- 1Department of Nuclear Medicine, The Affiliated Hospital of Southwest Medical University, Luzhou, Sichuan, China
- 2Nuclear Medicine and Molecular Imaging Key Laboratory of Sichuan Province, Luzhou, Sichuan, China
- 3Academician (Expert) Workstation of Sichuan Province, Luzhou, Sichuan, China
Theranostic in nuclear medicine combines diagnostic imaging and internal irradiation therapy using different therapeutic nuclear probes for visual diagnosis and precise treatment. GLP-1R is a popular receptor target in endocrine diseases, non-alcoholic steatohepatitis, tumors, and other areas. Likewise, it has also made breakthroughs in the development of molecular imaging. It was recognized that GLP-1R imaging originated from the study of insulinoma and afterwards was expanded in application including islet transplantation, pancreatic β-cell mass measurement, and ATP-dependent potassium channel-related endocrine diseases. Fortunately, GLP-1R molecular imaging has been involved in ischemic cardiomyocytes and neurodegenerative diseases. These signs illustrate the power of GLP-1R molecular imaging in the development of medicine. However, it is still limited to imaging diagnosis research in the current molecular imaging environment. The lack of molecular-targeted therapeutics related report hinders its radiology theranostic. In this article, the current research status, challenges, and emerging opportunities for GLP-1R molecular imaging are discussed in order to open a new path for theranostics and to promote the evolution of molecular medicine.
1 Introduction
GLP-1R belongs to the subfamily of G protein-coupled receptors (Holst, 2007). It is widely distributed in pancreatic islets, the gastrointestinal tract, the heart, kidney, liver, brain, and other tissue organs (Pabreja et al., 2014). Various physiological functions can perform when activated by GLP-1 or synthetic agonists. In addition to its principal activity in islet cells, ghrelin inhibits gastric secretion and gastrointestinal motility, delays gastric emptying, increases satiety and decreases food intake in gastrointestinal tissues (Brubaker and Drucker, 2002). Similarly, GLP-1R protects nerve cells, fights loss of appetite, and enhances memory. In the cardiovascular aspect, it can improve cardiovascular function and reduce inflammation (Laviola et al., 2012) (Figure 1). Obviously, such a powerful physiological function and general distribution characteristics make it a popular receptor target in recent years.
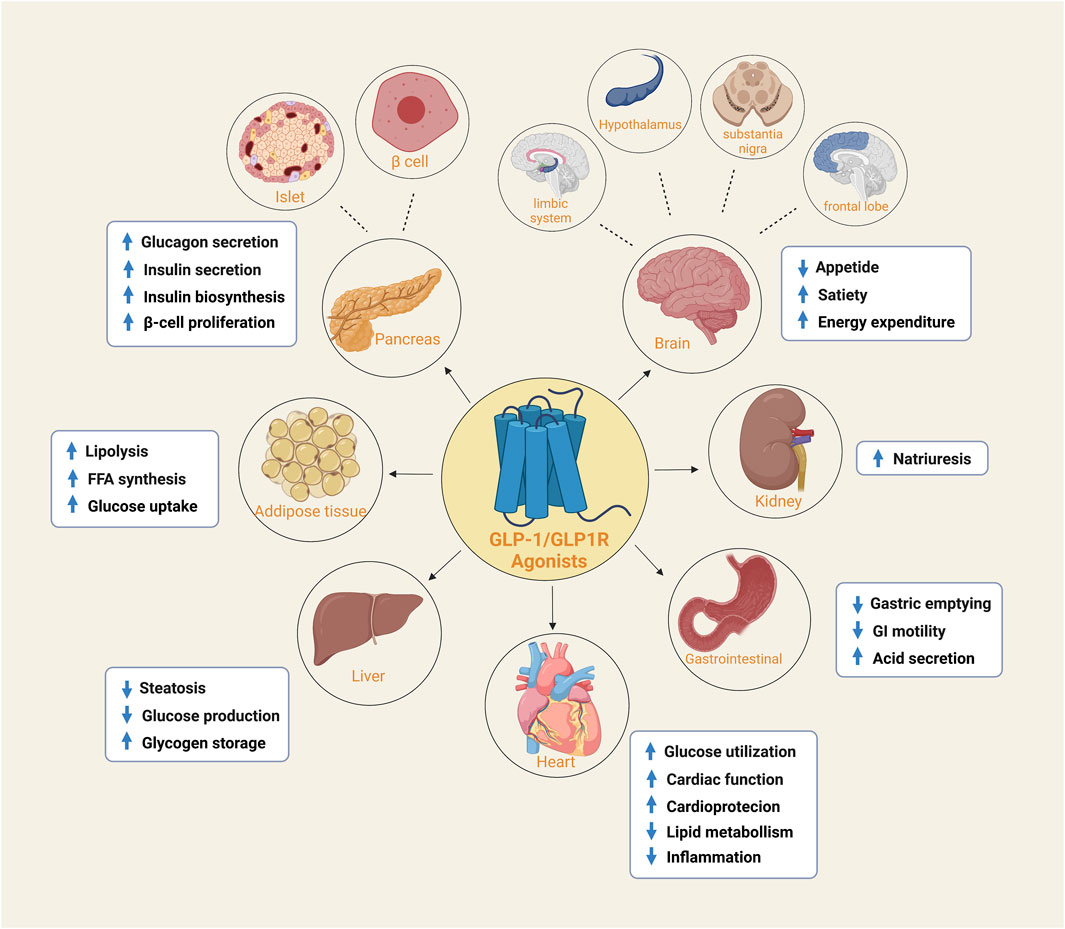
FIGURE 1. The expression of GLP-1/GLP-1R agonists in different organs and tissues. GLP1R is widely distributed and is expressed in both islets and beta cells of the pancreas, adipose tissue, heart, liver, kidney and gastroduodenum. It is also found in the frontal lobe, substantia nigra, hypothalamus and limbic system of the brain. They perform their respective functions in different tissue sites.
GLP1, the natural ligand of GLP-1R, is rapidly degraded and inactivated by dipeptidyl peptidase IV (DPP-IV) in vivo. Based on this, the advent of GLP-1 analog opens a new direction for molecular imaging. Except the GLP1 analogue exendin-3 and its antagonist exendin-(9–39) are involved in GLP-1R molecular imaging studies as GLP-1 ligands. The most successful and widely used ligand for current GLP1R molecular imaging is still exendin-4. As the first generation GLP1 analog, it is a 39-amino acid peptide structure, which was discovered and extracted from Gila monster saliva and shared 53% identity with human GLP1 (Joosten et al., 2018). Meanwhile, it has a longer half-life (9.57 h) than GLP1 (about 2 min) because it lacks a specific site for DPP-IV hydrolase and is resistant to the rapid enzymatic digestion of DPP-IV (Zhang and Chen, 2012). With this natural advantage, it has become the core probe for GLP-1R molecular imaging.
In the initial exploration of molecular imaging of GLP-1R, Korner (Korner et al., 2007) first labeled the radionuclide 125I on exendin-4. Excitingly, the biodistribution results demonstrated that the receptor is highly expressed in insulinoma, gastrinoma, pheochromocytoma, and medullary thyroid carcinoma (MTC). In addition, intracranial tumors such as meningiomas and astrocytomas have upregulated receptor manifestations. Among those neoplasms, the highest expression in benign insulinomas is consistent with Reubi’s autoradiography study (Reubi and Waser, 2003). Thus, the publication of these two findings were promoted directly with the development of molecular imaging on insulinoma. Although exendin-4, the most mature molecular probe for GLP-1R imaging, has been successful in insulinoma studies. We have aware that dependence on this tracer is currently the biggest challenge. The advancement of more comprehensive GLP-1R molecular imaging agents will also become an emerging opportunity. Fortunately, in recent years, research on it has gradually expanded to other fields such as medullary thyroid cancer, myocardial ischaemia, islet transplantation, islet beta cell mass monitoring, congenital hyperinsulinemia and neurodegenerative diseases (Figure 2). This article reviews the past and present development in GLP-1R molecular imaging. It puts forward the challenges encountered at this stage and new opportunities in the future, aiming to lay a solid theoretical basis for long-term theranostics.
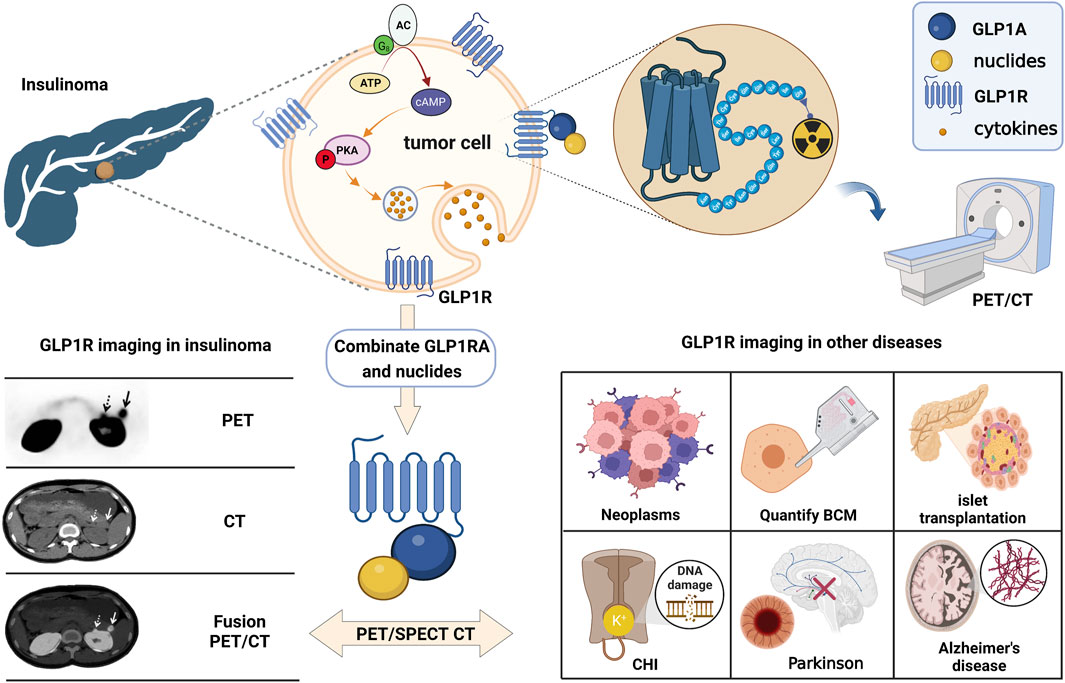
FIGURE 2. The schematic of GLP-1R molecular imaging. GLP1 analogues can bind competitively with GLP1 to GLP-1R targets and achieve GLP-1R molecular imaging by labelling with different radionuclides. Many studies of GLP-1R molecular imaging have been performed in tumours such as insulinoma, medullary thyroid cancer, myocardial ischaemia, islet transplantation, pancreatic beta-cell mass monitoring, congenital hyperinsulinemia and neurodegenerative diseases.
2 GLP-1R molecular imaging in insulinoma
Even before the discovery of exendin-4, its homolog exendin-3 was successfully synthesized by 125I labeling and utilized in two models of insulinoma (NEDH rats and RINm 5F cells) (Gotthardt et al., 2002), which opened the chapter of GLP-1R imaging for 21 years. During this period, molecular imaging in insulinomas has matured, thanks to the exploration of exendin-4. It acts as a double-edged sword, pushing GLP-1R forward while relying on it so that all current molecular imaging studies of insulinoma focus on exendin-4 and its derivatives. This section summarizes the previous 21 years of GLP-1R molecular imaging in insulinoma. Insulinoma is the most common cause of endogenous hyperinsulinemic hypoglycemia (EHH) (Mori et al., 2020). As a result, detecting and precisely localizing the lesion prior to surgery is essential for surgical treatment (Falconi et al., 2016). However, the sensitivity of conventional imaging techniques for insulinoma is low, and the imaging is blurred. These factors affect the detection rate of lesions. Therefore, the molecular imaging study of GLP-1R was carried out.
2.1 Preclinical study of GLP-1 receptor molecular imaging in insulinoma
Initially (Wild et al., 2006), Wild was the first to successfully visualize insulinomas in preclinical studies utilizing Ahx as a linker between DTPA and peptide by c-terminal extension of the Lys side chain coupling of exendin-4 (Lys40NH2 modifies Exendin-4), a landmark for GLP-1R molecular visualization. Wicki et al. (2007) later observed a 94% reduction in tumor volume within 8 days after injecting 28 MBq of radioactive peptide under the same molecular tracer. Such positive results raise expectations for driving molecular imaging in the theranostic of insulinoma. Nonetheless, the 111In is costly and has a significant radiation burden on patients. Therefore, an alternative radionuclide for SPECT/CT imaging, 99mTc, appeared in comparison research with 111In at Wild et al. (2010). The synthesized molecular compound that used exendin-4 as a substrate also demonstrated an ideal tumor background ratio and insulinoma sensitivity, making it an ideal choice for GLP-1R molecular imaging.
Regrettably, the above studies were all SPECT/CT imaging of nuclide markers until Wu’s two results (Wu et al., 2011; Wu et al., 2014) on 64Cu-exendin-4 PET/CT molecular imaging of insulinomas opened the window to new horizons of GLP-1R molecular imaging again. The ligands Cys-exendin-4 were respectively added to phosphate buffers containing BaMalSar and Mal2Sar at pH 7.0, and the coupling products BaMalSar-exendin-4 and Mal2Sar-(exendin-4)2 were purified by HPLC with stirring at room temperature in yields greater than 90% (Figure 3). Not only did they visualize insulinomas using this molecular imaging, but also successfully visualized portal islet transplantation models. However, negative findings could also reveal that the average effective dose of 64Cu was nearly ten times higher than that of 68Ga-exendin-4, which significantly increased the radiation dose and limited its application and translation. It suggests that 68Ga may be a nuclide probe with great potential for molecular imaging of GLP-1R.
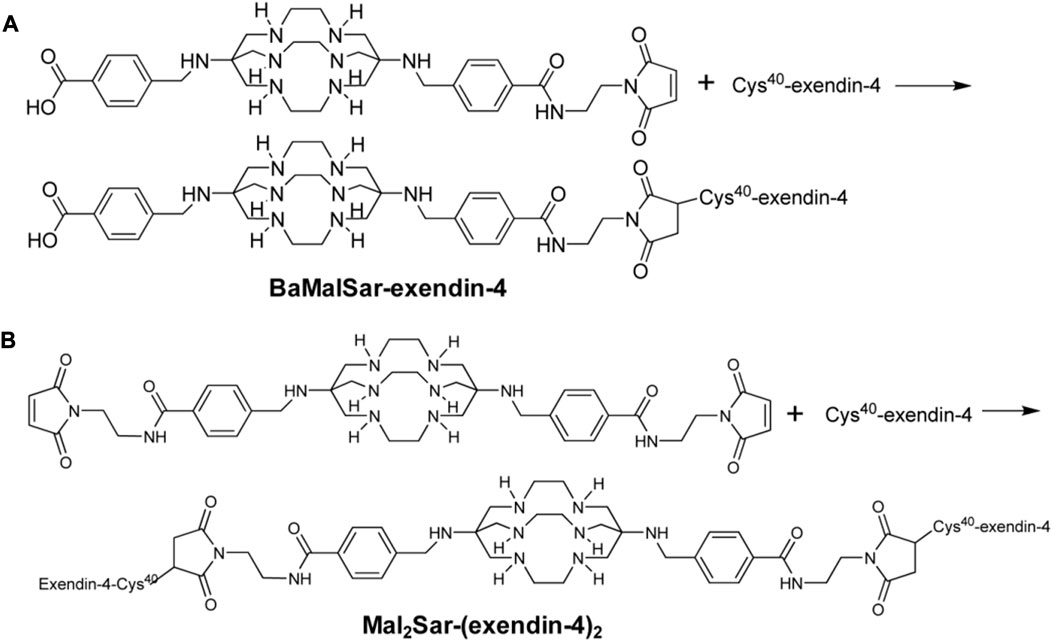
FIGURE 3. Synthesis scheme of monomeric and dimeric exendin-4 analogs. Two novel peptide structures BaMalSar-exendin-4 (A) and Mal2Sar-(exendin-4)2 (B) were respectively prepared by coupling the ligand Cys-exendin-4 with BaMalSar and Mal2Sar, in over 90% yield.
Before beginning insulinoma research with 68Ga-exendin-4, several results (Kiesewetter et al., 2012a; Kiesewetter et al., 2012b; Keliher et al., 2012; Wu et al., 2013; Yue et al., 2013; Xu et al., 2015; Li et al., 2020; Murakami et al., 2021a) with 18F indicated that 18F-exendin-4 is critical for developing molecular imaging. Surprisingly, in these 18F experiments, we saw one of the most significant drawbacks of current GLP-1R molecular imaging, namely, high renal uptake, which can lead to missed or misdiagnosed lesions located in the tail of the pancreas next to the kidney. Actually, the high renal radiation burden emerged early when Vegt et al. (2008) and Wild et al. (2010) reduced renal uptake by adding the albumin fragment FRALB, gelofusine, and polyglutamic acid infusion. However, the tedious and complicated preparation constrained subsequent development. In contrast, the 18F-exendin-4 study was performed by synthesizing new GLP-1R derivatives through diverse modifications of different amino acid structural sites of Exendin-4 to obtain a lower renal load developer. We have seen that successfully preparing different modified derivatives, including micron and nanomaterials [FBEM (Kiesewetter et al., 2012a), TCO (Keliher et al., 2012), TTCO (Wu et al., 2013), FPenM (Yue et al., 2013), and PEG (Li et al., 2020; Murakami et al., 2021a)], can reduce renal uptake to a certain extent yet the process is still complicated. It is possible that finding new analogs of GLP-1 with higher homology and a nephroprotective native structure will be a target for future molecular imaging works. Moreover, perhaps the second-generation GLP-1 analog semaglutide will be a new option.
Subsequently, the six preclinical studies (Brom et al., 2010; Sel et al., 2014; Bauman et al., 2015; Kaeppeli et al., 2019; Zhang et al., 2019) of 68Ga-exendin-4 held promise for further clinical translation and laid a solid foundation for its future unassailable position in insulinoma molecular imaging. In addition to the most common DOTA, using different chelators, including NOTA, NODAGA, DO3A, and DFO, has also compensated for the lack of singularity of other nuclide markers in the past. It was the first time in the Kaeppeli study (Kaeppeli et al., 2019) that exendin-4 was modified at position 14, where methionine (Met) was replaced by norleucine (Nle), to improve oxidative stability during labelling. And coupled to NODAGA to form [Nle14-Lys40-(NODAGA)NH2]exendin-4, referred to as Ex4NOD. A comparative study was carried out with Ex4DFO coupled to desferrioxamine DFO (Figure 4 of the two chemical structures). In particular, two comparative studies by Brom et al. (2010) and Wild et al. (2010) have shown that GLP-1R PET/CT molecular imaging has a better sensitivity and tumor background ratio for insulinoma than SPECT/CT.
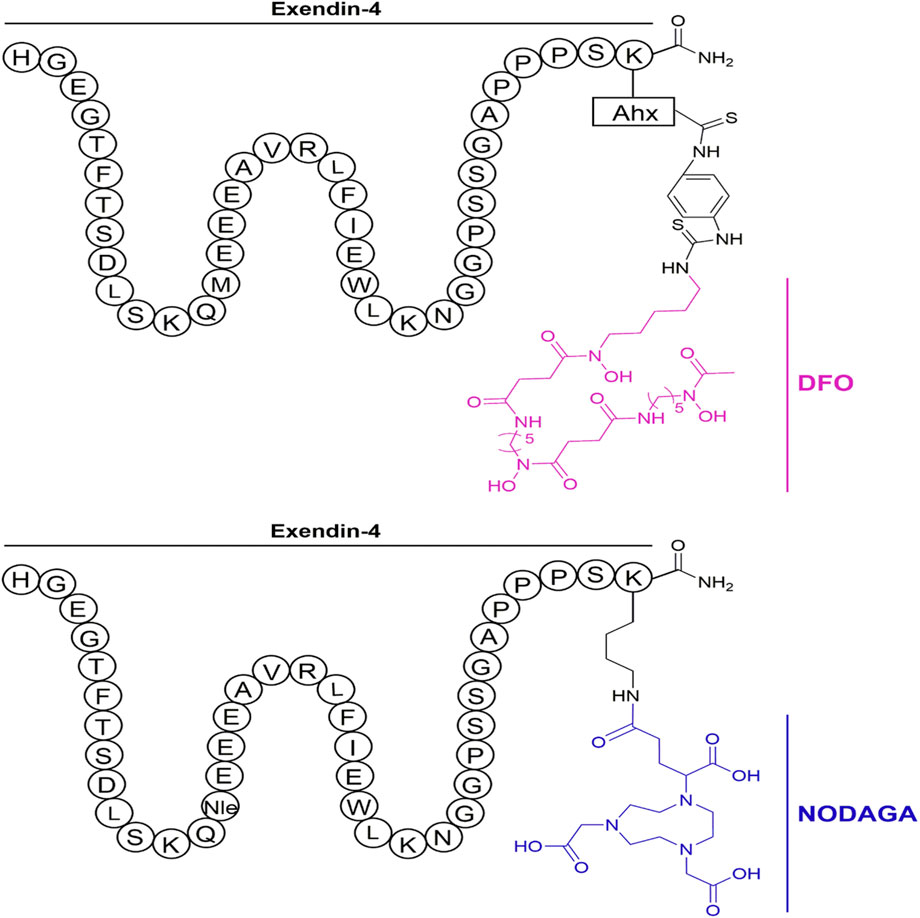
FIGURE 4. Schematic representation of the peptides investigated in the study. The top shows Ex4DFO and the bottom shows Ex4NOD. The chelators are depicted without coordinated radiometal.
We have summarized the previous preclinical studies of GLP1-R molecular imaging in insulinoma (Table 1) and discovered that from 111In in the early days of exploration to the now mature 68Ga. Even 89Zr (Bauman et al., 2015), a long half-life nucleophile, has been added to the list. It opens up more options for clinical research to be reviewed. Even so, the molecular probe for GLP-1R is still exendin-4. Such limitations and its high renal load are currently a challenge in this field, and perhaps the integration of new GLP-1R analogs into targeted therapy will become a more worthwhile prospect.
2.2 Clinical study of GLP-1 receptor molecular imaging in insulinoma
The theranostic of molecular medicine is the latest trend. Furthermore, the success of clinical translation more or less determines the future of molecular imaging of GLP-1R in insulinoma. We have likewise summarized all previous studies on insulinomas (Table 2). Nucleophiles18F, 64Cu, and 89Zr have restricted their clinical application due to some of the limitations mentioned in the previous section. Wild combines GLP-1R molecular imaging with the clinic for the first time (Wild et al., 2008; Christ et al., 2009; Wild et al., 2011), 2 years after its landmark 2006 preclinical study was published. Despite the small number of insulinoma patients included in the two clinical studies, 111In-DTPA-exendin-4 SEPCT/CT successfully detected lesions compared to the weak sensitivity of conventional imaging pairs. It opens new doors for molecular imaging of insulinomas. Subsequent SEPCT/CT molecular imaging investigations of 111In and 99mTc-labelled exendin-4 have followed (Sowa-Staszczak et al., 2013; Antwi et al., 2015; Senica et al., 2020). However, the most representative of these undoubtedly belongs to two large sample-size clinical trials by Christ in 2013 (Christ et al., 2013) and Sowa-Staszczak in 2016 (Sowa-Staszczak et al., 2016). Christ performed 111In-DTPA-exendin-4 molecular imaging in 20 of the 30 patients with EHH who were included to detect insulinomas and successfully detected 19 with a sensitivity of 95%, in contrast to 44% with conventional imaging. Such results are consistent with Sowa-Staszczak. The latter used 99mTC-HYNIC-exendin-4 in all 18 patients with insulinoma, significantly higher than the detection rate of 6/18 (33.3%) with conventional imaging. Such promising results are encouraging, and it indicates that GLP1-R molecular imaging is gradually replacing conventional imaging as the non-invasive modality of choice for the accurate preoperative localization of insulinoma.
Similarly, PET/CT studies, a representative of molecular imaging, have also revolved around 68Ga-exendin-4. Different chelator-targeted molecular probes have been successfully reported in several cases. Here, our team (Qiu et al., 2023) also successfully used 68Ga-DOTA-exendin-4 PET/CT to visualize a double lesion insulinoma in the pancreas’s tail, one of which was not detected on MRI (Figure 5). The clinical reports of Ga-exendin-4 are among the most diverse in terms of the chelating agents employed, and it has been an essential part of its success in clinical translation. Whether DOTA (Sood et al., 2019; Michalski et al., 2020; Das et al., 2021; Zhang et al., 2021), DO3A (Eriksson et al., 2014; Velikyan et al., 2017), or NOTA (Luo et al., 2015; Luo et al., 2016a; Warren et al., 2020) is reported on a case-by-case basis, the most convincing report and one that has driven the recognition of 68Ga-GLP-1R molecular imaging should be the publication of Luo’s seminal study in 2017 (Luo et al., 2016b). He prepared 68Ga-NOTA-MAL-cys40-exendin-4 by coupling NOTA-MAL with the ligand Cys40-exendin-4 and then successfully targeting 68Ga to the peptide at 100°C for 15 min. The results revealed that 68Ga-NOTA-exendin-4 PET/CT successfully detected lesions in 42 of 43 insulinoma patients, with a sensitivity of 97.7%, markedly higher than the 19.5% of 99mTc-HYNIC-TOC SPECT/CT. It is the first time that the two types of molecular imaging have been compared again in a clinical report, and PET/CT remains the obvious choice. Another study (Boss et al., 2019), also widely expected, showed a sensitivity of 85.3% for 68Ga-NODAGA-exendin-4 PET, greater than SSTR PET (54.5%) and conventional imaging (66.7%). At this point GLP-1R molecular imaging has entered the era of 68Ga-exendin-4, and their meaning here cannot be overstated.
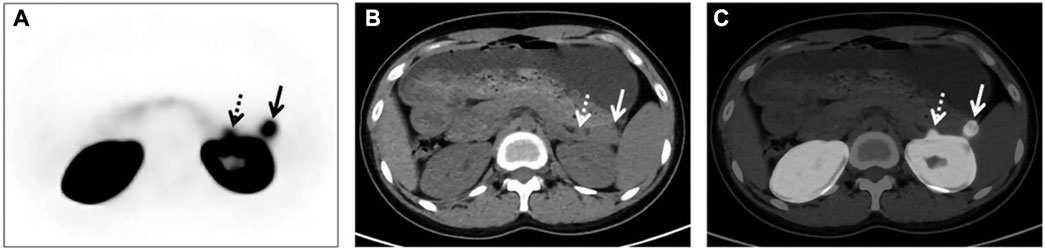
FIGURE 5. GLP-1R molecular imaging of double-primary insulinomas. Two insulinoma lesions in the tail of the pancreas, only one of which was detected in conventional imaging.68 Ga-DOTA-exendin-4 molecular imaging successfully detected both lesions and guided surgical resection. In the axial images [(A) PET, (B) CT, (C) fusion) in addition to the significantly higher uptake of the lesion in the tail of the pancreas, we see that GLP-1R imaging successfully detected the other lesion adjacent to the kidney, which was not detected in the MRI image due to the obscuration of the kidney. Thus, GLP-1R molecular imaging offers better advantages over conventional imaging in the preoperative precise localization of insulinomas.
Unfortunately, it has been greatly appreciated in molecular imaging as the most popular combination of 68Ga/177Lu for theranostics. The research is still vacant in insulinoma. As early as 2016 (Espes et al., 2016) [177Lu]-DO3A-VS-Cys40-Exendin-4 was used for the first time to successfully scan transplanted islets and quantify cell mass in an islet transplantation model. In 2019, Guleria et al. (2019) produced a molecule [177Lu]Lu-DOTA-Ahx-Lys40-Exendin-4 with high radiochemical purity. The biodistribution investigation revealed that the chemical initially accumulated in pancreatic tissue and was predominantly excreted by the kidney. Nevertheless, its substantial absorption in the kidney restricts its potential for further translation. The failure of the investigations above based on 177Lu-labeled exendin-4 to complete GLP-1R molecular imaging and targeted therapy in insulinoma models will present an emerging opportunity for our long-term research. It will also be the biggest obstacle to the current move towards GLP-1R molecular imaging into theranostic, and we need to pool our efforts to overcome this challenge.
3 Emerging opportunities for GLP-1R molecular imaging
Although most past studies have been limited to insulinomas, we tabulated radiolabeling options from all relevant studies (Table 3). It is easy to see that the preclinical studies cover a much wider choice of radionuclides, but the large radiation load of 64Cu, the stringent labelling conditions of 18F and the cumbersome preparation of these radionuclides, for example. These are all constraints to their clinical translation. The two long half-life radiolabels, 89Zr and 177Lu, have also failed to achieve clinical translation, even though they offer better therapeutic prospects. However, the greater renal burden of both and the limited stability of 89Zr due to the covalent bond breakage between chelator and protein have limited their potential use in GLP-1R imaging. Therefore both 111In and 99mTc in SPECT/CT and radionuclide 68Ga in PET/CT are better choices for GLP-1R radiolabelling. It also provides a guarantee and basis for future research in other areas of clinical translational research and diagnostic integration.
The current treatment modality is still mainly surgical excision. Therefore finding an integrated approach to GLP1-R molecular medicine treatment as soon as possible could provide more guidance to clinicians in their choice of intervention strategies. Can we explore the feasibility of GLP-1R molecular imaging in other diseases or fields and open the gateway to push on it? With this in mind, we have summarised and reviewed the current status of GLP-1R molecular imaging in other fields, and it may be an opportunity to explore its theranostic.
3.1 Other neoplasms
SSTR is highly expressed in malignant tumors but rarely in GLP-1R. The results of Bongetti’s research (Bongetti et al., 2018) gave us a great warning. Although benign insulinoma accounts for 90% of all insulinomas, 10% are still malignant. Therefore, for patients with negative imaging results who remain highly suspected of insulinoma, we should be alert to whether they are malignant. At this time, we need to take the receptor imaging technology of SSTR to identify them, such as 68Ga-Dotatate-exendin-4. Do not miss or misdiagnose the patients with negative GLP-1R imaging. The study of Korner et al. (2007), other than insulinoma, illustrated that GLP-1R is also high expression in gastrinoma, MTC, 60% of pheochromocytoma, and gastrinoma. Given this, is molecular imaging targeting GLP-1 analogs feasible in these tumors? A study has since given partial answers.
Pach et al. (2013) performed [Lys (Sood et al., 2019) (Ahx-HYNIC-(99m)Tc/EDDA)NH2]-Exendin-4 SPECT/CT imaging in four enrolled MTC patients for the first time. These results displayed that GLP-1R molecular imaging is not exclusive to insulinoma and plays a vital role in other diseases. Clinical experience has pointed to these tumors as high in receptor expression, and they all have a relatively low clinical incidence. Even with pheochromocytoma, which has a relatively high prevalence, the more established SSTR molecular imaging agent DOTATATE is now available. Hence, these factors have directly or indirectly hindered the exploration of GLP-1R molecular imaging in tumor diseases other than insulinoma. It is both a challenge and an opportunity, so searching for additional molecular probes may present promising results in these oncological diseases.
3.2 Myocardial ischemia
Currently, the most commonly used methods for the assessment of surviving myocardium after myocardial infarction in clinical practice include EHCO, CAG, CTCA, and MRI. In the past, these methods were used to assess myocardial survival after infarction from the level of myocardial dynamics and perfusion, but they were unable to analyze the metabolic level of the surviving myocardium from the molecular metabolism point of view in a more in-depth manner. The FDG currently used in PET/CT is taken up by myocardium with metabolic activity but not by necrotic myocardium. Thus, myocardial metabolism can be evaluated by this mechanism. Also PET dynamic myocardial perfusion imaging agents combined with 13N-NH3 provide more accurate judgment (Kim et al., 2015). In the cardiovascular, GLP-1R can improve cardiovascular function and reduce inflammation (Laviola et al., 2012). Moreover, it is involved in the protective effect of myocardial cells after myocardial ischemia. So, can infarcted myocardial cells be targeted by nuclide-labeled GLP-1R molecular imaging? The publication of two critical studies has also delivered on the promise of GLP-1R imaging in cardiovascular disease.
In the myocardial ischemia/reperfusion (MI/R) study (Gao et al., 2012), 18F-FBEM-Cys40-exendin-4 could monitor the dynamic changes of GLP-1R upregulation in the myocardial infarction/ischemia area. Meanwhile, the uptake changes observed by PET were consistent with the changes in receptor expression by Western blot analysis and histochemistry. Its protective mechanism and analogs in myocardial cells with ischemia injury are now better-understood thanks to this report, which is the first to image GLP-1R in myocardial cells that have undergone MI/R. It marks the triumphant entry of molecular imaging into the cardiovascular field. Another blockbuster study (Stahle et al., 2020) was the first application of the 68Ga-NODAGA-exendin-4 molecular probe in a myocardial infarction model. The encouraging conclusion indicated that the uptake of 68Ga-NODAGA-exendin-4 in the infarcted area was increased by 8.6 times compared with the sham operation area. The uptake of the imaging agent was correlated with the number of CD68-positive macrophages in the infarcted area and α-smooth muscle actin staining in the distal myocardium. Molecular imaging can dynamically monitor the protective effect of GLP-1R on ischemic cardiomyocytes, which brings a new auxiliary method for evaluating the therapeutic effect of myocardial ischemia and myocardial infarction.
And compared with FDG PET/CT, GLP1R molecular imaging is more friendly to patients with type 2 diabetes. It allows for a more visual assessment of GLP1R metabolic expression levels in the surviving myocardium at the molecular metabolic level. Therefore, in the future, it could complement the assessment of inflammation in the molecular metabolism of surviving post-infarction cardiomyocytes. And it can be used together with other routine tests to provide the evaluation value of surviving post-infarction cardiomyocytes from different perspectives including myocardial dynamics, perfusion and molecular metabolism. However, because only a few preclinical studies of GLP1R molecular imaging have been performed, there is a need for a larger future.
3.3 Endocrine-related fields
GLP-1/GLP-1R in the endocrine field has been accompanied by the introduction of semaglutide, which has become a principal receptor target. The initial release of the SUSTAIN and PIONEER phase III clinical trials established its landmark importance in treating type 2 diabetes mellitus (T2DM) and obesity (Meier, 2021). More recently, research on this new GLP-1 analog has gradually expanded into other areas, including diabetes, chronic kidney disease, cardiovascular disease, NASH, and Alzheimer’s disease (Knudsen and Lau, 2019). However, no molecular imaging studies with semaglutide have been performed, and the endocrine field is still dependent on exendin-4 and its derivatives for molecular imaging. This section reviews the progress and emerging opportunities for endocrine-related GLP-1R molecular imaging.
3.3.1 Quantified pancreatic beta (β)-cell mass (BCM) in diabetes
It is well known that a decrease in pancreatic islet beta cells causes diabetes (Moonschi et al., 2017). At first, unlabeled GLP-1 analogs were developed for glycemic control in patients with diabetes, whereas pancreatic beta-cell mass (BCM) is essential for controlling and treating diabetes. Visual evaluation of BCM was invasive in the past (Murakami et al., 2021b). Non-invasive molecular imaging of radionuclides targeting GLP-1R in nuclear medicine has brought light to the quantitative evaluation of BCM. It directly drives progress in endocrinological diabetes research (Table 4).
The GLP-1R antagonist exendin (Gotthardt et al., 2002; Wild et al., 2006; Wicki et al., 2007; Vegt et al., 2008; Wild et al., 2008; Christ et al., 2009; Brom et al., 2010; Wild et al., 2010; Wild et al., 2011; Wu et al., 2011; Kiesewetter et al., 2012a; Kiesewetter et al., 2012b; Keliher et al., 2012; Christ et al., 2013; Sowa-Staszczak et al., 2013; Wu et al., 2013; Yue et al., 2013; Sel et al., 2014; Wu et al., 2014; Antwi et al., 2015; Bauman et al., 2015; Xu et al., 2015; Falconi et al., 2016; Sowa-Staszczak et al., 2016; Kaeppeli et al., 2019; Zhang et al., 2019; Li et al., 2020; Mori et al., 2020; Senica et al., 2020; Murakami et al., 2021a; Qiu et al., 2023) is the most widely applied in diabetic BCM. It is a truncated form of the GLP-1 agonist exendin-4 and can significantly reduce the insulin-to-glucose ratio without affecting glucose tolerance or insulin sensitivity (Blevins et al., 2014). Earliest, Wang (Wang et al., 2012) combined 18F and FBA to 6-hydroxynitrile aldehyde at Lys27 of Exendin (Gotthardt et al., 2002; Wild et al., 2006; Wicki et al., 2007; Vegt et al., 2008; Wild et al., 2008; Christ et al., 2009; Brom et al., 2010; Wild et al., 2010; Wild et al., 2011; Wu et al., 2011; Kiesewetter et al., 2012a; Kiesewetter et al., 2012b; Keliher et al., 2012; Christ et al., 2013; Sowa-Staszczak et al., 2013; Wu et al., 2013; Yue et al., 2013; Sel et al., 2014; Wu et al., 2014; Antwi et al., 2015; Bauman et al., 2015; Xu et al., 2015; Falconi et al., 2016; Sowa-Staszczak et al., 2016; Kaeppeli et al., 2019; Zhang et al., 2019; Li et al., 2020; Mori et al., 2020; Senica et al., 2020; Murakami et al., 2021a; Qiu et al., 2023), synthesized the first molecular developer [18F]Ex (Gotthardt et al., 2002; Wild et al., 2006; Wicki et al., 2007; Vegt et al., 2008; Wild et al., 2008; Christ et al., 2009; Brom et al., 2010; Wild et al., 2010; Wild et al., 2011; Wu et al., 2011; Kiesewetter et al., 2012a; Kiesewetter et al., 2012b; Keliher et al., 2012; Christ et al., 2013; Sowa-Staszczak et al., 2013; Wu et al., 2013; Yue et al., 2013; Sel et al., 2014; Wu et al., 2014; Antwi et al., 2015; Bauman et al., 2015; Xu et al., 2015; Falconi et al., 2016; Sowa-Staszczak et al., 2016; Kaeppeli et al., 2019; Zhang et al., 2019; Li et al., 2020; Mori et al., 2020; Senica et al., 2020; Murakami et al., 2021a; Qiu et al., 2023) for quantifying BCM in the bio breeding diabetes-prone (BB-DP) animal model. The following year, Kimura (Kimura et al., 2018) exploited [18F]-FB40-Ex (Gotthardt et al., 2002; Wild et al., 2006; Wicki et al., 2007; Vegt et al., 2008; Wild et al., 2008; Christ et al., 2009; Brom et al., 2010; Wild et al., 2010; Wild et al., 2011; Wu et al., 2011; Kiesewetter et al., 2012a; Kiesewetter et al., 2012b; Keliher et al., 2012; Christ et al., 2013; Sowa-Staszczak et al., 2013; Wu et al., 2013; Yue et al., 2013; Sel et al., 2014; Wu et al., 2014; Antwi et al., 2015; Bauman et al., 2015; Xu et al., 2015; Falconi et al., 2016; Sowa-Staszczak et al., 2016; Kaeppeli et al., 2019; Zhang et al., 2019; Li et al., 2020; Mori et al., 2020; Senica et al., 2020; Murakami et al., 2021a; Qiu et al., 2023) for the quantification of diabetic BCM. It had a stronger targeting affinity and could visualize pancreatic β-cells. The initial conditions for a molecular probe for GLP-1 R imaging were fulfilled, and a new frontier was opened for non-invasive molecular imaging. Continuing the heat of exendin (Gotthardt et al., 2002; Wild et al., 2006; Wicki et al., 2007; Vegt et al., 2008; Wild et al., 2008; Christ et al., 2009; Brom et al., 2010; Wild et al., 2010; Wild et al., 2011; Wu et al., 2011; Kiesewetter et al., 2012a; Kiesewetter et al., 2012b; Keliher et al., 2012; Christ et al., 2013; Sowa-Staszczak et al., 2013; Wu et al., 2013; Yue et al., 2013; Sel et al., 2014; Wu et al., 2014; Antwi et al., 2015; Bauman et al., 2015; Xu et al., 2015; Falconi et al., 2016; Sowa-Staszczak et al., 2016; Kaeppeli et al., 2019; Zhang et al., 2019; Li et al., 2020; Mori et al., 2020; Senica et al., 2020; Murakami et al., 2021a; Qiu et al., 2023) quantifying the diabetic BCM, molecular imaging trials of exendin-4 in this field are also in full swing. Connolly (Connolly et al., 2012) is the first to attempt the composite 64Cu-DOTA-exendin-4. However, it was impacted by the tracer’s high uptake in the kidney, which obscured part of the pancreatic tissue and seriously affected the quantitative determination of BCM. Until two diabetes model studies (Brom et al., 2014; Kimura et al., 2017) completed the quantification of BCM under the derivatives ([Lys12(111In-BnDTPA-Ahx)]exendin-4) and guided the treatment of diabetes. It also signifies the value and significance of GLP-1R molecular imaging.
177-Lu, as an essential part of theranostics, does not perform satisfactorily in previous insulinoma imaging. Eriksson (Eriksson et al., 2017) initially applied [177Lu]Lu-DO3A-VS-Cys40Exendin-4 to the comparative study of pancreatic uptake in various species. The ratio of islets to exocrine pancreas varies between species; consequently, the crucial concept of IPR was proposed. IPR has also become an influential statistical factor in imaging pancreatic β-cells and determining BCM. Such results are promising. Compared to the therapeutic limitations of insulinoma, quantifying BCM and treating diabetes through GLP-1R molecular imaging have shed light on theranostics.
3.3.2 Islet transplantation
Before the development of mesenchymal stem cells and pluripotent stem cells for the treatment of diabetes, the most effective treatment for type 1 diabetes (T1D) was portal vein islet transplantation. It primarily administered donor islets to the liver via the portal vein. In the hepatic sinusoids, the islet cells could proliferate and secrete insulin. Portal vein islet transplantation has achieved good results in protecting patients from severe hypoglycemia. Regarding pancreatic β-cells, it makes sense to monitor graft survival in patients with pancreatic β-cell transplantation with GLP-1R molecular imaging. Previous studies have clarified (Hubalewska-Dydejczyk et al., 2015) that GLP-1R imaging helps monitor pancreatic beta cell quality in patients with fragile type I diabetes after islet transplantation. Indeed, few articles exist on molecular imaging of transplanted islets.
Initially, 111In-Exendin-3 SPECT/CT imaging (Eter et al., 2017) detected the quality of transplanted β-cells and measured the volume using C3H of heterotopic islet transplantation in the calf muscle. Pattou et al. (2010) featured a left brachioradialis islet transplantation case, and GLP-1R molecular imaging with Lys40 (Ahx-DTPA-111In)NH2-exendin-4 revealed locally high uptake in the left forearm islet graft. According to these two reports, 111In-exendin SPECT/CT imaging is anticipated to become a novel molecular probe for non-invasive quantitative detection of β-cell in human islet transplantation. In addition, several other radionuclide markers for islet graft imaging have been developed. Such as (Yue et al., 2013) F-TTCO-Cys (Sood et al., 2019)-exendin-4 (Wu et al., 2013) visualize the graft in liver imaging of islet transplantation, similar results were obtained for 177Lu-DO3A-Exendin-4 (Espes et al., 2016) and 18F-PTTCO-Exendin-4 (Li et al., 2020). These studies lack data from sufficiently large sample sizes, and the case studies remain inconclusive.
Moreover, a recent study (Juang et al., 2021) showed the interaction between manganese with engineered iron oxide nanoparticles (MnMEIO NPs) and Exendin-4. Successfully detecting the labeled Min-6 β-cells in the recipients after transplantation in combination with MRI. Unfortunately, they did not target labeling radionuclides. Exploring more GLP-1R derivatives and other analogs for islet transplantation has become necessary. Monitoring transplanted pancreatic β-cells to guide graft survival is a new benefit for islet transplantation patients. Such a gap also represents a worthwhile direction for future research in GLP-1R molecular imaging.
3.3.3 Congenital hyperinsulinemia (CHI)
Benign insulinoma is the most common cause of endogenous hyperinsulinemic hypoglycemia (EHH). GLP-1R molecular imaging will become the ideal choice for non-invasive localization and diagnosis of benign insulinoma patients in the future. The clinical presentation of CHI is similar to that of insulinoma, which can cause misdiagnosis. It must therefore be carefully excluded when diagnosing insulinoma with EHH symptoms. In the past, nesidioblastosis was synonymous with congenital hyperinsulinemia. Until 1995 (Ma et al., 2020), the genetic basis of CHI was thought to be an inactivating mutation in the subunit. That forms the β-cell plasma membrane ATP-dependent potassium channel. Thus, it is not the proliferation or the hyperinsulinemia of β-cell proliferation. Hence the name is defined as CHI. It is more common in neonates and children, but Shah et al. (2017) reported that it could also appear in adults. Due to the different treatment options between benign insulinoma and CHI, accurately identifying the two diseases can avoid omission and misdiagnosis to a greater extent.
Boss et al. (2022) compared the sensitivity of 68Ga-NODAGA-exendin-4 and 18F-DOPA-exendin-4 PET/CT for the diagnostic efficacy of 19 patients with CHI. The sensitivity of 68Ga-NODAGA-exendin-4 at 100% was significantly higher than that of 18F-DOPA-exendin-4 at 71%. It is the first to discuss whether GLP-1R molecular imaging is a good choice for the preoperative localization of CHI lesions. The research that made a molecular imaging breakthrough in this field is credited to Christ (C et al., 2020). In the study, the pancreatic islet cells with CHI lesions had an extreme expression of GLP-1R. He discovered that the intensity of CHI uptake in PET molecular images ranged between insulinoma and normal pancreatic tissue. It provides a more convenient non-invasive test for differentiating benign insulinoma and CHI. This result has brought a historic breakthrough in GLP-1R molecular imaging.
In recent years, several studies (Reubi et al., 2010; Kalff et al., 2021) using different molecular probes to visualize CHI presented results similar to Christ’s. Interestingly, a trial with a negative outcome caught our attention (Reubi et al., 2010). The results indicated that patients with the non-insulinoma pancreatic hypoglycemic syndrome (NIPHS) and post-gastric bypass hypoglycemic syndrome (PGBH) could not be distinguished via GLP-1R molecular imaging. A plausible explanation may be that in these diseases, EHH symptoms prevent pancreatic cells from over-expressing this receptor. Its molecular imaging offers a new diagnostic option for insulinomas and CHI, which are rare and hard to identify in clinical practice. Nevertheless, molecular imaging still has limitations and does not apply to patients with specific etiologies of EHH, such as NIPHS and PGBH. The road ahead for molecular medicine remains daunting, and we need to focus on trial and error in these gap areas.
3.3.4 Multiple endocrine neoplasia type 1 (MEN-1)
MEN-1 is an autosomal dominant genetic disease caused by mutations in its tumor suppressor gene. Most patients will gradually progress to multifocal functional or non-functional pancreatic neuroendocrine tumors. Therefore, seeking a non-invasive means of identification at an early stage is necessary. GLP-1R molecular imaging in patients with MEN-1 mutations is a subject of increasing research. It is a very positive sign for endocrinology. Molecular imaging can enhance the diagnosis of an ion channel or genetically inherited diseases such as CHI and MEN-1, which are rare in clinical practice.
In 2019, Antwi et al. (2019) studied six MEN-1 patients diagnosed by genetic testing among 52 recruited EHH patients. The sensitivity of 68Ga-DOTA-exendin-4 PET/CT combined with MRI (92.3%) is significantly higher than MRI (38.5%), and PET/CT (84.6%) alone, GLP-1R molecular imaging combined with MRI is a feasible method to detect the lesions of MEN-1 patients and guide the surgical treatment. Subsequently, two cases published (Luo et al., 2015; Luo et al., 2016a) successfully identified multiple insulinomas in confirmed MEN-1 patients with the tracer 68Ga-NOTA-exendin-4 PET/CT imaging. GLP-1R molecular imaging has become a potential direction for diagnosing insulinoma in MEN-1.
That said, the results of an earlier study (Shah et al., 2022) are worth considering. SSTR-targeted tracer 68Ga-DOTATATE PET/CT was utilized to compare the lesions in insulinomas and MEN-1 patients. The tracer had significantly higher sensitivity and positive predictive value in malignant insulinoma. It could guide the theranostic PRRT treatment other than MEN-1. SSTR molecular imaging is not applicable in MEN-1, so the value of GLP-1R molecular imaging for this disease becomes even more valuable. Furthermore, a first meta-analysis (Shah et al., 2021) last year concluded that GLP-1R could act as a molecular probe for accurate preoperative localization imaging in MEN-1 patients after summarizing all previous cases of MEN-1 patients. Due to the difficulty and rarity of diagnosing MEN-1 patients, the predictive value of GLP-1R imaging prior to pathological examination is especially relevant. Nonetheless, the labeled nuclide in MEN-1 continues to be 68Ga. Research on long half-life therapeutic nuclides such as 177Lu is still lacking, and it is challenging to integrate molecular medicine theranostics in this field.
In summary, from our summary of the current status of GLP-1R molecular imaging in endocrinology (Table 4), we realize that quantification of BCM in diabetes and islet transplantation may be the last winner and that current advances in molecular medicine in this field are full of promise. Diseases such as CHI and MEN-1, which are clinically uncommon and easily underdiagnosed, have also been studied and molecular imaging has given them crossover implications. We therefore have reason to believe that non-invasive molecular medicine in endocrinology may lead to a remarkable achievement in the theranostic.
3.4 Neurodegenerative disease
The expression of GLP-1R was initially detected in the nucleus tractus solitarius and hypothalamus. As the research progressed, so did other brain parts, such as the frontal cortex, hippocampus, amygdala, and substantia nigra. In addition to being primarily distributed in the pancreatic islet cell, it can appear selectively in the central nervous system (Jensen et al., 2018; Mansur et al., 2019; Gabery et al., 2020). The theranostic of central nervous system diseases targeting GLP-1R has been gradually developed. It is also a key area of focus for molecular medicine at this stage.
Molecular imaging has already become a crucial clinical tool in the diagnosis of AD and PD. For instance, the tracer 11C-PIB, as is now widely used for imaging AD age spots (Klunk et al., 2004). However, 11C has a half-life of only 20 min, limiting its use in routine examinations. 18F has a longer half-life. Therefore 18F-based PET imaging began a long quest in the disease assessment of AD. From the initial low specificity of FDG PET for pathological changes in AD to the drawbacks that amyloid PET imaging does not correlate with the severity of the disease. The unfolding of the Tau PET examination has provided significant value in the assessment of early pathological changes and disease severity in AD. And later with the 18F-florbetapir have been employed in AD as beta-amyloid (Aβ) imaging agents (Clark et al., 2012), while 18F-FDOPA has been used in PD diagnosis (Brooks, 2010). Two previous studies in PD models (Bertilsson et al., 2008; Isacson et al., 2011) have confirmed that exendin-4 can restore the nerve in the subventricular zone and hippocampus, thereby improving mice’s cognitive, motor, and memory abilities. Bu et al. (2021) still bound exendin-4 with AAV-9-A53T-α-synuclein in the PD model and observed that exendin-4 treatment attenuated the pathological accumulation of α-synuclein. In recent years, additional studies (Salameh et al., 2020; Lv et al., 2021; Zhang et al., 2022) have found that incretin receptor agonists (Exendin-4 and DA-JC4) and GLP-1/GIP dual receptor agonists DA5-CH can successfully cross the blood-brain barrier and reach the pathological area of PD, which is expected to become an ideal therapeutic drug. Exendin-4 has shown excellent results in improving cognitive function in Parkinson’s disease. The mechanism and efficacy of a novel GLP-1R analog liraglutide in PD are also evaluated (Kim et al., 2017; Mah et al., 2022; Chen et al., 2023). GLP-1R targets may usher in a new chapter in the theranostic of central nervous system diseases.
For the first time, Wang et al. (2018) illustrated the effect of age differences on GLP-1R distribution in the brain by synthesize [18F]AlF-NOTA-MAL-Cys39-exendin-4, the peptide was modified by coupling Cys39-exendin-4 to NOTA-MAL and was successfully synthesized using 18F-targeted labeling of the peptide(Figure 6). Its expression in the brains of aged mice was elevated reduced. The discovery of this molecular probe creates a better prospect for applying GLP-1R molecular imaging to neurodegenerative diseases such as PD and AD. In 2020, Liu et al. (2020) published the investigation via PET imaging in Alzheimer’s disease (AD) model, different derivatives with 18F labeling followed by microPET. [18F]FBEM-exendin-4 is more likely to cross the blood-brain barrier due to its better lipid solubility than [18F]AIF-exendin-4 based on previous findings. [18F]FBEM-Cys39-exendin-4 was compared with three other 18F-labeled derivatives of PET imaging. This tracer successfully evaluated the decrease of total GLP-1R expression in the AD model. It is the first report of GLP-1R molecular imaging in patients with neurodegenerative disease, which opens a good direction for the future in this still-unknown field. Deden et al. (2021) also used a 68Ga-labeled tracer to detect the difference in receptor intracranial distribution of obese patients compared with ordinary people. Unfortunately, 68Ga-NODAGA-exendin-4 PET was unsuitable for GLP-1R distribution imaging in the brain of obese patients.
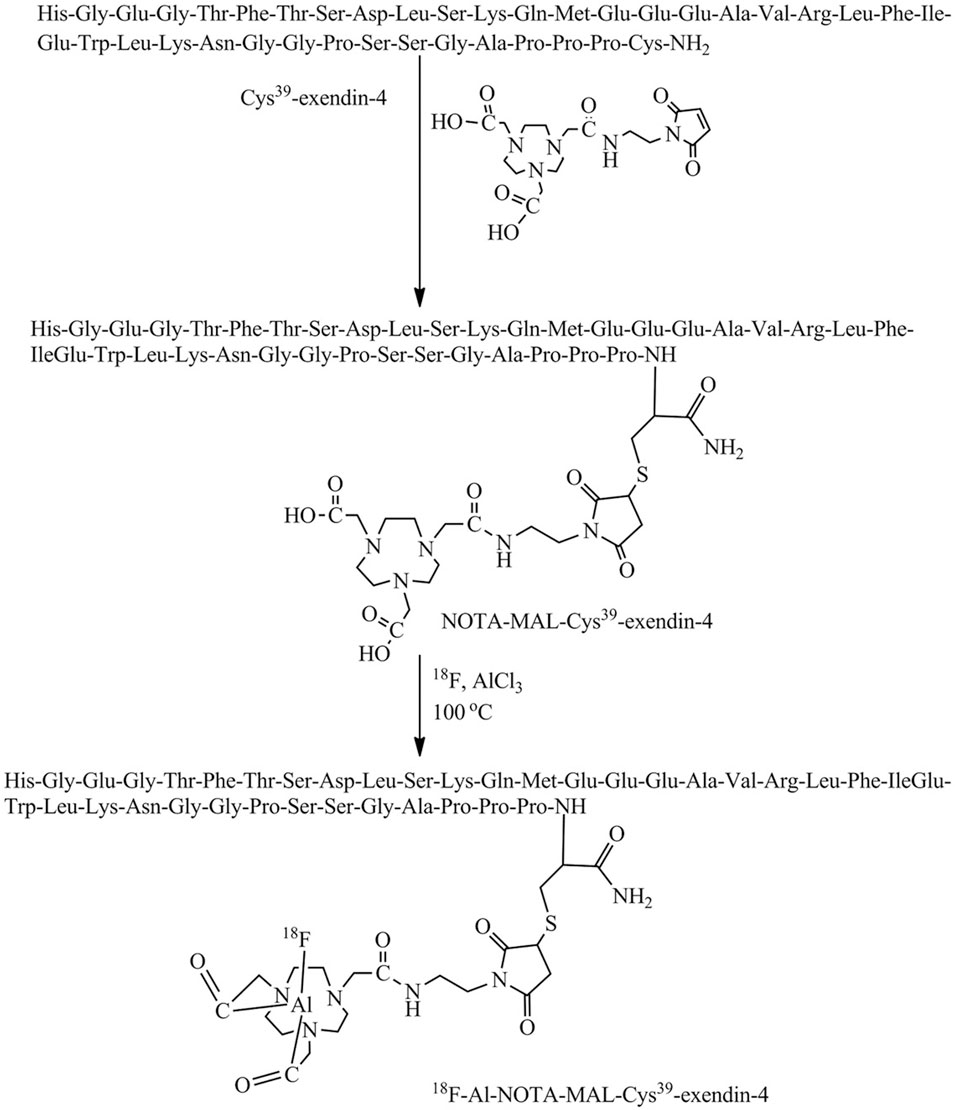
FIGURE 6. Scheme for the synthesis of [18F]AlF-NOTA-MAL-Cys39-exendin-4. Modified by coupling Cys39-exendin-4 to NOTA-MAL by addition of ionized target water containing 18F (740 MBq). Heated at 100°C for 10 min. Cool and dilute the mixture with sterile water and inject into a validated Varian BondElut C18 cartridge. 10 mL of sterile water washes the column twice and ethanol hydrochloride (0.3 mL) elutes the labelled peptide.
The research on GLP-1R molecular imaging in neurodegenerative diseases and other central nervous system diseases has not achieved more extraordinary breakthroughs, limited to preclinical studies and related studies of blood-brain barrier penetration. There remains a significant gap in the comparative study of GLP-1R and neurodegenerative mechanisms and the improvement of symptoms in clinical patients. The experimental investigation of combining GLP-1R molecular imaging with other related nervous system diseases is an emerging opportunity in that uncharted territory. It could drive the neurology theranostic.
4 GLP1R molecular visualization of ligands and chelator
As mentioned previously, ligand selection for GLP-1R molecular imaging is currently still centred on exendin-4. Although exendin-3 and exendin-(9–39) have also been involved as other ligands in studies of insulinoma and pancreatic β-cell mass determination. However, the structural form of the ligand for both exists only in the single form of Lys40-exendin-3, Lys27-exendin-(9–39) (Gasbjerg et al., 2021). Diverse ligand forms are currently being designed and developed around exendin-4. These include Lys39-exendin-4, Lys40-exendin-4, Nle14, Lys40-exenidn-4, Cys40-exendin-4 and other different ligand types. Table 5 lists the different types of ligands designed for GLP-1R molecular imaging and their corresponding radiolabels, linkers, conjugation structures, etc. Lys40-exendin-4 and Cys40-exendin-4 are the two most established and widely used ligands. They can show good stability with different chelating agents. In terms of linkers, Ahx has almost become the obvious choice for GLP-1R molecular imaging. The introduction of the hydrophobic linker Ahx between the peptide and the marker effectively protects the stability of the labelled peptide. It makes the conjugated structure and the peptide more stable, which facilitates the progress of subsequent studies.
A chelator is an organic molecule that can chelate with heavy metal ions. Both SPECT/CT and PET/CT present a more diverse selection of chelating agents to try in preclinical studies. Unfortunately the chelating agents that eventually translate to clinical use are mainly shown in Figure 7. One of the smallest molecular weights, Hydrazinonicontinamide (HYNIC), is an established bifunctional complexing agent. A past LC-MS study (King et al., 2007) has shown that the HYNIC adduct contains fewer ligand co-ligand molecules, making it the most suitable chelator for 99mTc labelling as it can efficiently meet the ligand requirements of technetium. DTPA accomplishes the DPTA modification of peptides mainly by chelating NH2 bonds on the benzene ring and amino acids with COOH.111In radiolabelling them is an important labelling strategy for GLP-1R molecular imaging studies. When it comes to chelating agents, DOTA must occupy an important place. The four nitrogen atoms and the carboxyl group in the acetic acid on the nitrogen atom can be coordinated with metal, which is a common class of bifunctional chelators. 111In and 68Ga in GLP-1R molecular imaging can be perfectly targeted with it. It has also become one of the most widely used chelating agents in molecular imaging conversion. Others include NOTA, NODAGA and DO3A chelating agents are new chelating agents that have emerged in recent years. They and their derivatives have improved structural remodeling to give more stability to the target-labeled peptides. Thus they are also starting to emerge in GLP-1R molecular imaging.
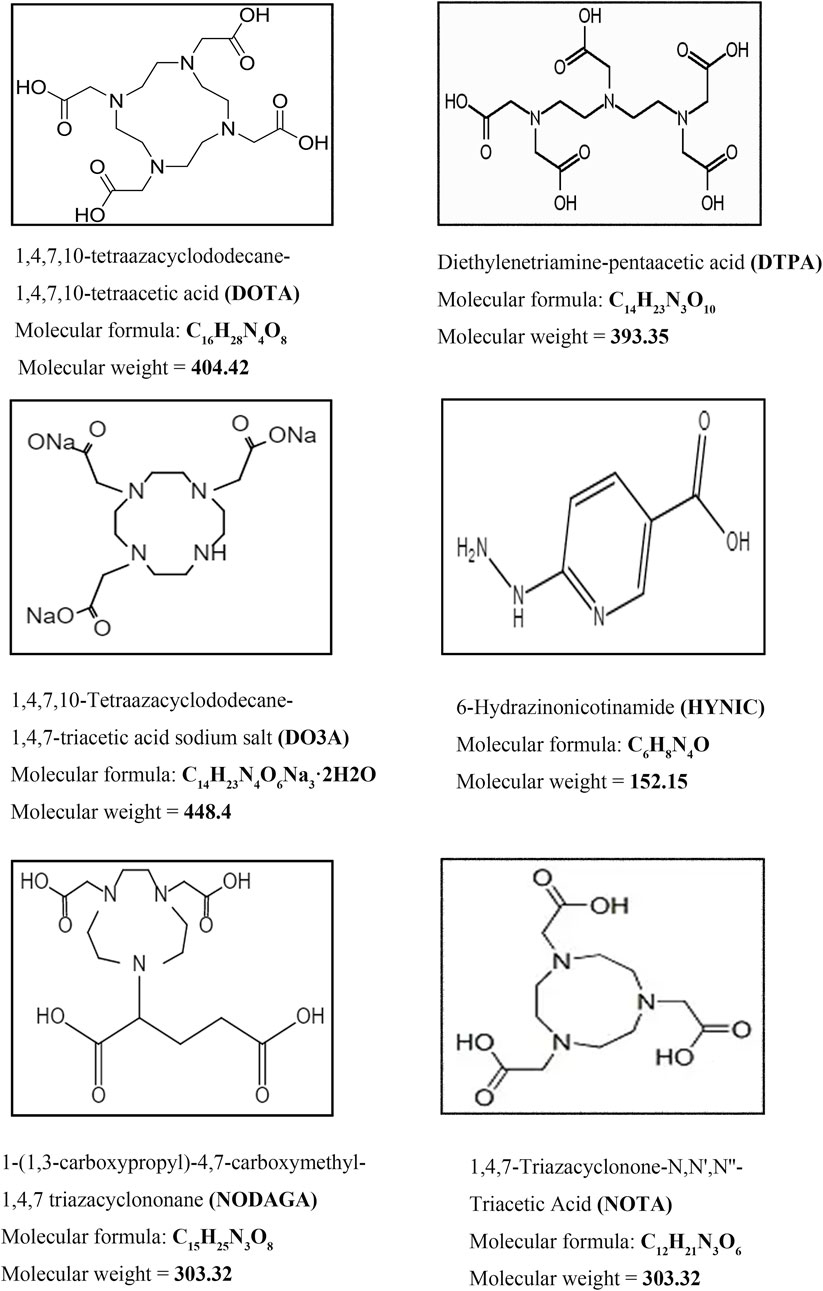
FIGURE 7. Commonly used chelating agents in GLP-1R molecular probes. The most classic chelators, DOTA, DTPA and the 99mTc HYNIC chelator, which were used in the first GLP-1R molecular imaging studies, have all been successfully translated and used in clinical studies. On this basis, the advantages of 68Ga radionuclides in GLP-1R imaging have been highlighted. New chelators, including DO3A, NOTA and NODAGA, are also beginning to make their mark in GLP-1R molecular imaging.
In contrast to translational clinical chelators, preclinical studies have included the successful preparation of a variety of derivative chelators with 18F targeting markers. However, these chelators remain limited to preclinical studies, which may be related to the high cost of 18F radionuclides, high radiation burden, and cumbersome preparation of derivatives. A similar phenomenon is also seen in ligand selection. Both for Lys40-exendin-4 and Cys-exendin-4, their ligands are limited to exendin-4 itself. Therefore, there is an urgent need to find chelators with better affinity for the target peptide and to explore the preparation of more diverse and stable ligands in the future. This is also the key to successful diagnostic integration in GLP-1R molecular imaging.
5 Discussion
GLP-1R molecular imaging is currently the most promising non-invasive method for detecting benign insulinoma and other GLP-1R-positive diseases. The experimental expansion of various molecular probes has become a trend, and 68Ga-exendin has emerged in clinical transformation, becoming an efficient target probe choice for the localization of insulinoma. The diversity of nuclides in preclinical studies is awkward in the transition to clinical studies, as shown by our summary of the molecular imaging results of insulinoma. The main radiolabels that have been successfully translated into clinical practice are 111In, 99mTc and 68Ga. This is mainly due to their relatively low radiation load, the relative ease of preparation and their economic cost. The long half-life nuclides 89Zr, 64Cu and 177Lu, on the other hand, have greatly hindered the progress of their integration into clinical translational therapy due to the disadvantages of their high renal burden. This is the main issue that we need to urgently address in the future in the field of GLP-1R therapy.
Meanwhile, the choice of ligand is also crucial in determining the labelling strategy and the prerequisite basis for peptide preparation. It has been mentioned many times that current research, both pre-clinical and clinical, is focused around exendin-4 ligands. The ligand, although based on exendin-4 taps into a more established type of more diverse forms including Lys40-exendin-4 and Cys40-exendin-4. However, the strategy of exendin-4-based peptide synthesis and preparation has not yet left the core of exendin-4. Is it possible to explore the future from the new generation of GLP-1R analogues such as semiglutide and use them as substrates to develop more ligands and peptides with better stability and lower renal radiation? Such a direction may become a new phase of exploration for GLP-1R molecular imaging afterwards. On the one hand, the high renal uptake of exendin-4 limits its clinical application and translation. Although the preparation of different derivatives to reduce renal uptake is still troublesome. It may lead to tumors in the distal tail of the pancreas that overlap with the kidneys. As a result, these lesions are missed during imaging, and the average uptake of duodenal Brunner’s glands may also be a source of confusion for tumor imaging with this tracer (Zhu et al., 2017; Hepprich et al., 2020). On the other hand, the treatment of choice for insulinoma remains surgery, leading to limitations in molecular imaging of therapeutic nuclei. Therefore it becomes the biggest obstacle to advancing GLP-1R molecular medicine treatment in insulinoma.
In previous studies, adverse reactions such as nausea, vomiting, and hypoglycemia have been discovered in patients after imaging (C et al., 2020; Antwi et al., 2019; Shah et al., 2021). Therefore, the possibility to explore some new molecular probes targeting GLP-1R besides exendin is an uncharted area that we need to overcome and develop in the future. Clinical trials in NASH, pancreatic cancer, obesity, and cardiovascular disease are in full swing (Jansen et al., 2019). This article provides an overview of the current status of GLP-1R molecular imaging in insulinoma, endocrinology and other related fields (Table 6). It is clear that GLP-1R molecular imaging research continues to be centered on exendin. The search for novel molecular probes has become vital to advancing therapeutics in molecular medicine. The most recent GLP-1RA of the second generation is semaglutide. Its introduction and the success of clinical trials in several areas have prompted us to wonder whether this new GLP-1RA can be labeled with nuclides (Knudsen and Lau, 2019; Meier, 2021; Smits and Van Raalte, 2021). It has more homology than exendin-4 and may replace it as a new target tracer for GLP-1R molecular imaging. Nevertheless, this is only our advice, continue to speak with research data. Developing new molecular probes will result in significant advances in GLP-1R molecular imaging, which is exciting.
The performance of GLP-1R molecular imaging in diabetic BCM gives us insight and hope for theranostic. Recent studies have discovered novel targets in neuroendocrine tumors, such as the glucose-dependent insulin-biophilic polypeptide receptor (GIPR), the same hormone receptor as GLP-1R. Because it is expressed in gastrinoma and bronchial lung cancer, it holds great promise for molecular imaging of NENs when the GLP-1R is negative (Papaefthymiou et al., 2022). The gallbladder hormone-2 (CCK2) receptor is overexpressed in MTC, small cell lung cancer, and other neuroendocrine tumors (Refardt et al., 2021) and is considered a promising new target for NENs therapy. Thus, our outlook and goal have been to develop novel peptide hormone receptors, apply novel GLP-1R drugs as molecular probes, and target GLP-1R diseases. These are emerging opportunities to open up gaps, even if the molecular medicine theranostic is challenging tomorrow.
Author contributions
YX: completed the review selection and wrote the first draft. WP and YW: collated the literature summary and produced the figures, and YC: supervised and revised the manuscript. All authors contributed to the article and approved the submitted version.
Conflict of interest
The authors declare that the research was conducted in the absence of any commercial or financial relationships that could be construed as a potential conflict of interest.
Publisher’s note
All claims expressed in this article are solely those of the authors and do not necessarily represent those of their affiliated organizations, or those of the publisher, the editors and the reviewers. Any product that may be evaluated in this article, or claim that may be made by its manufacturer, is not guaranteed or endorsed by the publisher.
Supplementary material
The Supplementary Material for this article can be found online at: https://www.frontiersin.org/articles/10.3389/fmolb.2023.1210347/full#supplementary-material
Abbreviations
GLP-1, Glucagon-like peptide-1; GLP-1R, Glucagon-like peptide-1 receptor; GLP-1R, Glucagon-like peptide-1 receptor agonists; DPP-IV, dipeptidyl peptidase IV; MTC, medullary thyroid carcinoma; EHH, endogenous hyperinsulinemic hypoglycemia; DTPA, Diethylene Triamine Pentaacetic Acid; HPLC, High Performance Liquid Chromatography; SSTR, somatostatin receptor; DOTATATE, DOTA-octreotide; EHCO, Echocardiogram; CAG, coronary angiography; CTCA, computed tomography coronary angiography; MRI, Magnetic Resonance Imaging; MI/R, myocardial ischemia/reperfusion; T2DM, type 2 diabetes mellitus; NASH, nonalcoholic steatohepatitis; AD, Alzheimer Disease; PD, Parkinson Diseaseto; BCM, beta (β)-cell mass; BB-DP, bio breeding diabetes-prone; CHI, Congenital Hyperinsulinemia; PGBH, post-gastric bypass hypoglycemic syndrome; NIPHS, non-insulinoma pancreatic hypoglycemic syndrome; MEN-1, Multiple endocrine neoplasia type 1; GIPR, glucose-dependent insulin-biophilic polypeptide receptor; CCK2, gallbladder hormone-2.
References
Antwi, K., Fani, M., Nicolas, G., Rottenburger, C., Heye, T., Reubi, J. C., et al. (2015). Localization of hidden insulinomas with ⁶⁸Ga-DOTA-Exendin-4 PET/CT: A pilot study. J. Nucl. Med. 56 (7), 1075–1078. doi:10.2967/jnumed.115.157768
Antwi, K., Nicolas, G., Fani, M., Heye, T., Pattou, F., Grossman, A., et al. (2019). 68Ga-Exendin-4 PET/CT detects insulinomas in patients with endogenous hyperinsulinemic hypoglycemia in MEN-1. J. Clin. Endocrinol. Metab. 104 (12), 5843–5852. doi:10.1210/jc.2018-02754
Bauman, A., Valverde, I. E., Fischer, C. A., Vomstein, S., and Mindt, T. L. (2015). Development of 68Ga- and 89Zr-labeled exendin-4 as potential radiotracers for the imaging of insulinomas by PET. J. Nucl. Med. 56 (10), 1569–1574. doi:10.2967/jnumed.115.159186
Bertilsson, G., Patrone, C., Zachrisson, O., Andersson, A., Dannaeus, K., Heidrich, J., et al. (2008). Peptide hormone exendin-4 stimulates subventricular zone neurogenesis in the adult rodent brain and induces recovery in an animal model of Parkinson's disease. J. Neurosci. Res. 86 (2), 326–338. doi:10.1002/jnr.21483
Blevins, J. E., Appleyard, S. M., Baskin, D. G., Schwartz, M. W., Morton, G. J., Hwang, B. H., et al. (2014). Hindbrain oxytocin receptors contribute to the effects of circulating oxytocin on food intake in male rats. Endocrinology 155 (8), 2845–2857. doi:10.1210/en.2014-1148
Bongetti, E., Lee, M. H., Pattison, D. A., Hicks, R. J., Norris, R., Sachithanandan, N., et al. (2018). Diagnostic challenges in a patient with an occult insulinoma:(68)Ga-DOTA-exendin-4 PET/CT and (68)Ga-DOTATATE PET/CT. Clin. Case Rep. 6 (4), 719–722. doi:10.1002/ccr3.1448
Boss, M., Mikkola, K., Buitinga, M., Brom, M., and Gotthardt, M. J. N. (2019). 68Ga-NODAGA-exendin-4 PET/CT for the localization of insulinomas. J. Nucl. Med. 63.
Boss, M., Rottenburger, C., Brenner, W., Blankenstein, O., Prasad, V., Prasad, S., et al. (2022). 68)Ga-NODAGA-Exendin-4 PET/CT improves the detection of focal congenital hyperinsulinism. J. Nucl. Med. 63 (2), 310–315. doi:10.2967/jnumed.121.262327
Brom, M., Oyen, W. J., Joosten, L., Gotthardt, M., and Boerman, O. C. (2010). 68Ga-labelled exendin-3, a new agent for the detection of insulinomas with PET. Eur. J. Nucl. Med. Mol. Imaging 37 (7), 1345–1355. doi:10.1007/s00259-009-1363-y
Brom, M., Woliner-van der Weg, W., Joosten, L., Frielink, C., Bouckenooghe, T., Rijken, P., et al. (2014). Non-invasive quantification of the beta cell mass by SPECT with ¹¹¹In-labelled exendin. Diabetologia 57 (5), 950–959. doi:10.1007/s00125-014-3166-3
Brooks, D. J. (2010). Imaging approaches to Parkinson disease. J. Nucl. Med. 51 (4), 596–609. doi:10.2967/jnumed.108.059998
Brubaker, P. L., and Drucker, D. J. (2002). Structure-function of the glucagon receptor family of G protein-coupled receptors: the glucagon, GIP, GLP-1, and GLP-2 receptors. Recept Channels 8 (3-4), 179–188. doi:10.1080/10606820213687
Bu, L. L., Liu, Y. Q., Shen, Y., Fan, Y., Yu, W. B., Jiang, D. L., et al. (2021). Neuroprotection of exendin-4 by enhanced autophagy in a parkinsonian rat model of α-synucleinopathy. Neurotherapeutics 18 (2), 962–978. doi:10.1007/s13311-021-01018-5
Christ, E., Antwi, K., Fani, M., and Wild, D. (2020). Innovative imaging of insulinoma: the end of sampling? A review. Endocr. Relat. Cancer 27 (4), R79–R92. doi:10.1530/ERC-19-0476
Chen, S. D., Chuang, Y. C., Lin, T. K., and Yang, J. L. (2023). Alternative role of glucagon-like Peptide-1 receptor agonists in neurodegenerative diseases. Eur. J. Pharmacol. 938, 175439. doi:10.1016/j.ejphar.2022.175439
Christ, E., Wild, D., Ederer, S., Behe, M., Nicolas, G., Caplin, M. E., et al. (2013). Glucagon-like peptide-1 receptor imaging for the localisation of insulinomas: A prospective multicentre imaging study. Lancet Diabetes Endocrinol. 1 (2), 115–122. doi:10.1016/S2213-8587(13)70049-4
Christ, E., Wild, D., Forrer, F., Brandle, M., Sahli, R., Clerici, T., et al. (2009). Glucagon-like peptide-1 receptor imaging for localization of insulinomas. J. Clin. Endocrinol. Metab. 94 (11), 4398–4405. doi:10.1210/jc.2009-1082
Clark, C. M., Pontecorvo, M. J., Beach, T. G., Bedell, B. J., Coleman, R. E., Doraiswamy, P. M., et al. (2012). Cerebral PET with florbetapir compared with neuropathology at autopsy for detection of neuritic amyloid-β plaques: A prospective cohort study. Lancet Neurology 11 (8), 669–678. doi:10.1016/S1474-4422(12)70142-4
Connolly, B. M., Vanko, A., McQuade, P., Guenther, I., Meng, X., Rubins, D., et al. (2012). Ex vivo imaging of pancreatic beta cells using a radiolabeled GLP-1 receptor agonist. Mol. Imaging Biol. 14 (1), 79–87. doi:10.1007/s11307-011-0481-7
Das, S. S., Thakral, P., Manda, D., Cb, V., Malik, D., and Sen, I. (2021). Radioguided surgery in insulinoma using (68)Ga labeled exendin-4: A case report. Nucl. Med. Mol. Imaging 55 (5), 253–256. doi:10.1007/s13139-021-00698-3
Deden, L. N., Booij, J., Grandjean, J., Homberg, J. R., Hazebroek, E. J., Gotthardt, M., et al. (2021). Brain imaging of the GLP-1 receptor in obesity using (68)Ga-NODAGA-Exendin-4 PET. Brain Sci. 11 (12), 1647. doi:10.3390/brainsci11121647
Eriksson, O., Rosenstrom, U., Selvaraju, R. K., Eriksson, B., and Velikyan, I. (2017). Species differences in pancreatic binding of DO3A-VS-Cys(40)-Exendin4. Acta Diabetol. 54 (11), 1039–1045. doi:10.1007/s00592-017-1046-2
Eriksson, O., Velikyan, I., Selvaraju, R. K., Kandeel, F., Johansson, L., Antoni, G., et al. (2014). Detection of metastatic insulinoma by positron emission tomography with [(68)ga]exendin-4-a case report. J. Clin. Endocrinol. Metab. 99 (5), 1519–1524. doi:10.1210/jc.2013-3541
Espes, D., Selvaraju, R., Velikyan, I., Krajcovic, M., Carlsson, P. O., and Eriksson, O. (2016). Quantification of beta-cell mass in intramuscular islet grafts using radiolabeled exendin-4. Transpl. Direct 2 (8), e93. doi:10.1097/TXD.0000000000000598
Eter, W. A., Van der Kroon, I., Andralojc, K., Buitinga, M., Willekens, S. M. A., Frielink, C., et al. (2017). Non-invasive in vivo determination of viable islet graft volume by (111)In-exendin-3. Sci. Rep. 7 (1), 7232. doi:10.1038/s41598-017-07815-3
Falconi, M., Eriksson, B., Kaltsas, G., Bartsch, D. K., Capdevila, J., Caplin, M., et al. (2016). ENETS consensus guidelines update for the management of patients with functional pancreatic neuroendocrine tumors and non-functional pancreatic neuroendocrine tumors. Neuroendocrinology 103 (2), 153–171. doi:10.1159/000443171
Gabery, S., Salinas, C. G., Paulsen, S. J., Ahnfelt-Ronne, J., Alanentalo, T., Baquero, A. F., et al. (2020). Semaglutide lowers body weight in rodents via distributed neural pathways. JCI Insight 5 (6), e133429. doi:10.1172/jci.insight.133429
Gao, H., Kiesewetter, D. O., Zhang, X., Huang, X., Guo, N., Lang, L., et al. (2012). PET of glucagonlike peptide receptor upregulation after myocardial ischemia or reperfusion injury. J. Nucl. Med. 53 (12), 1960–1968. doi:10.2967/jnumed.112.109413
Gasbjerg, L. S., Bari, E. J., Christensen, M., and Knop, F. K. (2021). Exendin(9-39)NH2: recommendations for clinical use based on a systematic literature review. Diabetes, Obes. Metabolism 23 (11), 2419–2436. doi:10.1111/dom.14507
Gotthardt, M., Fischer, M., Naeher, I., Holz, J. B., Jungclas, H., Fritsch, H. W., et al. (2002). Use of the incretin hormone glucagon-like peptide-1 (GLP-1) for the detection of insulinomas: initial experimental results. Eur. J. Nucl. Med. Mol. Imaging 29 (5), 597–606. doi:10.1007/s00259-002-0761-1
Guleria, M., Das, T., Amirdhanayagam, J., Sarma, H. D., and Dash, A. (2019). Preparation of [(177)Lu]Lu-DOTA-Ahx-Lys40-Exendin-4 for radiotherapy of insulinoma: A detailed insight into the radiochemical intricacies. Nucl. Med. Biol. 78-79, 31–40. doi:10.1016/j.nucmedbio.2019.11.003
Hepprich, M., Antwi, K., Waser, B., Reubi, J. C., Wild, D., and Christ, E. R. (2020). Brunner's gland hyperplasia in a patient after roux-Y gastric bypass: an important pitfall in GLP-1 receptor imaging. Case Rep. Endocrinol. 2020, 4510910. doi:10.1155/2020/4510910
Holst, J. J. (2007). The physiology of glucagon-like peptide 1. Physiol. Rev. 87 (4), 1409–1439. doi:10.1152/physrev.00034.2006
Hubalewska-Dydejczyk, A., Sowa-Staszczak, A., Tomaszuk, M., and Stefanska, A. (2015). GLP-1 and exendin-4 for imaging endocrine pancreas. a review. Labelled glucagon-like peptide-1 analogues: past, present and future. Q. J. Nucl. Med. Mol. Imaging 59 (2), 152–160.
Isacson, R., Nielsen, E., Dannaeus, K., Bertilsson, G., Patrone, C., Zachrisson, O., et al. (2011). The glucagon-like peptide 1 receptor agonist exendin-4 improves reference memory performance and decreases immobility in the forced swim test. Eur. J. Pharmacol. 650 (1), 249–255. doi:10.1016/j.ejphar.2010.10.008
Jansen, T. J. P., van Lith, S. A. M., Boss, M., Brom, M., Joosten, L., Behe, M., et al. (2019). Exendin-4 analogs in insulinoma theranostics. J. Label. Comp. Radiopharm. 62 (10), 656–672. doi:10.1002/jlcr.3750
Jensen, C. B., Pyke, C., Rasch, M. G., Dahl, A. B., Knudsen, L. B., and Secher, A. (2018). Characterization of the glucagonlike peptide-1 receptor in male mouse brain using a novel antibody and in situ hybridization. Endocrinology 159 (2), 665–675. doi:10.1210/en.2017-00812
Joosten, L., Brom, M., Peeters, H., Heskamp, S., Behe, M., Boerman, O., et al. (2018). Enhanced specific activity by multichelation of exendin-3 leads to improved image quality and in vivo beta cell imaging. Mol. Pharm. 15 (2), 486–494. doi:10.1021/acs.molpharmaceut.7b00853
Juang, J. H., Shen, C. R., Wang, J. J., Wu, S. T., Lin, S. H., Chen, C. Y., et al. (2021). Exendin-4-Conjugated manganese magnetism-engineered iron oxide nanoparticles as a potential magnetic resonance imaging contrast agent for tracking transplanted beta-cells. Nanomater. (Basel) 11 (11), 3145. doi:10.3390/nano11113145
Kaeppeli, S. A. M., Schibli, R., Mindt, T. L., and Behe, M. (2019). Comparison of desferrioxamine and NODAGA for the gallium-68 labeling of exendin-4. EJNMMI Radiopharm. Chem. 4 (1), 9. doi:10.1186/s41181-019-0060-9
Kalff, V., Iravani, A., Akhurst, T., Pattison, D. A., Eu, P., Hofman, M. S., et al. (2021). Utility of (68) Ga-DOTA-Exendin-4 positron emission tomography-computed tomography imaging in distinguishing between insulinoma and nesidioblastosis in patients with confirmed endogenous hyperinsulinaemic hypoglycaemia. Intern Med. J. 51 (10), 1657–1664. doi:10.1111/imj.15141
Keliher, E. J., Reiner, T., Thurber, G. M., Upadhyay, R., and Weissleder, R. (2012). Efficient (18)F-labeling of synthetic exendin-4 analogues for imaging beta cells. ChemistryOpen 1 (4), 177–183. doi:10.1002/open.201200014
Kiesewetter, D. O., Gao, H., Ma, Y., Niu, G., Quan, Q., Guo, N., et al. (2012a). 18F-radiolabeled analogs of exendin-4 for PET imaging of GLP-1 in insulinoma. Eur. J. Nucl. Med. Mol. Imaging 39 (3), 463–473. doi:10.1007/s00259-011-1980-0
Kiesewetter, D. O., Guo, N., Guo, J., Gao, H., Zhu, L., Ma, Y., et al. (2012b). Evaluation of an [(18)F]AlF-NOTA analog of exendin-4 for imaging of GLP-1 receptor in insulinoma. Theranostics 2 (10), 999–1009. doi:10.7150/thno.5276
Kim, D-Y., Kim, H. S., Reder, S., Zheng, J. H., Herz, M., Higuchi, T., et al. (2015). Comparison of 18F-labeled fluoroalkylphosphonium cations with 13N-NH3 for PET myocardial perfusion imaging. J. Nucl. Med. 56 (10), 1581–1586. doi:10.2967/jnumed.115.156794
Kim, D. S., Choi, H. I., Wang, Y., Luo, Y., Hoffer, B. J., and Greig, N. H. (2017). A new treatment strategy for Parkinson's disease through the gut-brain Axis: the glucagon-like peptide-1 receptor pathway. Cell Transpl. 26 (9), 1560–1571. doi:10.1177/0963689717721234
Kimura, H., Fujita, N., Kanbe, K., Matsuda, H., Watanabe, H., Arimitsu, K., et al. (2017). Synthesis and biological evaluation of an (111)In-labeled exendin-4 derivative as a single-photon emission computed tomography probe for imaging pancreatic beta-cells. Bioorg Med. Chem. 25 (20), 5772–5778. doi:10.1016/j.bmc.2017.09.005
Kimura, H., Ogawa, Y., Fujimoto, H., Mukai, E., Kawashima, H., Arimitsu, K., et al. (2018). Evaluation of (18)F-labeled exendin(9-39) derivatives targeting glucagon-like peptide-1 receptor for pancreatic beta-cell imaging. Bioorg Med. Chem. 26 (2), 463–469. doi:10.1016/j.bmc.2017.12.007
King, R. C., Surfraz, M. B-U., Biagini, S. C. G., Blower, P. J., and Mather, S. J. (2007). How do HYNIC-conjugated peptides bind technetium? Insights from LC-MS and stability studies. Dalton Trans. 43.
Klunk, W. E. E. H., Nordberg, A., Wang, Y., Blomqvist, G., Holt, D. P., et al. (2004). Imaging brain amyloid in Alzheimer's disease with Pittsburgh Compound-B. Ann. Neurol. 55 (3), 306–319. doi:10.1002/ana.20009
Knudsen, L. B., and Lau, J. (2019). The discovery and development of liraglutide and semaglutide. Front. Endocrinol. (Lausanne) 10, 155. doi:10.3389/fendo.2019.00155
Korner, M., Stockli, M., Waser, B., and Reubi, J. C. (2007). GLP-1 receptor expression in human tumors and human normal tissues: potential for in vivo targeting. J. Nucl. Med. 48 (5), 736–743. doi:10.2967/jnumed.106.038679
Laviola, L., Leonardini, A., Melchiorre, M., Orlando, M. R., Peschechera, A., Bortone, A., et al. (2012). Glucagon-like peptide-1 counteracts oxidative stress-dependent apoptosis of human cardiac progenitor cells by inhibiting the activation of the c-Jun N-terminal protein kinase signaling pathway. Endocrinology 153 (12), 5770–5781. doi:10.1210/en.2012-1461
Li, J., Peng, J., Tang, W., Rawson, J., Karunananthan, J., Jung, M., et al. (2020). Synthesis and evaluation of (18)F-PTTCO-Cys(40)-Exendin-4 for PET imaging of ectopic insulinomas in rodents. Bioorg Chem. 98, 103718. doi:10.1016/j.bioorg.2020.103718
Liu, Y., Xu, Y., Li, M., Pan, D., Li, Y., Wang, Y., et al. (2020). Clinicopathologic characterisation of myeloid neoplasms with concurrent spliceosome mutations and myeloproliferative-neoplasm-associated mutations. Neurosci. Lett. 73, 728–736. doi:10.1136/jclinpath-2020-206495
Luo, Y., Li, N., Kiesewetter, D. O., Chen, X., and Li, F. (2016a). 68Ga-NOTA-Exendin-4 PET/CT in localization of an occult insulinoma and appearance of coexisting esophageal carcinoma. Clin. Nucl. Med. 41 (4), 341–343. doi:10.1097/RLU.0000000000001087
Luo, Y., Pan, Q., Yao, S., Yu, M., Wu, W., Xue, H., et al. (2016b). Glucagon-Like peptide-1 receptor PET/CT with 68Ga-NOTA-Exendin-4 for detecting localized insulinoma: A prospective cohort study. J. Nucl. Med. 57 (5), 715–720. doi:10.2967/jnumed.115.167445
Luo, Y., Yu, M., Pan, Q., Wu, W., Zhang, T., Kiesewetter, D. O., et al. (2015). 68Ga-NOTA-exendin-4 PET/CT in detection of occult insulinoma and evaluation of physiological uptake. Eur. J. Nucl. Med. Mol. Imaging 42 (3), 531–532. doi:10.1007/s00259-014-2946-9
Lv, M., Xue, G., Cheng, H., Meng, P., Lian, X., Holscher, C., et al. (2021). The GLP-1/GIP dual-receptor agonist DA5-CH inhibits the NF-κB inflammatory pathway in the MPTP mouse model of Parkinson's disease more effectively than the GLP-1 single-receptor agonist NLY01. Brain Behav. 11 (8), e2231. doi:10.1002/brb3.2231
Ma, Z. Y., Gong, Y. F., Zhuang, H. K., Zhou, Z. X., Huang, S. Z., Zou, Y. P., et al. (2020). Pancreatic neuroendocrine tumors: A review of serum biomarkers, staging, and management. World J. Gastroenterol. 26 (19), 2305–2322. doi:10.3748/wjg.v26.i19.2305
Mahapatra, M. K., Karuppasamy, M., and Sahoo, B. M. (2022). Therapeutic potential of semaglutide, a newer GLP-1 receptor agonist, in abating obesity, non-alcoholic steatohepatitis and neurodegenerative diseases: A narrative review. Pharm. Res. 39 (6), 1233–1248. doi:10.1007/s11095-022-03302-1
Mansur, R. B., Fries, G. R., Trevizol, A. P., Subramaniapillai, M., Lovshin, J., Lin, K., et al. (2019). The effect of body mass index on glucagon-like peptide receptor gene expression in the post mortem brain from individuals with mood and psychotic disorders. Eur. Neuropsychopharmacol. 29 (1), 137–146. doi:10.1016/j.euroneuro.2018.10.007
Meier, J. J. (2021). Efficacy of semaglutide in a subcutaneous and an oral formulation. Front. Endocrinol. (Lausanne). 12, 645617. doi:10.3389/fendo.2021.645617
Michalski, K., Laubner, K., Stoykow, C., Omrane, M. A., Maecke, H. R., Seufert, J., et al. (2020). Detection of insulinomas using dual-time-point 68Ga-DOTA-Exendin 4 PET/CT. Clin. Nucl. Med. 45 (7), 519–524. doi:10.1097/RLU.0000000000003093
Moonschi, F. H., Hughes, C. B., Mussman, G. M., Fowlkes, J. L., Richards, C. I., and Popescu, I. (2017). Advances in micro- and nanotechnologies for the GLP-1-based therapy and imaging of pancreatic beta-cells. Acta Diabetol. 55 (5), 405–418. doi:10.1007/s00592-017-1086-7
Mori, Y., Matsui, T., Hirano, T., and Yamagishi, S. I. (2020). GIP as a potential therapeutic target for atherosclerotic cardiovascular disease-A systematic review. Int. J. Mol. Sci. 21 (4), 1509. doi:10.3390/ijms21041509
Murakami, T., Fujimoto, H., Hamamatsu, K., Yamauchi, Y., Kodama, Y., Fujita, N., et al. (2021a). Distinctive detection of insulinoma using [(18)F]FB(ePEG12)12-exendin-4 PET/CT. Sci. Rep. 11 (1), 15014. doi:10.1038/s41598-021-94595-6
Murakami, T., Fujimoto, H., and Inagaki, N. (2021b). Non-invasive beta-cell imaging: visualization, quantification, and beyond. Front. Endocrinol. (Lausanne) 12, 714348. doi:10.3389/fendo.2021.714348
Pabreja, K., Mohd, M. A., Koole, C., Wootten, D., and Furness, S. G. (2014). Molecular mechanisms underlying physiological and receptor pleiotropic effects mediated by GLP-1R activation. Br. J. Pharmacol. 171 (5), 1114–1128. doi:10.1111/bph.12313
Pach, D, Sowa-Staszczak, A, Jabrocka-Hybel, A, Stefanska, A, Tomaszuk, M, Mikolajczak, R, et al. (2013). Glucagon-Like peptide-1 receptor imaging with [Lys (40) (Ahx-HYNIC- (99 m) Tc/EDDA)NH 2 ]-Exendin-4 for the diagnosis of recurrence or dissemination of medullary thyroid cancer: A preliminary report. Int. J. Endocrinol. 2013, 384508. doi:10.1155/2013/384508
Papaefthymiou, A., Laskaratos, F. M., Koffas, A., Manolakis, A., Gkolfakis, P., Coda, S., et al. (2022). State of the art in endoscopic therapy for the management of gastroenteropancreatic neuroendocrine tumors. Curr. Treat. Options Oncol. 23 (7), 1014–1034. doi:10.1007/s11864-022-00986-w
Pattou, F., Kerr-Conte, J., and Wild, D. (2010). GLP-1-receptor scanning for imaging of human beta cells transplanted in muscle. N. Engl. J. Med. 363 (13), 1289–1290. doi:10.1056/NEJMc1004547
Qiu, L., Lan, L., Cai, L., Chen, L., and Chen, Y. (2023). 68Ga-DOTA-Exendin-4 PET/CT demonstrated a higher detection efficacy for double-primary insulinomas than MRI: A case report. Clin. Nucl. Med. 48 (3), 286–287. doi:10.1097/RLU.0000000000004508
Refardt, J., Hofland, J., Kwadwo, A., Nicolas, G. P., Rottenburger, C., Fani, M., et al. (2021). Theranostics in neuroendocrine tumors: an overview of current approaches and future challenges. Rev. Endocr. Metab. Disord. 22 (3), 581–594. doi:10.1007/s11154-020-09552-x
Reubi, J. C., Perren, A., Rehmann, R., Waser, B., Christ, E., Callery, M., et al. (2010). Glucagon-like peptide-1 (GLP-1) receptors are not overexpressed in pancreatic islets from patients with severe hyperinsulinaemic hypoglycaemia following gastric bypass. Diabetologia 53 (12), 2641–2645. doi:10.1007/s00125-010-1901-y
Reubi, J. C., and Waser, B. (2003). Concomitant expression of several peptide receptors in neuroendocrine tumours: molecular basis for in vivo multireceptor tumour targeting. Eur. J. Nucl. Med. Mol. Imaging 30 (5), 781–793. doi:10.1007/s00259-003-1184-3
Salameh, T. S., Rhea, E. M., Talbot, K., and Banks, W. A. (2020). Brain uptake pharmacokinetics of incretin receptor agonists showing promise as Alzheimer's and Parkinson's disease therapeutics. Biochem. Pharmacol. 180, 114187. doi:10.1016/j.bcp.2020.114187
Selvaraju, R. K., Velikyan, I., Asplund, V., Johansson, L., Wu, Z., Todorov, I., et al. (2014). Pre-clinical evaluation of [(68)Ga]Ga-DO3A-VS-Cys(40)-Exendin-4 for imaging of insulinoma. Nucl. Med. Biol. 41 (6), 471–476. doi:10.1016/j.nucmedbio.2014.03.017
Senica, K., Tomazic, A., Skvarca, A., Kolenc Peitl, P., Mikolajczak, R., Hubalewska-Dydejczyk, A., et al. (2020). Superior diagnostic performance of the GLP-1 receptor agonist [Lys(40)(AhxHYNIC-[(99m)Tc]/EDDA)NH(2)]-exendin-4 over conventional imaging modalities for localization of insulinoma. Mol. Imaging Biol. 22 (1), 165–172. doi:10.1007/s11307-019-01372-z
Shah, P., Rahman, S. A., Demirbilek, H., Guemes, M., and Hussain, K. (2017). Hyperinsulinaemic hypoglycaemia in children and adults. Lancet Diabetes Endocrinol. 5 (9), 729–742. doi:10.1016/S2213-8587(16)30323-0
Shah, R., Garg, R., Majmundar, M., Purandare, N., Malhotra, G., Patil, V., et al. (2021). Exendin-4-based imaging in insulinoma localization: systematic review and meta-analysis. Clin. Endocrinol. (Oxf). 95 (2), 354–364. doi:10.1111/cen.14406
Shah, R., Sehemby, M., Garg, R., Purandare, N., Hira, P., Mahajan, A., et al. (2022). 68) Ga-DOTATATE PET/CT imaging in endogenous hyperinsulinemic hypoglycemia: A tertiary endocrine centre experience. Clin. Endocrinol. (Oxf). 96 (2), 190–199. doi:10.1111/cen.14586
Smits, M. M., and Van Raalte, D. H. (2021). Safety of semaglutide. Front. Endocrinol. (Lausanne) 12, 645563. doi:10.3389/fendo.2021.645563
Sood, A., Basher, R. K., Kang, M., Shukla, J., Behera, A., Walia, R., et al. (2019). 68Ga-DOTA-Exendin PET-MRI fusion imaging in a case of insulinoma. Clin. Nucl. Med. 44 (7), e428–e430. doi:10.1097/RLU.0000000000002620
Sowa-Staszczak, A., Pach, D., Mikolajczak, R., Macke, H., Jabrocka-Hybel, A., Stefanska, A., et al. (2013). Glucagon-like peptide-1 receptor imaging with [Lys40(Ahx-HYNIC- 99mTc/EDDA)NH2]-exendin-4 for the detection of insulinoma. Eur. J. Nucl. Med. Mol. Imaging 40 (4), 524–531. doi:10.1007/s00259-012-2299-1
Sowa-Staszczak, A., Trofimiuk-Muldner, M., Stefanska, A., Tomaszuk, M., Buziak-Bereza, M., Gilis-Januszewska, A., et al. (2016). 99mTc labeled glucagon-like peptide-1-analogue (99mTc-GLP1) scintigraphy in the management of patients with occult insulinoma. PLoS One 11 (8), e0160714. doi:10.1371/journal.pone.0160714
Stahle, M., Kyto, V., Kiugel, M., Liljenback, H., Metsala, O., Kakela, M., et al. (2020). Glucagon-like peptide-1 receptor expression after myocardial infarction: imaging study using (68)Ga-NODAGA-exendin-4 positron emission tomography. J. Nucl. Cardiol. 27 (6), 2386–2397. doi:10.1007/s12350-018-01547-1
Vegt, E., van Eerd, J. E., Eek, A., Oyen, W. J., Wetzels, J. F., de Jong, M., et al. (2008). Reducing renal uptake of radiolabeled peptides using albumin fragments. J. Nucl. Med. 49 (9), 1506–1511. doi:10.2967/jnumed.108.053249
Velikyan, I., Rosenstrom, U., and Eriksson, O. (2017). Fully automated GMP production of [(68)Ga]Ga-DO3A-VS-Cys(40)-Exendin-4 for clinical use. Am. J. Nucl. Med. Mol. Imaging 7 (3), 111–125.
Wang, L., Liu, Y., Xu, Y., Sheng, J., Pan, D., Wang, X., et al. (2018). Age-related change of GLP-1R expression in rats can be detected by [(18)F]AlF-NOTA-MAL-Cys(39)-exendin-4. Brain Res. 1698, 213–219. doi:10.1016/j.brainres.2018.08.022
Wang, Y., Lim, K., Normandin, M., Zhao, X., Cline, G. W., and Ding, Y. S. (2012). Synthesis and evaluation of [18F]exendin (9-39) as a potential biomarker to measure pancreatic beta-cell mass. Nucl. Med. Biol. 39 (2), 167–176. doi:10.1016/j.nucmedbio.2011.07.011
Warren, A. M., Topliss, D. J., and Hamblin, P. S. (2020). Successful medical management of insulinoma with diazoxide for 27 years. Endocrinol. Diabetes Metab. Case Rep. 2020, 2020. doi:10.1530/edm-20-0132
Wicki, A., Wild, D., Storch, D., Seemayer, C., Gotthardt, M., Behe, M., et al. (2007). [Lys40(Ahx-DTPA-111In)NH2]-Exendin-4 is a highly efficient radiotherapeutic for glucagon-like peptide-1 receptor-targeted therapy for insulinoma. Clin. Cancer Res. 13 (12), 3696–3705. doi:10.1158/1078-0432.CCR-06-2965
Wild, D., Behe, M., Wicki, A., Storch, D., Waser, B., Gotthardt, M., et al. (2006). [Lys40(Ahx-DTPA-111In)NH2]exendin-4, a very promising ligand for glucagon-like peptide-1 (GLP-1) receptor targeting. J. Nucl. Med. 47 (12), 2025–2033.
Wild, D., Christ, E., Caplin, M. E., Kurzawinski, T. R., Forrer, F., Brandle, M., et al. (2011). Glucagon-like peptide-1 versus somatostatin receptor targeting reveals 2 distinct forms of malignant insulinomas. J. Nucl. Med. 52 (7), 1073–1078. doi:10.2967/jnumed.110.085142
Wild, D., Macke, H., Christ, E., Gloor, B., and Reubi, J. C. (2008). Glucagon-like peptide 1-receptor scans to localize occult insulinomas. N. Engl. J. Med. 359 (7), 766–768. doi:10.1056/NEJMc0802045
Wild, D., Wicki, A., Mansi, R., Behe, M., Keil, B., Bernhardt, P., et al. (2010). Exendin-4-based radiopharmaceuticals for glucagonlike peptide-1 receptor PET/CT and SPECT/CT. J. Nucl. Med. 51 (7), 1059–1067. doi:10.2967/jnumed.110.074914
Wu, Z., Liu, S., Hassink, M., Nair, I., Park, R., Li, L., et al. (2013). Development and evaluation of 18F-TTCO-Cys40-Exendin-4: A PET probe for imaging transplanted islets. J. Nucl. Med. 54 (2), 244–251. doi:10.2967/jnumed.112.109694
Wu, Z., Liu, S., Nair, I., Omori, K., Scott, S., Todorov, I., et al. (2014). 64)Cu labeled sarcophagine exendin-4 for microPET imaging of glucagon like peptide-1 receptor expression. Theranostics 4 (8), 770–777. doi:10.7150/thno.7759
Wu, Z., Todorov, I., Li, L., Bading, J. R., Li, Z., Nair, I., et al. (2011). In vivo imaging of transplanted islets with 64Cu-DO3A-VS-Cys40-Exendin-4 by targeting GLP-1 receptor. Bioconjug Chem. 22 (8), 1587–1594. doi:10.1021/bc200132t
Xu, Q., Zhu, C., Xu, Y., Pan, D., Liu, P., Yang, R., et al. (2015). Preliminary evaluation of [18F]AlF-NOTA-MAL-Cys39-exendin-4 in insulinoma with PET. J. Drug Target 23 (9), 813–820. doi:10.3109/1061186X.2015.1020808
Yue, X., Kiesewetter, D. O., Guo, J., Sun, Z., Zhang, X., Zhu, L., et al. (2013). Development of a new thiol site-specific prosthetic group and its conjugation with [Cys(40)]-exendin-4 for in vivo targeting of insulinomas. Bioconjug Chem. 24 (7), 1191–1200. doi:10.1021/bc400084u
Zhang, L., Li, C., Zhang, Z., Zhang, Z., Jin, Q. Q., Li, L., et al. (2022). DA5-CH and semaglutide protect against neurodegeneration and reduce α-synuclein levels in the 6-OHDA Parkinson's disease rat model. Park. Dis. 2022, 1428817. doi:10.1155/2022/1428817
Zhang, P., Zhao, Z., Zhang, L., Wu, W., Xu, Y., Pan, D., et al. (2019). [(68)Ga]Ga-NOTA-MAL-Cys(39)-exendin-4, a potential GLP-1R targeted PET tracer for the detection of insulinoma. Nucl. Med. Biol. 74-75, 19–24. doi:10.1016/j.nucmedbio.2019.08.002
Zhang, X., Jia, H., Li, F., Fang, C., Zhen, J., He, Q., et al. (2021). Ectopic insulinoma diagnosed by 68Ga-Exendin-4 PET/CT: A case report and review of literature. Med. Baltim. 100 (13), e25076. doi:10.1097/MD.0000000000025076
Zhang, Y., and Chen, W. (2012). Radiolabeled glucagon-like peptide-1 analogues: A new pancreatic beta-cell imaging agent. Nucl. Med. Commun. 33 (3), 223–227. doi:10.1097/MNM.0b013e32834e7f47
Keywords: GLP-1r, molecular imaging, theranostics, challenges, opportunities
Citation: Xie Y, Wang Y, Pei W and Chen Y (2023) Theranostic in GLP-1R molecular imaging: challenges and emerging opportunities. Front. Mol. Biosci. 10:1210347. doi: 10.3389/fmolb.2023.1210347
Received: 22 April 2023; Accepted: 06 September 2023;
Published: 15 September 2023.
Edited by:
Venkateswarlu Kanamarlapudi, Swansea University Medical School, United KingdomReviewed by:
Syed Muhammad Usama, University of Texas at San Antonio, United StatesGregg Stanwood, Florida State University, United States
Ming Yang, University of California, San Diego, United States
Copyright © 2023 Xie, Wang, Pei and Chen. This is an open-access article distributed under the terms of the Creative Commons Attribution License (CC BY). The use, distribution or reproduction in other forums is permitted, provided the original author(s) and the copyright owner(s) are credited and that the original publication in this journal is cited, in accordance with accepted academic practice. No use, distribution or reproduction is permitted which does not comply with these terms.
*Correspondence: Yue Chen, NDY0NDE4NjE3QHFxLmNvbQ==
†These authors have contributed equally to this work and share first authorship