- 1Grupo de Inmunobiología y Biología Celular, Facultad de Ciencias, Pontificia Universidad Javeriana, Bogotá, Colombia
- 2Versiti Blood Research Institute, Milwaukee, WI, United States
Leukemic cells acquire complex and often multifactorial mechanisms of resistance to treatment, including various metabolic alterations. Although the use of metabolic modulators has been proposed for several decades, their use in clinical practice has not been established. Natural products, the so-called botanical drugs, are capable of regulating tumor metabolism, particularly in hematopoietic tumors, which could partly explain the biological activity attributed to them for a long time. This review addresses the most recent findings relating to metabolic reprogramming—Mainly in the glycolytic pathway and mitochondrial activity—Of leukemic cells and its role in the generation of resistance to conventional treatments, the modulation of the tumor microenvironment, and the evasion of immune response. In turn, it describes how the modulation of metabolism by plant-derived extracts can counteract resistance to chemotherapy in this tumor model and contribute to the activation of the antitumor immune system.
1 Introduction
Acute leukemias (ALs) are a group of malignant diseases of the hematopoietic system characterized by disordered proliferation and clonal expansion of immature precursors, resulting in bone marrow failure with blockage in cell differentiation processes (Swerdlow et al., 2017). ALs are classified, considering their morphological, immunophenotypic, cytogenetic, and molecular characteristics, into two large groups: acute myeloid leukemia (AML) and acute lymphoblastic leukemia (ALL). AML is characterized by the accumulation and blocked differentiation of progenitors of myeloid, monocytic, erythroid, or megakaryocytic lineage, and ALL instead arises from the transformation of B-cell (B-ALL) or T-cell progenitors (T-ALL) (Arber et al., 2016).
Although the use of various chemotherapy schemes for the treatment of ALs has achieved a high percentage of survival in developed countries, in Colombia its implementation has shown discouraging results, with complete remission rates of 45% in AML and 61% in ALL in the adult population (Combariza et al., 2007; Ballesteros-Ramírez et al., 2020a; Sossa et al., 2021). Treatment options for patients who do not achieve a complete remission are limited, as even with salvage therapy followed by allogeneic stem cell transplantation (HSCT), outcomes remain poor (Thol, 2018). For four decades, the mortality rates of patients with ALs have been the highest when compared to the rates of other groups of leukemias (Shallis et al., 2019).
The lack of response to current chemotherapeutics has been attributed to different resistance mechanisms, among them: 1) the acquisition of new mutations or genetic alterations (clonal evolution) (Hackl et al., 2017); 2) the presence of leukemia-initiating cells (LICs) remaining after chemotherapy (Yeung and Radich, 2017); 3) the autophagy as a cooperative mechanism for the stability of oncoproteins or as a cytoprotective against the cytotoxic effects induced by drugs (Auberger and Puissant, 2017); 4) the overexpression of ATP-binding cassette transporters (ABC transporters) that allow drug efflux (Marin et al., 2016); 5) the microbiota affecting the metabolism, toxicity, and efficacy of certain drugs (Wilkinson et al., 2018); 6) inherent patient factors such as body mass index and age (Li et al., 2017), 7) the microenvironment through soluble factors or direct interaction between leukemic blasts and mesenchymal or stromal cells (Shafat et al., 2017); and 8) which has recently been recognized as one of the hallmarks of cancer, can also contribute to the evasion of the antitumor immune response (Desbats et al., 2020).
Reprogrammed metabolic activities of tumor cells that support survival, maintenance, and drug response are related to altered bioenergetic pathways, enhanced macromolecule biosynthesis, and maintenance of the redox balance (DeBerardinis and Chandel, 2016; Vander Heiden and Deberardinis, 2017). The addition of therapies that modulate tumor metabolism to conventional chemotherapy has been emerging, (Luppi et al., 2018; Winer and Stone, 2019), and more therapeutic strategies are needed that achieve tumor metabolic modulation that can lead to the activation of the immune system and an accentuated anti-neoplastic effect. Then, our group focused on seeking anti-leukemic therapies based on plant-derived extracts that serve as the basis for the preparation of botanical drugs or phytomedicines (Urueña et al., 2008; Castañeda et al., 2012; Sandoval et al., 2016; Ballesteros-Ramírez et al., 2020b). The use of plant-derived extracts, due to their multiple active components, offers a multi-target mechanism of action, unlike many current therapies that follow the classic “one drug, one target” pharmacological dogma and have fewer toxic side effects, so they are more tolerable (Herranz-López et al., 2018). In addition, its main components can be combined with conventional chemotherapy to reduce the development of chemoresistance through the modulation of metabolism (Kumar and Jaitak, 2019).
In this review, we describe the intrinsic and extrinsic mechanisms that have been associated with alterations in glycolytic metabolism and mitochondrial function in chemoresistant leukemic cells and how natural products could reverse resistance with a metabolic and immunomodulatory approach.
2 Reprogramming glycolytic metabolism induces chemoresistance in ALs
The final product of glucose oxidation can be lactate or carbon dioxide (CO2) (via mitochondrial respiration). Tumor cells, regardless of oxygen availability, increase glucose consumption and produce large amounts of lactate, which is known as the Warburg effect (Icard et al., 2018; Liu et al., 2021; Marcucci and Cristiano, 2021). The increase in the glycolytic pathway is advantageous for tumor cells because: first, it promotes uncontrolled proliferation due to biomass generation; second, it is a fast way to produce adenosine triphosphate (ATP), even more than oxidative phosphorylation (OxPhos); and third, it prevents damage from oxidative stress, directly by reducing mitochondrial respiration and therefore the generation of reactive oxygen species (ROS), and indirectly by the production of nicotinamide adenine reduced dinucleotide phosphate (NADPH) through the pentose pathway (PPP), which maintains glutathione (GSH) in a reduced state, the main non-protein thiol that acts as an intracellular redox regulator (Marcucci and Cristiano, 2021). Some intrinsic and extrinsic factors related to increased glycolysis described below are associated with chemoresistance mechanisms in various tumor models, including ALs (Supplementary Table S1).
2.1 ALL and AML cells resistant to chemotherapy and increase glycolysis
Most of the studies in cell lines and primary samples derived from myeloid and lymphoid leukemias demonstrate a relationship between the increase in the glycolytic pathway and resistance to different types of drugs, such as anthracyclines (daunorubicin, -DNR-, doxorubicin, -DOX-, and idarubicin, -IDA-), some glucocorticoids such as prednisolone, and tyrosine kinase inhibitors (TKIs) such as imatinib, among others.
In DNR-resistant cell lines such as HL60/DNR and CEM/R2, of myeloid and T-lymphoid origin, respectively, a higher glucose demand has been described together with a lower glutamine dependence and a lower rate of fatty acid oxidation compared with their non-resistant counterparts (Stäubert et al., 2015). Similarly, myeloid cell lines (K562-r and LAMA84-r) with aberrant expression of Breakpoint Cluster Region-Breakpoints in the Abelson (ABL)1 fusion (BCR-ABL1) and resistance to imatinib maintain a highly glycolytic metabolic phenotype with elevated lactate production (Kominsky et al., 2009). On the other hand, the TEX cell line, which mimics the characteristics of AML and LICs, is resistant to tigecycline (an antibiotic that induces an antitumor effect by inhibiting the translation of mitochondrial proteins) (Xu et al., 2016), and it has an increased glycolytic rate (Jhas et al., 2013). Importantly, in some B-ALL and T-ALL cell lines and cells derived from patients with B-ALL, reduction of the glycolytic rate using 2-deoxy-D-glucose (2-DG), lonidamine (LND), or 3-bromopyruvate (3-BrPA), can restore the sensitivity of these cells to glucocorticoids (Hulleman et al., 2009; Gu et al., 2017); and the combined treatment of a glycolytic inhibitor in conjunction with the silencing of anti-apoptotic proteins such as myeloid cell leukemia sequence 1 protein (MCL-1) can enhance the antitumor activity in cells resistant to prednisolone (Ariës et al., 2013).
In contrast to the above, a single publication by Herst et al. (2011) using primary AML cells describes that patients with highly glycolytic blasts had higher overall survival rates and longer rates of first complete remission compared to patients who had blasts with moderate glycolytic rate. Likewise, leukemic blasts that had a high glycolytic level were more susceptible to the anti-tumor activity of all-trans retinoic acid (ATRA) and arsenic trioxide (ATO). These data show us that tumor cells require homeostatic mechanisms to avoid scenarios of accumulation or depletion of secondary metabolites that can be toxic and that an excessive glycolytic flux can represent an unfavorable event for their survival and adaptation to different stress conditions. However, the direct relationship between the increase in glycolysis and chemoresistance in leukemic cells must be studied in depth at the mechanistic level.
2.2 Regulation of increased glycolytic flux: intrinsic mechanistic factors
Different intrinsic factors have been established that influence an improvement in the glycolytic pathway in chemoresistant leukemia cells (Figure 1). Genetic alterations that involve mutations in oncogenes and/or tumor suppressor genes lead to deregulated signaling pathways and consequently to the aberrant expression of transcription factors, enzymes, transporters, proteins (nuclear and cytoplasmic), and regulatory molecules of glucose metabolism (Lo Presti et al., 2020; Di Martino et al., 2021).
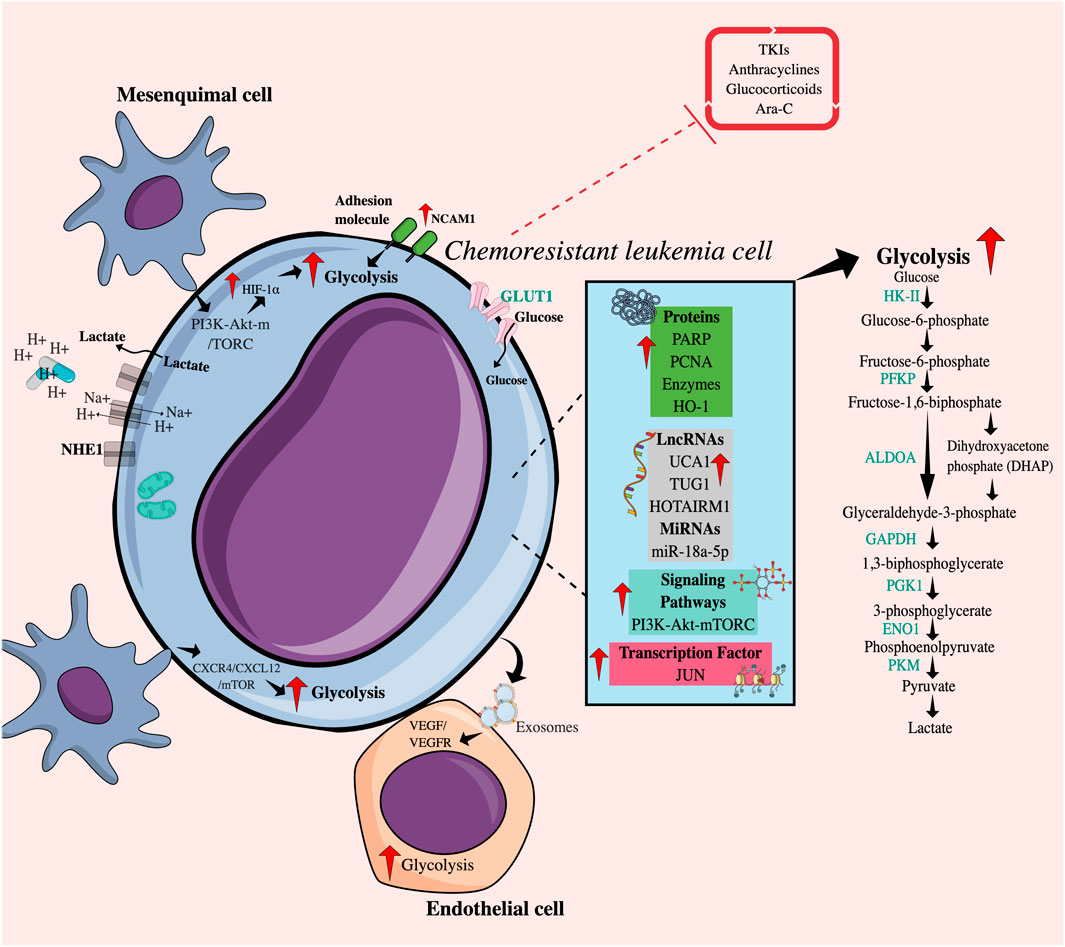
FIGURE 1. Intrinsic and extrinsic mechanisms related to increased glycolytic flux in chemoresistant leukemia cells. Intrinsically, chemoresistant leukemia cells overexpress cytoplasmic proteins, enzymes, RNA molecules, transcription factors, and receptors and present alterations in signaling pathways that favor an increase in glycolytic flow. Additionally, the direct or indirect interaction between chemoresistant leukemia cells and various cell populations of the tumor microenvironment, such as mesenchymal and endothelial cells, promotes glucose uptake achieved by tumor cells through the PI3K-mTORC pathway or the CXCR4/CXCL12 axis. The release of exosomes containing VEGF-RVEFG can also increase glycolysis in endothelial cells. Taken together, all these factors may regulate resistance to drugs including TKIs, anthracyclines, Ara-C, and glucocorticoids. TKIs, Tyrosine kinase inhibitors; Ara-C, Cytarabine; HIF-1, Hypoxia-induced factor; GLUT1, Glucose transporter 1; NCAM1, Neural cell adhesion molecule 1; NHE1, Na (+)/H (+) antiporter; HK-II, Hexokinase II; PFKP, Phosphofructokinase; GPI, Glucose phosphate isomerase; ALDOA, Aldolase A; GAPDH, Glyceraldehyde-3-phosphate dehydrogenase; PGK1, Phosphoglycerate kinase; ENO1, Enolase 1; LDHA, Lactate dehydrogenase A; PKM, Pyruvate kinase 2; HO-1, Heme oxygenase-1; PARP, Poly (ADP-ribose) polymerases; PCNA, Proliferating cell nuclear antigen; LncRNAs, Long non-coding RNAs; microRNAs, Short non-coding RNAs; UCA1, Associated with urothelial carcinoma; TUG1, Taurine upregulated gene 1; HOTAIRM1, Myeloid-specific antisense intergenic RNA HOX transcript 1.
The phosphoinositide 3-kinase-Akt-mammalian target of rapamycin (PI3K-Akt-mTOR) signaling pathway, commonly activated in cancer, including AML, contributes to the maintenance of glycolysis by translational and post-translational regulation of metabolic enzymes (Hoxhaj and Manning, 2020; Nepstad et al., 2020). Ryu et al. (2019) established that AKT activation by phosphatidylinositol 3,4,5-trisphosphate 3-phosphatase and dual-specificity protein phosphatase (PTEN) deficiency, a direct PI3K antagonist, maintained a refractory state of cells to treatment with cytarabine (Ara-C) and IDA through the improvement of glycolysis in the myeloid lines HL60 and KG1α.
A master transcription factor in the regulation of glycolysis is hypoxia-inducible factor 1 α (HIF-1α) because it promotes the transcription of glucose transporters, such as glucose transporter 1 (GLUT1), and glycolytic enzymes, such as hexokinase II (HK-II), and pyruvate kinase M2 (PKM2) (Yu et al., 2017). An increase in the expression levels of GLUT1, HIF1-α, and HK-II mRNA has been reported in primary AML cells from non-responders and HL60/DNR cells (Jhas et al., 2013; Song et al., 2014; Song et al., 2016). Also, recently, Valin et al. (2022) reported JUN, an oncogenic transcription factor, as a regulator of glycolytic metabolism in AML, since it increases the expression of hexokinase I (HKI) and HKII, glucose phosphate isomerase (GPI), phosphofructo-1-kinase (PFKP), aldolase A (ALDOA), glyceraldehyde-3-phosphate dehydrogenase (GAPDH), phosphoglycerate kinase (PGK1), enolase 1 (ENO1), and PKM. Similarly, in breast cancer, the c-JUN family of proteins has been associated with resistance to cisplatin (Xia et al., 2013).
Another molecule that promotes glycolysis is the proliferating cell nuclear antigen (PCNA), which is a nuclear protein synthesized in the early G1 phase and in the S phase of the cell cycle and is considered a marker of the proliferation index in some neoplasms (Cardano et al., 2020). It has been shown that the interaction between PCNA and nicotinamide phosphoribosyl transferase (NAMPT), an enzyme that participates in the rescue pathway for the generation of the nicotinamide adenine dinucleotide (NAD+) cofactor, can coordinate the increase in glycolysis, favoring the survival of DNR-resistant HL60 cells (Ohayon et al., 2016).
On the other hand, the enzyme heme oxygenase-1 (HO-1) catalyzes the degradation of the heme group, causing carbon monoxide (CO), biliverdin, and iron, where the heme group is a critical component of multiple hemoproteins involved in glucose metabolism and of lipids and proteins. Interestingly, elevated levels of HO-1 have been described in primary myeloid cells and HL60 cells resistant to Ara-C and DNR. HO-1 inhibition abrogates the expression of HIF-1α, and GLUT1, improving the sensitivity to the two drugs, apparently by decreasing the glycolysis (Zhe et al., 2015). Furthermore, HO-1 is involved in the generation of a resistant profile in patients with myelodysplastic syndrome (MDS) who can progress to AML (He et al., 2019). Also, the increased expression of the adhesion protein NCAM1 (Neural Cell Adhesion Molecule 1) in K562 BCR-ABL+ myeloid cells increases the IC50 for dasatinib (Sasca et al., 2019). Knockdown of NCAM1 decreases the expression of genes involved in glucose metabolism, and the reduction in their expression sensitizes NOMO shNCAM_3 leukemic cells to treatment with Ara-C in a murine NSG (NOD scid gamma mouse) model.
Poly (ADP-ribose) polymerases (PARP) family of polymerases is made up of proteins involved in many cellular processes, including DNA repair and apoptosis. Particularly, PARP14 can promote glycolysis in different tumor models, such as human hepatocellular carcinoma (HCC), by maintaining reduced PKM2 activity (Iansante et al., 2015); on the other hand, a close relationship between c-myc and AKT has been reported in B lymphomas (Cho et al., 2011) and AML through the NF-κB/HIF-1α axis (Zhu et al., 2022). Interestingly, the use of Niraparib, a PARP1/2 inhibitor, decreases resistance to ATO and hypomethylating agents (azacytidine and decitabine) in tumor promyelocytes (Giansanti et al., 2021).
Deregulation of LncRNAs and microRNAs (long and short non-coding RNAs, respectively), considered epigenetic regulators, contributes to therapeutic resistance through the regulation of energy metabolism (Agbu and Carthew, 2021; Taghvimi et al., 2022). The LncRNA associated with urothelial carcinoma (UCA1) is overexpressed in AML cells from patients who did not respond to DOX treatment and in the HL60/DNR cell line (Liu et al., 2014). UCA1 promotes glycolysis by inhibiting the action of mRNA125a, which usually functions as a tumor suppressor but also appears to be involved in blocking HK-II (Sun et al., 2017), which in turn could participate in the stabilization of HIF1-α (Zhang et al., 2018). Also, thanks to its oncogenic role, the increase in the expression of the LncRNA TUG1 (taurine-upregulated gene 1) is accompanied by the increase in the expression of the mRNAs of HK-II and PKM2 in HL60 cells resistant to doxorubicin (HL60/DOX) (Chen L. et al., 2019a). Another LncRNA, HOTAIRM1 (HOX transcript antisense intergenic RNA myeloid-specific 1), involved in myeloid lineage maturation and overexpressed in AML, has been compromised by resistance to Ara-C. Its deletion has been reported to improve drug activity and decrease glucose consumption and therefore lactate production, mainly mediated by the reduction of PFKP in HL60/WT and THP-1/WT cells (Chen et al., 2020).
Particularly in K562 cells, the expression level of miR-18a-5p in DOX-resistant cells is lower compared to K562 cells and normal lymphocytes, and strikingly, miR-18a-5p can inhibit the expression of HIF-1α and, in turn, induce a reduction in the production of pyruvate, ATP and affect the expression of GLUT1, HK-II, PKM2, and lactate dehydrogenase A (LDHA) (Wu et al., 2021).
2.3 Regulation of increased glycolytic flux: extrinsic mechanistic factors
In the bone marrow microenvironment, there are cellular and non-cellular components that influence the metabolic reprogramming of leukemia cells and can be considered external factors (Jiang and Nakada, 2016). Contact between mesenchymal cells (MSC) and/or endothelial cells (EC) and leukemic cells improves tumor survival in the presence of different chemotherapeutic agents (Boutin et al., 2020; Okamoto et al., 2022). Culturing primary B-ALL cells or Reh lymphoid cell line with bone marrow-derived MSCs under hypoxic conditions induces a higher expression of HIF-1α and therefore an acquisition of the glycolytic phenotype of leukemic cells, which in part is due to signaling-regulated by the AKT/mTOR pathway induced by stromal cells. Inhibition of mTOR using everolimus, an antiproliferative agent, reduces the expression of HIF-1α, decreases the glycolytic rate, and partially restores the sensitivity of ALL cells to vincristine under co-culture and hypoxic conditions (Frolova et al., 2012). Also, the interaction with stromal cells increases the glycolytic flux of AML cells from patients through the CXCR4/CXCL12/mTOR axis (Braun et al., 2016), and the use of selective inhibitors of this axis represents an opportunity to block MSC-mediated chemoresistance (Braun et al., 2016). In AML, leukemic cells have been reported to secrete VEGF/VEGFR-containing exosomes that induce glycolysis in endothelial cells -HUVECs-, leading to vascular remodeling and the acquisition of chemoresistance (Wang et al., 2019).
On the other hand, the Warburg effect implies an increase in the production of lactic acid and, therefore, an acidic microenvironment. Tumor cells express several families of pH regulators in the plasma membrane to protect themselves, such as NHE1 (Sodium-hydrogen antiporter 1), which exports H+ and contributes to the decrease in extracellular pH. Acidification in the microenvironment plays an immunosuppressive role and mediates the protonation of drugs, affecting their cell permeability (Altaf et al., 2017; de la Cruz-López et al., 2019). In T-ALL, increased NHE1 activity may promote resistance to DOX and imatinib (Man et al., 2014).
2.4 Association between increased glycolysis with clinical response and survival rates in ALs
Despite the strong evidence that glycolytic metabolism can promote chemoresistance in primary leukemic cells from non-responders and/or multiresistant cell lines, recent advances in metabolomics platforms have allowed further investigation into tumor metabolism at the systemic level in patients with acute leukemia (Grønningsæter et al., 2019; Kim et al., 2021), however, only a few studies have focused on the impact of glycolytic alterations on response to chemotherapy treatment, with mixed results. An increase in circulating serum glucose (α-glucose and β-glucose) in patients with different subtypes of AML—Including leukemias with monocytic differentiation characterized by having a poor prognosis—In comparison with healthy controls has been reported (Duan et al., 2022). In contrast, in plasma samples of bone marrow from pediatric ALL patients with positive minimal residual disease (MRD), a decrease in glucose and a higher concentration of other metabolites related to glycolysis, the PPP pathway, and the tricarboxylic acid (TCA) cycle were observed (Schraw et al., 2019). These results demonstrate the functional compartmentalization of glucose and the ability of tumor cells to influence systemic glucose regulation (Ye et al., 2018). Likewise, Chen et al. (2014) managed to identify a serum metabolic signature related mainly to the glycolysis pathway, where the decrease in glycerol-3-phosphate (glycerol-3P) and lactate, together with the increase in 2-hydroxyglutarate (2-HG), 2-oxoglutarate, and pyruvate, would be negatively related to the overall survival of patients with AML with a normal karyotype; while decreased citrate levels would positively impact, evidencing the clinical utility of the identification of energetic metabolic biomarkers. However, taking into account that the global metabolome of a patient is a complex, specialized metabolic network at different levels (Schmidt et al., 2021) and that it is not only influenced by the glycolytic metabolism of tumor cells, but the focus of metabolism studies in cancer with clinical utility must also have a comprehensive vision.
3 Chemoresistance-induced mitochondrial function in ALs
Mitochondria allow metabolic adaptation to various stress conditions as they are an integrating center of important cellular processes that include energy production through OxPhos, redox signaling, anabolic and catabolic reactions, epigenetic regulation, cell self-renewal and differentiation, initiation, and run-time programmed cell death (PCD) (Basak and Banerjee, 2015; Guerra et al., 2017; Bokil and Sancho, 2019; Grasso et al., 2020); and particularly in leukemias, they have emerged as a determinant for the progression and development of cancer as well as the response to chemotherapeutics (Basak and Banerjee, 2015). Interestingly, several studies have shown that AML and B-ALL cells have increased respiratory activity, increased mitochondrial mass, and significantly higher mitochondrial DNA (mtDNA) content than normal CD34+ cells and peripheral blood mononuclear cells (PBMCs) (Panina et al., 2019; Jain et al., 2022). However, they show a lower energy reserve capacity and reduced activity of complexes III, IV, and V, which could explain why they are more sensitive to mitochondrial damage than normal cells or other tumor models, such as ovarian cancer (Panina et al., 2019). In addition, synergistic and selective cytotoxicity has been reported in AML cell lines by combining components that target mitochondria (mitocans) with glycolytic inhibitors, TKIs, or microtubule destabilizers (Panina et al., 2020). Added to the above, cells with low levels of ROS, within which LICs could be, are unable to use glucose when mitochondrial respiration is inhibited (Lagadinou et al., 2013). For all the above, mitochondrial function is essential for the survival of leukemic cells and is currently a highly explored therapeutic target.
3.1 Mitochondria as a promoter of chemoresistant AML and ALL cells
Various publications have described the role of mitochondrial activity in resistance or sensitivity to chemotherapy treatment in ALs. Most of the reports currently described show that resistant leukemic cells have enhanced mitochondrial activity, while other works suggest that impaired or stable mitochondrial activity could be related to resistance. The association between mitochondrial activity and low sensitivity to anthracyclines, Ara-C, and Venetoclax has been documented in cell lines and primary leukemia cells and confirmed using in vivo models (Farge et al., 2017) (Figure 2).
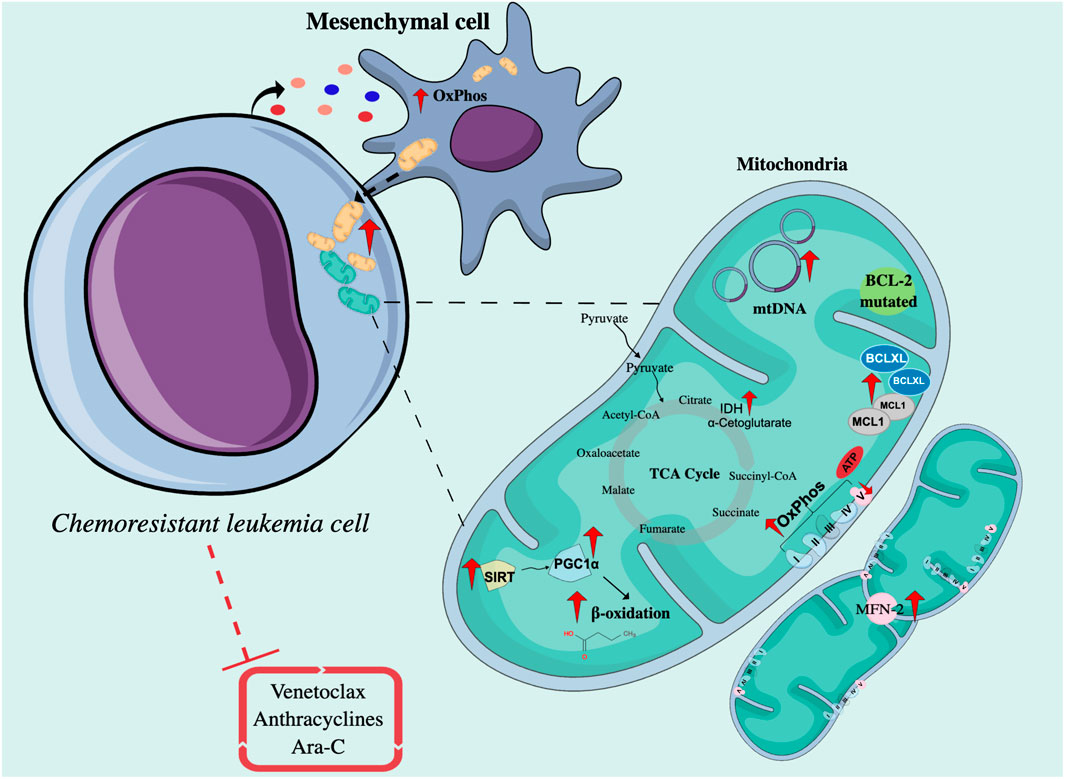
FIGURE 2. Intrinsic and extrinsic mechanisms related to mitochondrial function in chemoresistant leukemia cells. Chemoresistant leukemia cells exposed to drugs such as Venetoclax, Ara-C, and anthracyclines interact with mesenchymal cells through mitochondrial transfer to increase OxPhos and ATP production. On the other hand, the mitochondria of resistant cells are characterized by the upregulation of proteins involved in the regulation of apoptosis, mitochondrial biogenesis, and fatty acid metabolism, and present a higher number of mtDNA copies. OxPhos, Oxidative phosphorylation; Ara-C, Cytarabine; TCA cycle, Cycle of tricarboxylic acids; MFN-2, Mitofusin 2; SIRT, Sirtuins; PGC1α, PPARγ coactivator-1α; IDH, Isocitrate dehydrogenase; mtDNA, Mitochondrial DNA; BCL-2, B-Cell Leukemia/Lymphoma 2; Bax, Bcl-2-associated X protein; Bak, Bcl-2 homologous antagonist/killer; MCL1, Myeloid cell leukemia sequence 1 protein.
Regarding improved mitochondrial activity, AML cells persistent to treatment with Ara-C in an NSG mouse model presented a higher rate and a genetic signature associated with OxPhos, accompanied by an increase in mitochondrial mass, membrane potential, and ROS production (Farge et al., 2017). Through the use of metabolic sensors, in B-ALL, it was shown that cells resistant to Ara-C had greater mitochondrial respiration capacity (Chen et al., 2021). Several reports have shown that by inhibiting mitochondrial processes such as the oxidation of fatty acids, the activity of the electron transport chain (ETC), the replication of mtDNA, or the synthesis of mitochondrial proteins, the sensitivity to Ara-C increases in vitro and in vivo (Farge et al., 2017), and the proliferation of primary AML cells have slightly decreased (Vitkevičienė et al., 2019).
On the other hand, regarding stable or impaired mitochondrial activity, in Jurkat rho zero (ρ0) T lymphoid cells (cells without mitochondria), there is greater resistance to treatment with bleomycin, an antineoplastic antibiotic, compared to Jurkat cells with mitochondria (Yeung et al., 2015), reflecting a susceptibility to mitochondrial damage in these cells. And also, interestingly, Henkenius et al. (2017) reported that resistant HL60 and MV4-11 cells maintain stable mitochondrial activity after exposure to different concentrations of Ara-C and sorafenib, compared to sensitive HL60 cells, in which their mitochondrial activity is affected.
3.2 Intrinsic and extrinsic mechanistic factors of mitochondrial function mediating chemoresistance in ALs cells
There are also intrinsic and extrinsic factors that affect mitochondrial function and, therefore, mediate therapeutic sensitivity. Among the intrinsic factors are the sirtuins (SIRTs), a family of NAD+-dependent lysine deacetylases, some of which are exclusively expressed in mitochondria (SIRTs 3, 4, and 5) (Jaiswal et al., 2022). These enzymes mediate the response to cellular stress, inducing metabolic changes by regulating the activity of proteins such as PGC1α (PPARγ coactivator-1α) (Chalkiadaki and Guarente, 2015), which is a master in the regulation of mitochondrial metabolism and an activator of genes associated with the oxidation of fatty acids (Mattes et al., 2019). In AML, SIRT3 activity has been implicated in chemoresistance to Ara-C by regulating OxPhos in the myeloid cell line MV4-11 (Ma et al., 2019). In addition, it has been described that many AML patient samples are highly dependent on SIRT5. Genetic or pharmacological SIRT5 inhibition impairs in vitro transformation of mouse hematopoietic cells by several myeloid oncogenes, including MLL-AF9, and attenuates in vivo leukemogenesis. SIRT5 knockdown or pharmacological inhibition with NRD167 is associated with reduced OXPHOS, reduced GSH levels, and increased mitochondrial superoxide, suggesting that AML cells depend on SIRT5 to maintain redox homeostasis (Yan et al., 2021).
Another important enzyme in mitochondrial function is ATP synthase, a transmembrane enzyme that catalyzes the synthesis of ATP from ADP, a phosphate group, and the energy supplied by the flow of H+ supplied by the coenzymes NADH and reduced flavin adenine dinucleotide (FADH2). It is made up of two subunits: the Fo subunit, a component that crosses the inner mitochondrial membrane, and the F1 subunit, which protrudes into the mitochondrial matrix. The decrease in the expression of the ATP-F1-β subunit, probably due to epigenetic changes related to hypermethylation of the gene, has been reported in cells from refractory or relapsed AML patients and has been confirmed in DOX-resistant HL60 myeloid lines and DNR (Xiao et al., 2013; Song et al., 2016; Yang et al., 2016).
Mitochondrial fusion and fission are two opposite processes that go according to cellular metabolic requirements. Fusion allows mitochondria to connect with each other to form networks or fragments and is commonly associated with increased ATP production and protection against autophagy; on the contrary, fission allows mitochondria to constantly divide and is mainly related to apoptosis, facilitating the segregation of mtDNA in mitosis and eliminating defective mitochondria (Genovese et al., 2021). The survival of T-ALL cells after DOX treatment was correlated with the expression of Mitofusin-2 (MFN-2)—A GTPase protein that mediates mitochondrial fusion—With an increase in the expression of CTE complexes as well as a proportional increase in oxygen consumption rate (OCR), and increased sensitivity to DOX was evident upon inactivating MFN-2 by CRISPR (Decker et al., 2020).
The anti-apoptotic protein Bcl-2 homologous antagonist/killer (BCL-2), overexpressed in some subtypes of B-cell lymphomas, regulates mitochondrial function and mediates therapeutic resistance by inhibiting the oligomerization of the pro-apoptotic Bcl-2 homologous antagonist/killer (Bak) and Bcl-2-associated X protein (Bax) proteins and by facilitating the import of GSH and complex IV subunits to mitochondria (Mattes et al., 2019). The dependence of BCL-2 activity has been demonstrated in both chemosensitive and chemoresistant myeloblasts but not in normal hematopoietic stem cells (HSCs) (Vo et al., 2012); therefore, the use of BCL-2 inhibitors has recently emerged, especially the use of venetoclax in the treatment of AML (Griffioen et al., 2022). Despite its potential in vivo, factors such as the upregulation of fatty acid oxidation (FAO) (Stevens et al., 2020), mutations in BCL-2, overexpression of the anti-apoptotic proteins MCL1 or B-cell lymphoma extra-large (Bcl-xL) (Saliba et al., 2021), or the OPA1 (Mitochondrial Dynamin Like GTPase) fusion protein have been described as mediators of resistance to venetoclax (Chen X. et al., 2019b).
The enzymes isocitrate dehydrogenases (IDH) produce NADPH by metabolizing isocitrate to α-ketoglutarate (α-KG), which is an important factor in the TCA cycle. In AML, IDH mutations lead to the consumption of NADPH by converting isocitrate to 2-HG, considered an oncometabolite. The accumulation of 2-HG triggers the inhibition of α-KG-dependent enzymes, such as TET2 (Tet methylcytosine dioxygenase 2) demethylase, and consequently alters gene expression by favoring DNA hypermethylation (Raimondi et al., 2022). The improvement in mitochondrial oxidative metabolism has been associated with resistance to the use of IDH inhibitors and the concomitant use of OxPhos inhibitors improves the efficacy of treatment against IDH mutated in vivo in AML (Stuani et al., 2021).
Regarding external factors, microenvironment-induced tumor reprogramming also affects mitochondrial function. In AL, the transfer of mitochondria between leukemic cells and MSCs as a mechanism of tumor protection has been reported (Polak et al., 2015; Moschoi et al., 2016; Wang et al., 2018). Transfer inhibition using metformin improves the chemosensitivity of AML cells cocultured with MSCs to Ara-C (You et al., 2022). In fact, Ara-C increases mitochondrial transfer from MSCs to AML cells triggered by OxPhos inhibition, favoring tumor progression (Saito et al., 2021). Zhang et al. (2022) described that OCI-AML3 myeloid cells induce adipogenic differentiation in MSCs and reduce osteoblastic differentiation (Figure 2). This alteration is accompanied by a metabolic change from glycolysis to a more oxidative form given by a decrease in phosphoglycerate mutase 1 (PGAM1), ALDOA, LDHA/B, and an increase in succinate dehydrogenase (SDH) A/B/C/D from the ADP-forming beta subunit of succinate-CoA ligase (SUCLA2), aconitase 2 (ACO2), PDK, and pyruvate dehydrogenase phosphatase (PDP), among others.
3.3 Association between mitochondrial function and clinical response and survival rates in ALs
In AML, patients with lower rates of remission and overall survival have a proteogenomic profile characterized by high expression of mitochondrial proteins and a more complex I-dependent respiration (Jayavelu et al., 2022). Additionally, Nan et al. (2022) in a group with high-risk AML identified an increased mitochondrial gene signature: electron transfer flavoprotein subunit beta (ETFB), carnitine palmitoyl transferase 1A (CPT1A), 4-Hydroxyphenylpyruvate Dioxygenase Like (HPDL), and isocitrate Dehydrogenase NAD+ 3 Catalytic Subunit Alpha (IDH3A) (Jiang et al., 2022). Likewise, patients with mutations in CTE complexes have worse overall survival compared to those without mutations (Silkjaer et al., 2013). Also, a proteomic study on primary AML cells derived from patients in their first relapse shows enrichment of mitochondrial ribosomal proteins (Mitochondrial Ribosomal Protein L21 -MRPL21-, Mitochondrial Ribosomal Protein S33 -MRPS3-), and CTE proteins: Translocase of Inner Mitochondrial Membrane Domain Containing 1 (TIMMDC1), a chaperone protein involved in the assembly of Complex I, and Mitochondrial Import Inner Membrane Translocase subunit (Tim8B TIMM8B), a chaperone that participates in the import and insertion of some transmembrane proteins in the inner mitochondrial membrane (Aasebø et al., 2020). In addition, patients treated with chemotherapy show lasting changes in the expression of genes involved with OxPhos activation, which can be explained because tumor cells, after chemotherapy-induced damage, increase their demand for ATP for repair processes (Vellinga et al., 2015). Therefore, these studies in patients support the idea that enhanced mitochondrial metabolism is associated with a poor prognosis; however, further studies are needed to elucidate the role of mitochondria in chemoresistance in acute leukemia.
4 Relationship between altered energy metabolism and the immune system
Malignant transformation is accompanied by changes in cell metabolism that have an impact on immune system function, reducing the control of cancer cells (O’Sullivan et al., 2019; Patel et al., 2019). These alterations include competition for substrates, the abundant release of bioactive metabolites, and microenvironmental metabolic remodeling that favors the induction or survival of subsets of tolerogenic cells (Mougiakakos, 2019). Modulation of tumor metabolism can improve immune cell activation, allowing an efficacious antitumor response, which achieves an effective therapeutic approach against ALs.
Increased glycolysis has been associated with inflammatory effector phenotypes in a variety of activated immune cells. In contrast, mitochondrial oxidation programs are associated with memory, suppressor, or wound-healing immune cell phenotypes. Each subset of immune cells has been shown to have distinct metabolic requirements. Thus, although T cells, macrophages, and dendritic cells (DC) each have significant plasticity to engage in other metabolic pathways for energy generation, survival, and proliferation, these changes alter or impair immune function. These alterations in cell function are due to the needs of each immunological subset for specific metabolites, signaling intermediates, or epigenetic modifications mediated by metabolite levels. As metabolic intermediaries change, cell differentiation is not adequately induced, or stress response pathways feedback to restrict or alter the ultimate fate of cells (Andrejeva and Rathmell, 2017).
4.1 Tumor glycolytic metabolism and immune response
The metabolites and waste products excreted by tumor cells (lactate, CO2, H+, NH4+, nitric oxide, butyrate, polyamines, and ROS, among others) establish acidification of the microenvironment, that is, compatible with cell proliferation and dissemination, promoting several processes such as decreased cell adhesion, angiogenesis, and mesenchyme-epithelial transition (MET) in other tumor models.
Tumor cells can evade the surveillance of the immune system by secreting lactate. This extracellular metabolite generates acidification of the medium that reduces the pH inside immune cells, affecting various signaling pathways and inhibiting the activation and proliferation of CD4+, CD8+ T cells, NK cells, and DCs. In models of solid tumors, it has been described that the increase in lactate stimulates the polarization of resident macrophages to M2-type macrophages (immunosuppressants), promotes angiogenesis, and stimulates the production of hyaluronic acid by fibroblasts that can contribute to tumor invasion (Hirschhaeuser et al., 2011; Pavlova and Thompson, 2016; de la Cruz-López et al., 2019). It has been described that ROS and HIF can promote a “reverse Warburg effect” in cancer-associated fibroblasts (CAFs), which release lactate through the MCT4 (proton-linked monocarboxylate transporter) receptor, which is captured by tumor cells through MCT1, to be converted to pyruvate and metabolized at the mitochondrial level (Icard et al., 2018). In AML, it has been described that lactate could contribute to the induction of regulatory T lymphocytes and suppressor myeloid cells. (Mougiakakos, 2019). Cancer cells’ greed for glucose inactivates effector T cells and DCs, whereas programmed cell death ligand-1 (PD-L1) expressed by tumor cells stimulates glycolysis and the Akt pathway in cancer cells, thereby helping maintain its proliferation (Jayavelu et al., 2022).
4.2 Mitochondrial function and immune response
Mitochondria influence immunosurveillance through intrinsic and extrinsic mechanisms in cancer cells. On the one hand, mitochondria are the source of many danger signals released by dying cancer cells, and these signals are crucial for the DCs activation (Porporato et al., 2018) and tumor-associated macrophages (TAMs) (Icard et al., 2018), as well as the concentrations of succinate and citrate. M1 macrophages, which have a proinflammatory and anticancer role, are dependent on glycolysis and an abnormal TCA cycle that leads to citrate and succinate accumulation. Citrate accumulation increases the production of the main mediators of acute inflammation (nitric oxide, ROS, and prostaglandins), while succinate induces the production of IL-1β, a central molecule in inflammation (Icard et al., 2018).
Furthermore, mitochondrial metabolism is involved in many functions linked to cancer immunity, including (but not limited to) the activation of inflammasomes, the establishment of protective immunological memory, tumor subset differentiation, and macrophage-specific activity (Porporato et al., 2018). Mitochondria serve as sources of molecules that activate inflammatory pathways as well as signaling platforms to propagate these signals (Giampazolias and Tait, 2016).
2-HG has also been implicated in tumor immune evasion. 2-HG transport into T cells is facilitated by the sodium-dependent dicarboxylate transporter (SLC13A3), altering its effector function and cell proliferation. In addition, it interferes with the activation of activated T-cell nuclear factor 1 (NFATC1), a key transcription factor for activated T-cell function, which is linked to ATP deficiency. This oncometabolite also acts at the biochemical level in T cells by inhibiting ornithine decarboxylase, an enzyme that participates in the biosynthesis of polyamines (such as putrescine), molecules necessary for the proper functioning of T cells. This represents a self-perpetuating effect since putrescine can antagonize 2-HG, suppressing cell proliferation. 2-HG has also been shown to inhibit CD12 expression on DC and inhibit CXCL-10 secretion, thus preventing T-cell recruitment. In naive T cells, 2-HG is associated with the destabilization of HIF-1α, preservation of OxPhos, and increased differentiation of CD4+/CD25+/FOXP3+ regulatory T cells. This proceeds at the expense of differentiation into Th17 cells. Finally, 2-HG, through the stimulation of NF-kB, induces cell proliferation in a stromal niche for AML cells and, at relatively low concentrations, promotes fibroblast proliferation (Ježek, 2020).
5 Modulation of energy metabolism and activation of the immune system as targets of natural products
Substantial studies highlight the antineoplastic effect of natural products in addition to their use in the development of new drugs (Newman and Cragg, 2016; Sarwar et al., 2018), suggesting their great potential to become a coadjuvant alternative for the ALs treatment. According to their chemical composition, natural products can be subclassified into: alkaloids, carotenoids, nitrogen-containing compounds, organosulfur compounds, and phenolic compounds, the latter being the group that has the greatest in vitro effect on AML cells, perhaps because they are one of the substances more widespread among plants (Hwang et al., 2019).
Currently, there are natural products that have been used in the treatment of ALL and AML, such as vincristine, an alkaloid derived from Catharanthus roseus, and etoposide, a semisynthetic derivative of podophyllin obtained from Podophyllum (Lucas et al., 2010). In in vitro assays, there are various plants that show a cytotoxic effect on AML cells through different mechanisms (Hwang et al., 2019). Interestingly, the potentiation of the antineoplastic effect has been published when natural products and conventional chemotherapeutics are used in combination. In AML, there is a synergy between Ara-C and resveratrol. The latter can act by inhibiting the CTE complex III, affecting the pool of dNTPs, and reducing the proliferation of leukemic cells (Horvath et al., 2005).
In addition to the role of natural products in PCD, they may also prevent resistance by tumor cells to conventional chemotherapeutics (Yuan et al., 2017), thanks to their intervention in tumor metabolism (Figure 3). Plant-derived components can modulate energy metabolism at both the glycolytic (Hasanpourghadi et al., 2017) and mitochondrial (Tao et al., 2019) levels in different cancer models.
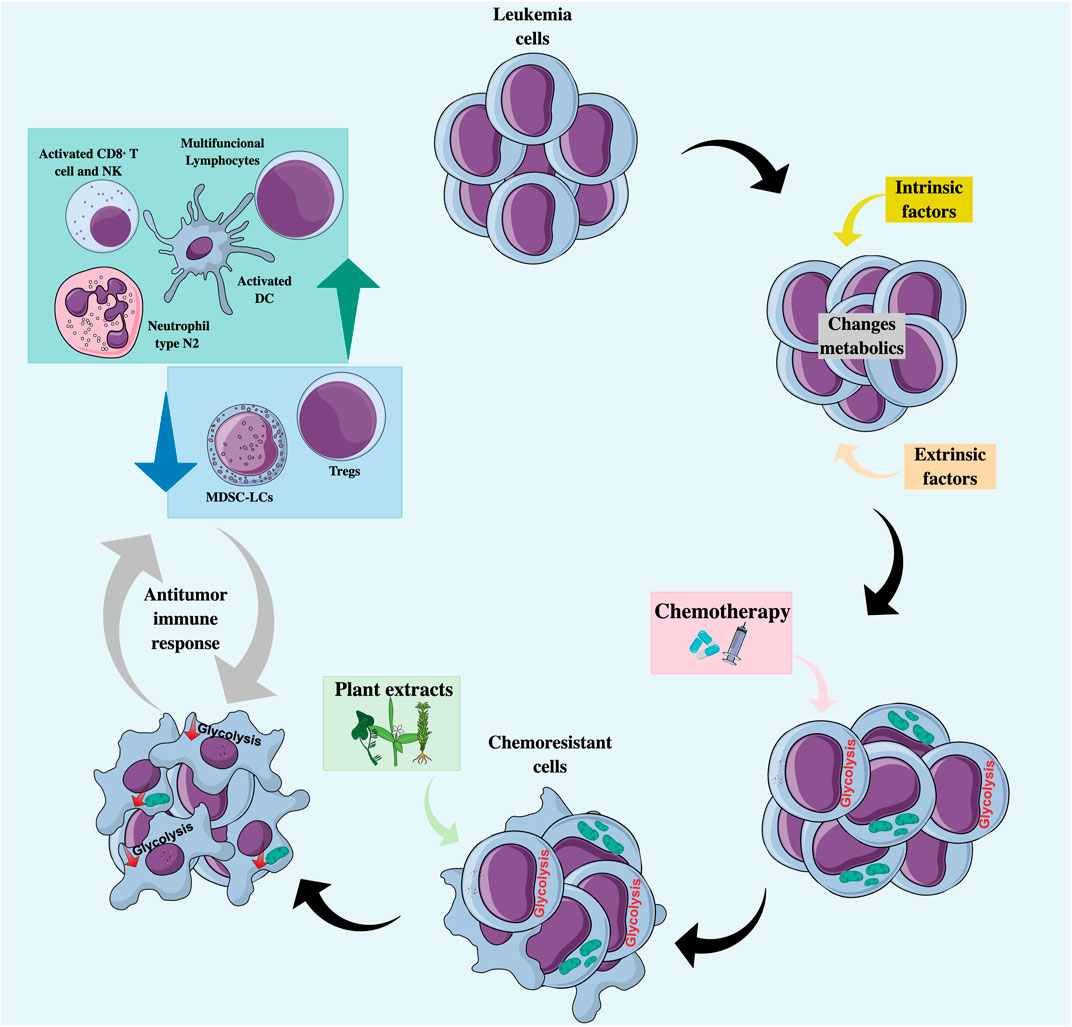
FIGURE 3. Natural products as co-adjuvant therapy in acute leukemia with a metabolic and immunomodulatory approach. Treatment with chemotherapeutic agents may favor the selection of chemoresistant clones characterized by an increase in glycolytic flux or an increase in mitochondrial activity. Combined treatment with natural products can lead to the regulation of tumor metabolism by decreasing glycolysis and/or altering mitochondrial function, making cells more sensitive to cell death, and promoting the recognition and elimination of tumor cells by cells of the immune system. DC, Dendritic cells; MDSC-LCs, Myeloid-derived suppressor-like cells; N2 neutrophils, Anti-tumor phenotype; NK, Natural killer; Tregs, Regulators T cells.
Focusing on the modulation of metabolism, fermented wheat germ extract is a complex mixture of biologically active molecules with an antimetastatic activity that inhibits the enzymes glucose-6-phosphate dehydrogenase (G6PDH) and transketolase, which regulate carbon flux in glycolysis and the pathway of the pentoses in Jurkat line cell (Comi-Anduix et al., 2022). Phenolic phytometabolites such as curcumin, apigenin, resveratrol, or some alkaloids such as alkeberberine or capsaicin target HIF-1, GLUTs, or some enzymes of the glycolytic pathway such as HK-II, PFKP, and PK, among others (Hasanpourghadi et al., 2017). However, in ALs, the role of natural compounds on glycolytic metabolic regulation has been poorly evaluated.
On the other hand, phenolic compounds can act as mitocans through different mechanisms that include inhibition of HK-II, BCL-2, electron transport chain complexes, induction of oxidative stress, voltage-gated anion channel (VDAC)/adenine nucleotide translocase (ANT) complex dysregulation, or as lipophilic cations targeting the inner mitochondrial membrane, some intermediates of the TCA cycle, or mitochondrial DNA (Guerra et al., 2018). One of the widely studied anti-tumor effects of phenolic compounds is the neutralization of ROS because of its integral role in carcinogenesis and mitochondria as its main source. The antioxidant effect is attributed to the modulating capacity of intracellular antioxidant enzymes, direct elimination of ROS by donation of electrons or hydrogens, chelation of transition metals, or induction of antioxidant pathways such as Kelch-like ECH-associated protein 1- Nuclear factor erythroid 2 related factor 2 (Keap1-Nrf2) (Stevens et al., 2018). Interestingly, phenolic compounds can also exert a prooxidant role that depends on the total number of hydroxyl groups and their substitution patterns. The dual effects of some compounds are promising in combination therapies.
An example of the modulation of mitochondrial metabolism in primary AML cells is the action of lipid B extracted from avocado, which can exert a selective cytotoxic effect on leukemic cells. This specificity can be attributed to increased mitochondrial mass and altered mitochondrial metabolism. Lipid B can enter the mitochondria through carnitine palmitoyl transferase I (CPT1), an enzyme that facilitates the transport of mitochondrial lipids, favoring their accumulation and consequently the inhibition of fatty acid oxidation and reduction in NADPH levels, leading to cell death (Lee et al., 2015).
Regarding the immunomodulatory potential of natural products in ALs, in in vitro assays, continuous treatment of HL60 cells with DOX induces a differentiation towards type N1 neutrophils (pro-tumor phenotype); however, the addition of berberine, an alkaloid, favors a change towards type N2 neutrophils (anti-tumor phenotype) (Zhang et al., 2020). Also, chrysophanol, one of the most important anthraquinone components isolated from plants of the genus Rheum, was proposed as an immunomodulator by Zhong et al. (2022), since they suggested that its antitumor effects against acute leukemia T cells were due to thorough regulation of the immunosuppressive molecule PD-L1 (Yin et al., 2020). In turn, resveratrol improved T lymphocyte proliferation and NK cell activity in a mouse leukemia model (L1210) (Li et al., 2007). Further studies of natural products as immunomodulators in AL could help discover promising compounds against immune checkpoints.
The central research area of our group is the study and development of phytomedicines from Colombian plants, among them Petiveria alliacea (P. alliacea), Caesalpinia spinosa (C. spinosa), Tillandsia usneoides (T. usneoides), and Piper nigrum (P. nigrum) (Lasso et al., 2018; Urueña et al., 2020; Lasso et al., 2022; Urueña et al., 2022). Each one of the extracts of these plants has been characterized from a chemical point of view, and the main compounds have been isolated and studied. The extracts are standardized to be developed as botanical drugs due to their interesting antitumor and immunomodulatory activity in animal models (Lasso et al., 2018; Urueña et al., 2020). C. spinosa has also been studied in normal individuals to assess its safety and in coronavirus disease 2019 (COVID-19) patients, where significant biological activity has been demonstrated (Duran et al., 2022; Urueña et al., 2022).
P. alliacea is a plant used in Central and South America as traditional medicine due to its antispasmodic, antirheumatic, anti-inflammatory, and analgesic activities. Regarding its activity on metabolism, traditional knowledge attributes hypoglycemic properties to it (Fiorentino and Urueña, 2018). We have found cytotoxic activity in tumor cell lines of murine and human origin for melanoma, breast cancer, and acute leukemia (Sandoval et al., 2016; Prieto et al., 2019; Ballesteros-Ramírez et al., 2020b). The proteomic analysis of tumor cells treated with aqueous extract of P. alliacea revealed an alteration of proteins that participate in cell proliferation and energy metabolism, as well as a decrease in glucose uptake and lactate production. This suggests that the extract uses multiple biological mechanisms to regulate tumor growth (Hernández et al., 2014). In the in vivo model, the extract slows the progression of murine mammary tumors after orthotopic transplantation with 4T1 cells (Hernández et al., 2014).
More recently, it has been observed that the activity of P. alliacea extract is specific to tumor cells, inducing a reduction in the expression of the enzyme β-F1-ATPase, the concentration of intracellular ATP, and mitochondrial respiration (Hernández et al., 2017). Like other compounds obtained from plants, P. alliacea can act on DCs, inducing their partial activation, which is evidenced by morphological and phenotypic changes, associated with a differential profile of secreted cytokines (Santander et al., 2012). Our group is currently delving into the tumor context of acute leukemias.
On the other hand, in traditional medicine, the seeds and pods of C. spinosa are used to treat tonsillitis, gastric ulcers, and skin infections due to their high content of hydrolyzable tannins (derived from gallic acid) and their antibacterial, antitumor, astringent, anti-inflammatory, and healing properties. They are also known for their high antioxidant capacity (Fiorentino and Urueña, 2018). It is distributed throughout Latin America, and in Colombia, it occurs naturally. The extract obtained from C. spinosa was called P2Et. This extract can induce apoptosis through depolarization of mitochondria, activation of caspase 3, chromatin condensation, and decreased clonogenic capacity in K562 leukemia cells and 4T1 breast cancer cells. In addition, when used in combination with DOX at sublethal concentrations, a reduction in IC50 is observed, representing an increase in the net antitumor activity of the drug (Castañeda et al., 2012; Urueña et al., 2013). Interestingly, in vitro, P2Et is cytotoxic both in resistant lines with the expression of Pgp+ and in Pgp-lines in 2D and 3D cultures (Sandoval et al., 2016). It has been possible to show that the marked antioxidant activity of C. spinosa may participate in the regulation of ROS production inside and outside the tumor mass in some cancer models, which would confer great antitumor potential (Prieto et al., 2019). An immunomodulatory effect has been attributed to P2Et since it can induce the expression of immunogenic cell death markers such as calreticulin and High Mobility Group-Box superfamily 1 (HMGB1) and the release of ATP. In the in vivo model, mice transplanted with 4T1 cells treated in vitro with P2Et showed reduced tumor growth, and ex vivo analysis revealed multifunctional CD4+ and CD8+ T lymphocytes (Urueña et al., 2013; Gomez-Cadena et al., 2016).
Focusing on ALs, cells isolated from patients with ALL or AML have been shown to be less sensitive to P2Et and more sensitive to P. alliacea. In addition, the treatment of leukemic blasts with each of the two extracts combined with some drugs increases the sensitivity to death even in tumor cells that, in some cases, do not respond to conventional therapies. These data show that, at least in vitro, the extracts of P. alliacea and C. spinosa are capable of acting on human primary tumors, improving the response to chemotherapy (Ballesteros-Ramírez et al., 2020b).
Other plants with emerging anti-leukemic activity in our group are the extracts of T. usneoides and P. nigrum. In a mouse model transplanted with DA-3/ER-GM murine acute myeloid leukemia cells, both P2Et and T. usneoides-derived extracts were found to decrease the tumor load in peripheral blood with respect to the control group (unpublished data). Furthermore, in the 4T1 murine breast cancer model, extracts derived from T. usneoides and P. nigrum modulate the immune response by increasing the frequency of DC and activated CD8+ T cells and decreasing myeloid-derived suppressor-like cells (MDSC-LCs) and regulators T cells (Tregs) in the tumor microenvironment, thus favoring control of tumor growth (Lasso et al., 2022). At the metabolic level, T. usneoides and P. nigrum extracts behave as prooxidants in both B16-F10 melanoma cells and 4T1 breast cancer cells (Lasso et al., 2022). Regarding glucose consumption, T. usneoides does not induce significant changes in glucose uptake in 4T1 cells; however, it induces an evident increase at 12 h in B16-F10 cells (Lasso et al., 2022). P. nigrum induces a dose-dependent decrease in intracellular glucose uptake, in contrast to a small increase in intracellular glucose uptake in B16-F10 cells (unpublished data). The mechanisms related to the intrinsic sensitivity of the different tumor cells to each of these extracts could be related to differences in their metabolic plasticity, so immunomodulation and involvement in metabolic changes are being studied in the acute leukemia model. Some extracts or isolated compounds derived from plants with the capacity to induce metabolic changes and/or with immunomodulatory potential evaluated in leukemias are summarized in Table 1 and in other tumor models in Table 2.
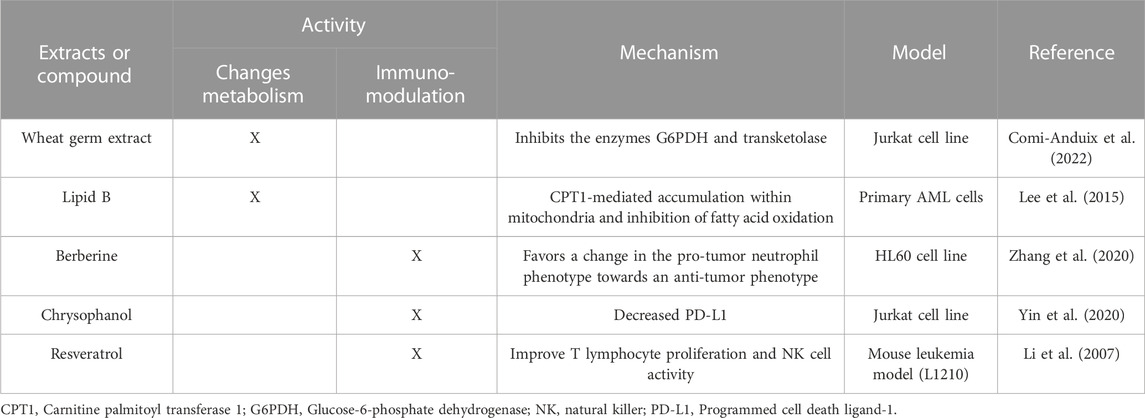
TABLE 1. Summary of plant-derived extracts or compounds that target tumor metabolism or are proven immunomodulators in acute leukemias.
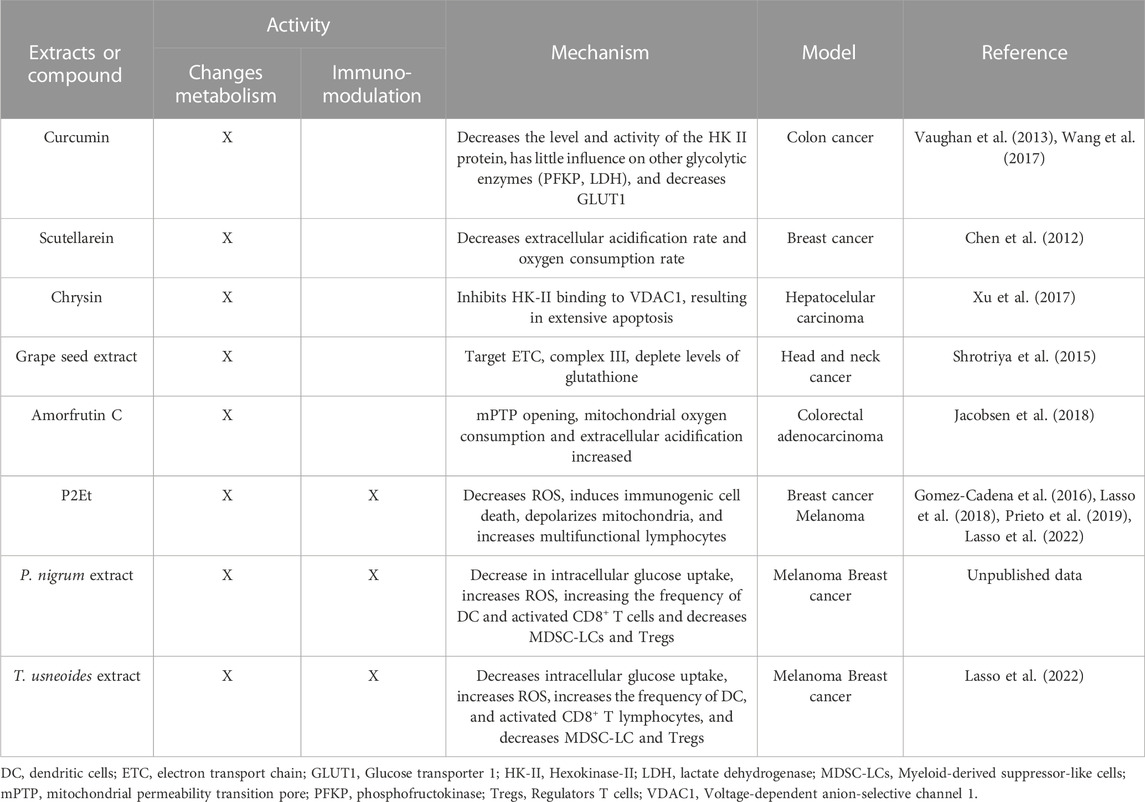
TABLE 2. Summary of some plant-derived extracts or compounds that target tumor metabolism or are proven immunomodulators in different cancer models.
6 Conclusion
Both an increase in glycolytic activity and mitochondrial changes may underlie tumor cell resistance to many types of drugs. Although mechanistic evidence has been mainly in tumor cell lines, there is evidence of increased glycolytic metabolism in patients with ALs related to poor response to treatment. However, Recent in vivo and systemic studies in cancer patients support the notion that mitochondria, through their role in metabolic adaptation, also play a pivotal role in chemoresistance in ALs rather than the glycolytic phenotype. These findings suggest that both ways can be implicated, and possibly other factors such as population differences (genetics, lifestyle, microbiome nutrition, co-infections, oncogenic mutations, etc.) could influence this modulation and resistance to chemotherapy. Therapeutic intervention using extracts derived from plants developed as botanical medicines to induce metabolic changes by altering glycolysis or mitochondrial function in resistant leukemic cells can sensitize the tumor cell, potentiating the action of conventional chemotherapeutics and resulting in an immunomodulatory effect that allows the activation of an antitumor immune response.
Author contributions
CA, SQ, and SF gave the idea and drafted the manuscript. CA performed the literature search and drafted figures. CA, NC-R, SQ, and SF edited the manuscript and supervised the whole study. All authors contributed to the article and approved the submitted version.
Funding
Ministerio de Ciencia, Tecnología e Innovación (Colciencias), Ministerio de Educación Nacional, Ministerio de Industria, Comercio y Turismo e ICETEX (792–2017 2a Convocatoria Ecosistema Científico—Colombia Científica para la Financiación de Proyectos de I + D + i), and Vicerrectoría de Investigaciones, Pontificia Universidad Javeriana, Bogotá D.C., Colombia (Contract no. FP44842-221-2018).
Acknowledgments
The authors would like to thank Pontificia Universidad Javeriana, the Grupo de Inmunobiología y Biología Celular, Bogotá D.C., Colombia, and the Programa de Colombia Científica (GAT), contract no. FP44842-221-2018.
Conflict of interest
The authors declare that the research was conducted in the absence of any commercial or financial relationships that could be construed as a potential conflict of interest.
Publisher’s note
All claims expressed in this article are solely those of the authors and do not necessarily represent those of their affiliated organizations, or those of the publisher, the editors and the reviewers. Any product that may be evaluated in this article, or claim that may be made by its manufacturer, is not guaranteed or endorsed by the publisher.
Supplementary material
The Supplementary Material for this article can be found online at: https://www.frontiersin.org/articles/10.3389/fmolb.2023.1229760/full#supplementary-material
References
Aasebø, E., Berven, F. S., Hovland, R., Døskeland, S. O., Øystein, B., Selheim, F., et al. (2020). The progression of acute myeloid leukemia from first diagnosis to chemoresistant relapse: A comparison of proteomic and phosphoproteomic profiles. Cancers (Basel) 12 (6), 1466. doi:10.3390/cancers12061466
Agbu, P., and Carthew, R. W. (2021). MicroRNA-mediated regulation of glucose and lipid metabolism. Nat. Rev. Mol. Cell Biol. 22 (6), 425–438. doi:10.1038/s41580-021-00354-w
Altaf, E., Huang, X., Xiong, J., Yang, X., Deng, X., Xiong, M., et al. (2017). NHE1 has a notable role in metastasis and drug resistance of T-cell acute lymphoblastic leukemia. Oncol. Lett. 14 (4), 4256–4262. doi:10.3892/ol.2017.6716
Andrejeva, G., and Rathmell, J. C. (2017). Similarities and distinctions of cancer and immune metabolism in inflammation and tumors. Cell Metab. 26 (1), 49–70. doi:10.1016/j.cmet.2017.06.004
Arber, D., Orazi, A., Hasserjian, R., Thiele, J., Borowitz, M., Le Beau, M., et al. (2016). The 2016 revision to the World Health Organization classification of myeloid neoplasms and acute leukemia. Blood 127 (20), 2391–2405. doi:10.1182/blood-2016-03-643544
Ariës, I. M., Hansen, B. R., Koch, T., Van Den Dungen, R., Evans, W. E., Pieters, R., et al. (2013). The synergism of MCL1 and glycolysis on pediatric acute lymphoblastic leukemia cell survival and prednisolone resistance. Haematologica 98 (12), 1905–1911. doi:10.3324/haematol.2013.093823
Auberger, P., and Puissant, A. (2017). Autophagy, a key mechanism of oncogenesis and resistance in leukemia. Blood 129 (5), 547–552. doi:10.1182/blood-2016-07-692707
Ballesteros-Ramírez, R., Aldana, E., Herrera, M. V., Urueña, C., Rojas, L. Y., Echeverri, L. F., et al. (2020b). Preferential activity of Petiveria alliacea extract on primary myeloid leukemic blast. Evid. Based Complement. Altern. Med. 2020, 4736206. doi:10.1155/2020/4736206
Ballesteros-Ramírez, R., Quijano, S., Solano, J., Ordoñez-Reyes, C., Herrera, M. V., Murillo, R., et al. (2020a). Influence of dose intensity in consolidation with HIDAC and other clinical and biological parameters in the survival of AML. J. Cancer Epidemiol. 2020, 8021095. doi:10.1155/2020/8021095
Basak, N. P., and Banerjee, S. (2015). Mitochondrial dependency in progression of acute myeloid leukemia. Mitochondrion 21, 41–48. doi:10.1016/j.mito.2015.01.006
Bokil, A., and Sancho, P. (2019). Mitochondrial determinants of chemoresistance. Cancer Drug Resist 2 (3), 634–646. doi:10.20517/cdr.2019.46
Boutin, L., Arnautou, P., Trignol, A., Ségot, A., Farge, T., Desterke, C., et al. (2020). Mesenchymal stromal cells confer chemoresistance to myeloid leukemia blasts through Side Population functionality and ABC transporter activation. Haematologica 105 (4), 987–9998. doi:10.3324/haematol.2018.214379
Braun, M., Qorraj, M., Büttner, M., Klein, F. A., Saul, D., Aigner, M., et al. (2016). CXCL12 promotes glycolytic reprogramming in acute myeloid leukemia cells via the CXCR4/mTOR axis. Leukemia 30 (8), 1788–1792. doi:10.1038/leu.2016.58
Cardano, M., Tribioli, C., and Prosperi, E. (2020). Targeting proliferating cell nuclear antigen (PCNA) as an effective strategy to inhibit tumor cell proliferation. Curr. Cancer Drug Targets 20 (4), 240–252. doi:10.2174/1568009620666200115162814
Castañeda, D. M., Pombo, L. M., Urueña, C. P., Hernandez, J. F., and Fiorentino, S. (2012). A gallotannin-rich fraction from Caesalpinia spinosa (Molina) Kuntze displays cytotoxic activity and raises sensitivity to doxorubicin in a leukemia cell line. BMC Complement. Altern. Med. 12, 38. doi:10.1186/1472-6882-12-38
Chalkiadaki, A., and Guarente, L. (2015). The multifaceted functions of sirtuins in cancer. Nat. Rev. Cancer 15 (10), 608–624. doi:10.1038/nrc3985
Chen, C., Hao, X., Lai, X., Liu, L., Zhu, J., Shao, H., et al. (2021). Oxidative phosphorylation enhances the leukemogenic capacity and resistance to chemotherapy of B cell acute lymphoblastic leukemia. Sci. Adv. 7 (11), eabd6280. doi:10.1126/sciadv.abd6280
Chen, L., Hu, N., Wang, C., and Zhao, H. (2020). HOTAIRM1 knockdown enhances cytarabine-induced cytotoxicity by suppression of glycolysis through the Wnt/β-catenin/PFKP pathway in acute myeloid leukemia cells. Arch. Biochem. Biophys. 680, 108244. doi:10.1016/j.abb.2019.108244
Chen, L., Zhao, H., Wang, C., and Hu, N. (2019a). TUG1 knockdown enhances adriamycin cytotoxicity by inhibiting glycolysis in adriamycin-resistant acute myeloid leukemia HL60/ADR cells. RSC Adv. 9 (19), 10897–10904. doi:10.1039/c9ra00306a
Chen, V., Staub, R. E., Baggett, S., Chimmani, R., Tagliaferri, M., Cohen, I., et al. (2012). Identification and analysis of the active phytochemicals from the anti-cancer botanical extract Bezielle. PLoS One 7 (1), e30107. doi:10.1371/journal.pone.0030107
Chen, W. L., Wang, J. H., Zhao, A. H., Xu, X., Wang, Y. H., Chen, T. L., et al. (2014). A distinct glucose metabolism signature of acute myeloid leukemia with prognostic value. Blood 124 (10), 1645–1654. doi:10.1182/blood-2014-02-554204
Chen, X., Glytsou, C., Zhou, H., Narang, S., Reyna, D. E., Lopez, A., et al. (2019b). Targeting mitochondrial structure sensitizes acute myeloid leukemia to venetoclax treatment. Cancer Discov. 9 (7), 890–909. doi:10.1158/2159-8290.CD-19-0117
Cho, S. H., Ahn, A. K., Bhargava, P., Lee, C-H., Eischen, C. M., Mcguinness, O., et al. (2011). Glycolytic rate and lymphomagenesis depend on PARP14, an ADP ribosyltransferase of the B aggressive lymphoma (BAL) family. Proc. Natl. Acad. Sci. U. S. A. 108 (38), 15972–15977. doi:10.1073/pnas.1017082108
Combariza, J. F., Casas, C. P., Rodríguez, M., Cardona, A. F., Ospina, É., and Grajales, M. (2007). Supervivencia en adultos con leucemia linfoide aguda de novo tratados con el esquema HyperCVAD en el Instituto Nacional de Cancerología (Colombia), entre enero de 2001 y junio de 2005. Rev. Colomb. Cancerol. 11, 92–100.
Comi-Anduix, B., Boros, L. G., Marin, S., Boren, J., Callol-Massot, C., Centelles, J. J., et al. (2022). Fermented wheat germ extract inhibits glycolysis/pentose cycle enzymes and induces apoptosis through poly(ADP-ribose) polymerase activation in Jurkat T-cell leukemia tumor cells. J. Biol. Chem. 277 (48), 46408. doi:10.1074/jbc.M206150200
de la Cruz-López, K. G., Castro-Muñoz, L. J., Reyes-Hernández, D. O., García-Carrancá, A., and Manzo-Merino, J. (2019). Lactate in the regulation of tumor microenvironment and therapeutic approaches. Front. Oncol. 9, 1143. doi:10.3389/fonc.2019.01143
DeBerardinis, R. J., and Chandel, N. S. (2016). Fundamentals of cancer metabolism. Sci. Adv. 2 (5), e1600200. doi:10.1126/sciadv.1600200
Decker, C. W., Garcia, J., Gatchalian, K., Arceneaux, D., Choi, C., Han, D., et al. (2020). Mitofusin-2 mediates doxorubicin sensitivity and acute resistance in Jurkat leukemia cells. Biochem. Biophys. Rep. 24, 100824. doi:10.1016/j.bbrep.2020.100824
Desbats, M. A., Giacomini, I., Prayer-Galetti, T., and Montopoli, M. (2020). Metabolic plasticity in chemotherapy resistance. Front. Oncol. 10, 281. doi:10.3389/fonc.2020.00281
Di Martino, L., Tosello, V., Peroni, E., and Piovan, E. (2021). Insights on metabolic reprogramming and its therapeutic potential in acute leukemia. Int. J. Mol. Sci. 22 (16), 8738. doi:10.3390/ijms22168738
Duan, Z., Chen, Y., Ye, M., Xiao, L., Chen, Y., Cao, Y., et al. (2022). Differentiation and prognostic stratification of acute myeloid leukemia by serum-based spectroscopy coupling with metabolic fingerprints. FASEB J. 36 (7), e22416. doi:10.1096/fj.202200487R
Duran, M. I., Ballesteros-Ramírez, R., Tellez, A., Torregrosa, L., Olejua, P. A., Galvis, S., et al. (2022). Safety evaluation in healthy Colombian volunteers of P2Et extract obtained from Caesalpinia spinosa: Design 3+3 phase I clinical trial. Evid. Based Complement. Altern. Med. 2022, 7943001. doi:10.1155/2022/7943001
Farge, T., Saland, E., de Toni, F., Aroua, N., Hosseini, M., Perry, R., et al. (2017). Chemotherapy-resistant human acute myeloid leukemia cells are not enriched for leukemic stem cells but require oxidative metabolism. Cancer Discov. 7 (7), 716–735. doi:10.1158/2159-8290.CD-16-0441
Fiorentino, S., and Urueña, C. (2018). La fitoterapia como fuente de medicamentos reguladores del metabolismo tumoral y activadores de la respuesta inmunitaria. Rev. Acad. Colomb. Cienc. Exact. Fis. Nat. 42 (163), 132. doi:10.18257/raccefyn.542
Frolova, O., Samudio, I., Benito, J. M., Jacamo, R., Kornblau, S. M., Markovic, A., et al. (2012). Regulation of HIF-1α signaling and chemoresistance in acute lymphocytic leukemia under hypoxic conditions of the bone marrow microenvironment. Cancer Biol. Ther. 13 (10), 858–870. doi:10.4161/cbt.20838
Genovese, I., Carinci, M., Modesti, L., Aguiari, G., Pinton, P., and Giorgi, C. (2021). Mitochondria: Insights into crucial features to overcome cancer chemoresistance. Int. J. Mol. Sci. 22 (9), 4770. doi:10.3390/ijms22094770
Giampazolias, E., and Tait, S. W. G. (2016). Mitochondria and the hallmarks of cancer. FEBS J. 283 (5), 803–814. doi:10.1111/febs.13603
Giansanti, M., De Gabrieli, A., Prete, S. P., Ottone, T., Divona, M. D., Karimi, T., et al. (2021). Poly(ADP-Ribose) polymerase inhibitors for arsenic trioxide–resistant acute promyelocytic leukemia: Synergistic in vitro antitumor effects with hypomethylating agents or high-dose vitamin C. J. Pharmacol. Exp. Ther. 377 (3), 385–397. doi:10.1124/jpet.121.000537
Gomez-Cadena, A., Uruenã, C., Prieto, K., Martinez-Usatorre, A., Donda, A., Barreto, A., et al. (2016). Immune-system-dependent anti-tumor activity of a plant-derived polyphenol rich fraction in a melanoma mouse model. Cell Death Dis. 7 (6), e2243. doi:10.1038/cddis.2016.134
Grasso, D., Zampieri, L., Capelôa, T., Van de Velde, J., and Sonveaux, P. (2020). Mitochondria in cancer. Cell Stress 4 (6), 114–146. doi:10.15698/cst2020.06.221
Griffioen, M. S., De Leeuw, D. C., Janssen, J. J. W. M., and Smit, L. (2022). Targeting acute myeloid leukemia with venetoclax; biomarkers for sensitivity and rationale for venetoclax-based combination therapies. Cancers (Basel). 14 (14), 3456. doi:10.3390/cancers14143456
Grønningsæter, I. S., Fredly, H. K., Gjertsen, B. T., Hatfield, K. J., and Øystein, B. (2019). Systemic metabolomic profiling of acute myeloid leukemia patients before and during disease-stabilizing treatment based on all-trans retinoic acid, valproic acid, and low-dose chemotherapy. Cells 8 (10), 1229. doi:10.3390/cells8101229
Gu, L., Yi, Z., Zhang, Y., Ma, Z., Zhu, Y., and Gao, J. (2017). Low dose of 2-deoxy-D-glucose kills acute lymphoblastic leukemia cells and reverses glucocorticoid resistance via N-linked glycosylation inhibition under normoxia. Oncotarget 8 (19), 30978–30991. doi:10.18632/oncotarget.16046
Guerra, A. R., Duarte, M. F., and Duarte, I. F. (2018). Targeting tumor metabolism with plant-derived natural products: Emerging trends in cancer therapy. J. Agric. Food Chem. 66 (41), 10663–10685. doi:10.1021/acs.jafc.8b04104
Guerra, F., Arbini, A. A., and Moro, L. (2017). Mitochondria and cancer chemoresistance. Biochim. Biophys. Acta Bioenerg. 1858 (8), 686–699. doi:10.1016/j.bbabio.2017.01.012
Hackl, H., Astanina, K., and Wieser, R. (2017). Molecular and genetic alterations associated with therapy resistance and relapse of acute myeloid leukemia. J. Hematol. Oncol. 10 (1), 51. doi:10.1186/s13045-017-0416-0
Hasanpourghadi, M., Yeng Looi, C., Kumar Pandurangan, A., Sethi, G., Fen Wong, W., and Rais Mustafa, M. (2017). Phytometabolites targeting the Warburg effect in cancer cells: A mechanistic review. Curr. Drug Targets 18 (9), 1086–1094. doi:10.2174/1389450117666160401124842
He, Z., Zhang, S., Ma, D., Fang, Q., Yang, L., Shen, S., et al. (2019). HO-1 promotes resistance to an EZH2 inhibitor through the pRB-E2F pathway: Correlation with the progression of myelodysplastic syndrome into acute myeloid leukemia. J. Transl. Med. 17 (1), 366. doi:10.1186/s12967-019-2115-9
Henkenius, K., Greene, B. H., Barckhausen, C., Hartmann, R., Märken, M., Kaiser, T., et al. (2017). Maintenance of cellular respiration indicates drug resistance in acute myeloid leukemia. Leuk. Res. 62, 56–63. doi:10.1016/j.leukres.2017.09.021
Hernández, J. F., Urueña, C. P., Cifuentes, M. C., Sandoval, T. A., Pombo, L. M., Castañeda, D., et al. (2014). A Petiveria alliacea standardized fraction induces breast adenocarcinoma cell death by modulating glycolytic metabolism. J. Ethnopharmacol. 153 (3), 641–649. doi:10.1016/j.jep.2014.03.013
Hernández, J. F., Urueña, C. P., Sandoval, T. A., Cifuentes, M. C., Formentini, L., Cuezva, J. M., et al. (2017). A cytotoxic Petiveria alliacea dry extract induces ATP depletion and decreases β-F1-ATPase expression in breast cancer cells and promotes survival in tumor-bearing mice. Braz. J. Pharmacogn. 27 (3), 306–314. doi:10.1016/j.bjp.2016.09.008
Herranz-López, M., Losada-Echeberría, M., and Barrajón-Catalán, E. (2018). The multitarget activity of natural extracts on cancer: Synergy and xenohormesis. Med. (Basel) 6 (1), 6. doi:10.3390/medicines6010006
Herst, P. M., Howman, R. A., Neeson, P. J., Berridge, M. V., and Ritchie, D. S. (2011). The level of glycolytic metabolism in acute myeloid leukemia blasts at diagnosis is prognostic for clinical outcome. J. Leukoc. Biol. 89 (1), 51–55. doi:10.1189/jlb.0710417
Hirschhaeuser, F., Sattler, U. G. A., and Mueller-Klieser, W. (2011). Lactate: A metabolic key player in cancer. Cancer Res. 71 (22), 6921–6925. doi:10.1158/0008-5472.CAN-11-1457
Horvath, Z., Saiko, P., Illmer, C., Madlener, S., Hoechtl, T., Bauer, W., et al. (2005). Synergistic action of resveratrol, an ingredient of wine, with Ara-C and tiazofurin in HL-60 human promyelocytic leukemia cells. Exp. Hematol. 33 (3), 329–335. doi:10.1016/j.exphem.2004.11.009
Hoxhaj, G., and Manning, B. D. (2020). The PI3K–AKT network at the interface of oncogenic signalling and cancer metabolism. Nat. Rev. Cancer 20 (2), 74–88. doi:10.1038/s41568-019-0216-7
Hulleman, E., Kazemier, K. M., Holleman, A., VanderWeele, D. J., Rudin, C. M., Broekhuis, M. J., et al. (2009). Inhibition of glycolysis modulates prednisolone resistance in acute lymphoblastic leukemia cells. Blood 113 (9), 2014–2021. doi:10.1182/blood-2008-05-157842
Hwang, D., Kim, M., Park, H., Jeong, M. I., Jung, W., and Kim, B. (2019). Natural products and acute myeloid leukemia: A review highlighting mechanisms of action. Nutrients 11 (5), 1010. doi:10.3390/nu11051010
Iansante, V., Choy, P. M., Fung, S. W., Liu, Y., Chai, J-G., Dyson, J., et al. (2015). PARP14 promotes the Warburg effect in hepatocellular carcinoma by inhibiting JNK1-dependent PKM2 phosphorylation and activation. Nat. Commun. 6, 7882. doi:10.1038/ncomms8882
Icard, P. S., Shulman, S., Farhat, D., Steyaert, J. M., Alifano, M., and Lincet, H. (2018). How the Warburg effect supports aggressiveness and drug resistance of cancer cells? Drug Resist Updat 38, 1–11. doi:10.1016/j.drup.2018.03.001
Jacobsen, K. M., Villadsen, N. L., Torring, T., Nielsen, C. B., Salomon, T., Nielsen, M. M., et al. (2018). APD-containing cyclolipodepsipeptides target mitochondrial function in hypoxic cancer cells. Cell Chem. Biol. 25, 1337–1349.e12. doi:10.1016/j.chembiol.2018.07.010
Jain, A., Katiyar, A., Singh, R., Bakhshi, S., Singh, H., Palanichamy, J. K., et al. (2022). Implications of mitochondrial DNA variants in pediatric B-cell acute lymphoblastic leukemia. Egypt J. Med. Hum. Genet. 23 (1), 133. doi:10.1186/s43042-022-00347-0
Jaiswal, A., Xudong, Z., Zhenyu, J., and Saretzki, G. (2022). Mitochondrial sirtuins in stem cells and cancer. FEBS J. 289 (12), 3393–3415. doi:10.1111/febs.15879
Jayavelu, A. K., Wolf, S., Buettner, F., Alexe, G., Häupl, B., Comoglio, F., et al. (2022). The proteogenomic subtypes of acute myeloid leukemia. Cancer Cell 40 (3), 301–317.e12. doi:10.1016/j.ccell.2022.02.006
Ježek, P. (2020). 2-Hydroxyglutarate in cancer cells. Antioxid. Redox Signal 33 (13), 903–926. doi:10.1089/ars.2019.7902
Jhas, B., Sriskanthadevan, S., Skrtic, M., Sukhai, M. A., Voisin, V., Jitkova, Y., et al. (2013). Metabolic adaptation to chronic inhibition of mitochondrial protein synthesis in acute myeloid leukemia cells. PLoS One 8 (3), e58367. doi:10.1371/journal.pone.0058367
Jiang, N., Zhang, X., Chen, Q., Kantawong, F., Wan, S., Liu, J., et al. (2022). Identification of a mitochondria-related gene signature to predict the prognosis in AML. Front. Oncol. 12, 823831. doi:10.3389/fonc.2022.823831
Jiang, Y., and Nakada, D. (2016). Cell intrinsic and extrinsic regulation of leukemia cell metabolism. Int. J. Hematol. 103 (6), 607–616. doi:10.1007/s12185-016-1958-6
Kim, H. K., Son, S. Y., Oh, J. S., Song, Y. N., Byun, J. M., Koh, Y., et al. (2021). Metabolic profiling during acute myeloid leukemia progression using paired clinical bone marrow serum samples. Metabolites 11 (9), 586. doi:10.3390/metabo11090586
Kominsky, D. J., Klawitter, J., Brown, J. L., Boros, L. G., Melo, J. V., Eckhardt, S. G., et al. (2009). Abnormalities in glucose uptake and metabolism in imatinib-resistant human BCR-ABL-positive cells. Clin. Cancer Res. 15 (10), 3442–3450. doi:10.1158/1078-0432.CCR-08-3291
Kumar, A., and Jaitak, V. (2019). Natural products as multidrug resistance modulators in cancer. Eur. J. Med. Chem. 176, 268–291. doi:10.1016/j.ejmech.2019.05.027
Lagadinou, E. D., Sach, A., Callahan, K., Rossi, R. M., Neering, S. J., Minhajuddin, M., et al. (2013). BCL-2 inhibition targets oxidative phosphorylation and selectively eradicates quiescent human leukemia stem cells. Cell Stem Cell 12 (3), 329–341. doi:10.1016/j.stem.2012.12.013
Lasso, P., Gomez-Cadena, A., Urueña, C., Donda, A., Martinez-Usatorre, A., Barreto, A., et al. (2018). Prophylactic vs. therapeutic treatment with P2Et polyphenol-rich extract has opposite effects on tumor growth. Front. Oncol. 8, 356. doi:10.3389/fonc.2018.00356
Lasso, P., Rojas, L., Arévalo, C., Urueña, C., Murillo, N., Barreto, A., et al. (2022). Tillandsia usneoides extract decreases the primary tumor in a murine breast cancer model but not in melanoma. Cancers (Basel) 14 (21), 5383. doi:10.3390/cancers14215383
Lee, E. A., Angka, L., Rota, S. G., Hanlon, T., Mitchell, A., Hurren, R., et al. (2015). Targeting mitochondria with avocatin B induces selective leukemia cell death. Cancer Res. 75 (12), 2478–2488. doi:10.1158/0008-5472.CAN-14-2676
Li, S., Chen, L., Jin, W., Ma, X., Ma, Y., Dong, F., et al. (2017). Influence of body mass index on incidence and prognosis of acute myeloid leukemia and acute promyelocytic leukemia: A meta-analysis. Sci. Rep. 7 (1), 17998. doi:10.1038/s41598-017-18278-x
Li, T., Fan, G. X., Wang, W., Li, T., and Yuan, Y. K. (2007). Resveratrol induces apoptosis, influences IL-6 and exerts immunomodulatory effect on mouse lymphocytic leukemia both in vitro and in vivo. Int. Immunopharmacol. 7 (9), 1221–1231. doi:10.1016/j.intimp.2007.05.008
Liu, C. J., Jin, Y., and Fan, Z. (2021). The mechanism of Warburg effect-induced chemoresistance in cancer. Front. Oncol. 11, 698023. doi:10.3389/fonc.2021.698023
Liu, S. M., Chen, W., and Wang, J. (2014). Distinguishing between cancer cell differentiation and resistance induced by all-trans retinoic acid using transcriptional profiles and functional pathway analysis. Sci. Rep. 4, 5577. doi:10.1038/srep05577
Lo Presti, C., Fauvelle, F., Mondet, J., and Mossuz, P. (2020). The differential activation of metabolic pathways in leukemic cells depending on their genotype and micro-environmental stress. Metabolomics 16 (1), 13. doi:10.1007/s11306-020-1633-z
Lucas, D. M., Still, P. C., Bueno Perez, L., Grever, M. R., and Douglas Kinghorn, A. (2010). Potential of plant-derived natural products in the treatment of leukemia and lymphoma. Curr. Drug Targets 11 (7), 812–822. doi:10.2174/138945010791320809
Luppi, M., Fabbiano, F., Visani, G., Martinelli, G., and Venditti, A. (2018). Novel agents for acute myeloid leukemia. Cancers (Basel) 10 (11), 429. doi:10.3390/cancers10110429
Ma, J., Liu, B., Yu, D., Zuo, Y., Cai, R., Yang, J., et al. (2019). SIRT3 deacetylase activity confers chemoresistance in AML via regulation of mitochondrial oxidative phosphorylation. Br. J. Haematol. 187 (1), 49–64. doi:10.1111/bjh.16044
Man, C. L., Sun, S. S., Chow, M. K., Gill, H. C., Kwong, H., Leung, Y. L. A. Y., et al. (2014). A novel tescalcin-sodium/hydrogen exchange axis underlying sorafenib resistance in FLT3-ITD+ AML. Blood 123 (16), 2530–2539. doi:10.1182/blood-2013-07-512194
Marcucci, F. R., and Cristiano, R. (2021). Glycolysis-induced drug resistance in tumors-A response to danger signals? Neoplasia 23 (2), 234–245. doi:10.1016/j.neo.2020.12.009
Marin, J. J. G., Briz, O., Rodríguez-Macias, G., Díez-Martín, J. L., and Macias, R. I. R. (2016). Role of drug transport and metabolism in the chemoresistance of acute myeloid leukemia. Blood Rev. 30 (1), 55–64. doi:10.1016/j.blre.2015.08.001
Mattes, K., Vellenga, E., and Schepers, H. (2019). Differential redox-regulation and mitochondrial dynamics in normal and leukemic hematopoietic stem cells: A potential window for leukemia therapy. Crit. Rev. Oncol./Hematol. 144, 102814. doi:10.1016/j.critrevonc.2019.102814
Moschoi, R., Imbert, V., Nebout, M., Chiche, J., Mary, D., Prebet, T., et al. (2016). Protective mitochondrial transfer from bone marrow stromal cells to acute myeloid leukemic cells during chemotherapy. Blood 128 (2), 253–264. doi:10.1182/blood-2015-07-655860
Mougiakakos, D. (2019). The induction of a permissive environment to promote T cell immune evasion in acute myeloid leukemia: The metabolic perspective. Front. Oncol. 9, 1166. doi:10.3389/fonc.2019.01166
Nepstad, I., Hatfield, K. J., Grønningsæter, I. S., and Reikvam, H. (2020). The PI3K-Akt-mTOR signaling pathway in human acute myeloid leukemia (AML) cells. Int. J. Mol. Sci. 21 (8), 2907. doi:10.3390/ijms21082907
Newman, D. J., and Cragg, G. M. (2016). Natural products as sources of new drugs from 1981 to 2014. J. Nat. Prod. 79 (3), 629–661. doi:10.1021/acs.jnatprod.5b01055
Ohayon, D., De Chiara, A., Chapuis, N., Candalh, C., Mocek, J., Ribeil, J. A., et al. (2016). Cytoplasmic proliferating cell nuclear antigen connects glycolysis and cell survival in acute myeloid leukemia. Sci. Rep. 6, 35561. doi:10.1038/srep35561
Okamoto, S., Miyano, K., Kitakaze, K., Kato, H., Yamauchi, A., Kajikawa, M., et al. (2022). Coculture in vitro with endothelial cells induces cytarabine resistance of acute myeloid leukemia cells in a VEGF-A/VEGFR-2 signaling-independent manner. Biochem. Biophys. Res. Commun. 587, 78–84. doi:10.1016/j.bbrc.2021.11.090
O’Sullivan, D., Sanin, D. E., Pearce, E. J., and Pearce, E. L. (2019). Metabolic interventions in the immune response to cancer. Nat. Rev. Immunol. 19 (5), 324–335. doi:10.1038/s41577-019-0140-9
Panina, S. B., Baran, N., Brasil da Costa, F. H., Konopleva, M., and Kirienko, N. V. (2019). A mechanism for increased sensitivity of acute myeloid leukemia to mitotoxic drugs. Cell Death Dis. 10 (8), 617. doi:10.1038/s41419-019-1851-3
Panina, S. B., Pei, J., Baran, N., Konopleva, M., and Kirienko, N. V. (2020). Utilizing synergistic potential of mitochondria-targeting drugs for leukemia therapy. Front. Oncol. 10, 435. doi:10.3389/fonc.2020.00435
Patel, C. H., Leone, R. D., Horton, M. R., and Powell, J. D. (2019). Targeting metabolism to regulate immune responses in autoimmunity and cancer. Nat. Rev. Drug Discov. 18 (9), 669–688. doi:10.1038/s41573-019-0032-5
Pavlova, N. N., and Thompson, C. B. (2016). The emerging hallmarks of cancer metabolism. Cell Metab. 23 (1), 27–47. doi:10.1016/j.cmet.2015.12.006
Polak, R., de Rooij, B., Pieters, R., and den Boer, M. L. (2015). B-cell precursor acute lymphoblastic leukemia cells use tunneling nanotubes to orchestrate their microenvironment. Blood 126 (21), 2404–2414. doi:10.1182/blood-2015-03-634238
Porporato, P. E., Filigheddu, N., Pedro, J. M. B. S., Kroemer, G., and Galluzzi, L. (2018). Mitochondrial metabolism and cancer. Cell Res. 28 (3), 265–280. doi:10.1038/cr.2017.155
Prieto, K., Cao, Y., Mohamed, E., Trillo-Tinoco, J., Sierra, R. A., Urueña, C., et al. (2019). Polyphenol-rich extract induces apoptosis with immunogenic markers in melanoma cells through the ER stress-associated kinase PERK. Cell Death Discov. 5, 134. doi:10.1038/s41420-019-0214-2
Raimondi, V., Ciotti, G., Gottardi, M., and Ciccarese, F. (2022). 2-Hydroxyglutarate in acute myeloid leukemia: A journey from pathogenesis to therapies. Biomedicines 10 (6), 1359. doi:10.3390/biomedicines10061359
Ryu, M. J., Han, J., Kim, S. J., Lee, M. J., Ju, X., Lee, Y. L., et al. (2019). PTEN/AKT signaling mediates chemoresistance in refractory acute myeloid leukemia through enhanced glycolysis. Oncol. Rep. 42 (5), 2149–2158. doi:10.3892/or.2019.7308
Saito, K., Zhang, Q., Yang, H., Yamatani, K., Ai, T., Ruvolo, V., et al. (2021). Exogenous mitochondrial transfer and endogenous mitochondrial fission facilitate AML resistance to OxPhos inhibition. Blood Adv. 5 (20), 4233–4255. doi:10.1182/bloodadvances.2020003661
Saliba, A. N., John, A., and Kaufmann, S. H. (2021). Resistance to venetoclax and hypomethylating agents in acute myeloid leukemia. Cancer Drug Resist 4 (1), 125–142. doi:10.20517/cdr.2020.95
Sandoval, T. A., Urueña, C. P., Llano, M., Gómez-Cadena, A., Hernández, J. F., Sequeda, L. G., et al. (2016). Standardized extract from Caesalpinia spinosa is cytotoxic over cancer stem cells and enhance anticancer activity of doxorubicin. Am. J. Chin. Med. 44 (8), 1693–1717. doi:10.1142/S0192415X16500956
Santander, S. P., Hernández, J. F., Cifuentes, B. C., Aoki, M., Moins-Teisserenc, H., and Fiorentino, S. (2012). Immunomodulatory effects of Aqueous and organic fractions from Petiveria alliacea on human dendritic cells. Am. J. Chin. Med. 40 (4), 833–844. doi:10.1142/S0192415X12500620
Sarwar, M. S., Zhang, H-J., and Tsang, S. W. (2018). Perspectives of plant natural products in inhibition of cancer invasion and metastasis by regulating multiple signaling pathways. Curr. Med. Chem. 25 (38), 5057–5087. doi:10.2174/0929867324666170918123413
Sasca, D., Szybinski, J., Schüler, A., Shah, V., Heidelberger, J., Haehnel, P. S., et al. (2019). NCAM1 (CD56) promotes leukemogenesis and confers drug resistance in AML. Blood 133 (21), 2305–2319. doi:10.1182/blood-2018-12-889725
Schmidt, D. R., Patel, R., Kirsch, D. G., Lewis, C. A., Vander Heiden, M. G., and Locasale, J. W. (2021). Metabolomics in cancer research and emerging applications in clinical oncology. CA Cancer J. Clin. 71 (4), 333–358. doi:10.3322/caac.21670
Schraw, J. M., Junco, J. J., Brown, A. L., Scheurer, M. E., Rabin, K. R., and Lupo, P. J. (2019). Metabolomic profiling identifies pathways associated with minimal residual disease in childhood acute lymphoblastic leukaemia. EBioMedicine 48, 49–57. doi:10.1016/j.ebiom.2019.09.033
Shafat, M. S., Gnaneswaran, B., Bowles, K. M., and Rushworth, S. A. (2017). The bone marrow microenvironment – home of the leukemic blasts. Blood Rev. 31 (5), 277–286. doi:10.1016/j.blre.2017.03.004
Shallis, R. M., Wang, R., Davidoff, A., Ma, X., and Zeidan, A. M. (2019). Epidemiology of acute myeloid leukemia: Recent progress and enduring challenges. Blood Rev. 36, 70–87. doi:10.1016/j.blre.2019.04.005
Shrotriya, S., Deep, G., Lopert, P., Patel, M., Agarwal, R., and Agarwal, C. (2015). Grape seed extract targets mitochondrial electron transport chain complex III and induces oxidative and metabolic stress leading to cytoprotective autophagy and apoptotic death in human head and neck cancer cells. Mol. Carcinog. 54 (12), 1734–1747. doi:10.1002/mc.22246
Silkjaer, T., Nørgaard, J. M., Aggerholm, A., Ebbesen, L. H., Kjeldsen, E., Hokland, P., et al. (2013). Characterization and prognostic significance of mitochondrial DNA variations in acute myeloid leukemia. Eur. J. Haematol. 90 (5), 385–396. doi:10.1111/ejh.12090
Song, K., Li, M., Xu, X., Xuan, L., Huang, G., and Liu, Q. (2016). Resistance to chemotherapy is associated with altered glucose metabolism in acute myeloid leukemia. Oncol. Lett. 12 (1), 334–342. doi:10.3892/ol.2016.4600
Song, K., Li, M., Xu, X. J., Xuan, L., Huang, G. N., Song, X. L., et al. (2014). HIF-1α and GLUT1 gene expression is associated with chemoresistance of acute myeloid leukemia. Asian Pac J. Cancer Prev. 15 (4), 1823–1829. doi:10.7314/apjcp.2014.15.4.1823
Sossa, C. A., Abello, V., Salazar, L., Rosales, M., Idrobo, H., Reyes-Castellanos, J., et al. (2021). AML-425: Acute myeloid leukemia: A multicenter experience in Colombia. Clin. Lymphoma Myeloma Leuk. 21, 2. doi:10.1016/S2152-2650(21)01735-3
Stäubert, C., Bhuiyan, H., Lindahl, A., Broom, O. J., Zhu, Y., Islam, S., et al. (2015). Rewired metabolism in drug-resistant leukemia cells: A metabolic switch hallmarked by reduced dependence on exogenous glutamine. J. Biol. Chem. 290 (13), 8348–8359. doi:10.1074/jbc.M114.618769
Stevens, B. M., Jones, C. L., Pollyea, D. A., Culp-Hill, R., D’Alessandro, A., Winters, A., et al. (2020). Fatty acid metabolism underlies venetoclax resistance in acute myeloid leukemia stem cells. Nat. Cancer 1 (12), 1176–1187. doi:10.1038/s43018-020-00126-z
Stevens, J. F., Revel, J. S., and Maier, C. S. (2018). Mitochondria-centric review of polyphenol bioactivity in cancer models. Antioxid. Redox Signal 29 (16), 1589–1611. doi:10.1089/ars.2017.7404
Stuani, L., Sabatier, M., Saland, E., Cognet, G., Poupin, N., Bosc, C., et al. (2021). Mitochondrial metabolism supports resistance to IDH mutant inhibitors in acute myeloid leukemia. J. Exp. Med. 218 (5), e20200924. doi:10.1084/jem.20200924
Sun, Z., Zhang, W., and Li, Q. (2017). miR-125a suppresses viability and glycolysis and induces apoptosis by targeting Hexokinase 2 in laryngeal squamous cell carcinoma. Cell Biosci. 7, 51. doi:10.1186/s13578-017-0178-y
Swerdlow, S. H., Campo, E., Harris, N. L., Jaffe, E. S., Pileri, S. A., Stein, H., et al. (2017). WHO Classification of tumors for haematopoietic and lymphoid tissues. Lyon, France: International Agency for Research on Cancer (IARC).
Taghvimi, S., Abbaszadeh, S., Banan, F. B., Fard, E. S., Jamali, Z., Najafabadi, M. A., et al. (2022). LncRNAs roles in chemoresistance of cancer cells. Curr. Mol. Med. 22 (8), 691–702. doi:10.2174/1566524021666211027090515
Tao, F., Zhang, Y., and Zhang, Z. (2019). The role of herbal bioactive components in mitochondria function and cancer therapy. Evid. Based Complement. Altern. Med. 2019, 3868354. doi:10.1155/2019/3868354
Thol, F. (2018). Can we forecast induction failure in acute myeloid leukemia? Haematologica 103 (3), 375–377. doi:10.3324/haematol.2018.187575
Urueña, C., Ballesteros-Ramírez, R., Gomez-Cadena, A., Barreto, A., Prieto, K., Quijano, S., et al. (2022). Randomized double-blind clinical study in patients with COVID-19 to evaluate the safety and efficacy of a phytomedicine (P2Et). Front. Med. 9, 991873. doi:10.3389/fmed.2022.991873
Urueña, C., Cifuentes, C., Castañeda, D., Arango, A., Kaur, P., Asea, A., et al. (2008). Petiveria alliacea extracts uses multiple mechanisms to inhibit growth of human and mouse tumoral cells. BMC Complement. Altern. Med. 8, 60. doi:10.1186/1472-6882-8-60
Urueña, C., Mancipe, J., Hernandez, J., Castañeda, D., Pombo, L., Gomez, A., et al. (2013). Gallotannin-rich Caesalpinia spinosa fraction decreases the primary tumor and factors associated with poor prognosis in a murine breast cancer model. BMC Complement. Altern. Med. 13, 74. doi:10.1186/1472-6882-13-74
Urueña, C., Sandoval, T. A., Lasso, P., Tawil, M., Barreto, A., Torregrosa, L., et al. (2020). Evaluation of chemotherapy and P2Et extract combination in ex-vivo derived tumor mammospheres from breast cancer patients. Sci. Rep. 10 (1), 19639. doi:10.1038/s41598-020-76619-9
Valin, J., Martinez, E., Huhn, J., Di, M., Orsola, S., Stephen, J., et al. (2022). Acute myeloid leukemia cells rely on the glycolytic enzyme PGK1 to support energy production and amino acid metabolism. Blood 140, 8694–8695. doi:10.1182/blood-2022-166659
Vander Heiden, M. G., and Deberardinis, R. J. (2017). Understanding the intersections between metabolism and cancer biology. Cell 168 (4), 657–669. doi:10.1016/j.cell.2016.12.039
Vaughan, R. A., Garcia-Smith, R., Dorsey, J., Griffith, J. K., Bisoffi, M., and Trujillo, K. A. (2013). Tumor necrosis factor alpha induces Warburg-like metabolism and is reversed by anti-inflammatory curcumin in breast epithelial cells. Int. J. Cancer 133 (10), 2504–2510. doi:10.1002/ijc.28264
Vellinga, T. T., De Boer, V. C. J., Fatrai, S., Van Schelven, S., Trumpi, K., Verheem, A., et al. (2015). SIRT1/PGC1a-Dependent increase in oxidative phosphorylation supports chemotherapy resistance of colon cancer. Clin. Cancer Res. 21 (12), 2870–2879. doi:10.1158/1078-0432.CCR-14-2290
Vitkevičienė, A., Janulis, V., Žučenka, A., Borutinskaitė, V., Kaupinis, A., Valius, M., et al. (2019). Oxidative phosphorylation inhibition induces anticancerous changes in therapy-resistant–acute myeloid leukemia patient cells. Mol. Carcinog. 58 (11), 2008–2016. doi:10.1002/mc.23092
Vo, T. T., Ryan, J., Carrasco, R., Neuberg, D., Rossi, D. J., Stone, R. M., et al. (2012). Relative mitochondrial priming of myeloblasts and normal HSCs determines chemotherapeutic success in AML. Cell 151 (2), 344–355. doi:10.1016/j.cell.2012.08.038
Wang, B., Wang, X., Hou, D., Huang, Q., Zhan, W., Chen, C., et al. (2019). Exosomes derived from acute myeloid leukemia cells promote chemoresistance by enhancing glycolysis-mediated vascular remodeling. J. Cell Physiol. 234 (7), 10602–10614. doi:10.1002/jcp.27735
Wang, G., Wang, J. J., Guan, R., Du, L., Gao, J., and Fu, X. L. (2017). Strategies to target glucose metabolism in tumor microenvironment on cancer by flavonoids. Nutr. Cancer 69 (4), 534–554. doi:10.1080/01635581.2017.1295090
Wang, J., Liu, X., Qiu, Y., Shi, Y., Cai, J., Wang, B., et al. (2018). Cell adhesion-mediated mitochondria transfer contributes to mesenchymal stem cell-induced chemoresistance on T cell acute lymphoblastic leukemia cells. Hematol. Oncol. 11 (1), 11. doi:10.1186/s13045-018-0554-z
Wilkinson, E. M., Ilhan, Z. E., and Herbst-Kralovetz, M. M. (2018). Microbiota–drug interactions: Impact on metabolism and efficacy of therapeutics. Maturitas 112, 53–63. doi:10.1016/j.maturitas.2018.03.012
Winer, E. S., and Stone, R. M. (2019). Novel therapy in acute myeloid leukemia (AML): Moving toward targeted approaches. Ther. Adv. Hematol. 10, 2040620719860645. doi:10.1177/2040620719860645
Wu, K., Guo, C., Li, Y., Yang, J., Zhou, Q., Cheng, S., et al. (2021). MicroRNA-18a-5p regulates the Warburg effect by targeting hypoxia-inducible factor 1α in the K562/ADM cell line. Exp. Ther. Med. 22 (4), 1069. doi:10.3892/etm.2021.10503
Xia, Y., Yang, W., Bu, W., Ji, H., Zhao, X., Zheng, Y., et al. (2013). Differential regulation of c-jun protein plays an instrumental role in chemoresistance of cancer cells. J. Biol. Chem. 288 (27), 19321–19329. doi:10.1074/jbc.M113.475442
Xiao, X., Yang, J., Li, R., Liu, S., Xu, Y., Zheng, W., et al. (2013). Deregulation of mitochondrial ATPsyn-β in acute myeloid leukemia cells and with increased drug resistance. PLoS One 8 (12), e83610. doi:10.1371/journal.pone.0083610
Xu, D., Jin, J., Yu, H., Zhao, Z., Ma, D., Zhang, C., et al. (2017). Chrysin inhibited tumor glycolysis and induced apoptosis in hepatocellular carcinoma by targeting hexokinase-2. J. Exp. Clin. Cancer Res. 36 (1), 44. doi:10.1186/s13046-017-0514-4
Xu, Z., Yan, Y., Li, Z., Qian, L., and Gong, Z. (2016). The antibiotic drug tigecycline: A focus on its promising anticancer properties. Front. Pharmacol. 7, 473. doi:10.3389/fphar.2016.00473
Yan, D., Franzini, A., Pomicter, A. D., Halverson, B. J., Antelope, O., Mason, C. C., et al. (2021). SIRT5 is a druggable metabolic vulnerability in acute myeloid leukemia. Blood cancer Discov. 2 (3), 266–287. doi:10.1158/2643-3230.BCD-20-0168
Yang, J., Xiao, X., Li, R., Li, Z., Deng, M., and Zhang, G. (2016). Hypermethylation of CpG sites at the promoter region is associated with deregulation of mitochondrial ATPsyn-β and chemoresistance in acute myeloid leukemia. Cancer Biomark. 16 (1), 81–88. doi:10.3233/CBM-150543
Ye, H., Adane, B., Khan, N., Alexeev, E., Nusbacher, N., Minhajuddin, M., et al. (2018). Subversion of systemic glucose metabolism as a mechanism to support the growth of leukemia cells. Cancer Cell 34 (4), 659–673.e6. doi:10.1016/j.ccell.2018.08.016
Yeung, C. C. S., and Radich, J. (2017). Predicting chemotherapy resistance in AML. Curr. Hematol. Malig. Rep. 12 (6), 530–536. doi:10.1007/s11899-017-0378-x
Yeung, M., Hurren, R., Nemr, C., Wang, X., Hershenfeld, S., Gronda, M., et al. (2015). Mitochondrial DNA damage by bleomycin induces AML cell death. Apoptosis 20 (6), 811–820. doi:10.1007/s10495-015-1119-z
Yin, J., Yin, Q., Liang, B., Mi, R., Ai, H., Chen, L., et al. (2020). Chrysophanol suppresses growth and metastasis of T cell acute lymphoblastic leukemia via miR-9/PD-L1 axis. Naunyn-Schmiedeberg's archives Pharmacol. 393 (2), 273–286. doi:10.1007/s00210-019-01778-0
You, R., Wang, B., Chen, P., Zheng, X., Hou, D., Wang, X., et al. (2022). Metformin sensitizes AML cells to chemotherapy through blocking mitochondrial transfer from stromal cells to AML cells. Cancer Lett. 532, 215582. doi:10.1016/j.canlet.2022.215582
Yu, L., Chen, X., Sun, X., Wang, L., and Chen, S. (2017). The glycolytic switch in tumors: How many players are involved? J. Cancer 8 (17), 3430–3440. doi:10.7150/jca.21125
Yuan, R., Hou, Y., Sun, W., Yu, J., Liu, X., Niu, Y., et al. (2017). Natural products to prevent drug resistance in cancer chemotherapy: A review. Ann. N. Y. Acad. Sci. 1401 (1), 19–27. doi:10.1111/nyas.13387
Zhang, L., Zhao, Q., Cang, H., Wang, Z., Hu, X., Pan, R., et al. (2022). Acute myeloid leukemia cells educate mesenchymal stromal cells toward an adipogenic differentiation propensity with leukemia promotion capabilities. Adv. Sci. (Weinh). 9 (16), 2105811. doi:10.1002/advs.202105811
Zhang, S., Zhou, L., Zhang, M., Wang, Y., Wang, M., Du, J., et al. (2020). Berberine maintains the neutrophil N1 phenotype to reverse cancer cell resistance to doxorubicin. Front. Pharmacol. 10, 1658. doi:10.3389/fphar.2019.01658
Zhang, Y., Liu, Y., and Xu, X. (2018). Knockdown of LncRNA-UCA1 suppresses chemoresistance of pediatric AML by inhibiting glycolysis through the microRNA-125a/hexokinase 2 pathway. J. Cell Biochem. 119 (7), 6296–6308. doi:10.1002/jcb.26899
Zhe, N., Wang, J., Chen, S., Lin, X., Chai, Q., Zhang, Y., et al. (2015). Heme oxygenase-1 plays a crucial role in chemoresistance in acute myeloid leukemia. Hematology 20 (7), 384–391. doi:10.1179/1607845414Y.0000000212
Zhu, Y., Liu, Z., Wan, Y., Zou, L., Liu, L., Ding, S., et al. (2022). PARP14 promotes the growth and glycolysis of acute myeloid leukemia cells by regulating HIF-1α expression. Clin. Immunol. 242, 109094. doi:10.1016/j.clim.2022.109094
Glossary
Keywords: resistance, acute leukemias, metabolism, natural products, botanical drugs
Citation: Arévalo CM, Cruz-Rodriguez N, Quijano S and Fiorentino S (2023) Plant-derived extracts and metabolic modulation in leukemia: a promising approach to overcome treatment resistance. Front. Mol. Biosci. 10:1229760. doi: 10.3389/fmolb.2023.1229760
Received: 27 May 2023; Accepted: 30 June 2023;
Published: 13 July 2023.
Edited by:
Ann Saada, Hebrew University of Jerusalem, IsraelReviewed by:
Sovannarith Korm, Marine Biological Laboratory (MBL), United StatesXiuling Cao, Zhejiang Agriculture and Forestry University, China
Copyright © 2023 Arévalo, Cruz-Rodriguez, Quijano and Fiorentino. This is an open-access article distributed under the terms of the Creative Commons Attribution License (CC BY). The use, distribution or reproduction in other forums is permitted, provided the original author(s) and the copyright owner(s) are credited and that the original publication in this journal is cited, in accordance with accepted academic practice. No use, distribution or reproduction is permitted which does not comply with these terms.
*Correspondence: Susana Fiorentino, c3VzYW5hLmZpb3JlbnRpbm9AamF2ZXJpYW5hLmVkdS5jbw==