- Neurofarba Department, Section of Pharmaceutical Sciences, University of Florence, Florence, Italy
While the carbonic anhydrase (CA, EC 4.2.1.1) superfamily of enzymes has been described primarily as involved only in pH regulation for decades, it also has many other important functions. CO2, bicarbonate, and protons, the physiological substrates of CA, are indeed the main buffering system in organisms belonging to all life kingdoms; however, in the last period, relevant progress has been made in the direction of elucidating the involvement of the eight genetically distinct CA families in chemical sensing, metabolism, and several other crucial physiological processes. Interference with CA activity, both by inhibiting and activating these enzymes, has thus led to novel applications for CA inhibitors and activators in the field of innovative biomedicine and environment and health. In this perspective article, I will discuss the recent advances which have allowed for a deeper understanding of the biochemistry of these versatile enzymes and various applications of their modulators of activity.
1 Introduction
Discovered 90 years ago in the human blood (Meldrum and Roughton, 1933), during experiments aimed to understand the transport of gases in the vertebrates’ blood, the enzyme carbonic anhydrase (CA, EC 4.2.1.1) was considered for decades the quintessential metalloenzyme and a relevant tool for studying the physiology and biochemistry of the crucial molecules/ions acting as its substrates or reaction products (Krebs and Roughton, 1948; Supuran, 2023a). CA catalyzes the reversible interconversion between CO2 and bicarbonate, effectively hydrating this metabolic gas, generated in oxidative processes in all organisms, to soluble products: bicarbonate and protons (Lindskog, 1997; Supuran, 2008). The vertebrate enzymes, which all belong to the α-CA family, indeed use a zinc hydroxide mechanism for achieving this reaction at a physiological pH, with a huge efficacy, some of them being amongst the best catalysts known in nature, with kcat/KM values >108 M−1 s−1 and kcat values >106 s−1 (Lindskog, 1997; Supuran, 2016). During the years, novel CA genetic families have been discovered in organisms all over the phylogenetic tree, and currently, eight of them are known: α-, β-, γ-, δ-, ζ-, η-, θ-, and ι-CAs (Alber and Ferry, 1994; Cox et al., 2000; Xu et al., 2008; Del Prete et al., 2014; Capasso and Supuran, 2015; Kikutani et al., 2016; Jensen et al., 2019; Jin et al., 2020; Hirakawa et al., 2021). There are in fact very few organisms in which these enzymes were absent: few bacteria and one archaeon (Smith et al., 1999; Ueda et al., 2012). All of them, except ι-CAs, are metalloenzymes, and in addition to Zn(II), Cd(II), Fe(II), and Co(II) ions are found in their active sites, with the corresponding apo-enzymes being catalytically totally inactive (Lindskog, 1997; Ferry, 2010; Supuran, 2016) and the active-site metal hydroxide playing a crucial catalytic role (Lindskog, 1997). In fact, a water molecule coordinated at the metal ion is activated upon nucleophilic attack on the CO2 molecule bound in a hydrophobic pocket nearby, at the bottom of the active site cavity (Domsic et al., 2008; Aggarwal et al., 2015). This water molecule coordinated to the metal ion within the enzyme cavity has a pKa value of approximately 7, thus being orders of magnitude more nucleophilic than bulk water (Lindskog, 1997; Supuran, 2008; 2016). The rate-determining step of the entire catalytic cycle is, on the other hand, a proton transfer reaction from the metal-coordinated water molecule to the environment, which is assisted by an active-site amino acid residue with the appropriate pKa value, a His in most α-CAs (Steiner et al., 1975; Tu et al., 1989). However, Hirakawa et al. (2021) recently characterized ι-CAs from two different organisms, the cyanobacterium, Anabaena sp. PCC 7120, and the chlorarachniophyte alga, Bigelowiella natans, demonstrating that no metal ions are present in these CAs. As for the remaining seven CA genetic families, CO2 hydration is achieved by an active site-activated water molecule; however, as shown in Figure 1, the activation is performed without metal ions by three amino acid residues conserved in all ι-CAs investigated so far: Thr106, Ser199, and Tyr124 (Nocentini et al., 2021).
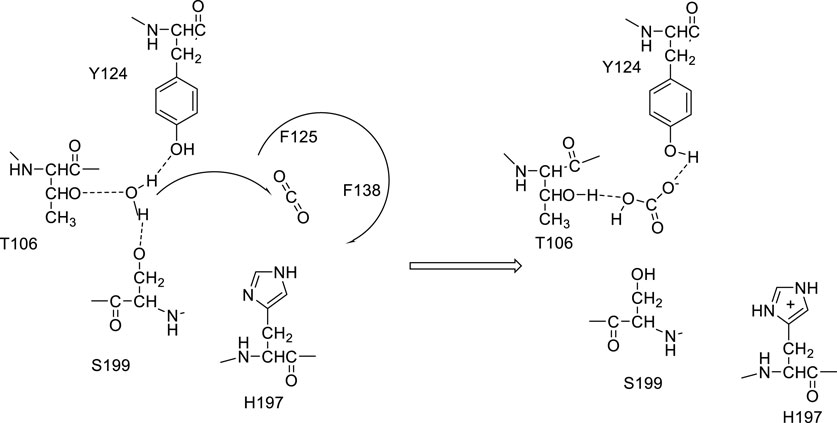
FIGURE 1. Proposed catalytic mechanism of ι-CAs: the water molecule is activated upon nucleophilic attack on the CO2 molecule bound in a hydrophobic pocket, through H-bond formation with T106, S199, and Y124. Proton transfer is presumably achieved by H197.
2 CAs and pH regulation
The CA-catalyzed reaction generates from two neutral molecules, CO2 and water, a weak base (bicarbonate) and a strong acid (H+ ions). This system is universally used for pH regulation for at least two reasons: 1) the facile and general availability of CO2, which as a metabolic gas is also possible to eliminate from the system rather easily, and 2) the widespread presence of CAs in most cells, tissues, and organisms and their huge catalytic activity with CO2 as a substrate (Supuran, 2023a). Thus, under both physiological conditions, in normal cells (Occhipinti and Boron, 2019) or in pathologic states, e.g., tumors (Neri and Supuran, 2011), CAs promote rapid buffering and the tight control/stability of pH-sensitive processes. In teleost fish, CA activity in muscle capillaries short-circuits pH regulation in red blood cells, acidifying the erythrocytes, which unloads O2 from hemoglobin, providing elevated tissue oxygenation, a phenomenon known as the Root effect (Rummer et al., 2013).
3 CO2/bicarbonate sensing
CO2 is a primary product of respiration, possibly playing other physiological roles, some of which are poorly understood, whereas its conversion to bicarbonate under the action of CAs triggers significant responses in most organisms that sense these two molecules in different ways (Cummins et al., 2014; Strowitzki et al., 2022). Altered levels of either CO2 or bicarbonate elicit the activation of multiple adaptive pathways both in prokaryotes and eukaryotes. Thus, in bacterial and fungal pathogens, CO2/bicarbonate sensing is correlated with increased virulence and/or pathogenicity (Bahn et al., 2005; Abuaita and Withey, 2009; Cottier et al., 2013). In insects, this chemosensing process is involved in prey-seeking behavior (Cummins et al., 2014), whereas in vertebrates, it is involved in taste perception (Chandrashekar et al., 2009), lung function (Kunert et al., 2022), and the control of immunity (Strowitzki et al., 2022). In algae, aquatic plants, diatoms, and cyanobacteria, CO2/bicarbonate sensing is highly relevant in order to supply sufficient CO2 for photosynthesis, and hence, sophisticated carbon-concentrating mechanisms have independently evolved in many such organisms (Momayyezi et al., 2020; Santhanagopalan et al., 2021; Zhang et al., 2022; Liao et al., 2023; Shimakawa et al., 2023).
4 Metabolism
In recent years, the involvement of CAs in metabolism has been considered and investigated in detail (Supuran, 2018). Several biosynthetic processes, which involve CO2/bicarbonate as substrates, including various carboxylation reactions and gluconeogenesis, are assisted by different CA isoforms, leading to the production of metabolic intermediates such as pyruvate, succinate, and fatty acids (Supuran, 2022). By using mitochondrial CA-selective inhibitors, it has been demonstrated that pyruvate metabolism was the most dramatically affected, followed by fatty acid metabolism and succinate metabolism (Arechederra et al., 2013). Santi et al. (2013) also showed that in tumors, bicarbonate formed from CO2 by hydration in the presence of CA IX or XII supplies cancer cells with intermediates utilized for sustaining their high proliferation rate through transformations in metabolic intermediates, as those described previously. The involvement of CAs in the metabolism of pathogenic bacteria, fungi, and protozoans was less investigated, but it seems to be as relevant as for mammalian cells (Supuran and Capasso, 2021; Supuran, 2023b).
5 Discussion and conclusion
In this section, I will discuss several applications of these enzymes and their modulation of activity in the field of biomedicine and environment and health. Its application in crop engineering, i.e., integrating CAs involved in C4 or crassulacean acid metabolism or algal carbon-concentrating mechanisms (in which various CAs are also involved) into cultivars for boosting agricultural yields has been recently demonstrated to be feasible (Findinier and Grossman AR, 2023; Förster et al., 2023) and might be of crucial relevance in a planet with >8 billion inhabitants. The field is in its infancy, but the recent breakthrough mentioned previously as well as other similar research studies (He et al., 2023) showed that both α- and β-CAs of rice (Oryza sativa) are essential for photosynthesis, providing the possibility for engineering plants for high-yielding crops.
In the era of global warming, CO2 capture has been seriously considered a possibility to relieve the long-term consequences of anthropic hot greenhouse gas emissions (Migliardini et al., 2014; Del Prete et al., 2016; Del Prete et al., 2019; Talekar et al., 2022; Huili et al., 2023; Villa et al., 2023). Various enzymes, many derived from extremophilic organisms, which provided highly thermostable CAs, have been proposed for such a purpose, either for transforming CO2 into bicarbonate used for algal growth, precipitating it as CaCO3, or transforming it into organic compounds, such as oxaloacetate (Del Prete et al., 2016).
Inhibiting human CAs has been clinically used for several decades, with many available drugs acting as diuretic, antiglaucoma, antiepileptic, antiobesity, and antitumor agents or as drugs for acute mountain sickness and idiopathic intracranial hypertension (Supuran, 2021). Other novel applications are also intensely studied and may soon lead to relevant developments for inflammation, cerebral ischemia, and cognition disturbances, among others.
Data availability statement
The original contributions presented in the study are included in the article/supplementary material; further inquiries can be directed to the corresponding author.
Author contributions
CS: Conceptualization and writing–original draft.
Funding
The author(s) declare that no financial support was received for the research, authorship, and/or publication of this article.
Conflict of interest
The authors declare that the research was conducted in the absence of any commercial or financial relationships that could be construed as a potential conflict of interest.
Publisher’s note
All claims expressed in this article are solely those of the authors and do not necessarily represent those of their affiliated organizations, or those of the publisher, the editors, and the reviewers. Any product that may be evaluated in this article, or claim that may be made by its manufacturer, is not guaranteed or endorsed by the publisher.
References
Abuaita, B. H., and Withey, J. H. (2009). Bicarbonate Induces Vibrio cholerae virulence gene expression by enhancing ToxT activity. Infect. Immun. 77 (9), 4111–4120. doi:10.1128/IAI.00409-09
Aggarwal, M., Chua, T. K., Pinard, M. A., Szebenyi, D. M., and McKenna, R. (2015). Carbon dioxide "trapped" in a β-carbonic anhydrase. Biochemistry 54 (43), 6631–6638. doi:10.1021/acs.biochem.5b00987
Alber, B. E., and Ferry, J. G. (1994). A carbonic anhydrase from the archaeon Methanosarcina thermophila. Proc. Natl. Acad. Sci. U. S. A. 91 (15), 6909–6913. doi:10.1073/pnas.91.15.6909
Arechederra, R. L., Waheed, A., Sly, W. S., Supuran, C. T., and Minteer, S. D. (2013). Effect of sulfonamides as carbonic anhydrase VA and VB inhibitors on mitochondrial metabolic energy conversion. Bioorg Med. Chem. 21 (6), 1544–1548. doi:10.1016/j.bmc.2012.06.053
Bahn, Y. S., Cox, G. M., Perfect, J. R., and Heitman, J. (2005). Carbonic anhydrase and CO2 sensing during Cryptococcus neoformans growth, differentiation, and virulence. Curr. Biol. 15, 2013–2020. doi:10.1016/j.cub.2005.09.047
Capasso, C., and Supuran, C. T. (2015). An overview of the alpha-beta- and gamma-carbonic anhydrases from Bacteria: can bacterial carbonic anhydrases shed new light on evolution of bacteria? J. Enzyme Inhib. Med. Chem. 30, 325–332. doi:10.3109/14756366.2014.910202
Chandrashekar, J., Yarmolinsky, D., von Buchholtz, L., Oka, Y., Sly, W., Ryba, N. J., et al. (2009). The taste of carbonation. Science 326 (5951), 443–445. doi:10.1126/science.1174601
Cottier, F., Leewattanapasuk, W., Kemp, L. R., Murphy, M., Supuran, C. T., Kurzai, O., et al. (2013). Carbonic anhydrase regulation and CO(2) sensing in the fungal pathogen Candida glabrata involves a novel Rca1p ortholog. Bioorg Med. Chem. 21 (6), 1549–1554. doi:10.1016/j.bmc.2012.05.053
Cox, E. H., McLendon, G. L., Morel, F. M., Lane, T. W., Prince, R. C., Pickering, I. J., et al. (2000). The active site structure of Thalassiosira weissflogii carbonic anhydrase 1. Biochemistry 39, 12128–12130. doi:10.1021/bi001416s
Cummins, E. P., Selfridge, A. C., Sporn, P. H., Sznajder, J. I., and Taylor, C. T. (2014). Carbon dioxide-sensing in organisms and its implications for human disease. Cell Mol. Life Sci. 71 (5), 831–845. doi:10.1007/s00018-013-1470-6
Del Prete, S., De Luca, V., Capasso, C., Supuran, C. T., and Carginale, V. (2016). Recombinant thermoactive phosphoenolpyruvate carboxylase (PEPC) from Thermosynechococcus elongatus and its coupling with mesophilic/thermophilic bacterial carbonic anhydrases (CAs) for the conversion of CO2 to oxaloacetate. Bioorg Med. Chem. 24 (2), 220–225. doi:10.1016/j.bmc.2015.12.005
Del Prete, S., Merlo, R., Valenti, A., Mattossovich, R., Rossi, M., Carginale, V., et al. (2019). Thermostability enhancement of the α-carbonic anhydrase from Sulfurihydrogenibium yellowstonense by using the anchoring-and-self-labelling-protein-tag system (ASLtag). J. Enzyme Inhib. Med. Chem. 34 (1), 946–954. doi:10.1080/14756366.2019.1605991
Del Prete, S., Vullo, D., Fisher, G. M., Andrews, K. T., Poulsen, S. A., Capasso, C., et al. (2014). Discovery of a new family of carbonic anhydrases in the malaria pathogen Plasmodium falciparum--the η-carbonic anhydrases. Bioorg Med. Chem. Lett. 24 (18), 4389–4396. doi:10.1016/j.bmcl.2014.08.015
Domsic, J. F., Avvaru, B. S., Kim, C. U., Gruner, S. M., Agbandje-McKenna, M., Silverman, D. N., et al. (2008). Entrapment of carbon dioxide in the active site of carbonic anhydrase II. J. Biol. Chem. 283 (45), 30766–30771. doi:10.1074/jbc.M805353200
Ferry, J. G. (2010). The gamma class of carbonic anhydrases. Biochim. Biophys. Acta 1804 (2), 374–381. doi:10.1016/j.bbapap.2009.08.026
Findinier, J., and Grossman, A. R. (2023). One step further toward a crop CO2-concentrating mechanism. J. Exp. Bot. 74 (12), 3402–3405. doi:10.1093/jxb/erad200
Förster, B., Rourke, L. M., Weerasooriya, H. N., Pabuayon, I. C. M., Rolland, V., Au, E. K., et al. (2023). The Chlamydomonas reinhardtii chloroplast envelope protein LCIA transports bicarbonate in planta. J. Exp. Bot. 74 (12), 3651–3666. doi:10.1093/jxb/erad116
He, Y., Duan, W., Xue, B., Cong, X., Sun, P., Hou, X., et al. (2023). OsαCA1 affects photosynthesis, yield potential, and water use efficiency in rice. Int. J. Mol. Sci. 24 (6), 5560. doi:10.3390/ijms24065560
Hirakawa, Y., Senda, M., Fukuda, K., Yu, H. Y., Ishida, M., Taira, M., et al. (2021). Characterization of a novel type of carbonic anhydrase that acts without metal cofactors. BMC Biol. 19 (1), 105. doi:10.1186/s12915-021-01039-8
Huili, Z., Li, K., and Nguyen, K. V. (2023). Climate change: approach to intervention using expression vector for carbonic anhydrase via glycosylphosphatidylinositol. Nucleosides Nucleotides Nucleic Acids, 1–12. doi:10.1080/15257770.2023.2238781
Jensen, E. L., Clement, R., Kosta, A., Maberly, S. C., and Gontero, B. (2019). A new widespread subclass of carbonic anhydrase in marine phytoplankton. ISME J. 13 (8), 2094–2106. doi:10.1038/s41396-019-0426-8
Jin, S., Vullo, D., Bua, S., Nocentini, A., Supuran, C. T., and Gao, Y. G. (2020). Structural and biochemical characterization of novel carbonic anhydrases from Phaeodactylum tricornutum. Acta Crystallogr. D. Struct. Biol. 76, 676–686. doi:10.1107/S2059798320007202
Kikutani, S., Nakajima, K., Nagasato, C., Tsuji, Y., Miyatake, A., and Matsuda, Y. (2016). Thylakoid luminal theta-carbonic anhydrase critical for growth and photosynthesis in the marine diatom Phaeodactylum tricornutum. Proc. Natl. Acad. Sci. U. S. A. 113, 9828–9833. doi:10.1073/pnas.1603112113
Krebs, H. A., and Roughton, F. J. (1948). Carbonic anhydrase as a tool in studying the mechanism of reactions involving H2CO3, CO2 or HCO3-. Biochem. J. 43, 550–555. doi:10.1042/bj0430550
Kunert, E., Joyce, W., Pan, Y. K., Chen, A., Perry, S. F., and Gilmour, K. M. (2022). Role of cytosolic carbonic anhydrase Ca17a in cardiorespiratory responses to CO2 in developing zebrafish (Danio rerio). Am. J. Physiol. Regul. Integr. Comp. Physiol. 323 (4), R532–R546. doi:10.1152/ajpregu.00050.2022
Liao, L., Chen, B., Deng, K., He, Q., Lin, G., Guo, J., et al. (2023). Effect of the N-hexanoyl-L-homoserine lactone on the carbon fixation capacity of the algae-bacteria system. Int. J. Environ. Res. Public Health 20 (6), 5047. doi:10.3390/ijerph20065047
Lindskog, S. (1997). Structure and mechanism of carbonic anhydrase. Pharmacol. Ther. 74 (1), 1–20. doi:10.1016/s0163-7258(96)00198-2
Meldrum, N. U., and Roughton, F. J. (1933). Carbonic anhydrase. Its preparation and properties. J. Physiol. 80, 113–142. doi:10.1113/jphysiol.1933.sp003077
Migliardini, F., De Luca, V., Carginale, V., Rossi, M., Corbo, P., Supuran, C. T., et al. (2014). Biomimetic CO2 capture using a highly thermostable bacterial α-carbonic anhydrase immobilized on a polyurethane foam. J. Enzyme Inhib. Med. Chem. 29 (1), 146–150. doi:10.3109/14756366.2012.761608
Momayyezi, M., McKown, A. D., Bell, S. C. S., and Guy, R. D. (2020). Emerging roles for carbonic anhydrase in mesophyll conductance and photosynthesis. Plant J. 101 (4), 831–844. doi:10.1111/tpj.14638
Neri, D., and Supuran, C. T. (2011). Interfering with pH regulation in tumours as a therapeutic strategy. Nat. Rev. Drug Discov. 10 (10), 767–777. doi:10.1038/nrd3554
Nocentini, A., Supuran, C. T., and Capasso, C. (2021). An overview on the recently discovered iota-carbonic anhydrases. J. Enzyme Inhib. Med. Chem. 36 (1), 1988–1995. doi:10.1080/14756366.2021.1972995
Occhipinti, R., and Boron, W. F. (2019). Role of carbonic anhydrases and inhibitors in acid-base physiology: insights from mathematical modeling. Int. J. Mol. Sci. 20 (15), 3841. doi:10.3390/ijms20153841
Rummer, J. L., McKenzie, D. J., Innocenti, A., Supuran, C. T., and Brauner, C. J. (2013). Root effect hemoglobin may have evolved to enhance general tissue oxygen delivery. Science 340 (6138), 1327–1329. doi:10.1126/science.1233692
Santhanagopalan, I., Wong, R., Mathur, T., and Griffiths, H. (2021). Orchestral manoeuvres in the light: crosstalk needed for regulation of the Chlamydomonas carbon concentration mechanism. J. Exp. Bot. 72 (13), 4604–4624. doi:10.1093/jxb/erab169
Santi, A., Caselli, A., Paoli, P., Corti, D., Camici, G., Pieraccini, G., et al. (2013). The effects of CA IX catalysis products within tumor microenvironment. Cell Commun. Signal 11, 81. doi:10.1186/1478-811X-11-81
Shimakawa, G., Okuyama, A., Harada, H., Nakagaito, S., Toyoshima, Y., Nagata, K., et al. (2023). Pyrenoid-core CO2-evolving machinery is essential for diatom photosynthesis in elevated CO2. Plant Physiol., kiad475. doi:10.1093/plphys/kiad475
Smith, K. S., Jakubzick, C., Whittam, T. S., and Ferry, J. G. (1999). Carbonic anhydrase is an ancient enzyme widespread in prokaryotes. Proc. Natl. Acad. Sci. U. S. A. 96 (26), 15184–15189. doi:10.1073/pnas.96.26.15184
Steiner, H., Jonsson, B. H., and Lindskog, S. (1975). The catalytic mechanism of carbonic anhydrase. Hydrogen-isotope effects on the kinetic parameters of the human C isoenzyme. Eur. J. Biochem. 59, 253–259. doi:10.1111/j.1432-1033.1975.tb02449.x
Strowitzki, M. J., Nelson, R., Garcia, M. P., Tuffs, C., Bleul, M. B., Fitzsimons, S., et al. (2022). Carbon dioxide sensing by immune cells occurs through carbonic anhydrase 2-dependent changes in intracellular pH. J. Immunol. 208 (10), 2363–2375. doi:10.4049/jimmunol.2100665
Supuran, C. T. (2008). Carbonic anhydrases: novel therapeutic applications for inhibitors and activators. Nat. Rev. Drug Discov. 7 (2), 168–181. doi:10.1038/nrd2467
Supuran, C. T. (2016). Structure and function of carbonic anhydrases. Biochem. J. 473, 2023–2032. doi:10.1042/BCJ20160115
Supuran, C. T. (2018). Carbonic anhydrases and metabolism. Metabolites 8 (2), 25. doi:10.3390/metabo8020025
Supuran, C. T. (2021). Emerging role of carbonic anhydrase inhibitors. Clin. Sci. (Lond). 135 (10), 1233–1249. doi:10.1042/cs20210040
Supuran, C. T. (2022). Anti-obesity carbonic anhydrase inhibitors: challenges and opportunities. J. Enzyme Inhib. Med. Chem. 37 (1), 2478–2488. doi:10.1080/14756366.2022.2121393
Supuran, C. T. (2023a). A simple yet multifaceted 90 years old, evergreen enzyme: carbonic anhydrase, its inhibition and activation. Bioorg Med. Chem. Lett. 93:129411, doi:10.1016/j.bmcl.2023.129411
Supuran, C. T. (2023b). An overview of novel antimicrobial carbonic anhydrase inhibitors. Expert Opin. Ther. Targets, 1–14. (in press). doi:10.1080/14728222.2023.2263914
Supuran, C. T., and Capasso, C. (2021). A highlight on the inhibition of fungal carbonic anhydrases as drug targets for the antifungal armamentarium. Int. J. Mol. Sci. 22 (9), 4324. doi:10.3390/ijms22094324
Talekar, S., Jo, B. H., Dordick, J. S., and Kim, J. (2022). Carbonic anhydrase for CO2 capture, conversion and utilization. Curr. Opin. Biotechnol. 74, 230–240. doi:10.1016/j.copbio.2021.12.003
Tu, C., Silverman, D. N., Forsman, C., Jonsson, B. H., and Lindskog, S. (1989). Role of histidine 64 in the catalytic mechanism of human carbonic anhydrase II studied with a site-specific mutant. Biochemistry 28, 7913–7918. doi:10.1021/bi00445a054
Ueda, K., Nishida, H., and Beppu, T. (2012). Dispensabilities of carbonic anhydrase in proteobacteria. Int. J. Evol. Biol. 2012, 324549. doi:10.1155/2012/324549
Villa, R., Nieto, S., Donaire, A., and Lozano, P. (2023). Direct biocatalytic processes for CO2 capture as a green tool to produce value-added chemicals. Molecules 28 (14), 5520. doi:10.3390/molecules28145520
Xu, Y., Feng, L., Jeffrey, P. D., Shi, Y., and Morel, F. M. (2008). Structure and metal exchange in the cadmium carbonic anhydrase of marine diatoms. Nature 452, 56–61. doi:10.1038/nature06636
Keywords: carbonic anhydrase, pH regulation, CO2/bicarbonate sensing, metabolism, inhibitor
Citation: Supuran CT (2023) Carbonic anhydrase versatility: from pH regulation to CO2 sensing and metabolism. Front. Mol. Biosci. 10:1326633. doi: 10.3389/fmolb.2023.1326633
Received: 23 October 2023; Accepted: 26 October 2023;
Published: 10 November 2023.
Edited by:
Andrea Mozzarelli, University of Parma, ItalyReviewed by:
René Csuk, Martin Luther University of Halle-Wittenberg, GermanyCopyright © 2023 Supuran. This is an open-access article distributed under the terms of the Creative Commons Attribution License (CC BY). The use, distribution or reproduction in other forums is permitted, provided the original author(s) and the copyright owner(s) are credited and that the original publication in this journal is cited, in accordance with accepted academic practice. No use, distribution or reproduction is permitted which does not comply with these terms.
*Correspondence: Claudiu T. Supuran, Y2xhdWRpdS5zdXB1cmFuQHVuaWZpLml0