- 1Pathology, Icahn School of Medicine at Mount Sinai, New York, NY, United States
- 2Oncological Sciences, Icahn School of Medicine at Mount Sinai, New York, NY, United States
- 3Genetics and Genomics, Icahn School of Medicine at Mount Sinai, New York, NY, United States
- 4Icahn Genomics Institute, Icahn School of Medicine at Mount Sinai, New York, NY, United States
Emerging evidence suggests that brain derived extracellular vesicles (EVs) and particles (EPs) can cross blood-brain barrier and mediate communication among neurons, astrocytes, microglial, and other cells of the central nervous system (CNS). Yet, a complete understanding of the molecular landscape and function of circulating EVs & EPs (EVPs) remain a major gap in knowledge. This is mainly due to the lack of technologies to isolate and separate all EVPs of heterogeneous dimensions and low buoyant density. In this review, we aim to provide a comprehensive understanding of the neurosecretome, including the extracellular vesicles that carry the molecular signature of the brain in both its microenvironment and the systemic circulation. We discuss the biogenesis of EVPs, their function, cell-to-cell communication, past and emerging isolation technologies, therapeutics, and liquid-biopsy applications. It is important to highlight that the landscape of EVPs is in a constant state of evolution; hence, we not only discuss the past literature and current landscape of the EVPs, but we also speculate as to how novel EVPs may contribute to the etiology of addiction, depression, psychiatric, neurodegenerative diseases, and aid in the real time monitoring of the “living brain”. Overall, the neurosecretome is a concept we introduce here to embody the compendium of circulating particles of the brain for their function and disease pathogenesis. Finally, for the purpose of inclusion of all extracellular particles, we have used the term EVPs as defined by the International Society of Extracellular Vesicles (ISEV).
1 Introduction
From eukaryotes to prokaryotes, all cells secrete extracellular vesicles and particles (EVPs) as part of their regular homeostasis, intercellular communication, and cargo disposal (Trams et al., 1981; Pan et al., 1985; Nieuwland and Sturk, 2010). These EVPs may include but are not limited to apoptotic bodies (Kerr et al., 1972), ectosomes/microvesicles (Dalton, 1975; Thery et al., 2018), exosomes (Trams et al., 1981; Pegtel and Gould, 2019), mitochondria-derived vesicles (D'Acunzo et al., 2021; Miller et al., 2022; Popov, 2022; Nakamya et al., 2022), exomeres (Zhang et al., 2018), supermeres (Zhang et al., 2021a), high and low density-lipoproteins (HDL/LDL) (Claude, 1970), ribonucleic proteins (Barrieux et al., 1976), enveloped and non-enveloped viruses (Dalton, 1975; Alem et al., 2021; Dogra et al., 2021), and other cell free proteins/DNA/RNA (Figure 1) (Lo, 2009; Mathivanan et al., 2010; Toden et al., 2020). Recent literature shows that EVPs carry distinct proteo-transcriptomic signatures from their cell of origin. (Chen et al., 2022a). Subsequently, EVPs shuttle around the body as part of a coordinated system of communication between the cells (Simpson and Sonne, 1982; Simpson et al., 2009; Kowal et al., 2014; Thery et al., 2018; Murillo et al., 2019). EVPs are enriched with tissue-specific biomarkers derived from blood, (Dogra et al., 2020; Chen et al., 2022a), urine (Nilsson et al., 2009; Smith et al., 2018), cerebrospinal fluid (Saman et al., 2012; Norman et al., 2021; Sandau et al., 2022), cell culture media (Valadi et al., 2007; Wei et al., 2017; Chen et al., 2022a), and a variety of other fluids (Trams et al., 1981; Valadi et al., 2007; Skog et al., 2008; Nilsson et al., 2009; Simpson et al., 2009; Palanisamy et al., 2010; Bellingham et al., 2012; Raposo and Stoorvogel, 2013; Kowal et al., 2014; DeRita et al., 2017; Shurtleff et al., 2017). These discoveries have brought immense excitement to novel mechanisms of EVP-derived cellular communication, therapy, and liquid-biopsy applications (Fruhbeis et al., 2012; Song et al., 2020; Gaglani et al., 2021).
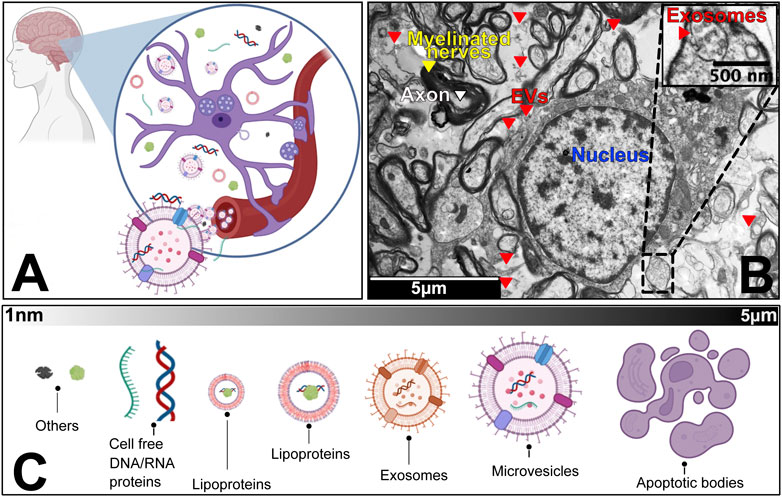
FIGURE 1. The human neurosecretome. (A) Schematic zoom into the universe of extracellular vesicles and particles in the brain microenvironment. We highlight that neurons, microglia and other accompanying cells co-exist with populations of vesicles and particles that are released and up taken by circulatory system. (B) Transmission Electron Microscopy of a transversal cut of brain tissue, showing axons (white), myelinated nerves (yellow) and cell nucleus (blue), surrounded by extracellular vesicles (red). Embedded scale shows 5 micro meter distance. (C) Overview of the most common subpopulations of EVPs, from smallest (∼1 nano meter) to largest components (5 micro meter).
Groundbreaking evidence shows that brain-EVPs allow information exchange between the cells of the CNS (Figure 2) (Takeda et al., 2015; Dickens et al., 2017; Song et al., 2020). First described in 2006 (Faure et al., 2006), neurons and astrocytes release EVPs that have a regulatory function at the synapse, which allows intercellular molecular exchange within the brain (Faure et al., 2006; Skog et al., 2008; Song et al., 2020). Recently, plasma, serum and CSF neuronal-enriched EVPs of patients with Alzheimer’s, Parkinson’s, addiction, and glioblastoma were reported to exhibit modulated levels of phosphorylated (p) tau (Kapogiannis et al., 2019a; Palmqvist et al., 2020; Palmqvist et al., 2023), APoE4 (Palmqvist et al., 2023) and Aβ42 (Li et al., 2022; Palmqvist et al., 2023), α-synuclein (Niu et al., 2020), and multiple miRNAs/mRNAs (Lopez et al., 2017; Song et al., 2020) (A detailed list of brain-derived biomarkers is provided on Table 1). Consequently, brain derived EVPs have emerged as key mediators of communication and disposal mechanisms among the CNS. However, there remains a major gap in knowledge with incomplete understanding of the molecular landscape of circulating EVPs that reside in the tissue microenvironment and systemic circulation. To date, most of these secretory particles remain uncharacterized mainly due to lack of technologies to isolate and separate all EVPs, given their variable nanoscale dimensions and low buoyant density (Smith et al., 2018; Murillo et al., 2019; Norman et al., 2021). Thus, the function of several EVPs in disease pathogenesis remains unknown and elusive.
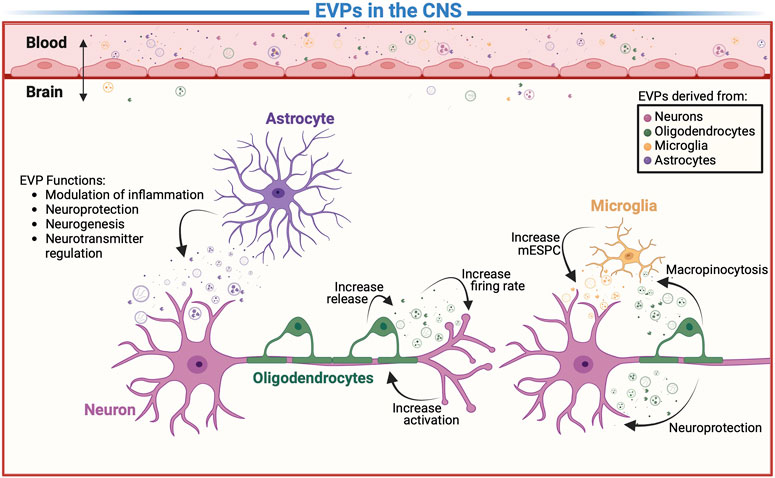
FIGURE 2. Extracellular vesicles and particles (EVPs) in communication. A detail representation of EVP-mediated mechanism including intracellular communication between the cells of CNS and modulation of molecule transport across the BBB. However, it is unclear which EVPs contribute to etiology of addiction, depression, psychiatric, neurodegenerative diseases, and aid in the real time monitoring of the “living brain”.
In this review, we focus on the biogenesis, function, and pathogenesis of the complete EVPs of the neurosecretome. We discuss the extracellular nanoparticles that carry molecular signatures of the brain in its microenvironment and systemic circulation. We elaborate on the current status of technologies used to isolate and separate EVPs of variable sizes and buoyant densities and discuss that every secretory particle may have a distinct cargo and function. It is important to note that the technologies to isolate EVPs are frequently advancing and as a consequence, the complete landscape of EVPs is constantly evolving. Nonetheless, we delve into exploratory and validation studies that have investigated several potential biomarkers of brain diseases circulating within various EVPs. Finally, we employ past literatures and the current landscape of the EVPs to speculate their role in the etiology of addiction (Nakamura et al., 2019; Doncheck et al., 2020; Odegaard et al., 2020; Chand et al., 2021; Odegaard et al., 2022), depression (Saeedi et al., 2021; van der Zee et al., 2022), psychiatric (Du et al., 2022; Nakamya et al., 2022), neurodegenerative diseases (Thompson et al., 2016; Lazo et al., 2021; Noren Hooten et al., 2022; Sandau et al., 2022), and application in real time monitoring of the “living brain”. (Odegaard et al., 2020; Nakamya et al., 2022; van der Zee et al., 2022).
2 Extracellular vesicles, particles, and their subtypes
As part of the neurosecretome, we depict an overview of extracellular vesicles, particles, and their diverse subtypes (Figure 1). As a matter of clarity, we begin with the largest vesicles and end with the smallest known particles.
2.1 Large vesicles (microvesicles, apoptotic bodies, ectosomes, oncosomes, and beyond)
2.1.1 Microvesicles
Cell activation and cytokine stimulation triggers the formation of microvesicles (MVs) from the plasma membrane that are packaged with cellular components and released into the extracellular environment (Basso and Bonetto, 2016; Greening et al., 2017). The membrane budding formation is initiated by the translocation of phosphatidylserine to the outer membrane (Combes et al., 2010; Basso and Bonetto, 2016). Actin-myosin interactions complete the budding process by contracting cytoskeletal structures (Muralidharan-Chari et al., 2009; Basso and Bonetto, 2016). Consequently, the membrane proteins found on MVs would resemble the receptors and proteins found on specific regions of the plasma membrane of the cell of origin. (Doyle and Wang, 2019). This makes identification of the universal MV markers for all cells types a strenuous challenge (Lotvall et al., 2014; Thery et al., 2018). In addition, the use of markers is extremely essential to identifying MVs since their size range (50–2000 nm) overlaps with that of apoptotic bodies and exosomes (Basso and Bonetto, 2016; Ciardiello et al., 2020). The most frequently employed microvesicle markers are mainly integral membrane proteins and cytoskeletal proteins, including KIF23, RACGAP, CSE1L, ARF6, and EMMPRIN (Muralidharan-Chari et al., 2009; Antonyak et al., 2012; Li et al., 2012; Ghossoub et al., 2014; Greening et al., 2017). Several studies suggest that MVs carry a wide variety of cargo including cell surface receptors, cytosolic signaling proteins, metabolic enzymes, and nuclear proteins indicating their role in intercellular communication (Antonyak et al., 2011; Kreger et al., 2016). Specifically, they contain mRNA, lncRNAs and miRNAs, dsDNAs, cytoplasmic proteins suggesting their potential role in exchanging genetic material between cells (Balaj et al., 2011; Greening et al., 2017). Neural stem cells derived MVs have been shown to influence synaptic activity, nerve protection and regeneration, and neuronal development (Marzesco et al., 2005; Lai and Breakefield, 2012). Studies have also demonstrated the pleiotropic effects of MVs by delivering pluripotent transcription factors (Ratajczak et al., 2006a; Ratajczak et al., 2006b). However, shedding of MVs derived from endothelial cells (Minagar et al., 2001; Schindler et al., 2014), neurons (Bianco et al., 2005; Horstman et al., 2007; Colombo et al., 2012), glial cells (Saijo and Glass, 2011; Colombo et al., 2012; Verderio et al., 2012; Agosta et al., 2014), and platelets (Lee et al., 1993; Geiser et al., 1998) have been reported with regards to incidences of stress, oxygen radicals, inflammation, ischemia, and other stimuli. (Porro et al., 2015). Therefore, spike in MVs would likely be associated with stroke, vascular dementia, inflammatory, and other neurodegenerative diseases (Doeuvre et al., 2009; Porro et al., 2015). For instance, increase in MV production is observed in Alzheimer patients with mild cognitive impairment. These Alzheimer associated MVs are also characterized for their increase in toxicity (Verderio et al., 2012; Garzetti et al., 2014; Joshi et al., 2014). Likewise, the role of oligodendroglioma derived MVs as signal transductor has been shown to trigger neuronal apoptosis and suppressing neuronal sprouting (Porro et al., 2015; D'Agostino et al., 2006). Given that MVs derive from the plasma membrane, they could effectively reflect the intercellular activities in the microenvironment, exhibiting characteristics as prominent markers of the pathophysiology of the CNS (Porro et al., 2015).
2.1.2 Apoptotic bodies
Apoptotic cells also release extracellular particles called apoptotic bodies (Kakarla et al., 2020). These particles are generally described as vesicles that carry nuclear fragments and cellular organelles due to apoptosis (Kakarla et al., 2020). Being one of the largest extracellular particle, their size ranges from 800 to 5000 nm in diameter (Serrano-Heras et al., 2020). Originally these particles were considered cell debris and were often overlooked in studies of circulating vesicles (Kakarla et al., 2020). However, apoptotic bodies purified from neurological patients did not differ in morphology or size distribution from those purified from healthy volunteers (Serrano-Heras et al., 2020). Additionally, they identified neurological apoptotic body biomarkers that were consistent regardless of the health condition of their cell of origin (Serrano-Heras et al., 2020). These studies are not conclusive that these vesicles have a distinct role in pathological conditions; hence, further studies of the functionality of these particles is needed.
2.1.3 Ectosomes, oncosomes, and beyond
Although classification of large vesicles is evolving, ectosomes and large oncosomes have been widely studied (Keerthikumar et al., 2015). Ectosomes are released from the plasma membrane and are implicated to have role in cancer (Keerthikumar et al., 2015; Thery et al., 2018). Large oncosomes are produced from several cancer cells when stimulated involving EGFR and overexpression of membrane-targeted Ak1t (Di Vizio et al., 2012). These vesicles differ in morphology and biogenesis from oncosomes which are any EV subtype that carries oncogenic cargo (Di Vizio et al., 2012). Large Oncosomes are 1,000–10,000 nm in diameter and are derived from ameboid tumor cells (Di Vizio et al., 2012). These cancer cells use amoeboid movement in order to invade other cells through narrow 3 μm-wide microchannels at a fast velocity that cannot be stopped by integrin inhibition (Wu et al., 2021). Additionally, amoeboid cancer cells have been suggested to have drug-resistant properties (Graziani et al., 2022). Considering their highly invasive properties, better ways of targeting them in clinics are needed. Further research should investigate how large oncosomes found in biofluids can be used as a liquid biopsy alternative to detect the presence of ameboid tumors.
2.2 Exosomes
Exosomes are nano sized EVPs (∼30–200 nm) of endocytic origin that have demonstrated clinical potential as therapeutic agents given their role in pathogenesis and the biologically active molecules they encapsulate. (Kalluri and LeBleu, 2020; Fan et al., 2022). Exosomes are intraluminal vesicles derived from inward budding of endosomal multivesicular bodies that are released into the extracellular space via exocytosis. (Alvarez-Erviti et al., 2011; Kahlert and Kalluri, 2013; Basso and Bonetto, 2016; van Niel et al., 2018; Jeppesen et al., 2019). The process of sorting exosomal cargo mainly revolves around the endosomal sorting complex (ESCRT), comprised of four complexes (ESCRT-0, I, II, and III) derived from up to thirty protein accessories (Greening et al., 2017; van Niel et al., 2018; Mathieu et al., 2019). Likewise, another major component are the Rab GTPase proteins, largely involved in modulations of intercellular vesicle transportation and budding and release of vesicles (Colombo et al., 2014; Greening et al., 2017; Mathieu et al., 2019; Kalluri and LeBleu, 2020). As a result, common surface markers unique to exosomes include endosome related components like flotillin, CD63, TSG101, and Alix (Meckes et al., 2013; Clark et al., 2015; Costa-Silva et al., 2015; Kowal et al., 2016; Song et al., 2020). Like MVs, exosomes have been found to contain proteins and nucleic acids (Zhang et al., 2019a). However, it has been found that they differ in their lipid, protein, RNA, and DNA composition, emphasizing their potential unique roles (Wei et al., 2017; Pegtel and Gould, 2019).
In the context of brain, exosomes facilitates intercellular communication, synaptic plasticity, neurogenesis, and neuronal stress response by transferring cell type specific coding and non-coding RNAs, miRNAs, proteins, and lipids (Lachenal et al., 2011; Bahrini et al., 2015; Li et al., 2018a; Saeedi et al., 2019). Consequently, there have been reports of pathogenic amyloids and protein deposits found within and outside of brain cells associated with neurodegenerative diseases (Sweeney et al., 2017; Saeedi et al., 2019). Thus, access to biofluids containing exosomes with pathogenic protein aggregates may be capable of profiling the heterogeneity of neurological diseases and disorders via simple and non-invasive liquid biopsy (Lai and Breakefield, 2012; Hornung et al., 2020; Younas et al., 2022).
Researchers have investigated the associations between exosomes and progression of neurodegenerative diseases, including both synucleinopathies (Kunadt et al., 2015; Stuendl et al., 2016) and tauopathies (Saman et al., 2012; Guix et al., 2018), and prion diseases (Bellingham et al., 2012; Chiasserini et al., 2014). Exosomes derived from neurons and glia constitute a sophisticated and interconnected network that influences physiological functions of the CNS (Holm et al., 2018; Fan et al., 2022). This makes them an indispensable component in a series of protective mechanisms of the CNS including angiogenesis (van Balkom et al., 2013; Liang et al., 2016), inhibition of the neural apoptosis (Song et al., 2019), neuroimmune regulation (Kolios and Moodley, 2013; Doeppner et al., 2015), formation of myelin sheath (Boecker et al., 2018; Madison and Robinson, 2019; Yin et al., 2021), growth of axon (Li et al., 2018b; Delpech et al., 2019; Guo et al., 2019; Fan et al., 2022). Nevertheless, in tumor microenvironment or during progression of neurodegenerative diseases, exosomes are linked to distribution of amyloid-β peptides and α-synuclein and tumor metastasis (El Andaloussi et al., 2013; Kalluri and LeBleu, 2020; Fan et al., 2022).
2.3 Viruses
The viruses must also be considered as a subset of the vast EVP canopy (Ghosh et al., 2020; Dogra et al., 2021). Many viruses follow the late lysosome or multivesicular body (MVB) followed by exocytosis mechanism for egress (Alem et al., 2021; Dogra et al., 2021; Elmore et al., 2021). As shown by Dogra et al. (2021) the biogenesis of SARS-coV-2 have an egress pathway identical to the exosomes. While, other viruses, such as HIV, pinch off the plasma membrane (Brügger et al., 2006). As a result, all viruses fall under the classification of either microvesicles/ectosomes (pinching off the plasma membrane), exosomes (exocytosis), or other pathways EVP release.
2.4 Mitochondrial derived-extracellular vesicles
The mitochondria may function as a communication channel between neurons and provide neuroprotective and neurorecovery functions (Hayakawa et al., 2016). However, it is unknown whether communication is achieved through the secretion of whole mitochondria, mitochondria-encapsulated microvesicles, or EVPs packed with mitochondrial cargo. Researchers have identified vesicles carrying cargo of mitochondrial proteins, lipids, and mtDNA (Miller et al., 2022; Popov, 2022). These vesicles have been potentially misinterpreted as exosomes. Recent studies have identified that these vesicles are not exosomes but rather, a subtype of EVs called mitochondrial derived-vesicles (MDV) (D'Acunzo et al., 2021; Nakamya et al., 2022). It has been described that neurological diseases, including Alzheimer’s, Parkinson’s, substance use disorder and down syndrome, can affect mitochondrial structure and function (D'Acunzo et al., 2023).
Two types of MDVs have been described–mitochondrial derived-intracellular vesicles (MDIV) and mitochondrial derived-extracellular vesicles (MDEV), also known as mitovesicles (D'Acunzo et al., 2021; Nakamya et al., 2022; Heyn et al., 2023). MDIVs are single-layer vesicles that originated from the outer membrane, whereas the MDEVs are bilayer vesicles due to its origin from both the inner and outer membrane (Sugiura et al., 2014; Heyn et al., 2023). Given their difference in biogenesis, electron microscopy is frequently employed to distinguish between MDIV and MDEV (Sugiura et al., 2014; Heyn et al., 2023). Likewise, differences in lipid content that stems from varying EVP biogenesis processes can also distinguish MDVs from other EVPs (D'Acunzo et al., 2021). For example, MDIVs are TOMM20-positive, PDH-negative and MDEVs are TOMM20-negative, PDH-positive (Heyn et al., 2023). Furthermore, MDEVs and MDIVs do not express exosome or microvesicle markers (e.g., Annexin A1, Annexin A2, Alix, TSG101, CD63), thus, implying they belong to their own subtype. Furthermore, recent research has revealed that MDEVs transport distinct cargo in healthy versus diseased states, as observed in both humans and rodents with Down Syndrome and Cocaine Use Disorder (D'Acunzo et al., 2021; D'Acunzo et al., 2023). Although the field of MDEVs is still emerging, further research is necessary to comprehend the role of these subtypes in the etiology and diagnosis of mitochondrial abnormalities (D'Acunzo et al., 2021; D'Acunzo et al., 2023). Nevertheless, the current research confirms previous findings of the association between physiological disorders and mitochondrial abnormalities (D'Acunzo et al., 2021; Nakamya et al., 2022; D'Acunzo et al., 2023).
2.5 Exomeres
First considered as cellular debris, exomeres (<50 nm), unlike other EVs are non-membranous nanoparticles (Zhang et al., 2018; Zhang et al., 2019b). Since these vesicles are lacking a lipid bilayer and expression of ESCRT, it is suggested that they do not originate from the plasma membrane or endocytic pathway like the other subtypes (Zhang et al., 2019b). Regardless of their small size, they have been detected to contain proteins, lipids, and nucleic acids (Zhang et al., 2018). It is suggested from their protein enrichment that their cargo is closely associated with the endoplasmic reticulum, mitochondria, and cytoskeletal microtubules (Zhang et al., 2019b). In vitro studies have shown that exomeres can contain functional cargo that can alter recipient cells (Zhang et al., 2019b). Yet, the biogenesis and the exact function of exomeres remain unknown. Given the ambiguity in their biogenesis, several questions are being raised regarding the formation of additional particles during their rigorous isolation process.
2.6 Supermeres
A recent study conducted by Zhang et al., reported the discovery of a new distinct nanoparticle named supermeres (Zhang et al., 2019b; Zhang et al., 2021a). While ultracentrifugation of the supernatant of exomeres at 376,000 × g for 16 h the authors discovered a small pellet (Zhang et al., 2019b). After analyzing this pellet authors found that these nanoparticles were distinct from exosomes in size, morphology, composition, and cellular interactions. As a result, the authors termed the subcategory of nanoparticles supermeres–supernatant of exomeres. These nanoparticles are suggested to have a functional application since they are potentially enriched in clinically relevant proteins that were previously reported in exosomes (e.g., amyloid precursor protein (APP), cellular-mesenchymal-epithelial transition factor (MET), glypican 1 (GPC1), argonaute-2 (AGO2), TGFβ-induced (TGFBI), numerous glycolytic enzymes) and extracellular RNA (exRNA; miR-1246)) (Zhang et al., 2021a). Further studies verifying the existence of these particles are required since there is no clear understanding of how supermeres are formed and what is their function. Ultracentrifugation could lead to the formation of additional particles, and it is not clear whether supermeres are formed during ultracentrifugation or not.
2.7 High and low density lipoproteins
Cholesterol containing particles have been comprehensibly studied for their size, biogenesis, and disease pathology, mainly related to cardiovascular (Ishibashi et al., 1993) and Alzheimer’s diseases (Tcw et al., 2022).These particles are classified as very low density lipoproteins (VLDL) (35–200 nm), low-density lipoproteins (LDL) (20–26 nm), and high-density lipoproteins (HDL) (5–8 nm) and other subtypes of them (Claude, 1970; Liangsupree et al., 2021). In a recent study Murillo et al. investigated the exRNA of variable particles and it was shown that HDL and LDL carry distinct RNA, while argonaute proteins and vesicles of different density cargo unique RNA signatures (Murillo et al., 2019). Furthermore, It is important to note that LDL is up taken and released by cells through receptor-mediated endocytosis, a similar process to exosomes secretion (Tcw et al., 2022).
3 Advances in isolation technologies for EVPs
Common sources of EVPs include tissue, cell supernatant, and a wide variety of biofluids including blood, saliva, urine, breast milk, etc. (Valadi et al., 2007; Keller et al., 2011; Lässer et al., 2011; Crescitelli et al., 2021). In contrast to tissue and cell supernatant samples, biofluids are more accessible and less invasive. However, samples of bodily fluid generally have larger starting volumes, resulting in challenges associated with dilution or low EVP yield and purity (Ramirez et al., 2018). This issue is further exacerbated when attempting to isolate cell-type specific EVPs or specific EVP subpopulations from biofluids (Su et al., 2017; Antes et al., 2018; van Niel et al., 2018). Therefore, the key feature of a clinically applicable and reliable EVP isolation method would need to address: 1) sensitivity to individual subpopulations of EVPs; 2) purity and throughput of isolated EVPs; 3) reproducibility, standardization, and scalability; 4) external validity when considering various clinical settings and samples used (Younas et al., 2022). Currently, the established isolation methods include differential ultracentrifugation (UC), density gradient ultracentrifugation (UC-DG), size exclusion chromatography (SEC), immunoprecipitation via beads, field-flow fractionation (FFF), tangential flow filtration (TFF), nanofluidic deterministic lateral displacement (nanoDLD), and acoustic trapping technology (Wunsch et al., 2016; Kim et al., 2017; Smith et al., 2018; Liangsupree et al., 2021).
3.1 Differential centrifugation (UC), density gradient ultracentrifugation (UC-DG)
UC and UC-DG remain the most frequently implemented method due to its high EVP yields (Royo et al., 2020; Liangsupree et al., 2021). UC utilizes a series of gradually increasing centrifugation to sequentially pellet, remove debris and isolate EVPs in a stepwise manner, allowing for efficient isolation even given a large starting volume (Carnino et al., 2019; Brennan et al., 2020). This method also does not require any additional chemical reagents ensuring the functionality of the EVPs isolated (Liangsupree et al., 2021). Nevertheless, the resulting pellet from UC are expected to cause EVP aggregations and would include a mixture of all EVPs, suggesting lower purity for selected subtypes (Szatanek et al., 2015; Carnino et al., 2019). Individual biofluids’ inherent biochemical compositions also interfere with this isolation process (Ramirez et al., 2018); the polymeric-Tam Horsfall protein, frequently found in urine, tends to bind to EVPs which causes the complex to pellet at lower centrifugation speed, decreasing the overall EVP yield and purity (Wachalska et al., 2016); EVP pellet isolated from breast milk via UC appears to solidify due to higher concentration of whey and casein protein, thus posing great difficulty for EVP resuspension (Ramirez et al., 2018).
In contrast, UC-DG implements sucrose, iohexol, or iodixanol-based density gradient on top of the UC protocol. (Cvjetkovic et al., 2014). This allows for further segregation of the UC isolated EVP pellet based on the individual buoyant density of the subpopulations found within the pellet (Konoshenko et al., 2018; Carnino et al., 2019). Nonetheless, in exchange for the improved segregation, the set up for UC-DG are exceedingly sophisticated and time consuming, requiring up to 2 days for completion (Théry et al., 2009; Konoshenko et al., 2018). Additionally, studies have reported EVP throughput of both UC and UC-DG is significantly dependent on the centrifuge and rotors used (Théry et al., 2006; Momen-Heravi et al., 2013; Witwer et al., 2013; Cvjetkovic et al., 2014; Gardiner et al., 2016; Konoshenko et al., 2018).
3.2 Size exclusion chromatography (SEC)
To further simplify the EVP isolation process, SEC-based methods are developed. SEC employs a column filled with porous resin of pre-determined diameter to effectively drain and isolate all EVP and its subpopulations based on their hydrodynamic radius found within the loaded biofluid (Barth et al., 1994). Isolated EVP subtypes are then eluted as separated fractions in the order of decreasing diameters. This mechanism prevents EVP aggregates and preserves their functionality (Gámez-Valero et al., 2016). Most importantly, SEC minimizes the amount of time and effort needed considerably for EVP isolation (Gámez-Valero et al., 2016). This can make or break the clinical applicability of EVP based liquid biopsy. Despite the rigorous segregation by size, SEC offers limited separation of EVP given the overlapping diameters of various subgroups (Carnino et al., 2019; Brennan et al., 2020). In addition, the eluting solutions used for SEC would further dilute the concentration of each EVP fractions (Brennan et al., 2020; Liangsupree et al., 2021).
3.3 Immunoprecipitation (IP)
Immunoprecipitation is a fast and simple affinity-based method that targets the surface protein markers of the EVPs via magnetic beads to isolate the selected EVP population. This method is frequently used in conjunction with other isolation methods as a purification step (Carnino et al., 2019). The specificity of the method could be adjusted by attaching specific antibodies to the magnetic beads in correspondence to EVP subtypes or different cell types, thus providing high purity sample output (Tauro et al., 2012). Consequently, the high selectivity of this method is highly contingent on presence of sufficient number of beads and proper optimization of the ligands to allow for maximal binding (Carnino et al., 2019). Thus, this suggests immunoprecipitation would be less efficient in biofluids with complex compositions of varying enzymes and molecules, for instance, plasma, due to binding competition (Hage et al., 1993; Carnino et al., 2019). There is also the need to account for the high cost of beads with specific ligands and difficulties with detachment of antibodies from EVPs for preservation of integrity (Heath et al., 2018).
3.4 Field-flow fractionation (FFF) and as.ymmetrical flow field-flow fractionation (AF4)
FFF is a term used to describe a plethora of flow-based separation technique that applies an external perpendicular force on the flow direction of the sample causing accumulation of particles along the bottom wall of the narrow channel (Schallinger et al., 1985). Correspondingly, the counteracting Brownian motion of the particles within the fluid would diffuse, separating the particles into layers based on their diffusion coefficient and allowing for elution at different time point (Zhang and Lyden, 2019). The smaller particles with higher diffusion coefficient would be near the upper layer thus eluting faster than larger particles near the bottom (Sitar et al., 2015). The most frequently used FFF for the purpose of EVP isolation is the asymmetrical flow field-flow fractionation (AF4) that utilizes cross-flow as the external force and replaced the bottom wall with a pre-determined pore size permeable (Zhang and Lyden, 2019). The biggest advantage of AF4 relative to other size-based isolation techniques is its flexibility; the cross-flow could be adjusted between runs to accommodate for fluid samples of varying degrees of particle heterogeneity (Sitar et al., 2015). Likewise, the solvent used in AF4 could also be replaced with PBS or the original EV formulation buffer to preserve EV integrity for functionality experiments. However, similar to SEC, AF4 has the tendency to dilute the samples post isolation. Additionally, AF4 is not catered towards large volume sample isolation as this could likely result in self-association and overloading effects (Liangsupree et al., 2021).
3.5 Tangential flow filtration (TFF)
TFF, otherwise known as cross-flow filtration, is an improved size-based filtration technique that implements solvent flowing in the direction tangent to the semi-permeable membrane, effectively preventing the buildup of larger particles and filter cake formation (Busatto et al., 2018; McNamara et al., 2018). The particles smaller than the pores would then travel across the membrane due to the transmembrane pressure (Kim et al., 2021). A combination of multiple membranes of different pore sizes could achieve proper EVP isolation and effectively segregate the subpopulations. Additionally, utilization of TFF is often coupled with other methods to further concentrate the resulting isolated EVPs, providing stronger signals for downstream analysis. This suggests TFF is highly efficient in isolation of EVPs in large volume of diluted samples (Liangsupree et al., 2021). The isolation process also effectively preserves the integrity and biological activity of the EVPs (Jia et al., 2022). Nevertheless, similar to other size-based isolation techniques, TFF has limited separation of EVP subpopulations given overlapping diameters of the subtypes.
3.6 Nanofluidic deterministic lateral displacement (nanoDLD)
The nanofluidic device nanoDLD (Smith et al., 2018), is a size-based isolation method that use asymmetric pillar arrays to deflect particles in specific trajectories in accordance with their size (Kim et al., 2017). Smaller particles would exhibit less disruption and would flow through the pillars in a “zigzag” pattern (Kim et al., 2017). Meanwhile, larger particles would likely be disrupted by the pillars, thus, would typically travel in a “bumping” pattern (Wunsch et al., 2016; Kim et al., 2017; Smith et al., 2018). The displacement in the larger particles allows for effective segregation between EVPs of varying hydrodynamic diameter (Wunsch et al., 2016; Kim et al., 2017; Smith et al., 2018; Liangsupree et al., 2021). This mechanism makes high purity particle isolation via nanoDLD extremely efficient and reproducible. However, it requires the use of a silicon chip which significantly limits the amount of sample it could process in a single run (Wunsch et al., 2016). Correspondingly, similar to filtration-based methods, nanoDLD is prone to clogging, therefore, prefiltration of larger particles is required (Liangsupree et al., 2021).
3.7 Acoustic trapping
Acoustic trapping technology, on the other hand, heavily relies on ultrasonic wave scattering to effectively cluster and separate EVPs based on their size, density, and compressibility (Rezeli et al., 2016; Bryl-Górecka et al., 2018; Ku et al., 2018; Ku et al., 2019). This method requires addition and retainment of seeding particles, most commonly polystyrene beads, using the acoustic standing wave prior to loading the samples. Once the sample is loaded, the particles within would aspirate and cluster with the seeding particles using the secondary acoustic wave through particle-particle interactions. To retrieve the isolated EVP subtypes, the clusters are washed and released from the beads once the acoustic wave is turned off (Ku et al., 2018). Acoustic trapping has demonstrated high efficacy and strong enrichment performances for sample volume as low as 12.5 ul (Bryl-Górecka et al., 2018; Liangsupree et al., 2021). Nevertheless, the set up and maintenance requires large amount of funding and the device itself are only functional when high power inputs are available (Hammarström et al., 2021).
4 Extracellular vesicles and particles in the healthy central nervous system
While many publications have exclusively used the terms “exosomes” or “ectosomes” etc., the isolation methods used in the respective studies do not exclusively separate such particles, but a heterogenous population. For the purpose of inclusion of all particles, in this review, we have used the term EVPs as defined by the International Society of Extracellular Vesicles (ISEV) (Thery et al., 2018).
4.1 Oligodendrocytes and neurons
Oligodendrocytes are specialized glial cells that wrap around the axons of neurons forming myelin sheaths in the CNS (Pegtel et al., 2014). The myelination of axons is crucial for proper conduction of impulses (Xiao et al., 2021). During the myelination process axons and oligodendrocytes intercommunicate in order to maintain axon integrity and survival (Pegtel et al., 2014). The relationship is evident in demyelinating diseases where myelin damage is strongly associated with neuronal and axonal degeneration (Xiao et al., 2021). Research suggests that oligodendrocyte-derived EVPs mediate signaling between oligodendrocytes and neurons (Frühbeis et al., 2013; Pegtel et al., 2014; Delpech et al., 2019; Pistono et al., 2021).
Studies suggest that oligo-EVPs are released as a result of neuron activation starting a cascade of events (Delpech et al., 2019). First, glutamatergic signaling from neurons occurs (Delpech et al., 2019). This results in glutamate inducing a Ca2+ influx in oligodendrocytes, which causes the activation of GTPase Rab35 that in turn leads to the release of oligo-EVPs (Lachenal et al., 2011; Delpech et al., 2019) Neurons exposed to oligo-EVPs display increased firing rates as well as altered gene expression. Therefore, the increased activation of neurons increases the release of oligo-EVPs, which then enhances the activation of the neuron, fueling a cyclical relationship (Krämer-Albers, 2020). Hyperactivation of neurons has been associated with neurodegenerative disorders (Xiao et al., 2021). Yet, oligo-EVPs cause an enhancement of neuronal firing without causing excitotoxicity in healthy brains (Krämer-Albers, 2020). In fact, oligo-EVPs may have a neuroprotective role (Krämer-Albers, 2020; Oyarce et al., 2022). A study found that neurons exposed to oligo-EVPs under conditions of oxidative stress and nutrient deprivation had higher metabolic activity than neurons exposed to HEK293T-derived exosomes or artificial liposomes (Frühbeis et al., 2013). Thus, suggesting that oligo-EVPs uniquely aid neuronal health. Further research should be done to understand how the release of oligo-EVPs affects the signaling of other neurotransmitters and its role in regulation and possible clinical application.
The purpose of oligo-EVP uptake may be different between cell types. In vitro oligo-EVPs were seen to be taken up by neurons and microglia, and infrequently by astrocytes or oligodendrocytes (Figure 2) (Krämer-Albers, 2020). In order to validate these findings an in vivo study was done using transgenic mice and oligo-EVPs carrying Cre-recombinase (Frühbeis et al., 2013). Given the activation of the reporter by Cre-recombinase requires the release of Cre from the endosome and translocation to the nucleus, any expression of Cre would directly correlate to oligo-EVP uptake; increased levels of Cre expression were observed in neurons correspondingly (Frühbeis et al., 2013). However, in the earlier studies microglia were also seen to take up oligo-EVPs by macropinocytosis. (Krämer-Albers, 2020). It is believed that these EVPs are then trafficked to lysosomes for degradation in order to clear oligodendroglial myelin membrane debris through their EVP uptake (Delpech et al., 2019). Therefore, the lack of visualization using the Cre reporter, is most likely due to the degradation of EVP-cargo in the endo-lysosomal system. In neurons, Cre was expressed to demonstrate that oligo-EVPs can be internalized in vivo (Frühbeis et al., 2013). Additionally, the number of recombined neurons did not change due to uptake at axonal and soma-dentric sites, indicating both sites may be used (Frühbeis et al., 2013). As a result, it is clear that the cargo of oligo-EVPs can alter gene expression of neurons (Frühbeis et al., 2013). However, since both uptake sites contain receptors that regulate neuronal signaling, research is needed to investigate whether oligo-EVPs alter the membrane of these sites and can activate these receptors.
4.2 Microglia and neurons
Microglia are the immune cells of the CNS (Williams et al., 1994; Sousa et al., 2017). To ensure homeostasis is maintained they produce soluble factors that mediate inflammatory responses (e.g., chemokines, cytokines, and free radicals) (Kettenmann et al., 2011; Paolicelli et al., 2019). These biomolecules can be exchanged between cells through the use of microglia-derived extracellular vesicles (microglia-EVPs) in order to communicate support for infection and brain damage (Paolicelli et al., 2019). Additionally, microglia-EVPs can signal neuronal activation through surface components (Ceccarelli et al., 2021; Picciolini et al., 2021). While neuroinflammation aims to solve an injury and restore brain homeostasis, the dysregulation of such responses can become unfavorable and even neurotoxic (Brites and Fernandes, 2015). Through the study of microglia-EVPs we can gain a deeper understanding of how healthy conditions are maintained.
Microglia-EVPs have also been suggested to play a role in neurite outgrowth, modulating neuronal activity, and orchestrating innate immunity (Delpech et al., 2019). The surface components of microglia-EVPs, rather than their cargo content, have been found to have a direct effect on neurotransmission, causing an increase in miniature excitatory postsynaptic current (mEPSC) (Delpech et al., 2019; Ceccarelli et al., 2021). Specifically, microglia-EVPs enrich ceramide and sphingosine production in neurons and increase synaptic activity by facilitating SNARE and synaptic vesicle release (Delpech et al., 2019; Aires et al., 2021). Further research is needed to understand how microglia-EVPs supportive role in synaptic vesicle release may be dysregulated in psychiatric chemical imbalances. Microglia-EVPs have also been shown to alter neurotransmission by transporting hydrophobic ligands on their surface (Paolicelli et al., 2019; Ceccarelli et al., 2021). For example, it was found that microglia-EVPs carry endocannabinoid N-arachidonoylethanolamine (AEA) on their surface which can be used to activate type-1 cannabinoid receptors (CB1) (Gabrielli et al., 2015a; Gabrielli et al., 2015b; Paolicelli et al., 2019). The activation of CB1 receptors on GABAergic neurons leads to the inhibition of presynaptic transmission (Manzoni and Bockaert, 2001; Mackie, 2006). A variety of neurological activities, such as mood, memory, and cognition, are mediated by CB1 receptors (Kendall and Yudowski, 2016; Franzen et al., 2022). Thus, altered expressions of the CB1 receptors have been observed in various neurodegenerative diseases, such as Alzheimer’s disease, Parkinson’s disease, and Huntington’s disease (Bisogno and Di Marzo, 2010; Vasincu et al., 2022). These alterations may be associated with microglia-EVPs (Aires et al., 2021). Correspondingly, the communication between microglia-EVPs and neurons are bidirectional; microglia-EVPs are capable of being able to activate neurons and vice versa (Figure 2) (Albertini et al., 2020). When serotonergic signaling occurs, 5-HT is released from neurons, binding to 5-HT receptors on microglia, and releasing EVPs (Glebov et al., 2015; Albertini et al., 2020). Depression which has been associated with low levels of serotonin has also been associated with neuroinflammation (Brites and Fernandes, 2015; Cowen and Browning, 2015). A deficiency of serotonin may lead to modification of microglia-EVPs, which in turn suppresses the neuroimmune system. However, this association needs to be further investigated.
4.3 Astrocytes and neurons
Astrocytes are known to play important roles in various neurological processes, including the support and maintenance of neurons, the regulation of brain blood flow, and the formation and repair of the blood-brain barrier (Sidoryk-Wegrzynowicz et al., 2011; Gharbi et al., 2020). There is increasing evidence that astrocyte-derived EVPs (astro-EVPs) may be involved in a number of neurological processes, including the modulation of inflammation, the promotion of neurogenesis, and the repair of damaged tissue (Figure 2) (Gharbi et al., 2020; Upadhya et al., 2020). In addition, astro-EVPs appear to regulate the concentration of neurotransmitters (Upadhya et al., 2020; You et al., 2020). Astro-EVPs’ cargo could be further investigated to understand better their neuroprotective mechanisms.
Upon changes in the environment, astrocytes increase EVP release and modify EVP cargo in order to maintain homeostasis (Gharbi et al., 2020). Jovicic et al. suggests that miRNAs contained in EVPs secreted by mouse astrocytes differed from those present in the cell of origin (Jovicic and Gitler, 2017). According to these findings, astrocytes select specific miRNAs for EVP transport in correspondence to varying functions (Upadhya et al., 2020). For example, when in an environment with cytokines IL-1β and TNF-α, astro-EVPs will contain miRNAs that support neuronal function (Upadhya et al., 2020). Like miR-125a-5p and miR-16-5p which activate the kinase receptor for neurotrophin 3 thereby promoting neuronal survival and differentiation (Upadhya et al., 2020). In stressful conditions, astro-EVPs have been found to carry cytoprotective heat shock protein 70 (HSP70) (Guha et al., 2019a; Upadhya et al., 2020). Whereas, in conditions of hypoxia and hypoglycemia, astro-EVPs have been found to carry functional prion proteins (Guitart et al., 2016; Upadhya et al., 2020). According to this study, these EVPs are involved in activating specific signaling pathways that enhance neuronal protection (Upadhya et al., 2020).
Neurotransmitter regulation is a complex process that is essential for maintaining normal brain function (Borodinsky et al., 2014). In order to regulate the concentration of glutamate, astrocytes utilize excitatory amino-acid transporter (EAATs) proteins on their surface (Murphy-Royal et al., 2017; Mahmoud et al., 2019; Todd and Hardingham, 2020). These transporter proteins have also been found in astro-EVPs indicating they may maintain neuronal homeostasis by reducing excitotoxicity in the brain, often associated with numerous neuropathological conditions (Upadhya et al., 2020). Further research is needed for therapeutic applications of astro-EVPs containing EAAT.
5 Extracellular particles in the pathological central nervous system
5.1 Neurodegenerative diseases and aging
There is evidence that EVPs may play a role in the development and progression of neurodegenerative diseases, which are conditions characterized by the progressive loss of function and death of neurons in the brain and nervous system (Thompson et al., 2016). These diseases include conditions such as Alzheimer’s disease (AD), and Parkinson’s disease (PD). Aging is a major risk factor for the development of neurodegenerative diseases, and it has been suggested that the aging process itself may be influenced by EVPs (Robbins, 2017; Hou et al., 2019).
Since accelerated aging may lead to neurodegeneration and other physical declines (Hou et al., 2019), biomarkers may offer a means to monitor aging and ensure that preventative care is provided. It is suggested that mitochondrial abnormalities occur with age and neurodegeneration (Lazo et al., 2021). Scientists analyzed EVP mtDNA from individuals aged 30–64 using both cross-sectional and longitudinal methods to determine whether human mtDNA levels vary with age (Lazo et al., 2021). They discovered the inverse relationship between the levels of EVP-associated mtDNA and aging (Chomyn and Attardi, 2003; Lazo et al., 2021). Thus, monitoring the aging process may be possible through the decline in mtDNA levels. Likewise, both AD and PD are associated with mitochondrial abnormalities, making mtDNA in EVPs a valuable insight into these diseases (Bose and Beal, 2016; Perez Ortiz and Swerdlow, 2019; Nakamya et al., 2022). In PD, mitochondrial dysfunction can increase oxidative stress, disrupt cellular material trafficking, impair electron transport chain function, cause calcium imbalances, and disrupt mitophagy (Nakamya et al., 2022). There is interesting evidence suggesting mitophagy is initiated through the PINK1–Parkin pathway, mutations of which are linked to the early onset of recessive Parkinson’s disease (Nakamya et al., 2022). In AD, toxicity associated with Aβ aggregates has been reported to damage mitochondria and cause mitochondrial dysfunction (Reddy and Beal, 2008; Kim et al., 2020). Isolated brain EVPs exposed to Aβ aggregates and H2O2 have been found to contain mitochondrial structures, RNA and proteins (Kim et al., 2020). This suggests that EVPs may deliver toxic mitochondrial components from damaged mitochondria, promoting cellular pathologies and AD (Kim et al., 2020). A comparison of mitochondrial cargo within EVPs derived from neurodegenerative patients with healthy samples is necessary to determine whether these abnormalities can serve as biomarkers. Further, the mitochondrial cargo of EVPs from aging individuals should also be examined for neurodegenerative-associated components.
Changes in the microenvironment have been linked to neurodegeneration (Delpech et al., 2019; Aires et al., 2021). For example, when midbrain cultures are incubated with IFN-γ and LPS, there is an increase in EVP release and activation of microglia inducing dopaminergic degeneration associated with PD (Aires et al., 2021). It has been suggested that miR-34a carried by astro-EVPs may enter dopaminergic neurons and target the anti-apoptotic Bcl-2 protein, which may also aid the progression of PD (Upadhya et al., 2020). Both microglia-EVPs and astro-EVPs have also been implicated in the pathogenesis of AD (Upadhya et al., 2020; Aires et al., 2021). The activation of P2X7R in microglial cells has been linked to the release of EVPs (Ruan et al., 2020). There is both cognitive improvement and EVP release hindrance when P2X7R is inhibited, emphasizing that EVPs are involved in the promotion of AD (Ruan et al., 2020). Astro-EVPs have been identified as carriers of aggregated proteins such as amyloid beta oligomers (AβO) and protofibrils (Aβ) in AD (Upadhya et al., 2020; Gomes et al., 2022). EVPs isolated from the CSF and blood of patients with AD and PD have been found to contain protein aggregates of Aβ, tau, and α-synuclein (Joshi et al., 2014; Guix et al., 2018; Cicognola et al., 2019; Niu et al., 2020; Upadhya et al., 2020; Li et al., 2022). Thus, it has been suggested that the release of neurotoxic proteins in EVPs allows cells to deliver the protein aggregates to other cells with a higher degradation capacity (Upadhya et al., 2020).
The presence of neurodegenerative proteins in EVPs has led researchers to consider their use as liquid biopsy (Abdel-Haq, 2020). A number of small-scale studies have been conducted, but in order for the results to be valid, larger studies are needed (Kapogiannis et al., 2015). In order to meet this need researchers collected 887 plasma samples from 128 individuals who eventually developed AD and 222 matched healthy controls (Kapogiannis et al., 2019a). They used neuron-EVP biomarker data from these samples which were collected up to 9 years before the onset of AD symptoms, to build a model that could accurately predict future AD diagnoses (Kapogiannis et al., 2019a). Surprisingly, the study found that levels of Aβ42, previously linked to neurotoxicity and neurodegeneration (Upadhya et al., 2020), were not significantly different between individuals with future AD and healthy controls (Kapogiannis et al., 2019a). Validating earlier findings the model did include tau biomarkers (Upadhya et al., 2020) and insulin-receptor-substrate-1 (IRS-1) phosphorylation as predictors, with pSer312-IRS-1 and pY-IRS-1 being powerful individual predictors (Bomfim et al., 2012; Kapogiannis et al., 2015; Mullins et al., 2017; Kapogiannis et al., 2019a; Akhtar and Sah, 2020). These findings support the use of neuron-derived EVP biomarkers as a potential tool for early diagnosis and treatment of AD, and further development as a clinical blood test for the disease is warranted.
5.2 Drug addiction
The cycle of substance abuse refers to the repeating pattern of drug use and negative consequences that often occurs in individuals with substance (Feltenstein and See, 2008; Koob and Volkow, 2016). This initial use can then lead to a dependence on the drug, which can cause physical and psychological symptoms of withdrawal when the drug is not taken, reenforcing the need for the drug (Baker et al., 2004; Koob and Volkow, 2016). EVPs have been used to understand the physiological conditions at each stage of the cycle (Chivero et al., 2021).
New insights emphasis that EVP modulated astroglia dysfunction and activation of CB1 enhance dopamine release, thereby contributing to cocaine addiction (Nakamura et al., 2019; Jarvis et al., 2020). In an in vivo cocaine addiction model, it was discovered that cocaine reduced the internalization of neuron-EVPs into astrocytes, resulting in less miR-124-3p delivered and decreased glutamate transporter-1 (GLT1) and GFAP levels (Jarvis et al., 2020). A decrease in GLT1 expression may inhibit glutamate reuptake, resulting in greater excitatory response and an increase in dopamine release, furthering addiction (Scofield and Kalivas, 2014; Jarvis et al., 2020). Additionally, the reduced expression of GLT1 and GFAP has also been implicated in several psychiatric disorders, such as anxiety (Jarvis et al., 2020; Jia et al., 2020). This change in EVP cargo may explain the correlation between withdrawal and psychological symptoms. Likewise, both in cocaine treated mice and cells, it has been seen that there is a stimulation of EVP release in the ventral tegmental area (VTA) (Nakamura et al., 2019). These EVPs are suggested to carry 2-Arachidonoylglycerol (2-AG) that binds to CB1, inhibiting GABA release and enhancing dopamine release to promote cocaine dependence (Covey et al., 2016; Nakamura et al., 2019).
As a result of tolerance to opioids, larger doses of the drug may be needed to achieve the same effects, increasing the likelihood of overdose (Chang et al., 2007; Boyer, 2012). Drug resistance and morphine tolerance appear to be linked to upregulation of sonic hedgehog (SHH) proteins in morphine-stimulated astro-EVPs activating SHH signaling in astrocytes via primary cilia (Huang, 2021; Ma et al., 2021; Luxmi and King, 2022). Researchers found that inhibiting either EVP release or primary cilia decreased morphine tolerance indicating its potential therapeutic role (Ma et al., 2021). The choroid plexus (ChP) and CSF has been associated with SHH (Huang et al., 2009; Li et al., 2016; Yang et al., 2021). Since astrocytes can take up ChP-EVPs, this mechanism may also have a role in mediating drug tolerance (Su and Pasternak, 2013; Pauwels et al., 2022). The relationship between neurological and psychiatric disorders with ChP is currently being studied including its role in opioid tolerance and nicotine addiction (Su and Pasternak, 2013; Ochoa et al., 2015; Lallai et al., 2019; Kenny et al., 2022). Additionally, the intercommunication between the ChP and astrocytes mediated by EVPs may contribute to the widespread ChP contamination in the sampling and profiling of brain tissue (Olney et al., 2022).
Research has shown that EVPs can serve as an effective tool for both screening and monitoring individuals with substance abuse disorders (Nakamura et al., 2019; Doncheck et al., 2020; Odegaard et al., 2020; Zhang et al., 2021b; Chen et al., 2022b; Odegaard et al., 2022). For example, a human study utilized plasma EVPs to monitor withdrawal syndrome of heroin and methamphetamine users at 3-month and 12-month stages (Chand et al., 2021; Chen et al., 2022b). Recent studies have found that the expression of EVP miRNA signatures reflects the differences in time point (Li et al., 2018c; Chen et al., 2022b). These EVP miRNAs may be revealed to contribute to psychiatric symptoms if validated by future studies (Chand et al., 2021; Sil et al., 2021; Chen et al., 2022b). Additionally, this data was used to predict the substance patients were using (Chen et al., 2022b). For example, miRNA signature hsa-mia-451a can be used to identify heroin-dependent patients, whereas hsa-mir-21a can be used to identify methamphetamine-dependent patients (Chen et al., 2022b). Another recent and comprehensive study aimed to delineate the role of an extracellular vesicle-associated microRNA-29a in chronic methamphetamine use disorder (Chand et al., 2021). Similarly, using serum EVPs, a team of researchers has successfully distinguished rats dependent on methamphetamine and from those dependent on ketamine (Li et al., 2018c). The unique miRNA profiles of each group could potentially be strongly linked to drug addiction and may help to elucidate the distinct addiction processes involved (Li et al., 2018c). Moreover, another recent study demonstrated the successful use of EVPs to monitor the synaptic genesis of fetuses that were exposed to oxycodone in utero in a rodent model (Odegaard et al., 2022). These results underscore the potential use of EVs as a tool for both screening and monitoring in the future.
It has been shown in postmortem studies that high levels of hyperphosphorylated tau correlate with the activation of microglia in opiate abusers, suggesting an accelerated AD progression (Kovacs et al., 2015). An investigation of morphine-dependent rhesus macaques provided insight into how EVPs may play a role in mediating this effect (Sil et al., 2021). In the presence of morphine, HIF-1α is seen as a regulator of BACE1 expression–an enzyme essential for the generation of β-amyloid–leading to neuroinflammation (Sil et al., 2021). This inflammation causes the release of amyloid cargo via astro-EVPs, which can lead to the production of Tau (Sil et al., 2021; Gomes et al., 2022). As an indication of the link between neurodegeneration and long-term oxycodone abuse, EVPs isolated from the plasma of non-human primates exposed to the drug were found to contain neurodegenerative and pro-inflammatory biomarkers (Kumar et al., 2021). These biomarkers have a potential clinical application as a risk monitoring tool (Kumar et al., 2021). Furthermore, a study using astro-derived EVPs from morphine-stimulated rodents found that miR-138 activates toll-like receptor 7 (TLR7) leading to neuroinflammation through microglia activation (Liao et al., 2020a). Since neuroinflammation is associated with the risk of developing AD, those who need to take pain relievers long-term may find benefit from silencing TLR7 through EVPs (Liao et al., 2020a).
In conclusion, EVPs have emerged as promising tools for understanding the complex mechanisms of substance abuse and addiction. The cargo of these EVPs, including miRNAs and other biomarkers, have been found to play a critical role in various stages of drug addiction, from initiation to withdrawal symptoms and the risk of developing neurodegenerative disorders (Nakamura et al., 2019; Doncheck et al., 2020; Odegaard et al., 2020; Zhang et al., 2021b; Chen et al., 2022b; Odegaard et al., 2022). Moreover, the use of EVs as a screening and monitoring tool may offer significant clinical benefits, for instance early identification of drug dependence and personalized therapeutic interventions. As further research is conducted in this area, the potential of EVPs to contribute to a better understanding of substance abuse and addiction continues to expand.
5.3 Major depression disorder
The most common neuropsychiatric disorder in the general population is Major Depressive Disorder (MDD) which is associated with functional impairment, morbidity, and low quality of life (Ferrari et al., 2013). It is currently being explored whether EVPs can detect drug response for MDD. A larger study was done with 60 participant to investigate miRNA cargo of MDD neuron-EVPs for drug response, indicating that they are reflective of the performance of antidepressant drug post treatment (Saeedi et al., 2021). Further research is need to determine whether miRNA signatures can forecast drug response prior to prescription. Additionally, miR-139-5p found in blood EVPs may play a role in the pathogenesis of depression, as demonstrated by the depressive-like behavior exhibited by mice following the transplantation of blood exosomes from MDD patients (Wei et al., 2020). Therefore, emphasizing its promising use in the diagnosis and treatment of MDD. A study discovered that MDD patients have higher levels of Neuron-EVPs compared to healthy controls (Nasca et al., 2021). The release of these EVPs has been linked to an increase in glutamate levels, which emphasizes the relationship between MDD and abnormal glutamatergic neurotransmission (Frye et al., 2007; Deschwanden et al., 2011; Lachenal et al., 2011; Lee et al., 2013). The study also found that Neuron-EVPs have a higher concentration of insulin receptor substrate-1 (IRS-1) associated with suicidality and anhedonia, confirming previous research linking MDD to insulin resistance, which also impacts glutamate levels (Grillo et al., 2011; Al-Hakeim et al., 2018; Hamer et al., 2019; Nasca et al., 2021). Further research is needed to understand how EVPs modulate glutamate levels in MDD patients, as well as how this knowledge can relate to new treatment methods targeting glutamate pathways like ketamine (Lener et al., 2017; Murrough et al., 2017). In addition to neuron-EVPs, astro-EVPs are also being studied in relation to MDD (Xie et al., 2022). A study found that MDD patients have an increase of inflammatory markers in Astro-EVPs when compared to healthy controls, supporting previous research linking depression to neuroinflammation (Brites and Fernandes, 2015; Troubat et al., 2021; Xie et al., 2022). These studies highlights the potential of EVPs to provide new understanding of the living brain and MDD.
5.4 Bipolar disorder
Bipolar disorder (BD) is a mental illness that affects about 1%–5% of the population that involves recurrent episodes of mania, hypomania, and depression and is also associated with a high risk of sucide (Dome et al., 2019). Selective serotonin reuptake inhibitors (SSRIs) often triggering a manic episode (Gitlin, 2019) in patients suffering from BD-related depression, indicating the need for alternative treatment. Through analyzing the content of neuron-EVPs, researchers have proven that infliximab (TNF blocker) can treat BD anhedonia by reducing neuroinflammation (Lee et al., 2021; Mansur et al., 2021). In addition to reducing anhedonia, infliximab treated patient derived-EVPs are associated with insulin cascades in neurons, indicating insulin as a relevant potential target for BD intervention (Mansur et al., 2021). Likewise, by analyzing blood-derived neuron-EVP metabolites a study found a connection between glucose metabolism dysfunction and BD (Du et al., 2022). These metabolites can potentially be used to classify samples from patients with BD, SCZ, MDD and healthy subjects, improving current psychotic diagnosis (Singh and Rajput, 2006). Similarly, research is being done on using miRNA signatures as biomarkers for BD diagnosis (Ceylan et al., 2020). For example, plasma EVPs were used to compare patients in depressive, manic, and euthymic states against a healthy control (Ceylan et al., 2020). 13 miRNAs showed significant differences between patients with BD and healthy individuals, with no significant alterations among different states of BD (Ceylan et al., 2020). Additional studies focusing on neuron-EVPs are necessary to identify potential biomarkers for detecting the transition from a depressive episode to a manic episode and vice versa, which can lead to improved treatment options.
5.5 Schizophrenia
Schizophrenia (SCZ) is a chronic incurable mental disorder characterized by abnormal thought processes and behaviors, such as delusions and hallucinations (Du et al., 2019). In order to better understand the epistemology, potential biomarkers, and treatments of SCZ, EVPs are employed in SCZ research (Oraki Kohshour et al., 2022). Previously, insulin sensitization was believed to be a side effect of SCZ prescriptions, but recent studies suggest this may be due to excitotoxicity caused by the disorder (Plitman et al., 2014; Kapogiannis et al., 2019b; Pomytkin et al., 2019). As a result of studying peripheral blood neuron-EVPs, scientists were able to determine that insulin resistance exists in vivo, even in the absence of drug treatment, emphasizing its role in the dysfunction of brain development and maturation in SCZ (Kapogiannis et al., 2019b; Wijtenburg et al., 2019). To understand the pathophysiology of SCZ and identify potential biomarkers, the first genome-wide miRNA expression profiling in serum-derived EVPs from SCZ patients was performed (Du et al., 2019). The signatures were found to be enriched for genes associated with protein glycosylation, neurotransmitter receptors, and dendrite spine development (Du et al., 2019). Furthermore, hsa-miR-206, a regulator of neutrophic factor expression, was found to be upregulated in blood EVPs of SCZ patients (Du et al., 2019). This data was used to find 11 miRNA signatures that were highly accurate in predicting SCZ and could serve as biomarkers (Du et al., 2019). A follow-up study identified 25 metabolites from neuron-EVPs that can be used to classify samples from patients and controls accurately (Du et al., 2021). These metabolites were enriched in SCZ pathways, such as glycerophospholipid metabolism (Du et al., 2021). These findings point to an important role for EVP metabolite dysregulation in SCZ pathophysiology and indicate a strong potential for their use in SCZ diagnosis. EVPs have also demonstrated treatment potentials. In one study, mesenchymal stem cell-derived EVPs were administered to a rodent model of SCZ, reducing SCZ-like behaviors and neurotoxic levels of glutamate (Tsivion-Visbord et al., 2020). Overall, research focusing on EVPs has contributed to our comprehension of the pathophysiology, potential biomarkers, and treatments of schizophrenia, underscoring the need for follow up studies to enhance diagnosis and management.
5.6 Viral infections
There is evidence that EVPs may play a role in the infection and replication of neuronal viruses (Kutchy et al., 2020). For example, research has shown that EVPs can transfer viral particles and genetic material to target cells, potentially contributing to the spread of infection (Kutchy et al., 2020). The role of EVPs in the infection process is greater emphasized in research being done on HIV (Hu et al., 2016; Dagur et al., 2020; Hu et al., 2020). HIV-associated neurocognitive disorders (HAND) are a group of conditions that can affect the nervous, even if there is no detectable viral load (Campbell and Mocchetti, 2021). While neurons are less susceptible to direct infection, infected microglia can contribute to neuronal damage through the release of EVPs (Kannan et al., 2022) One study demonstrates that HIV protein Tat can cause the release of EVPs from microglia, which carry proinflammatory protein NLRP3 cargo (Kannan et al., 2022). When these microglia-EVPs are taken up by neurons, they can lead to synaptodendritic injury and functional impairment, as indicated by decreased mEPSCs (Campbell and Mocchetti, 2021; Kannan et al., 2022). Additionally, Tat has been found to alter the EVP cargo from astrocytes, resulting in impairment of the synaptic architecture of neurons (Kannan et al., 2022). These findings suggest that EVPs may play a mediating role in the microenvironment in the development of HAND (Guha et al., 2019b; Kannan et al., 2022).
5.7 Brain tumors
There are various types of brain cancers including glioma, meningioma, astrocytoma, and metastatic brain cancer (Passiglia et al., 2018; Boire et al., 2020). The most well studied of these cancers is a type of glioma referred to as glioblastoma (GBM) (Tominaga et al., 2015; Hallal et al., 2020; Ricklefs et al., 2020; Rana et al., 2021a; Rana et al., 2021b; Ricklefs et al., 2022). GBM is a type of brain cancer that is aggressive and treatment-resistant (Simon et al., 2020). It is characterized by the presence of cancer stem cells (CSCs) and a complex and dynamic microenvironment that includes endothelial cells, astrocytes, and immune cells (Yekula et al., 2020). In the context of GBM, EVPs have been shown to contribute to the maintenance and survival of CSCs and promote GBM recurrence (Simon et al., 2020). Understanding the mechanisms by which EVPs are secreted and target recipient cells in the GBM microenvironment may provide new insights into the disease. For example, GBM-EVPs have been shown to alter the phenotype of normal astrocytes to acquire tumor-supporting capabilities (Zeng et al., 2020; Nieland et al., 2021; Zhou et al., 2022). One study found that EVPs containing miR-19a, delivered from astrocytes to tumor cells, could downregulate PTEN expression, thus upregulating brain metastasis (Simon et al., 2020). Similarly, GBM-EVPs are suggested to stimulate tumor-promoting M2 phenotypes in microglia, as opposed to immune-supporting M1 phenotypes (Simon et al., 2020). GBM-EVPs may also serve as biomarkers for diagnosis, prognosis, and therapeutic response and may be used as a means of drug delivery to the target site (Hallal et al., 2019). For example, a study using serum-derived EVPs found that 7 miRNA signatures can distinguish GBM samples from healthy controls (Ebrahimkhani et al., 2018). Furthermore, miRNA signature miR-9 was found to be upregulated in Temozolomide drug-resistant cells (Simon et al., 2020). In order to reverse this effect, scientists delivered anti-miR-9 to cells via EVP delivery (Simon et al., 2020). As a result of their study, anti-miRNA appears to be a promising therapeutic to counteract these outcomes (Simon et al., 2020). While EVP application for GBM has been extensively researched, EVPs are also showing potential as diagnostic tools for other brain cancers and could aid as a medium to better understand their etiology (Tominaga et al., 2015; Hallal et al., 2020; Ricklefs et al., 2020; Rana et al., 2021a; Rana et al., 2021b; Ricklefs et al., 2022). For example, a study examining brain metastatic cancer has indicated that EVPs containing miRNA-181-c might have the ability to disrupt the blood brain barrier (BBB), thereby facilitating cancer metastasis (Tominaga et al., 2015). Likewise, Graziano et al. has detected varying levels of miR-1, miR-206, miR-663 in the blood EVs of meningioma grade II patients in accordance to patient conditions pre- and post-surgery (Graziano et al., 2021). By conducting research on all types of brain cancer using EVPs, we can gain a deeper understanding of cancer’s underlying mechanisms and develop more effective treatment strategies.
5.8 Prion disorders
Prions are misfolded proteins that are transmissible and causative agents for neurodegenerative diseases (Prusiner et al., 1998). Prions are found on the surface of many cells and have been discovered to be associated with EVPs (Bellingham et al., 2012; Cheng et al., 2018). The precise mechanism of prion infections remain a matter of intense debate and EVPs are implicated to have a role in prion infection (Fevrier et al., 2004).
6 Clinical application
6.1 Liquid biopsy
Cerebrospinal fluid (CSF) biomarkers have been used for the diagnosis of neurodegenerative diseases, but the invasive nature of lumbar puncture collection makes it an impractical choice for routine screening (Wright et al., 2012). Instead, blood is the preferred biofluid for these tests because it can be easily and routinely obtained from patients. However, blood is in contact with the entire body, making it difficult to isolate brain-derived EVPs from those of other tissues (Monteiro-Reis et al., 2021). This introduce the need for CSF biomarkers that can be found in blood (Alawode et al., 2021; Mankhong et al., 2022). Blood-based EVP biomarkers have several advantages as diagnostic tools (Wang et al., 2017). They can be collected using a minimally-invasive procedure and can be repeatedly sampled to monitor changes in the molecular landscape of a disease or treatment outcome (Wang et al., 2017). In addition, this approach may uncover underlying pathological mechanisms that were previously unnoticed (Monteiro-Reis et al., 2021). There are some limitations to this approach, including issues with the isolation and purification of EVPs and the fact that some biomarkers, including miRNA, may change throughout different stages of a disease (Abdel-Haq, 2020). These problems, however, may be alleviated by further research. Many studies have investigated miRNAs, proteins, and metabolites that are useful in diagnosing psychiatric and neurodegenerative disorders (see Table 1). The use of blood-based EVP biomarkers as diagnostic tools in neurological diseases has the potential to enable early detection and treatment, as well as to allow for the monitoring of treatment outcomes and disease progression (Abdel-Haq, 2020).
6.2 Therapeutics
Currently, the treatment options for psychiatric and neurological disorders are often limited and may not be effective for all individuals (Howes et al., 2022; Miller and Raison, 2023). In fact, the only approved disease-modifying medication for AD, Aducanumab, has faced controversy in its approval and the minimal data they do have on its effectiveness is limited to those with early onset AD (Howard and Liu, 2020; Beshir et al., 2022). In addition, some therapies can have significant side effects, which potentially reduce significantly quality of life (McCammon and Sive, 2015; Miller and Raison, 2023). For example, many of those with schizophrenia, bipolar disorder, and personality disorders are prescribed antipsychotics which have severe, unpleasant and even lethal side effects including tardive dyskinesia, neuroleptic malignant syndrome, weight gain, diabetes, sedation, emotional blunting and even sudden cardiac death (Moncrieff et al., 2020). It is due to the mental and behavioral changes that many people do not feel like themselves on their medications and avoid taking them (Moncrieff et al., 2020). This highlights the need for therapies with higher sensitivity, higher accuracy, and with no or fewer side effects.
When developing new therapeutics it is important that the drug is selective, does not create an immune response or cause toxicity, and is effective (Strovel et al., 2004; Huggins et al., 2012; Elsharkasy et al., 2020). Recent research has shown that EVPs may be cable of meeting these criteria. The emerging role of EVPs as a therapeutic has been greater understood through studies done on routes of administration, types of cargo that can be delivered, and their inherent nature (Mulcahy et al., 2014; Escude Martinez de Castilla et al., 2021).
According to studies conducted on ways of administration, EVPs enable molecules to cross the BBB, which would not have been possible without them (Qu et al., 2018). Due to the fact that dopamine cannot cross the BBB, scientist examined the effects of intravenously administered dopamine-loaded blood EVPs versus administration of free-dopamine on mice (Cestelli et al., 2001). In comparison with those given free-dopamine after 6 h, those given dopamine-loaded blood EVPs had a 15-fold higher distribution of dopamine in the brain (Qu et al., 2018). Additionally, there was no sign of toxicity in the hippocampus, liver, spleen, and lung and there was a minimal immune response (Qu et al., 2018). Other routes of administration have also been investigated. It has been found that intrathecal administration leads to the highest concentration of the drug reaching the brain; however, this route is invasive and not practical for drugs that need multiple doses (Gratpain et al., 2021). Accordingly, researchers are investigating the intranasal route as a second-best route (Gratpain et al., 2021). Since this route goes directly to the brain it has been considered efficient (Liao et al., 2020b; Gratpain et al., 2021). For example, a study administering IFNγ-dendrictic cell-EVPs found the intranasal route targeted the CNS better than the intravenous route and also lead to less accumulation in the liver (Pusic et al., 2021). Additionally, the EVPs have been found to protect the drug from being metabolized by nasal mucosa enzymes (Gratpain et al., 2021). Yet, there is a limitation of how much fluid can be administered before it is drained into the esophagu (Gratpain et al., 2021).
Due to the fact that EVPs are selectively absorbed, they have the potential to be used as targeted drugs. For example, research done on administering IFNγ-dendrictic cell-EVPs intranasally suggest that these EVPs were preferentially taken up by oligodendrocytes, indicating that dendritic cell-EVPs maybe used to target oligodendrocytes (Pusic et al., 2021). It is suggested that the selectivity of EVPs is due to proteins on their surface (Liu et al., 2015). Accordingly, researchers are investigating how these membranes can be edited to be more target (Liu et al., 2015). For example, scientists found that EVPs expressing the neuron-specific rabies viral glycoprotein (RVG) peptide on the membrane could successfully enter cells expressing the acetylcholine receptor on their membranes and not those without it (Liu et al., 2015). Thus, suggesting ways to target specific neurons within the CNS. Likewise, scientists have been striving to develop glioma-targeting EVPs to transport therapeutics exclusively to cancerous cells (Jia et al., 2018; Lino et al., 2021). For example, one study demonstrated how engineered EVPs, containing therapeutic siRNA, could target glioma cells by expressing a protein that binds to neuropilin-1 (NRP-1), which is recognized for its overexpression on the surface of these cells (Jia et al., 2018). This marks a significant shift in cancer therapy from the conventional use of chemotherapy that tends to target all cells (Rébé and Ghiringhelli, 2015). In spite of this, damage to the structure can occur during membrane modification, making large-scale use of these techniques difficult (Herrmann et al., 2021). Therefore, finding EVPs that naturally target specific cells may be a more practical solution.
Although EVPs can be used to administer a variety of cargo including miRNA, proteins, and molecules, a myriad studies have been focusing on their delivery of siRNA. One study administering mu-opioid receptor (MOR) siRNA-loaded EVPs to mice have uncover its ability to prevent morphine relapse (Liu et al., 2015). In another study, EVPs loaded with siRNA targeting Huntingtin mRNA were effectively internalized by mouse primary cortical neurons and significantly silenced Huntingtin mRNA and proteins, further emphasizing the use of siRNA-loaded EVPs for knockdowns (Didiot et al., 2016). Neurological research often employs stereotaxic surgery to infect rodents with viruses that will lead to gene knockdowns. (Nectow and Nestler, 2020). However, this approach is not translational in the human model due to its invasiveness and potential immune response to the virus (Cetin et al., 2006; Sack and Herzog, 2009). It is clear from these earlier studies, that through the use of EVP-loaded siRNA, these knockdowns can still be achieved while also having the potential for therapeutic application in the future.
7 Conclusion and future directions
Collectively, in this review we describe the Universe of EVPs, while providing evidence that neuroregulatory EVPs convey neuroprotective and neurodegenerative effects. The diversity of EVP populations reflects their origin, complexity, and roles in multiple neurological processes. While an unprecedented amount of scientific work has propelled the development of cell-based strategies to protect and repair the injured brain. Current clinical outcomes from treatments in patients’ psychiatric and neurodegenerative disorders such as Alzheimer’s, Parkinson’s disease or schizophrenia, have not reached the anticipated and strong success of preclinical trials. Patient heterogeneity and lack of standardized procedures might have contributed to this adverse scenario. Immune response, immune compatibility, dosing, and administration route may help explain the limited translatability of current therapies. EVPs have brought excitement to new diagnostics and therapeutical alternatives due the incremental evidence of their role in signal transmission and amplification as vehicles of cell communication, healthy phenotypes, aging and pathological states and diseases.
Therefore, there is a critical need to further characterize the function of the neurosecretome with the aim to characterize mechanisms that allow to sustain recovery in psychiatric and neurodegenerative disorders. In the clinical and preclinical settings, it is essential to identify the vesicles, particles and their subpopulations carrying novel and key molecules or group of molecules responsible for the known therapeutic effects, define the relationship between cells and their secreted factors, and elucidate the temporal window of application and dynamics of release.
A major argument in the field is whether there are biomarkers associated with brain derived EVPs and if so then how is one to evaluate their presence in the biofluids. In this context, we evaluated over ∼20 different studies that have isolated brain-derived EVPs from various biofluids (blood, serum, plasma, CSF, tissue, and in-vitro tissue cultures) using different EVP isolation methods. A detailed list of brain-derived biomarkers that are being studied in EVPs is provided on Table 1. This comprehensive and cumulative study allowed us to envision brain derived EVPs with a global view. Primarily, Aβ42 (Li et al., 2022), P-tau (Palmqvist et al., 2020), APOE4 (for AD) (Palmqvist et al., 2023), α-synuclein (for PD) (Niu et al., 2020), many miRNAs (for AD (Wiedrick et al., 2019), addiction (Chand et al., 2021), SCZ (Du et al., 2019), GBM (Ebrahimkhani et al., 2018)) were discovered in the isolated EVPs. Although most published studies on Table 1 suggested the presence of brain-derived biomarkers in the systemic circulation, it was not entirely clear if there is a linear correlation between the biomarkers and the disease. However, given the diversity of the isolation methods and biofluids it is difficult to come to concrete conclusion. Overall, emerging evidence suggest that brain derived biomarkers are present in the various EVPs and we’re embarking on the journey of reproducible detection and direct correlation of EVPs with brain cancers, psychiatric, neurodegenerative diseases. The incremental knowledge derived from neurosecretome studies will help to design improved liquid-biopsy assays and therapies either using EVPs or their bioengineering analogs to deliver efficiently beneficial molecules for repairing the injured brain. Innovative and groundbreaking research has unveiled the immense potential of EVPs in treating psychopathological phenotypes, paving the way for an exciting new era of clinical benefits for patients.
Author contributions
Conceptualization: ND and TS Writing—original draft preparation: TS, T-YC, EG-K, and ND. Writing—review and editing: T-YC, EG-K, TS, and ND Data curation: T-YC, EG-K, TS, and ND Visualization: T-YC, EG-K, TS, and ND Supervision: EG-K and ND Funding acquisition: ND All authors have read and agreed to the published version of the manuscript.
Funding
ND, EG-K, and T-YC are funded from NIH/NIA, R21AG07848. ND is also funded from Alzheimer’s disease research center (ADRC) and Friedman Brain Institute (FBI) at Icahn School of Medicine at Mount Sinai.
Acknowledgments
Figures were constructed using the BioRender program (Agreement number: PA22VC7880) and Affinity Designer (available in https://affinity.serif.com/en-gb/). We would like to acknowledge Ronald E. Gordon for help electron microscopy. Alex Charney, Carlos Cordon-Cardo, Panagiotis Roussos, John Fullard, Nikolaos Robakis, Stephen Salton, Brian Kopell, Bojan Losic, Gustavo Stolovitzky, Susmita Sahoo, and Natasha Kyprianou for several useful discussions. The authors thank following funding agencies and foundations: National Institutes of Health NHLBI, R01HL148786 (ND, SL, and SS); The Alzheimer’s Disease Research Center and Friedman Brain Institute at Mount Sinai (ND); R01CA232574/National Institutes of Health/NCI (NK), the Deane Prostate Health and The Arthur M. Blank Family Foundation (AKT).
Conflict of interest
The authors declare that the research was conducted in the absence of any commercial or financial relationships that could be construed as a potential conflict of interest.
Publisher’s note
All claims expressed in this article are solely those of the authors and do not necessarily represent those of their affiliated organizations, or those of the publisher, the editors and the reviewers. Any product that may be evaluated in this article, or claim that may be made by its manufacturer, is not guaranteed or endorsed by the publisher.
References
Abdel-Haq, H. (2020). The potential of liquid biopsy of the brain using blood extracellular vesicles: The first step toward effective neuroprotection against neurodegenerative diseases. Mol. Diagn Ther. 24 (6), 703–713. doi:10.1007/s40291-020-00493-4
Agliardi, C., Guerini, F. R., Zanzottera, M., Bianchi, A., Nemni, R., and Clerici, M. (2019). SNAP-25 in serum is carried by exosomes of neuronal origin and is a potential biomarker of Alzheimer's disease. Mol. Neurobiol. 56 (8), 5792–5798. doi:10.1007/s12035-019-1501-x
Agosta, F., Dalla Libera, D., Spinelli, E. G., Finardi, A., Canu, E., Bergami, A., et al. (2014). Myeloid microvesicles in cerebrospinal fluid are associated with myelin damage and neuronal loss in mild cognitive impairment and Alzheimer disease. Ann. Neurol. 76 (6), 813–825. doi:10.1002/ana.24235
Aires, I. D., Ribeiro-Rodrigues, T., Boia, R., Ferreira-Rodrigues, M., Girao, H., Ambrosio, A. F., et al. (2021). Microglial extracellular vesicles as vehicles for neurodegeneration spreading. Biomolecules 11 (6), 770. doi:10.3390/biom11060770
Akhtar, A., and Sah, S. P. (2020). Insulin signaling pathway and related molecules: Role in neurodegeneration and Alzheimer's disease. Neurochem. Int. 135, 104707. doi:10.1016/j.neuint.2020.104707
Al-Hakeim, H. K., Al-Kufi, S. N., Al-Dujaili, A. H., and Maes, M. (2018). Serum interleukin levels and insulin resistance in major depressive disorder. CNS Neurol. Disord. Drug Targets 17 (8), 618–625. doi:10.2174/1871527317666180720155300
Alawode, D. O. T., Heslegrave, A. J., Ashton, N. J., Karikari, T. K., Simren, J., Montoliu-Gaya, L., et al. (2021). Transitioning from cerebrospinal fluid to blood tests to facilitate diagnosis and disease monitoring in Alzheimer's disease. J. Intern Med. 290 (3), 583–601. doi:10.1111/joim.13332
Albertini, G., Etienne, F., and Roumier, A. (2020). Regulation of microglia by neuromodulators: Modulations in major and minor modes. Neurosci. Lett. 733, 135000. doi:10.1016/j.neulet.2020.135000
Alem, F., Olanrewaju, A. A., Omole, S., Hobbs, H. E., Ahsan, N., Matulis, G., et al. (2021). Exosomes originating from infection with the cytoplasmic single-stranded RNA virus Rift Valley fever virus (RVFV) protect recipient cells by inducing RIG-I mediated IFN-B response that leads to activation of autophagy. Cell Biosci. 11 (1), 220. doi:10.1186/s13578-021-00732-z
Alvarez-Erviti, L., Seow, Y., Schapira, A. H., Gardiner, C., Sargent, I. L., Wood, M. J., et al. (2011). Lysosomal dysfunction increases exosome-mediated alpha-synuclein release and transmission. Neurobiol. Dis. 42 (3), 360–367. doi:10.1016/j.nbd.2011.01.029
Antes, T. J., Middleton, R. C., Luther, K. M., Ijichi, T., Peck, K. A., Liu, W. J., et al. (2018). Targeting extracellular vesicles to injured tissue using membrane cloaking and surface display. J. Nanobiotechnology 16 (1), 61. doi:10.1186/s12951-018-0388-4
Antonyak, M. A., Li, B., Boroughs, L. K., Johnson, J. L., Druso, J. E., Bryant, K. L., et al. (2011). Cancer cell-derived microvesicles induce transformation by transferring tissue transglutaminase and fibronectin to recipient cells. Proc. Natl. Acad. Sci. U. S. A. 108 (12), 4852–4857. doi:10.1073/pnas.1017667108
Antonyak, M. A., Wilson, K. F., and Cerione, R. A. (2012). R(h)oads to microvesicles. Small GTPases 3 (4), 219–224. doi:10.4161/sgtp.20755
Bahrini, I., Song, J.-h., Diez, D., and Hanayama, R. (2015). Neuronal exosomes facilitate synaptic pruning by up-regulating complement factors in microglia. Sci. Rep. 5 (1), 7989. doi:10.1038/srep07989
Baker, T. B., Piper, M. E., McCarthy, D. E., Majeskie, M. R., and Fiore, M. C. (2004). Addiction motivation reformulated: An affective processing model of negative reinforcement. Psychol. Rev. 111 (1), 33–51. doi:10.1037/0033-295X.111.1.33
Balaj, L., Lessard, R., Dai, L., Cho, Y.-J., Pomeroy, S. L., Breakefield, X. O., et al. (2011). Tumour microvesicles contain retrotransposon elements and amplified oncogene sequences. Nat. Commun. 2 (1), 180. doi:10.1038/ncomms1180
Barrieux, A., Ingraham, H. A., Nystul, S., and Rosenfeld, M. G. (1976). Characterization of the association of specific proteins with messenger ribonucleic acid. Biochemistry 15 (16), 3523–3528. doi:10.1021/bi00661a020
Barth, H. G., Boyes, B. E., and Jackson, C. (1994). Size exclusion chromatography. Anal. Chem. 66 (12), 595R–620R. doi:10.1021/ac00084a022
Basso, M., and Bonetto, V. (2016). Extracellular vesicles and a novel form of communication in the brain. Front. Neurosci. 10, 127. doi:10.3389/fnins.2016.00127
Bellingham, S. A., Coleman, B. M., and Hill, A. F. (2012). Small RNA deep sequencing reveals a distinct miRNA signature released in exosomes from prion-infected neuronal cells. Nucleic Acids Res. 40 (21), 10937–10949. doi:10.1093/nar/gks832
Beshir, S. A., Aadithsoorya, A. M., Parveen, A., Goh, S. S. L., Hussain, N., and Menon, V. B. (2022). Aducanumab therapy to treat Alzheimer's disease: A narrative review. Int. J. Alzheimer's Dis. 2022, 9343514. doi:10.1155/2022/9343514
Bianco, F., Pravettoni, E., Colombo, A., Schenk, U., Möller, T., Matteoli, M., et al. (2005). Astrocyte-derived ATP induces vesicle shedding and IL-1 beta release from microglia. J. Immunol. 174 (11), 7268–7277. doi:10.4049/jimmunol.174.11.7268
Bisogno, T., and Di Marzo, V. (2010). Cannabinoid receptors and endocannabinoids: Role in neuroinflammatory and neurodegenerative disorders. CNS Neurol. Disord. Drug Targets 9 (5), 564–573. doi:10.2174/187152710793361568
Boecker, A. H., Bozkurt, A., Kim, B. S., Altinova, H., Tank, J., Deumens, R., et al. (2018). Cell-enrichment with olfactory ensheathing cells has limited local extra beneficial effects on nerve regeneration supported by the nerve guide Perimaix. J. Tissue Eng. Regen. Med. 12 (11), 2125–2137. doi:10.1002/term.2731
Boire, A., Brastianos, P. K., Garzia, L., and Valiente, M. (2020). Brain metastasis. Nat. Rev. Cancer 20 (1), 4–11. doi:10.1038/s41568-019-0220-y
Bomfim, T. R., Forny-Germano, L., Sathler, L. B., Brito-Moreira, J., Houzel, J. C., Decker, H., et al. (2012). An anti-diabetes agent protects the mouse brain from defective insulin signaling caused by Alzheimer's disease-associated Aβ oligomers. J. Clin. Invest 122 (4), 1339–1353. doi:10.1172/JCI57256
Borodinsky, L. N., Belgacem, Y. H., Swapna, I., and Sequerra, E. B. (2014). Dynamic regulation of neurotransmitter specification: Relevance to nervous system homeostasis. Neuropharmacology 78, 75–80. doi:10.1016/j.neuropharm.2012.12.005
Bose, A., and Beal, M. F. (2016). Mitochondrial dysfunction in Parkinson's disease. J. Neurochem. 139 (1), 216–231. doi:10.1111/jnc.13731
Boyer, E. W. (2012). Management of opioid analgesic overdose. N. Engl. J. Med. 367 (2), 146–155. doi:10.1056/NEJMra1202561
Brennan, K., Martin, K., FitzGerald, S. P., O’Sullivan, J., Wu, Y., Blanco, A., et al. (2020). A comparison of methods for the isolation and separation of extracellular vesicles from protein and lipid particles in human serum. Sci. Rep. 10 (1), 1039. doi:10.1038/s41598-020-57497-7
Brites, D., and Fernandes, A. (2015). Neuroinflammation and depression: Microglia activation, extracellular microvesicles and microRNA dysregulation. Front. Cell. Neurosci. 9, 476. doi:10.3389/fncel.2015.00476
Brügger, B., Glass, B., Haberkant, P., Leibrecht, I., Wieland, F. T., and Kräusslich, H. G. (2006). The HIV lipidome: A raft with an unusual composition. Proc. Natl. Acad. Sci. 103 (8), 2641–2646. doi:10.1073/pnas.0511136103
Bryl-Górecka, P., Sathanoori, R., Al-Mashat, M., Olde, B., Jögi, J., Evander, M., et al. (2018). Effect of exercise on the plasma vesicular proteome: A methodological study comparing acoustic trapping and centrifugation. Lab. Chip 18 (20), 3101–3111. doi:10.1039/c8lc00686e
Busatto, S., Vilanilam, G., Ticer, T., Lin, W. L., Dickson, D. W., Shapiro, S., et al. (2018). Tangential flow filtration for highly efficient concentration of extracellular vesicles from large volumes of fluid. Cells 7 (12), 273. doi:10.3390/cells7120273
Campbell, L. A., and Mocchetti, I. (2021). Extracellular vesicles and HIV-associated neurocognitive disorders: Implications in neuropathogenesis and disease diagnosis. Neurotox. Res. 39 (6), 2098–2107. doi:10.1007/s12640-021-00425-y
Carnino, J. M., Lee, H., and Jin, Y. (2019). Isolation and characterization of extracellular vesicles from broncho-alveolar lavage fluid: A review and comparison of different methods. Respir. Res. 20 (1), 240. doi:10.1186/s12931-019-1210-z
Ceccarelli, L., Giacomelli, C., Marchetti, L., and Martini, C. (2021). Microglia extracellular vesicles: Focus on molecular composition and biological function. Biochem. Soc. Trans. 49 (4), 1779–1790. doi:10.1042/BST20210202
Cestelli, A., Catania, C., D'Agostino, S., Di Liegro, I., Licata, L., Schiera, G., et al. (2001). Functional feature of a novel model of blood brain barrier: Studies on permeation of test compounds. J. Control Release 76 (1-2), 139–147. doi:10.1016/s0168-3659(01)00431-x
Cetin, A., Komai, S., Eliava, M., Seeburg, P. H., and Osten, P. (2006). Stereotaxic gene delivery in the rodent brain. Nat. Protoc. 1 (6), 3166–3173. doi:10.1038/nprot.2006.450
Ceylan, D., Tufekci, K. U., Keskinoglu, P., Genc, S., and Özerdem, A. (2020). Circulating exosomal microRNAs in bipolar disorder. J. Affect. Disord. 262, 99–107. doi:10.1016/j.jad.2019.10.038
Chand, S., Gowen, A., Savine, M., Moore, D., Clark, A., Huynh, W., et al. (2021). A comprehensive study to delineate the role of an extracellular vesicle-associated microRNA-29a in chronic methamphetamine use disorder. J. Extracell. Vesicles 10 (14), e12177. doi:10.1002/jev2.12177
Chang, G., Chen, L., and Mao, J. (2007). Opioid tolerance and hyperalgesia. Med. Clin. North Am. 91 (2), 199–211. doi:10.1016/j.mcna.2006.10.003
Chen, F., Xu, Y., Shi, K., Zhang, Z., Xie, Z., Wu, H., et al. (2022). Multi-omics study reveals associations among neurotransmitter, extracellular vesicle-derived microRNA and psychiatric comorbidities during heroin and methamphetamine withdrawal. Biomed. Pharmacother. 155, 113685. doi:10.1016/j.biopha.2022.113685
Chen, T.-Y., Gonzalez-Kozlova, E., Soleymani, T., La Salvia, S., Kyprianou, N., Sahoo, S., et al. (2022). Extracellular vesicles carry distinct proteo-transcriptomic signatures that are different from their cancer cell of origin. iScience 25 (6), 104414. doi:10.1016/j.isci.2022.104414
Cheng, L., Zhao, W., and Hill, A. F. (2018). Exosomes and their role in the intercellular trafficking of normal and disease associated prion proteins. Mol. Asp. Med. 60, 62–68. doi:10.1016/j.mam.2017.11.011
Chiasserini, D., van Weering, J. R. T., Piersma, S. R., Pham, T. V., Malekzadeh, A., Teunissen, C. E., et al. (2014). Proteomic analysis of cerebrospinal fluid extracellular vesicles: A comprehensive dataset. J. Proteomics 106, 191–204. doi:10.1016/j.jprot.2014.04.028
Chivero, E. T., Dagur, R. S., Peeples, E. S., Sil, S., Liao, K., Ma, R., et al. (2021). Biogenesis, physiological functions and potential applications of extracellular vesicles in substance use disorders. Cell. Mol. Life Sci. 78, 4849–4865. doi:10.1007/s00018-021-03824-8
Chomyn, A., and Attardi, G. (2003). MtDNA mutations in aging and apoptosis. Biochem. Biophys. Res. Commun. 304 (3), 519–529. doi:10.1016/s0006-291x(03)00625-9
Ciardiello, C., Migliorino, R., Leone, A., and Budillon, A. (2020). Large extracellular vesicles: Size matters in tumor progression. Cytokine & Growth Factor Rev. 51, 69–74. doi:10.1016/j.cytogfr.2019.12.007
Cicognola, C., Brinkmalm, G., Wahlgren, J., Portelius, E., Gobom, J., Cullen, N. C., et al. (2019). Novel tau fragments in cerebrospinal fluid: Relation to tangle pathology and cognitive decline in Alzheimer's disease. Acta Neuropathol. 137 (2), 279–296. doi:10.1007/s00401-018-1948-2
Clark, D. J., Fondrie, W. E., Liao, Z., Hanson, P. I., Fulton, A., Mao, L., et al. (2015). Redefining the breast cancer exosome proteome by tandem mass tag quantitative proteomics and multivariate cluster analysis. Anal. Chem. 87 (20), 10462–10469. doi:10.1021/acs.analchem.5b02586
Claude, A. (1970). Growth and differentiation of cytoplasmic membranes in the course of lipoprotein granule synthesis in the hepatic cell. I. Elaboration of elements of the Golgi complex. J. Cell Biol. 47 (3), 745–766. doi:10.1083/jcb.47.3.745
Colombo, E., Borgiani, B., Verderio, C., and Furlan, R. (2012). Microvesicles: Novel biomarkers for neurological disorders. Front. Physiol. 3, 63. doi:10.3389/fphys.2012.00063
Colombo, M., Raposo, G., and Théry, C. (2014). Biogenesis, secretion, and intercellular interactions of exosomes and other extracellular vesicles. Annu. Rev. Cell Dev. Biol. 30, 255–289. doi:10.1146/annurev-cellbio-101512-122326
Combes, V., El-Assaad, F., Faille, D., Jambou, R., Hunt, N. H., and Grau, G. E. R. (2010). Microvesiculation and cell interactions at the brain–endothelial interface in cerebral malaria pathogenesis. Prog. Neurobiol. 91 (2), 140–151. doi:10.1016/j.pneurobio.2010.01.007
Costa-Silva, B., Aiello, N. M., Ocean, A. J., Singh, S., Zhang, H., Thakur, B. K., et al. (2015). Pancreatic cancer exosomes initiate pre-metastatic niche formation in the liver. Nat. Cell Biol. 17 (6), 816–826. doi:10.1038/ncb3169
Covey, D. P., Zlebnik, N. E., and Cheer, J. F. (2016). Endocannabinoid regulation of cocaine reinforcement: An upper or downer? Neuropsychopharmacology 41 (9), 2189–2191. doi:10.1038/npp.2016.25
Cowen, P. J., and Browning, M. (2015). What has serotonin to do with depression? World Psychiatry 14 (2), 158–160. doi:10.1002/wps.20229
Crescitelli, R., Lässer, C., and Lötvall, J. (2021). Isolation and characterization of extracellular vesicle subpopulations from tissues. Nat. Protoc. 16 (3), 1548–1580. doi:10.1038/s41596-020-00466-1
Cumba Garcia, L. M., Peterson, T. E., Cepeda, M. A., Johnson, A. J., and Parney, I. F. (2019). Isolation and analysis of plasma-derived exosomes in patients with glioma. Front. Oncol. 9, 651. doi:10.3389/fonc.2019.00651
Cvjetkovic, A., Lötvall, J., and Lässer, C. (2014). The influence of rotor type and centrifugation time on the yield and purity of extracellular vesicles. J. Extracell. Vesicles 3, 23111. doi:10.3402/jev.v3.23111
D'Acunzo, P., Pérez-González, R., Kim, Y., Hargash, T., Miller, C., Alldred, M. J., et al. (2021). Mitovesicles are a novel population of extracellular vesicles of mitochondrial origin altered in Down syndrome. Sci. Adv. 7 (7), eabe5085. doi:10.1126/sciadv.abe5085
D'Acunzo, P., Ungania, J. M., Kim, Y., Barreto, B. R., DeRosa, S., Pawlik, M., et al. (2023). Cocaine perturbs mitovesicle biology in the brain. J. Extracell. Vesicles 12 (1), e12301. doi:10.1002/jev2.12301
D'Agostino, S., Salamone, M., Di Liegro, I., and Vittorelli, M. L. (2006). Membrane vesicles shed by oligodendroglioma cells induce neuronal apoptosis. Int. J. Oncol. 29 (5), 1075–1085.
Dagur, R. S., Liao, K., Sil, S., Niu, F., Sun, Z., Lyubchenko, Y. L., et al. (2020). Neuronal-derived extracellular vesicles are enriched in the brain and serum of HIV-1 transgenic rats. J. Extracell. Vesicles 9 (1), 1703249. doi:10.1080/20013078.2019.1703249
Dalton, A. J. (1975). Microvesicles and vesicles of multivesicular bodies versus "virus-like" particles. J. Natl. Cancer Inst. 54 (5), 1137–1148. doi:10.1093/jnci/54.5.1137
Delpech, J. C., Herron, S., Botros, M. B., and Ikezu, T. (2019). Neuroimmune Crosstalk through extracellular vesicles in health and disease. Trends Neurosci. 42 (5), 361–372. doi:10.1016/j.tins.2019.02.007
DeRita, R. M., Zerlanko, B., Singh, A., Lu, H., Iozzo, R. V., Benovic, J. L., et al. (2017). c-Src, insulin-like growth factor I receptor, G-protein-coupled receptor kinases and focal adhesion kinase are enriched into prostate cancer cell exosomes. J. Cell Biochem. 118 (1), 66–73. doi:10.1002/jcb.25611
Deschwanden, A., Karolewicz, B., Feyissa, A. M., Treyer, V., Ametamey, S. M., Johayem, A., et al. (2011). Reduced metabotropic glutamate receptor 5 density in major depression determined by [(11)C]ABP688 PET and postmortem study. Am. J. Psychiatry 168 (7), 727–734. doi:10.1176/appi.ajp.2011.09111607
Di Vizio, D., Morello, M., Dudley, A. C., Schow, P. W., Adam, R. M., Morley, S., et al. (2012). Large oncosomes in human prostate cancer tissues and in the circulation of mice with metastatic disease. Am. J. Pathol. 181 (5), 1573–1584. doi:10.1016/j.ajpath.2012.07.030
Dickens, A. M., Tovar, Y. R. L. B., Yoo, S. W., Trout, A. L., Bae, M., Kanmogne, M., et al. (2017). Astrocyte-shed extracellular vesicles regulate the peripheral leukocyte response to inflammatory brain lesions. Sci. Signal 10 (473), eaai7696. doi:10.1126/scisignal.aai7696
Didiot, M. C., Hall, L. M., Coles, A. H., Haraszti, R. A., Godinho, B. M., Chase, K., et al. (2016). Exosome-mediated delivery of hydrophobically modified siRNA for Huntingtin mRNA silencing. Mol. Ther. 24 (10), 1836–1847. doi:10.1038/mt.2016.126
Doeppner, T. R., Herz, J., Görgens, A., Schlechter, J., Ludwig, A. K., Radtke, S., et al. (2015). Extracellular vesicles improve post-stroke neuroregeneration and prevent postischemic immunosuppression. Stem Cells Transl. Med. 4 (10), 1131–1143. doi:10.5966/sctm.2015-0078
Doeuvre, L., Plawinski, L., Toti, F., and Anglés-Cano, E. (2009). Cell-derived microparticles: A new challenge in neuroscience. J. Neurochem. 110 (2), 457–468. doi:10.1111/j.1471-4159.2009.06163.x
Dogra, N., Ahsen, M. E., Kozlova, E. G., Chen, T., Allette, K., Olsen, R., et al. (2020). exRNA signatures in extracellular vesicles and their tumor-lineage from prostate cancer. MedRxiv.
Dogra, N., Ledesma-Feliciano, C., and Sen, R. (2021). Developmental aspects of SARS-CoV-2, potential role of exosomes and their impact on the human transcriptome. J. Dev. Biol. 9 (4), 54. doi:10.3390/jdb9040054
Dome, P., Rihmer, Z., and Gonda, X. (2019). Suicide risk in bipolar disorder: A brief review. Med. Kaunas. 55 (8), 403. doi:10.3390/medicina55080403
Doncheck, E. M., Liddiard, G. T., Konrath, C. D., Liu, X., Yu, L., Urbanik, L. A., et al. (2020). Sex, stress, and prefrontal cortex: Influence of biological sex on stress-promoted cocaine seeking. Neuropsychopharmacology 45 (12), 1974–1985. doi:10.1038/s41386-020-0674-3
Doyle, L. M., and Wang, M. Z. (2019). Overview of extracellular vesicles, their origin, composition, purpose, and methods for exosome isolation and analysis. Cells 8 (7), 727. doi:10.3390/cells8070727
Du, Y., Chen, L., Li, X. S., Li, X. L., Xu, X. D., Tai, S. B., et al. (2021). Metabolomic identification of exosome-derived biomarkers for schizophrenia: A large multicenter study. Schizophr. Bull. 47 (3), 615–623. doi:10.1093/schbul/sbaa166
Du, Y., Dong, J. H., Chen, L., Liu, H., Zheng, G. E., Chen, G. Y., et al. (2022). Metabolomic identification of serum exosome-derived biomarkers for bipolar disorder. Oxid. Med. Cell Longev. 2022, 5717445. doi:10.1155/2022/5717445
Du, Y., Yu, Y., Hu, Y., Li, X. W., Wei, Z. X., Pan, R. Y., et al. (2019). Genome-wide, integrative analysis implicates exosome-derived MicroRNA dysregulation in schizophrenia. Schizophr. Bull. 45 (6), 1257–1266. doi:10.1093/schbul/sby191
Ebrahimkhani, S., Vafaee, F., Hallal, S., Wei, H., Lee, M. Y. T., Young, P. E., et al. (2018). Deep sequencing of circulating exosomal microRNA allows non-invasive glioblastoma diagnosis. NPJ Precis. Oncol. 2, 28. doi:10.1038/s41698-018-0071-0
El Andaloussi, S., Mäger, I., Breakefield, X. O., and Wood, M. J. A. (2013). Extracellular vesicles: Biology and emerging therapeutic opportunities. Nat. Rev. Drug Discov. 12 (5), 347–357. doi:10.1038/nrd3978
Elmore, Z. C., Patrick Havlik, L., Oh, D. K., Anderson, L., Daaboul, G., Devlin, G. W., et al. (2021). The membrane associated accessory protein is an adeno-associated viral egress factor. Nat. Commun. 12 (1), 6239. doi:10.1038/s41467-021-26485-4
Elsharkasy, O. M., Nordin, J. Z., Hagey, D. W., de Jong, O. G., Schiffelers, R. M., et al. (2020). Extracellular vesicles as drug delivery systems: Why and how? Adv. Drug Deliv. Rev. 159, 332–343. doi:10.1016/j.addr.2020.04.004
Escude Martinez de Castilla, P., Tong, L., Huang, C., Sofias, A. M., Pastorin, G., Chen, X., et al. (2021). Extracellular vesicles as a drug delivery system: A systematic review of preclinical studies. Adv. Drug Deliv. Rev. 175, 113801. doi:10.1016/j.addr.2021.05.011
Fan, Y., Chen, Z., and Zhang, M. (2022). Role of exosomes in the pathogenesis, diagnosis, and treatment of central nervous system diseases. J. Transl. Med. 20 (1), 291. doi:10.1186/s12967-022-03493-6
Faure, J., Lachenal, G., Court, M., Hirrlinger, J., Chatellard-Causse, C., Blot, B., et al. (2006). Exosomes are released by cultured cortical neurones. Mol. Cell Neurosci. 31 (4), 642–648. doi:10.1016/j.mcn.2005.12.003
Feltenstein, M. W., and See, R. E. (2008). The neurocircuitry of addiction: An overview. Br. J. Pharmacol. 154 (2), 261–274. doi:10.1038/bjp.2008.51
Ferrari, A. J., Somerville, A. J., Baxter, A. J., Norman, R., Patten, S. B., Vos, T., et al. (2013). Global variation in the prevalence and incidence of major depressive disorder: A systematic review of the epidemiological literature. Psychol. Med. 43 (3), 471–481. doi:10.1017/S0033291712001511
Fevrier, B., Vilette, D., Archer, F., Loew, D., Faigle, W., Vidal, M., et al. (2004). Cells release prions in association with exosomes. Proc. Natl. Acad. Sci. U. S. A. 101 (26), 9683–9688. doi:10.1073/pnas.0308413101
Franzen, J. M., Vanz, F., Werle, I., Guimaraes, F. S., and Bertoglio, L. J. (2022). Cannabidiol impairs fear memory reconsolidation in female rats through dorsal hippocampus CB1 but not CB2 receptor interaction. Eur. Neuropsychopharmacol. 64, 7–18. doi:10.1016/j.euroneuro.2022.08.002
Fruhbeis, C., Frohlich, D., and Kramer-Albers, E. M. (2012). Emerging roles of exosomes in neuron-glia communication. Front. Physiol. 3, 119. doi:10.3389/fphys.2012.00119
Frühbeis, C., Fröhlich, D., Kuo, W. P., Amphornrat, J., Thilemann, S., Saab, A. S., et al. (2013). Neurotransmitter-triggered transfer of exosomes mediates oligodendrocyte-neuron communication. PLoS Biol. 11 (7), e1001604. doi:10.1371/journal.pbio.1001604
Frye, M. A., Tsai, G. E., Huggins, T., Coyle, J. T., and Post, R. M. (2007). Low cerebrospinal fluid glutamate and glycine in refractory affective disorder. Biol. Psychiatry 61 (2), 162–166. doi:10.1016/j.biopsych.2006.01.024
Gabrielli, M., Battista, N., Riganti, L., Prada, I., Antonucci, F., Cantone, L., et al. (2015). Active endocannabinoids are secreted on the surface of microglial microvesicles. Springerplus 4 (1), L29. doi:10.1186/2193-1801-4-S1-L29
Gabrielli, M., Battista, N., Riganti, L., Prada, I., Antonucci, F., Cantone, L., et al. (2015). Active endocannabinoids are secreted on extracellular membrane vesicles. EMBO Rep. 16 (2), 213–220. doi:10.15252/embr.201439668
Gaglani, S., Gonzalez-Kozlova, E., Lundon, D. J., Tewari, A. K., Dogra, N., and Kyprianou, N. (2021). Exosomes as A Next-Generation diagnostic and therapeutic tool in prostate cancer. Int. J. Mol. Sci. 22 (18), 10131. doi:10.3390/ijms221810131
Gámez-Valero, A., Monguió-Tortajada, M., Carreras-Planella, L., Franquesa, M. l., Beyer, K., and Borràs, F. E. (2016). Size-Exclusion Chromatography-based isolation minimally alters Extracellular Vesicles’ characteristics compared to precipitating agents. Sci. Rep. 6 (1), 33641. doi:10.1038/srep33641
Gardiner, C., Di Vizio, D., Sahoo, S., Théry, C., Witwer, K. W., Wauben, M., et al. (2016). Techniques used for the isolation and characterization of extracellular vesicles: Results of a worldwide survey. J. Extracell. Vesicles 5, 32945. doi:10.3402/jev.v5.32945
Garzetti, L., Menon, R., Finardi, A., Bergami, A., Sica, A., Martino, G., et al. (2014). Activated macrophages release microvesicles containing polarized M1 or M2 mRNAs. J. Leukoc. Biol. 95 (5), 817–825. doi:10.1189/jlb.0913485
Geiser, T., Sturzenegger, M., Genewein, U., Haeberli, A., and Beer, J. H. (1998). Mechanisms of cerebrovascular events as assessed by procoagulant activity, cerebral microemboli, and platelet microparticles in patients with prosthetic heart valves. Stroke 29 (9), 1770–1777. doi:10.1161/01.str.29.9.1770
Gharbi, T., Zhang, Z., and Yang, G. Y. (2020). The function of astrocyte mediated extracellular vesicles in central nervous system diseases. Front. Cell Dev. Biol. 8, 568889. doi:10.3389/fcell.2020.568889
Ghosh, S., Dellibovi-Ragheb, T. A., Kerviel, A., Pak, E., Qiu, Q., Fisher, M., et al. (2020). β-Coronaviruses use lysosomes for egress Instead of the biosynthetic secretory pathway. Cell 183 (6), 1520–1535. doi:10.1016/j.cell.2020.10.039
Ghossoub, R., Lembo, F., Rubio, A., Gaillard, C. B., Bouchet, J., Vitale, N., et al. (2014). Syntenin-ALIX exosome biogenesis and budding into multivesicular bodies are controlled by ARF6 and PLD2. Nat. Commun. 5, 3477. doi:10.1038/ncomms4477
Gitlin, M. J. (2019). Antidepressants in bipolar depression: An enduring controversy. Focus (Am Psychiatr. Publ. 17 (3), 278–283. doi:10.1176/appi.focus.17306
Glebov, K., Löchner, M., Jabs, R., Lau, T., Merkel, O., Schloss, P., et al. (2015). Serotonin stimulates secretion of exosomes from microglia cells. GLIA 63 (4), 626–634. doi:10.1002/glia.22772
Goetzl, E. J., Abner, E. L., Jicha, G. A., Kapogiannis, D., and Schwartz, J. B. (2018). Declining levels of functionally specialized synaptic proteins in plasma neuronal exosomes with progression of Alzheimer's disease. FASEB J. 32 (2), 888–893. doi:10.1096/fj.201700731R
Gomes, P., Tzouanou, F., Skolariki, K., Vamvaka-Iakovou, A., Noguera-Ortiz, C., Tsirtsaki, K., et al. (2022). Extracellular vesicles and Alzheimer's disease in the novel era of precision medicine: Implications for disease progression, diagnosis and treatment. Exp. Neurol. 358, 114183. doi:10.1016/j.expneurol.2022.114183
Gratpain, V., Mwema, A., Labrak, Y., Muccioli, G. G., van Pesch, V., and des Rieux, A. (2021). Extracellular vesicles for the treatment of central nervous system diseases. Adv. Drug Deliv. Rev. 174, 535–552. doi:10.1016/j.addr.2021.05.006
Graziani, V., Rodriguez-Hernandez, I., Maiques, O., and Sanz-Moreno, V. (2022). The amoeboid state as part of the epithelial-to-mesenchymal transition programme. Trends Cell Biol. 32 (3), 228–242. doi:10.1016/j.tcb.2021.10.004
Graziano, F., Iacopino, D. G., Cammarata, G., Scalia, G., Campanella, C., Giannone, A. G., et al. (2021). The triad hsp60-miRNAs-extracellular vesicles in brain tumors: Assessing its components for understanding tumorigenesis and monitoring patients. Appl. Sci. 11 (6), 2867. doi:10.3390/app11062867
Greening, D. W., Xu, R., Gopal, S. K., Rai, A., and Simpson, R. J. (2017). Proteomic insights into extracellular vesicle biology - defining exosomes and shed microvesicles. Expert Rev. Proteomics 14 (1), 69–95. doi:10.1080/14789450.2017.1260450
Grillo, C. A., Piroli, G. G., Kaigler, K. F., Wilson, S. P., Wilson, M. A., and Reagan, L. P. (2011). Downregulation of hypothalamic insulin receptor expression elicits depressive-like behaviors in rats. Behav. Brain Res. 222 (1), 230–235. doi:10.1016/j.bbr.2011.03.052
Guha, D., Lorenz, D. R., Misra, V., Chettimada, S., Morgello, S., and Gabuzda, D. (2019). Proteomic analysis of cerebrospinal fluid extracellular vesicles reveals synaptic injury, inflammation, and stress response markers in HIV patients with cognitive impairment. J. Neuroinflammation 16 (1), 254. doi:10.1186/s12974-019-1617-y
Guha, D., Mukerji, S. S., Chettimada, S., Misra, V., Lorenz, D. R., Morgello, S., et al. (2019). Cerebrospinal fluid extracellular vesicles and neurofilament light protein as biomarkers of central nervous system injury in HIV-infected patients on antiretroviral therapy. AIDS 33 (4), 615–625. doi:10.1097/QAD.0000000000002121
Guitart, K., Loers, G., Buck, F., Bork, U., Schachner, M., and Kleene, R. (2016). Improvement of neuronal cell survival by astrocyte-derived exosomes under hypoxic and ischemic conditions depends on prion protein. Glia 64 (6), 896–910. doi:10.1002/glia.22963
Guix, F. X., Corbett, G. T., Cha, D. J., Mustapic, M., Liu, W., Mengel, D., et al. (2018). Detection of aggregation-competent tau in neuron-derived extracellular vesicles. Int. J. Mol. Sci. 19 (3), 663. doi:10.3390/ijms19030663
Guo, S., Perets, N., Betzer, O., Ben-Shaul, S., Sheinin, A., Michaelevski, I., et al. (2019). Intranasal delivery of mesenchymal stem cell derived exosomes loaded with phosphatase and tensin homolog siRNA repairs complete spinal cord injury. ACS Nano 13 (9), 10015–10028. doi:10.1021/acsnano.9b01892
Hage, D. S., Thomas, D. H., and Beck, M. S. (1993). Theory of a sequential addition competitive binding immunoassay based on high-performance immunoaffinity chromatography. Anal. Chem. 65 (11), 1622–1630. doi:10.1021/ac00059a023
Hallal, S., Ebrahim Khani, S., Wei, H., Lee, M. Y. T., Sim, H. W., Sy, J., et al. (2020). Deep sequencing of small RNAs from neurosurgical extracellular vesicles substantiates miR-486-3p as a circulating biomarker that distinguishes glioblastoma from lower-grade astrocytoma patients. Int. J. Mol. Sci. 21 (14), 4954. doi:10.3390/ijms21144954
Hallal, S., Ebrahimkhani, S., Shivalingam, B., Graeber, M. B., Kaufman, K. L., and Buckland, M. E. (2019). The emerging clinical potential of circulating extracellular vesicles for non-invasive glioma diagnosis and disease monitoring. Brain Tumor Pathol. 36 (2), 29–39. doi:10.1007/s10014-019-00335-0
Hamer, J. A., Testani, D., Mansur, R. B., Lee, Y., Subramaniapillai, M., and McIntyre, R. S. (2019). Brain insulin resistance: A treatment target for cognitive impairment and anhedonia in depression. Exp. Neurol. 315, 1–8. doi:10.1016/j.expneurol.2019.01.016
Hammarström, B., Skov, N. R., Olofsson, K., Bruus, H., and Wiklund, M. (2021). Acoustic trapping based on surface displacement of resonance modes. J. Acoust. Soc. Am. 149 (3), 1445. doi:10.1121/10.0003600
Hayakawa, K., Esposito, E., Wang, X., Terasaki, Y., Liu, Y., Xing, C., et al. (2016). Transfer of mitochondria from astrocytes to neurons after stroke. Nature 535 (7613), 551–555. doi:10.1038/nature18928
Heath, N., Grant, L., De Oliveira, T. M., Rowlinson, R., Osteikoetxea, X., Dekker, N., et al. (2018). Rapid isolation and enrichment of extracellular vesicle preparations using anion exchange chromatography. Sci. Rep. 8 (1), 5730. doi:10.1038/s41598-018-24163-y
Herrmann, I. K., Wood, M. J. A., and Fuhrmann, G. (2021). Extracellular vesicles as a next-generation drug delivery platform. Nat. Nanotechnol. 16 (7), 748–759. doi:10.1038/s41565-021-00931-2
Heyn, J., Heuschkel, M. A., and Goettsch, C. (2023). Mitochondrial-derived vesicles-link to extracellular vesicles and implications in cardiovascular disease. Int. J. Mol. Sci. 24 (3), 2637. doi:10.3390/ijms24032637
Holm, M. M., Kaiser, J., and Schwab, M. E. (2018). Extracellular vesicles: Multimodal envoys in neural maintenance and repair. Trends Neurosci. 41 (6), 360–372. doi:10.1016/j.tins.2018.03.006
Hornung, S., Dutta, S., and Bitan, G. (2020). CNS-derived blood exosomes as a promising source of biomarkers: Opportunities and challenges. Front. Mol. Neurosci. 13, 38. doi:10.3389/fnmol.2020.00038
Horstman, L. L., Jy, W., Minagar, A., Bidot, C. J., Jimenez, J. J., Alexander, J. S., et al. (2007). Cell-derived microparticles and exosomes in neuroinflammatory disorders. Int. Rev. Neurobiol. 79, 227–268. doi:10.1016/S0074-7742(07)79010-4
Hou, Y., Dan, X., Babbar, M., Wei, Y., Hasselbalch, S. G., Croteau, D. L., et al. (2019). Ageing as a risk factor for neurodegenerative disease. Nat. Rev. Neurol. 15 (10), 565–581. doi:10.1038/s41582-019-0244-7
Howard, R., and Liu, K. Y. (2020). Questions EMERGE as Biogen claims aducanumab turnaround. Nat. Rev. Neurol. 16 (2), 63–64. doi:10.1038/s41582-019-0295-9
Howes, O. D., Thase, M. E., and Pillinger, T. (2022). Treatment resistance in psychiatry: State of the art and new directions. Mol. Psychiatry 27 (1), 58–72. doi:10.1038/s41380-021-01200-3
Hu, G., Niu, F., Liao, K., Periyasamy, P., Sil, S., Liu, J., et al. (2020). HIV-1 tat-induced astrocytic extracellular vesicle miR-7 impairs synaptic architecture. J. Neuroimmune Pharmacol. 15 (3), 538–553. doi:10.1007/s11481-019-09869-8
Hu, G., Yang, L., Cai, Y., Niu, F., Mezzacappa, F., Callen, S., et al. (2016). Emerging roles of extracellular vesicles in neurodegenerative disorders: Focus on HIV-associated neurological complications. Cell Death Dis. 7 (11), e2481. doi:10.1038/cddis.2016.336
Huang, X., Ketova, T., Fleming, J. T., Wang, H., Dey, S. K., Litingtung, Y., et al. (2009). Sonic hedgehog signaling regulates a novel epithelial progenitor domain of the hindbrain choroid plexus. Development 136 (15), 2535–2543. doi:10.1242/dev.033795
Huang, Y. (2021). Extracellular vesicles and ciliogenesis as novel targets to stop opioid tolerance. Biol. Psychiatry 90 (8), e39–e40. doi:10.1016/j.biopsych.2021.08.007
Huggins, D. J., Sherman, W., and Tidor, B. (2012). Rational approaches to improving selectivity in drug design. J. Med. Chem. 55 (4), 1424–1444. doi:10.1021/jm2010332
Ishibashi, S., Brown, M. S., Goldstein, J. L., Gerard, R. D., Hammer, R. E., and Herz, J. (1993). Hypercholesterolemia in low density lipoprotein receptor knockout mice and its reversal by adenovirus-mediated gene delivery. J. Clin. Invest 92 (2), 883–893. doi:10.1172/JCI116663
Jarvis, R., Tamashiro-Orrego, A., Promes, V., Tu, L., Shi, J., and Yang, Y. (2020). Cocaine self-administration and extinction inversely alter neuron to glia exosomal dynamics in the nucleus accumbens. Front. Cell. Neurosci. 13, 581. doi:10.3389/fncel.2019.00581
Jeppesen, D. K., Fenix, A. M., Franklin, J. L., Higginbotham, J. N., Zhang, Q., Zimmerman, L. J., et al. (2019). Reassessment of exosome composition. Cell 177 (2), 428–445. doi:10.1016/j.cell.2019.02.029
Jia, G., Han, Y., An, Y., Ding, Y., He, C., Wang, X., et al. (2018). NRP-1 targeted and cargo-loaded exosomes facilitate simultaneous imaging and therapy of glioma in vitro and in vivo. Biomaterials 178, 302–316. doi:10.1016/j.biomaterials.2018.06.029
Jia, Y. F., Wininger, K., Ho, A. M., Peyton, L., Baker, M., and Choi, D. S. (2020). Astrocytic glutamate transporter 1 (GLT1) deficiency reduces anxiety- and depression-like behaviors in mice. Front. Behav. Neurosci. 14, 57. doi:10.3389/fnbeh.2020.00057
Jia, Y., Yu, L., Ma, T., Xu, W., Qian, H., Sun, Y., et al. (2022). Small extracellular vesicles isolation and separation: Current techniques, pending questions and clinical applications. Theranostics 12 (15), 6548–6575. doi:10.7150/thno.74305
Joshi, P., Turola, E., Ruiz, A., Bergami, A., Libera, D. D., Benussi, L., et al. (2014). Microglia convert aggregated amyloid-β into neurotoxic forms through the shedding of microvesicles. Cell Death Differ. 21 (4), 582–593. doi:10.1038/cdd.2013.180
Jovicic, A., and Gitler, A. D. (2017). Distinct repertoires of microRNAs present in mouse astrocytes compared to astrocyte-secreted exosomes. PLoS One 12 (2), e0171418. doi:10.1371/journal.pone.0171418
Kahlert, C., and Kalluri, R. (2013). Exosomes in tumor microenvironment influence cancer progression and metastasis. J. Mol. Med. Berl. 91 (4), 431–437. doi:10.1007/s00109-013-1020-6
Kakarla, R., Hur, J., Kim, Y. J., Kim, J., and Chwae, Y. J. (2020). Apoptotic cell-derived exosomes: Messages from dying cells. Exp. Mol. Med. 52, 1–6. doi:10.1038/s12276-019-0362-8
Kalluri, R., and LeBleu, V. S. (2020). The biology, function, and biomedical applications of exosomes. Science 367 (6478), eaau6977. doi:10.1126/science.aau6977
Kannan, M., Singh, S., Chemparathy, D. T., Oladapo, A. A., Gawande, D. Y., Dravid, S. M., et al. (2022). HIV-1 Tat induced microglial EVs leads to neuronal synaptodendritic injury: Microglia-neuron cross-talk in neuroHIV. Extracell. Vesicles Circulating Nucleic Acids 3 (2), 133–149. doi:10.20517/evcna.2022.14
Kapogiannis, D., Boxer, A., Schwartz, J. B., Abner, E. L., Biragyn, A., Masharani, U., et al. (2015). Dysfunctionally phosphorylated type 1 insulin receptor substrate in neural-derived blood exosomes of preclinical Alzheimer's disease. FASEB J. 29 (2), 589–596. doi:10.1096/fj.14-262048
Kapogiannis, D., Dobrowolny, H., Tran, J., Mustapic, M., Frodl, T., Meyer-Lotz, G., et al. (2019). Insulin-signaling abnormalities in drug-naïve first-episode schizophrenia: Transduction protein analyses in extracellular vesicles of putative neuronal origin. Eur. Psychiatry 62, 124–129. doi:10.1016/j.eurpsy.2019.08.012
Kapogiannis, D., Mustapic, M., Shardell, M. D., Berkowitz, S. T., Diehl, T. C., Spangler, R. D., et al. (2019). Association of extracellular vesicle biomarkers with alzheimer disease in the baltimore longitudinal study of aging. JAMA Neurol. 76 (11), 1340–1351. doi:10.1001/jamaneurol.2019.2462
Keerthikumar, S., Gangoda, L., Liem, M., Fonseka, P., Atukorala, I., Ozcitti, C., et al. (2015). Proteogenomic analysis reveals exosomes are more oncogenic than ectosomes. Oncotarget 6 (17), 15375–15396. doi:10.18632/oncotarget.3801
Keller, S., Ridinger, J., Rupp, A. K., Janssen, J. W., and Altevogt, P. (2011). Body fluid derived exosomes as a novel template for clinical diagnostics. J. Transl. Med. 9, 86. doi:10.1186/1479-5876-9-86
Kendall, D. A., and Yudowski, G. A. (2016). Cannabinoid receptors in the central nervous system: Their signaling and roles in disease. Front. Cell Neurosci. 10, 294. doi:10.3389/fncel.2016.00294
Kenny, P., Caligiuri, S., Bali, P., Smith, A., Chen, Z., Voren, G., et al. (2022). Choroid plexus regulation of nicotine reward and aversion. Biol. Psychiatry 91 (9), S14. doi:10.1016/j.biopsych.2022.02.054
Kerr, J. F., Wyllie, A. H., and Currie, A. R. (1972). Apoptosis: A basic biological phenomenon with wide-ranging implications in tissue kinetics. Br. J. Cancer 26 (4), 239–257. doi:10.1038/bjc.1972.33
Kettenmann, H., Hanisch, U. K., Noda, M., and Verkhratsky, A. (2011). Physiology of microglia. Physiol. Rev. 91 (2), 461–553. doi:10.1152/physrev.00011.2010
Kim, K. M., Meng, Q., Perez de Acha, O., Mustapic, M., Cheng, A., Eren, E., et al. (2020). Mitochondrial RNA in Alzheimer's disease circulating extracellular vesicles. Front. Cell Dev. Biol. 8, 581882. doi:10.3389/fcell.2020.581882
Kim, K., Park, J., Jung, J. H., Lee, R., Park, J. H., Yuk, J. M., et al. (2021). Cyclic tangential flow filtration system for isolation of extracellular vesicles. Apl. Bioeng. 5 (1), 016103. doi:10.1063/5.0037768
Kim, S. C., Wunsch, B. H., Hu, H., Smith, J. T., Austin, R. H., and Stolovitzky, G. (2017). Broken flow symmetry explains the dynamics of small particles in deterministic lateral displacement arrays. Proc. Natl. Acad. Sci. U. S. A. 114 (26), E5034–e5041. doi:10.1073/pnas.1706645114
Kolios, G., and Moodley, Y. (2013). Introduction to stem cells and regenerative medicine. Respiration 85 (1), 3–10. doi:10.1159/000345615
Konoshenko, M. Y., Lekchnov, E. A., Vlassov, A. V., and Laktionov, P. P. (2018). Isolation of extracellular vesicles: General methodologies and latest trends. Biomed. Res. Int. 2018, 8545347. doi:10.1155/2018/8545347
Koob, G. F., and Volkow, N. D. (2016). Neurobiology of addiction: A neurocircuitry analysis. Lancet Psychiatry 3 (8), 760–773. doi:10.1016/S2215-0366(16)00104-8
Kovacs, G. G., Horvath, M. C., Majtenyi, K., Lutz, M. I., Hurd, Y. L., and Keller, E. (2015). Heroin abuse exaggerates age-related deposition of hyperphosphorylated tau and p62-positive inclusions. Neurobiol. Aging 36 (11), 3100–3107. doi:10.1016/j.neurobiolaging.2015.07.018
Kowal, J., Arras, G., Colombo, M., Jouve, M., Morath, J. P., Primdal-Bengtson, B., et al. (2016). Proteomic comparison defines novel markers to characterize heterogeneous populations of extracellular vesicle subtypes. Proc. Natl. Acad. Sci. U. S. A. 113 (8), E968–E977. doi:10.1073/pnas.1521230113
Kowal, J., Tkach, M., and Théry, C. (2014). Biogenesis and secretion of exosomes. Curr. Opin. Cell Biol. 29, 116–125. doi:10.1016/j.ceb.2014.05.004
Krämer-Albers, E. M. (2020). Extracellular vesicles in the oligodendrocyte microenvironment. Neurosci. Lett. 725, 134915. doi:10.1016/j.neulet.2020.134915
Kreger, B. T., Dougherty, A. L., Greene, K. S., Cerione, R. A., and Antonyak, M. A. (2016). Microvesicle cargo and function changes upon induction of cellular transformation. J. Biol. Chem. 291 (38), 19774–19785. doi:10.1074/jbc.M116.725705
Ku, A., Lim, H. C., Evander, M., Lilja, H., Laurell, T., Scheding, S., et al. (2018). Acoustic enrichment of extracellular vesicles from biological fluids. Anal. Chem. 90 (13), 8011–8019. doi:10.1021/acs.analchem.8b00914
Ku, A., Ravi, N., Yang, M., Evander, M., Laurell, T., Lilja, H., et al. (2019). A urinary extracellular vesicle microRNA biomarker discovery pipeline; from automated extracellular vesicle enrichment by acoustic trapping to microRNA sequencing. PLoS One 14 (5), e0217507. doi:10.1371/journal.pone.0217507
Kumar, A., Kim, S., Su, Y., Sharma, M., Kumar, P., Singh, S., et al. (2021). Brain cell-derived exosomes in plasma serve as neurodegeneration biomarkers in male cynomolgus monkeys self-administrating oxycodone. EBioMedicine 63, 103192. doi:10.1016/j.ebiom.2020.103192
Kunadt, M., Eckermann, K., Stuendl, A., Gong, J., Russo, B., Strauss, K., et al. (2015). Extracellular vesicle sorting of α-Synuclein is regulated by sumoylation. Acta Neuropathol. 129 (5), 695–713. doi:10.1007/s00401-015-1408-1
Kutchy, N. A., Peeples, E. S., Sil, S., Liao, K., Chivero, E. T., Hu, G., et al. (2020). Extracellular vesicles in viral infections of the nervous system. Viruses 12, 700. doi:10.3390/v12070700
Lachenal, G., Pernet-Gallay, K., Chivet, M., Hemming, F. J., Belly, A., Bodon, G., et al. (2011). Release of exosomes from differentiated neurons and its regulation by synaptic glutamatergic activity. Mol. Cell. Neurosci. 46 (2), 409–418. doi:10.1016/j.mcn.2010.11.004
Lai, C. P., and Breakefield, X. O. (2012). Role of exosomes/microvesicles in the nervous system and use in emerging therapies. Front. Physiol. 3, 228. doi:10.3389/fphys.2012.00228
Lallai, V., Grimes, N., Fowler, J. P., Sequeira, P. A., Cartagena, P., Limon, A., et al. (2019). Nicotine acts on cholinergic signaling mechanisms to directly modulate choroid plexus function. eNeuro 6 (2). doi:10.1523/ENEURO.0051-19.2019
Lässer, C., Alikhani, V. S., Ekström, K., Eldh, M., Paredes, P. T., Bossios, A., et al. (2011). Human saliva, plasma and breast milk exosomes contain RNA: Uptake by macrophages. J. Transl. Med. 9, 9. doi:10.1186/1479-5876-9-9
Lazo, S., Noren Hooten, N., Green, J., Eitan, E., Mode, N. A., Liu, Q. R., et al. (2021). Mitochondrial DNA in extracellular vesicles declines with age. Aging Cell 20 (1), e13283. doi:10.1111/acel.13283
Lee, Y. J., Jy, W., Horstman, L. L., Janania, J., Reyes, Y., Kelley, R. E., et al. (1993). Elevated platelet microparticles in transient ischemic attacks, lacunar infarcts, and multiinfarct dementias. Thrombosis Res. 72 (4), 295–304. doi:10.1016/0049-3848(93)90138-e
Lee, Y., Mansur, R. B., Brietzke, E., Kapogiannis, D., Delgado-Peraza, F., Boutilier, J. J., et al. (2021). Peripheral inflammatory biomarkers define biotypes of bipolar depression. Mol. Psychiatry 26 (7), 3395–3406. doi:10.1038/s41380-021-01051-y
Lee, Y., Son, H., Kim, G., Kim, S., Lee, D. H., Roh, G. S., et al. (2013). Glutamine deficiency in the prefrontal cortex increases depressive-like behaviours in male mice. J. Psychiatry Neurosci. 38 (3), 183–191. doi:10.1503/jpn.120024
Lener, M. S., Kadriu, B., and Zarate, C. A. (2017). Ketamine and beyond: Investigations into the potential of glutamatergic agents to treat depression. Drugs 77 (4), 381–401. doi:10.1007/s40265-017-0702-8
Li, B., Antonyak, M. A., Zhang, J., and Cerione, R. A. (2012). RhoA triggers a specific signaling pathway that generates transforming microvesicles in cancer cells. Oncogene 31 (45), 4740–4749. doi:10.1038/onc.2011.636
Li, D., Zhang, P., Yao, X., Li, H., Shen, H., Li, X., et al. (2018). Exosomes derived from miR-133b-modified mesenchymal stem cells promote recovery after spinal cord injury. Front. Neurosci. 12, 845. doi:10.3389/fnins.2018.00845
Li, H., Li, C., Zhou, Y., Luo, C., Ou, J., Li, J., et al. (2018). Expression of microRNAs in the serum exosomes of methamphetamine-dependent rats vs. ketamine-dependent rats. Exp. Ther. Med. 15 (4), 3369–3375. doi:10.3892/etm.2018.5814
Li, J. J., Wang, B., Kodali, M. C., Chen, C., Kim, E., Patters, B. J., et al. (2018). In vivo evidence for the contribution of peripheral circulating inflammatory exosomes to neuroinflammation. J. Neuroinflammation 15 (1), 8. doi:10.1186/s12974-017-1038-8
Li, L., Grausam, K. B., Wang, J., Lun, M. P., Ohli, J., Lidov, H. G., et al. (2016). Sonic Hedgehog promotes proliferation of Notch-dependent monociliated choroid plexus tumour cells. Nat. Cell Biol. 18 (4), 418–430. doi:10.1038/ncb3327
Li, T. R., Yao, Y. X., Jiang, X. Y., Dong, Q. Y., Yu, X. F., Wang, T., et al. (2022). β-Amyloid in blood neuronal-derived extracellular vesicles is elevated in cognitively normal adults at risk of Alzheimer's disease and predicts cerebral amyloidosis. Alzheimers Res. Ther. 14 (1), 66. doi:10.1186/s13195-022-01010-x
Liang, X., Zhang, L., Wang, S., Han, Q., and Zhao, R. C. (2016). Exosomes secreted by mesenchymal stem cells promote endothelial cell angiogenesis by transferring miR-125a. J. Cell Sci. 129 (11), 2182–2189. doi:10.1242/jcs.170373
Liangsupree, T., Multia, E., and Riekkola, M.-L. (2021). Modern isolation and separation techniques for extracellular vesicles. J. Chromatogr. A 1636, 461773. doi:10.1016/j.chroma.2020.461773
Liao, K., Niu, F., Dagur, R. S., He, M., Tian, C., and Hu, G. (2020). Intranasal delivery of lincRNA-cox2 siRNA loaded extracellular vesicles decreases lipopolysaccharide-induced microglial proliferation in mice. J. Neuroimmune Pharmacol. 15 (3), 390–399. doi:10.1007/s11481-019-09864-z
Liao, K., Niu, F., Hu, G., Yang, L., Dallon, B., Villarreal, D., et al. (2020). Morphine-mediated release of miR-138 in astrocyte-derived extracellular vesicles promotes microglial activation. J. Extracell. Vesicles 10 (1), e12027. doi:10.1002/jev2.12027
Lino, M. M., Simões, S., Tomatis, F., Albino, I., Barrera, A., Vivien, D., et al. (2021). Engineered extracellular vesicles as brain therapeutics. J. Control Release 338, 472–485. doi:10.1016/j.jconrel.2021.08.037
Liu, Y., Li, D., Liu, Z., Zhou, Y., Chu, D., Li, X., et al. (2015). Targeted exosome-mediated delivery of opioid receptor Mu siRNA for the treatment of morphine relapse. Sci. Rep. 5, 17543. doi:10.1038/srep17543
Lo, Y. M. (2009). Noninvasive prenatal detection of fetal chromosomal aneuploidies by maternal plasma nucleic acid analysis: A review of the current state of the art. BJOG 116 (2), 152–157. doi:10.1111/j.1471-0528.2008.02010.x
Lopez, J. P., Fiori, L. M., Cruceanu, C., Lin, R., Labonte, B., Cates, H. M., et al. (2017). MicroRNAs 146a/b-5 and 425-3p and 24-3p are markers of antidepressant response and regulate MAPK/Wnt-system genes. Nat. Commun. 8, 15497. doi:10.1038/ncomms15497
Lotvall, J., Hill, A. F., Hochberg, F., Buzas, E. I., Di Vizio, D., Gardiner, C., et al. (2014). Minimal experimental requirements for definition of extracellular vesicles and their functions: A position statement from the international society for extracellular vesicles. J. Extracell. Vesicles 3, 26913. doi:10.3402/jev.v3.26913
Luxmi, R., and King, S. M. (2022). Cilia-derived vesicles: An ancient route for intercellular communication. Semin. Cell Dev. Biol. 129, 82–92. doi:10.1016/j.semcdb.2022.03.014
Ma, R., Kutchy, N. A., and Hu, G. (2021). Astrocyte-derived extracellular vesicle–mediated activation of primary ciliary signaling contributes to the development of morphine tolerance. Biol. Psychiatry 90 (8), 575–585. doi:10.1016/j.biopsych.2021.06.009
Mackie, K. (2006). Mechanisms of CB1 receptor signaling: Endocannabinoid modulation of synaptic strength. Int. J. Obes. 30, S19–S23. doi:10.1038/sj.ijo.0803273
Madison, R. D., and Robinson, G. A. (2019). Muscle-derived extracellular vesicles influence motor neuron regeneration accuracy. Neuroscience 419, 46–59. doi:10.1016/j.neuroscience.2019.08.028
Mahmoud, S., Gharagozloo, M., Simard, C., and Gris, D. (2019). Astrocytes maintain glutamate homeostasis in the CNS by controlling the balance between glutamate uptake and release. Cells 8 (2), 184. doi:10.3390/cells8020184
Mamdani, F., Weber, M. D., Bunney, B., Burke, K., Cartagena, P., Walsh, D., et al. (2022). Identification of potential blood biomarkers associated with suicide in major depressive disorder. Transl. Psychiatry 12 (1), 159. doi:10.1038/s41398-022-01918-w
Mankhong, S., Kim, S., Lee, S., Kwak, H. B., Park, D. H., Joa, K. L., et al. (2022). Development of Alzheimer's disease biomarkers: From CSF- to blood-based biomarkers. Biomedicines 10 (4), 850. doi:10.3390/biomedicines10040850
Mansur, R. B., Delgado-Peraza, F., Subramaniapillai, M., Lee, Y., Iacobucci, M., Nasri, F., et al. (2021). Exploring brain insulin resistance in adults with bipolar depression using extracellular vesicles of neuronal origin. J. Psychiatric Res. 133, 82–92. doi:10.1016/j.jpsychires.2020.12.007
Mansur, R. B., Delgado-Peraza, F., Subramaniapillai, M., Lee, Y., Iacobucci, M., Rodrigues, N., et al. (2020). Extracellular vesicle biomarkers reveal inhibition of neuroinflammation by infliximab in association with antidepressant response in adults with bipolar depression. Cells 9 (4), 895. doi:10.3390/cells9040895
Manzoni, O. J., and Bockaert, J. (2001). Cannabinoids inhibit GABAergic synaptic transmission in mice nucleus accumbens. Eur. J. Pharmacol. 412 (2), R3–R5. doi:10.1016/s0014-2999(01)00723-3
Marzesco, A. M., Janich, P., Wilsch-Bräuninger, M., Dubreuil, V., Langenfeld, K., Corbeil, D., et al. (2005). Release of extracellular membrane particles carrying the stem cell marker prominin-1 (CD133) from neural progenitors and other epithelial cells. J. Cell Sci. 118 (13), 2849–2858. doi:10.1242/jcs.02439
Mathieu, M., Martin-Jaular, L., Lavieu, G., and Théry, C. (2019). Specificities of secretion and uptake of exosomes and other extracellular vesicles for cell-to-cell communication. Nat. Cell Biol. 21 (1), 9–17. doi:10.1038/s41556-018-0250-9
Mathivanan, S., Lim, J. W., Tauro, B. J., Ji, H., Moritz, R. L., and Simpson, R. J. (2010). Proteomics analysis of A33 immunoaffinity-purified exosomes released from the human colon tumor cell line LIM1215 reveals a tissue-specific protein signature. Mol. Cell Proteomics 9 (2), 197–208. doi:10.1074/mcp.M900152-MCP200
McCammon, J. M., and Sive, H. (2015). Challenges in understanding psychiatric disorders and developing therapeutics: A role for zebrafish. Dis. Model Mech. 8 (7), 647–656. doi:10.1242/dmm.019620
McNamara, R. P., Caro-Vegas, C. P., Costantini, L. M., Landis, J. T., Griffith, J. D., Damania, B. A., et al. (2018). Large-scale, cross-flow based isolation of highly pure and endocytosis-competent extracellular vesicles. J. Extracell. Vesicles 7 (1), 1541396. doi:10.1080/20013078.2018.1541396
Meckes, D. G., Gunawardena, H. P., Dekroon, R. M., Heaton, P. R., Edwards, R. H., Ozgur, S., et al. (2013). Modulation of B-cell exosome proteins by gamma herpesvirus infection. Proc. Natl. Acad. Sci. U. S. A. 110 (31), E2925–E2933. doi:10.1073/pnas.1303906110
Miller, A. H., and Raison, C. L. (2023). Burning down the house: Reinventing drug discovery in psychiatry for the development of targeted therapies. Mol. Psychiatry 28 (1), 68–75. doi:10.1038/s41380-022-01887-y
Miller, C. E., Xu, F., Zhao, Y., Luo, W., Zhong, W., Meyer, K., et al. (2022). Hydrogen peroxide promotes the production of radiation-derived EVs containing mitochondrial proteins. Antioxidants (Basel) 11 (11), 2119. doi:10.3390/antiox11112119
Minagar, A., Jy, W., Jimenez, J. J., Sheremata, W. A., Mauro, L. M., Mao, W. W., et al. (2001). Elevated plasma endothelial microparticles in multiple sclerosis. Neurology 56 (10), 1319–1324. doi:10.1212/wnl.56.10.1319
Momen-Heravi, F., Balaj, L., Alian, S., Mantel, P. Y., Halleck, A. E., Trachtenberg, A. J., et al. (2013). Current methods for the isolation of extracellular vesicles. Biol. Chem. 394 (10), 1253–1262. doi:10.1515/hsz-2013-0141
Moncrieff, J., Gupta, S., and Horowitz, M. A. (2020). Barriers to stopping neuroleptic (antipsychotic) treatment in people with schizophrenia, psychosis or bipolar disorder. Ther. Adv. Psychopharmacol. 10, 2045125320937910–204512532093791. doi:10.1177/2045125320937910
Monteiro-Reis, S., Carvalho-Maia, C., Bart, G., Vainio, S. J., Pedro, J., Silva, E. R., et al. (2021). Secreted extracellular vesicle molecular cargo as a novel liquid biopsy diagnostics of central nervous system diseases. Int. J. Mol. Sci. MDPI AG 22, 3267. doi:10.3390/ijms22063267
Mulcahy, L. A., Pink, R. C., and Carter, D. R. (2014). Routes and mechanisms of extracellular vesicle uptake. J. Extracell. Vesicles 3, 24641. doi:10.3402/jev.v3.24641
Mullins, R. J., Mustapic, M., Goetzl, E. J., and Kapogiannis, D. (2017). Exosomal biomarkers of brain insulin resistance associated with regional atrophy in Alzheimer's disease. Hum. Brain Mapp. 38 (4), 1933–1940. doi:10.1002/hbm.23494
Muralidharan-Chari, V., Clancy, J., Plou, C., Romao, M., Chavrier, P., Raposo, G., et al. (2009). ARF6-regulated shedding of tumor cell-derived plasma membrane microvesicles. Curr. Biol. 19 (22), 1875–85. doi:10.1016/j.cub.2009.09.059
Muraoka, S., DeLeo, A. M., Sethi, M. K., Yukawa-Takamatsu, K., Yang, Z., Ko, J., et al. (2020). Proteomic and biological profiling of extracellular vesicles from Alzheimer's disease human brain tissues. Alzheimers Dement. 16 (6), 896–907. doi:10.1002/alz.12089
Murillo, O. D., Thistlethwaite, W., Rozowsky, J., Subramanian, S. L., Lucero, R., Shah, N., et al. (2019). exRNA atlas analysis reveals distinct extracellular RNA cargo types and their carriers present across human biofluids. Cell 177 (2), 463–477. doi:10.1016/j.cell.2019.02.018
Murphy-Royal, C., Dupuis, J., Groc, L., and Oliet, S. H. R. (2017). Astroglial glutamate transporters in the brain: Regulating neurotransmitter homeostasis and synaptic transmission. J. Neurosci. Res. 95 (11), 2140–2151. doi:10.1002/jnr.24029
Murrough, J. W., Abdallah, C. G., and Mathew, S. J. (2017). Targeting glutamate signalling in depression: Progress and prospects. Nat. Rev. Drug Discov. 16 (7), 472–486. doi:10.1038/nrd.2017.16
Nakamura, Y., Dryanovski, D. I., Kimura, Y., Jackson, S. N., Woods, A. S., Yasui, Y., et al. (2019). Cocaine-induced endocannabinoid signaling mediated by sigma-1 receptors and extracellular vesicle secretion. Elife 8, e47209. doi:10.7554/eLife.47209
Nakamya, M. F., Sil, S., Buch, S., and Hakami, R. M. (2022). Mitochondrial extracellular vesicles in CNS disorders: New Frontiers in understanding the neurological disorders of the brain. Front. Mol. Biosci. 9, 840364. doi:10.3389/fmolb.2022.840364
Nasca, C., Dobbin, J., Bigio, B., Watson, K., de Angelis, P., Kautz, M., et al. (2021). Insulin receptor substrate in brain-enriched exosomes in subjects with major depression: On the path of creation of biosignatures of central insulin resistance. Mol. Psychiatry 26 (9), 5140–5149. doi:10.1038/s41380-020-0804-7
Nectow, A. R., and Nestler, E. J. (2020). Viral tools for neuroscience. Nat. Rev. Neurosci. 21 (12), 669–681. doi:10.1038/s41583-020-00382-z
Nieland, L., Morsett, L. M., Broekman, M. L. D., Breakefield, X. O., and Abels, E. R. (2021). Extracellular vesicle-mediated bilateral communication between glioblastoma and astrocytes. Trends Neurosci. 44 (3), 215–226. doi:10.1016/j.tins.2020.10.014
Nieuwland, R., and Sturk, A. (2010). Why do cells release vesicles? Thromb. Res. 125 (1), S49–51. doi:10.1016/j.thromres.2010.01.037
Nilsson, J., Skog, J., Nordstrand, A., Baranov, V., Mincheva-Nilsson, L., Breakefield, X. O., et al. (2009). Prostate cancer-derived urine exosomes: A novel approach to biomarkers for prostate cancer. Br. J. Cancer 100 (10), 1603–7. doi:10.1038/sj.bjc.6605058
Niu, M., Li, Y., Li, G., Zhou, L., Luo, N., Yao, M., et al. (2020). A longitudinal study on alpha-synuclein in plasma neuronal exosomes as a biomarker for Parkinson's disease development and progression. Eur. J. Neurol. 27 (6), 967–974. doi:10.1111/ene.14208
Noren Hooten, N., Torres, S., Mode, N. A., Zonderman, A. B., Ghosh, P., Ezike, N., et al. (2022). Association of extracellular vesicle inflammatory proteins and mortality. Sci. Rep. 12 (1), 14049. doi:10.1038/s41598-022-17944-z
Norman, M., Ter-Ovanesyan, D., Trieu, W., Lazarovits, R., Kowal, E. J. K., Lee, J. H., et al. (2021). L1CAM is not associated with extracellular vesicles in human cerebrospinal fluid or plasma. Nat. Methods 18 (6), 631–634. doi:10.1038/s41592-021-01174-8
Ochoa, V., Loeffler, A. J., and Fowler, C. D. (2015). Emerging role of the cerebrospinal fluid - neuronal interface in neuropathology. Neuro 2, 92–98. doi:10.17140/NOJ-2-118
Odegaard, K. E., Gallegos, G., Koul, S., Schaal, V. L., Vellichirammal, N. N., Guda, C., et al. (2022). Distinct synaptic vesicle proteomic signatures associated with pre- and post-natal oxycodone-exposure. Cells 11 (11), 1740. doi:10.3390/cells11111740
Odegaard, K. E., Schaal, V. L., Clark, A. R., Koul, S., Sankarasubramanian, J., Xia, Z., et al. (2020). A holistic systems approach to characterize the impact of pre- and post-natal oxycodone exposure on neurodevelopment and behavior. Front. Cell Dev. Biol. 8, 619199. doi:10.3389/fcell.2020.619199
Olney, K. C., Todd, K. T., Pallegar, P. N., Jensen, T. D., Cadiz, M. P., Gibson, K. A., et al. (2022). Widespread choroid plexus contamination in sampling and profiling of brain tissue. Mol. Psychiatry 27 (3), 1839–1847. doi:10.1038/s41380-021-01416-3
Oraki Kohshour, M., Papiol, S., Delalle, I., Rossner, M. J., and Schulze, T. G. (2022). Extracellular vesicle approach to major psychiatric disorders. Eur. Arch. Psychiatry Clin. Neurosci. 2022. doi:10.1007/s00406-022-01497-3
Oyarce, K., Cepeda, M. Y., Lagos, R., Garrido, C., Vega-Letter, A. M., Garcia-Robles, M., et al. (2022). Neuroprotective and neurotoxic effects of glial-derived exosomes. Front. Cell Neurosci. 16, 920686. doi:10.3389/fncel.2022.920686
Palanisamy, V., Sharma, S., Deshpande, A., Zhou, H., Gimzewski, J., and Wong, D. T. (2010). Nanostructural and transcriptomic analyses of human saliva derived exosomes. PLoS One 5 (1), e8577. doi:10.1371/journal.pone.0008577
Palmqvist, S., Janelidze, S., Quiroz, Y. T., Zetterberg, H., Lopera, F., Stomrud, E., et al. (2020). Discriminative accuracy of plasma phospho-tau217 for alzheimer disease vs other neurodegenerative disorders. JAMA 324 (8), 772–781. doi:10.1001/jama.2020.12134
Palmqvist, S., Stomrud, E., Cullen, N., Janelidze, S., Manuilova, E., Jethwa, A., et al. (2023). An accurate fully automated panel of plasma biomarkers for Alzheimer's diseas e. Alzheimers Dement. 19, 1204–1215. doi:10.1002/alz.12751
Pan, B. T., Teng, K., Wu, C., Adam, M., and Johnstone, R. M. (1985). Electron microscopic evidence for externalization of the transferrin receptor in vesicular form in sheep reticulocytes. J. Cell Biol. 101 (3), 942–8. doi:10.1083/jcb.101.3.942
Paolicelli, R. C., Bergamini, G., and Rajendran, L. (2019). Cell-to-cell communication by extracellular vesicles: Focus on microglia. Neuroscience 405, 148–157. doi:10.1016/j.neuroscience.2018.04.003
Passiglia, F., Caglevic, C., Giovannetti, E., Pinto, J. A., Manca, P., Taverna, S., et al. (2018). Primary and metastatic brain cancer genomics and emerging biomarkers for immunomodulatory cancer treatment. Semin. Cancer Biol. 52 (2), 259–268. doi:10.1016/j.semcancer.2018.01.015
Pauwels, M. J., Xie, J., Ceroi, A., Balusu, S., Castelein, J., Van Wonterghem, E., et al. (2022). Choroid plexus-derived extracellular vesicles exhibit brain targeting characteristics. Biomaterials 290, 121830. doi:10.1016/j.biomaterials.2022.121830
Pegtel, D. M., and Gould, S. J. (2019). Exosomes. Annu. Rev. Biochem. 88, 487–514. doi:10.1146/annurev-biochem-013118-111902
Pegtel, D. M., Peferoen, L., and Amor, S. (2014). Extracellular vesicles as modulators of cell-to-cell communication in the healthy and diseased brain. Philosophical Trans. R. Soc. B Biol. Sci. 369, 20130516. doi:10.1098/rstb.2013.0516
Perez Ortiz, J. M., and Swerdlow, R. H. (2019). Mitochondrial dysfunction in Alzheimer's disease: Role in pathogenesis and novel therapeutic opportunities. Br. J. Pharmacol. 176 (18), 3489–3507. doi:10.1111/bph.14585
Picciolini, S., Gualerzi, A., Carlomagno, C., Cabinio, M., Sorrentino, S., Baglio, F., et al. (2021). An SPRi-based biosensor pilot study: Analysis of multiple circulating extracellular vesicles and hippocampal volume in Alzheimer's disease. J. Pharm. Biomed. Anal. 192, 113649. doi:10.1016/j.jpba.2020.113649
Pistono, C., Bister, N., Stanová, I., and Malm, T. (2021). Glia-derived extracellular vesicles: Role in central nervous system communication in health and disease. Front. Cell Dev. Biol. 8, 623771. doi:10.3389/fcell.2020.623771
Plitman, E., Nakajima, S., de la Fuente-Sandoval, C., Gerretsen, P., Chakravarty, M. M., Kobylianskii, J., et al. (2014). Glutamate-mediated excitotoxicity in schizophrenia: A review. Eur. Neuropsychopharmacol. 24 (10), 1591–605. doi:10.1016/j.euroneuro.2014.07.015
Pomytkin, I., Krasil'nikova, I., Bakaeva, Z., Surin, A., and Pinelis, V. (2019). Excitotoxic glutamate causes neuronal insulin resistance by inhibiting insulin receptor/Akt/mTOR pathway. Mol. Brain 12 (1), 112. doi:10.1186/s13041-019-0533-5
Popov, L. D. (2022). Mitochondrial-derived vesicles: Recent insights. J. Cell Mol. Med. 26 (12), 3323–3328. doi:10.1111/jcmm.17391
Porro, C., Trotta, T., and Panaro, M. A. (2015). Microvesicles in the brain: Biomarker, messenger or mediator? J. Neuroimmunol. 288, 70–8. doi:10.1016/j.jneuroim.2015.09.006
Prusiner, S. B., Scott, M. R., DeArmond, S. J., and Cohen, F. E. (1998). Prion protein biology. Cell 93 (3), 337–48. doi:10.1016/s0092-8674(00)81163-0
Pusic, K. M., Kraig, R. P., and Pusic, A. D. (2021). IFNγ-stimulated dendritic cell extracellular vesicles can be nasally administered to the brain and enter oligodendrocytes. PLoS ONE 16 (8), e0255778. doi:10.1371/journal.pone.0255778
Qu, M., Lin, Q., Huang, L., Fu, Y., Wang, L., He, S., et al. (2018). Dopamine-loaded blood exosomes targeted to brain for better treatment of Parkinson's disease. J. Control Release 287, 156–166. doi:10.1016/j.jconrel.2018.08.035
Ramirez, M. I., Amorim, M. G., Gadelha, C., Milic, I., Welsh, J. A., Freitas, V. M., et al. (2018). Technical challenges of working with extracellular vesicles. Nanoscale 10 (3), 881–906. doi:10.1039/c7nr08360b
Rana, R., Joon, S., Chauhan, K., Rathi, V., Ganguly, N. K., Kumari, C., et al. (2021). Role of extracellular vesicles in glioma progression: Deciphering cellular biological processes to clinical applications. Curr. Top. Med. Chem. 21 (8), 696–704. doi:10.2174/1568026620666201207100139
Rana, R., Sharma, S., and Ganguly, N. K. (2021). Comprehensive overview of extracellular vesicle proteomics in meningioma: Future strategy. Mol. Biol. Rep. 48 (12), 8061–8074. doi:10.1007/s11033-021-06740-z
Raposo, G., and Stoorvogel, W. (2013). Extracellular vesicles: Exosomes, microvesicles, and friends. J. Cell Biol. 200 (4), 373–83. doi:10.1083/jcb.201211138
Ratajczak, J., Miekus, K., Kucia, M., Zhang, J., Reca, R., Dvorak, P., et al. (2006). Embryonic stem cell-derived microvesicles reprogram hematopoietic progenitors: Evidence for horizontal transfer of mRNA and protein delivery. Leukemia 20 (5), 847–856. doi:10.1038/sj.leu.2404132
Ratajczak, J., Wysoczynski, M., Hayek, F., Janowska-Wieczorek, A., and Ratajczak, M. Z. (2006). Membrane-derived microvesicles: Important and underappreciated mediators of cell-to-cell communication. Leukemia 20 (9), 1487–1495. doi:10.1038/sj.leu.2404296
Rébé, C., and Ghiringhelli, F. (2015). Cytotoxic effects of chemotherapy on cancer and immune cells: How can it be modulated to generate novel therapeutic strategies? Future Oncol. 11 (19), 2645–2654. doi:10.2217/fon.15.198
Reddy, P. H., and Beal, M. F. (2008). Amyloid beta, mitochondrial dysfunction and synaptic damage: Implications for cognitive decline in aging and Alzheimer's disease. Trends Mol. Med. 14 (2), 45–53. doi:10.1016/j.molmed.2007.12.002
Rezeli, M., Gidlöf, O., Evander, M., Bryl-Górecka, P., Sathanoori, R., Gilje, P., et al. (2016). Comparative proteomic analysis of extracellular vesicles isolated by acoustic trapping or differential centrifugation. Anal. Chem. 88 (17), 8577–86. doi:10.1021/acs.analchem.6b01694
Ricklefs, F. L., Maire, C. L., Matschke, J., Dührsen, L., Sauvigny, T., Holz, M., et al. (2020). FASN is a biomarker enriched in malignant glioma-derived extracellular vesicles. Int. J. Mol. Sci. 21 (6), 1931. doi:10.3390/ijms21061931
Ricklefs, F. L., Maire, C. L., Wollmann, K., Dührsen, L., Fita, K. D., Sahm, F., et al. (2022). Diagnostic potential of extracellular vesicles in meningioma patients. Neuro Oncol. 24 (12), 2078–2090. doi:10.1093/neuonc/noac127
Robbins, P. D. (2017). Extracellular vesicles and aging. Stem Cell Investig. 4, 98. doi:10.21037/sci.2017.12.03
Royo, F., Théry, C., Falcón-Pérez, J. M., Nieuwland, R., and Witwer, K. W. (2020). Methods for separation and characterization of extracellular vesicles: Results of a worldwide survey performed by the ISEV rigor and standardization subcommittee. Cells 9 (9), 1955. doi:10.3390/cells9091955
Ruan, Z., Delpech, J. C., Venkatesan Kalavai, S., Van Enoo, A. A., Hu, J., Ikezu, S., et al. (2020). P2RX7 inhibitor suppresses exosome secretion and disease phenotype in P301S tau transgenic mice. Mol. Neurodegener. 15 (1), 47. doi:10.1186/s13024-020-00396-2
Sack, B. K., and Herzog, R. W. (2009). Evading the immune response upon in vivo gene therapy with viral vectors. Curr. Opin. Mol. Ther. 11 (5), 493–503.
Saeedi, S., Israel, S., Nagy, C., and Turecki, G. (2019). The emerging role of exosomes in mental disorders. Transl. Psychiatry 9 (1), 122. doi:10.1038/s41398-019-0459-9
Saeedi, S., Nagy, C., Ibrahim, P., Théroux, J. F., Wakid, M., Fiori, L. M., et al. (2021). Neuron-derived extracellular vesicles enriched from plasma show altered size and miRNA cargo as a function of antidepressant drug response. Mol. Psychiatry 26 (12), 7417–7424. doi:10.1038/s41380-021-01255-2
Saijo, K., and Glass, C. K. (2011). Microglial cell origin and phenotypes in health and disease. Nat. Rev. Immunol. 11 (11), 775–787. doi:10.1038/nri3086
Saman, S., Kim, W., Raya, M., Visnick, Y., Miro, S., Jackson, B., et al. (2012). Exosome-associated tau is secreted in tauopathy models and is selectively phosphorylated in cerebrospinal fluid in early Alzheimer disease. J. Biol. Chem. 287 (6), 3842–9. doi:10.1074/jbc.M111.277061
Sandau, U. S., McFarland, T. J., Smith, S. J., Galasko, D. R., Quinn, J. F., and Saugstad, J. A. (2022). Differential effects of APOE genotype on MicroRNA cargo of cerebrospinal fluid extracellular vesicles in females with Alzheimer's disease compared to males. Front. Cell Dev. Biol. 10, 864022. doi:10.3389/fcell.2022.864022
Schallinger, L. E., Gray, J. E., Wagner, L. W., Knowlton, S., and Kirkland, J. J. (1985). Preparative isolation of plasmid DNA with sedimentation field flow fractionation. J. Chromatogr. 342 (1), 67–77. doi:10.1016/s0378-4347(00)84489-8
Schindler, S. M., Little, J. P., and Klegeris, A. (2014). Microparticles: A new perspective in central nervous system disorders. Biomed. Res. Int. 2014, 756327. doi:10.1155/2014/756327
Scofield, M. D., and Kalivas, P. W. (2014). Astrocytic dysfunction and addiction: Consequences of impaired glutamate homeostasis. Neuroscientist 20 (6), 610–22. doi:10.1177/1073858413520347
Serpente, M., Fenoglio, C., D'Anca, M., Arcaro, M., Sorrentino, F., Visconte, C., et al. (2020). MiRNA profiling in plasma neural-derived small extracellular vesicles from patients with Alzheimer's disease. Cells 9 (6), 1443. doi:10.3390/cells9061443
Serrano-Heras, G., Díaz-Maroto, I., Castro-Robles, B., Carrión, B., Perona-Moratalla, A. B., Gracia, J., et al. (2020). Isolation and quantification of blood apoptotic bodies, a non-invasive tool to evaluate apoptosis in patients with ischemic stroke and neurodegenerative diseases. Biol. Proced. Online 22 (1), 17. doi:10.1186/s12575-020-00130-8
Shurtleff, M. J., Yao, J., Qin, Y., Nottingham, R. M., Temoche-Diaz, M. M., Schekman, R., et al. (2017). Broad role for YBX1 in defining the small noncoding RNA composition of exosomes. Proc. Natl. Acad. Sci. U. S. A. 114 (43), E8987–E8995. doi:10.1073/pnas.1712108114
Sidoryk-Wegrzynowicz, M., Wegrzynowicz, M., Lee, E., Bowman, A. B., and Aschner, M. (2011). Role of astrocytes in brain function and disease. Toxicol. Pathol. 39 (1), 115–23. doi:10.1177/0192623310385254
Sil, S., Singh, S., Chemparathy, D. T., Chivero, E. T., Gordon, L., and Buch, S. (2021). Astrocytes & astrocyte derived extracellular vesicles in morphine induced amyloidopathy: Implications for cognitive deficits in opiate abusers. Aging Dis. 12 (6), 1389–1408. doi:10.14336/AD.2021.0406
Simon, T., Jackson, E., and Giamas, G. (2020). Breaking through the glioblastoma micro-environment via extracellular vesicles. Oncogene 39, 4477–4490. doi:10.1038/s41388-020-1308-2
Simpson, I. A., and Sonne, O. (1982). A simple, rapid, and sensitive method for measuring protein concentration in subcellular membrane fractions prepared by sucrose density ultracentrifugation. Anal. Biochem. 119 (2), 424–7. doi:10.1016/0003-2697(82)90608-x
Simpson, R. J., Lim, J. W., Moritz, R. L., and Mathivanan, S. (2009). Exosomes: Proteomic insights and diagnostic potential. Expert Rev. Proteomics 6 (3), 267–83. doi:10.1586/epr.09.17
Singh, T., and Rajput, M. (2006). Misdiagnosis of bipolar disorder. Psychiatry (Edgmont) 3 (10), 57–63.
Sitar, S., Kejžar, A., Pahovnik, D., Kogej, K., Tušek-Žnidarič, M., Lenassi, M., et al. (2015). Size characterization and quantification of exosomes by asymmetrical-flow field-flow fractionation. Anal. Chem. 87 (18), 9225–33. doi:10.1021/acs.analchem.5b01636
Skog, J., Würdinger, T., van Rijn, S., Meijer, D. H., Gainche, L., Sena-Esteves, M., et al. (2008). Glioblastoma microvesicles transport RNA and proteins that promote tumour growth and provide diagnostic biomarkers. Nat. Cell Biol. 10 (12), 1470–6. doi:10.1038/ncb1800
Smith, J. T., Wunsch, B. H., Dogra, N., Ahsen, M. E., Lee, K., Yadav, K. K., et al. (2018). Integrated nanoscale deterministic lateral displacement arrays for separation of extracellular vesicles from clinically-relevant volumes of biological samples. Lab. Chip 18 (24), 3913–3925. doi:10.1039/c8lc01017j
Song, Y., Li, Z., He, T., Qu, M., Jiang, L., Li, W., et al. (2019). M2 microglia-derived exosomes protect the mouse brain from ischemia-reperfusion injury via exosomal miR-124. Theranostics 9 (10), 2910–2923. doi:10.7150/thno.30879
Song, Z., Xu, Y., Deng, W., Zhang, L., Zhu, H., Yu, P., et al. (2020). Brain derived exosomes are a double-edged sword in Alzheimer's disease. Front. Mol. Neurosci. 13, 79. doi:10.3389/fnmol.2020.00079
Sousa, C., Biber, K., and Michelucci, A. (2017). Cellular and molecular characterization of microglia: A unique immune cell population. Front. Immunol. 8, 198. doi:10.3389/fimmu.2017.00198
Strovel, J., Sittampalam, S., Coussens, N. P., Hughes, M., Inglese, J., Kurtz, A., et al. (2004). “Early drug discovery and development guidelines: For academic researchers, collaborators, and start-up companies,” in Assay guidance manual (Bethesda, MD: Eli Lilly & Company and the National Center for Advancing Translational Sciences).
Stuendl, A., Kunadt, M., Kruse, N., Bartels, C., Moebius, W., Danzer, K. M., et al. (2016). Induction of α-synuclein aggregate formation by CSF exosomes from patients with Parkinson's disease and dementia with Lewy bodies. Brain 139 (2), 481–494. doi:10.1093/brain/awv346
Su, S. A., Xie, Y., Fu, Z., Wang, Y., Wang, J. A., and Xiang, M. (2017). Emerging role of exosome-mediated intercellular communication in vascular remodeling. Oncotarget 8 (15), 25700–25712. doi:10.18632/oncotarget.14878
Su, W., and Pasternak, G. W. (2013). The role of multidrug resistance-associated protein in the blood-brain barrier and opioid analgesia. Synapse 67 (9), 609–19. doi:10.1002/syn.21667
Sugiura, A., McLelland, G. L., Fon, E. A., and McBride, H. M. (2014). A new pathway for mitochondrial quality control: Mitochondrial-derived vesicles. Embo J. 33 (19), 2142–56. doi:10.15252/embj.201488104
Sweeney, P., Park, H., Baumann, M., Dunlop, J., Frydman, J., Kopito, R., et al. (2017). Protein misfolding in neurodegenerative diseases: Implications and strategies. Transl. Neurodegener. 6, 6. doi:10.1186/s40035-017-0077-5
Szatanek, R., Baran, J., Siedlar, M., and Baj-Krzyworzeka, M. (2015). Isolation of extracellular vesicles: Determining the correct approach (Review). Int. J. Mol. Med. 36 (1), 11–7. doi:10.3892/ijmm.2015.2194
Takeda, S., Wegmann, S., Cho, H., DeVos, S. L., Commins, C., Roe, A. D., et al. (2015). Neuronal uptake and propagation of a rare phosphorylated high-molecular-weight tau derived from Alzheimer's disease brain. Nat. Commun. 6, 8490. doi:10.1038/ncomms9490
Tauro, B. J., Greening, D. W., Mathias, R. A., Ji, H., Mathivanan, S., Scott, A. M., et al. (2012). Comparison of ultracentrifugation, density gradient separation, and immunoaffinity capture methods for isolating human colon cancer cell line LIM1863-derived exosomes. Methods 56 (2), 293–304. doi:10.1016/j.ymeth.2012.01.002
Tcw, J., Qian, L., Pipalia, N. H., Chao, M. J., Liang, S. A., Shi, Y., et al. (2022). Cholesterol and matrisome pathways dysregulated in astrocytes and microglia. Cell 185 (13), 2213–2233 e25. doi:10.1016/j.cell.2022.05.017
Théry, C., Amigorena, S., Raposo, G., and Clayton, A. (2006). Isolation and characterization of exosomes from cell culture supernatants and biological fluids. Curr. Protoc. Cell Biol. 3, 3.22. doi:10.1002/0471143030.cb0322s30
Théry, C., Ostrowski, M., and Segura, E. (2009). Membrane vesicles as conveyors of immune responses. Nat. Rev. Immunol. 9 (8), 581–593. doi:10.1038/nri2567
Thery, C., Witwer, K. W., Aikawa, E., Alcaraz, M. J., Anderson, J. D., Andriantsitohaina, R., et al. (2018). Minimal information for studies of extracellular vesicles 2018 (MISEV2018): A position statement of the international society for extracellular vesicles and update of the MISEV2014 guidelines. J. Extracell. Vesicles 7 (1), 1535750. doi:10.1080/20013078.2018.1535750
Thompson, A. G., Gray, E., Heman-Ackah, S. M., Mager, I., Talbot, K., Andaloussi, S. E., et al. (2016). Extracellular vesicles in neurodegenerative disease - pathogenesis to biomarkers. Nat. Rev. Neurol. 12 (6), 346–57. doi:10.1038/nrneurol.2016.68
Todd, A. C., and Hardingham, G. E. (2020). The regulation of astrocytic glutamate transporters in health and neurodegenerative diseases. Int. J. Mol. Sci. 21 (24), 9607. doi:10.3390/ijms21249607
Toden, S., Zhuang, J., Acosta, A. D., Karns, A. P., Salathia, N. S., Brewer, J. B., et al. (2020). Noninvasive characterization of Alzheimer's disease by circulating, cell-free messenger RNA next-generation sequencing. Sci. Adv. 6 (50), eabb1654. doi:10.1126/sciadv.abb1654
Tominaga, N., Kosaka, N., Ono, M., Katsuda, T., Yoshioka, Y., Tamura, K., et al. (2015). Brain metastatic cancer cells release microRNA-181c-containing extracellular vesicles capable of destructing blood-brain barrier. Nat. Commun. 6, 6716. doi:10.1038/ncomms7716
Trams, E. G., Lauter, C. J., Salem, N., and Heine, U. (1981). Exfoliation of membrane ecto-enzymes in the form of micro-vesicles. Biochim. Biophys. Acta 645 (1), 63–70. doi:10.1016/0005-2736(81)90512-5
Troubat, R., Barone, P., Leman, S., Desmidt, T., Cressant, A., Atanasova, B., et al. (2021). Neuroinflammation and depression: A review. Eur. J. Neurosci. 53 (1), 151–171. doi:10.1111/ejn.14720
Tsivion-Visbord, H., Perets, N., Sofer, T., Bikovski, L., Goldshmit, Y., Ruban, A., et al. (2020). Mesenchymal stem cells derived extracellular vesicles improve behavioral and biochemical deficits in a phencyclidine model of schizophrenia. Transl. Psychiatry 10 (1), 305. doi:10.1038/s41398-020-00988-y
Upadhya, R., Zingg, W., Shetty, S., and Shetty, A. K. (2020). Astrocyte-derived extracellular vesicles: Neuroreparative properties and role in the pathogenesis of neurodegenerative disorders. J. Control Release 323, 225–239. doi:10.1016/j.jconrel.2020.04.017
Valadi, H., Ekström, K., Bossios, A., Sjöstrand, M., Lee, J. J., and Lötvall, J. O. (2007). Exosome-mediated transfer of mRNAs and microRNAs is a novel mechanism of genetic exchange between cells. Nat. Cell Biol. 9 (6), 654–9. doi:10.1038/ncb1596
van Balkom, B. W., de Jong, O. G., Smits, M., Brummelman, J., den Ouden, K., et al. (2013). Endothelial cells require miR-214 to secrete exosomes that suppress senescence and induce angiogenesis in human and mouse endothelial cells. Blood 121 (19), 3997–4006. doi:10.1182/blood-2013-02-478925
van der Zee, Y. Y., Eijssen, L. M. T., Mews, P., Ramakrishnan, A., Alvarez, K., Lardner, C. K., et al. (2022). Blood miR-144-3p: A novel diagnostic and therapeutic tool for depression. Mol. Psychiatry 27 (11), 4536–4549. doi:10.1038/s41380-022-01712-6
van Niel, G., D'Angelo, G., and Raposo, G. (2018). Shedding light on the cell biology of extracellular vesicles. Nat. Rev. Mol. Cell Biol. 19 (4), 213–228. doi:10.1038/nrm.2017.125
Vasincu, A., Rusu, R. N., Ababei, D. C., Larion, M., Bild, W., Stanciu, G. D., et al. (2022). Endocannabinoid modulation in neurodegenerative diseases: In pursuit of certainty. Biol. (Basel) 11 (3), 440. doi:10.3390/biology11030440
Verderio, C., Muzio, L., Turola, E., Bergami, A., Novellino, L., Ruffini, F., et al. (2012). Myeloid microvesicles are a marker and therapeutic target for neuroinflammation. Ann. Neurol. 72 (4), 610–24. doi:10.1002/ana.23627
Wachalska, M., Koppers-Lalic, D., van Eijndhoven, M., Pegtel, M., Geldof, A. A., Lipinska, A. D., et al. (2016). Protein complexes in urine interfere with extracellular vesicle biomarker studies. J. Circ. Biomark. 5, 4. doi:10.5772/62579
Wang, D., Wang, P., Bian, X., Xu, S., Zhou, Q., Zhang, Y., et al. (2020). Elevated plasma levels of exosomal BACE1-AS combined with the volume and thickness of the right entorhinal cortex may serve as a biomarker for the detection of Alzheimer's disease. Mol. Med. Rep. 22 (1), 227–238. doi:10.3892/mmr.2020.11118
Wang, J., Chang, S., Li, G., and Sun, Y. (2017). Application of liquid biopsy in precision medicine: Opportunities and challenges. Front. Med. 11 (4), 522–527. doi:10.1007/s11684-017-0526-7
Wei, Z., Batagov, A. O., Schinelli, S., Wang, J., Wang, Y., El Fatimy, R., et al. (2017). Coding and noncoding landscape of extracellular RNA released by human glioma stem cells. Nat. Commun. 8 (1), 1145. doi:10.1038/s41467-017-01196-x
Wei, Z. X., Xie, G. J., Mao, X., Zou, X. P., Liao, Y. J., Liu, Q. S., et al. (2020). Exosomes from patients with major depression cause depressive-like behaviors in mice with involvement of miR-139-5p-regulated neurogenesis. Neuropsychopharmacology 45 (6), 1050–1058. doi:10.1038/s41386-020-0622-2
Wiedrick, J. T., Phillips, J. I., Lusardi, T. A., McFarland, T. J., Lind, B., Sandau, U. S., et al. (2019). Validation of MicroRNA biomarkers for Alzheimer's disease in human cerebrospinal fluid. J. Alzheimers Dis. 67 (3), 875–891. doi:10.3233/JAD-180539
Wijtenburg, S. A., Kapogiannis, D., Korenic, S. A., Mullins, R. J., Tran, J., Gaston, F. E., et al. (2019). Brain insulin resistance and altered brain glucose are related to memory impairments in schizophrenia. Schizophr. Res. 208, 324–330. doi:10.1016/j.schres.2019.01.031
Williams, K., Ulvestad, E., and Antel, J. (1994). Immune regulatory and effector properties of human adult microglia studies in vitro and in situ. Adv. Neuroimmunol. 4 (3), 273–81. doi:10.1016/s0960-5428(06)80267-6
Witwer, K. W., Buzás, E. I., Bemis, L. T., Bora, A., Lässer, C., Lötvall, J., et al. (2013). Standardization of sample collection, isolation and analysis methods in extracellular vesicle research. J. Extracell. Vesicles 2, 20360. doi:10.3402/jev.v2i0.20360
Wright, B. L., Lai, J. T., and Sinclair, A. J. (2012). Cerebrospinal fluid and lumbar puncture: A practical review. J. Neurol. 259 (8), 1530–45. doi:10.1007/s00415-012-6413-x
Wu, J. s., Jiang, J., Chen, B. j., Wang, K., Tang, Y. l., and Liang, X. h. (2021). Plasticity of cancer cell invasion: Patterns and mechanisms. Transl. Oncol. 14, 100899. doi:10.1016/j.tranon.2020.100899
Wunsch, B. H., Smith, J. T., Gifford, S. M., Wang, C., Brink, M., Bruce, R. L., et al. (2016). Nanoscale lateral displacement arrays for the separation of exosomes and colloids down to 20 nm. Nat. Nanotechnol. 11 (11), 936–940. doi:10.1038/nnano.2016.134
Xiao, Y., Wang, S. K., Zhang, Y., Rostami, A., Kenkare, A., Casella, G., et al. (2021). Role of extracellular vesicles in neurodegenerative diseases. Prog. Neurobiol. 201, 102022. doi:10.1016/j.pneurobio.2021.102022
Xie, X. H., Lai, W. T., Xu, S. X., Di Forti, M., Zhang, J. Y., Chen, M. M., et al. (2022). Hyper-inflammation of astrocytes in patients of major depressive disorder: Evidence from serum astrocyte-derived extracellular vesicles. Brain Behav. Immun. 109, 51–62. doi:10.1016/j.bbi.2022.12.014
Yang, C., Qi, Y., and Sun, Z. (2021). The role of sonic hedgehog pathway in the development of the central nervous system and aging-related neurodegenerative diseases. Front. Mol. Biosci. 8, 711710. doi:10.3389/fmolb.2021.711710
Yekula, A., Yekula, A., Muralidharan, K., Kang, K., Carter, B. S., and Balaj, L. (2020). Extracellular vesicles in glioblastoma tumor microenvironment. Front. Immunol. Front. Media S.A. 10, 3137. doi:10.3389/fimmu.2019.03137
Yin, G., Yu, B., Liu, C., Lin, Y., Xie, Z., Hu, Y., et al. (2021). Exosomes produced by adipose-derived stem cells inhibit schwann cells autophagy and promote the regeneration of the myelin sheath. Int. J. Biochem. Cell Biol. 132, 105921. doi:10.1016/j.biocel.2021.105921
You, Y., Borgmann, K., Edara, V. V., Stacy, S., Ghorpade, A., and Ikezu, T. (2020). Activated human astrocyte-derived extracellular vesicles modulate neuronal uptake, differentiation and firing. J. Extracell. Vesicles 9 (1), 1706801. doi:10.1080/20013078.2019.1706801
Younas, N., Fernandez Flores, L. C., Hopfner, F., Höglinger, G. U., and Zerr, I. (2022). A new paradigm for diagnosis of neurodegenerative diseases: Peripheral exosomes of brain origin. Transl. Neurodegener. 11 (1), 28. doi:10.1186/s40035-022-00301-5
Zeng, A., Wei, Z., Rabinovsky, R., Jun, H. J., El Fatimy, R., Deforzh, E., et al. (2020). Glioblastoma-derived extracellular vesicles facilitate transformation of astrocytes via reprogramming oncogenic metabolism. iScience 23, 101420. doi:10.1016/j.isci.2020.101420
Zhang, H., Freitas, D., Kim, H. S., Fabijanic, K., Li, Z., Chen, H., et al. (2018). Identification of distinct nanoparticles and subsets of extracellular vesicles by asymmetric flow field-flow fractionation. Nat. Cell Biol. 20 (3), 332–343. doi:10.1038/s41556-018-0040-4
Zhang, H., and Lyden, D. (2019). Asymmetric-flow field-flow fractionation technology for exomere and small extracellular vesicle separation and characterization. Nat. Protoc. 14 (4), 1027–1053. doi:10.1038/s41596-019-0126-x
Zhang, Q., Higginbotham, J. N., Jeppesen, D. K., Yang, Y. P., Li, W., McKinley, E. T., et al. (2019). Transfer of functional cargo in exomeres. Cell Rep. 27 (3), 940–954. doi:10.1016/j.celrep.2019.01.009
Zhang, Q., Jeppesen, D. K., Higginbotham, J. N., Graves-Deal, R., Trinh, V. Q., Ramirez, M. A., et al. (2021). Supermeres are functional extracellular nanoparticles replete with disease biomarkers and therapeutic targets. Nat. Cell Biol. 23 (12), 1240–1254. doi:10.1038/s41556-021-00805-8
Zhang, Y., Liu, Y., Liu, H., and Tang, W. H. (2019). Exosomes: Biogenesis, biologic function and clinical potential. Cell & Biosci. 9 (1), 19. doi:10.1186/s13578-019-0282-2
Zhang, Z., Wu, H., Peng, Q., Xie, Z., Chen, F., Ma, Y., et al. (2021). Integration of molecular inflammatory interactome analyses reveals dynamics of circulating cytokines and extracellular vesicle long non-coding RNAs and mRNAs in heroin addicts during acute and protracted withdrawal. Front. Immunol. 12, 730300. doi:10.3389/fimmu.2021.730300
Keywords: extracellular vesicle (EV), exosome (vesicle), brain, therapy, liquid biopsy
Citation: Soleymani T, Chen T-Y, Gonzalez-Kozlova E and Dogra N (2023) The human neurosecretome: extracellular vesicles and particles (EVPs) of the brain for intercellular communication, therapy, and liquid-biopsy applications. Front. Mol. Biosci. 10:1156821. doi: 10.3389/fmolb.2023.1156821
Received: 01 February 2023; Accepted: 25 April 2023;
Published: 17 May 2023.
Edited by:
Ramin M. Hakami, George Mason University, United StatesReviewed by:
Luksana Chaiswing, University of Kentucky, United StatesSowmya Yelamanchili, University of Nebraska Medical Center, United States
Copyright © 2023 Soleymani, Chen, Gonzalez-Kozlova and Dogra. This is an open-access article distributed under the terms of the Creative Commons Attribution License (CC BY). The use, distribution or reproduction in other forums is permitted, provided the original author(s) and the copyright owner(s) are credited and that the original publication in this journal is cited, in accordance with accepted academic practice. No use, distribution or reproduction is permitted which does not comply with these terms.
*Correspondence: Edgar Gonzalez-Kozlova, ZWRnYXIuZ29uemFsZXota296bG92YUBtc3NtLmVkdQ==; Navneet Dogra, bmF2bmVldC5kb2dyYUBtc3NtLmVkdQ==
†These authors have contributed equally to this work
‡Lead contact