- Section of Cell Biology and Functional Genomics, Division of Diabetes, Endocrinology and Metabolism, Department of Metabolism, Digestion and Reproduction, Imperial College London, London, United Kingdom
Type 2 diabetes (T2D) and obesity have reached epidemic proportions. Incretin therapy is the second line of treatment for T2D, improving both blood glucose regulation and weight loss. Glucagon-like peptide-1 (GLP-1) and glucose-stimulated insulinotropic polypeptide (GIP) are the incretin hormones that provide the foundations for these drugs. While these therapies have been highly effective for some, the results are variable. Incretin therapies target the class B G protein-coupled receptors GLP-1R and GIPR, expressed mainly in the pancreas and the hypothalamus, while some therapeutical approaches include additional targeting of the related glucagon receptor (GCGR) in the liver. The proper functioning of these receptors is crucial for incretin therapy success and here we review several mechanisms at the cellular and molecular level that influence an individual’s response to incretin therapy.
Introduction
Type 2 diabetes (T2D) is one of the major health concerns affecting ∼9% of the global population, putting those affected at risk of cardiovascular-associated mortality (Editors, 2021). T2D is characterized by elevated blood glucose levels resulting from a combined failure of pancreatic beta cell function (reduced or dysregulated insulin secretion) and glucose clearance by peripheral tissues (insulin resistance) (James et al., 2021).
The incretins glucagon-like peptide-1 (GLP-1) and glucose-stimulated insulinotropic polypeptide (GIP) are peptide hormones secreted from intestinal enteroendocrine cells (Baggio and Drucker, 2007) that regulate blood glucose levels by potentiating insulin secretion in response to nutrient intake as well as promoting beta cell survival (Nauck and Meier, 2018), with the “incretin effect” referring to the greater amount of insulin secreted in response to an oral glucose load relative to an equal intravenous dose. Incretin mimetics are ideal candidate therapies for T2D as they exert their actions specifically during hyperglycaemic conditions and have the additional capacity to regulate body weight (Kim and Egan, 2008).
Since 2005, six incretin therapy drugs have been approved by the FDA (United States) and the MHRA (United Kingdom) for T2D treatment: Exenatide, Lixisenatide, Liraglutide, Dulaglutide, Semaglutide and Tirzepatide, all of which are administered daily or once weekly via subcutaneous injection; although Semaglutide is now also available as an oral medication (Latif et al., 2022). Initially, incretin therapies focused on synthesizing GLP-1 analogues that were resistant to degradation by dipeptidyl peptidase-4 (DPP-4), which rapidly breaks down endogenous GLP-1 (Röhrborn et al., 2015). Exenatide, purified from the Gila monster Heloderma suspectum, has an increased binding affinity to the GLP-1 receptor (GLP-1R) and reduced rate of degradation by DPP-4 compared to GLP-1, increasing its half-life from 1 min to over 2 h (Gao and Jusko, 2011).
Three classes of DPP-4 inhibitors (Gliptins) are in clinical use to augment the endogenous incretin effect, and these provide 80%–90% efficacy in inhibiting the peptidase; however, DPP-4 inhibitors are not administered in conjunction with incretin therapies (Gallwitz, 2019).
All GLP-1R agonists reduce food intake, body weight, and HbA1c and improve both blood pressure and blood lipid profiles. These benefits were highlighted in 2019 when the Lancet published a meta-analysis of seven clinical trials showing that these treatments reduced hospital admission by 9%, all-cause mortality by 12% and deaths associated with kidney disease by 17% (Kristensen et al., 2019). Then, in 2021, Semaglutide administered once weekly over 68 weeks was shown to result in 32% of participants losing >20% of their body mass compared to only 1.7% in controls. This study also showed that 86.4% of individuals lost ≥ 5% of their body mass (Wilding et al., 2021). Moreover, last year a separate study showed that 91% of obese individuals receiving a weekly dose of 15 mg Tirzepatide—a recently developed GLP-1R/GIPR dual agonist, lost 5% of their body mass with 36% of participants on the same dosage losing at least 25% (Jastreboff et al., 2022).
While these data are astounding—with yearly progress in efficacy, we should consider that around 9% of patients receiving these game-changer treatments (Killworth, 2021) were not able to lose even 5% of their body mass over 72 weeks, and some trials are still struggling to get participants’ HbA1c below 7% (Table 1). Additionally, incretin therapies can have overbearing negative side effects including hypoglycaemia (often in combination with other glucose-lowering treatments), nausea, vomiting, gastrointestinal upset (including diarrhoea) and injection site reactions that contribute to therapy discontinuation (Trujillo et al., 2021); this review highlights the current research investigating the modifiable and non-modifiable factors contributing to the ‘non-responder’ phenotype to incretin therapies.
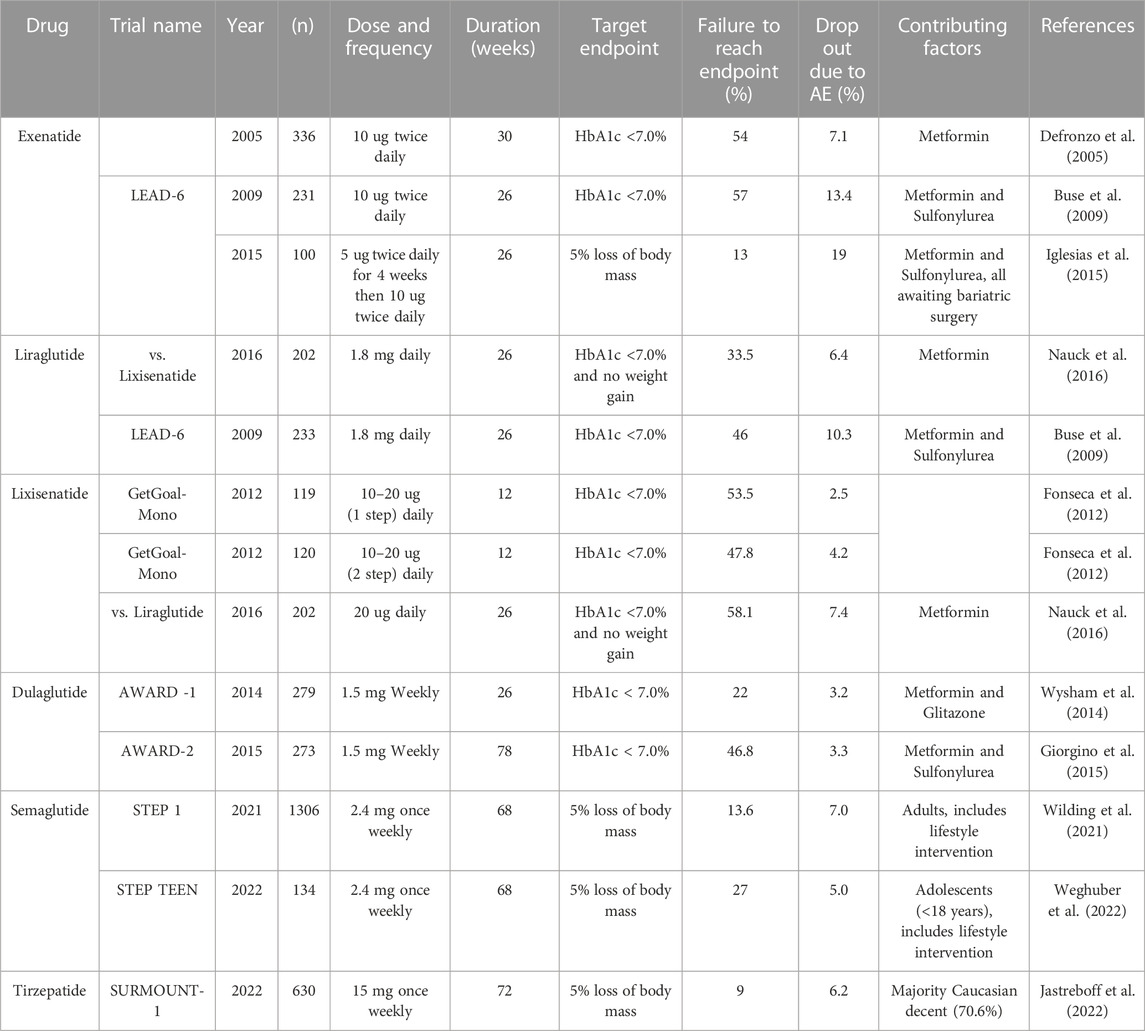
TABLE 1. Incretin therapy studies including percentage of participants who failed to reach the desired endpoint.
Incretin signalling and biased agonism: Basic concepts
Both GLP-1 and GIP stimulate insulin secretion from pancreatic beta cells, with GIP also stimulating alpha cells to secrete glucagon (Manchanda et al., 2022). These functions occur via binding and activation of their cognate secretin-like (class B) G protein-coupled receptors (GPCRs). Orthosteric binding of incretin peptides to the N-terminus (extracellular) region of the receptors causes a conformational change, stabilizing a binding site in the intracellular region that allows coupling to and activation of heterotrimeric guanine nucleotide-binding protein (G proteins) (Chen and Tesmer, 2022). Heterotrimeric G protein activation causes their a and βγ subunits to dissociate triggering different signalling cascades. For the GLP-1R and the GIPR [and also relevant for the other member of the family, the glucagon receptor (GCGR)], the predominant G protein recruited is Gαs that activates adenylate cyclase (mainly isoforms 5 and 8), triggering the production of the second messenger cyclic adenosine monophosphate (cAMP) from intracellular ATP (Anton et al., 2022) and subsequent downstream signalling. Alternatively, Gαi subunits inhibit adenylate cyclase activity and Gαq triggers the mobilisation of Ca2+ ions via phospholipase Cβ activity that produces inositol-1,4,5 triphosphate (IP3) from phosphatidylinositol 4-5-bisphosphate (PIP2). Additionally, dissociated Gβγ subunits can also trigger signalling that leads to activation of both the calcium and the extracellular signal-regulated kinase (ERK1/2) pathways (Lymperopoulos et al., 2022).
After activation of signal transduction, the process is then turned off in a stepwise manner. G protein-coupled receptor kinases (GRKs) phosphorylate specific sites at the receptor C-terminal tail, providing binding sites for β-arrestins (Arcones et al., 2021), scaffold proteins involved in the desensitization and internalization of GPCRs, as well as engaging in their specific signalling (van Gastel et al., 2018). When coupled to β-arrestins, GPCRs are desensitized by abrogated coupling to G proteins and trafficked away from the cell surface, destined for either re-sensitization following their recycling back to the plasma membrane, or for final signal termination resulting from their degradation in lysosomes (McCorvy et al., 2017). Additionally, internalized receptors and GPCR:β-arrestin complexes can continue to signal from the endosome, with this process potentially engaging alternative signalling cascades (Caengprasath et al., 2020). In this context, ligand-associated biased agonism is defined as the observation that certain ligands (in comparison to a reference compound) specifically favour either G protein or β-arrestin transducer pathways—within a particular GPCR (Jones, 2021; Kolb et al., 2022), an effect that has been described for the GLP-1R and exploited by some GLP-1R agonists in clinical use such as Tirzepatide (Willard et al., 2020). While beneficial effects are observed when compounds trigger bias at the GLP-1R towards G protein signalling (Jones et al., 2018), complete blockade of β-arrestin action is detrimental (Kuna et al., 2013). This beneficial effect is evident in the G protein-biased agonist Exendin-phe1, derived from a single phenylalanine substitution at position 1 in Exenatide, which displays improved receptor recycling, reduced receptor desensitization, and sustained incretin-stimulated insulin secretion (Jones et al., 2018). Similar G protein-biased agonists have also been developed for the GIPR and the GCGR, but the full extent of their effects on beta cell function is still unknown (Jones et al., 2021).
Genetic variation at the incretin receptors
The most direct answer to why people fail to respond to incretin therapies is that they might express non-functional incretin receptors. Genetic missense variants in GLP-1R, GIPR and GCGR have been identified, with both gain of function (GoF) and loss of function (LoF) effects. GoF receptors are often characterised by a greater propensity for G protein versus β-arrestin recruitment and signalling, slower rate of receptor internalisation and rapid recycling back to the plasma membrane, with these characteristics being independent of their surface expression levels (Liu et al., 2022). LoF variants may be misfolded, reducing successful biosynthesis and surface expression; or they might display enhanced β-arrestin recruitment, higher degradation rates and/or defective ligand binding ability (Tao and Conn, 2014). A missense variant in the GIPR, E354Q—whereby a glutamine (Q) at amino acid position 354 replaces a glutamic acid (E), identified in a genome-wide association study (GWAS), is linked with increased risk of T2D and higher BMI (Speliotes et al., 2010). GIPR E354Q was characterized in differentiated adipocytes as having a higher rate of desensitization and reduced recycling capacity, all markers of enhanced β-arrestin recruitment. While these features are associated with a LoF phenotype, other studies suggest that both GIPR agonists and antagonists may provide benefits to T2D and obesity (Yang et al., 2022), indicating a complex mechanism of GIPR action within the processes of blood glucose and weight regulation. A suggested mechanism involves prolonged GIPR agonism causing receptor degradation, reducing sustained GIP signalling and, when combined with GLP-1R agonism, causing increased weight loss (Killion et al., 2020). However, it remains an open question how both activation and inhibition of GIPR can lead to beneficial effects, and whether the presence of the E354Q variant at the GIPR may affect the efficacy of GLP-1R agonists (GLP-1RAs).
The GLP-1R has itself several LoF variants including R380C, shown by two independent groups to have normal surface expression but reduced affinity for Exenatide (Wootten et al., 2016; Hegron et al., 2023). Another LoF variant, R421W, displays reduced coupling to mini-Gαs proteins in response to various endogenous and clinical agonists (Lagou et al., 2021). Additionally, the T149M variant is associated with increased T2D risk and has normal surface expression but reduced binding affinity for GLP-1 and Exenatide, as well as reduced cAMP production (Beinborn et al., 2005), although a study was able to rescue both cAMP and ERK1/2 signalling for this variant using the allosteric modulator Compound 2 (Koole et al., 2011). On the other hand, the GLP-1R GoF variant R131Q is associated with decreased T2D risk in the Japanese population (Suzuki et al., 2019). Patients heterozygous for R131Q secrete at least double the amount of insulin in response to GLP-1 during a hyperglycaemic clamp (Sathananthan et al., 2010). Another GoF variant, A316T, shows a 2-fold increase in cAMP accumulation (Hegron et al., 2023), and increased Ca2+ mobilization (which is essential for insulin secretion). Furthermore, A316T shows enhanced recruitment of Gαs and endocytosis in response to oxyntomodulin, GLP-1, Semaglutide and Tirzepatide, but not Exenatide (Lagou et al., 2021). Ongoing research efforts into this variant aim to uncover the molecular factors associated with its benefits, focusing on the analysis of transducer factor recruitment and receptor trafficking patterns in response to a range of clinical and non-clinical GLP-1RAs.
Membrane composition and incretin receptor action
Dietary polyunsaturated fatty acids are essential to human health, they play important roles in both membrane phospholipid composition, which increases plasma membrane fluidity, and provide the backbone to several signalling lipids (Baccouch et al., 2023). The ability of receptors to move within the cellular plasma membrane and into intracellular compartments is vital to regulate their function (Fang et al., 2020) and an individual’s cellular membrane composition may be altered because of diet, medications and genetic factors (Sabapathy et al., 2022). For example, mice fed a high-fat diet for 21 days have both lipid and protein compositional changes in cellular plasma membranes that result in an impaired capacity for oxidative phosphorylation, increased reactive oxygen species (ROS) production, hepatosteatosis and insulin resistance (Kahle et al., 2015). In humans, dietary related alterations in hepatocyte membrane composition, including an increase in phosphatidylethanolamines, have been found to contribute to metabolic-associated fatty liver disease through mitochondrial dysfunction causing the production of ROS, lipid accumulation and loss of insulin sensitivity (Shama et al., 2023). Additionally, in obese humans, visceral fat deposits accumulate where the membrane composition regulating protein ATB8B1 (or flippase) is upregulated (Motahari-Rad et al., 2022). These studies all indicate that diet can influence the make-up of cell membranes and thus, we hypothesise that it may have a potential direct regulatory impact on incretin receptor function in target cells.
Cholesterol is a particularly important lipid for the regulation of incretin receptor function. Lipid rafts (or cholesterol-rich nanodomains) are regions within the plasma membrane where weak binding between cholesterol and other phospholipids allows specific protein interactions to occur (Ho et al., 2022). The GLP-1R forms clusters within lipid rafts when activated with Exenatide, an effect not observed for the GIPR, which presents with a higher level of pre-clustering in basal conditions (Buenaventura et al., 2019). Palmitoylation of the GLP-1R, referring to the reversible attachment of a palmitate moiety to a cysteine residue in the C-terminal tail of the receptor, also contributes to its stabilisation within lipid rafts. Clustering is also important for the recurring activation of GLP-1R, and this capacity appears to be regulated by GLP-1R localization to these cholesterol-rich membrane nanodomains (Buenaventura et al., 2019). Although cholesterol is essential for lipid raft formation, the effects of cholesterol on GPCR function are complex (Kiriakidi et al., 2019). Results from a study where methyl-β-cyclodextrin was used to deplete cellular cholesterol showed that this lipid is required for serotonin receptor stability and preservation of signal potential (Saxena and Chattopadhyay, 2012), as well as for effective serotonin control of food intake and prevention of hyperphagia (van Galen et al., 2021). For the GCGR, however, cholesterol levels are negatively associated with receptor function as demonstrated by the fact that treatment with the cholesterol-lowering drug simvastatin increases ex vivo cAMP levels in response to GCG stimulation in murine hepatocytes (McGlone et al., 2022). Following these results for the GCGR, we are currently investigating the effects of statin treatment on GLP-1R function in the pancreas in vivo and ex vivo. Our preliminary findings suggest that prescribed statins may indeed be a factor that can regulate responses to GLP-1RAs. Therefore, exposure to these cholesterol-lowering drugs may impact the effectiveness of incretin-based therapies.
Receptor trafficking involves the internalisation of the active receptor: Ligand complex towards endocytic compartments, followed by its deactivation/dephosphorylation and recycling back to the cell membrane, leading to its re-sensitisation, or trafficking to lysosomal organelles for its degradation and final signal termination (McGlone et al., 2021). Additional receptor destinations involve retrograde transport towards the Golgi apparatus (Bonifacino and Rojas, 2006) or receptor incorporation into exosomes intended to be secreted, potentially playing a role in inter-cellular communication (Isola and Chen, 2016).
Evidence from primary mouse islets and clonal beta cells (INS-1 832/3) shows that Exenatide causes a greater amount of GLP-1R degradation compared to endogenous GLP-1, with this effect apparently due to differences in the susceptibility of the agonists for degradation by the endopeptidase endothelin converting enzyme 1 (ECE1) (Fang et al., 2020). Also, Huntingtin-interacting protein-1 (HIP1), a protein essential to the regulation of clathrin-mediated endocytosis (Metzler et al., 2001) is associated with the control of GLP-1R function. Data from our laboratory (Buenaventura et al., 2018) shows that HIP1 knockdown reduces G protein recruitment to the GLP-1R, with HIP1 being required for effective incretin-stimulated insulin secretion from murine and human beta cell lines and primary human islets. Additionally, in this study we also showed that the endosomal sorting nexin proteins, SNX1 and SNX27, regulate GLP-1R endosomal trafficking, with SNX1 decreasing receptor recycling to the cell membrane while SNX27 having the opposite effect. Therefore, changes in the expression levels of these endocytic trafficking regulators, or mutations in their genes resulting in changes in their function could additionally impact the capacity of GLP-1RAs to exert beneficial effects in some patients.
Effect of the microbiome
The gut microbiome consists of trillions of microorganisms that contribute to the host digestive system, generating metabolites of which the short-chain fatty acids (SCFAs): acetate, propionate and butyrate, are primary components, produced through the fermentation of indigestible polysaccharides (O’Donnell et al., 2023). Short-chain fatty acids are, among other things, ligands for the rhodopsin-like (Class A) GPCRs free fatty acid receptors (FFARs), expressed in the gut and the endocrine pancreas. FFA2 is activated predominantly by propionate and FFA3 by butyrate [EC50 for acetate, propionate and butyrate at FFA2 are 431, 290 and 371 μM respectively, and at FFA3 393, 41 and 33 μM (Brown et al., 2003)].
The gut microbiome is altered in obesity and T2D, an effect known as dysbiosis, where the ratio of the main bacterial phyla (Firmicutes and Bacteroidetes) becomes imbalanced, reducing diversity while the relative abundance of certain bacteria, including opportunistic pathogens, increases (Larsen et al., 2010). A pilot study investigating gut microbiomes of 52 patients receiving either Dulaglutide or Liraglutide determined a microbial signature of GLP-1RA responders (n = 34) and non-responders (n = 18) (Tsai et al., 2022). After adjusting for baseline HbA1c and serum C-peptide, the bacteria Bacteroides dorei and Lachnoclostridium sp, both of which are associated with anti-inflammatory immunomodulation and improved gut barrier integrity (Yoshida et al., 2018), were positively associated with responders. Alternatively, Mitsuokella multacida, a bacterium previously shown to produce trimethylamine oxide, which contributes to atherosclerosis formation (Fu et al., 2020), correlated with GLP-1RA non-responders.
SCFAs are known to indirectly influence the incretin effect (Arora et al., 2021) through the activation of FFA2 and FFA3 on both L and K enteroendocrine cells, triggering the secretion of GLP-1 and GIP, respectively (Ørgaard et al., 2019). Both FFA2 and FFA3 are expressed in pancreatic islets (DiGruccio et al., 2016), and their direct effect on insulin responses has so far provided mixed results. Ex vivo analysis of both murine and human islets has linked acetate and propionate to both increased and decreased glucose-stimulated insulin secretion (GSIS) (Priyadarshini et al., 2015; Tang et al., 2015; Pingitore et al., 2017). Butyrate administered in pharmacological doses (5 mM) directly to ex vivo rat islets can increase GSIS (Wang et al., 2022), but this effect might potentially be due to mechanisms unrelated to its binding to FFARs, such as on chromatin remodelling (Bridgeman et al., 2021). Furthermore (Priyadarshini et al., 2015), found that primary isolated wild-type mouse islets secrete 50% more insulin in a GSIS assay following co-stimulation with acetate and Exendin-4 compared to FFA2 KO islets. In an FFA3 study, propionate signalling via Gαi negatively impacted GLP-1R function, significantly reducing Exendin-4-stimulated potentiation of insulin secretion (Priyadarshini and Layden, 2015). This study also showed that a global KO of FFA3 results in the downregulation of GLP-1R levels in mouse islets. The authors suggest that FFA3 might act to prevent hyperphagia-induced over-secretion of insulin that would cause beta cell exhaustion during dietary-related stress (Priyadarshini et al., 2020). While some progress is being made linking the gut microbiome with obesity and T2D (Perry et al., 2016; Sanna et al., 2019; Utzschneider et al., 2020), a major question remains: Do SCFAs produced in the gut reach the pancreas in relevant concentrations to influence local receptors? Most butyrate synthesized in the gut is utilized by colonocytes, with only 1–12 µM reaching systemic circulation (Stevens and Hume, 1998). Propionate is entirely metabolised by hepatocytes (Guarner and Malagelada, 2003), and acetate, a major substrate for lipogenesis, reaches a systemic concentration of 19–160 µM (Bloemen et al., 2009), meaning that only a small fraction of the acetate produced by the microbiome would be available to reach the pancreatic islets. So, while the gut microbiome is topical, microbial metabolites might have a challenging time directly affecting incretin responses at the pancreas. Alternatively, the pancreas may host its own microbiome, synthesizing SCFAs that might directly activate cognate receptors located in pancreatic islet endocrine cells. In this context, del Castillo et al. (2019) have compared the similarities between rRNA sequences isolated from the pancreas and duodenum of 50 pancreatic cancer and 34 non-cancer patients. Their findings show that the microbial signatures of these two tissues do not differ significantly, and both contain highly diverse microbial communities. They identified that cancer patients have significantly less Lactobacillus in their pancreas. Lactobacilli are lactic acid-producing bacteria capable of generating both acetate and butyrate (Hati et al., 2019). Future investigations tackling the role of the microbiome in the control of blood glucose levels in healthy and T2D conditions, as well as its influence on obesity, may consider including the potential effect that local microbiome metabolites such as SCFAs might have on modulating incretin receptor responses. Overall, the influence of the microbiome on pancreatic islet function and incretin mimetic treatments warrant further investigation.
Functional interactions of incretin receptors with other GPCRs
To understand the function of a specific GPCR, it is fundamental to consider the context in which this receptor is signalling, including both physical and functional interactions with other co-expressed receptors (Figure 1). GPCRs can form dimers and oligomers both with themselves and with other GPCRs, suggesting the existence of mechanisms whereby one receptor can influence the function of another, a concept known as receptor crosstalk (Hur and Kim, 2002).
Multiple previous studies have shown that incretin receptors can form heterodimers, as demonstrated, for example, using bioluminescence resonance energy transfer (BRET) (Schelshorn et al., 2012; Whitaker et al., 2012; Dale et al., 2022). In particular (Roed et al., 2015), have shown that both the GIPR and the GCGR form heteromers with the GLP-1R when expressed in HEK-293 cells. This process negatively affects cAMP production and modulates β-arrestin recruitment to the GLP-1R. Another study also carried out in HEK-293 cells showed that incretin heterodimers can form constitutively and that stimulation of GLP-1R with GLP-1 promotes incretin heterodimerisation whereas GIP stimulation reduces it (Al-Zaid et al., 2022). This study additionally showed that GIP stimulation reduces Gαq and β-arrestin recruitment to the GLP-1R whereas GLP-1 stimulation enhances recruitment of Gαs to the GIPR. Future work is required to determine these effects in pancreatic endocrine cells under endogenous levels of receptor expression.
Additionally, there is potential for functional interaction between incretin receptors and other GPCRs, including the SCFA-activated FFARs, to occur in the islets of Langerhans (as previously discussed), but also in the gut and the hypothalamus, all of which could influence incretin therapy responses. Other examples of potential functional interactions might include the receptor for long-chain saturated fatty acids, FFA1, which is also expressed in pancreatic beta cells, where its activation leads to GSIS potentiation via Gαq transducer pathways (Kimura et al., 2020), as well as in hepatocytes (Secor et al., 2021) and enteroendocrine L and K cells, where it contributes to incretin hormone secretion, with FFA1 KO mice exhibiting obesity on a low-fat diet (Lu et al., 2021). It is also conceivable that GLP-1R functional interactions might occur in appetite control centres of the hypothalamus: For example, FFA4—the receptor for long-chain polyunsaturated fatty acids, that can regulate energy homeostasis (Dragano et al., 2017), and is expressed alongside the GLP-1R in the arcuate nucleus (Singh et al., 2022). Furthermore, the gut-brain-pancreas axis is connected via the parasympathetic vagus nerve with afferent neurons linking the intestinal mucosa, portal vein and hypothalamus. FFA3 is expressed in the enteric sympathetic ganglion (Kimura et al., 2011) and the vagal ganglion (Nøhr et al., 2015) and the GLP-1R is expressed in vagal afferent neurons including the nodose ganglion (Zhang et al., 2020), suggesting the possibility of functional interactions between these two receptors at these neuronal locations. Intraperitoneal injection of butyrate has been shown to regulate food intake in mice via the vagus nerve, causing ERK1/2 phosphorylation in nodose ganglion neurons (Goswami et al., 2018); therefore, investigation of potential individual-specific FFAR/incretin receptor functional interactions in the different locations where these receptors are co-expressed is paramount to determine if co-stimulation of both GPCR families might results in enhanced, reduced or biased GLP-1RA responses, an effect which might be regulated by the action of the microbiome.
Conclusion
In this review, we have discussed several factors that might contribute to an individual’s ability to respond to incretin therapy. Weight loss of at least 5%–10% is known to reduce complications of T2D and improve quality of life (Apovian et al., 2019), and achieving an HbA1c level below 7% (preferably 6.5%) is indicative of sustained blood glucose regulation; therefore, failure to reach these targets under incretin treatment should define a reasonable threshold beyond which a personalised medicine approach needs to be considered. Identifying genetic factors in “non-responders” will help guide treatments, and further research is required to identify muti-targeted approaches that can improve incretin therapies under these conditions. In the meantime, novel dual and tri-incretin agonists are already in development. For example, MEDI0382, a synthetic palmitoylated dual agonist of GLP-1R and GCGR derived from oxyntomodulin, achieved both significant weight loss and blood glucose lowering effects in obese and T2D patients during phase 2 trials (Ambery et al., 2018). G49 is another GLP-1R/GCGR dual agonist shown to improve liver regeneration in non-alcoholic fatty liver disease (Valdecantos et al., 2016). Additionally, a single molecule tri-agonist of GLP-1R, GIPR and GCGR can increase insulin secretion and improve blood glucose handling in mice beyond the cumulative effects of the three individual agonists separately (Khajavi et al., 2018). These benefits were associated with increased Ca2+ mobilisation observed in human clonal pancreatic beta cells. Increased Ca2+ mobilization may be achieved in future novel treatment strategies by co-targeting Gαq-coupled GPCRs present in beta cells, including FFA1 and 2.
The relevance of optimised incretin therapies extends beyond their action on blood glucose regulation and control of body weight, with GLP-1RAs providing beneficial effects for blood pressure disorders (Martins et al., 2020) and neurodegenerative conditions including Alzheimer’s (Bomfim et al., 2012) and Parkinson’s disease (de Pablo-Fernandez et al., 2018). Therefore, with the current strain on health and social care services around the globe, particular investment should be funnelled into this research topic.
Author contributions
All authors listed have made a substantial, direct, and intellectual contribution to the work and approved it for publication.
Funding
This research is supported by MRC grant number MR/R010676/1.
Conflict of interest
The authors declare that the research was conducted in the absence of any commercial or financial relationships that could be construed as a potential conflict of interest.
Publisher’s note
All claims expressed in this article are solely those of the authors and do not necessarily represent those of their affiliated organizations, or those of the publisher, the editors and the reviewers. Any product that may be evaluated in this article, or claim that may be made by its manufacturer, is not guaranteed or endorsed by the publisher.
References
Al-Zaid, B., Chacko, S., Ezeamuzie, C. I., Bünemann, M., Krasel, C., Karimian, T., et al. (2022). Differential effects of glucose-dependent insulinotropic polypeptide receptor/glucagon-like peptide-1 receptor heteromerization on cell signaling when expressed in HEK-293 cells. Pharmacol. Res. Perspect. 10, e01013. doi:10.1002/prp2.1013
Ambery, P., Parker, V. E., Stumvoll, M., Posch, M. G., Heise, T., Plum-Moerschel, L., et al. (2018). MEDI0382, a GLP-1 and glucagon receptor dual agonist, in obese or overweight patients with type 2 diabetes: A randomised, controlled, double-blind, ascending dose and phase 2a study. Lancet 391, 2607–2618. doi:10.1016/S0140-6736(18)30726-8
Anton, S. E., Kayser, C., Maiellaro, I., Nemec, K., Möller, J., Koschinski, A., et al. (2022). Receptor-associated independent cAMP nanodomains mediate spatiotemporal specificity of GPCR signaling. Cell 185, 1130–1142.e11. doi:10.1016/j.cell.2022.02.011
Apovian, C. M., Okemah, J., and O’Neil, P. M. (2019). Body weight considerations in the management of type 2 diabetes. Adv. Ther. 36, 44–58. doi:10.1007/s12325-018-0824-8
Arcones, A. C., Vila-Bedmar, R., Mirasierra, M., Cruces-Sande, M., Vallejo, M., Jones, B., et al. (2021). GRK2 regulates GLP-1R-mediated early phase insulin secretion in vivo. BMC Biol. 19, 40. doi:10.1186/s12915-021-00966-w
Arora, T., Vanslette, A. M., Hjorth, S. A., and Bäckhed, F. (2021). Microbial regulation of enteroendocrine cells. Med 2, 553–570. doi:10.1016/j.medj.2021.03.018
Baccouch, R., Shi, Y., Vernay, E., Mathelié-Guinlet, M., Taib-Maamar, N., Villette, S., et al. (2023). The impact of lipid polyunsaturation on the physical and mechanical properties of lipid membranes. Biochim. Biophys. Acta Biomembr. 1865, 184084. doi:10.1016/j.bbamem.2022.184084
Baggio, L. L., and Drucker, D. J. (2007). Biology of incretins: GLP-1 and GIP. Gastroenterology 132, 2131–2157. doi:10.1053/j.gastro.2007.03.054
Beinborn, M., Worrall, C. I., McBride, E. W., and Kopin, A. S. (2005). A human glucagon-like peptide-1 receptor polymorphism results in reduced agonist responsiveness. Regul. Pept. 130, 1–6. doi:10.1016/j.regpep.2005.05.001
Bloemen, J. G., Venema, K., van de Poll, M. C., Olde Damink, S. W., Buurman, W. A., and Dejong, C. H. (2009). Short chain fatty acids exchange across the gut and liver in humans measured at surgery. Clin. Nutr. 28, 657–661. doi:10.1016/j.clnu.2009.05.011
Bomfim, T. R., Forny-Germano, L., Sathler, L. B., Brito-Moreira, J., Houzel, J.-C., Decker, H., et al. (2012). An anti-diabetes agent protects the mouse brain from defective insulin signaling caused by Alzheimer’s disease-associated Aβ oligomers. J. Clin. Invest. 122, 1339–1353. doi:10.1172/JCI57256
Bonifacino, J. S., and Rojas, R. (2006). Retrograde transport from endosomes to the trans-Golgi network. Nat. Rev. Mol. Cell Biol. 7, 568–579. doi:10.1038/nrm1985
Bridgeman, S., Ellison, G., Newsholme, P., Mamotte, C., Karpinski, P., Sasiadek, M. M., et al. (2021). The HDAC inhibitor butyrate impairs beta cell function and activates the disallowed gene hexokinase I. Int. J. Mol. Sci. 22, 13330. Page 13330 22. doi:10.3390/ijms222413330
Brown, A. J., Goldsworthy, S. M., Barnes, A. A., Eilert, M. M., Tcheang, L., Daniels, D., et al. (2003). The orphan G protein-coupled receptors GPR41 and GPR43 are activated by propionate and other short chain carboxylic acids. J. Biol. Chem. 278, 11312–11319. doi:10.1074/jbc.M211609200
Buenaventura, T., Bitsi, S., Laughlin, W. E., Burgoyne, T., Lyu, Z., Oqua, A. I., et al. (2019). Agonist-induced membrane nanodomain clustering drives GLP-1 receptor responses in pancreatic beta cells. PLoS Biol. 17, e3000097. doi:10.1371/journal.pbio.3000097
Buenaventura, T., Kanda, N., Douzenis, P. C., Jones, B., Bloom, S. R., Chabosseau, P., et al. (2018). A targeted RNAi screen identifies endocytic trafficking factors that control GLP-1 receptor signaling in pancreatic beta cells. Diabetes 67, 385–399. doi:10.2337/db17-0639
Buse, J. B., Rosenstock, J., Sesti, G., Schmidt, W. E., Montanya, E., Brett, J. H., et al. (2009). Liraglutide once a day versus exenatide twice a day for type 2 diabetes: A 26-week randomised, parallel-group, multinational, open-label trial (LEAD-6). Lancet 374, 39–47. doi:10.1016/S0140-6736(09)60659-0
Caengprasath, N., Gonzalez-Abuin, N., Shchepinova, M., Ma, Y., Inoue, A., Tate, E. W., et al. (2020). Internalization-Dependent free fatty acid receptor 2 signaling is essential for propionate-induced anorectic gut hormone release. iScience 23, 101449. doi:10.1016/j.isci.2020.101449
Chen, Q., and Tesmer, J. J. G. (2022). G protein-coupled receptor interactions with arrestins and GPCR kinases: The unresolved issue of signal bias. J. Biol. Chem. 298, 102279. doi:10.1016/j.jbc.2022.102279
Dale, N. C., Johnstone, E. K. M., and Pfleger, K. D. G. (2022). GPCR heteromers: An overview of their classification, function and physiological relevance. Front. Endocrinol. (Lausanne) 13, 931573. doi:10.3389/fendo.2022.931573
de Pablo-Fernandez, E., Goldacre, R., Pakpoor, J., Noyce, A. J., and Warner, T. T. (2018). Association between diabetes and subsequent Parkinson disease: A record-linkage cohort study. Neurology 91, e139–e142. doi:10.1212/WNL.0000000000005771
Defronzo, R. A., Ratner, R. E., Han, J., Kim, D. D., Fineman, M. S., and Baron, A. D. (2005). Effects of exenatide (Exendin-4) on glycemic control and weight over 30 Weeks in metformin-treated patients with type 2 diabetes. Diabetes Care 28 (5), 1092–1100. doi:10.2337/diacare.28.5.1092
del Castillo, E., Meier, R., Chung, M., Koestler, D. C., Chen, T., Paster, B. J., et al. (2019). The microbiomes of pancreatic and duodenum tissue overlap and are highly subject specific but differ between pancreatic cancer and noncancer subjects. Cancer Epidemiol. Biomarkers Prev. 28, 370–383. doi:10.1158/1055-9965.EPI-18-0542
DiGruccio, M. R., Mawla, A. M., Donaldson, C. J., Noguchi, G. M., Vaughan, J., Cowing-Zitron, C., et al. (2016). Comprehensive alpha, beta and delta cell transcriptomes reveal that ghrelin selectively activates delta cells and promotes somatostatin release from pancreatic islets. Mol. Metab. 5, 449–458. doi:10.1016/j.molmet.2016.04.007
Dragano, N. R. V., Solon, C., Ramalho, A. F., de Moura, R. F., Razolli, D. S., Christiansen, E., et al. (2017). Polyunsaturated fatty acid receptors, GPR40 and GPR120, are expressed in the hypothalamus and control energy homeostasis and inflammation. J. Neuroinflammation 14, 91. doi:10.1186/s12974-017-0869-7
Fang, Z., Chen, S., Manchanda, Y., Bitsi, S., Pickford, P., David, A., et al. (2020). Ligand-specific factors influencing glp-1 receptor post-endocytic trafficking and degradation in pancreatic beta cells. Int. J. Mol. Sci. 21, 8404–8424. doi:10.3390/ijms21218404
Fonseca, V. A., Alvarado-Ruiz, R., Raccah, D., Boka, G., Miossec, P., Gerich, J. E., et al. (2012). Efficacy and safety of the once-daily GLP-1 receptor agonist lixisenatide in monotherapy: A randomized, double-blind, placebo-controlled trial in patients with type 2 diabetes (GetGoal-Mono). Diabetes Care 35, 1225–1231. doi:10.2337/dc11-1935
Fu, B. C., Hullar, M. A., Randolph, T. W., Franke, A. A., Monroe, K. R., Cheng, I., et al. (2020). Associations of plasma trimethylamine N-oxide, choline, carnitine, and betaine with inflammatory and cardiometabolic risk biomarkers and the fecal microbiome in the Multiethnic Cohort Adiposity Phenotype Study. Am. J. Clin. Nutr. 111, 1226–1234. doi:10.1093/ajcn/nqaa015
Gallwitz, B. (2019). Clinical use of DPP-4 inhibitors. Front. Endocrinol. 10. (Lausanne). doi:10.3389/fendo.2019.00389
Gao, W., and Jusko, W. J. (2011). Pharmacokinetic and pharmacodynamic modeling of exendin-4 in type 2 diabetic Goto-Kakizaki rats. J. Pharmacol. Exp. Ther. 336, 881–890. doi:10.1124/jpet.110.175752
Giorgino, F., Benroubi, M., Sun, J. H., Zimmermann, A. G., and Pechtner, V. (2015). Efficacy and safety of once-weekly dulaglutide versus insulin glargine in patients with type 2 Diabetes on metformin and glimepiride (AWARD-2). Diabetes Care 38, 2241–2249. doi:10.2337/dc14-1625
Goswami, C., Iwasaki, Y., and Yada, T. (2018). Short-chain fatty acids suppress food intake by activating vagal afferent neurons. J. Nutr. Biochem. 57, 130–135. doi:10.1016/j.jnutbio.2018.03.009
Guarner, F., and Malagelada, J.-R. (2003). Gut flora in health and disease. Lancet 361, 512–519. doi:10.1016/S0140-6736(03)12489-0
Hati, S., Patel, M., Mishra, B. K., and Das, S. (2019). Short-chain fatty acid and vitamin production potentials of Lactobacillus isolated from fermented foods of Khasi Tribes, Meghalaya, India. Ann. Microbiol. 69, 1191–1199. doi:10.1007/s13213-019-01500-8
Hegron, A., Hou, G., Charpentier, G., Hospitalier, C., Francilien, S., Derhourhi, M., et al. (2023). Human GLP1R variants affecting GLP1R cell surface expression contribute to impaired glucose control and increased adiposity. Pre Print.
Ho, T. H., Nguyen, T. T., and Huynh, L. K. (2022). Formation of lipid raft nanodomains in homogeneous ternary lipid mixture of POPC/DPSM/cholesterol: Theoretical insights. Biochim. Biophys. Acta Biomembr. 1864, 184027. doi:10.1016/j.bbamem.2022.184027
Hur, E. M., and Kim, K. T. (2002). G protein-coupled receptor signalling and cross-talk: Achieving rapidity and specificity. Cell Signal 14, 397–405. doi:10.1016/s0898-6568(01)00258-3
Iglesias, P., Civantos, S., Vega, B., Pavón, I., Guijarro, G., and Monereo, S. (2015). Clinical effectiveness of exenatide in diabetic patients waiting for bariatric surgery. Obes. Surg. 25, 575–578. doi:10.1007/s11695-014-1563-9
Isola, A. L., and Chen, S. (2016). Exosomes: The link between GPCR activation and metastatic potential? Front. Genet. 7, 56. doi:10.3389/fgene.2016.00056
James, D. E., Stöckli, J., and Birnbaum, M. J. (2021). The aetiology and molecular landscape of insulin resistance. Nat. Rev. Mol. Cell Biol. 22, 751–771. doi:10.1038/s41580-021-00390-6
Jastreboff, A. M., Aronne, L. J., Ahmad, N. N., Wharton, S., Connery, L., Alves, B., et al. (2022). Tirzepatide once weekly for the treatment of obesity. N. Engl. J. Med. 387, 205–216. doi:10.1056/NEJMoa2206038
Jones, B., Buenaventura, T., Kanda, N., Chabosseau, P., Owen, B. M., Scott, R., et al. (2018). Targeting GLP-1 receptor trafficking to improve agonist efficacy. Nat. Commun. 9, 1602. doi:10.1038/s41467-018-03941-2
Jones, B., McGlone, E. R., Fang, Z., Pickford, P., Corrêa, I. R., Oishi, A., et al. (2021). Genetic and biased agonist-mediated reductions in β-Arrestin recruitment prolong cAMP signaling at glucagon family receptors. J. Biol. Chem. 296, 100133. doi:10.1074/jbc.RA120.016334
Kahle, M., Schäfer, A., Seelig, A., Schultheiß, J., Wu, M., Aichler, M., et al. (2015). High fat diet-induced modifications in membrane lipid and mitochondrial-membrane protein signatures precede the development of hepatic insulin resistance in mice. Mol. Metab. 4, 39–50. doi:10.1016/j.molmet.2014.11.004
Khajavi, N., Finan, B., Kluth, O., Müller, T. D., Mergler, S., Schulz, A., et al. (2018). An incretin-based tri-agonist promotes superior insulin secretion from murine pancreatic islets via PLC activation. Cell Signal 51, 13–22. doi:10.1016/j.cellsig.2018.07.006
Killion, E. A., Chen, M., Falsey, J. R., Sivits, G., Hager, T., Atangan, L., et al. (2020). Chronic glucose-dependent insulinotropic polypeptide receptor (GIPR) agonism desensitizes adipocyte GIPR activity mimicking functional GIPR antagonism. Nat. Commun. 11, 4981–5017. doi:10.1038/s41467-020-18751-8
Killworth, H. (2021). Gamechanger” drug for treating obesity cuts body weight by 20% [WWW Document]. London: University College London.
Kim, W., and Egan, J. M. (2008). The role of incretins in glucose homeostasis and diabetes treatment. Pharmacol. Rev. 60 (4), 470–512. doi:10.1124/pr.108.000604
Kimura, I., Ichimura, A., Ohue-Kitano, R., and Igarashi, M. (2020). Free fatty acid receptors in health and disease. Physiol. Rev. 100, 171–210. doi:10.1152/physrev.00041.2018
Kimura, I., Inoue, D., Maeda, T., Hara, T., Ichimura, A., Miyauchi, S., et al. (2011). Short-chain fatty acids and ketones directly regulate sympathetic nervous system via G protein-coupled receptor 41 (GPR41). Proc. Natl. Acad. Sci. U. S. A. 108, 8030–8035. doi:10.1073/pnas.1016088108
Kiriakidi, S., Kolocouris, A., Liapakis, G., Ikram, S., Durdagi, S., and Mavromoustakos, T. (2019). “Effects of cholesterol on GPCR function: Insights from computational and experimental studies,” in Advances in experimental medicine and biology, 89–103.
Kolb, P., Kenakin, T., Alexander, S. P. H., Bermudez, M., Bohn, L. M., Breinholt, C. S., et al. (2022). Community guidelines for GPCR ligand bias: IUPHAR review 32. Br. J. Pharmacol. 179 (14), 3651–3674. doi:10.1111/bph.15811
Koole, C., Wootten, D., Simms, J., Valant, C., Miller, L. J., Christopoulos, A., et al. (2011). Polymorphism and ligand dependent changes in human glucagon-like peptide-1 receptor (GLP-1R) function: Allosteric rescue of loss of function mutation. Mol. Pharmacol. 80, 486–497. doi:10.1124/mol.111.072884
Kristensen, S. L., Rørth, R., Jhund, P. S., Docherty, K. F., Sattar, N., Preiss, D., et al. (2019). Cardiovascular, mortality, and kidney outcomes with GLP-1 receptor agonists in patients with type 2 diabetes: A systematic review and meta-analysis of cardiovascular outcome trials. Lancet Diabetes Endocrinol. 7, 776–785. doi:10.1016/S2213-8587(19)30249-9
Kuna, R. S., Girada, S. B., Asalla, S., Vallentyne, J., Maddika, S., Patterson, J. T., et al. (2013). Glucagon-like peptide-1 receptor-mediated endosomal cAMP generation promotes glucose-stimulated insulin secretion in pancreatic β-cells. Am. J. Physiol. Endocrinol. Metab. 305, E161–E170. doi:10.1152/ajpendo.00551.2012
Lagou, V., Jiang, L., Ulrich, A., Zudina, L., Sofia Gutiérrez González, K., Balkhiyarova, Z., et al. (2021). Random glucose GWAS in 493,036 individuals provides insights into diabetes pathophysiology, complications and treatment stratification. MedRxiv Prepr. 82.
Larsen, N., Vogensen, F. K., van den Berg, F. W. J., Sandris Nielsen, D., Andreasen, A. S., Pedersen, B. K., et al. (2010). Gut microbiota in human adults with type 2 diabetes differs from non-diabetic adults. PLoS One 5, e9085. doi:10.1371/journal.pone.0009085
Latif, W., Lambrinos, K. J., and Rodriguez, R. (2022). Compare and contrast the glucagon-like peptide-1 receptor agonists (GLP1RAs). StatPearls.
Liu, T., Ji, R. L., and Tao, Y. X. (2022). Naturally occurring mutations in G protein-coupled receptors associated with obesity and type 2 diabetes mellitus. Pharmacol. Ther. 234, 108044. doi:10.1016/j.pharmthera.2021.108044
Lu, Z., Li, Y., Syn, W. K., Li, A. J., Ritter, W. S., Wank, S. A., et al. (2021). GPR40 deficiency is associated with hepatic FAT/CD36 upregulation, steatosis, inflammation, and cell injury in C57BL/6 mice. Am. J. Physiol. Endocrinol. Metab. 320, E30–E42. doi:10.1152/ajpendo.00257.2020
Lymperopoulos, A., Suster, M. S., and Borges, J. I. (2022). Short-chain fatty acid receptors and cardiovascular function. Int. J. Mol. Sci. 23, 3303. doi:10.3390/ijms23063303
Manchanda, Y., Bitsi, S., Chen, S., Broichhagen, J., Bernardino De La Serna, J., Jones, B., et al. (2022). An examination of the divergent spatiotemporal signaling of GLP-1R versus GIPR in pancreatic beta cells. BioRxix PrePrint.
Martins, F. L., Bailey, M. A., and Girardi, A. C. C. (2020). Endogenous activation of glucagon-like peptide-1 receptor contributes to blood pressure control: Role of proximal tubule Na+/H+Exchanger isoform 3, renal angiotensin II, and insulin sensitivity. Hypertension 76, 839–848. doi:10.1161/HYPERTENSIONAHA.120.14868
McCorvy, J. D., Butler, K. V., Kelly, B., Rechsteiner, K., Karpiak, J., Betz, R. M., et al. (2017). Structure-inspired design of β-arrestin-biased ligands for aminergic GPCRs. Nature Publishing Group.
McGlone, E. R., Ansell, T. B., Dunsterville, C., Song, W., Carling, D., Tomas, A., et al. (2022). Hepatocyte cholesterol content modulates glucagon receptor signalling. Mol. Metab. 63, 101530. doi:10.1016/j.molmet.2022.101530
McGlone, E. R., Manchanda, Y., Jones, B., Pickford, P., Inoue, A., Carling, D., et al. (2021). Receptor Activity-Modifying Protein 2 (RAMP2) alters glucagon receptor trafficking in hepatocytes with functional effects on receptor signalling. Mol. Metab. 53, 101296. doi:10.1016/j.molmet.2021.101296
Metzler, M., Legendre-Guillemin, V., Gan, L., Chopra, V., Kwok, A., McPherson, P. S., et al. (2001). HIP1 functions in clathrin-mediated endocytosis through binding to clathrin and adaptor protein 2. J. Biol. Chem. 276 (42), 39271–39276. doi:10.1074/jbc.C100401200
Motahari-Rad, H., Subiri, A., Soler, R., Ocaña, L., Alcaide, J., Rodríguez-Capitan, J., et al. (2022). The effect of sex and obesity on the gene expression of lipid flippases in adipose tissue. J. Clin. Med. 11, 3878. doi:10.3390/jcm11133878
Nauck, M. A., and Meier, J. J. (2018). Incretin hormones: Their role in health and disease. Diabetes Obes. Metab. 20, 5–21. doi:10.1111/dom.13129
Nauck, M., Rizzo, M., Johnson, A., Bosch-Traberg, H., Madsen, J., and Cariou, B. (2016). Once-daily liraglutide versus lixisenatide as add-on to metformin in type 2 diabetes: A 26-week randomized controlled clinical trial. Diabetes Care 39, 1501–1509. doi:10.2337/dc15-2479
Nøhr, M. K., Egerod, K. L., Christiansen, S. H., Gille, A., Offermanns, S., Schwartz, T. W., et al. (2015). Expression of the short chain fatty acid receptor GPR41/FFAR3 in autonomic and somatic sensory ganglia. Neuroscience 290, 126–137. doi:10.1016/j.neuroscience.2015.01.040
O’Donnell, J. A., Zheng, T., Meric, G., and Marques, F. Z. (2023). The gut microbiome and hypertension. Nat. Rev. Nephrol. 19, 153–167. doi:10.1038/s41581-022-00654-0
Ørgaard, A., Jepsen, S. L., and Holst, J. J. (2019). Short-chain fatty acids and regulation of pancreatic endocrine secretion in mice. Islets 11, 103–111. doi:10.1080/19382014.2019.1587976
Perry, R. J., Peng, L., Barry, N. A., Cline, G. W., Zhang, D., Cardone, R. L., et al. (2016). Acetate mediates a microbiome-brain-β-cell axis to promote metabolic syndrome.
Pingitore, A., Chambers, E. S., Hill, T., Maldonado, I. R., Liu, B., Bewick, G., et al. (2017). The diet-derived short chain fatty acid propionate improves beta-cell function in humans and stimulates insulin secretion from human islets in vitro. Diabetes Obes. Metab. 19, 257–265. doi:10.1111/dom.12811
Priyadarshini, M., Cole, C., Oroskar, G., Ludvik, A., Wicksteed, B., He, C., et al. (2020). Free fatty acid receptor 3 differentially contributes to β-cell compensation under high-fat diet and streptozotocin stress. Am. J. Physiol. Regul. Integr. Comp. Physiol. 318, 691–700. doi:10.1152/ajpregu.00128.2019
Priyadarshini, M., and Layden, B. T. (2015). FFAR3 modulates insulin secretion and global gene expression in mouse islets. Islets 7, e1045182. doi:10.1080/19382014.2015.1045182
Priyadarshini, M., Villa, S. R., Fuller, M., Wicksteed, B., Mackay, C. R., Alquier, T., et al. (2015). An acetate-specific GPCR, FFAR2, regulates insulin secretion. Mol. Endocrinol. 29, 1055–1066. doi:10.1210/me.2015-1007
Roed, S. N., Nøhr, A. C., Wismann, P., Iversen, H., Bräuner-Osborne, H., Knudsen, S. M., et al. (2015). Functional consequences of glucagon-like peptide-1 receptor cross-talk and trafficking. J. Biol. Chem. 290, 1233–1243. doi:10.1074/jbc.M114.592436
Röhrborn, D., Wronkowitz, N., and Eckel, J. (2015). DPP4 in diabetes. Front. Immunol. 6, 386. doi:10.3389/fimmu.2015.00386
Sabapathy, T., Helmerhorst, E., Ellison, G., Bridgeman, S. C., and Mamotte, C. D. (2022). High-fat diet induced alterations in plasma membrane cholesterol content impairs insulin receptor binding and signalling in mouse liver but is ameliorated by atorvastatin. Biochim. Biophys. Acta Mol. Basis Dis. 1868, 166372. doi:10.1016/j.bbadis.2022.166372
Sanna, S., van Zuydam, N. R., Mahajan, A., Kurilshikov, A., Vich Vila, A., Võsa, U., et al. (2019). Causal relationships among the gut microbiome, short-chain fatty acids and metabolic diseases. Nat. Genet. 51, 600–605. doi:10.1038/s41588-019-0350-x
Sathananthan, A., Man, C. D., Micheletto, F., Zinsmeister, A. R., Camilleri, M., Giesler, P. D., et al. (2010). Common genetic variation in GLP1R and insulin secretion in response to exogenous GLP-1 in nondiabetic subjects: A pilot study. Diabetes Care 33, 2074–2076. doi:10.2337/dc10-0200
Saxena, R., and Chattopadhyay, A. (2012). Membrane cholesterol stabilizes the human serotonin(1A) receptor. Biochim. Biophys. Acta 1818, 2936–2942. doi:10.1016/j.bbamem.2012.07.032
Schelshorn, D., Joly, F., Mutel, S., Hampe, C., Breton, B., Mutel, V., et al. (2012). Lateral allosterism in the glucagon receptor family: Glucagon-like peptide 1 induces G-protein-coupled receptor heteromer formation. Mol. Pharmacol. 81, 309–318. doi:10.1124/mol.111.074757
Secor, J. D., Fligor, S. C., Tsikis, S. T., Yu, L. J., and Puder, M. (2021). Free fatty acid receptors as mediators and therapeutic targets in liver disease. Front. Physiol. 12, 656441. doi:10.3389/fphys.2021.656441
Shama, S., Jang, H., Wang, X., Zhang, Y., Shahin, N. N., Motawi, T. K., et al. (2023). Phosphatidylethanolamines are associated with nonalcoholic fatty liver disease (NAFLD) in obese adults and induce liver cell metabolic perturbations and hepatic stellate cell activation. Int. J. Mol. Sci. 24, 1034. doi:10.3390/ijms24021034
Singh, I., Wang, L., Xia, B., Liu, J., Tahiri, A., El Ouaamari, A., et al. (2022). Activation of arcuate nucleus glucagon-like peptide-1 receptor-expressing neurons suppresses food intake. Cell Biosci. 12, 178. doi:10.1186/s13578-022-00914-3
Speliotes, E. K., Willer, C. J., Berndt, S. I., Monda, K. L., Thorleifsson, G., Jackson, A. U., et al. (2010). Association analyses of 249,796 individuals reveal 18 new loci associated with body mass index. Nat. Genet. 42, 937–948. doi:10.1038/ng.686
Stevens, C. E., and Hume, I. D. (1998). Contributions of microbes in vertebrate gastrointestinal tract to production and conservation of nutrients. Physiol. Rev. 78, 393–427. doi:10.1152/physrev.1998.78.2.393
Suzuki, K., Akiyama, M., Ishigaki, K., Kanai, M., Hosoe, J., Shojima, N., et al. (2019). Identification of 28 new susceptibility loci for type 2 diabetes in the Japanese population. Nat. Genet. 51, 379–386. doi:10.1038/s41588-018-0332-4
Tang, C., Ahmed, K., Gille, A., Lu, S., Gröne, H. J., Tunaru, S., et al. (2015). Loss of FFA2 and FFA3 increases insulin secretion and improves glucose tolerance in type 2 diabetes. Nat. Med. 21, 173–177. doi:10.1038/nm.3779
Tao, Y.-X., and Conn, P. M. (2014). Chaperoning G protein-coupled receptors: From cell biology to therapeutics. Endocr. Rev. 35, 602–647. doi:10.1210/er.2013-1121
Trujillo, J. M., Nuffer, W., and Smith, B. A. (2021). GLP-1 receptor agonists: An updated review of head-to-head clinical studies. Ther. Adv. Endocrinol. Metab. 12, 2042018821997320. doi:10.1177/2042018821997320
Tsai, C. Y., Lu, H. C., Chou, Y. H., Liu, P. Y., Chen, H. Y., Huang, M. C., et al. (2022). Gut microbial signatures for glycemic responses of GLP-1 receptor agonists in type 2 diabetic patients: A pilot study. Front. Endocrinol. (Lausanne) 12, 814770. doi:10.3389/fendo.2021.814770
Utzschneider, K. M., Kratz, M., Damman, C. J., and Hullarg, M. (2020). Mechanisms linking the gut microbiome and glucose metabolism. J. Clin. Endocrinol. Metabolism 101, 1445–1454. doi:10.1210/jc.2015-4251
Valdecantos, M. P., Pardo, V., Ruiz, L., Castro-S Anchez, L., Lanz, B., Fern Andez-Mill, E., et al. (2016). A novel glucagon-like peptide 1/glucagon receptor dual agonist improves steatohepatitis and liver regeneration in mice.
van Galen, K. A., ter Horst, K. W., and Serlie, M. J. (2021). Serotonin, food intake, and obesity. Obes. Rev. 22, e13210. doi:10.1111/obr.13210
van Gastel, J., Hendrickx, J. O., Leysen, H., Santos-Otte, P., Luttrell, L. M., Martin, B., et al. (2018). β-Arrestin based receptor signaling paradigms: Potential therapeutic targets for complex age-related disorders. Front. Pharmacol. 9, 1369. doi:10.3389/fphar.2018.01369
Wang, S., Yuan, M., Zhang, L., Zhu, K., Sheng, C., Zhou, F., et al. (2022). Sodium butyrate potentiates insulin secretion from rat islets at the expense of compromised expression of β cell identity genes. Cell Death Dis. 13, 67. doi:10.1038/s41419-022-04517-1
Weghuber, D., Barrett, T., Barrientos-Pérez, M., Gies, I., Hesse, D., Jeppesen, O. K., et al. (2022). Once-weekly Semaglutide in adolescents with obesity. N. Engl. J. Med. 387, 2245–2257. doi:10.1056/nejmoa2208601
Whitaker, G. M., Lynn, F. C., McIntosh, C. H. S., and Accili, E. A. (2012). Regulation of GIP and GLP1 receptor cell surface expression by N-glycosylation and receptor heteromerization. PLoS One 7, e32675. doi:10.1371/journal.pone.0032675
Wilding, J. P. H., Batterham, R. L., Calanna, S., Davies, M., van Gaal, L. F., Lingvay, I., et al. (2021). Once-weekly Semaglutide in adults with overweight or obesity. N. Engl. J. Med. 384, 989–1002. doi:10.1056/NEJMoa2032183
Willard, F. S., Douros, J. D., Gabe, M. B. N., Showalter, A. D., Wainscott, D. B., Suter, T. M., et al. (2020). Tirzepatide is an imbalanced and biased dual GIP and GLP-1 receptor agonist. JCI Insight 5, e140532. doi:10.1172/jci.insight.140532
Wootten, D., Reynolds, C. A., Smith, K. J., Mobarec, J. C., Koole, C., Savage, E. E., et al. (2016). The extracellular surface of the GLP-1 receptor is a molecular trigger for biased agonism. Cell 165, 1632–1643. doi:10.1016/j.cell.2016.05.023
Wysham, C., Blevins, T., Arakaki, R., Colon, G., Garcia, P., Atisso, C., et al. (2014). Efficacy and safety of dulaglutide added onto pioglitazone and metformin versus exenatide in type 2 diabetes in a randomized controlled trial (AWARD-1). Diabetes Care 37, 2159–2167. doi:10.2337/dc13-2760
Yang, B., Gelfanov, V. M., El, K., Chen, A., Rohlfs, R., DuBois, B., et al. (2022). Discovery of a potent GIPR peptide antagonist that is effective in rodent and human systems. Mol. Metab. 66, 101638. doi:10.1016/j.molmet.2022.101638
Yoshida, N., Emoto, T., Yamashita, T., Watanabe, H., Hayashi, T., Tabata, T., et al. (2018). Bacteroides vulgatus and Bacteroides dorei reduce gut microbial lipopolysaccharide production and inhibit atherosclerosis. Circulation 138, 2486–2498. doi:10.1161/CIRCULATIONAHA.118.033714
Keywords: incretin—based therapy, GLP-1 (glucagon-like peptide-1), GIP (glucose-dependent insulinotropic polypeptide), T2D (type 2 diabetes), obesity, incretin receptors
Citation: Austin GO and Tomas A (2023) Variation in responses to incretin therapy: Modifiable and non-modifiable factors. Front. Mol. Biosci. 10:1170181. doi: 10.3389/fmolb.2023.1170181
Received: 20 February 2023; Accepted: 28 March 2023;
Published: 07 April 2023.
Edited by:
Catalina Dirney Alba Soto, University of Buenos Aires, ArgentinaReviewed by:
Jacek Mokrosinski, Novo Nordisk Research Center Indianapolis, United StatesBrian Hudson, University of Glasgow, United Kingdom
Copyright © 2023 Austin and Tomas. This is an open-access article distributed under the terms of the Creative Commons Attribution License (CC BY). The use, distribution or reproduction in other forums is permitted, provided the original author(s) and the copyright owner(s) are credited and that the original publication in this journal is cited, in accordance with accepted academic practice. No use, distribution or reproduction is permitted which does not comply with these terms.
*Correspondence: Alejandra Tomas, YS50b21hcy1jYXRhbGFAaW1wZXJpYWwuYWMudWs=