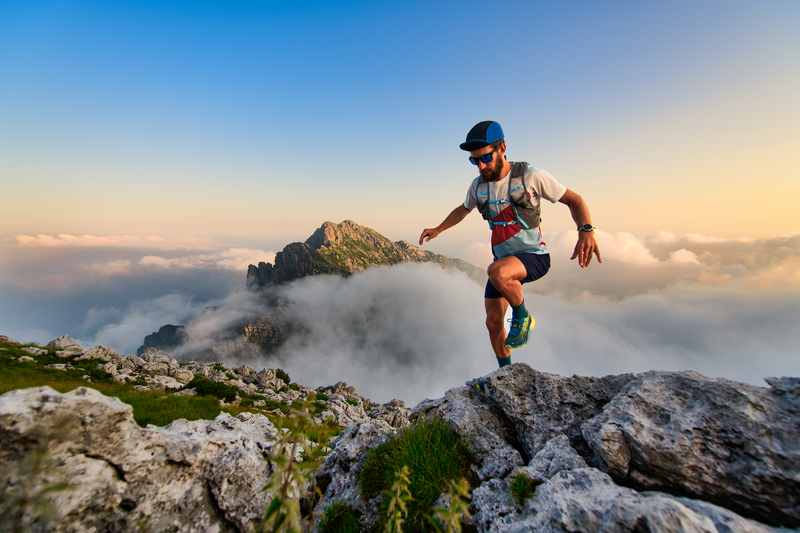
94% of researchers rate our articles as excellent or good
Learn more about the work of our research integrity team to safeguard the quality of each article we publish.
Find out more
REVIEW article
Front. Mol. Biosci. , 04 April 2024
Sec. Molecular Diagnostics and Therapeutics
Volume 11 - 2024 | https://doi.org/10.3389/fmolb.2024.1365760
This article is part of the Research Topic Interplay Between Cancer and the Nervous System: Molecular and Cellular Mechanisms View all 4 articles
The lungs are a key organ in the respiratory system. They are regulated by a complex network of nerves that control their development, structure, function, and response to various pathological stimuli. Accumulating evidence suggests the involvement of a neural mechanism in different pathophysiological conditions in the lungs and the development and progression of common respiratory diseases. Lung diseases are the chief source of death globally. For instance, lung cancer is the second most commonly diagnosed malignancy, after prostate cancer in men and breast cancer in women, and is the most lethal cancer worldwide. However, although airway nerves are accepted as a mechanistically and therapeutically important feature that demands appropriate emphasizing in the context of many respiratory diseases, significantly less is known about the role of the neuroglial cells in lung physiology and pathophysiology, including lung cancer. New data have uncovered some cellular and molecular mechanisms of how Schwann cells, as fundamental components of the peripheral nervous system, may regulate lung cancer cells’ survival, spreading, and invasiveness in vitro and in vivo. Schwann cells control the formation and maintenance of the lung cancer microenvironment and support metastasis formation. It was also reported that the number of lung cancer-associated Schwann cells correlates with patients’ survival. Different factors secreted by Schwann cells, including microRNA, are known to sharpen the lung cancer environment by regulating the tumor-neuro-immune axis. Further clinical and experimental studies are required to elucidate the detailed role of Schwann cells in creating and maintaining pulmonary tumor-neuro-immune axis, which will advance our understanding of the pathogenesis of lung cancer and may inform therapeutic hypotheses aiming neoplasms and metastases in the lung.
Respiratory illnesses are among the most widespread medical obstacles in the world and have a substantial worldwide health influence. For instance, chronic obstructive pulmonary disease (COPD) is the third leading cause of death affecting about 300 million people globally and contributing to more than 3 million deaths (Ruvuna and Sood, 2020). Asthma affects more than 340 million people and an additional 100 million may be affected by 2025 (Dharmage et al., 2019). An estimated ∼11 million people contract tuberculosis every year, and more than 1.5 million people die from tuberculosis annually making it the world’s leading cause of death from an infectious agent (Natarajan et al., 2020). Lung cancer is one of the most commonly diagnosed malignancies and is the most lethal cancer with an estimated annual 2 million new cases and ∼1.8 million deaths worldwide (Thai et al., 2021).
Lungs, as the foundational organs of the respiratory system, are innervated by the peripheral nervous system (PNS) and the vagus nerve that grants the lungs to perform their vital function in the respiratory system, which also includes the nose, oropharynx, larynx, trachea, bronchi, and bronchioles. The airways and lungs are heavily innervated with specific aiming of airway epithelium, smooth muscles, glands, and vasculature. Pulmonary innervation is responsible for dilating and constricting the airway via contraction of smooth muscles in bronchi and bronchioles, bronchial secretions from submucosal glands, vascular permeability, and blood flow. Sensory innervation of the visceral pleura is involved in stretch detection (Arreola-Ramírez et al., 2013).
One important aspect of the functioning of airway nerves is the involvement of a neural mechanism in different pathophysiological conditions in the lungs and the development or progression of common respiratory diseases. The well-established anatomical structure and integrity of airway innervation underscore the contribution of nerves to both the normal airway function and disease pathways that control airway tone, mucus secretion, inflammatory reaction, fibrosis, and other key characteristics (Canning and Spina, 2009; Kistemaker and Prakash, 2019). Accumulating evidence supports the concept that the respiratory neural network may be activated or dysregulated in COPD, asthma, chronic pulmonary fibrosis, pulmonary pain, chronic cough, lung infection, and other acute and chronic inflammatory respiratory diseases (van der Velden and Hulsmann, 1999; Undem and Kollarik, 2005; Undem and Nassenstein, 2009; Mazzone and Undem, 2016; Audrit et al., 2017; Kistemaker and Prakash, 2019; Yegen et al., 2023) For instance, airway nerves not only regulate airway physiology, they also express and secrete various pro- and anti-inflammatory molecules and may control attraction, homing, and activation of different subsets of immune cells (Verhein et al., 2009). Recent data revealed the essential role of local sympathetic innervations in negatively altering the lung’s innate immune reactions (Liu et al., 2020). Similarly, other data demonstrated significant activation of sympathetic nerve activity in patients with pulmonary artery hypertension, which may be associated with disease severity (Velez-Roa et al., 2004; Vaillancourt et al., 2017).
Thus, although airway nerves are accepted as a mechanistically and therapeutically important aspect that demands appropriate emphasizing in the context of many respiratory diseases, significantly less is done to reveal the role of PNS glial cells in lung physiology and pathophysiology.
Schwann cells (SCs), discovered and described by German physiologist, Theodor Schwann, also known as neurolemmocytes, are primary non-neuronal cells, or neuroglial cells, of the PNS that support and regulate the viability and functions of peripheral neurons. Satellite cells, olfactory ensheathing cells, enteric glia, and glial cells at the endings of the sensory nerves are also members of the glial cells in the PNS. SCs differentiate from specific precursor cells in the neural crest and can be segregated into two types: myelinating and non-myelinating SCs. The myelinating SCs produce myelin for axon insulation and form cytoplasmic sheaths surrounding the axon of a single nerve. The non-myelinating Schwann cells line axons with their cytoplasm but do not produce myelin. SCs sustain peripheral nerve longevity under homeostatic conditions, independent of myelination (Eid et al., 2023).
SCs advance the saltatory transmission of nerve impulses as the velocity of electrical impulses along axons is up to ten times enhanced by the myelin sheath. SCs also account for supplying trophic support for neurons. SCs also play a crucial role in nerve regeneration (Balakrishnan et al., 2020). During damage of the PNS neurons, SCs first participate in direct or macrophage-mediated phagocytosis of broken or injured axons, a process known as Wallerian degeneration, associated with SC dedifferentiation, denervation, and proliferation. These repair SCs then form an endoneurial tunnel, which works as a guide track toward regenerating axons growing at a rate of about 1 mm per day. Importantly, axons die if SCs fail to interact with them (Jessen and Mirsky, 2016).
A growing body of evidence on the communication between SCs and immune cells implies SC’s contribution to inflammatory processes. Activated SCs can induce and control local immune reactions by presenting antigens and by expressing cytokines and chemokines that attract immune cells to the site of trauma or injury. Cross-talk with immune cells allows SCs to shape immune reactions that can be advantageous for inflammatory and autoimmune neuropathies (Meyer zu Hörste et al., 2008; Ydens et al., 2013). SCs are now known as antigen-presenting cells supporting neuron survival and preserving axons (Hartlehnert et al., 2017). For instance, recent data characterizing repair SCs revealed high levels of expression of MHC class II molecules and co-stimulatory and co-inhibitory molecules, including CD40, CD80, CD86 CD58 (or LFA-3), and CD276 (or B7-H3) (Berner et al., 2022). The ability of these SCs to produce both pro- and anti-inflammatory cytokines and chemokines, regulate PD-L1-mediated T cell inhibition, and control the activation state of T cells suggest their functional similarities with professional antigen-presenting cells and immune regulators of T cell responses during nerve repair process (Berner et al., 2022). SCs interaction with peripheral nerve resident macrophages may be responsible for cancer-induced pain, at least in some experimental systems (De Logu et al., 2021).
Because SCs have been proven to play an important role in neurotransmission, axon de/regeneration, protection of nerves in the PNS, interaction with immune cells, and shaping the tumor microenvironment, they are a cluster of glial cells of great clinical significance. Different disorders are related to the deterioration, damage, hyperactivation, or malfunction of SCs including demyelinating neuropathies (e.g., inherited, inflammatory, autoimmune), spinal cord injury, neuropathic pain, infections, and cancer. Understanding cellular and molecular mechanisms of SC-associated pathophysiology and progression of these diseases is well justified for improving disease diagnostics, predicting patient outcomes, and developing effective therapeutic approaches. Furthermore, targeting pathological SCs or adoptive transfer of normal or modified SCs is a novel therapeutic modality that should be available soon.
The lungs are highly innervated by the sympathetic, parasympathetic, and sensory branches of the PNS (Downing and Lee, 1980). They are innervated from the pulmonary plexus in the root of each lung and the phrenic nerve, which comes from C3,4,5 cervical nerve roots. Branches from the vagus nerve and sympathetic branches from the cervical cardiac nerves (sympathetic trunk) merge and establish the pulmonary plexus (Figure 1). The plexus is then subdivided into anterior and posterior branches matching the hilum of the lung supplying the bronchi and the visceral pleura (Belvisi, 2002). The vagus nerves, which provide all parasympathetic and most of the sensory nerve fibers to the airways, divide into the superior laryngeal and recurrent laryngeal nerves, which carry sensory fibers and preganglionic parasympathetic fibers to the trachea and main bronchi. Smaller branches of the vagus then supply the rest of the airways. Preganglionic autonomic fibers are myelinated, whereas the postganglionic part is usually unmyelinated. Sympathetic nerves innervating the lung begin in the upper thoracic sections of the spinal cord and the sympathetic ganglia synapse innervating bronchial blood vessels and submucosal glands (Figure 1). The sympathetic nervous system regulates bronchodilation and mucous secretion, while the parasympathetic system regulates bronchoconstriction.
Figure 1. Schematic illustration of lung innervation. The lungs are innervated by the sympathetic, parasympathetic, and sensory branches of the peripheral nervous system. The vagus nerves supply parasympathetic, originated in the medulla oblongata, and sensory, via the nodose and jugular ganglia, nerve fibers to the airways. Additional sensory nerves travel from the dorsal root ganglia of the spinal cord and travel with spinal sympathetic nerves. Sympathetic efferents of the thoracic ganglia of the sympathetic trunk originate in the ventral horn of the spinal cord and run via the pulmonary plexus. Sympathetic nerves: adrenergic. Parasympathetic nerve: cholinergic.
Vagal sensory neurons establish the main afferent stream to the airways and lungs: approximately 20% of afferent vagus nerves terminate in the airways and lungs (Mazzone and Undem, 2016). Nociceptive afferent nerve endings localized in the lung parenchyma and near the airways respond to different noxious stimuli including irritants, allergens, and pathogens. Some more minor sensory innervation originates from the T1-T6 dorsal root ganglia and these fibers run with spinal sympathetic nerves (Lee and Yu, 2014).
Thus, three types of nerve fibers link the lungs to the autonomic part of the PNS (Kistemaker and Prakash, 2019). Two efferent fibers: the parasympathetic fibers in the vagus nerve provide motor stimuli to the airways and lungs inducing bronchiolar muscle contraction, vasodilatation, and gland secretion; and the sympathetic fibers responsible for relaxation of bronchial muscles, vasoconstriction, and suppression of glandular secretion. The third type of nerve fibers in the lung are afferent neurons that are subdivided into A- and C-fibers and the so-called cough receptors (Audrit et al., 2017). The afferent A-fibers in the vagus nerve connect stretch receptors in the airways and the lungs with the pulmonary plexus. The axons of these receptors are myelinated and have high conduction velocities. Another class of airway sensory fiber is the unmyelinated bronchial or pulmonary C-fiber (McGarvey, 2015). Pulmonary C-fiber endings are found in the lung parenchyma, while bronchial C-fibers are seen within the airway mucosa (Canning and Spina, 2009; Su et al., 2021).
Clinical data suggest that nerves in the lungs can be affected by different diseases; for instance, lung cancer can spread to the nerve trunks. Respiratory dysfunctions resulting from neurological diseases are well described (Michael et al., 1999). New studies also highlight the roles of lung-innervating neurons in controlling immune cell activity in asthma, rhinitis, chronic obstructive pulmonary disorder, and lung infections (Blake et al., 2019; De Virgiliis and Di Giovanni, 2020). For instance, vagotomy has been reported to worsen lung infection, inflammation, and injury implying that the pulmonary parasympathetic inflammatory reflex can control the extent of lung infection and inflammation (Huang et al., 2019). Interestingly, PNS nerves have been suggested as an important neuroanatomical component of the bi-directorial brain-lung axis (Li et al., 2023). Functional communication between the lung and the brain is associated with many disorders including lung diseases and CNS diseases.
Lung innervation was also investigated in resected lung specimens from lung adenocarcinoma patients and the results demonstrated a negative correlation between the density of neural fiber expression and recurrence-free survival in patients with lung adenocarcinoma (Shao et al., 2016). Interestingly, sympathetic nerve fiber density was higher in the peritumor area, while parasympathetic nerve fibers were located in the tumor bed. Thus, PNS nerve densities associated with lung adenocarcinoma are negatively correlated with disease prognosis. These data correlate with the report that a comprehensive suppression of sympathetic activity may decrease the risk of developing primary pulmonary tumors in smokers and nicotine addicts (McCarty et al., 2015). Other data revealed that non-small cell lung cancer (NSCLC) patients who used beta-blockers have better disease-free survival and lower rate of metastasis than patients on conventional cancer therapy (Coelho et al., 2020). In fact, beta-blocker propranolol was shown to significantly attenuate the proliferation of lung cancer cell lines (Al-Wadei et al., 2012). Similarly, the blockage of muscarinic cholinergic signaling has been demonstrated to inhibit small cell lung cancer (SCLC) and NSCLC growth in different model systems (Zhao et al., 2015; Spindel, 2016). Similar clinical and experimental results were also reported for different types of solid tumors (Hutchings et al., 2020).
Although the lung hosts both myelinating and non-myelinating SCs, the latter correspond to the amplest SC subpopulation in the lung (Heredia et al., 2023) since almost 90% of nerves in the lung are unmyelinated (Jammes et al., 1982). Non-myelinating SCs in the lung were first described in 1957 (Agostoni et al., 1957) (Table 1), and electron microscopy and immunohistochemical analyses of lung innervation reliably reveal a spatial location of SCs and the axons of pulmonary nerves (Hung et al., 1972; Sparrow et al., 1999; Suarez-Mier and Buckwalter, 2015; Heredia et al., 2023). For instance, S-100-positive glial cells were described as cells surrounding peripheral nerves in the lung in man and three other mammalian species (Sheppard et al., 1983). Description of the morphology, tissue distribution, and connection of S-100-positive glial cells with pulmonary nerves was also confirmed in transgenic mice that express a green fluorescent protein (GFP) in the lung under the control of glial fibrillary acidic protein (GFAP) promoter, a marker of non-myelinating SCs (Suarez-Mier and Buckwalter, 2015). Glia-ensheathed nerves create a netting that surrounds large and small bronchi (Suarez-Mier and Buckwalter, 2015). Glia of non-myelinating SCs have been also found ensheathing blood vessel nerves (Table 1).
During lung development, in addition to the vagal neurons, neural crest cells also migrate to develop parasympathetic ganglia constituting both neurons and glial cells (Burns et al., 2008). Expression of glia markers was documented during respiratory neurogenesis (Aven and Ai, 2013). Neural crest-derived SOX10+PHOX2B+ SC precursors enter the lung, travel toward ganglionic positions, and differentiate into parasympathetic neurons and immature SCs in the bronchial interstitium (Dyachuk et al., 2014; Sountoulidis et al., 2023). In humans, an association of ganglia and nerve trunks with SCs in the developing airways was demonstrated by the middle of the first trimester (Sparrow et al., 1999).
The presence of SCs in the lungs is also demonstrated by the identification of pulmonary schwannomas - nerve sheath tumors comprised of neoplastic SCs. Schwannomas, also known as neurilemmomas or neurinomas of Verocay, are seen as encapsulated benign tumors expressing SC differentiation morphology and markers, including S100 and SOX10 protein, that develop from myelinated peripheral nerves (Belakhoua and Rodriguez, 2021). Because primary lung nerve cell tumors are very rare, accounting for ∼0.2% of all lung neoplasms, only a few reports describe cases of pulmonary schwannoma (Roviaro et al., 1983; Ohtsuka et al., 2005; Abbas et al., 2023).
Recent data suggest that non-myelinating SCs in the lung not only provide axon support and protection but are also actively involved in coordinating lung inflammation (Heredia et al., 2023). Remarkably, characterization of mouse lungs myelinating and non-myelinating SC transcriptome using single-cell RNA sequencing revealed the presence of two non-myelinating SC populations that participate in pathogen recognition. Comprehensive analysis of proinflammatory response of lung SCs both in vitro and in vivo uncovered that lung non-myelinating SCs, but not myelinating SCs, were actively involved in the innate immune response in the lung and lung inflammation (Heredia et al., 2023).
Furthermore, during lung cancer development and progression, SCs in the local lung microenvironment may play a quite different and specific role by interacting with both the malignant cells and infiltrating effector and regulatory immune cells.
SCs were detected in the lung tumors and in the tumor surrounding area both in experimental animals and human specimens (Silva et al., 2019; Cao et al., 2022). Furthermore, the retrospective analysis of SC identification in NSCLC tissues comprising 90 cases of adenocarcinoma and 51 cases of squamous cell carcinoma revealed higher levels of SCs in cancerous tissues compared to adjacent tissues (Zhou et al., 2019). Importantly, increased homing of detectable SCs positively correlated with a worse patient prognosis. Furthermore, it was also observed that advanced stages of lung cancer exhibited the highest levels of SC detection (Zhou et al., 2019). Although the identification of SCs in different solid tumors and their role in the development and metastasis formation in different types of cancer have been repeatedly reported and reviewed (Bunimovich et al., 2017; Deborde and Wong, 2022; Sun et al., 2022; Yurteri et al., 2022; Xue et al., 2023), data describing the functional significance of tumor-associated SCs (TA-SCs) in lung cancer are scarce (Table 1).
Earlier reports utilizing experimental animal models showed that SCs could regulate the motility and invasiveness of lung cancer cells in vitro and the development of metastases in vivo. SCs have been shown to endorse the epithelial-mesenchymal transition (EMT) and transmigration of lung cancer cells via CXCR2 by releasing chemokine CXCL5, augmenting expression of Snail and Twist, functional markers of EMT, and activating the PI3K/AKT/GSK-3β/Snail-Twist signaling pathway in lung cancer cells (Zhou et al., 2018). Furthermore, treatment of tumor cells with SC conditioning medium before the tumor cell inoculation resulted in a significant acceleration of metastasis formation in the regional lymph nodes in mice (Zhou et al., 2018). However, after knocking down CXCL5 signaling, a significant reduction in metastasis formation was observed. These data confirmed a new role of the PNS and specifically SCs in the functional organization of the lung tumor microenvironment and tumor progression (Figure 2).
Figure 2. Tumor-associated Schwann cells can promote development and progression of lung cancer. Schwann cells have been identified in the lung tumor specimens, and their number has been shown to correlated with the prognosis of disease. Tumor-associated remodeled Schwann cells can be reprogrammed by tumor-derived factors to the repair-like phenotype and activated to express and release cytokines, chemokines, growth and neurotrophic factors, matrix metalloproteinases, and exosomes. These and other factors modulate the tumor microenvironment by (i) attracting and polarizing immune cells to the regulatory (immunosuppressive) phenotype, (ii) remodeling the extracellular matrix, and (iii) directly altering the functional activity of malignant cells. Schwann cell-derived chemokines can upregulated EMT in lung cancer cells and stimulate their transmigratory potential. microRNA from Schwann cell exosomes promote proliferation, motility, and invasiveness of lung cancer cells by blocking specific mRNA in malignant cells. Altogether, tumor-associated Schwann cells can promote lung tumor growth in vivo and support formation of distant metastases. EMT, epithelial-mesenchymal transition; M2, alternatively activated tumor-associated macrophages; MDSC, myeloid-derived suppressor cells; MMP, matrix metalloproteinases; regDC, regulatory dendritic cells; Treg, regulatory T cells.
Cellular mechanisms of the pro-tumorigenic activity of SC include both the direct effect on malignant cells and the modulation of infiltrating immune cells in the tumor immunoenvironment (Figure 2). Attraction and polarization of conventional dendritic cells, immature myeloid cells, and T cells into immunosuppressive regulatory dendritic cells, myeloid-derived suppressor cells (MDSC), and T regulatory (Treg) cells, respectively, have been reported in different tumor models (Martyn et al., 2019; Zhang et al., 2020; Shurin et al., 2022). For lung cancer, bioinformatic analysis of the transcriptome of SCs demonstrated the secretion of high levels of CCL2, CXCL5, CXCL12, and CXCL8, and functional data suggested that CCL2 promoted the M2 polarization of macrophages (Zhou et al., 2020). Furthermore, SC-polarized M2 macrophages augmented the proliferation of lung cancer cells, demonstrating another functional path of the tumor-PNS/glia-immune axis.
According to the genome-scale biomolecular network analysis of SCs in lung adenocarcinoma and lung squamous cell carcinoma, pathways of SC dedifferentiation were upregulated while the expression of genes representing SC migration inhibition system was decreased. Interestingly, miRNAs targeting these pathways were also significantly altered (Silva et al., 2019). These results suggest that identified intratumoral SC-associated molecules may be used as systems biomarkers for screening in perineural invasion of lung cancer or for potential therapeutic purposes (Silva et al., 2019). Importantly, new data focusing on the ability of human TA-SCs to regulate the functional activity of human SCLC cell lines in vitro revealed that TA-SCs upregulated longevity, proliferation, migration, and invasiveness of tumor cells (Cao et al., 2022). In vivo studies confirmed these findings by demonstrating that SCs promote the growth of SCLC in mice. Further analysis of human TA-SCs revealed that they exhibit a repair-like phenotype, confirming results reported for SC analysis in melanoma specimens (Shurin et al., 2019). This allows distinguishing TA-SCs as so-called tumor-associated repair or repair-like SCs. Investigation of differentially expressed genes in lung cancer-activated repair-like SCs allowed the construction of unique networks of messenger-, micro-, and long non-coding RNA (mRNA-miRNA-lncRNA) that brought new insights into our understanding of potential molecular mechanisms of pro-tumorigenic activity of lung cancer-associated SCs. Furthermore, using the gene ontology (GO) enrichment annotations for the analysis of differentially expressed mRNAs in the SC-treated SCLC cells revealed a series of significant alterations that were categorized in all three common groups: biological process, cellular component, and molecular function (Cao et al., 2022). These new findings identified a new functional phenotype of SCs in cancer and brought new development into a better characterization of the nervous system-tumor crosstalk.
New data provide an interesting mechanistic explanation of how SCs can interact with malignant cells in the lungs. It is generally accepted that SCs utilize extracellular vesicles or exosomes to build and maintain the nerve microenvironment. Exosomes, as a means of intercellular communication, are extracellular vesicles that are consistently or inducibly released by many cell types to transfer protein, lipids, nucleic acids, glycoconjugates, membrane-bound molecules, and other biologically active factors to surrounding or distant cells and tissues (Edgar, 2016). This pathway of intercellular vesicle traffic plays important roles in many aspects of SC’s ability to transfer distinct cargoes that employ either neuroprotective and neuroregenerative or pathogenic influence on the recipient cells. For instance, the role of SC-derived exosomes in promoting axonal regeneration has been experimentally proven (Lopez-Verrilli et al., 2013; López-Leal et al., 2020; Wong et al., 2022). Mutations in the exosome biogenesis-related proteins causing a reduced number of exosomes and excretion of exosomal proteins in SCs were implicated in the molecular pathogenesis of Charcot-Marie-Tooth disease, a common hereditary neurological disorder of the PNS (Zhu et al., 2013). Interestingly, apart from different neuropathies, the potential contribution of SC-derived exosomes in carcinogenesis has also been suggested (Wong et al., 2022). A case study of schwannomatosis revealed a promoting effect on prostate-specific antigen secretion and a growth-accelerating outcome on a cognate schwannoma after resection of a prominent schwannoma (Chignon-Sicard et al., 2019). This was explained by exosomes secreted from schwannoma cells and tumor-adjacent resident SCs that targeted prostate cells and distant schwannoma cells. In addition, the possibility that SC’s exosomes can mediate the formation of a pre-metastatic niche has also been speculated (Wong et al., 2022). However, direct evidence of tumor cell stimulation by SC-derived exosomes has only been published recently.
The results of a new study, using the in vitro and xenogeneic in vivo models of NSCLC, revealed that the promotion of tumor cell proliferation, survival, motility, and transmigration by human SC-conditioned medium was due to SC-derived exosomes (Zhou et al., 2024). Evaluation of the expression and functional activity of SC exosomal microRNA demonstrated that hsa-miRNA-21-5P is responsible for upregulation of NSCLC cell longevity and motility. In fact, the necessity of exosome secretion from SCs was verified by knocking down Rab small GTPases Rab27A and Rab27B, which are known to control exosome secretion in human cells (Ostrowski et al., 2010) by shRNA-based targeting. These in vitro results were further confirmed by assessing the function of tumor cells transduced with miRNA-21-5p mimic and inhibitor. Additional analysis of tumor cells for intracellular signaling affected by miRNA-21 revealed that reversion-inducing cysteine-rich protein with Kazal motifs (RECK), a matrix metalloproteinase (MMP) inhibitor, not only blocked the interaction with exosomal miR-21-5p but completely prevented activation of tumor cells when transfected into RECK knockout tumor cells in a mutant form (Zhou et al., 2024).
The in vivo analysis of exosomal microRNA from SCs utilizing Cell Line-derived Tumor Xenograft models demonstrated that the presence of exosomal miRNA-21-5p was required for SC exosome-mediated stimulation of NSCLC growth in vivo. Importantly, by using EGFP-transfected tumor cells, it was also reported that SC exosomes significantly promoted the metastatic activity of tumor cells in vivo when miRNA-21-5p activity was not inhibited (Zhou et al., 2024). Finally, the confirmation of the co-expression of S100B positive SCs and miRNA-21 in human lung cancer tissues and the demonstration of an association between hsa-miRNA-21-5P expression levels and poor prognosis for NSCLC patients supported the clinical significance and high translational potential of these new findings.
Thus, these data demonstrate that exosomal microRNAs released from TA-SCs may potentiate proliferation, migration, and invasiveness of lung cancer cells, and upregulate tumor growth and metastasis formation of lung cancer. Together with other findings showing that SC-derived factors support lung cancer progression, these data provide a prospect to assess novel cellular and molecular targets for lung cancer therapy.
Our recognition of the relevance of SC activity and lung cancer is still in its infancy although the important role of SCs in other cancer types has already been demonstrated (Deborde and Wong, 2017; Martyn et al., 2019; Shurin et al., 2021; Deborde and Wong, 2022; Yurteri et al., 2022). Analysis of SC biology in prostate, pancreatic, cervical, oral, colon tumors, melanoma, and other cancer types revealed their involvement in perineural invasion, cancer pain, and tumor cell proliferation, dispersion, invasion, and metastasizing (Demir et al., 2014; Deborde et al., 2016; Shan et al., 2016; Shurin et al., 2019; Huang et al., 2020; Salvo et al., 2021; Santi et al., 2022). It was also shown that the proregenerative state of tumor-activated SCs is important for the stimulation of tumor development, progression, and metastasis (Shurin et al., 2020; Pascual et al., 2021; Deborde and Wong, 2022). Nevertheless, there are convincing findings concerning the significance of cytokines, chemokines, and exosomes in SC’s ability to control lung cancer development, progression, and metastasis formation directly or via modulating the tumor immunoenvironment. These data include reports showing that lung cancer-associated or activated/polarized SCs: i) are strongly associated with a poor prognosis in patients with lung cancer, ii) promote longevity, motility, and spreading of different lung cancer cell lines in vitro, iii) endorse EMT of lung cancer cells, iv) accelerate lung cancer cell growth and metastasis formation in vivo, v) express cytokines and chemokines that upregulate polarization of regulatory immune cells, vi) release exosomes containing microRNA that modulate various functions of lung cancer cells, and vii) regulate lncRNA-miRNA-mRNA competitive endogenous RNA (ceRNA) networks that play critical roles in development of lung neoplasms.
Collectively, available data suggest that SCs regulate lung cancer progression, indicating that manipulation of resident and, potentially, adoptively transferred SCs may provide a useful tool to improve lung cancer patients’ outcomes. Future studies in this field will permit a better understanding of SC-controlling pathways in the lung cancer milieu in search of new multimodal diagnostic and therapeutic approaches in patients with SCLC and NSCLC. New strategies targeting repair-like TA-SCs or SC-derived exosomes as therapeutics and the development of diagnostic panels evaluating TA-SC-associated ceRNA networks as new biomarkers will contribute to improving outcomes of patients with lung cancer.
MS: Conceptualization, Project administration, Visualization, Writing–original draft. SW: Formal Analysis, Project administration, Writing–review and editing. GS: Investigation, Methodology, Validation, Writing–original draft. HZ: Data curation, Funding acquisition, Project administration, Writing–review and editing. YZ: Conceptualization, Supervision, Writing–original draft.
The author(s) declare that financial support was received for the research, authorship, and/or publication of this article. This work was supported by the Department of Pathology, University of Pittsburgh Medical Center, Shanghai Chest Hospital, Shanghai Municipal Health Commission (No. 2022YQ039, to YZ), and Shanghai “Rising Stars of Medical Talents” Youth Development Program (to YZ).
The authors declare that the research was conducted in the absence of any commercial or financial relationships that could be construed as a potential conflict of interest.
The author(s) declared that they were an editorial board member of Frontiers, at the time of submission. This had no impact on the peer review process and the final decision.
All claims expressed in this article are solely those of the authors and do not necessarily represent those of their affiliated organizations, or those of the publisher, the editors and the reviewers. Any product that may be evaluated in this article, or claim that may be made by its manufacturer, is not guaranteed or endorsed by the publisher.
Abbas, M., Fatimi, A. S., Hassan, S. I., Aamir, F. B., Arshad, S., and Fatimi, S. H. (2023). Intrapulmonary schwannoma presenting as an asymptomatic lung mass: a case report. J. Med. Case Rep. 17 (1), 538. doi:10.1186/s13256-023-04234-z
Agostoni, E., Chinnock, J. E., De Daly, M. B., and Murray, J. G. (1957). Functional and histological studies of the vagus nerve and its branches to the heart, lungs and abdominal viscera in the cat. J. Physiol. 135 (1), 182–205. doi:10.1113/jphysiol.1957.sp005703
Al-Wadei, H. A. N., Ullah, M. F., and Al-Wadei, M. H. (2012). Intercepting neoplastic progression in lung malignancies via the beta adrenergic (β-AR) pathway: implications for anti-cancer drug targets. Pharmacol. Res. 66 (1), 33–40. doi:10.1016/j.phrs.2012.03.014
Arreola-Ramírez, J. L., Morales-Hernández, P. E., Falcón-Rodríguez, C. I., and Segura-Medina, P. (2013). General aspects of pulmonary innervation. Gac. Med. Mex. 149 (5), 502–508.
Audrit, K. J., Delventhal, L., Aydin, Ö., and Nassenstein, C. (2017). The nervous system of airways and its remodeling in inflammatory lung diseases. Cell Tissue Res. 367 (3), 571–590. doi:10.1007/s00441-016-2559-7
Aven, L., and Ai, X. (2013). Mechanisms of respiratory innervation during embryonic development. Organogenesis 9 (3), 194–198. doi:10.4161/org.24842
Balakrishnan, A., Belfiore, L., Chu, T. H., Fleming, T., Midha, R., Biernaskie, J., et al. (2020). Insights into the role and potential of Schwann cells for peripheral nerve repair from studies of development and injury. Front. Mol. Neurosci. 13, 608442. doi:10.3389/fnmol.2020.608442
Belakhoua, S. M., and Rodriguez, F. J. (2021). Diagnostic Pathology of tumors of peripheral nerve. Neurosurgery 88 (3), 443–456. doi:10.1093/neuros/nyab021
Belvisi, M. G. (2002). Overview of the innervation of the lung. Curr. Opin. Pharmacol. 2 (3), 211–215. doi:10.1016/s1471-4892(02)00145-5
Berner, J., Weiss, T., Sorger, H., Rifatbegovic, F., Kauer, M., Windhager, R., et al. (2022). Human repair-related Schwann cells adopt functions of antigen-presenting cells in vitro. Glia 70 (12), 2361–2377. doi:10.1002/glia.24257
Blake, K. J., Jiang, X. R., and Chiu, I. M. (2019). Neuronal regulation of immunity in the skin and lungs. Trends Neurosci. 42 (8), 537–551. doi:10.1016/j.tins.2019.05.005
Bunimovich, Y. L., Keskinov, A. A., Shurin, G. V., and Shurin, M. R. (2017). Schwann cells: a new player in the tumor microenvironment. Cancer Immunol. Immunother. 66 (8), 959–968. doi:10.1007/s00262-016-1929-z
Burns, A. J., Thapar, N., and Barlow, A. J. (2008). Development of the neural crest-derived intrinsic innervation of the human lung. Am. J. Respir. Cell Mol. Biol. 38 (3), 269–275. doi:10.1165/rcmb.2007-0246OC
Canning, B. J., and Spina, D. (2009). Sensory nerves and airway irritability. Handb. Exp. Pharmacol. 194 (194), 139–183. doi:10.1007/978-3-540-79090-7_5
Cao, S., Wang, Y., Zhou, Y., Zhang, Y., Ling, X., Zhang, L., et al. (2022). A novel therapeutic target for small-cell lung cancer: tumor-associated repair-like Schwann cells. Cancers (Basel) 14 (24), 6132. doi:10.3390/cancers14246132
Chignon-Sicard, B., Hofman, V., Chevallier, D., Cucchi, J.-M., Ilié, M., Dadone-Montaudié, B., et al. (2019). Age-related schwannomatosis with potential exosome-mediated contribution to prostate hyperplasia: a case report and mini-review. Ther. Adv. Urology 11, 1756287219875578. doi:10.1177/1756287219875578
Coelho, M., Squizzato, A., Cassina, N., Marino, F., Ribeiro, L. V., and Cosentino, M. (2020). Effect of beta-blockers on survival of lung cancer patients: a systematic review and meta-analysis. Eur. J. Cancer Prev. 29 (4), 306–314. doi:10.1097/CEJ.0000000000000544
Deborde, S., Omelchenko, T., Lyubchik, A., Zhou, Y., He, S., McNamara, W. F., et al. (2016). Schwann cells induce cancer cell dispersion and invasion. J. Clin. Invest. 126 (4), 1538–1554. doi:10.1172/jci82658
Deborde, S., and Wong, R. J. (2017). How Schwann cells facilitate cancer progression in nerves. Cell Mol. Life Sci. 74 (24), 4405–4420. doi:10.1007/s00018-017-2578-x
Deborde, S., and Wong, R. J. (2022). The role of Schwann cells in cancer. Adv. Biol. (Weinh) 6 (9), e2200089. doi:10.1002/adbi.202200089
De Logu, F., Marini, M., Landini, L., Souza Monteiro de Araujo, D., Bartalucci, N., Trevisan, G., et al. (2021). Peripheral nerve resident macrophages and Schwann cells mediate cancer-induced pain. Cancer Res. 81 (12), 3387–3401. doi:10.1158/0008-5472.Can-20-3326
Demir, I. E., Boldis, A., Pfitzinger, P. L., Teller, S., Brunner, E., Klose, N., et al. (2014). Investigation of Schwann cells at neoplastic cell sites before the onset of cancer invasion. JNCI J. Natl. Cancer Inst. 106 (8), dju184. doi:10.1093/jnci/dju184
De Virgiliis, F., and Di Giovanni, S. (2020). Lung innervation in the eye of a cytokine storm: neuroimmune interactions and COVID-19. Nat. Rev. Neurol. 16 (11), 645–652. doi:10.1038/s41582-020-0402-y
Dharmage, S. C., Perret, J. L., and Custovic, A. (2019). Epidemiology of asthma in children and adults. Front. Pediatr. 7, 246. doi:10.3389/fped.2019.00246
Downing, S. E., and Lee, J. C. (1980). Nervous control of the pulmonary circulation. Annu. Rev. Physiol. 42, 199–210. doi:10.1146/annurev.ph.42.030180.001215
Dyachuk, V., Furlan, A., Shahidi, M. K., Giovenco, M., Kaukua, N., Konstantinidou, C., et al. (2014). Neurodevelopment. Parasympathetic neurons originate from nerve-associated peripheral glial progenitors. Science 345 (6192), 82–87. doi:10.1126/science.1253281
Edgar, J. R. (2016). Q&A: what are exosomes, exactly? BMC Biol. 14 (1), 46. doi:10.1186/s12915-016-0268-z
Eid, S. A., Noureldein, M., Kim, B., Hinder, L. M., Mendelson, F. E., Hayes, J. M., et al. (2023). Single-cell RNA-seq uncovers novel metabolic functions of Schwann cells beyond myelination. J. Neurochem. 166 (2), 367–388. doi:10.1111/jnc.15877
Hartlehnert, M., Derksen, A., Hagenacker, T., Kindermann, D., Schäfers, M., Pawlak, M., et al. (2017). Schwann cells promote post-traumatic nerve inflammation and neuropathic pain through MHC class II. Sci. Rep. 7 (1), 12518. doi:10.1038/s41598-017-12744-2
Heredia, J. E., Jung, M., Balestrini, A., Doerr, J., Paler-Martinez, A., Mozzarelli, A., et al. (2023). Single-cell transcriptomic analysis links nonmyelinating Schwann cells to proinflammatory response in the lung. J. Immunol. 211 (5), 844–852. doi:10.4049/jimmunol.2200946
Huang, T., Fan, Q., Wang, Y., Cui, Y., Wang, Z., Yang, L., et al. (2020). Schwann cell-derived CCL2 promotes the perineural invasion of cervical cancer. Front. Oncol. 10, 19. doi:10.3389/fonc.2020.00019
Huang, Y., Zhao, C., and Su, X. (2019). Neuroimmune regulation of lung infection and inflammation. QJM Int. J. Med. 112 (7), 483–487. doi:10.1093/qjmed/hcy154
Hung, K. S., Hertweck, M. S., Hardy, J. D., and Loosli, C. G. (1972). Innervation of pulmonary alveoli of the mouse lung: an electron microscopic study. Am. J. Anat. 135 (4), 477–495. doi:10.1002/aja.1001350404
Hutchings, C., Phillips, J. A., and Djamgoz, M. B. A. (2020). Nerve input to tumours: pathophysiological consequences of a dynamic relationship. Biochimica Biophysica Acta (BBA) - Rev. Cancer 1874 (2), 188411. doi:10.1016/j.bbcan.2020.188411
Jammes, Y., Fornaris, E., Mei, N., and Barrat, E. (1982). Afferent and efferent components of the bronchial vagal branches in cats. J. Auton. Nerv. Syst. 5 (2), 165–176. doi:10.1016/0165-1838(82)90037-6
Jessen, K. R., and Mirsky, R. (2016). The repair Schwann cell and its function in regenerating nerves. J. Physiol. 594 (13), 3521–3531. doi:10.1113/jp270874
Kistemaker, L. E. M., and Prakash, Y. S. (2019). Airway innervation and plasticity in asthma. Physiol. (Bethesda) 34 (4), 283–298. doi:10.1152/physiol.00050.2018
Lee, L.-Y., and Yu, J. (2014). Sensory nerves in lung and airways. Compr. Physiol. 4, 287–324. doi:10.1002/cphy.c130020
Li, C., Chen, W., Lin, F., Li, W., Wang, P., Liao, G., et al. (2023). Functional two-way crosstalk between brain and lung: the brain–lung Axis. Cell. Mol. Neurobiol. 43 (3), 991–1003. doi:10.1007/s10571-022-01238-z
Liu, T., Yang, L., Han, X., Ding, X., Li, J., and Yang, J. (2020). Local sympathetic innervations modulate the lung innate immune responses. Sci. Adv. 6 (20), eaay1497. doi:10.1126/sciadv.aay1497
López-Leal, R., Díaz-Viraqué, F., Catalán, R. J., Saquel, C., Enright, A., Iraola, G., et al. (2020). Schwann cell reprogramming into repair cells increases miRNA-21 expression in exosomes promoting axonal growth. J. Cell Sci. 133 (12), jcs239004. doi:10.1242/jcs.239004
Lopez-Verrilli, M. A., Picou, F., and Court, F. A. (2013). Schwann cell-derived exosomes enhance axonal regeneration in the peripheral nervous system. Glia 61 (11), 1795–1806. doi:10.1002/glia.22558
Martyn, G. V., Shurin, G. V., Keskinov, A. A., Bunimovich, Y. L., and Shurin, M. R. (2019). Schwann cells shape the neuro-immune environs and control cancer progression. Cancer Immunol. Immunother. 68 (11), 1819–1829. doi:10.1007/s00262-018-02296-3
Mazzone, S. B., and Undem, B. J. (2016). Vagal afferent innervation of the airways in health and disease. Physiol. Rev. 96 (3), 975–1024. doi:10.1152/physrev.00039.2015
McCarty, M. F., O’Keefe, J. H., and DiNicolantonio, J. J. (2015). Carvedilol and spirulina may provide important health protection to smokers and other nicotine addicts: a call for pertinent research. Mo Med. 112 (1), 72–75.
McGarvey, L. (2015). Seeing is believing. Sensing real progress in the study of human airway nerves. Am. J. Respir. Crit. Care Med. 192 (1), 1–2. doi:10.1164/rccm.201505-0873ED
Meyer zu Hörste, G., Hu, W., Hartung, H. P., Lehmann, H. C., and Kieseier, B. C. (2008). The immunocompetence of Schwann cells. Muscle Nerve 37 (1), 3–13. doi:10.1002/mus.20893
Michael, I. P., Rebecca, A. L., John, M., and Leigh, P. N. (1999). Respiratory aspects of neurological disease. J. Neurology, Neurosurg. & Psychiatry 66, 5–15. doi:10.1136/jnnp.66.1.5
Natarajan, A., Beena, P. M., Devnikar, A. V., and Mali, S. (2020). A systemic review on tuberculosis. Indian J. Tuberc. 67 (3), 295–311. doi:10.1016/j.ijtb.2020.02.005
Ohtsuka, T., Nomori, H., Naruke, T., Orikasa, H., Yamazaki, K., and Suemasu, K. (2005). Intrapulmonary schwannoma. Jpn. J. Thorac. Cardiovasc. Surg. 53 (3), 154–156. doi:10.1007/s11748-005-0023-9
Ostrowski, M., Carmo, N. B., Krumeich, S., Fanget, I., Raposo, G., Savina, A., et al. (2010). Rab27a and Rab27b control different steps of the exosome secretion pathway. Nat. Cell Biol. 12 (1), 19–30. doi:10.1038/ncb2000
Pascual, G., Domínguez, D., Elosúa-Bayes, M., Beckedorff, F., Laudanna, C., Bigas, C., et al. (2021). Dietary palmitic acid promotes a prometastatic memory via Schwann cells. Nature 599 (7885), 485–490. doi:10.1038/s41586-021-04075-0
Roviaro, G., Montorsi, M., Varoli, F., Binda, R., and Cecchetto, A. (1983). Primary pulmonary tumours of neurogenic origin. Thorax 38 (12), 942–945. doi:10.1136/thx.38.12.942
Ruvuna, L., and Sood, A. (2020). Epidemiology of chronic obstructive pulmonary disease. Clin. Chest Med. 41 (3), 315–327. doi:10.1016/j.ccm.2020.05.002
Salvo, E., Tu, N. H., Scheff, N. N., Dubeykovskaya, Z. A., Chavan, S. A., Aouizerat, B. E., et al. (2021). TNFα promotes oral cancer growth, pain, and Schwann cell activation. Sci. Rep. 11 (1), 1840. doi:10.1038/s41598-021-81500-4
Santi, M. D., Zhang, M., Salvo, E., Asam, K., Viet, C. T., Xie, T., et al. (2022). Schwann cells induce phenotypic changes in oral cancer cells. Adv. Biol. (Weinh) 6 (9), e2200187. doi:10.1002/adbi.202200187
Shan, C., Wei, J., Hou, R., Wu, B., Yang, Z., Wang, L., et al. (2016). Schwann cells promote EMT and the Schwann-like differentiation of salivary adenoid cystic carcinoma cells via the BDNF/TrkB axis. Oncol. Rep. 35 (1), 427–435. doi:10.3892/or.2015.4366
Shao, J.-X., Wang, B., Yao, Y.-N., Pan, Z.-J., Shen, Q., and Zhou, J.-Y. (2016). Autonomic nervous infiltration positively correlates with pathological risk grading and poor prognosis in patients with lung adenocarcinoma. Thorac. Cancer 7 (5), 588–598. doi:10.1111/1759-7714.12374
Sheppard, M. N., Kurian, S. S., Henzen-Logmans, S. C., Michetti, F., Cocchia, D., Cole, P., et al. (1983). Neurone-specific enolase and S-100: new markers for delineating the innervation of the respiratory tract in man and other mammals. Thorax 38 (5), 333–340. doi:10.1136/thx.38.5.333
Shurin, G. V., Kruglov, O., Ding, F., Lin, Y., Hao, X., Keskinov, A. A., et al. (2019). Melanoma-induced reprogramming of Schwann cell signaling aids tumor growth. Cancer Res. 79 (10), 2736–2747. doi:10.1158/0008-5472.CAN-18-3872
Shurin, G. V., Vats, K., Kruglov, O., Bunimovich, Y. L., and Shurin, M. R. (2022). Tumor-induced T cell polarization by Schwann cells. Cells 11 (22), 3541. doi:10.3390/cells11223541
Shurin, M. R., Baraldi, J. H., and Shurin, G. V. (2021). Neuroimmune regulation of surgery-associated metastases. Cells 10 (2), 454. doi:10.3390/cells10020454
Shurin, M. R., Shurin, G. V., Zlotnikov, S. B., and Bunimovich, Y. L. (2020). The neuroimmune Axis in the tumor microenvironment. J. Immunol. 204 (2), 280–285. doi:10.4049/jimmunol.1900828
Silva, V. M., Gomes, J. A., Tenório, L. P. G., de Omena Neta, G. C., da Costa Paixão, K., Duarte, A. K. F., et al. (2019). Schwann cell reprogramming and lung cancer progression: a meta-analysis of transcriptome data. Oncotarget 10 (68), 7288–7307. doi:10.18632/oncotarget.27204
Sountoulidis, A., Marco Salas, S., Braun, E., Avenel, C., Bergenstrahle, J., Theelke, J., et al. (2023). A topographic atlas defines developmental origins of cell heterogeneity in the human embryonic lung. Nat. Cell Biol. 25 (2), 351–365. doi:10.1038/s41556-022-01064-x
Sparrow, M. P., Weichselbaum, M., and McCray, P. B. (1999). Development of the innervation and airway smooth muscle in human fetal lung. Am. J. Respir. Cell Mol. Biol. 20 (4), 550–560. doi:10.1165/ajrcmb.20.4.3385
Spindel, E. R. (2016). Cholinergic targets in lung cancer. Curr. Pharm. Des. 22 (14), 2152–2159. doi:10.2174/1381612822666160127114237
Su, Y., Barr, J., Jaquish, A., Xu, J., Verheyden, J. M., and Sun, X. (2021). Identification of lung innervating sensory neurons and their target specificity. Am. J. Physiology-Lung Cell. Mol. Physiology 322 (1), L50–L63. doi:10.1152/ajplung.00376.2021
Suarez-Mier, G. B., and Buckwalter, M. S. (2015). Glial fibrillary acidic protein-expressing glia in the mouse lung. ASN neuro 7 (5), 1759091415601636. doi:10.1177/1759091415601636
Sun, L., Chen, S., and Chen, M. (2022). Schwann cells in the tumor microenvironment: need more attention. J. Oncol. 2022, 1058667. doi:10.1155/2022/1058667
Thai, A. A., Solomon, B. J., Sequist, L. V., Gainor, J. F., and Heist, R. S. (2021). Lung cancer. Lancet 398 (10299), 535–554. doi:10.1016/S0140-6736(21)00312-3
Undem, B. J., and Kollarik, M. (2005). The role of vagal afferent nerves in chronic obstructive pulmonary disease. Proc. Am. Thorac. Soc. 2 (4), 355–360. doi:10.1513/pats.200504-033SR
Undem, B. J., and Nassenstein, C. (2009). Airway nerves and dyspnea associated with inflammatory airway disease. Respir. Physiol. Neurobiol. 167 (1), 36–44. doi:10.1016/j.resp.2008.11.012
Vaillancourt, M., Chia, P., Sarji, S., Nguyen, J., Hoftman, N., Ruffenach, G., et al. (2017). Autonomic nervous system involvement in pulmonary arterial hypertension. Respir. Res. 18 (1), 201. doi:10.1186/s12931-017-0679-6
van der Velden, V. H., and Hulsmann, A. R. (1999). Autonomic innervation of human airways: structure, function, and pathophysiology in asthma. Neuroimmunomodulation 6 (3), 145–159. doi:10.1159/000026376
Velez-Roa, S., Ciarka, A., Najem, B., Vachiery, J.-L., Naeije, R., and van de Borne, P. (2004). Increased sympathetic nerve activity in pulmonary artery hypertension. Circulation 110 (10), 1308–1312. doi:10.1161/01.CIR.0000140724.90898.D3
Verhein, K. C., Fryer, A. D., and Jacoby, D. B. (2009). Neural control of airway inflammation. Curr. Allergy Asthma Rep. 9 (6), 484–490. doi:10.1007/s11882-009-0071-9
Wong, F. C., Ye, L., Demir, I. E., and Kahlert, C. (2022). Schwann cell-derived exosomes: janus-faced mediators of regeneration and disease. Glia 70 (1), 20–34. doi:10.1002/glia.24087
Xue, M., Zhu, Y., Jiang, Y., Han, L., Shi, M., Su, R., et al. (2023). Schwann cells regulate tumor cells and cancer-associated fibroblasts in the pancreatic ductal adenocarcinoma microenvironment. Nat. Commun. 14 (1), 4600. doi:10.1038/s41467-023-40314-w
Ydens, E., Lornet, G., Smits, V., Goethals, S., Timmerman, V., and Janssens, S. (2013). The neuroinflammatory role of Schwann cells in disease. Neurobiol. Dis. 55, 95–103. doi:10.1016/j.nbd.2013.03.005
Yegen, C. H., Marchant, D., Bernaudin, J. F., Planes, C., Boncoeur, E., and Voituron, N. (2023). Chronic pulmonary fibrosis alters the functioning of the respiratory neural network. Front. Physiol. 14, 1205924. doi:10.3389/fphys.2023.1205924
Yurteri, Ü., Çifcibaşı, K., Friess, H., Ceyhan, G. O., Istvanffy, R., and Demir, I. E. (2022). Schwann cells in peripheral cancers: bystanders or promoters? Adv. Biol. (Weinh) 6, e2200033. doi:10.1002/adbi.202200033
Zhang, S. H., Shurin, G. V., Khosravi, H., Kazi, R., Kruglov, O., Shurin, M. R., et al. (2020). Immunomodulation by Schwann cells in disease. Cancer Immunol. Immunother. 69 (2), 245–253. doi:10.1007/s00262-019-02424-7
Zhao, Q., Gu, X., Zhang, C., Lu, Q., Chen, H., and Xu, L. (2015). Blocking M2 muscarinic receptor signaling inhibits tumor growth and reverses epithelial-mesenchymal transition (EMT) in non-small cell lung cancer (NSCLC). Cancer Biol. Ther. 16 (4), 634–643. doi:10.1080/15384047.2015.1029835
Zhou, Y., Li, J., Han, B., Zhong, R., and Zhong, H. (2020). Schwann cells promote lung cancer proliferation by promoting the M2 polarization of macrophages. Cell. Immunol. 357, 104211. doi:10.1016/j.cellimm.2020.104211
Zhou, Y., Shurin, G. V., Zhong, H., Bunimovich, Y. L., Han, B., and Shurin, M. R. (2018). Schwann cells augment cell spreading and metastasis of lung cancer. Cancer Res. 78 (20), 5927–5939. doi:10.1158/0008-5472.CAN-18-1702
Zhou, Y., Zhang, Y., Xu, J., Wang, Y., Yang, Y., Wang, W., et al. (2024). Schwann cell-derived exosomes promote lung cancer progression via miRNA-21-5p. Glia 72, 692–707. doi:10.1002/glia.24497
Zhou, Y., Zhong, H., and Han, B. (2019). P2.01-85 Schwann cells are overexpressed and inversely correlated with survival of non-small cell lung cancer patients. J. Thorac. Oncol. 14 (10), S673–S674. doi:10.1016/j.jtho.2019.08.1428
Keywords: Schwann cells, tumor innervation, metastasis, lung cancer, pulmonary diseases
Citation: Shurin MR, Wheeler SE, Shurin GV, Zhong H and Zhou Y (2024) Schwann cells in the normal and pathological lung microenvironment. Front. Mol. Biosci. 11:1365760. doi: 10.3389/fmolb.2024.1365760
Received: 04 January 2024; Accepted: 21 March 2024;
Published: 04 April 2024.
Edited by:
Bojana Milutinovic, University of Texas MD Anderson Cancer Center, United StatesReviewed by:
Michael Rehman, Yale University, United StatesCopyright © 2024 Shurin, Wheeler, Shurin, Zhong and Zhou. This is an open-access article distributed under the terms of the Creative Commons Attribution License (CC BY). The use, distribution or reproduction in other forums is permitted, provided the original author(s) and the copyright owner(s) are credited and that the original publication in this journal is cited, in accordance with accepted academic practice. No use, distribution or reproduction is permitted which does not comply with these terms.
*Correspondence: Michael R. Shurin, c2h1cmlubXJAdXBtYy5lZHU=; Yan Zhou, eXpob3U3MTZAMTYzLmNvbQ==
Disclaimer: All claims expressed in this article are solely those of the authors and do not necessarily represent those of their affiliated organizations, or those of the publisher, the editors and the reviewers. Any product that may be evaluated in this article or claim that may be made by its manufacturer is not guaranteed or endorsed by the publisher.
Research integrity at Frontiers
Learn more about the work of our research integrity team to safeguard the quality of each article we publish.