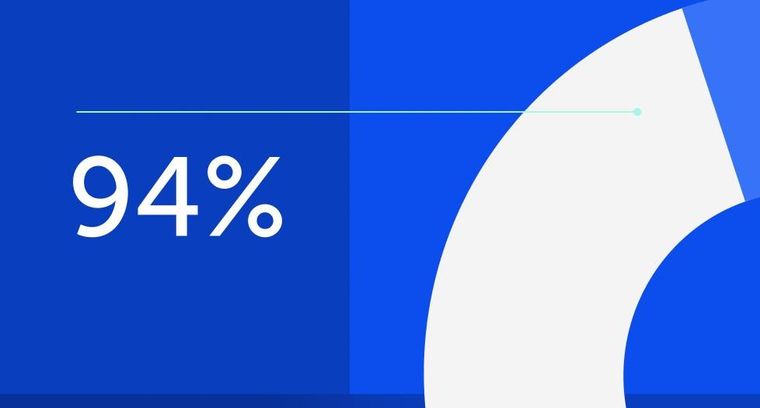
94% of researchers rate our articles as excellent or good
Learn more about the work of our research integrity team to safeguard the quality of each article we publish.
Find out more
ORIGINAL RESEARCH article
Front. Mol. Biosci., 23 February 2024
Sec. Cellular Biochemistry
Volume 11 - 2024 | https://doi.org/10.3389/fmolb.2024.1303379
The theory of aging is primarily concerned with oxidative stress caused by an imbalance in reactive oxygen species generation and cellular antioxidants. To alleviate the oxidative stress, we investigated the protective effect of diosgenin (DSG) for D-galactose (D-gal) using 20 and 40 mg of DSG/kg/day/orally for 42 days. The findings showed that D-gal caused brain and liver oxidative injuries by upregulating aging and oxidative markers. To counteract the oxidative stress caused by D-gal, DSG upregulated glutathione peroxidase-1, superoxide dismutase-1, and glutathione S-transferase-α. DSG also diminished the expression of p53, p21, Bcl-2-associated X protein, caspase-3, and mammalian target of rapamycin in brain and liver, as well as the build-up of β-galactosidase. DSG, in a dose-dependent manner, decreased the oxidative aging effects of D-gal in brain and liver tissues through targeting of aging and apoptotic marker genes. Finally, it should be noted that consuming DSG supplements is a suggesting natural preventative agent that may counteract aging and preserve health through improvement of body antioxidant status and control aging associated inflammation and cellular apoptosis.
Aging is a complex biological process of gradually deteriorating an organism’s physiological functions over time (Rodríguez-Rodero et al., 2011). Aging is a progressive decline in cellular repair, leading to increased vulnerability to disease and, eventually, death (López-Otín et al., 2013). As we age at risk of severe cellular damage, two primary pathways contributing to oxidative stress and inflammation occur (Guzik and Touyz, 2017). Inflammaging is a hallmark of aging where the inflammatory molecules accumulate in cells, causing tissue damage, impairing organ function, and contributing to age-related illnesses (Ferrucci and Fabbri, 2018). Generation of reactive oxygen species (ROS) due to regular cellular metabolism can harm cellular DNA, proteins, and lipids. Over time, oxidative damage adds to cellular malfunction and aging (Juan et al., 2021). ROS are very reactive chemicals that can harm the activities of various cellular components (Birben et al., 2012).
D-galactose (D-gal)-induced aging is a model of accelerated aging in animals that involves administering D-gal to cause aging-like symptoms. This model is used in research to study the effects of aging and potential treatments for age-related conditions (Hou et al., 2019; de Almeida Rezende et al., 2021; Remigante et al., 2022; Wang et al., 2022). D-gal reacts with free amines of amino acids in proteins through nonenzymatic glycation to form advanced glycation end products. Also, chronic administration of D-gal could contribute to ROS generation through D-gal oxidative metabolism and glycation end products (Song et al., 1999; Parameshwaran et al., 2010).
D-gal-induced aging has been used to explore possible interventions like antioxidants, anti-inflammatory agents, and anti-aging compounds to mitigate the effects of aging (El-Far et al., 2020; El-Far et al., 2021; El-Far et al., 2022; de Almeida Rezende et al., 2021; Saafan et al., 2023). Due to their lack of addictive and poisonous properties, phytopharmaceuticals are becoming increasingly critical in allopathic and conventional medicine (Subhashini et al., 2011). Diosgenin (DSG) is a naturally occurring aglycone of the steroid saponin that is present in Costus speciosus, Dioscorea species Smilax menispermoidea, Helicteres isora, Paris species, Aletris, Trigonella, and Trillium (Zitka et al., 2012; Chiang et al., 2020). It has been tested pharmacologically for its ability to reduce blood sugar (McAnuff et al., 2005) and its anti-inflammatory and antioxidant potentials (Son et al., 2007; Al-Matubsi et al., 2011). Son et al. (2007) reported that, DSG increased total superoxide dismutase (T-SOD) glutathione peroxidase (GPx), and catalase in high-cholesterol fed rats. In addition, DSG significantly increased the antioxidative effect of dietary chromium chloride supplementation on high-cholesterol fed Japanese quails (Al-Matubsi et al., 2011). Besides, DSG inhibited the ER stress-induced inflammation in aorta in experimental diabetic rats (Prasad et al., 2022). The biological activities of DSG were stated in Supplementary Material S1 retrieved from the Comparative Toxicogenomics Database (CTD; http://ctdbase.org/), showing the preventive and curative roles of DSG in different diseases and the target genes. While DSG has been studied for its health benefits, its direct anti-aging effects are not well-established. Therefore, the current study was assigned to investigate the protective effects of DSG on D-gal induced aging in rats’ brains and liver through targeting of aging and apoptotic markers mRNA expressions.
The Faculty of Veterinary Medicine Ethics Committee at Damanhour University in Egypt has been accepted all techniques (DMU/VetMed-2023/028).
Fifty male Wistar rats were bought from the Medical Research Institute of Alexandria University in Egypt, weighing between 90 and 110 g B.W. Rats were received water and basal meal (Table 1) under controlled environmental conditions. Rats were housed 10 days before the trial for acclimatization.
Five groups of ten rats each were at random allotted into five equal groups (Scheme 1). Rats in the control group received daily subcutaneous injections of physiological saline solution (0.9%) for 42 days. Rats in the vehicle group received daily oral supplements of corn oil and saline injection subcutaneously for 42 days. Rats in the D-gal group received corn oil orally and daily subcutaneous injected with saline contains 200 mg of D-gal/kg body weight (B.W) (Fan et al., 2017; El-Far et al., 2021; 2022; Saafan et al., 2023) for 42 days. Rats in the D-gal + DSG20 group received 20 mg of DSG dissolved in corn per kg B.W. orally (Jagadeesan et al., 2012) and daily subcutaneous injected with saline contains 200 mg of D-gal/kg B.W. Rats in the D-gal + DSG40 group received 40 mg of DSG dissolved in corn per kg B.W. orally in addition to daily subcutaneous injected with saline contains 200 mg of D-gal/kg B.W. for 42 days.
At the end of experiment, rats were inhaled isoflurane and cervical dislocation. The brains and livers were quickly rinsed with cold saline and cut into three pieces. One of the parts was used for histological study and was fixed in neutral buffered formaldehyde for 48 h, 10%, while the other two pieces were kept at −80°C for biochemical and mRNA expression analyses.
Caspase-3 (CASP3), B-cell lymphoma-2 (Bcl2), p53, p21, Bcl-2-associated X protein (Bax), mammalian target of rapamycin (mTOR), and β-actin were all expressed as mRNA in the first portion using RT-PCR. Malondialdehyde (MDA), superoxide dismutase 1 (SOD1), GPx-1, and glutathione S-transferase-α (GST-α) were all determined using ELISA in the second portion.
The fixed brain and liver tissues were processed using the conventional paraffin embedding procedure. Then, 4 µm thick sections were stained by Hematoxylin and Eosin (H and E) (Bancroft and Layton, 2013). The brain and hepatic lesions were semi-quantitatively scored by examining 10 fields selected from each rat’s slide. The lesions were scored blindly as follows [Score scale: 0 = normal; 1 ≤ 25%; 2 = 26–50%; 3 = 51–75%; 4 = 76–100%] (Gibson-Corley et al., 2013).
Immunohistochemical assessment of β-galactosidase and 8-hydroxy-2′-deoxyguanosine (8-OHdG) was done following Noreldin et al. (2018) using antibodies listed in Table 2. The slices were photographed under a microscope (Leica DM500) using a digital camera (Leica EC3, Leica, Germany). We used the ImageJ software (National Institutes of Health, Bethesda, MD, United States) to quantify the immunostaining intensities according to Sysel et al. (2013). Furthermore, the inverse mean densities of 10 different fields from different sections were assessed according to Vis et al. (2000).
Using chilled 0.1 M phosphate buffer saline and 20% (w/v), homogenates of the brain and liver were subjected to determination of MDA (Buege and Aust, 1978) and the protein levels of SOD1, GPx-1, and GST-α using ELISA kits (Fine Test, Wuhan, Hubei, China). All samples’ protein contents were assessed using the Bradford technique.
Total RNA was isolated from the tissue samples using the Easy spin kit for total RNA extraction following the manufacturer’s instructions (INTRON Biotechnology, Korea). Nanodrop spectrophotometer (Genway Nanodrop, Germany) was used to determine RNA’s purities and concentrations. Using the RT-Premix Kit, 1 µg of RNA was used to produce cDNA (INTRON Biotechnology). A final volume of 20 µL was created by combining 2 µL of RT product with 10 µL of SYBR-Green master mix, 0.5 mM of each forward and reverse primer (Table 3), and nuclease-free water. All reactions were done at 95°C for 10 min, then 40 cycles of 95°C for 15 s, 58°C for 15 s, and 72°C for 30 s using a 7,500 Applied Biosystems, United States. The housekeeper gene β-actin was used to standardize the relative expression of mRNA. The 2−ΔΔCt approach, which Livak and Schmittgen (2001) developed, was used to calculate the fold changes in mRNA expression.
The three-dimension structures of RAC-alpha serine/threonine-protein kinase (AKT1), AKT2, AKT3, caspase-8, caspase-9, caspase-3, interleukin-6 receptor subunit alpha (IL6RA), IL6RB, mTOR, phosphatidylinositol 4,5-bisphosphate 3-kinase catalytic subunit alpha (PK3CA), and PK3CB were obtained from AlphaFold (https://alphafold.ebi.ac.uk/) protein structure database. Molecular Operating Environment (MOE 2015.10) software (Vilar et al., 2008) were used to prepare proteins for docking. In addition, the three-dimension structure of diosgenin was retrieved from PubChem (https://pubchem.ncbi.nlm.nih.gov/) database. Furthermore, MOE software did the molecular docking, protein-ligand interactions, and visualization.
GraphPad Prism v.9 (https://www.graphpad.com/) (GraphPad, San Diego, CA, United States) was used to analyze the data using a one-way ANOVA with Tukey’s post hoc multiple range testing. p < 0.05 was required for all significance declarations.
The dentate gyrus was found to be normal in the control and vehicle groups’ hippocampus (Figures 1A, B). Nevertheless, necrotic neurons with pycnotic nuclei, hyperchromatic neurons, and very few normal neurons were recognized in the dentate gyrus of D-gal-treated rats (Figure 1C). In rats given D-gal + DSG20 and D-gal + DSG40, the dentate gyrus shape was enhanced, and there were fewer degenerating neurons (Figures 1D, E).
FIGURE 1. Representative photomicrograph of rat hippocampus. (A) Control group and (B) Vehicle group; rats showing normal histoarchitecture of the dentate gyrus. (C) D-gal-treated rats revealing shrunken neurons with pyknotic nuclei (thick arrow) and hyperchromatic neurons (thin arrow). (D) D-gal + DSG20-treated and (E) D-gal + DSG40-treated rats revealing improved hippocampal architecture. (F) Hippocampal necrotic scores. Data were analyzed with a one-way ANOVA followed by Tukey’s multiple comparison test. ns = nonsignificant, *p < 0.05, ***p < 0.001, and ****p < 0.0001. Error bars represent mean ± SD. (HE, Scale bar= 50 µm). D-gal; D-galactose. DSG; diosgenin.
We detected a typical cerebellar architecture comprising molecular layer, Purkinje cell, and granular layers when we examined the rats’ cerebella in the control and vehicle groups (Figures 2A, B). Rats given D-gal demonstrated focal loss of granular layer neurons and total loss of necrotic nuclei in the Purkinje cell layer (Figure 2C). However, the histologic structure of the cerebellum in the D-gal + DSG20-treated rats was better and had fewer pyknotic Purkinje cells (Figure 2D). Additionally, animals given D-gal + DSG40 treatment displayed virtually health cerebellar architecture with very few deteriorated Purkinje cells (Figure 2E).
FIGURE 2. Representative photomicrograph of rat cerebellum. (A) Control group and (B) Vehicle group; rats illustrating normal histologic structure of cerebellum. (C) D-gal-treated rats revealing pyknotic Purkinje cells (arrowhead) in Purkinje cells layer (PCL), focal depletion of neurons (thin arrow) in granular layer (GL) and necrotic neurons (thick arrow) in molecular layer (ML). (D) D-gal + DSG20-treated rats showing nearly normal histologic structure with normal Purkinje cells (arrow) and a few lost or pyknotic (arrowhead) Purkinje cells. (E) D-gal + DSG40-treated rats showing normal hippocampal histoarchitecture with minimal degenerated (arrowhead) Purkinje cell. (F) Cerebellar necrotic scores. Data were analyzed with a one-way ANOVA followed by Tukey’s multiple comparison test. ns = nonsignificant, **p < 0.01, and ****p < 0.0001. Error bars represent mean ± SD. (HE, Scale bar = 50 µm). D-gal; D-galactose. DSG; diosgenin.
No histopathological hepatic abnormalities were found in the control and vehicle groups (Figures 3A, B). Conversely, the hepatic tissues of the D-gal group revealed hydropic degeneration, a build-up of inflammatory cells, and a dilated and blocked central vein (Figure 3C). The hepatic architecture improved in the D-gal + DSG20 group, and there were fewer pyknotic nuclei (Figure 3D). Additionally, compared with the control group, rats given D-gal + DSG40 treatment displayed a normal hepatic arch (Figure 3E). As represented in Figure 3F, the D-gal group exhibited a significantly higher vacuolation compared with the control group. On the other hand, hepatic lesions score significantly decreased with D-gal + DSG20 and D-gal + DSG40.
FIGURE 3. Representative photomicrograph of rat liver. (A) Control group. (B) vehicle group. (C) D-gal group showing hydropic degeneration (thick arrow) and necrosis of hepatocytes (arrowhead). (D) D-gal + DSG20. (E) D-gal + DSG40. (F) Hepatic necrotic scores. Data were analyzed with a one-way ANOVA followed by Tukey’s multiple comparison test. ns = nonsignificant. ****p < 0.0001. Error bars represent mean ± SD. (HE, Scale bar = 50 µm). D-gal; D-galactose. DSG; diosgenin.
The hippocampus (Figures 4A1, A2 and Figures 5A1, A2), cerebellum (Figures 4B1, B2 and Figures 5B1, B2), and liver (Figures 4C1, C2 and Figures 5C1, C2) revealed negative immune reactions for β-galactosidase and 8-OHdG, respectively in the control and vehicle groups. Conversely, the D-gal group displayed an abundance of β-galactosidase and 8-OHdG immune reactive nuclei in the brain regions (Figures 4A3, B3 and Figures 5A3, B3, respectively) and liver (Figure 4C3 and Figure 5C3, respectively).
FIGURE 4. Representative photomicrograph demonstrated immunohistochemical expression of β-galactosidase in hippocampus (A1–A5), cerebellum (B1–B5), and liver (C1–C5) in control (A1, B1, C1), vehicle (A2, B2, C2), D-gal- treated (A3, B3, C3), D-gal + DSG20-treated (A4, B4, C4), and D-gal + DSG40-treated (A5, B5, C5). Arrowheads indicate positive immune expression in D-gal-treated rats either with or without co-treatment with DSG20 or DSG40. (A6) Hippocampal β-galactosidase (Area %). (B6) Cerebellar β-galactosidase (Area %). (C6) Hepatic β-galactosidase (Area %). Data were analyzed with a one-way ANOVA followed by Tukey’s multiple comparison test. ns = nonsignificant, *p < 0.05, **p < 0.01, and ****p < 0.0001. Error bars represent mean ± SD. Scale bar = 50 mm. D-gal; D-galactose. DSG; diosgenin.
FIGURE 5. Representative photomicrograph demonstrated immunohistochemical expression of 8-hydroxy-2′-deoxyguanosine (8-OHdG) in hippocampus (A1–A5), cerebellum (B1–B5), and liver (C1–C5) from control (A1, B1, C1), vehicle (A2, B2, C2), D-gal-treated (A3, B3, C3), D-gal + DSG20-treated (A4, B4, C4), and D-gal + DSG40-treated (A5, B5, C5). Arrowheads indicate positive immune expression in D-gal-treated rats either with or without co-treatment with DSG20 or DSG40. (A6) Hippocampal 8-OHdG (Area %). (B6) Cerebellar 8-OHdG (Area %). (C6) Hepatic8-OHdG (Area %). Data were analyzed with a one-way ANOVA followed by Tukey’s multiple comparison test. ns = nonsignificant, ***p < 0.001, and ****p < 0.0001. Error bars represent mean ± SD. Scale bar = 50 mm. D-gal; D-galactose. DSG; diosgenin.
In contrast, the D-gal group showed a higher distribution of β-galactosidase- and 8-OHdG-reacted nuclei than the D-gal + DSG20 group (Figures 4A4, B4, C4 and Figures 5A4, B4, C4, respectively). Additionally, the D-gal + DSG40 group showed the lowest distribution of nuclei reacting to β-galactosidase and 8-OHdG (Figures 4A5, B5, C5 and Figures 4A6, B6, C6, respectively). Rats treated with D-gal exhibit significantly higher expression than control rats in the area % of β-galactosidase and 8-OHdG’s immunohistochemical reacted nuclei. In the D-gal + DSG20 and D-gal + DSG40 groups, this expression was significantly reduced (Figures 4A6, B6, C6 and Figures 5A6, B6, C6, respectively).
Regarding the data shown in Figure 6A, the brain MDA concentration increased significantly (p < 0.0001) in the D-gal group compared with the control and vehicle groups. While it decreased significantly (p < 0.0001) in the D-gal + DSG20 and D-gal + DSG40 groups. Compared with the control and vehicle groups, there was no apparent difference between the D-gal + DSG20 and D-gal + DSG40 groups.
FIGURE 6. Brain oxidative stress and antioxidant status. (A) Brain malondialdehyde (MDA). (B) Brain superoxide dismutase (SOD1). (C) Brain glutathione peroxidase (GPx-1). (D) Brain glutathione S-transferase (GST-α). Data were analyzed with a one-way ANOVA followed by Tukey’s multiple comparison test. ns = nonsignificant, *p < 0.05, **p < 0.01, ***p < 0.001, and ****p < 0.0001. Error bars represent mean ± SD. n = 4. D-gal; D-galactose. DSG; diosgenin.
According to the data in Figure 6B, brain SOD1 concentrations were significantly lower in the D-gal group than the control (p < 0.05) and vehicle groups (p < 0.01) and higher in the D-gal + DSG20 (p < 0.001) and D-gal + DSG40 groups (p < 0.0001) than in the D-gal group.
Its concentration was not changed considerably in the D-gal + DSG20 group relative to the control group, but it was significantly higher in the D-gal + DSG40 group (p < 0.001) than the control group.
According to the data in Figure 6C, the brain GPx-1 levels were significantly (p < 0.05) lower in the D-gal group than in the control and vehicle groups. In contrast, it was significantly (p < 0.0001) higher in the D-gal + DSG20 and D-gal + DSG40 groups than in the D-gal group. Additionally, its concentration was considerably higher in the D-gal + DSG40 group in comparison with the control, vehicle, and D-gal + DSG20 groups (p < 0.0001), (p < 0.0001), and (p < 0.001), respectively.
According to the data shown in Figure 6D, the brain GST-α levels were significantly (p < 0.001) lower in the D-gal group compared with the control and vehicle groups. At the same time, it was significantly (p < 0.01) higher in the D-gal + DSG20 and D-gal + DSG40 groups compared with the D-gal group. Furthermore, compared with the control and vehicle groups, there was no considerable difference in their concentration in the D-gal + DSG20 and D-gal + DSG40 groups.
MDA levels were higher (p < 0.0001) in the D-gal group (Figure 7A). SOD1 (Figure 7B), GPx-1 (Figure 7C), and GST-α (Figure 7D) levels were all considerably lower (p < 0.0001, p < 0.01, and p < 0.01, respectively). The D-gal treated with DSG demonstrated dose-dependently substantial increases in SOD1, GPx-1, and GST-α and significant decreases in MDA in the D-gal + DSG20 and D-gal + DSG40 groups (p < 0.0001).
FIGURE 7. Hepatic oxidative stress and antioxidant status. (A) Hepatic MDA. (B) Hepatic SOD1. (C) Hepatic GPx-1. (D) Hepatic GST-α. Data were analyzed with a one-way ANOVA followed by Tukey’s multiple comparison test. ns = nonsignificant, *p < 0.05, **p < 0.01, ***p < 0.001, and ****p < 0.0001. Error bars represent mean ± SD. n = 4. D-gal; D-galactose. DSG; diosgenin.
Relative to the control and vehicle groups, the brain’s p53 mRNA levels in the D-gal group were considerably higher (p < 0.0001). P53 expressions were significantly lower in the D-gal + DSG20 (p < 0.01) and D-gal + DSG40 (p < 0.0001) groups compared with the D-gal group. Also, compared with the control (p < 0.05) and D-gal + DSG20 groups, its expression levels were considerably lower in the D-gal + DSG40 group (Figure 8A).
FIGURE 8. mRNA relative fold change expression of brain tissue. (A) p53. (B) p21. (C) Mammalian target of rapamycin (mTOR). (D) caspase-3 (CASP3). (E) Bcl2-associated X protein (Bax). (F) And B-cell lymphoma 2 (Bcl2). Data were analyzed with a one-way ANOVA followed by Tukey’s multiple comparison test. ns = nonsignificant, *p < 0.05, **p < 0.01, ***p < 0.001, and ****p < 0.0001. Error bars represent mean ± SD. n = 6. D-gal; D-galactose. DSG; diosgenin.
Related to the control and vehicle groups, the brain p21 mRNA expressions in the D-gal group were considerably higher (p < 0.0001). However, as compared with the D-gal group, the brain p21 mRNA expressions in the D-gal + DSG20 and D-gal + DSG40 groups were significantly lower (p < 0.0001) than in the D-gal group (Figure 8B).
Relative to the control and vehicle groups, the brain mTOR mRNA expressions in the D-gal group were considerably higher (p < 0.0001). However, when compared with the D-gal group, its expressions were dramatically reduced (p < 0.0001) in the D-gal + DSG20 and D-gal + DSG40 groups (Figure 8C).
Compared with the control and vehicle groups, the brain CASP3 mRNA expressions in the D-gal group were considerably higher (p < 0.0001). Figure 8D shows that the levels in the D-gal + DSG20 and D-gal + DSG40 groups were significantly lower (p < 0.0001) than those in the D-gal group.
Compared with the control and vehicle groups, the brain Bax mRNA expressions in the D-gal group were considerably higher (p < 0.0001). At the same time, the brain Bax mRNA expressions in the D-gal + DSG20 and D-gal + DSG40 groups were significantly lower (p < 0.0001) than those in the D-gal group. The brain Bax mRNA expressions were considerably down in the D-gal + DSG20 (p < 0.01) and D-gal + DSG40 (p < 0.001) than the control group (Figure 8E).
When compared with the control and vehicle groups, the brain Bcl2 mRNA levels in the D-gal group were considerably lower (p < 0.0001) and lower (p < 0.001). On the other hand, relative to the D-gal group, the Bcl2 mRNA expressions in the brain were significantly higher in the D-gal + DSG20 and D-gal + DSG40 groups (p < 0.0001). Additionally, compared with the control, vehicle, and D-gal + DSG20 groups, Bcl2 expressions were considerably (p < 0.0001) higher in the D-gal + DSG40 group (Figure 8F).
Like how Bcl2 (Figure 9F) expression was dramatically reduced (p < 0.01), hepatic p53 (Figure 9A), p21 (Figure 9B), mTOR (Figure 9C), CASP3 (Figure 9D), and Bax (Figure 9E) mRNA expression was significantly elevated in the D-gal group (p < 0.0001) compared with control. In contrast, Bcl2 expression was dramatically elevated in a dosage-dependent manner in the D-gal + DSG20 and D-gal + DSG40 groups than the D-gal group, while p53, p21, CASP3, Bax, and mTOR mRNA levels were significantly lowered.
FIGURE 9. mRNA relative fold change expression of hepatic tissue. (A) p53. (B) p21. (C) Mammalian target of rapamycin (mTOR). (D) caspase-3 (CASP3). (E) Bcl2-associated X protein (Bax). (F) And B-cell lymphoma 2 (Bcl2). Data were analyzed with a one-way ANOVA followed by Tukey’s multiple comparison test. ns = nonsignificant, *p < 0.05, **p < 0.01, ***p < 0.001, and ****p < 0.0001. Error bars represent mean ± SD. n = 6. D-gal; D-galactose. DSG; diosgenin.
Diosgenin interacted with the binding sites of rats’ AKT1 (Figure 10A), AKT2 (Figure 10B), AKT3 (Figure 10C), caspase-8 (Figure 10D), caspase-9 (Figure 10E), caspase-3 (Figure 10F), IL6RA (Figure 10G), IL6RB (Figure 10H), mTOR (Figure 10I), PK3CA (Figure 10J), and PK3CB (Figure 10J) by binding energies of −6.68, −6.26, −5.79, −7.06, −4.66, −5.72, −5.78, −7.02, −5.75, −6.71, and −6.77 kcal/mol, respectively.
FIGURE 10. Molecular docking interaction of diosgenin with (A) protein kinase B1 (AKT1), (B) protein kinase B2 (AKT2), (C) protein kinase B3 (AKT3), (D) caspase-8, (E) caspase-9, (F) caspase-3, (G) interleukin-6 receptor subunit alpha (IL6RA), (H) interleukin-6 receptor subunit beta (IL6RB), (I) mammalian target of rapamycin (mTOR), (J) phosphatidylinositol 4,5-bisphosphate 3-kinase catalytic subunit alpha (PK3CA), and (K) phosphatidylinositol 4,5-bisphosphate 3-kinase catalytic subunit beta (PK3CB).
Cellular hallmarks of aging include accumulation of β-galactosidase and overexpression of p53 and p21. Expression levels of p53 and p21 mRNA were upregulated along with considerable β-galactosidase immunostaining expression in the D-gal group. El-Far et al. (2021, 2022) and Saafan et al. (2023) found that rats in the D-gal groups had significantly higher levels of p53 and p21 expression in their brains. Additionally, mice given D-gal injections showed an increase in brain p21, according to Sun et al. (2018). Further, p53 and p21 were upregulated in the pancreas and kidneys of rats that had received D-gal injections to accelerate aging (El-Far et al., 2020). Western blot research has shown that rats given D-gal treatment have considerably higher p53, p21, and β-galactosidase protein expression in their liver (Huang et al., 2013). Furthermore, a significant build-up of β-galactosidase in the aged rats’ hippocampus (Li et al., 2016).
Neurodegenerative disorders and the natural aging process have oxidative stress as a significant contributing element (Yin et al., 2009). Increased ROS levels are likely to trigger cellular senescence (Liguori et al., 2018). However, the precise mechanism of oxidative stress-induced aging is uncertain. In the current investigation, D-gal markedly reduced SOD1, GPx-1, and GST levels in the brain and liver. Similarly, significant increases in the expression of 8-OHdG levels were reported by Du et al. (2019). The authors recognized reduction in total SOD and GPx activities in the D-gal-induced aging paradigm. Similar to this, animals treated with D-gal had their brain GPx and GST activities significantly reduced (El-Far et al., 2022). Also, SOD1 protein (Kuo et al., 2022) and SOD activity (Kuo et al., 2022) were decreased in the brain of rats injected with D-gal. Significant drops in the levels of Sirt1, Bcl2, CAT, and GPx were reported by Motevalian et al. (2021) in D-gal-treated mice.
In the present study, DSG overcame the oxidative and apoptotic alterations induced by D-gal in the brain and hepatic tissues. Moreover, molecular docking assessment stated the binding affinity of DSG to control apoptotic and inflammatory targets. By lowering a build-up, increasing SOD activity, and minimizing lipid peroxidation, and DSG prevented brain cell death. Similarly, Koh et al. (2016) reported significant enhancement in SOD activity with reduction in MDA levels due to DSG in the brain with neuronal damage induced by Aβ-42 accumulation and neurotoxicant injection. Also, DSG suppressed D-gal-induced neuronal Fas-dependent and mitochondria-dependent apoptotic pathways in rats (Cheng et al., 2020). Moreover, DSG successfully protected the dopaminergic neurons from LPS-induced neuroinflammation that was monitored by significant reduction in tumor necrosis factor-α and inducible nitric oxide synthase (Lee et al., 2021).
Free radicals produced lipid peroxidation, deactivation of enzymes, apoptosis and DNA breakup (Valko et al., 2007). In the current investigation, we reported significant reductions in Bcl2 expression and increased apoptosis, as evidenced by overexpression of CASP3 and Bax expression, in the hippocampus of rats. Atef et al. (2022) found increased immunostaining expression caspase-3 in the brain. Moreover, caspase-3, Bcl2, Bax, and CASP3 levels in the brain and heart were also increased in rats treated with D-gal (El-Far et al., 2021). Similarly, in our previous study, we reported significant reductions in Bcl2 expression and increased apoptosis as evidenced by overexpression of CASP3, Bax, and Bax protein (El-Far et al., 2021).
Natural compounds have been extensively employed in D-gal-inducing models for their anti-aging properties (El-Far et al., 2020; El-Far et al., 2021; El-Far et al., 2022; Saafan et al., 2023). The current investigation dramatically improved the antioxidant status of the rats’ brains and livers by adding DSG to the D-gal-treated rats. In the same setting, D-gal significantly raised the Bax/Bcl-2 ratio and caspase-3 in the brains of mice (Zhang et al., 2019) and rats (Ullah et al., 2015). On the contrary, DSG may have neuroprotective effects for preventing D-gal-induced brain aging by enhancing the Bcl-2 family (Cheng et al., 2020). Also, the mTOR is known to control various signs of aging (Weichhart, 2018). We reported a substantial overexpression of mTOR in the current investigation. Similarly, mTOR expression was elevated due to D-gal (Saafan et al., 2023).
Inflammation and oxidative stress are recognized as the two primary aging-related processes. Through the downregulation of aging markers (p53, p21, and β-galactosidase) and apoptotic markers (Bax and CASP3), as well as the improvement of antioxidant status, histomorphology, and immunohistochemical evaluation of the brain and hepatic tissues, DSG may have been able to mitigate the oxidative stress caused by D-gal in the rat brain and liver tissues. Besides, DSG exhibited a high affinity to inhibit apoptotic and inflammatory target proteins. Our findings imply that DSG successfully slowed down the rats’ brain and liver tissue aging through targeting of aging and apoptotic genes along with enhancement of cellular antioxidant status and overcome of inflammatory process, making it a potentially effective natural anti-aging supplement.
The raw data supporting the conclusions of this article will be made available by the authors, without undue reservation.
The Faculty of Veterinary Medicine Ethics Committee at Damanhour University in Egypt has accepted all techniques in compliance with the ARRIVE guidelines (https://arriveguidelines.org) (DMU/VetMed-2023/028). The studies were conducted in accordance with the local legislation and institutional requirements. Written informed consent was obtained from the owners for the participation of their animals in this study.
AE-F: Conceptualization, Data curation, Formal Analysis, Funding acquisition, Investigation, Methodology, Resources, Software, Supervision, Validation, Visualization, Writing–original draft, Writing–review and editing. ME: Conceptualization, Data curation, Funding acquisition, Investigation, Methodology, Resources, Validation, Writing–review and editing. SM: Data curation, Formal Analysis, Investigation, Methodology, Resources, Software, Supervision, Validation, Visualization, Writing–review and editing. AN: Formal Analysis, Investigation, Methodology, Resources, Validation, Visualization, Writing–original draft, Writing–review and editing. YE: Conceptualization, Data curation, Formal Analysis, Investigation, Methodology, Resources, Software, Visualization, Writing–review and editing. SJ: Writing–review and editing, Resources, Validation, Visualization. AA: Conceptualization, Data curation, Formal Analysis, Investigation, Methodology, Project administration, Resources, Software, Supervision, Validation, Visualization, Writing–original draft, Writing–review and editing.
The author(s) declare that no financial support was received for the research, authorship, and/or publication of this article.
The authors declare that the research was conducted in the absence of any commercial or financial relationships that could be construed as a potential conflict of interest.
All claims expressed in this article are solely those of the authors and do not necessarily represent those of their affiliated organizations, or those of the publisher, the editors and the reviewers. Any product that may be evaluated in this article, or claim that may be made by its manufacturer, is not guaranteed or endorsed by the publisher.
The Supplementary Material for this article can be found online at: https://www.frontiersin.org/articles/10.3389/fmolb.2024.1303379/full#supplementary-material
Al-Matubsi, H. Y., Nasrat, N. A., Oriquat, G. A., Abu-Samak, M., Al-Mzain, K. A., and Salim, M. (2011). The hypocholesterolemic and antioxidative effect of dietary diosgenin and chromium chloride supplementation on high-cholesterol fed Japanese quails. Pak. J. Biol. Sci. 14, 425–432. doi:10.3923/PJBS.2011.425.432
Atef, M. M., Emam, M. N., Abo El Gheit, R. E., Elbeltagi, E. M., Alshenawy, H. A., Radwan, D. A., et al. (2022). Mechanistic insights into ameliorating effect of geraniol on d-galactose induced memory impairment in rats. Neurochem. Res. 47, 1664–1678. doi:10.1007/s11064-022-03559-3
Bancroft, J. D. J., and Layton, C. (2013). “The Hematoxylin and eosin,” in Theory Practice of histological techniques Editors S. K. Suvarna, C. Layton, and J. D. Bancroft 7 (Philadelphia: Philadelphia: Churchill Livingstone of El Sevier).
Birben, E., Sahiner, U. M., Sackesen, C., Erzurum, S., and Kalayci, O. (2012). Oxidative stress and antioxidant defense. World Allergy Organ. J. 5, 9–19. doi:10.1097/WOX.0b013e3182439613
Buege, J. A., and Aust, S. D. (1978). Microsomal lipid peroxidation. Methods Enzymol. 52, 302–310. doi:10.1016/s0076-6879(78)52032-6
Cheng, S.-M., Ho, Y.-J., Yu, S.-H., Liu, Y.-F., Lin, Y.-Y., Huang, C.-Y., et al. (2020). Anti-apoptotic effects of diosgenin in D-galactose-induced aging brain. Am. J. Chin. Med. 48, 391–406. doi:10.1142/S0192415X20500202
Chiang, Y. R., Wei, S. T. S., Wang, P. H., Wu, P. H., and Yu, C. P. (2020). Microbial degradation of steroid sex hormones: implications for environmental and ecological studies. Microb. Biotechnol. 13, 926–949. doi:10.1111/1751-7915.13504
de Almeida Rezende, M. S., Oliveira de Almeida, A. J. P., Gonçalves, T. A. F., de Azevedo, F. de L. A. A., Dantas, S. H., Silva, S. de L., et al. (2021). D-(+)-Galactose-induced aging: a novel experimental model of erectile dysfunction. PLoS One 16, e0249487. doi:10.1371/journal.pone.0249487
Du, Z., Yu, S., Qi, Y., Qu, T. F., He, L., Wei, W., et al. (2019). NADPH oxidase inhibitor apocynin decreases mitochondrial dysfunction and apoptosis in the ventral cochlear nucleus of D-galactose-induced aging model in rats. Neurochem. Int. 124, 31–40. doi:10.1016/J.NEUINT.2018.12.008
El-Far, A. H., Elewa, Y. H. A., Abdelfattah, E.-Z. A., Alsenosy, A.-W. A., Atta, M. S., Abou-Zeid, K. M., et al. (2020). Quercetin attenuates pancreatic and renal D-galactose-induced aging-related oxidative alterations in rats. Int. J. Mol. Sci. 21, 6839. doi:10.3390/ijms21124348
El-Far, A. H., Elewa, Y. H. A., Abdelfattah, E.-Z. A., Alsenosy, A.-W. A., Atta, M. S., Abou-Zeid, K. M., et al. (2021). Thymoquinone and curcumin defeat aging-associated oxidative alterations induced by d-galactose in rats’ brain and heart. Int. J. Mol. Sci. 22, 6839. doi:10.3390/ijms22136839
El-Far, A. H., Mohamed, H. H., Elsabagh, D. A., Mohamed, S. A., Noreldin, A. E., Al Jaouni, S. K., et al. (2022). Eugenol and carvacrol attenuate brain d-galactose-induced aging-related oxidative alterations in rats. Environ. Sci. Pollut. Res. 29, 47436–47447. doi:10.1007/s11356-022-18984-8
Fan, J., Yang, X., Li, J., Shu, Z., Dai, J., Liu, X., et al. (2017). Spermidine coupled with exercise rescues skeletal muscle atrophy from D-gal-induced aging rats through enhanced autophagy and reduced apoptosis via AMPK-FOXO3a signal pathway. Oncotarget 8, 17475–17490. doi:10.18632/oncotarget.15728
Ferrucci, L., and Fabbri, E. (2018). Inflammageing: chronic inflammation in ageing, cardiovascular disease, and frailty. Nat. Rev. Cardiol. 15, 505–522. doi:10.1038/s41569-018-0064-2
Gibson-Corley, K. N., Olivier, A. K., and Meyerholz, D. K. (2013). Principles for valid histopathologic scoring in research. Vet. Pathol. 50, 1007–1015. doi:10.1177/0300985813485099
Guzik, T. J., and Touyz, R. M. (2017). Oxidative stress, inflammation, and vascular aging in hypertension. Hypertension 70, 660–667. doi:10.1161/HYPERTENSIONAHA.117.07802
Hou, J., Yun, Y., Xue, J., Sun, M., and Kim, S. (2019). D-galactose induces astrocytic aging and contributes to astrocytoma progression and chemoresistance via cellular senescence. Mol. Med. Rep. 20, 4111–4118. doi:10.3892/mmr.2019.10677
Huang, C.-C. C.-Y., Chiang, W.-D., Huang, W.-C., Huang, C.-C. C.-Y., Hsu, M.-C., and Lin, W.-T. (2013). Hepatoprotective effects of swimming exercise against D-galactose-induced senescence rat model. Evidence-Based Complement. Altern. Med. 2013, 275431–275439. doi:10.1155/2013/275431
Jagadeesan, J., Nandakumar, N., Rengarajan, T., and Balasubramanian, M. P. (2012). Diosgenin, a steroidal saponin, exhibits anticancer activity by attenuating lipid peroxidation via enhancing antioxidant defense system during NMU-induced breast carcinoma. J. Environ. Pathol. Toxicol. Oncol. 31, 121–129. doi:10.1615/JENVIRONPATHOLTOXICOLONCOL.V31.I2.40
Juan, C. A., Pérez de la Lastra, J. M., Plou, F. J., and Pérez-Lebeña, E. (2021). The chemistry of reactive oxygen species (ROS) revisited: outlining their role in biological macromolecules (DNA, lipids and proteins) and induced pathologies. Int. J. Mol. Sci. 22, 4642. doi:10.3390/ijms22094642
Koh, E.-K., Yun, W.-B., Kim, J.-E., Song, S.-H., Sung, J.-E., Lee, H.-A., et al. (2016). Beneficial effect of diosgenin as a stimulator of NGF on the brain with neuronal damage induced by Aβ-42 accumulation and neurotoxicant injection. Lab. Anim. Res. 32, 105–115. doi:10.5625/lar.2016.32.2.105
Kuo, H.-C., Tong, S.-Y., Chao, M.-W., and Tseng, C.-Y. (2022). Ganoderma tsugae prevents cognitive impairment and attenuates oxidative damage in d-galactose-induced aging in the rat brain. PLoS One 17, e0266331. doi:10.1371/journal.pone.0266331
Lee, S.-L., Tu, S.-C., Hsu, M.-Y., and Chin, T.-Y. (2021). Diosgenin prevents microglial activation and protects dopaminergic neurons from lipopolysaccharide-induced neural damage in vitro and in vivo. Int. J. Mol. Sci. 22, 10361. doi:10.3390/ijms221910361
Li, X., Chen, Y. Y., Shao, S., Tang, Q., Chen, W., Chen, Y. Y., et al. (2016). Oxidative stress induces the decline of brain EPO expression in aging rats. Exp. Gerontol. 83, 89–93. doi:10.1016/J.EXGER.2016.07.012
Liguori, I., Russo, G., Curcio, F., Bulli, G., Aran, L., Della-Morte, D., et al. (2018). Oxidative stress, aging, and diseases. Clin. Interv. Aging 13, 757–772. doi:10.2147/CIA.S158513
Livak, K. J., and Schmittgen, T. D. (2001). Analysis of relative gene expression data using real-time quantitative PCR and the 2(-Delta Delta C(T)) Method. methods 25, 402–408. doi:10.1006/meth.2001.1262
López-Otín, C., Blasco, M. A., Partridge, L., Serrano, M., and Kroemer, G. (2013). The hallmarks of aging. Cell 153, 1194–1217. doi:10.1016/j.cell.2013.05.039
McAnuff, M. A., Harding, W. W., Omoruyi, F. O., Jacobs, H., Morrison, E. Y., and Asemota, H. N. (2005). Hypoglycemic effects of steroidal sapogenins isolated from Jamaican bitter yam, Dioscorea polygonoides. Food Chem. Toxicol. 43, 1667–1672. doi:10.1016/J.FCT.2005.05.008
Motevalian, M., Tekyeh Maroof, N., Nematollahi, M. H., Khajehasani, F., and Fatemi, I. (2021). Atorvastatin modulates the expression of aging-related genes in the brain of aging induced by D-galactose in mice. Iran. J. Basic Med. Sci. 24, 1388–1394. doi:10.22038/IJBMS.2021.58502.12996
Noreldin, A. E., Elewa, Y. H. A., Kon, Y., Warita, K., and Hosaka, Y. Z. (2018). Immunohistochemical localization of osteoblast activating peptide in the mouse kidney. Acta histochem. 120, 323–328. doi:10.1016/j.acthis.2018.03.001
Parameshwaran, K., Irwin, M. H., Steliou, K., and Pinkert, C. A. (2010). D-galactose effectiveness in modeling aging and therapeutic antioxidant treatment in mice. Rejuvenation Res. 13, 729–735. doi:10.1089/rej.2010.1020
Prasad, M., Jayaraman, S., Rajagopal, P., Veeraraghavan, V. P., Kumar, P. K., Piramanayagam, S., et al. (2022). Diosgenin inhibits ER stress-induced inflammation in aorta via iRhom2/TACE mediated signaling in experimental diabetic rats: an in vivo and in silico approach. Chem. Biol. Interact. 358, 109885. doi:10.1016/j.cbi.2022.109885
Remigante, A., Spinelli, S., Trichilo, V., Loddo, S., Sarikas, A., Pusch, M., et al. (2022). d-Galactose induced early aging in human erythrocytes: role of band 3 protein. J. Cell. Physiol. 237, 1586–1596. doi:10.1002/jcp.30632
Rodríguez-Rodero, S., Fernández-Morera, J. L., Menéndez-Torre, E., Calvanese, V., Fernández, A. F., and Fraga, M. F. (2011). Aging genetics and aging. Aging Dis. 2, 186–195.
Saafan, S. M., Mohamed, S. A., Noreldin, A. E., El Tedawy, F. A., Elewa, Y. H. A., Fadly, R. S., et al. (2023). Rutin attenuates D-galactose-induced oxidative stress in rats’ brain and liver: molecular docking and experimental approaches. Food Funct. 14, 5728–5751. doi:10.1039/D2FO03301A
Son, I. S., Kim, J. H., Sohn, H. Y., Son, K. H., Kim, J. S., and Kwon, C. S. (2007). Antioxidative and hypolipidemic effects of diosgenin, a steroidal saponin of yam (Dioscorea spp.), on high-cholesterol fed rats. Biosci. Biotechnol. Biochem. 71, 3063–3071. doi:10.1271/bbb.70472
Song, X., Bao, M., Li, D., and Li, Y. M. (1999). Advanced glycation in d-galactose induced mouse aging model. Mech. Ageing Dev. 108, 239–251. doi:10.1016/S0047-6374(99)00022-6
Subhashini, N., Nagarajan, G., and Kavimani, S. (2011). Anti-inflammatory and in vitro antioxidant property of Trigonella foenum graecum seeds. J. Pharmacol. Toxicol. 6, 371–380. doi:10.3923/JPT.2011.371.380
Sun, K., Yang, P., Zhao, R., Bai, Y., and Guo, Z. (2018). Matrine attenuates D-galactose-induced aging-related behavior in mice via inhibition of cellular senescence and oxidative stress. Oxid. Med. Cell. Longev. 2018, 7108604–7108612. doi:10.1155/2018/7108604
Sysel, A. M., Valli, V. E., Nagle, R. B., and Bauer, J. A. (2013). Immunohistochemical quantification of the vitamin B12 transport protein (TCII), cell surface receptor (TCII-R) and Ki-67 in human tumor xenografts. Anticancer Res. 33, 4203–4212.
Ullah, F., Ali, T., Ullah, N., and Kim, M. O. (2015). Caffeine prevents d-galactose-induced cognitive deficits, oxidative stress, neuroinflammation and neurodegeneration in the adult rat brain. Neurochem. Int. 90, 114–124. doi:10.1016/j.neuint.2015.07.001
Valko, M., Leibfritz, D., Moncol, J., Cronin, M. T. D. D., Mazur, M., and Telser, J. (2007). Free radicals and antioxidants in normal physiological functions and human disease. Int. J. Biochem. Cell Biol. 39, 44–84. doi:10.1016/j.biocel.2006.07.001
Vilar, S., Cozza, G., and Moro, S. (2008). Medicinal chemistry and the molecular operating environment (MOE): application of QSAR and molecular docking to drug discovery. Curr. Top. Med. Chem. 8, 1555–1572. doi:10.2174/156802608786786624
Vis, A. N., Kranse, R., Nigg, A. L., and van der Kwast, T. H. (2000). Quantitative analysis of the decay of immunoreactivity in stored prostate needle biopsy sections. Am. J. Clin. Pathol. 113, 369–373. doi:10.1309/CQWY-E3F6-9KDN-YV36
Wang, S., Zhang, X., Ke, Z., Wen, X., Li, W., Liu, W., et al. (2022). D-galactose-induced cardiac ageing: a review of model establishment and potential interventions. J. Cell. Mol. Med. 26, 5335–5359. doi:10.1111/jcmm.17580
Weichhart, T. (2018). mTOR as regulator of lifespan, aging, and cellular senescence: a mini-review. Gerontology 64, 127–134. doi:10.1159/000484629
Yin, S. T., Tang, M. L., Deng, H. M., Xing, T. R., Chen, J. T., Wang, H. L., et al. (2009). Epigallocatechin-3-gallate induced primary cultures of rat hippocampal neurons death linked to calcium overload and oxidative stress. Naunyn. Schmiedeb. Arch. Pharmacol. 379, 551–564. doi:10.1007/s00210-009-0401-4
Zhang, X., Wu, J. Z., Lin, Z. X., Yuan, Q. J., Li, Y. C., Liang, J. L., et al. (2019). Ameliorative effect of supercritical fluid extract of Chrysanthemum indicum Linnén against D-galactose induced brain and liver injury in senescent mice via suppression of oxidative stress, inflammation and apoptosis. J. Ethnopharmacol. 234, 44–56. doi:10.1016/j.jep.2018.12.050
Keywords: diosgenin, oxidative stress, aging, antioxidants, brain, liver
Citation: El-Far AH, Elghaity MM, Mohamed SA, Noreldin AE, Elewa YHA, Al Jaouni SK and Alsenosy AA (2024) Diosgenin alleviates D-galactose-induced oxidative stress in rats’ brain and liver targeting aging and apoptotic marker genes. Front. Mol. Biosci. 11:1303379. doi: 10.3389/fmolb.2024.1303379
Received: 27 September 2023; Accepted: 26 January 2024;
Published: 23 February 2024.
Edited by:
Muhammad Sajid Hamid Akash, Government College University, Faisalabad, PakistanReviewed by:
Hoda Ibrahim Bahr, Suez Canal University, EgyptCopyright © 2024 El-Far, Elghaity, Mohamed, Noreldin, Elewa, Al Jaouni and Alsenosy. This is an open-access article distributed under the terms of the Creative Commons Attribution License (CC BY). The use, distribution or reproduction in other forums is permitted, provided the original author(s) and the copyright owner(s) are credited and that the original publication in this journal is cited, in accordance with accepted academic practice. No use, distribution or reproduction is permitted which does not comply with these terms.
*Correspondence: Ali H. El-Far, YWxpLmVsZmFyQGRhbWFuaG91ci5lZHUuZWc=; Soad Khalil Al Jaouni, c2FsamFvdW5pQGthdS5lZHUuc2E=
†These authors have contributed equally to this work and share first authorship
Disclaimer: All claims expressed in this article are solely those of the authors and do not necessarily represent those of their affiliated organizations, or those of the publisher, the editors and the reviewers. Any product that may be evaluated in this article or claim that may be made by its manufacturer is not guaranteed or endorsed by the publisher.
Research integrity at Frontiers
Learn more about the work of our research integrity team to safeguard the quality of each article we publish.