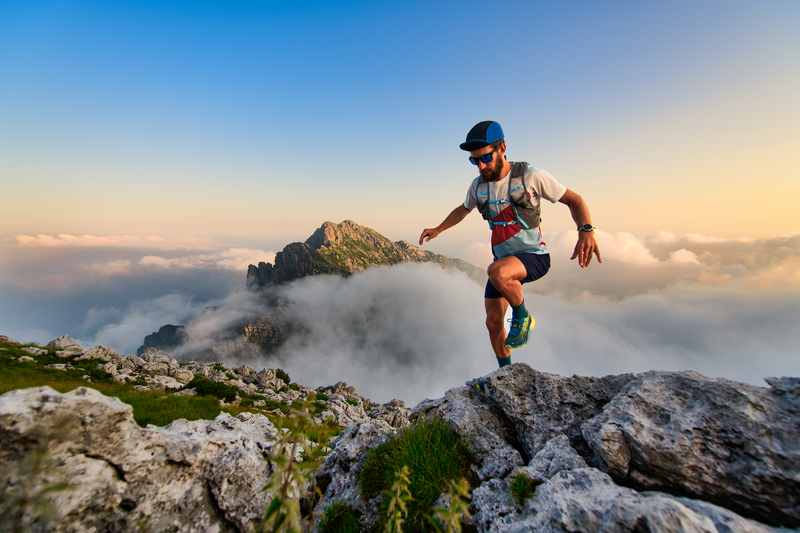
94% of researchers rate our articles as excellent or good
Learn more about the work of our research integrity team to safeguard the quality of each article we publish.
Find out more
REVIEW article
Front. Mol. Biosci. , 06 June 2024
Sec. Molecular Diagnostics and Therapeutics
Volume 11 - 2024 | https://doi.org/10.3389/fmolb.2024.1387576
This article is part of the Research Topic Crosstalk between Metabolism and Immunity View all 3 articles
All organisms have various circadian, behavioral, and physiological 24-h periodic rhythms, which are controlled by the circadian clock. The circadian clock controls various behavioral and physiological rhythms. In mammals, the primary circadian clock is present in the suprachiasmatic nucleus of the hypothalamus. The rhythm of the circadian clock is controlled by the interaction between negative and positive feedback loops, consisting of crucial clock regulators (including Bmal1 and Clock), three cycles (mPer1, mPer2, and mPer3), and two cryptochromes (Cry1 and Cry2). The development of early mammalian embryos is an ordered and complex biological process that includes stages from fertilized eggs to blastocysts and undergoes important morphological changes, such as blastocyst formation, cell multiplication, and compaction. The circadian clock affects the onset and timing of embryonic development. The circadian clock affects many biological processes, including eating time, immune function, sleep, energy metabolism, and endocrinology, therefore, it is also crucial for overall health, growth and development after birth. This review summarized the effects of the circadian clock in the body’s physiological activities. A new strategy is proposed for the prevention of malformations or diseases by regulating the circadian clock or changing circadian rhythms.
The circadian clock is organized in a hierarchical manner and is distributed within organs, cells, and tissues throughout the body. The central clock, known as the suprachiasmatic nucleus (SCN), is at the top of this hierarchy (Moreno et al., 2019). Several prokaryotes and most eukaryotes, whether bacteria or humans, have circadian clocks that manifest in the daily rhythms of physiology, behavior, and biochemistry (Rida et al., 2004). The mammalian circadian clock consists of a set of transcriptional circuits that oscillate in a 24-h cycle to maintain the rhythm of metabolism, sleep-wake cycle, and cell proliferation (Miller et al., 2007). The circadian clock receives daily signals from external environmental sources, including darkness and daylight, to drive circadian rhythms. Ultimately, circadian rhythms control the normal physiological, behavioral, and biochemical transmission of the organism (Maiese, 2017). In mammals, the length of a cycle of circadian rhythms is determined by the feedback loop regulation of circadian genes per (1 and 2), clock, cry (1 and 2), and bmal1 transcription in the middle of the circadian rhythm within the SCN. The length of this time is the transcription of cry 1 and 2 and per 1 and 2, starting with clock and bmal1 (Amano et al., 2020). The circadian clock cycle in mammals (including humans) is approximately 24 h. Therefore, circadian clocks require external input (time hints) to move phases to synchronize (or implicate) the environment (for example, light/dark cycles) (Moore and Lenn, 1972). The circadian clock receives optical and non-optical signals (Morris et al., 2012). Changes in eating habits, such as eating late at night, can result in inconsistencies between peripheral and central clocks. The optimal function relies on proper alignment between the central circadian clock (the SCN), chiaroscuro period, behavioral outputs (for example, activity, sleep, and diet), and the peripheral clock (Hastings et al., 2003). The development of early mammalian embryos is an ordered and complex biological process that includes stages from fertilized eggs to blastocysts and important morphological changes, such as blastocyst formation, cell multiplication, and compaction (Yong et al., 2021). Circadian rhythms extensively control physiological and pathological processes, including various human diseases and embryonic development in mammals (Jensen and Cao, 2013). The development and growth of the body are complicated processes that are affected by a number of factors, such as genetic background, the endocrine system, and environmental conditions (Cirillo et al., 2020). Studies have shown that the circadian clock affects many biological processes, including eating time, immune function, sleep, energy metabolism, and endocrinology (Luo et al., 2021). Healthy sleep is essential for overall health, development, and growth (Blaty and DelRosso, 2022). Our review explores the influence of the circadian clock to the embryonic development and body growth and development. It has also been shown that 2%–10% of mammalian genes are clock-controlled, and that they are mostly related to organ function and exhibit tissue-specific expression (Chu et al., 2011). Studies have shown that the circadian clock function is important for the cell cycle, in vivo tumor suppression, and DNA damage responses (Fu and Lee, 2003). Disorders of circadian rhythms can lead to serious health problems such as metabolic syndrome, organ fibrosis, aging, cancer, and osteopenia (Egstrand et al., 2020) These studies show that the circadian clock can not only regulate the growth and development of embryos and organs, but also has important implications for body health and disease development. Therefore, our review discusses the influence of circadian clock on the life process of the body, and provides new strategies and new ideas for the prevention and treatment of malformations and diseases.
The circadian clock affects the onset and timing of growth and development (Dunlap and Loros, 2006; Dunlap et al., 2007). It has been suggested that the cell cycle itself may be a degenerate circadian clock (Hunt and Sassone-Corsi, 2007). Light affects the circadian rhythms of all mammals (Dauchy et al., 2018). Other environmental factors such as temperature fluctuations, maternal nutrition, and stressors also influence circadian rhythm (Risely et al., 2021; Thompson et al., 2021; Tchekalarova et al., 2022). Important findings by Weiss et al. suggested that circadian rhythms of the eye may be involved in the regulation of eye growth (Chakraborty et al., 2018). The photoperiod, a seasonal and daily oscillating rhythm, can delay or inhibit sexual maturation and alter the ovarian developmental cycle (Dickey and Swanson, 1998; Rodríguez et al., 2005). Several previous studies have shown that both reducing and increasing light affect follicle development compared to a normal photoperiod (Shahed and Young, 2013; Murphy et al., 2015). Other studies have shown that associated signaling pathways and the photoperiod affect the secretion of sex hormones and development of the reproductive system (Vasal and Sundararaj, 1976). Numerous studies have reported that long-term (more than 6 weeks) exposure to light can affect ovarian development in rats, sheep, mice, cattle, and other mammals (Platt et al., 1983; Amaral et al., 2014). Based on the reflection of light by circadian rhythms, a prolonged artificial photoperiod can affect ovarian development (Chakraborty et al., 2018). In breast tissue, the expression pattern of the core clock gene changes in the female reproductive state and appears to be related to changes in the glandular development stage (Metz et al., 2006; Casey et al., 2014). Sleep disorders are often associated with children with neuropsychiatric disorders, and persistent sleep disorders can lead to impaired neuronal damage and brain development (Alfano and Gamble, 2009; Baddam et al., 2018). The interaction of clock genes and synapses may be involved in the pathogenesis of neuropsychiatric disorders, although sleep is considered to be necessary for brain maturation and synaptic development (Landgraf et al., 2014). Circadian rhythm disturbances affect brain stem development. Typically, Autism spectrum disorder first appears in infancy. Autism spectrum disorder is now considered a neurodevelopmental disorder. spatiotemporal disturbances of brainstem development may be the primary cause of Autism spectrum disorder that spread to the cerebral cortex (Takumi et al., 2020). Circadian rhythm disturbances are considered to be a common factor in developmental models of neuropsychiatric disorders (Marco et al., 2016). Sleep and circadian rhythms are constantly changing over the course of development, the dynamic relationship between sleep and circadian rhythms is necessary to understand sleep problems associated with ASD throughout development (Glickman, 2010).
The circadian clock transcription system has been reported to regulate cell proliferation intricately at the epigenetic level (Miller et al., 2007). The clock genes CLOCK (NPAS2) and BMAL1 may be the most important regulators of circadian rhythm. After translation and activation, they trigger the transcription of other CCGs. The dimerization of CLOCK and BMAL1 is achieved by helix-loop-helix motif on the per-arnt-sim domain of the CLOCK protein. Further epigenetic alterations, sumoylation, and phosphorylation of BMAL1 (Kondratov et al., 2003; Cardone et al., 2005) enhance the function of both proteins. After dimerization, they enter the nucleus to trigger the expression of PER and CRY genes (Gekakis et al., 1998). DNA methylation plays an important role in the regulation of PER2 gene and dysregulation can lead to metabolic disorders (Milagro et al., 2012). PER is thought to be phosphorylated by casein kinase I (CKI), while CRY is phosphorylated by adenosine monophosphate activated protein kinase (AMPK) (Lamia et al., 2009). CKI mutations lead to shorter daily cycles due to the accumulation of undegraded PER proteins, whose high levels eventually lead to an acceleration of the circadian rhythm, whose high levels eventually lead to an acceleration of the circadian rhythm (Lowrey et al., 2000). A gene-based approach has detected significant associations of CpG methylation pattern in PER2 gene with blood glucose and insulin resistance (Peng et al., 2019). Similar observations were made for the CLOCK and BMAL genes. Another result suggests that Bmal1 controls gene expression in inflammatory activation responses by regulating the epigenetic status of enhancers (Oishi et al., 2017). There is evidence that exposure to air pollution particles during pregnancy alters the methylation status of core circadian factors (CLOCK-BMAL1) and may be associated with circadian disruption (Nawrot et al., 2018). Nocturnin has been shown to be a clock-controlled deadenylase in mammals (Baggs and Green, 2003). Satoshi NISHIKAWA et al. found that early embryos and MⅡ oocytes of mice expressed nocturnin in circadian rhythms, and that accurate expression of nocturnin is imperative for the normal pre-implantation development of embryos (Nishikawa et al., 2013). Disrupting the circadian rhythm of the mother through exposure to chronic phase shifts in the photoperiod can have a lifelong effect on fetal metabolic homeostasis, leading to hyperinsulinemia in the offspring, increased obesity, and decreased insulin and glucose tolerance (Varcoe et al., 2013). Clock gene transcripts are components of maternal genetic transcripts and are often required for the first stage of embryonic development (Vatine et al., 2011). There is evidence that light can increase embryonic and post-hatch weights and pectoral muscle weight (Rozenboim et al., 2004). The nucleoplasmic shuttle of the clock in the E13–15 pancreas may be involved in the timing of pancreatic (Varcoe et al., 2013)rate of differentiation, which are regulated by circadian elements such as the clock and time (Li et al., 2007). Changes in light conditions (spectrum and intensity) affect the development of fish larvae and embryos in an underwater environment, and persistent red light or dark/light can lead to increased mortality and deformities (Sánchez-Vázquez et al., 2019). Tamara J. Varcoe et al. showed that pregnant female mice exposed to chronic phase shifts in the photoperiod profoundly alter the metabolism and expression of circadian rhythm genes in fetuses and mothers, potentially negatively affecting developing fetuses (Varcoe et al., 2013). Shift work of mothers can severely affect food consumption and the timing of activities, which can have a lasting impact on developing babies, making these offspring susceptible to metabolic disorders in adulthood (Varcoe et al., 2013). Tao et al. found that disturbances in circadian rhythms in pregnant mice also delayed enamel development in babies (Tao et al., 2016). Circadian oscillators have also been reported to guide early embryonic development. Cytokines and growth factors secreted and expressed by the embryo itself may be influenced by circadian transcription factors, thereby exerting autocrine control over embryonic development and paracrine stimulation of endometrial tolerance (Hardy and Spanos, 2002; Vlismas et al., 2016) (Figure 1). Maternal hormonal disturbances due to circadian rhythm changes or lack of sleep may disrupt fetal growth (Roman and Karlsson, 2013) (Table 1). Per3 is a main components of the circadian clock system. Mariko Noda et al. showed that the haploid dysfunction of Per3 may be associated with the onset of neuropsychiatric disorders, and Per3 plays an important role in brain development. In the neocortex of mice, the circadian clock gene regulates the onset of brain development after birth. In the developing mouse cerebral cortex, when Per3 was silenced, in addition to the abnormal position, the formation of dendritic protrusions and the projection of axons between the brain hemispheres were also canceled (Noda et al., 2019). As for the functional significance of circadian clock proteins in cortical development, oligophrenin-1, which is known to regulate dendritic spine morphology, interacts with Nr1d1 and is involved in neuronal function and structure during brain development (Valnegri et al., 2011). Lasse Dahl Jensen et al. recently found that circadian oscillations control angiogenesis in a developing zebrafish embryo model (Jensen and Cao, 2013). Bmal1 and Per2 have been reported to play roles in the regulation of angiogenesis in zebrafish development (Jensen et al., 2012). Jensen LD et al. exposed FLI1:EGFP zebrafish embryos to 12 h dark/12 h light (LD), persistent dark (DD), or persistent light (LL) environments. Exposure of zebrafish embryos to LL, in contrast to DD and LD, resulted in delayed angiogenesis of intersegmental vessels (ISV). DD exposure did not markedly alter ISV growth compared to that of LL. These results show that circadian rhythm disturbances may regulate angiogenesis in zebrafish embryos (Jensen et al., 2012). Studies have shown that the function of Bmal1 is inhibited by its specific morpholine, resulting in significant inhibition of ISV growth in zebrafish embryos (Jensen and Cao, 2013; Dunlap et al., 2007). In drosophila (Allada et al., 1998) and mice (King et al., 1997), homozygous deletion of the clock gene is fata to embryos. Throughout the embryonic development process, the core circadian rhythm genes represented by Clock are expressed in harmony with tissue differentiation and can be used as a timing device through regulation of Notch- and Wnt-based components and cell cycle regulation (Li et al., 2007). Studies have reported that the Clock gene Timeless is involved in branching morphogenesis in mouse urethral buds (Xiao et al., 2003). Homozygote Tim (−/−) embryos die early in the womb, with a general lack of cell tissue and necrotic cell death (Gotter et al., 2000). Rigzin et al. used genome-wide methods to identify indirect and direct targets for the clock-controlled transcription factor ADV-1 and light response in Brucella. A large proportion of the ADV-1 target was found to be clock-controlled and rich in genes associated with development and cell growth (Dekhang et al., 2017). In peripheral tissue cells, the circadian clock regulates gene expression by regulating circadian rhythms, such as Mdm2, C-myc, Cyclind1, P53, and Gadd45a, controlling apoptosis and proliferation (Hunt and Sassone-Corsi, 2007). Clock-△19 mice exhibited a point mutation that led to the downregulation of the Clock-Bmal1 target gene, which manifested as a decrease in the growth rate of young mice and an increase in neonatal mortality (Kennaway et al., 2004; Dolatshad et al., 2006). In contrast, melatonin promotes mitochondrial biogenesis by activating the SIRT1/PGC-1α pathway, compensating for energy deficiency and mitochondrial depletion, and promoting early embryonic development (Niu et al., 2020). The circadian clock and Smad2/3/4-mediated nodal signals regulate several pathological and physiological processes. Sha-Sha Bian et al. found that Clock1a coordinates the original blood supply and development of the mesoderm in zebrafish embryos by directly upregulating the Nodal-Smad3 signal (Bian et al., 2017). It has been reported that oscillations of circadian gene expression may serve as the master controllers of other potential timing oscillators, such as WNT and Notch signaling rings, as well as cell cycle regulation, and as the main regulator of the embryonic differentiation clock (Li et al., 2007). Studies have shown that interruptions in molecular clocks or changes in light conditions lead to changes in INR/TOR signals, which impact the growth and proliferation of neural stem cells in the brain of developing Drosophila (Dapergola et al., 2021).
Figure 1. Circadian oscillators have been reported to guide early embryo division. Cytokines and growth factors secreted and expressed by the embryo itself may be influenced by circadian transcription factors, thereby exerting autocrine control over embryonic development and paracrine stimulation of endometrial tolerance.
A study by Gotter et al. demonstrated that a possible mouse homology gene, mimless (mtim), is essential for embryonic development, and homozygous mtim (−/−) embryos die early in the uterus and developmental abnormalities are found as early as E5.5 embryos, suggesting that mtim is important before and after embryonic implantation (Gotter et al., 2000; Xiao et al., 2003). A study by Hanbin Dan et al. established the role of the fetal circadian clock as a regulator of the developmental timer, the number of renal units, and the branching rate, thus playing a fundamental role in kidney development (Dan et al., 2020). The circadian rhythm gene Timeless is involved in epithelial morphogenesis during kidney development (Li et al., 2007). Studies have reported that normal kidney development requires a complete fetal clock. Structural abnormalities found in Bmal1 tissue-specific mutations and overall mutations in the kidney suggest that circadian clock dysfunction may contribute to congenital defects. Disruption of the fetal renal rhythmic expression of genes is also related to mammalian phenotypes (Dan et al., 2020) Local or integral deletion of fetal Bmal1 produces recognizable phenotypic abnormalities and disrupts clock-correlated oscillatory expression of key developmental genes. The fetal clock described herein can provide a layer of regulation that coordinates the time of a variety of developmental processes (Dan et al., 2020). High-resolution 3D analysis of embryonic kidneys indicates that fetal clock interruptions, whether local or global, can lead to phenotypic defects, similar to congenital urinary tract abnormalities and congenital kidneys, which account for more than 50% of kidney failures and are a group of malformations that lead to child transplantation and dialysis (Dugoff, 2002; Verbitsky et al., 2019). These studies indicate that circadian clock transcription factors play an important role in the regulation of kidney branching morphogenesis, growth, and development (Dan et al., 2020).
Melatonin is secreted primarily by the pineal glands and is a multifunctional hormone regulated by circadian rhythms. It also plays a key role in embryonic development, and oocyte fertilization and maturation (Yong et al., 2021). Photoperiod information is converted from the pineal gland to rhythmic secretion of melatonin, which is released into the circulatory system at high concentrations at night and at low concentrations during the day. The rhythm of melatonin is controlled by the circadian clock and is considered an important output of the circadian rhythm system (Sánchez-Vázquez et al., 2019). Melatonin, a neuroendocrine hormone secreted by the pineal gland, transmits information about the ambient photoperiod (seasons and circadian rhythms) to relevant organs and tissues in the body (Hunt and Sassone-Corsi, 2007). Studies have reported that melatonin can protect against the development of vitrified embryos, which is related to its increased endogenous glutathione levels, anti-apoptosis, and direct free radical scavenging activity (Gao et al., 2012). Recent studies have also shown that melatonin affects gonadal development in mice (Kasahara et al., 2010), as well as the formation of teeth, bones, adrenal glands, and testicles (Torres-Farfan et al., 2006; Kasahara et al., 2010). Melatonin secreted by the salivary glands of embryos can affect organ development. In the early embryonic stage, melatonin maintains the shape of the salivary glands until the beginning of the branch morphology. In addition, the circadian rhythm of melatonin may affect the development of salivary glands (Obana-Koshino et al., 2015). Melatonin supplementation has been shown to significantly improve mitochondrial dysfunction, DNA damage, and zearalenone-induced oxidative stress early in embryonic development (Xu et al., 2019). Niu et al. showed that the beneficial effects of melatonin on early embryos depend on two crucial aspects. First, melatonin can prevent excessive mitochondrial biosynthesis induced by hyperoxia environments under sub-physiological conditions (Niu et al., 2020). Second, melatonin therapy protects fetal growth, placental perfusion, and fetal cardiovascular function during pregnancy (Itani et al., 2016). In malnourished pregnant rats, maternal melatonin supplementation increased birth weight (Richter et al., 2009). Therefore, the regulation of circadian rhythm disturbances may play an important role in the normal development of embryos.
Jennette P. Moreno et al. argued that children exhibit healthy seasonal patterns of height and weight gain controlled by an annual clock, whereas the summer vacation environment may favor circadian rhythm disorders caused by changes in social needs, promoting accelerated weight gain in children during the summer months (Moreno et al., 2019). There is evidence that weight gain and growth (height) in children are seasonal, suggesting that an endogenous rhythm of weight gain and growth exists in children (Bogin, 1978; Bogin, 1979). Since Bmal1 and Clock downregulate many genes other than Crys and Pers (10% of transcription genes), daily rhythms, such as sleep, are created (Buhr and Takahashi, 2013). Individuals such as shift workers experience circadian rhythm disorders frequently and/or chronically because their waking/sleeping and eating/fasting cycles are out of sync with the circadian timing system (Morris et al., 2012). When social needs such as school or work require individuals to live according to a schedule that does not conform to their inner rhythm, it can make it difficult to wake up and fall asleep during socially mandated work or school hours. Social jet lag is an example of a chronic circadian rhythm disorder. This leads to unmet sleep needs, which can accumulate sleep debt (for example, social jet lag) during work or school. On weekends, different waking times may bring more variability in sleep, meals, and activity patterns, resulting in a disorder of peripheral and body central clocks, leading to negative health consequences, such as cardiovascular disease, obesity, and cancer (Garaulet et al., 2005; Laermans and Depoortere, 2016; Roenneberg and Merrow, 2016). Disorders of circadian rhythms can lead to serious health problems such as metabolic syndrome, organ fibrosis, aging, cancer, and osteopenia (Egstrand et al., 2020) (Figure 2). Studies have shown that normal rhythmic damage favors gene expression and activation of pro-inflammatory responses, which may also play a role in neurodegenerative changes (De Lazzari et al., 2018). It has also been shown that 2%–10% of mammalian genes are clock-controlled, and that they are mostly related to organ function and exhibit tissue-specific expression (Chu et al., 2011). Studies have shown that the circadian clock function is important for the cell cycle, in vivo tumor suppression, and DNA damage responses (Fu and Lee, 2003). Several studies have reported the expression of circadian clock genes in reproductive tissues, including the ovaries (Sellix and Menaker, 2010). Female Clock-mutant mice have irregular estrous cycles and carry 51 amino acid deletions in the transcriptional activation region of the clock gene, without a normal luteinizing hormone surge in the pre-estrus period (Miller et al., 2004). Blood pressure, the cardiovascular system, endothelial function, heart rate, and thrombosis are all regulated by the circadian clock (Hu et al., 2004). Twenty-four-hour rhythm disturbances can lead to cardiovascular diseases, including fibrosis, heart failure, arrhythmias, and myocardial infarction (Martino et al., 2008). In Dahl salt-sensitive rats fed a high-salt diet for 6 weeks, the development of hypertrophy was associated with decreased diurnal expression of the core clock gene of the heart (Mohri et al., 2003). Similarly, hamster models with ubiquitous circadian cycle changes can develop accelerated senile cardiac kidney disease (Martino et al., 2008). Consistent with these rodent models of circadian dysregulation, shift workers are at a significantly higher risk of cardiovascular disease (as well as various features of cardiometabolic syndrome) (Knutsson, 1995; Härmä and Ilmarinen, 1999). Circadian clock genes play a key role in dementia and neurodegenerative diseases (Cronin et al., 2017; Vaccaro et al., 2017). Studies have shown that long-term levodopa therapy may impair circadian rhythm function and lead to cognitive dysfunction. In this regard, simulating long-space flights alters circadian rhythms, leading to potential neuronal damage and cognitive decline (Wu et al., 2017). Circadian rhythm disorders that lead to sleep fragmentation may lead to the further progression of neurodegenerative diseases. Circadian disruption and sleep disorders exacerbate neurodegeneration, which in turn can disrupt circadian rhythms and sleep (Shen et al., 2023). Increasing data suggest circadian rhythm plays a crucial role in regulating metabolism and neurological diseases and Bmal1 is considered to be a key regulator of circadian transcription (Gao et al., 2023). Compared with C57BL/6 J mice, BMAL1 KO showed a significantly reduced seizure threshold (Gerstner et al., 2014). Deletion of Per2 gene disrupts the circadian rhythm system, resulting in dysregulation of the HPA stress axis, which is subsequently associated with increased depression-like behavior and deficient starling response (Russell et al., 2021). Circadian rhythm alterations are commonly reported in individuals with psychiatric disorders, including major depressive disorder (MDD), bipolar disorder (BD), anxiety, and schizophrenia (SZ). In turn, circadian disruption may trigger or exacerbate symptoms in individuals with a predisposition for mental health disorders (Walker et al., 2020). Cerebrospinal fluid promotes the entire brain through a lymphatic pathway involving the perivascular network, which is necessary to clear metabolic waste products during sleep. This system relies on glial cells, and sleep deprivation can lead to the development of neurodegenerative diseases and dysfunction of the system (Benveniste et al., 2017). Alterations in circadian clock genes have been shown to cause age-related cognitive and motor impairments, as well as the progression and onset of Alzheimer’s disease (AD) (Maiese, 2021). A large number of studies have shown that aging is accompanied by the weakening of circadian rhythm and the change of sleep pattern (Stowe and McClung, 2023). For example, sleep-wake disruptions may be associated with an increase in Alzheimer’s disease-related biomarkers. AD is characterized by dampened circadian rhythms with a delay in circadian phase and overall decrease in amplitude (Stowe and McClung, 2023). Aging is associated with dysregulation of the circadian system and cognitive decline (Adler et al., 2019). Circadian disruption has been linked to hippocampal dependent memory impairment, and it is conceivable that age-induced changes in circadian regulation, a key process in hippocampal function, could contribute to this decline. The current study further supports the key role of circadian molecules in regulating cognitive processing and ROS/RNS homeostasis. Loss of normal sleep/wake cycles is a leading cause of hospitalization for dementia and has even been considered a contributing factor and/or preclinical symptom of neurodegenerative diseases such as Alzheimer’s and Huntington’s disease (Gerstner, 2010).
Different tissues have different clock-rhythmic output genes, allowing circadian rhythm control of the proper activity and function of each organ (Casey et al., 2021). In breast tissue, the time expression pattern of the clock gene of the core protein changes with changes in the female reproductive state, which seems to be related to changes in the stage of gland development (Casey et al., 2014). The circadian clock relies on the modulation of circadian clock genes, and mice with Per2 defects exhibit fewer bifurcations, and lack of catheter-deficient distal migration is evidence of the involvement of Per2 in breast branch morphogenesis (McQueen et al., 2018) (Table 2). Clock mutant mice exhibit lactation defects (Hoshino et al., 2006). In addition, the reduction in postnatal survival in Clock-△19 mice is associated with impaired breast development (Casey et al., 2021). In epidermal stem cells, it has been demonstrated that the circadian clock can adjust the Wnt/β-catenin pathway (Janich et al., 2011). Alterations in gene expression levels in the Wnt pathway may be a potential cause of the impaired mammary epithelial stem cell function observed in Clock-△19 mice (Yang et al., 2017) and significant premature aging in Bmal1−/− mice (Gimble et al., 2009). Bmal1 is necessary for bone marrow stem cell survival and growth (Burgermeister et al., 2019). Systemic knockout of mice with the core clock gene Bmal1 results in severe deficiencies in muscle and growth function (Kondratov et al., 2006; Andrews et al., 2010). Studies have shown that CAMK-1 deletion strains from ascomycete germination grow slowly, whereas analysis of CAMK-1 deletion strains has shown that CAMK-1 deletion affects the cycle of conidia circadian rhythm, phase, and light that induce phase shifts (Yang et al., 2001). Gene deletions of core circadian transcription factors (TFs), Clock and Bmal1, lead to a significant shortening of lifespan and premature aging (Maryanovich and Frenette, 2016). Research by Ebert et al. and others showed that the core circadian factor Bmal1 and Clock are necessary for the self-renewal and growth of leukemia stem cells (Puram et al., 2016). Data from Dapergola et al. suggested that disruption of molecular clocks or altered light conditions leads to alterations in INR/TOR signals that have an impact on the growth and proliferative properties of neural stem cells in the brains of developing Drosophila (Dapergola et al., 2021). In addition, mice with Per2 mutations are susceptible to cancer, whereas ectopic expression of Per1 and Per2 can lead to growth inhibition (Gery et al., 2005; Gery et al., 2006). The PER protein binds to nuclear receptors, such as REV-ERBα and PPARα, regulating life activities, including growth and development, through nuclear receptor function (Grimaldi et al., 2010; Schmutz et al., 2010). In mice treated with 6-OHDA, mutations in the circadian clock element mEV-ERBα (mBmal1 transcriptional inhibitory factor) promoted 6-OHDA-dependent motor deficits, neuroinflammation in the spinal midbrain, and dopaminergic neurodegeneration (Kim et al., 2018). Aged Per0 mutant flies exhibited impaired motor ability, increased sensitivity to oxidative damage, and expanded vacuolarization in the central brain. The loss of functional dPer exacerbates damage from neurodegenerative predisposition mutants, suggesting that in Drosophila, the Clock gene acts as a protective factor during aging (Krishnan et al., 2009). Reproductive dysfunction has been studied in Bmal1−/− mice and mice with Clock mutations (Chu et al., 2011). Studies showed that mouse Per2 or Per1 loss-of-function mutations have a lower reproductive success rate in 9-month-old male mice (Pilorz and Steinlechner, 2008). Y ating Zheng et al. found that the number of follicles in Per2/Per1 mutants decreased significantly from mid-year onward, compared to that is the litter control group. Per2/Per1 deletion mutations may alter the expression of genes involved in follicle development, leading to a decreased reproductive capacity and depletion of follicular reserves (Zheng et al., 2019). Studies have shown that deletion of Per2 or Per1 affects fertilization rates in females aged 9–12 months, manifested by decreased implantation success rates and abnormal estrous cycles (Pilorz and Steinlechner, 2008). Clock-△19 mice exhibited a point mutation that led to downregulation of the Clock-Bmal1 target gene, which was manifested by a decrease in lactation ability, with an increase in neonatal mortality and a decrease in growth rate in mice (Dolatshad et al., 2006). Using shRNA to reduce clock proteins in breast epithelial cell lines affected cell growth and reduced the expression of factors related to milk synthesis and breast differentiation (Casey et al., 2021). Bmal1 gene-deficient mice have a severely shortened lifespan and are sterile, weighing less than wild-type mice, and exhibit various characteristics of premature aging, such as arthropathy and early cataracts (Lee et al., 2010). Accelerated aging is a prominent feature in animal models with modified and missing core clock genes (Uth and Sleigh, 2014). Studies have reported that a non-coding polymorphism in the 3-UTR region of the human clock gene is linked to adult hyperactivity disorder and attention deficit (Kissling et al., 2008).
The circadian clock signal plays a key role in adjusting the mineralization and thickness of tooth enamel (Said et al., 2020). Immunohistochemical (IHC) studies have shown that Clock, Per1, Bmal1, and Per2 proteins are expressed in developing dental organs (Zheng et al., 2011). The circadian clock regulates a wide range of physiological processes (King and Takahashi, 2000; Schibler and Sassone-Corsi, 2002) including the processes by which other mineralized tissues affect bone growth and homeostasis (Fu et al., 2005). The periodicity observed in enamel suggests that the molecular basis that coordinates enamel development may be affected by the circadian clock (Lacruz et al., 2012). To determine the clock gene and diurnal rhythm of Amelx, Rodrigo et al. used mouse glazed cells for serum synchronization and analyzed the expression of circadian transcription factors Bmal1 and Per2 using real-time quantitative polymerase chain reaction (qPCR). The results showed that these crucial gene regulators of the circadian clock were expressed in synchronized cultured mouse glazed cells, and their expression patterns followed the reverse circadian rhythm pattern of the two gene transcripts (Lacruz et al., 2012). The ablation of the superopedial nucleus in rats led to the destruction of the incremental line in dentin (Anresen line), supporting the participation of the circadian clock in the formation of this tissue (Ohtsuka-Isoya et al., 2001). Rodrigo et al. found that in cultured glazed cell LS8, the clock genes Bmal1 and Per2 oscillate in reverse over a 48-h period. They also demonstrated the expression of Cry1 and Bmal1 in developing glazed cells (Lacruz et al., 2012). In addition, they measured daily fluctuations in the expression of amelogenin (Amelx) in the entire developing mouse tooth grinding process, which is a specific product of amelo-blast cell differentiation (Lacruz et al., 2012). Immunocytochemical (ICC) results showed that the expression of amelx and melatonin receptors (MTS) was markedly delayed in newborn mice grown in a full-light or all-dark environment, as well as during enamel development. The development of tooth enamel was also delayed, showing significant immature histology (Tao et al., 2016; Li et al., 2007). Studies have shown that the disruption of SOCE (store operated Ca2+ entry) significantly affects the molecular circadian clock of glazed cells, suggesting that alterations in circadian clocks may be associated with the development of stromal interaction molecule 1 (STM1)-mediated AI (amelogenesis imperfecta) (Said et al., 2020).
Bone is a dynamic tissue that constantly responds to environmental changes such as mechanical stress and nutrition (Chan et al., 2021). Bone homeostasis in adult life is maintained through bone reconstruction, which is a balanced and controlled process involving bone resorption by osteoblasts and osteoclasts (Chan et al., 2021). Over the past decade, several studies have revealed complex interactions between skeletal systems and the circadian clock (Gonçalves and Meng, 2019). Disturbances in the circadian clock of people with sleep restrictions and night workers have been linked to osteoporosis and abnormal bone metabolism (Feskanich et al., 2009). Osteoblasts at the site of fracture healing and tissue homeostasis are affected by the circadian clock (Chan et al., 2021) (Figure 3). The timing of eating and circadian rhythm disorders can alter bone metabolism (Egstrand et al., 2020). In recent years, from structural maintenance to functional regulation, several studies have reported that the clock system in skeletal muscle plays a key role in critical aspects of skeletal muscle physiology (Andrews et al., 2010; Chatterjee et al., 2013; Chatterjee et al., 2015). Studies in mice with mutations in the clock gene have shown a clear link between maintaining the entire molecular clock circuitry and skeletal muscle growth and development (Chatterjee and Ma, 2016). In vitro culture studies of flat and long bones, Per2 was found to have a 24-h rhythmic expression at the site of fracture healing (Kunimoto et al., 2016). Studies have reported that Bmal1 deficiency can lead to severe aging-related osteoporosis, and at 9 months of age, the loss of the Bmal1 gene leads to an approximate 50% weight loss (Kondratov et al., 2006). Clock mutant mice exhibited significant structural muscular pathology, skeletal muscle weakness, altered muscle filament structure, and altered mitochondrial volume (Miller et al., 2007). Molecular clocks play an important role in maintaining correct time regulation of muscle clock control genes (CCGs). In the first stage of myoblast differentiation and growth, myogenic differentiation 1 (MyoD1) is activated and becomes CCG dependent on its unique diurnal expression trends in adult muscles (Riley and Esser, 2017). Ablation of Bmal1 or Clock, key elements of the molecular clock, impairs its target genes and circadian expression of MyoD1, which can lead to altered sarcosmosamus fibrous tissue and disruption of muscle contractility (Andrews et al., 2010). The modulation of myogenic progenitor cells by Bmal1 has been shown to be imperative for the regeneration of muscle tissue after injury. In the muscle injury-induced regeneration model, animals lacking Bmal1 exhibited defects in the regenerative muscle generation process, accompanied by alterations in the process of satellite cell expansion and a decrease in repair capacity (Chatterjee et al., 2015). In mice with Clock mutations and Bmal1 gene defects, the finding of similar functional defects produced by muscle-specific forces suggests that clocks play a synergistic role in this basic skeletal muscle function (Bunger et al., 2000; Kondratov et al., 2006). In addition, mice with ROR, Per1, and Per2 deficiency, were found to have associated pathologies such as muscle movement, contraction defects, and weakness in muscle function and structure (Zheng et al., 1999; Zhang et al., 2015). Studies have shown that adult Bmal1 gene deficient mice may experience significant muscle weight loss due to impaired hypertrophic growth and developmental defects (Chatterjee and Ma, 2016). The opposite effects of the clock suppressor Rev-erbα and activator Bmal1 on myogenic development and the effect of RoRα on myogenic pathways strongly suggest the role of circadian clock genes in regulating myogenic precursor development (Chatterjee and Ma, 2016). Jeffrey et al. measured muscle growth in live zebrafish larvae over a 12-h period and found that muscles grew more during the day than at night. The expression of dominant negative CLOCK (△clk) reduces muscle growth, inhibits molecular clock function, and eliminates circadian rhythm differences (Kelu et al., 2020). Another important insight provided by growth plate research is the influence of the circadian clock on developmental signaling pathways and its regulation of osteogenesis in cartilage (Samsa et al., 2017). Some chondrocyte-specific genes, such as Col10a1 and Col2a1, are expressed in a diurnal pattern in ATDC5 chondrocytes, as well as in growth plates and articular cartilage, in addition to core clock genes (Gossan et al., 2013; Okubo et al., 2013). Parathyroid hormone (PTH) can reset the body clock of the femur in a dose- and time-dependent manner (Hanyu et al., 2011; Okubo et al., 2015). PTH modulates the expression of mouse Per2 and Per1 through the PKA-CREB signaling pathway (Hinoi et al., 2006). Therefore, there may be a peripheral clock specific to chondrocytes that is essential for growth plate development. In mice with Bmal1 gene defects, Ihh-mediated chondrocyte differentiation is impaired, and in Bmal1 gene-defective mice, the tibia and femur are significantly shortened (Takarada et al., 2012; Samsa et al., 2016). In addition, chondrocyte-specific removal of Bmal1 led to severe cartilage degeneration in 3-month-old rats (Samsa et al., 2017). One study showed that conditional knockout of Bmal1 in cartilage affects the integrity of cartilage and makes joints more susceptible to osteoarthritis (Dudek et al., 2016). Another study indicated that in mouse nucleus medullary cells, Bmal1 controls the transcriptional activity of HIF1α and, once removed, inhibits nuclear medullary growth (Suyama et al., 2016). Zheng et al. found that bmal1 plays a key role in cartilage development by modulating the Hif1α-vascular endothelial cell growth factor signaling pathway (Ma et al., 2019). Ma et al. demonstrated that the deletion of a specific Bmal1 in postnatal cartilage leads to decreased chondrocyte proliferation, disorders of cartilage formation in growth plates, and hypertrophy of chondrocytes that hinder the growth of longitudinal bone (Ma et al., 2019). Another study showed that a conditioned loss of Bmal1 in chondrocytes resulted in shorter tibia and femur lengths than control mice, with growth retardation associated with reduced expression of Indian hedgehog (IHH) (Takarada et al., 2012). Studies have identified the core circadian rhythm gene Bmal1 as being essential for maintaining normal intrachondral osteogenesis (Ma et al., 2019), and have shown that Bmal1 binds directly to the OPG promoter and upregulates its expression to inhibit osteoclast differentiation (Zhou et al., 2018).
Figure 3. Effect of the circadian clock on bone remodeling in adulthood in maintaining bone integrity and homeostasis.
There is growing evidence that failure of the circadian clock system is correlated with the pathogenesis of cancer (Greene, 2012; Sahar and Sassone-Corsi, 2012). There are several examples illustrating the link between circadian rhythms, circadian rhythm genes, age-related phenotypes, and cancer (Lee et al., 2010). Studies have shown that mice with Clock mutations resulting in rhythmic loss exhibit many phenotypic abnormalities, such as an increase in the incidence of underlying cancer or an increase in the incidence of cancer after genetic stress (Lee et al., 2010). The results of Puram et al. revealed that the survival and growth of leukemia cells depend on clock mechanisms (Puram et al., 2016). Nurses who work night shifts have higher rates of colorectal, breast, endometrial, and rectal cancer (Schernhammer et al., 2001). This risk increases as shift hours increase (Papantoniou et al., 2016). An increased risk of prostate cancer was also observed in male night shift workers, and the aggressiveness of the disease was found to be associated with night shift time (Papantoniou et al., 2015). Studies have reported that people who travel by air have a higher risk of developing cancer, which is associated with jet lag. One meta-analysis showed that female flight attendants who often experience endogenous circadian rhythm disturbances have an increased risk of malignant melanoma and breast cancer (Tokumaru et al., 2006). Mouse models of chronic jet lag exhibit changes in liver metabolism, which can lead to liver cancer (Kettner et al., 2016). Studies have shown that an increasing number of diagnosed cases of hepatocellular carcinoma are thought to be related to circadian clock disorders, obesity and non-alcoholic fatty liver disease (Crespo et al., 2021). The occurrence and progression of liver cancer relies on multiple molecular mechanisms, involving genetic and epigenetic alterations of oncogenes and tumor suppressor genes, as well as disorder control, abnormal functions and inappropriate interactions of key molecular cascades such as Hedgehog, MAPK and PI3K/AKT/mTOR signaling pathways (Skrlec and Talapko, 2022). Studies have reported that decreased levels of melatonin or decreased excretion of its main metabolite, 6-thiooxidative melatonin, are associated with an increased risk of cancer, suggesting the anticancer effects of this indoleamine. Overall, published data strongly support the inhibitory effect of melatonin on different types of tumors, including liver cancer (Fernández-Palanca et al., 2021). The inhibitory effect of melatonin in liver tumors involves a variety of different cellular processes and molecules, including cell cycle arrest, decreased cell growth, limiting vascular metastasis and production, and promoting cell death. In addition, melatonin has been reported to increase the sensitivity of liver cancer cells to existing treatments (Prieto-Domínguez et al., 2017). In addition, increased expression of the circadian rhythm gene hClock may promote tumorigenesis by enhancing the expression of genes associated with angiogenesis, such as those involved in metastasis of colorectal cancer (Wang et al., 2017). The five-repeat variant allele of the human Per3 gene 4/5 repeat polymorphism is associated with a triple risk of breast cancer in premenopausal women (Uth and Sleigh, 2014). Studies have reported that in breast cancer, the expression level of Znf704 is inversely associated with the expression level of Per2, and high levels of Znf704 are correlated with lymph node positivity, poor prognosis, and histological grading in patients with breast cancer (Yang et al., 2020). Baharan et al. revealed that Bmal1 and P2-HNF4α are incompatible in liver cancer and that forcing the expression of Bmal1 in HNF4α-positive liver cancer can stop tumor growth in vivo (Fekry et al., 2018). Studies have reported that PFKFB3 mediates circadian rhythm control of tumor growth, underscoring the significance of time-based PFKFB3 inhibition in cancer treatment (Chen et al., 2016). Studies have reported a lack of tumor suppression and cell cycle disorders in mice with Per2 gene defects, suggesting that Per2 plays a tumor-suppressive role through the DNA damage response pathway (Morgan et al., 2019). Another study found that Per1 knockout increased the expression of MMP9 and MMP2 and also reduced the expression of TIMP-2, which may lead to the observed increase in tumor cell invasion and migration (Li et al., 2016). The immune system provides defense mechanisms to eliminate or curb the appearance of tumor cells in healthy tissues and prevent the further development of life-threatening cancers (Gajewski et al., 2013; Chen and Mellman, 2017). Both innate and adaptive immune responses exhibit circadian rhythms (about 24 h) (Scheiermann et al., 2018; Palomino-Segura and Hidalgo, 2021), There is evidence that the circadian clock components of cancer cells are disturbed, which drives cancer development (Papagiannakopoulos et al., 2016). Using immunodeficient mice and mice lacking lineage-specific circadian functions, study has found that CD8+ T cells and dendritic cells (DCs) exert circadian anti-tumour functions that control melanoma volume (Wang et al., 2023). Melanoma and Breast cancer mouse models show that circadian rhythm disturbance enhances cancer cell proliferation, stemness, spread and metastasis, induces immunosuppressive TME by increasing the proportion of TAM and regulatory T cells (TREGs), and promotes the differentiation of macrophages into anti-inflammatory phenotype, resulting in reduced infiltration of active CD8+ T cells (Aiello et al., 2020; Hadadi et al., 2020). RORγ activation inhibits tumor growth and prolongs survival by enhancing Th17 cell differentiation and function and reducing Treg levels (Hu et al., 2016).
Circadian rhythms control several pathological and physiological processes, including various human diseases and embryonic development in mammals (Jensen and Cao, 2013; Dunlap et al., 2007). Targeting the components of circadian clock has received much attention as a novel approach to treating chronic diseases such as metabolic syndrome, chronic inflammatory diseases and cancer (Roenneberg and Merrow, 2016; Rijo-Ferreira and Takahashi, 2019). In theory, there are two drug approaches that could target the circadian clock: either by directly regulating the core circadian rhythm gene, or by targeting its regulators. However, since BMAL1 and CLOCK are transcription factors, it is challenging to directly target these circadian core genes (Takahashi, 2017; Trott and Menet, 2018). Therefore, pharmacological drugs that target proteins responsible for phosphorylating or degrading the clock components that negatively regulate BMAL1 and CLOCK have been developed as antagonists or agonists to disrupt the circadian network. Advances have been made in the chronopharmacology of those agents that target cancer, immunity, metabolism, coagulation, the cardiovascular system, and inflammatory and rheumatologic processes (Hermida et al., 2019; Tamimi et al., 2022; Civelek et al., 2023; Leung et al., 2023). Supplement dosing and schedule recommendations with circadian rhythm are recommended to improve drug safety and efficacy. The study of circadian drug responses has become a continuum from cell cultures to organoids, animal models, and patients (Lévi et al., 2024). Meaningful improvements in drug tolerability and/or efficacy through appropriate timing of administration have been demonstrated for immunotherapy and chemotherapy for cancer, as well as pharmacological agents commonly used in cardiovascular, metabolic, inflammatory, and neurological diseases (Lévi et al., 2024). However, few compounds have entered clinic trials that can evaluate the true efficacy of patients, and the dependence of these therapeutic effects on clock regulation still needs further investigation (Zhou et al., 2022).
BL: Writing–original draft. JS: Formal Analysis, Writing–original draft. JZ: Formal Analysis, Writing–original draft. JH: Methodology, Writing–original draft. XZ: Writing–review and editing. LC: Writing–review and editing.
The author(s) declare financial support was received for the research, authorship, and/or publication of this article. This work was supported by the National Natural Science Foundation of China for Excellent Young Scholars (31725011, to LC) and Key Program Projects (82030070, to LC), the National Natural Science Fund (82001026, to XZ).
The authors declare that the research was conducted in the absence of any commercial or financial relationships that could be construed as a potential conflict of interest.
All claims expressed in this article are solely those of the authors and do not necessarily represent those of their affiliated organizations, or those of the publisher, the editors and the reviewers. Any product that may be evaluated in this article, or claim that may be made by its manufacturer, is not guaranteed or endorsed by the publisher.
Adler, P., Chiang, C. K., Mayne, J., Ning, Z., Zhang, X., Xu, B., et al. (2019). Aging disrupts the circadian patterns of protein expression in the murine Hippocampus. Front. aging Neurosci. 11, 368. doi:10.3389/fnagi.2019.00368
Aiello, I., Fedele, M. L. M., Román, F., Marpegan, L., Caldart, C., Chiesa, J. J., et al. (2020). Circadian disruption promotes tumor-immune microenvironment remodeling favoring tumor cell proliferation. Sci. Adv. 6, eaaz4530. doi:10.1126/sciadv.aaz4530
Alfano, C. A., and Gamble, A. L. (2009). The role of sleep in childhood psychiatric disorders. Child youth care forum 38, 327–340. doi:10.1007/s10566-009-9081-y
Allada, R., White, N. E., So, W. V., Hall, J. C., and Rosbash, M. (1998). A mutant Drosophila homolog of mammalian Clock disrupts circadian rhythms and transcription of period and timeless. Cell. 93, 791–804. doi:10.1016/s0092-8674(00)81440-3
Amano, T., Ripperger, J. A., and Albrecht, U. (2020). Changing the light schedule in late pregnancy alters birth timing in mice. Theriogenology 154, 212–222. doi:10.1016/j.theriogenology.2020.05.032
Amaral, F. G., Castrucci, A. M., Cipolla-Neto, J., Poletini, M. O., Mendez, N., Richter, H. G., et al. (2014). Environmental control of biological rhythms: effects on development, fertility and metabolism. J. Neuroendocrinol. 26, 603–612. doi:10.1111/jne.12144
Andrews, J. L., Zhang, X., McCarthy, J. J., McDearmon, E. L., Hornberger, T. A., Russell, B., et al. (2010). CLOCK and BMAL1 regulate MyoD and are necessary for maintenance of skeletal muscle phenotype and function. Proc. Natl. Acad. Sci. U. S. A. 107, 19090–19095. doi:10.1073/pnas.1014523107
Baddam, S. K. R., Canapari, C. A., van Noordt, S. J. R., and Crowley, M. J. (2018). Sleep disturbances in child and adolescent mental health disorders: a review of the variability of objective sleep markers. Med. Sci. Basel, Switz. 6, 46. doi:10.3390/medsci6020046
Baggs, J. E., and Green, C. B. (2003). Nocturnin, a deadenylase in Xenopus laevis retina: a mechanism for posttranscriptional control of circadian-related mRNA. Curr. Biol. 13, 189–198. doi:10.1016/s0960-9822(03)00014-9
Benveniste, H., Lee, H., and Volkow, N. D. (2017). The glymphatic pathway: waste removal from the CNS via cerebrospinal fluid transport. Neurology Psychiatry 23, 454–465. doi:10.1177/1073858417691030
Bian, S. S., Zheng, X. L., Sun, H. Q., Chen, J. H., Lu, Y. L., Liu, Y. Q., et al. (2017). Clock1a affects mesoderm development and primitive hematopoiesis by regulating Nodal-Smad3 signaling in the zebrafish embryo. J. Biol. Chem. 292, 14165–14175. doi:10.1074/jbc.M117.794289
Blaty, J. L., and DelRosso, L. M. (2022). Tourette disorder and sleep. Biomed. J. 45, 240–249. doi:10.1016/j.bj.2022.01.002
Bogin, B. (1979). Monthly changes in the gain and loss of growth in weight of children living in Guatemala. Am. J. Phys. Anthropol. 51, 287–291. doi:10.1002/ajpa.1330510215
Bogin, B. A. (1978). Seasonal pattern in the rate of growth in height of children living in Guatemala. Am. J. Phys. Anthropol. 49, 205–210. doi:10.1002/ajpa.1330490208
Buhr, E. D., and Takahashi, J. S. (2013). “Molecular components of the Mammalian circadian clock,” in Handbook of experimental pharmacology, 3–27.
Bunger, M. K., Wilsbacher, L. D., Moran, S. M., Clendenin, C., Radcliffe, L. A., Hogenesch, J. B., et al. (2000). Mop3 is an essential component of the master circadian pacemaker in mammals. Cell 103, 1009–1017. doi:10.1016/s0092-8674(00)00205-1
Burgermeister, E., Battaglin, F., Eladly, F., Wu, W., Herweck, F., Schulte, N., et al. (2019). Aryl hydrocarbon receptor nuclear translocator-like (ARNTL/BMAL1) is associated with bevacizumab resistance in colorectal cancer via regulation of vascular endothelial growth factor A. EBioMedicine 45, 139–154. doi:10.1016/j.ebiom.2019.07.004
Cardone, L., Hirayama, J., Giordano, F., Tamaru, T., Palvimo, J. J., and Sassone-Corsi, P. (2005). Circadian clock control by SUMOylation of BMAL1. Sci. (New York, NY) 309, 1390–1394. doi:10.1126/science.1110689
Casey, T., Suarez-Trujillo, A., Cummings, S., Huff, K., Crodian, J., Bhide, K., et al. (2021). Core circadian clock transcription factor BMAL1 regulates mammary epithelial cell growth, differentiation, and milk component synthesis. PloS one 16, e0248199. doi:10.1371/journal.pone.0248199
Casey, T. M., Crodian, J., Erickson, E., Kuropatwinski, K. K., Gleiberman, A. S., and Antoch, M. P. (2014). Tissue-specific changes in molecular clocks during the transition from pregnancy to lactation in mice. Biol. reproduction 90, 127. doi:10.1095/biolreprod.113.116137
Chakraborty, R., Ostrin, L. A., Nickla, D. L., Iuvone, P. M., Pardue, M. T., and Stone, R. A. (2018). Circadian rhythms, refractive development, and myopia. Ophthalmic and physiological Opt. J. Br. Coll. Ophthalmic Opt. (Optometrists). 38, 217–245. doi:10.1111/opo.12453
Chan, W. C. W., Tan, Z., To, M. K. T., and Chan, D. (2021). Regulation and role of transcription factors in osteogenesis. Int. J. Mol. Sci. 22, 5445. doi:10.3390/ijms22115445
Chatterjee, S., and Ma, K. (2016). Circadian clock regulation of skeletal muscle growth and repair. F1000Research 5, 1549. doi:10.12688/f1000research.9076.1
Chatterjee, S., Nam, D., Guo, B., Kim, J. M., Winnier, G. E., Lee, J., et al. (2013). Brain and muscle Arnt-like 1 is a key regulator of myogenesis. J. Cell Sci. 126, 2213–2224. doi:10.1242/jcs.120519
Chatterjee, S., Yin, H., Nam, D., Li, Y., and Ma, K. (2015). Brain and muscle Arnt-like 1 promotes skeletal muscle regeneration through satellite cell expansion. Exp. Cell Res. 331, 200–210. doi:10.1016/j.yexcr.2014.08.041
Chen, D. S., and Mellman, I. (2017). Elements of cancer immunity and the cancer-immune set point. Nature 541, 321–330. doi:10.1038/nature21349
Chen, L., Zhao, J., Tang, Q., Li, H., Zhang, C., Yu, R., et al. (2016). PFKFB3 control of cancer growth by responding to circadian clock outputs. Sci. Rep. 6, 24324. doi:10.1038/srep24324
Chu, G., Yoshida, K., Narahara, S., Uchikawa, M., Kawamura, M., Yamauchi, N., et al. (2011). Alterations of circadian clockworks during differentiation and apoptosis of rat ovarian cells. Chronobiology Int. 28, 477–487. doi:10.3109/07420528.2011.589933
Cirillo, F., Catellani, C., Lazzeroni, P., Sartori, C., and Street, M. E. (2020). The role of MicroRNAs in influencing body growth and development. Hormone Res. Paediatr. 93, 7–15. doi:10.1159/000504669
Civelek, E., Ozturk Civelek, D., Akyel, Y. K., Kaleli Durman, D., and Okyar, A. (2023). Circadian dysfunction in adipose tissue: chronotherapy in metabolic diseases. Biology 12, 1077. doi:10.3390/biology12081077
Crespo, M., Leiva, M., and Sabio, G. (2021). Circadian clock and liver cancer. Cancers 13, 3631. doi:10.3390/cancers13143631
Cronin, P., McCarthy, M. J., Lim, A. S. P., Salmon, D. P., Galasko, D., Masliah, E., et al. (2017). Circadian alterations during early stages of Alzheimer's disease are associated with aberrant cycles of DNA methylation in BMAL1. Alzheimer's dementia J. Alzheimer's Assoc. 13, 689–700. doi:10.1016/j.jalz.2016.10.003
Dan, H., Ruan, T., and Sampogna, R. V. (2020). Circadian clock regulation of developmental time in the kidney. Cell Rep. 31, 107661. doi:10.1016/j.celrep.2020.107661
Dapergola, E., Menegazzi, P., Raabe, T., and Hovhanyan, A. (2021). Light stimuli and circadian clock affect neural development in Drosophila melanogaster. Front. Cell Dev. Biol. 9, 595754. doi:10.3389/fcell.2021.595754
Dauchy, R. T., Wren-Dail, M. A., Dupepe, L. M., Hill, S. M., Xiang, S., Anbalagan, M., et al. (2018). Effect of daytime blue-enriched LED light on the nighttime circadian melatonin inhibition of hepatoma 7288CTC warburg effect and progression. Comp. Med. 68, 269–279. doi:10.30802/AALAS-CM-17-000107
Dekhang, R., Wu, C., Smith, K. M., Lamb, T. M., Peterson, M., Bredeweg, E. L., et al. (2017). The neurospora transcription factor ADV-1 transduces light signals and temporal information to control rhythmic expression of genes involved in cell fusion. G3 (Bethesda, Md) 7, 129–142. doi:10.1534/g3.116.034298
De Lazzari, F., Bisaglia, M., Zordan, M. A., and Sandrelli, F. (2018). Circadian rhythm abnormalities in Parkinson's disease from humans to flies and back. Int. J. Mol. Sci. 19, 3911. doi:10.3390/ijms19123911
Dickey, J. T., and Swanson, P. (1998). Effects of sex steroids on gonadotropin (FSH and LH) regulation in coho salmon (Oncorhynchus kisutch). J. Mol. Endocrinol. 21, 291–306. doi:10.1677/jme.0.0210291
Dolatshad, H., Campbell, E. A., O'Hara, L., Maywood, E. S., Hastings, M. H., and Johnson, M. H. (2006). Developmental and reproductive performance in circadian mutant mice. Hum. Reprod. Oxf. Engl. 21, 68–79. doi:10.1093/humrep/dei313
Dudek, M., Gossan, N., Yang, N., Im, H. J., Ruckshanthi, J. P., Yoshitane, H., et al. (2016). The chondrocyte clock gene Bmal1 controls cartilage homeostasis and integrity. J. Clin. investigation 126, 365–376. doi:10.1172/JCI82755
Dugoff, L. (2002). Ultrasound diagnosis of structural abnormalities in the first trimester. Prenat. Diagn. 22, 316–320. doi:10.1002/pd.309
Dunlap, J. C., and Loros, J. J. (2006). How fungi keep time: circadian system in Neurospora and other fungi. Curr. Opin. Microbiol. 9, 579–587. doi:10.1016/j.mib.2006.10.008
Dunlap, J. C., Loros, J. J., Colot, H. V., Mehra, A., Belden, W. J., Shi, M., et al. (2007). A circadian clock in Neurospora: how genes and proteins cooperate to produce a sustained, entrainable, and compensated biological oscillator with a period of about a day. Cold Spring Harb. symposia quantitative Biol. 72, 57–68. doi:10.1101/sqb.2007.72.072
Egstrand, S., Olgaard, K., and Lewin, E. (2020). Circadian rhythms of mineral metabolism in chronic kidney disease-mineral bone disorder. Curr. Opin. Nephrol. Hypertens. 29, 367–377. doi:10.1097/MNH.0000000000000611
Fekry, B., Ribas-Latre, A., Baumgartner, C., Deans, J. R., Kwok, C., Patel, P., et al. (2018). Incompatibility of the circadian protein BMAL1 and HNF4α in hepatocellular carcinoma. Nat. Commun. 9, 4349. doi:10.1038/s41467-018-06648-6
Fernández-Palanca, P., Méndez-Blanco, C., Fondevila, F., Tuñón, M. J., Reiter, R. J., Mauriz, J. L., et al. (2021). Melatonin as an antitumor agent against liver cancer: an updated systematic review. Antioxidants Basel, Switz. 10, 103. doi:10.3390/antiox10010103
Feskanich, D., Hankinson, S. E., and Schernhammer, E. S. (2009). Nightshift work and fracture risk: the nurses' health study. Osteoporos. Int. 20, 537–542. doi:10.1007/s00198-008-0729-5
Fu, L., and Lee, C. C. (2003). The circadian clock: pacemaker and tumour suppressor. Nat. Rev. Cancer 3, 350–361. doi:10.1038/nrc1072
Fu, L., Patel, M. S., Bradley, A., Wagner, E. F., and Karsenty, G. (2005). The molecular clock mediates leptin-regulated bone formation. Cell 122, 803–815. doi:10.1016/j.cell.2005.06.028
Gajewski, T. F., Schreiber, H., and Fu, Y. X. (2013). Innate and adaptive immune cells in the tumor microenvironment. Nat. Immunol. 14, 1014–1022. doi:10.1038/ni.2703
Gao, C., Han, H. B., Tian, X. Z., Tan, D. X., Wang, L., Zhou, G. B., et al. (2012). Melatonin promotes embryonic development and reduces reactive oxygen species in vitrified mouse 2-cell embryos. J. pineal Res. 52, 305–311. doi:10.1111/j.1600-079X.2011.00944.x
Gao, X., Wei, Y., Sun, H., Hao, S., Ma, M., Sun, H., et al. (2023). Role of Bmal1 in type 2 diabetes mellitus-related glycolipid metabolic disorder and neuropsychiatric injury: involved in the regulation of synaptic plasticity and circadian rhythms. Mol. Neurobiol. 60, 4595–4617. doi:10.1007/s12035-023-03360-5
Garaulet, M., Ordovás, J. M., and Madrid, J. A. (2005)2010). The chronobiology, etiology and pathophysiology of obesity. Int. J. Obes. 34, 1667–1683. doi:10.1038/ijo.2010.118
Gekakis, N., Staknis, D., Nguyen, H. B., Davis, F. C., Wilsbacher, L. D., King, D. P., et al. (1998). Role of the CLOCK protein in the mammalian circadian mechanism. Sci. (New York, NY) 280, 1564–1569. doi:10.1126/science.280.5369.1564
Gerstner, J. R. (2010). The aging clock: to 'BMAL'icious toward learning and memory. Aging 2, 251–254. doi:10.18632/aging.100144
Gerstner, J. R., Smith, G. G., Lenz, O., Perron, I. J., Buono, R. J., and Ferraro, T. N. (2014). BMAL1 controls the diurnal rhythm and set point for electrical seizure threshold in mice. Front. Syst. Neurosci. 8, 121. doi:10.3389/fnsys.2014.00121
Gery, S., Gombart, A. F., Yi, W. S., Koeffler, C., Hofmann, W. K., and Koeffler, H. P. (2005). Transcription profiling of C/EBP targets identifies Per2 as a gene implicated in myeloid leukemia. Blood 106, 2827–2836. doi:10.1182/blood-2005-01-0358
Gery, S., Komatsu, N., Baldjyan, L., Yu, A., Koo, D., and Koeffler, H. P. (2006). The circadian gene per1 plays an important role in cell growth and DNA damage control in human cancer cells. Mol. Cell 22, 375–382. doi:10.1016/j.molcel.2006.03.038
Gimble, J. M., Floyd, Z. E., and Bunnell, B. A. (2009). The 4th dimension and adult stem cells: can timing be everything? J. Cell. Biochem. 107, 569–578. doi:10.1002/jcb.22153
Glickman, G. (2010). Circadian rhythms and sleep in children with autism. Neurosci. Biobehav. Rev. 34, 755–768. doi:10.1016/j.neubiorev.2009.11.017
Gonçalves, C. F., and Meng, Q. J. (2019). Timing metabolism in cartilage and bone: links between circadian clocks and tissue homeostasis. J. Endocrinol. 243, R29–R46. doi:10.1530/JOE-19-0256
Gossan, N., Zeef, L., Hensman, J., Hughes, A., Bateman, J. F., Rowley, L., et al. (2013). The circadian clock in murine chondrocytes regulates genes controlling key aspects of cartilage homeostasis. Arthritis rheumatism 65, 2334–2345. doi:10.1002/art.38035
Gotter, A. L., Manganaro, T., Weaver, D. R., Kolakowski, L. F., Possidente, B., Sriram, S., et al. (2000). A time-less function for mouse timeless. Nat. Neurosci. 3, 755–756. doi:10.1038/77653
Greene, M. W. (2012). Circadian rhythms and tumor growth. Cancer Lett. 318, 115–123. doi:10.1016/j.canlet.2012.01.001
Grimaldi, B., Bellet, M. M., Katada, S., Astarita, G., Hirayama, J., Amin, R. H., et al. (2010). PER2 controls lipid metabolism by direct regulation of PPARγ. Cell metab. 12, 509–520. doi:10.1016/j.cmet.2010.10.005
Hadadi, E., Taylor, W., Li, X. M., Aslan, Y., Villote, M., Rivière, J., et al. (2020). Chronic circadian disruption modulates breast cancer stemness and immune microenvironment to drive metastasis in mice. Nat. Commun. 11, 3193. doi:10.1038/s41467-020-16890-6
Hanyu, R., Hayata, T., Nagao, M., Saita, Y., Hemmi, H., Notomi, T., et al. (2011). Per-1 is a specific clock gene regulated by parathyroid hormone (PTH) signaling in osteoblasts and is functional for the transcriptional events induced by PTH. J. Cell. Biochem. 112, 433–438. doi:10.1002/jcb.22957
Hardy, K., and Spanos, S. (2002). Growth factor expression and function in the human and mouse preimplantation embryo. J. Endocrinol. 172, 221–236. doi:10.1677/joe.0.1720221
Härmä, M. I., and Ilmarinen, J. E. (1999). Towards the 24-hour society--new approaches for aging shift workers? Scand. J. work, Environ. health 25, 610–615. doi:10.5271/sjweh.488
Hastings, M. H., Reddy, A. B., and Maywood, E. S. (2003). A clockwork web: circadian timing in brain and periphery, in health and disease. Nat. Rev. Neurosci. 4, 649–661. doi:10.1038/nrn1177
Hermida, R. C., Crespo, J. J., Domínguez-Sardiña, M., Otero, A., Moyá, A., Ríos, M. T., et al. (2019). Bedtime hypertension treatment improves cardiovascular risk reduction: the Hygia Chronotherapy Trial. Eur. Heart J. 41, 4565–4576. doi:10.1093/eurheartj/ehz754
Hinoi, E., Ueshima, T., Hojo, H., Iemata, M., Takarada, T., and Yoneda, Y. (2006). Up-regulation of per mRNA expression by parathyroid hormone through a protein kinase A-CREB-dependent mechanism in chondrocytes. J. Biol. Chem. 281, 23632–23642. doi:10.1074/jbc.M512362200
Hoshino, K., Wakatsuki, Y., Iigo, M., and Shibata, S. (2006). Circadian Clock mutation in dams disrupts nursing behavior and growth of pups. Endocrinology 147, 1916–1923. doi:10.1210/en.2005-1343
Hu, K., Ivanov, P., Hilton, M. F., Chen, Z., Ayers, R. T., Stanley, H. E., et al. (2004). Endogenous circadian rhythm in an index of cardiac vulnerability independent of changes in behavior. Proc. Natl. Acad. Sci. U. S. A. 101, 18223–18227. doi:10.1073/pnas.0408243101
Hu, X., Liu, X., Moisan, J., Wang, Y., Lesch, C. A., Spooner, C., et al. (2016). Synthetic RORγ agonists regulate multiple pathways to enhance antitumor immunity. Oncoimmunology 5, e1254854. doi:10.1080/2162402X.2016.1254854
Hunt, T., and Sassone-Corsi, P. (2007). Riding tandem: circadian clocks and the cell cycle. Cell 129, 461–464. doi:10.1016/j.cell.2007.04.015
Itani, N., Skeffington, K. L., Beck, C., Niu, Y., and Giussani, D. A. (2016). Melatonin rescues cardiovascular dysfunction during hypoxic development in the chick embryo. J. pineal Res. 60, 16–26. doi:10.1111/jpi.12283
Janich, P., Pascual, G., Merlos-Suárez, A., Batlle, E., Ripperger, J., Albrecht, U., et al. (2011). The circadian molecular clock creates epidermal stem cell heterogeneity. Nature 480, 209–214. doi:10.1038/nature10649
Jensen, L. D., and Cao, Y. (2013). Clock controls angiogenesis. Cell cycleGeorget. Tex 12, 405–408. doi:10.4161/cc.23596
Jensen, L. D., Cao, Z., Nakamura, M., Yang, Y., Bräutigam, L., Andersson, P., et al. (2012). Opposing effects of circadian clock genes bmal1 and period2 in regulation of VEGF-dependent angiogenesis in developing zebrafish. Cell Rep. 2, 231–241. doi:10.1016/j.celrep.2012.07.005
Kasahara, T., Abe, K., Mekada, K., Yoshiki, A., and Kato, T. (2010). Genetic variation of melatonin productivity in laboratory mice under domestication. Proc. Natl. Acad. Sci. U. S. A. 107, 6412–6417. doi:10.1073/pnas.0914399107
Kelu, J. J., Pipalia, T. G., and Hughes, S. M. (2020). Circadian regulation of muscle growth independent of locomotor activity. Proc. Natl. Acad. Sci. U. S. A. 117, 31208–31218. doi:10.1073/pnas.2012450117
Kennaway, D. J., Boden, M. J., and Voultsios, A. (2004). Reproductive performance in female Clock Delta19 mutant mice. Reproduction, Fertil. Dev. 16, 801–810. doi:10.1071/rd04023
Kettner, N. M., Voicu, H., Finegold, M. J., Coarfa, C., Sreekumar, A., Putluri, N., et al. (2016). Circadian homeostasis of liver metabolism suppresses hepatocarcinogenesis. Cancer Cell 30, 909–924. doi:10.1016/j.ccell.2016.10.007
Kim, J., Jang, S., Choi, M., Chung, S., Choe, Y., Choe, H. K., et al. (2018). Abrogation of the circadian nuclear receptor REV-erbα exacerbates 6-hydroxydopamine-induced dopaminergic neurodegeneration. Mol. cells 41, 742–752. doi:10.14348/molcells.2018.0201
King, D. P., and Takahashi, J. S. (2000). Molecular genetics of circadian rhythms in mammals. Annu. Rev. Neurosci. 23, 713–742. doi:10.1146/annurev.neuro.23.1.713
King, D. P., Vitaterna, M. H., Chang, A. M., Dove, W. F., Pinto, L. H., Turek, F. W., et al. (1997). The mouse Clock mutation behaves as an antimorph and maps within the W19H deletion, distal of Kit. Genetics 146, 1049–1060. doi:10.1093/genetics/146.3.1049
Kissling, C., Retz, W., Wiemann, S., Coogan, A. N., Clement, R. M., Hünnerkopf, R., et al. (2008). A polymorphism at the 3'-untranslated region of the CLOCK gene is associated with adult attention-deficit hyperactivity disorder. Am. J. Med. Genet. Part B, Neuropsychiatric Genet. 147, 333–338. doi:10.1002/ajmg.b.30602
Knutsson, A. (1995). Increased risk of ischaemic heart disease in shift workers. Occup. Med. Oxf. Engl. 45, 55. doi:10.1093/occmed/45.1.55-a
Kondratov, R. V., Chernov, M. V., Kondratova, A. A., Gorbacheva, V. Y., Gudkov, A. V., and Antoch, M. P. (2003). BMAL1-dependent circadian oscillation of nuclear CLOCK: posttranslational events induced by dimerization of transcriptional activators of the mammalian clock system. Genes and Dev. 17, 1921–1932. doi:10.1101/gad.1099503
Kondratov, R. V., Kondratova, A. A., Gorbacheva, V. Y., Vykhovanets, O. V., and Antoch, M. P. (2006). Early aging and age-related pathologies in mice deficient in BMAL1, the core componentof the circadian clock. Genes and Dev. 20, 1868–1873. doi:10.1101/gad.1432206
Krishnan, N., Kretzschmar, D., Rakshit, K., Chow, E., and Giebultowicz, J. M. (2009). The circadian clock gene period extends healthspan in aging Drosophila melanogaster. Aging 1, 937–948. doi:10.18632/aging.100103
Kunimoto, T., Okubo, N., Minami, Y., Fujiwara, H., Hosokawa, T., Asada, M., et al. (2016). A PTH-responsive circadian clock operates in ex vivo mouse femur fracture healing site. Sci. Rep. 6, 22409. doi:10.1038/srep22409
Lacruz, R. S., Hacia, J. G., Bromage, T. G., Boyde, A., Lei, Y., Xu, Y., et al. (2012). The circadian clock modulates enamel development. J. Biol. rhythms 27, 237–245. doi:10.1177/0748730412442830
Laermans, J., and Depoortere, I. (2016). Chronobesity: role of the circadian system in the obesity epidemic. Obes. Rev. official J. Int. Assoc. Study Obes. 17, 108–125. doi:10.1111/obr.12351
Lamia, K. A., Sachdeva, U. M., DiTacchio, L., Williams, E. C., Alvarez, J. G., Egan, D. F., et al. (2009). AMPK regulates the circadian clock by cryptochrome phosphorylation and degradation. Sci. (New York, NY) 326, 437–440. doi:10.1126/science.1172156
Landgraf, D., McCarthy, M. J., and Welsh, D. K. (2014). Circadian clock and stress interactions in the molecular biology of psychiatric disorders. Curr. psychiatry Rep. 16, 483. doi:10.1007/s11920-014-0483-7
Lee, S., Donehower, L. A., Herron, A. J., Moore, D. D., and Fu, L. (2010). Disrupting circadian homeostasis of sympathetic signaling promotes tumor development in mice. PloS one 5, e10995. doi:10.1371/journal.pone.0010995
Leung, C., Gérard, C., and Gonze, D. (2023). Modeling the circadian control of the cell cycle and its consequences for cancer chronotherapy. Biology 12, 612. doi:10.3390/biology12040612
Lévi, F. A., Okyar, A., Hadadi, E., Innominato, P. F., and Ballesta, A. (2024). Circadian regulation of drug responses: toward sex-specific and personalized chronotherapy. Annu. Rev. Pharmacol. Toxicol. 64, 89–114. doi:10.1146/annurev-pharmtox-051920-095416
Li, H. X., Fu, X. J., Yang, K., Chen, D., Tang, H., and Zhao, Q. (2016). The clock gene PER1 suppresses expression of tumor-related genes in human oral squamous cell carcinoma. Oncotarget 7, 20574–20583. doi:10.18632/oncotarget.7827
Li, Z., Ruan, L., Lin, S., and Gittes, G. K. (2007). Clock controls timing of mouse pancreatic differentiation through regulation of Wnt- and Notch-based and cell division components. Biochem. biophysical Res. Commun. 359, 491–496. doi:10.1016/j.bbrc.2007.05.156
Lowrey, P. L., Shimomura, K., Antoch, M. P., Yamazaki, S., Zemenides, P. D., Ralph, M. R., et al. (2000). Positional syntenic cloning and functional characterization of the mammalian circadian mutation tau. Sci. (New York, NY) 288, 483–492. doi:10.1126/science.288.5465.483
Luo, B., Zhou, X., Tang, Q., Yin, Y., Feng, G., Li, S., et al. (2021). Circadian rhythms affect bone reconstruction by regulating bone energy metabolism. J. Transl. Med. 19, 410. doi:10.1186/s12967-021-03068-x
Ma, Z., Jin, X., Qian, Z., Li, F., Xu, M., Zhang, Y., et al. (2019). Deletion of clock gene Bmal1 impaired the chondrocyte function due to disruption of the HIF1α-VEGF signaling pathway. Cell cycleGeorget. Tex 18, 1473–1489. doi:10.1080/15384101.2019.1620572
Maiese, K. (2017). Moving to the rhythm with clock (circadian) genes, autophagy, mTOR, and SIRT1 in degenerative disease and cancer. Curr. neurovascular Res. 14, 299–304. doi:10.2174/1567202614666170718092010
Maiese, K. (2021). Cognitive impairment and dementia: gaining insight through circadian clock gene pathways. Biomolecules 11, 1002. doi:10.3390/biom11071002
Marco, E. M., Velarde, E., Llorente, R., and Laviola, G. (2016). Disrupted circadian rhythm as a common player in developmental models of neuropsychiatric disorders. Curr. Top. Behav. Neurosci. 29, 155–181. doi:10.1007/7854_2015_419
Maronde, E., Schilling, A. F., Seitz, S., Schinke, T., Schmutz, I., van der Horst, G., et al. (2010). The clock genes Period 2 and Cryptochrome 2 differentially balance bone formation. PLoS One 5 (7), e11527. doi:10.1371/journal.pone.0011527
Martino, T. A., Oudit, G. Y., Herzenberg, A. M., Tata, N., Koletar, M. M., Kabir, G. M., et al. (2008). Circadian rhythm disorganization produces profound cardiovascular and renal disease in hamsters. Am. J. physiology Regul. Integr. Comp. physiology 294, R1675–R1683. doi:10.1152/ajpregu.00829.2007
Maryanovich, M., and Frenette, P. S. (2016). A time bomb for leukemia. Cell 165, 262–263. doi:10.1016/j.cell.2016.03.024
McQueen, C. M., Schmitt, E. E., Sarkar, T. R., Elswood, J., Metz, R. P., Earnest, D., et al. (2018). PER2 regulation of mammary gland development. Dev. Camb. Engl. 145, dev157966. doi:10.1242/dev.157966
Metz, R. P., Qu, X., Laffin, B., Earnest, D., and Porter, W. W. (2006). Circadian clock and cell cycle gene expression in mouse mammary epithelial cells and in the developing mouse mammary gland. Dev. Dyn. official Publ. Am. Assoc. Anatomists 235, 263–271. doi:10.1002/dvdy.20605
Milagro, F. I., Gómez-Abellán, P., Campión, J., Martínez, J. A., Ordovás, J. M., and Garaulet, M. (2012). CLOCK, PER2 and BMAL1 DNA methylation: association with obesity and metabolic syndrome characteristics and monounsaturated fat intake. Chronobiology Int. 29, 1180–1194. doi:10.3109/07420528.2012.719967
Miller, B. H., McDearmon, E. L., Panda, S., Hayes, K. R., Zhang, J., Andrews, J. L., et al. (2007). Circadian and CLOCK-controlled regulation of the mouse transcriptome and cell proliferation. Proc. Natl. Acad. Sci. U. S. A. 104, 3342–3347. doi:10.1073/pnas.0611724104
Miller, B. H., Olson, S. L., Turek, F. W., Levine, J. E., Horton, T. H., and Takahashi, J. S. (2004). Circadian clock mutation disrupts estrous cyclicity and maintenance of pregnancy. Curr. Biol. CB 14, 1367–1373. doi:10.1016/j.cub.2004.07.055
Mohri, T., Emoto, N., Nonaka, H., Fukuya, H., Yagita, K., Okamura, H., et al. (2003). Alterations of circadian expressions of clock genes in Dahl salt-sensitive rats fed a high-salt diet. Hypertens. (Dallas, Tex 1979) 42, 189–194. doi:10.1161/01.HYP.0000082766.63952.49
Moore, R. Y., and Lenn, N. J. (1972). A retinohypothalamic projection in the rat. J. Comp. neurology 146, 1–14. doi:10.1002/cne.901460102
Moreno, J. P., Crowley, S. J., Alfano, C. A., Hannay, K. M., Thompson, D., and Baranowski, T. (2019). Potential circadian and circannual rhythm contributions to the obesity epidemic in elementary school age children. Int. J. Behav. Nutr. Phys. activity 16, 25. doi:10.1186/s12966-019-0784-7
Morgan, M. N., Dvuchbabny, S., Martinez, C. A., Kerr, B., Cistulli, P. A., and Cook, K. M. (2019). The cancer clock is (not) ticking: links between circadian rhythms and cancer. Clocks sleep 1, 435–458. doi:10.3390/clockssleep1040034
Morris, C. J., Aeschbach, D., and Scheer, F. A. (2012). Circadian system, sleep and endocrinology. Mol. Cell. Endocrinol. 349, 91–104. doi:10.1016/j.mce.2011.09.003
Murphy, B. A., Blake, C. M., Brown, J. A., Martin, A. M., Forde, N., Sweeney, L. M., et al. (2015). Evidence of a molecular clock in the ovine ovary and the influence of photoperiod. Theriogenology 84, 208–216. doi:10.1016/j.theriogenology.2015.03.008
Nawrot, T. S., Saenen, N. D., Schenk, J., Janssen, B. G., Motta, V., Tarantini, L., et al. (2018). Placental circadian pathway methylation and in utero exposure to fine particle air pollution. Environ. Int. 114, 231–241. doi:10.1016/j.envint.2018.02.034
Nishikawa, S., Hatanaka, Y., Tokoro, M., Shin, S. W., Shimizu, N., Nishihara, T., et al. (2013). Functional analysis of nocturnin, a circadian deadenylase, at maternal-to-zygotic transition in mice. J. reproduction Dev. 59, 258–265. doi:10.1262/jrd.2013-001
Niu, Y. J., Zhou, W., Nie, Z. W., Shin, K. T., and Cui, X. S. (2020). Melatonin enhances mitochondrial biogenesis and protects against rotenone-induced mitochondrial deficiency in early porcine embryos. J. pineal Res. 68, e12627. doi:10.1111/jpi.12627
Noda, M., Iwamoto, I., Tabata, H., Yamagata, T., Ito, H., and Nagata, K. I. (2019). Role of Per3, a circadian clock gene, in embryonic development of mouse cerebral cortex. Sci. Rep. 9, 5874. doi:10.1038/s41598-019-42390-9
Obana-Koshino, A., Ono, H., Miura, J., Sakai, M., Uchida, H., Nakamura, W., et al. (2015). Melatonin inhibits embryonic salivary gland branching morphogenesis by regulating both epithelial cell adhesion and morphology. PloS one 10, e0119960. doi:10.1371/journal.pone.0119960
Ohtsuka-Isoya, M., Hayashi, H., and Shinoda, H. (2001). Effect of suprachiasmatic nucleus lesion on circadian dentin increment in rats. Am. J. physiology Regul. Integr. Comp. physiology 280, R1364–R1370. doi:10.1152/ajpregu.2001.280.5.R1364
Oishi, Y., Hayashi, S., Isagawa, T., Oshima, M., Iwama, A., Shimba, S., et al. (2017). Bmal1 regulates inflammatory responses in macrophages by modulating enhancer RNA transcription. Sci. Rep. 7, 7086. doi:10.1038/s41598-017-07100-3
Okubo, N., Fujiwara, H., Minami, Y., Kunimoto, T., Hosokawa, T., Umemura, Y., et al. (2015). Parathyroid hormone resets the cartilage circadian clock of the organ-cultured murine femur. Acta Orthop. 86, 627–631. doi:10.3109/17453674.2015.1029393
Okubo, N., Minami, Y., Fujiwara, H., Umemura, Y., Tsuchiya, Y., Shirai, T., et al. (2013). Prolonged bioluminescence monitoring in mouse ex vivo bone culture revealed persistent circadian rhythms in articular cartilages and growth plates. PloS one 8, e78306. doi:10.1371/journal.pone.0078306
Palomino-Segura, M., and Hidalgo, A. (2021). Circadian immune circuits. J. Exp. Med. 218, e20200798. doi:10.1084/jem.20200798
Papagiannakopoulos, T., Bauer, M. R., Davidson, S. M., Heimann, M., Subbaraj, L., Bhutkar, A., et al. (2016). Circadian rhythm disruption promotes lung tumorigenesis. Cell metab. 24, 324–331. doi:10.1016/j.cmet.2016.07.001
Papantoniou, K., Castaño-Vinyals, G., Espinosa, A., Aragonés, N., Pérez-Gómez, B., Ardanaz, E., et al. (2016). Breast cancer risk and night shift work in a case-control study in a Spanish population. Eur. J. Epidemiol. 31, 867–878. doi:10.1007/s10654-015-0073-y
Papantoniou, K., Castaño-Vinyals, G., Espinosa, A., Aragonés, N., Pérez-Gómez, B., Burgos, J., et al. (2015). Night shift work, chronotype and prostate cancer risk in the MCC-Spain case-control study. Int. J. cancer 137, 1147–1157. doi:10.1002/ijc.29400
Peng, H., Zhu, Y., Goldberg, J., Vaccarino, V., and Zhao, J. (2019). DNA methylation of five core circadian genes jointly contributes to glucose metabolism: a gene-set analysis in monozygotic twins. Front. Genet. 10, 329. doi:10.3389/fgene.2019.00329
Pilorz, V., and Steinlechner, S. (2008). Low reproductive success in Per1 and Per2 mutant mouse females due to accelerated ageing? Reprod. Camb. Engl. 135, 559–568. doi:10.1530/REP-07-0434
Platt, T. E., Foster, G. S., Tarnavsky, G. K., and Reeves, J. J. (1983). Effects of photoperiod and estradiol on tonic gonadotropins in ovariectomized ewes. J. animal Sci. 56, 1180–1185. doi:10.2527/jas1983.5651180x
Prieto-Domínguez, N., Méndez-Blanco, C., Carbajo-Pescador, S., Fondevila, F., García-Palomo, A., González-Gallego, J., et al. (2017). Melatonin enhances sorafenib actions in human hepatocarcinoma cells by inhibiting mTORC1/p70S6K/HIF-1α and hypoxia-mediated mitophagy. Oncotarget 8, 91402–91414. doi:10.18632/oncotarget.20592
Puram, R. V., Kowalczyk, M. S., de Boer, C. G., Schneider, R. K., Miller, P. G., McConkey, M., et al. (2016). Core circadian clock genes regulate leukemia stem cells in AML. Cell 165, 303–316. doi:10.1016/j.cell.2016.03.015
Richter, H. G., Hansell, J. A., Raut, S., and Giussani, D. A. (2009). Melatonin improves placental efficiency and birth weight and increases the placental expression of antioxidant enzymes in undernourished pregnancy. J. pineal Res. 46, 357–364. doi:10.1111/j.1600-079X.2009.00671.x
Rida, P. C., Le Minh, N., and Jiang, Y. J. (2004). A Notch feeling of somite segmentation and beyond. Dev. Biol. 265, 2–22. doi:10.1016/j.ydbio.2003.07.003
Rijo-Ferreira, F., and Takahashi, J. S. (2019). Genomics of circadian rhythms in health and disease. Genome Med. 11, 82. doi:10.1186/s13073-019-0704-0
Riley, L. A., and Esser, K. A. (2017). The role of the molecular clock in skeletal muscle and what it is teaching us about muscle-bone crosstalk. Curr. Osteoporos. Rep. 15, 222–230. doi:10.1007/s11914-017-0363-2
Risely, A., Wilhelm, K., Clutton-Brock, T., Manser, M. B., and Sommer, S. (2021). Diurnal oscillations in gut bacterial load and composition eclipse seasonal and lifetime dynamics in wild meerkats. Nat. Commun. 12, 6017. doi:10.1038/s41467-021-26298-5
Rodríguez, L., Begtashi, I., Zanuy, S., and Carrillo, M. (2005). Long-term exposure to continuous light inhibits precocity in European male sea bass (Dicentrarchus labrax, L.): hormonal aspects. General Comp. Endocrinol. 140, 116–125. doi:10.1016/j.ygcen.2004.10.011
Roenneberg, T., and Merrow, M. (2016). The circadian clock and human health. Curr. Biol. CB 26, R432–R443. doi:10.1016/j.cub.2016.04.011
Roman, E., and Karlsson, O. (2013). Increased anxiety-like behavior but no cognitive impairments in adult rats exposed to constant light conditions during perinatal development. Upsala J. Med. Sci. 118, 222–227. doi:10.3109/03009734.2013.821191
Rozenboim, I., Piestun, Y., Mobarkey, N., Barak, M., Hoyzman, A., and Halevy, O. (2004). Monochromatic light stimuli during embryogenesis enhance embryo development and posthatch growth. Poult. Sci. 83, 1413–1419. doi:10.1093/ps/83.8.1413
Russell, A. L., Miller, L., Yi, H., Keil, R., Handa, R. J., and Wu, T. J. (2021). Knockout of the circadian gene, Per2, disrupts corticosterone secretion and results in depressive-like behaviors and deficits in startle responses. BMC Neurosci. 22, 5. doi:10.1186/s12868-020-00607-y
Sahar, S., and Sassone-Corsi, P. (2012). Regulation of metabolism: the circadian clock dictates the time. Trends Endocrinol. metabolism TEM 23, 1–8. doi:10.1016/j.tem.2011.10.005
Said, R., Lobanova, L., Papagerakis, S., and Papagerakis, P. (2020). Calcium sets the clock in ameloblasts. Front. physiology 11, 920. doi:10.3389/fphys.2020.00920
Samsa, W. E., Vasanji, A., Midura, R. J., and Kondratov, R. V. (2016). Deficiency of circadian clock protein BMAL1 in mice results in a low bone mass phenotype. Bone 84, 194–203. doi:10.1016/j.bone.2016.01.006
Samsa, W. E., Zhou, X., and Zhou, G. (2017). Signaling pathways regulating cartilage growth plate formation and activity. Seminars Cell and Dev. Biol. 62, 3–15. doi:10.1016/j.semcdb.2016.07.008
Sánchez-Vázquez, F. J., López-Olmeda, J. F., Vera, L. M., Migaud, H., López-Patiño, M. A., and Míguez, J. M. (2019). Environmental cycles, melatonin, and circadian control of stress response in fish. Front. Endocrinol. 10, 279. doi:10.3389/fendo.2019.00279
Scheiermann, C., Gibbs, J., Ince, L., and Loudon, A. (2018). Clocking in to immunity. Nat. Rev. Immunol. 18, 423–437. doi:10.1038/s41577-018-0008-4
Schernhammer, E. S., Laden, F., Speizer, F. E., Willett, W. C., Hunter, D. J., Kawachi, I., et al. (2001). Rotating night shifts and risk of breast cancer in women participating in the nurses' health study. J. Natl. Cancer Inst. 93, 1563–1568. doi:10.1093/jnci/93.20.1563
Schibler, U., and Sassone-Corsi, P. (2002). A web of circadian pacemakers. Cell 111, 919–922. doi:10.1016/s0092-8674(02)01225-4
Schmutz, I., Ripperger, J. A., Baeriswyl-Aebischer, S., and Albrecht, U. (2010). The mammalian clock component PERIOD2 coordinates circadian output by interaction with nuclear receptors. Genes and Dev. 24, 345–357. doi:10.1101/gad.564110
Sellix, M. T., and Menaker, M. (2010). Circadian clocks in the ovary. Trends Endocrinol. metabolism TEM 21, 628–636. doi:10.1016/j.tem.2010.06.002
Shahed, A., and Young, K. A. (2013). Anti-Müllerian hormone (AMH), inhibin-α, growth differentiation factor 9 (GDF9), and bone morphogenic protein-15 (BMP15) mRNA and protein are influenced by photoperiod-induced ovarian regression and recrudescence in Siberian hamster ovaries. Mol. reproduction Dev. 80, 895–907. doi:10.1002/mrd.22215
Shen, Y., Lv, Q. K., Xie, W. Y., Gong, S. Y., Zhuang, S., Liu, J. Y., et al. (2023). Circadian disruption and sleep disorders in neurodegeneration. Transl. Neurodegener. 12, 8. doi:10.1186/s40035-023-00340-6
Skrlec, I., and Talapko, J. (2022). Hepatitis B and circadian rhythm of the liver. World J. gastroenterology 28, 3282–3296. doi:10.3748/wjg.v28.i27.3282
Stowe, T. A., and McClung, C. A. (2023). How does chronobiology contribute to the development of diseases in later life. Clin. interventions aging 18, 655–666. doi:10.2147/CIA.S380436
Suyama, K., Silagi, E. S., Choi, H., Sakabe, K., Mochida, J., Shapiro, I. M., et al. (2016). Circadian factors BMAL1 and RORα control HIF-1α transcriptional activity in nucleus pulposus cells: implications in maintenance of intervertebral disc health. Oncotarget 7, 23056–23071. doi:10.18632/oncotarget.8521
Takahashi, J. S. (2017). Transcriptional architecture of the mammalian circadian clock. Nat. Rev. Genet. 18, 164–179. doi:10.1038/nrg.2016.150
Takarada, T., Kodama, A., Hotta, S., Mieda, M., Shimba, S., Hinoi, E., et al. (2012). Clock genes influence gene expression in growth plate and endochondral ossification in mice. J. Biol. Chem. 287, 36081–36095. doi:10.1074/jbc.M112.408963
Takumi, T., Tamada, K., Hatanaka, F., Nakai, N., and Bolton, P. F. (2020). Behavioral neuroscience of autism. Neurosci. Biobehav. Rev. 110, 60–76. doi:10.1016/j.neubiorev.2019.04.012
Tamimi, Z., Abusamak, M., Al-Waeli, H., Al-Tamimi, M., Al Habashneh, R., Ghanim, M., et al. (2022). NSAID chronotherapy after impacted third molar extraction: a randomized controlled trial. Oral Maxillofac. Surg. 26, 663–672. doi:10.1007/s10006-021-01029-8
Tao, J., Zhai, Y., Park, H., Han, J., Dong, J., Xie, M., et al. (2016). Circadian rhythm regulates development of enamel in mouse mandibular first molar. PloS one 11, e0159946. doi:10.1371/journal.pone.0159946
Tchekalarova, J., Kortenska, L., Marinov, P., and Ivanova, N. (2022). Sex-dependent effects of piromelatine treatment on sleep-wake cycle and sleep structure of prenatally stressed rats. Int. J. Mol. Sci. 23, 10349. doi:10.3390/ijms231810349
Thompson, R. S., Gaffney, M., Hopkins, S., Kelley, T., Gonzalez, A., Bowers, S. J., et al. (2021). Ruminiclostridium 5, Parabacteroides distasonis, and bile acid profile are modulated by prebiotic diet and associate with facilitated sleep/clock realignment after chronic disruption of rhythms. Brain, Behav. Immun. 97, 150–166. doi:10.1016/j.bbi.2021.07.006
Tokumaru, O., Haruki, K., Bacal, K., Katagiri, T., Yamamoto, T., and Sakurai, Y. (2006). Incidence of cancer among female flight attendants: a meta-analysis. J. travel Med. 13, 127–132. doi:10.1111/j.1708-8305.2006.00029.x
Torres-Farfan, C., Valenzuela, F. J., Germain, A. M., Viale, M. L., Campino, C., Torrealba, F., et al. (2006). Maternal melatonin stimulates growth and prevents maturation of the capuchin monkey fetal adrenal gland. J. pineal Res. 41, 58–66. doi:10.1111/j.1600-079X.2006.00331.x
Trott, A. J., and Menet, J. S. (2018). Regulation of circadian clock transcriptional output by CLOCK:BMAL1. PLoS Genet. 14, e1007156. doi:10.1371/journal.pgen.1007156
Uth, K., and Sleigh, R. (2014). Deregulation of the circadian clock constitutes a significant factor in tumorigenesis: a clockwork cancer. Part II. in vivo studies. Biotechnol. Biotechnol. Equip. 28, 379–386. doi:10.1080/13102818.2014.925298
Vaccaro, A., Issa, A. R., Seugnet, L., Birman, S., and Klarsfeld, A. (2017). Drosophila clock is required in brain pacemaker neurons to prevent premature locomotor aging independently of its circadian function. PLoS Genet. 13, e1006507. doi:10.1371/journal.pgen.1006507
Valnegri, P., Khelfaoui, M., Dorseuil, O., Bassani, S., Lagneaux, C., Gianfelice, A., et al. (2011). A circadian clock in hippocampus is regulated by interaction between oligophrenin-1 and Rev-erbα. Nat. Neurosci. 14, 1293–1301. doi:10.1038/nn.2911
Varcoe, T. J., Boden, M. J., Voultsios, A., Salkeld, M. D., Rattanatray, L., and Kennaway, D. J. (2013). Characterisation of the maternal response to chronic phase shifts during gestation in the rat: implications for fetal metabolic programming. PloS one 8, e53800. doi:10.1371/journal.pone.0053800
Vasal, S., and Sundararaj, B. I. (1976). Response of the ovary in the catfish, Heteropneustes fossilis (Bloch), to various combinations of photoperiod and temperature. J. Exp. zoology 197, 247–263. doi:10.1002/jez.1401970206
Vatine, G., Vallone, D., Gothilf, Y., and Foulkes, N. S. (2011). It's time to swim! Zebrafish and the circadian clock. FEBS Lett. 585, 1485–1494. doi:10.1016/j.febslet.2011.04.007
Verbitsky, M., Westland, R., Perez, A., Kiryluk, K., Liu, Q., Krithivasan, P., et al. (2019). The copy number variation landscape of congenital anomalies of the kidney and urinary tract. Nat. Genet. 51, 117–127. doi:10.1038/s41588-018-0281-y
Vlismas, A., Bletsa, R., Mavrogianni, D., Mamali, G., Pergamali, M., Dinopoulou, V., et al. (2016). Microarray analyses reveal marked differences in growth factor and receptor expression between 8-cell human embryos and pluripotent stem cells. Stem cells Dev. 25, 160–177. doi:10.1089/scd.2015.0284
Walker, W. H., Walton, J. C., DeVries, A. C., and Nelson, R. J. (2020). Circadian rhythm disruption and mental health. Transl. psychiatry 10, 28. doi:10.1038/s41398-020-0694-0
Wang, C., Barnoud, C., Cenerenti, M., Sun, M., Caffa, I., Kizil, B., et al. (2023). Dendritic cells direct circadian anti-tumour immune responses. Nature 614, 136–143. doi:10.1038/s41586-022-05605-0
Wang, Y., Sun, N., Lu, C., Bei, Y., Qian, R., and Hua, L. (2017). Upregulation of circadian gene 'hClock' contribution to metastasis of colorectal cancer. Int. J. Oncol. 50, 2191–2199. doi:10.3892/ijo.2017.3987
Wu, X., Li, D., Liu, J., Diao, L., Ling, S., Li, Y., et al. (2017). Dammarane sapogenins ameliorates neurocognitive functional impairment induced by simulated long-duration spaceflight. Front. Pharmacol. 8, 315. doi:10.3389/fphar.2017.00315
Xiao, J., Li, C., Zhu, N. L., Borok, Z., and Minoo, P. (2003). Timeless in lung morphogenesis. Dev. Dyn. official Publ. Am. Assoc. Anatomists 228, 82–94. doi:10.1002/dvdy.10346
Xu, Y., Zhang, K. H., Sun, M. H., Lan, M., Wan, X., Zhang, Y., et al. (2019). Protective effects of melatonin against zearalenone toxicity on porcine embryos in vitro. Front. Pharmacol. 10, 327. doi:10.3389/fphar.2019.00327
Yang, C., Wu, J., Liu, X., Wang, Y., Liu, B., Chen, X., et al. (2020). Circadian rhythm is disrupted by ZNF704 in breast carcinogenesis. Cancer Res. 80, 4114–4128. doi:10.1158/0008-5472.CAN-20-0493
Yang, N., Williams, J., Pekovic-Vaughan, V., Wang, P., Olabi, S., McConnell, J., et al. (2017). Cellular mechano-environment regulates the mammary circadian clock. Nat. Commun. 8, 14287. doi:10.1038/ncomms14287
Yang, Y., Cheng, P., Zhi, G., and Liu, Y. (2001). Identification of a calcium/calmodulin-dependent protein kinase that phosphorylates the Neurospora circadian clock protein FREQUENCY. J. Biol. Chem. 276, 41064–41072. doi:10.1074/jbc.M106905200
Yong, W., Ma, H., Na, M., Gao, T., Zhang, Y., Hao, L., et al. (2021). Roles of melatonin in the field of reproductive medicine. Biomed. Pharmacother. = Biomedecine Pharmacother. 144, 112001. doi:10.1016/j.biopha.2021.112001
Yuan, G., Xu, L., Cai, T., Hua, B., Sun, N., Yan, Z., et al. (2019). Clock mutant promotes osteoarthritis by inhibiting the acetylation of NFκB. Osteoarthritis Cartilage 27 (6), 922–931. doi:10.1016/j.joca.2019.01.012
Zhang, Y., Fang, B., Emmett, M. J., Damle, M., Sun, Z., Feng, D., et al. (2015). GENE REGULATION. Discrete functions of nuclear receptor Rev-erbα couple metabolism to the clock. Sci. (New York, NY) 348, 1488–1492. doi:10.1126/science.aab3021
Zheng, B., Larkin, D. W., Albrecht, U., Sun, Z. S., Sage, M., Eichele, G., et al. (1999). The mPer2 gene encodes a functional component of the mammalian circadian clock. Nature 400, 169–173. doi:10.1038/22118
Zheng, L., Papagerakis, S., Schnell, S. D., Hoogerwerf, W. A., and Papagerakis, P. (2011). Expression of clock proteins in developing tooth. Gene Expr. patterns GEP. 11, 202–206. doi:10.1016/j.gep.2010.12.002
Zheng, Y., Liu, C., Li, Y., Jiang, H., Yang, P., Tang, J., et al. (2019). Loss-of-function mutations with circadian rhythm regulator Per1/Per2 lead to premature ovarian insufficiency. Biol. reproduction 100, 1066–1072. doi:10.1093/biolre/ioy245
Zhou, L., Zhang, Z., Nice, E., Huang, C., Zhang, W., and Tang, Y. (2022). Circadian rhythms and cancers: the intrinsic links and therapeutic potentials. J. Hematol. Oncol. 15, 21. doi:10.1186/s13045-022-01238-y
Keywords: circadian clock, circadian rhythm, growth and development, metabolism, biological processes
Citation: Luo B, Song J, Zhang J, Han J, Zhou X and Chen L (2024) The contribution of circadian clock to the biological processes. Front. Mol. Biosci. 11:1387576. doi: 10.3389/fmolb.2024.1387576
Received: 18 February 2024; Accepted: 20 May 2024;
Published: 06 June 2024.
Edited by:
Jie Xiong, Shanghai Jiao Tong University, ChinaReviewed by:
Shilpi Minocha, Indian Institute of Technology Delhi, IndiaCopyright © 2024 Luo, Song, Zhang, Han, Zhou and Chen. This is an open-access article distributed under the terms of the Creative Commons Attribution License (CC BY). The use, distribution or reproduction in other forums is permitted, provided the original author(s) and the copyright owner(s) are credited and that the original publication in this journal is cited, in accordance with accepted academic practice. No use, distribution or reproduction is permitted which does not comply with these terms.
*Correspondence: Xin Zhou, emhvdXhpbl83MDlAMTYzLmNvbQ==; Lili Chen, Y2hlbmxpbGkxMDMwQGh1c3QuZWR1LmNu
†These authors have contributed equally to this work and share first authorship
Disclaimer: All claims expressed in this article are solely those of the authors and do not necessarily represent those of their affiliated organizations, or those of the publisher, the editors and the reviewers. Any product that may be evaluated in this article or claim that may be made by its manufacturer is not guaranteed or endorsed by the publisher.
Research integrity at Frontiers
Learn more about the work of our research integrity team to safeguard the quality of each article we publish.