- 1Integrated Program in Neuroscience, McGill University, Montreal, QC, Canada
- 2McGill Group for Suicide Studies, Douglas Mental Health University Institute, Verdun, QC, Canada
- 3Department of Psychiatry, McGill University, Montreal, QC, Canada
Aerobic exercise promotes beneficial effects in the brain including increased synaptic plasticity and neurogenesis and regulates neuroinflammation and stress response via the hypothalamic-pituitary-adrenal axis. Exercise can have therapeutic effects for numerous brain-related pathologies, including major depressive disorder (MDD). Beneficial effects of aerobic exercise are thought to be mediated through the release of “exerkines” including metabolites, proteins, nucleic acids, and hormones that communicate between the brain and periphery. While the specific mechanisms underlying the positive effects of aerobic exercise on MDD have not been fully elucidated, the evidence suggests that exercise may exert a direct or indirect influence on the brain via small extracellular vesicles which have been shown to transport signaling molecules including “exerkines” between cells and across the blood-brain barrier (BBB). sEVs are released by most cell types, found in numerous biofluids, and capable of crossing the BBB. sEVs have been associated with numerous brain-related functions including neuronal stress response, cell-cell communication, as well as those affected by exercise like synaptic plasticity and neurogenesis. In addition to known exerkines, they are loaded with other modulatory cargo such as microRNA (miRNA), an epigenetic regulator that regulates gene expression levels. How exercise-induced sEVs mediate exercise dependent improvements in MDD is unknown. Here, we perform a thorough survey of the current literature to elucidate the potential role of sEVs in the context of neurobiological changes seen with exercise and depression by summarizing studies on exercise and MDD, exercise and sEVs, and finally, sEVs as they relate to MDD. Moreover, we describe the links between peripheral sEV levels and their potential for infiltration into the brain. While literature suggests that aerobic exercise is protective against the development of mood disorders, there remains a scarcity of data on the therapeutic effects of exercise. Recent studies have shown that aerobic exercise does not appear to influence sEV size, but rather influence their concentration and cargo. These molecules have been independently implicated in numerous neuropsychiatric disorders. Taken together, these studies suggest that concentration of sEVs are increased post exercise, and they may contain specifically packaged protective cargo representing a novel therapeutic for MDD.
Introduction
Major depressive disorder (MDD) is one of the leading causes of disability worldwide (Smith, 2014). The World Health Organization estimates more than 300 million people worldwide suffer from MDD (Soares et al., 2021). Depressive disorders are characterized by mood changes, anhedonia, disrupted sleep, cognitive impairments, and suicidal thoughts (Dean and Keshavan, 2017). Without efficient treatments, depression is likely to be associated with several other physical comorbidities such as cancer, stroke, and acute coronary syndrome (Kang et al., 2015). Current treatments rely on antidepressant drugs, however only 30%–40% of depressed patients achieve a complete remission with treatment, and unremitted patients are at a higher risk for suicide (Block and Nemeroff, 2014). Given that traditional treatments are inadequate and have several pharmacological side effects (Alemi et al., 2021), there is an urgent need to better characterize molecular pathways involved in MDD to find novel therapeutic targets. Furthermore, the global prevalence of MDD is twice as common in women than in men (Albert, 2015). This differential risk arises from a combination of physiological, psychological, and environmental, including cultural factors, with sex as a biological variable (SABV) as a central factor (Salk et al., 2017). However, the underlying neurobiological mechanisms are not fully understood, and more research is needed to characterize the biological sex differences in MDD for better and more targeted treatment options (Bangasser and Cuarenta, 2021).
Exercise is one of the most effective non-pharmacological therapeutic means to sustain a healthy mind (Cotman et al., 2007; Jaworska et al., 2019), and has been used as a treatment for 26 various illnesses including depression, anxiety, and schizophrenia (Pedersen and Saltin, 2015).
Physical exercise (PE) is classified into aerobic and anaerobic PE depending on the level of intensity, the types of muscle fibers used, and the recruited metabolic energy system (CCA, 2017). Aerobic exercise in particular has been linked to several mental health benefits (Paluska and Schwenk, 2000). It rescues many features of neuropsychiatric disorders (Gujral et al., 2017), reduces depression and anxiety (Belvederi Murri et al., 2019), promotes hippocampal plasticity (Cooper et al., 2018), increases learning and memory (Hötting and Röder, 2013), and delays the onset of neurodegenerative symptoms (Valenzuela et al., 2020). Strong evidence shows that aerobic exercise is an effective treatment for depression (Kvam et al., 2016; Mandolesi et al., 2018), and the American Psychiatric Association recommends it as a non-pharmacological antidepressant treatment. However, the neural molecular mechanisms responsible for exercise-induced benefits on depressive symptoms are elusive. Since MDD, like other complex disorders, has numerous causal factors, and treatments are not equally effective in all patients, there has been increased interest in individualized treatments in psychiatry (Rush et al., 2006). Consequently, understanding the peripheral and central effects of exercise, and their interplay, will be an asset for pinpointing personalized treatment strategies. Interestingly, some of the beneficial effects of exercise are mediated by the release of extracellular vesicles (EVs) into circulation (Nederveen et al., 2021). Extracellular vesicles are released by nearly all cells in the body and carry molecular cargo that can influence the recipient cell. Many different molecules have been associated with EVs including, DNA, RNA, proteins, lipids, and microRNAs (miRNAs), the latter an epigenetic regulator whose dysregulation has been associated with many disease states including depression (Smalheiser et al., 2014; Belzeaux et al., 2017; Saeedi et al., 2021). EVs are found in numerous biofluids and are capable of crossing the blood-brain barrier which positions them as strong potential biomarkers, particularly for psychiatric phenotypes (Saeedi et al., 2021). Mounting evidence suggests that EVs, and particularly their cargo play a role in numerous brain-related functions including synaptic plasticity, neuronal stress response, cell-to-cell communication, and neurogenesis (Lafourcade et al., 2016; Luarte et al., 2017; Saeedi et al., 2019).
Recent studies show that after a single bout of aerobic physical activity, skeletal muscles release EVs into circulation (Frühbeis et al., 2015) that are thought to be taken up by other tissues, including the brain (Chen et al., 2016; Vechetti et al., 2021). An interesting demonstration of the ongoing interactions between the central nervous system and the periphery is the ability to isolate neuronal-origin EVs from plasma (Mustapic et al., 2017). Likewise, there is reason to believe peripheral EVs can exert their effects centrally (Shi et al., 2019). As such, there is increasing interest in the role of EVs as drivers of inter-organ communication associated with exercise, specifically through the transport of molecular signals. Given that, both exercise and EVs are shown to have a mediating role in mental illnesses, and the known link between exercise and EV release, we posit that exercise-induced EV release mediates the effect of PE on MDD.
In this perspective review, we demonstrate the potential of aerobic exercise as an adjunct treatment option for major depression. We delve into the mechanisms by which exercise affects brain health, including its impact on synaptic plasticity, neuroinflammation, and monoamine neurotransmission. While other manuscripts have focused on the triad of exercise, EVs, and depression (Soares et al., 2021), here, we expand on this triad by providing additional perspectives on the therapeutic effects of exercise factors and skeletal muscle-released small EVs (sEVs) on MDD. We explore multiple mechanisms beyond inflammation, shedding the light on other potential pathways implicated in the therapeutic effects of exercise-induced sEVs. Specifically, we highlight the potential role of exercise-induced sEVs in promoting neural plasticity, and neurogenesis, drawing insights from a range of both animal and human studies.
Moreover, we emphasize the significance of sex as a crucial biological variable in understanding the interplay between exercise, sEVs, and MDD. Most importantly, our review takes an additional step in this novel field by exploring the different mechanisms underlying the passage of sEVs across the BBB, going beyond the current focus on exercise, sEVs, and MDD. This discussion aims to provide a more nuanced understanding of the relationship between exercise and sEV-mediated effects on the nervous system.
Physical exercise in alleviating symptoms of major depression
Research into the beneficial effects of exercise on depression dates to the early 1900s, with studies by Franz and Hamilton (1905), Vaux (1926), and Ström-Olsen (1937). Paffenbarger et al. (1994) conducted a study on 31,000 Harvard College graduates aged 35–74 years, following them for 23–27 years. The study revealed that physically active individuals (walking, climbing, descending stairs, etc.) and sports players had 17%–28% lower incidence of depression compared to their sedentary counterparts (Paffenbarger et al., 1994). Similarly, a recent study on 5,877 participants aged 15–54 years found that regular exercise was associated with a 25% decrease in the likelihood of major depressive disorder (Goodwin, 2003). Additionally, exercise has been found to have a protective effect against depression regardless of age or geographical location, as reported in a study by Schuch et al. (2018). Indeed, prospective surveys suggest that exercise is protective against MDD, however, the causality and direction of this relationship are not explicit. Nevertheless, a genome-wide association study (GWAS) from 600,000 adults showed that people with genetic markers associated with MDD (44 independent genome-wide significant SNPs) were not less likely to exercise (objective accelerometer-based physical activity), hence MDD does not make one intrinsically less likely to exercise (Choi et al., 2019).
The exercise regimens used in research vary widely across studies leading to inconsistent findings or interpretations of the data. Nonetheless, there have been numerous studies looking at the therapeutic role of aerobic exercise in the context of MDD which have provided consistent, comparable, and measurable outcomes (Carter et al., 2015; Jaworska et al., 2019; Morres et al., 2019). Aerobic exercise can be defined by aerobic metabolism, the use of large muscle groups, prolonged duration, and consistent rhythm. Patients with MDD engaging in aerobic treadmill training 3 times a week for 16 weeks at home or in a supervised setting to exhaustion were found to have reduced Hamilton Depression Rating Scales (HAMD) scores similar to effects observed in patients treated with antidepressants (Blumenthal et al., 2007). Similarly, inactive and unmedicated 18–24 year olds with depression who underwent a 12-week program of aerobic exercise (3x/week; 1 h/session) showed an increased cardiovascular score and approximately 50% decreased depression HAMD scores (Jaworska et al., 2019). A systematic review and meta-analysis on the dose-response relationship between PE and depression suggests that small doses of aerobic exercise (2.5 h/week of brisk walking) are sufficient to lower risks of depression (Pearce et al., 2022), and several other studies have demonstrated that in some cases it is as effective as antidepressants (Netz, 2017). However, in severely depressed patients receiving pharmacologic therapy, exercise is considered as an adjunct treatment along with cognitive-behavioral therapies for managing symptoms of depression (Craft and Perna, 2004). Nonetheless, Stanton and colleagues showed that the use of moderate-intensity aerobic exercise three times a week for a minimum of 9 weeks could lower the symptoms of depression in adults aged 18–65 years with a diagnosis of depression (Stanton and Reaburn, 2014). Moreover, Knubben and colleagues showed that patients diagnosed with MDD may quickly show a significant improvement in their mood: the Bech-Rafaelsen Melancholy Scale (BMRS) scores dropped by 36% after 10 days of endurance exercise that consisted of daily walking on a treadmill (Knubben et al., 2007).
Exercise-induced benefits on MDD are most commonly associated with certain neuro-molecular mechanisms including; increasing the levels of serotonin and norepinephrine (Ross et al., 2023), increasing the expression of neurotrophins such as brain-derived neurotrophic factor (Russo-Neustadt et al., 2000), regulating the hypothalamic-pituitary-adrenal (HPA) axis activity (Lopresti et al., 2013), as well as reducing inflammation (Paolucci et al., 2018). The beneficial effects of exercise are thought to be mediated through the release of “exerkines” which encompass multiple signaling factors including metabolites, proteins, nucleic acids, and hormones that crosstalk between the brain and the periphery (Lee et al., 2019; Stephan and Sleiman, 2021; Chow et al., 2022). Various exercise-induced factors released into the bloodstream from muscle, liver, and bone, have been shown to cross the blood-brain barrier (BBB) enhancing brain-derived neurotrophic factor (BDNF) signaling, and alleviating the symptoms of depression (Sleiman et al., 2016; Karnib et al., 2019; Rentz et al., 2020). Additionally, aerobic exercise regulates miRNA expression in the prefrontal cortex and the hippocampus (Fernandes et al., 2018; Qu et al., 2020). Many mechanisms and pathways related to depression are influenced by exerkine related-factors of which, many are speculated to be transported in circulation in sEVs supporting the potential cross-talk between peripheral organs or between peripheral organs and the central nervous (Safdar and Tarnopolsky, 2018).
There have been many excellent reviews focusing on the efficacy of different exercise variables such as intensity (Rethorst et al., 2013), duration and frequency (Pearce et al., 2022) in the context of exercise and depression. These broader factors are outside the scope of this review as we will focus on changes in small extracellular vesicles (sEVs) characteristics during exercise and their impact on depression.
The effect of exercise on synaptic plasticity, neuroinflammation, and the monoamine neurotransmitters
Synaptic plasticity is shown to be disrupted in the hippocampi of individuals with depression (Liu et al., 2017). Interestingly, exercise positively affects brain function via the induction of synaptic plasticity (Mandolesi et al., 2017). Studies on humans and animals using magnetic resonance imaging (MRI) have shown that prolonged voluntary exercise increases the volume of the hippocampal region in mice (Islam et al., 2020), and long-term moderate-intensity exercise increases the volume of several brain regions in humans (Nauer et al., 2020). By triggering several different systems, such as the inflammatory system, the monoamine system, the neurotrophic pathway, and the HPA axis, exercise can mediate its favorable effects on the brain. Among these systems, the brain-derived neurotrophic factor (BDNF) signaling pathways is one of the most significant mechanisms activated by PE. BDNF is a protein that promotes the growth and survival of neurons (Miranda et al., 2019). Postmortem human studies of individuals with depression and/or who have died by suicide show reduced BDNF protein levels in the hippocampus and other brain regions, contrary to individuals receiving antidepressant treatment (Chen et al., 2001; Duman and Monteggia, 2006; Kim et al., 2007). In humans, aerobic exercise training triggers a rise in serum BDNF levels, which is linked to the increase of hippocampal volume, enhancement of spatial memory (Erickson et al., 2011; Khoury et al., 2023), and a reduction of depressive symptoms (Erickson et al., 2012). However, exercise-induced neuronal plasticity is inhibited in mice with the single nucleotide polymorphism (SNP) in the BDNF gene Val66Met (BDNFMet/Met) (Hariri et al., 2003), or when the BDNF signaling is blocked (Vaynman et al., 2004).
It has been demonstrated that crosstalk between the periphery and the brain can be triggered by exercise resulting from the release of molecules and factors from the liver, muscles, and bones into the bloodstream to support neuronal plasticity (Stephan & Sleiman, 2021) (Figure 1). These exercise-induced factors activate convergent neural pathways, with BDNF signaling in the central role of this coordinated response (Fakhoury et al., 2022). Upon exercise, the muscle secretes several factors that activate BDNF signaling and regulate neural plasticity such as the myokine fibronectin type III domain-containing protein 5 (FNDC5) (Wrann et al., 2013), and lactate (El Hayek et al., 2019).
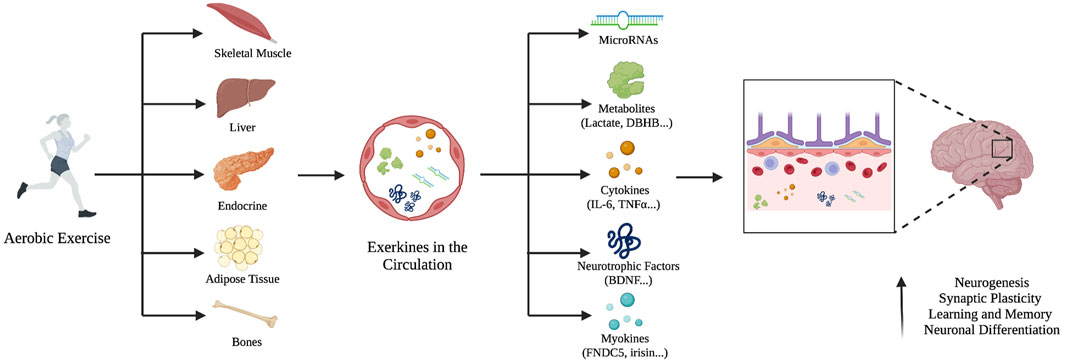
FIGURE 1. The systemic release of exerkines and their effect on the brain. Aerobic exercise stimulates different peripheral tissues to release exerkines into the bloodstream such as microRNAs, metabolites, cytokines, neurotrophic factors, and myokines. They can cross the blood-brain barrier, increase neurogenesis, improve synaptic plasticity, and neural differentiation.
Moderate to vigorous exercise increases lactate production and accumulation in the muscle and enhances the uptake of skeletal muscle-derived lactate by the brain, where it exerts its function in several ways (Xue et al., 2022). Exercise-induced lactate is transported to the hippocampus via the bloodstream where it binds to monocarboxylate transporters (MCT 1-4s), modulates hippocampal class I histone deacetylase (HDAC2/3) levels, which results in reduced depressive-like symptoms in mice (Proia et al., 2016; Karnib et al., 2019). It has also been suggested that lactate activates G Protein-Coupled Receptor 81 (GPR81) (Lauritzen et al., 2014), to promote anti-inflammatory effects (Hoque et al., 2014), and inhibit GABAergic transmission (Bozzo et al., 2013). Lactate can also increase the expression levels of the lysine deacetylase Sirtuin 1 (SIRT1), to activate the peroxisome proliferator-activated receptor gamma coactivator 1-alpha and the estrogen related receptor alpha complex (PGC1alpha/ERRa), causing an increase in the expression levels of FNDC5 in the hippocampus (Wrann et al., 2013). FNDC5 and its cleavage product irisin activate BDNF transcription via intermediate pathways, enhancing learning and memory formation (El Hayek et al., 2019). Four weeks of moderate physical exercise in adult female mice raised hippocampal FNDC5/irisin levels and elicited an antidepressant like effect in these mice (Gruhn et al., 2021). The same treadmill training protocol promoted hippocampal cell proliferation, neuronal differentiation, and cell survival, while increasing hippocampal levels of FNDC5 of female mice (Siteneski et al., 2020). However, again BDNF SNP Val66Met (BDNFMet/Met) inhibits the beneficial effects of exercise-induced increase in the hippocampal mRNA levels of FNDC5 and BDNF (Ieraci et al., 2016). A recent review suggests that the disruption of the endogenous BDNF activity such as the Val66Met BDNF variant potentiates sensitivity to stress and stress-inducible disorders. The authors argue regulators of BDNF may orchestrate sensitivity to stress, and trauma (Notaras and van den Buuse, 2020). For example, in mice exposed to chronic social defeat stress, overexpression of the PGC-1 alpha/FNDC5/BDNF signaling pathway in skeletal muscles contributes to mice’s resilience to stress (Zhan et al., 2018). Interestingly, exercise also causes bones to secrete osteocalcin, an endocrine hormone that activates the G Protein-Coupled Receptor, GPR158, increasing the levels of irisin, and in turn, BDNF in the brain (Khrimian et al., 2017; Nicolini et al., 2020). Of note, the genetic deletion of osteocalcin leads to increased depressive-like behaviors in mice (Oury et al., 2013).
Inflammatory processes are also involved in the complex interplay of pathways conferring PE’s positive effects on depression. Numerous lines of clinical and translational research demonstrate the link between depression and heightened immune activation through increased numbers of proinflammatory cytokines such as IL-6, and TNF-α (Raison et al., 2006; Nobis et al., 2020). On the other hand, physical exercise has been shown to reduce pro-inflammatory responses through increased expression of the transcriptional activator PGC1 alpha (Peroxisome proliferator-activated receptor gamma coactivator 1-alpha) (Handschin and Spiegelman, 2008). In line with this, it was demonstrated that moderate-intensity exercise can lower the proinflammatory cytokine TNF- α blood levels, and reduce depression scores (Paolucci et al., 2018).
Another hypothesized system to benefit from the effects of exercise on MDD is monoamines. Historically, the monoaminergic system was targeted for treatment interventions as it was consistently found that a depressed state was caused by an insufficient supply of the monoamine neurotransmitters serotonin, norepinephrine, and dopamine in the central nervous system (Boku et al., 2018). Exercise is known to restore the levels of these three main monoamine neurotransmitters dopamine, norepinephrine, and serotonin (Lin and Kuo, 2013), hence contributing to exercise’s anti-depressive effects.
Possible sex differences in exercise efficacy on brain health
Few studies on exercise and brain health have specifically addressed sex differences, and it is still not known whether the postulated mechanisms underlying the beneficial effects of exercise on the CNS differ between sexes. Barha and colleagues conducted a systematic review on aerobic exercise and found that the positive effects on cognitive functions, including decision making, were directly related to the number of female participants in the study. This suggests that certain beneficial outcomes of exercise are sex-specific (Barha et al., 2017). Similarly, a controlled trial by Baker and colleagues found major sex differences in the response to aerobic exercise in older adults with mild cognitive impairment (MCI). Specifically, the results revealed that females in the aerobic exercise group had increased BDNF serum levels and exhibited significant improvements in cognitive function compared to the control group. To the contrary, there were no significant changes in BDNF levels or cognitive function in males within the aerobic exercise group. These sex differences imply that aerobic exercise have a more positive impact on cognitive performance in females with MCI compared to males (Baker et al., 2010). As previously mentioned, aerobic exercise improves brain health by enhancing neural plasticity, and hippocampal neurogenesis, which is also influenced by sex differences. For example, one study found that adult female and male rodents show differences in neurogenesis following spatial training. Male rats outperformed female rats in the spatial test, and the training increased the quantity of BrdU-labeled cells in male rats but not in female rats (Chow et al., 2013). Furthermore, BDNF protein levels vary during the estrus cycle in rodents and the menstrual cycle in humans, as higher estradiol levels are associated with higher BDNF expression. BDNF and estrogen have been linked to various neurological and psychiatric disorders, which may have an impact on sex differences and disorders such as addiction (Harte-Hargrove et al., 2013). Indeed, a randomized controlled trial showed that a 6-month aerobic exercise training leads to higher serum levels of BDNF in females aged 55 years and older with mild cognitive impairment but not in males of the same age range (Barha et al., 2017). This suggests that the physiological response to aerobic training is not the same in males and females, making it crucial to move beyond the “one size fits all” approach and consider sex differences in exercise efficacy in developing efficient and personalized exercise interventions to promote a healthy brain.
The influence of exercise on these various biological processes lends support to the neuroprotective and anti-depressive properties of exercise. As such, exercise represents an important potential mediator of depression and future research should focus on the interactions of “exerkines” with the known underlying neurobiology of major depression.
The role of extracellular vesicles in the systemic adaptation to exercise
As described above, exercise’s health benefits are partially attributed to the plethora of bioactive molecules released of exerkines or exercise factors into the bloodstream. Aside from the traditional peptide secretion pathway, little is known about how these factors are delivered in circulation from their tissue of origin to close-by or distal destinations. Extracellular vesicles represent a possible delivery mechanism as they have been found to carry exerkine-type molecules including different RNA species, proteins, and metabolites (Chow et al., 2022). Extracellular vesicles can be classified into different subpopulations based on their biogenesis, and properties such as size, protein composition, miRNA content, and cellular origin (van Niel et al., 2018). The three main types of EVs are: small extracellular vesicles (sEVs), microvesicles, and apoptotic bodies (Gheinani et al., 2018). sEVs (40–200 nm) are the smallest population of EVs, they originate from the inward luminal budding of the membrane, followed by the formation of multivesicular bodies and fusion with the plasma membrane which leads to the release of these sEVs into the extracellular space (Lee et al., 2012). Microvesicles (50–1,000 nm) originate from the direct budding off the plasma membrane, to deliver cargo to recipient cells (Lee et al., 2012). Finally, apoptotic bodies (500–2000 nm), are membrane-bound vesicles that originate from the disassembly of apoptotic cells (Xu et al., 2019).
With just less than a decade of research exploring the relationship between exercise and sEVs, the field is still in its infancy, and we are only starting to elucidate its role in exercise. However, skeletal muscle has been extensively studied as an endocrine organ, and it is becoming increasingly clear that skeletal muscles release myokines and exerkines (IL-6, IL-15, myostatin, BDNF, and muscle-specific microRNAs) in response to muscle contractions (Vechetti et al., 2021). There is evidence that exercise’s beneficial effects on mood are partially mediated through skeletal muscle-brain communication via myokines such as IL-1β, IL-6, TNF-α, and IL-10 (cytokines released by muscle tissue) (Mucher et al., 2021). Exercise-induced myokines are taken up by other tissues including the brain where they regulate several functions, including mood, memory, locomotion, and neuronal injury protection, demonstrating muscle-brain cross-talk (Scisciola et al., 2021). Following different high and moderate intensity exercise protocols, it was demonstrated that moderate intensity exercise can temporarily elicit both pro- and anti-inflammatory temporary responses in the blood (Cerqueira et al., 2019). For example, in otherwise healthy college students, moderate-intensity exercise was optimal for improving depressive symptoms which appears to be mediated through a decrease in TNF-α levels in the blood (Paolucci et al., 2018). On the other hand, in individuals with treatment-resistant depression, a rise in TNF-α levels was positively correlated with the antidepressant effect of a 12-week aerobic exercise (Rethorst et al., 2013).
Recently, the mechanisms through which skeletal muscle operates as an endocrine organ have been broadened to include sEVs (Rome et al., 2019). In vivo research on sEVs originating from skeletal muscle is challenging due to the current inability to specifically label and monitor sEVs released from this tissue. However, multiple in vitro studies have clearly shown that myoblasts and myotubes release proteins using sEVs (Forterre et al., 2014; Choi et al., 2016). Muscle-derived sEVs have been shown to serve as a cell-free therapeutic approach for muscle regeneration, a biological cue for stem cell differentiation (Choi et al., 2016), as well as, a modulator of metabolism (Takafuji et al., 2020).
In the context of EV cargo, D’souza and colleagues demonstrated that specific populations of miRNAs from sEVs in muscles, plasma, and circulation (Table 1) increased significantly in ten healthy young men (18–35 years old) following high-intensity exercise in the form of cycling (D’Souza et al., 2018). Likewise, comparing acute exercise in active vs. inactive men on a cycle ergometer for 40 min, resulted in 7 differentially expressed miRNAs immediately post-exercise and 8 miRNAs 3 h post-exercise. The miRNA signature post-acute exercise was shown to be correlated with the activation of IGF-1 signaling in the exercise group (Nair et al., 2020). In rats, acute aerobic treadmill training for 40 min/day for 1 week resulted in the differential expression of twelve miRNAs and a concurrent rise in serum sEV concentration (Oliveira et al., 2018).
In a very intriguing study by Bei and colleagues, exercise-induced sEVs were injected into sedentary mice at an exercise-relevant concentration resulting in a significant reduction in the infarct size in vivo, and cardiomyocyte apoptosis in vitro. The authors found an enhancement in the cardioprotective effect against acute ischemia/reperfusion injury in mice driven by sEV (Bei et al., 2017). Another study showed that intravenous injection of exercise-induced sEVs reduced tumor volume by 35% in a rat model of metastatic prostate cancer (Sadovska et al., 2022). Several other studies show promise for the use of extracellular vesicles in clinical applications both as biomarkers and as therapeutic delivery vehicles (O’Brien et al., 2020). According to a study by Pierdoná and colleagues, obese and sedentary adolescents (15–16 years old) that respond to acute aerobic exercise produce larger-sized plasma EVs with a higher protein yield (Pierdoná et al., 2022).
Future treatments for obesity and type 2 diabetes mellitus (T2DM) may use modified small extracellular vesicles loaded with exerkines, which have been shown to reduce obesity and T2DM and positively affect metabolic health (Safdar et al., 2016). These results confirm the notion that aerobic exercise does indeed influence miRNA expression in sEVs, and that sEVs play a key role in mediating the systemic beneficial effects of exercise. However, more studies investigating exercise-related factors such as intensity, type, and frequency, are needed for a better understanding of the link between exercise and sEVs.
The data summarized in Table 1 and Table 2 represent recent trials evaluating the circulating EV patterns following physical exercise in humans, and rodents, respectively. Nanoparticle tracking analysis (NTA) of plasma EVs showed increased concentration in human male subjects that underwent incremental cycle ergometry, intense treadmill running. Post-exercise plasma analysis showed a 5.2-fold surge in sEV markers: FLOT1 and HSP/Hsc70 (Frühbeis et al., 2015). Additionally, an exercise stress test caused a rapid increase in plasma EVs in human subjects (Bei et al., 2017).
However, a similar study showed no significant alterations in NTA particle size or number following a cycling ergometer test in the blood; but there was an increase in several sEV markers including CD9, CD63, and CD81 (Brahmer et al., 2019). In animal studies, aerobic exercise causes an increase in BDNF and CD63 EV levels but does not alter EV size or concentration in aged rats (Barcellos et al., 2020). Acute aerobic treadmill exercise in rats was found to increase EV concentration in the blood and alter miRNA cargo that targets the MAPK pathway (Oliveira et al., 2018). Likewise, a systemic analysis of 19 in vivo and ex vivo studies concluded that aerobic exercise increases sEV markers (Alix, CD63, CD81, and Flot-1), and stimulates their release into circulation without changing its vesicle size or concentration (Estébanez et al., 2021). These results imply that physical exercise has more influence on sEV content and concentration, rather than size (Figure 2).
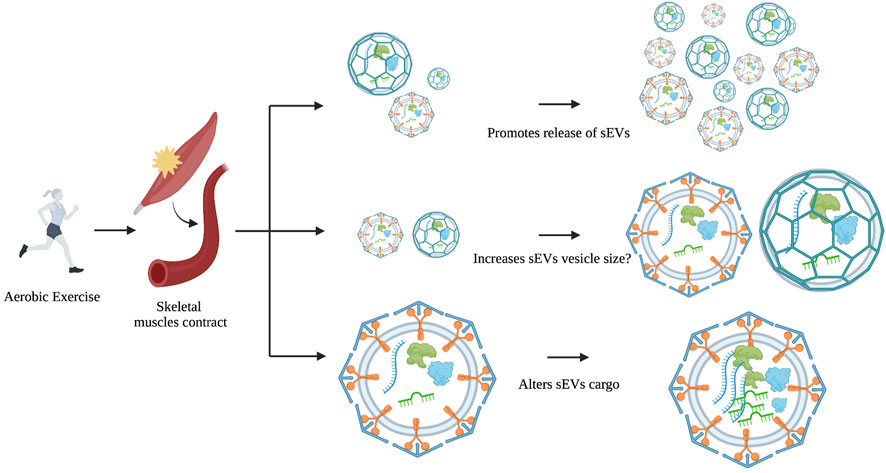
FIGURE 2. The possible effects of exercise on EV size, concentration, and cargo. During or after physical exercise, skeletal muscles release sEVs into the bloodstream. Evidence to date suggests that exercise is more likely to affect cargo and concentration and not size.
Extracellular vesicles and major depressive disorder
Circulating EVs and particularly the smaller subtype, have garnered a lot of attention as a potential diagnostic tool in psychiatric disorders (Kano et al., 2019). Intercellular communication mediated by sEVs has been linked to neurogenesis (Luarte et al., 2017), neuronal plasticity (Lafourcade et al., 2016), neuroinflammation (Dalvi et al., 2017), and transcriptional regulation (Ung et al., 2014). Alterations to the above-mentioned neurobiological processes have previously been implicated in MDD, which has led researchers to hypothesize that sEVs might be involved in the pathology of MDD. Multiple studies suggest that sEVs carry miRNAs throughout the body (Tian et al., 2017; Liu et al., 2019; Munir et al., 2020), and most of the miRNA targets are associated with different pathologic pathways in depression (Gruzdev et al., 2019).
The effect of sEVs on neurogenesis
While still controversial, some studies have shown that adult hippocampal neurogenesis (AHN) is disrupted in individuals with depression (Kim & Park, 2021) with studies pointing to specific miRNAs as contributors to this disruption (Ortega et al., 2021). In this context, certain miRNAs found in sEVs released by astrocytes were found to be elevated (miR-9, miR-26b, mi-29a, miR-125b, miR-145, and miR-543) and others, downregulated (miR-26a, miR-221), in stressful circumstances. Notably, these miRNAs have been implicated in regulating neuronal differentiation and proliferation by targeting different molecular targets such as Notch signaling genes, and BMP/TGFβ signaling genes (Luarte et al., 2017). Likewise, others have shown BDNF, VEGF, Notch1, miR-124, and miR-9 — molecules known to regulate neurogenesis—have been identified as cargo present in sEVs from various peripheral tissues (Zhang and Xu, 2022). Serum and sEV BDNF levels measured by ELISA were found to be decreased in depressed patients compared to healthy controls (Dwivedi, 2009; Gelle et al., 2021). Intranasal administration of EVs from neural stem cells (NSCs) derived from human induced pluripotent stem cells into adult rats and mice resulted in the incorporation of EVs by neurons, microglia, and astrocytes in all brain regions and promoted hippocampal neurogenesis via several enriched proteins such as Agrin and pentraxin-3 (Upadhya et al., 2020). This suggests that proteins from EVs play a role in increasing neurogenesis, which might be disrupted in individuals with MDD (Saeedi et al., 2019). On the other hand, injecting sEVs containing amyloid precursor protein, a cargo considered to be pathogenic, into the dentate gyrus of mice, weakened adult hippocampal neurogenesis (Zheng et al., 2017). Genome-wide miRNA profiling of blood-derived sEVs from individuals with MDD and healthy controls showed an upregulation of miR-139-5p expression in peripheral sEVs in individuals with depression. Injection of a miR-139-5p antagomir rescued depressive-like symptoms and increased hippocampal neurogenesis in stressed mice (Wei et al., 2020). Serum sEV-derived miR-139-5p was found to be one of the most differentially expressed miRNAs between MDD and healthy patients by Liang and colleagues (Liang et al., 2020). Inhibition of miR-139-5p plays an antidepressant-like role by enhancing the levels of nuclear receptor subfamily 3 group C member 1 (NRC1), BDNF, and the phosphorylated/total tyrosine kinase receptor B (p-TrKB/TrKB) (Su et al., 2022). Taken together, these studies suggests that sEVs are implicated in promoting or inhibiting adult hippocampal neurogenesis, and thus, potentially influencing the depressive state.
The effect of sEVs on synaptic plasticity
sEVs released from depolarized neurons have been found to regulate synaptic plasticity. For example, proteomic analysis of neuron-derived sEVs found the synaptic-plasticity-associated protein MAP1b to be enriched in these vesicles (Goldie et al., 2014). Specifically, proteins such as transmembrane protein proline-rich 7 (PRR7) are secreted by neurons by sEVs, and are taken up by a recipient cell, resulting in the elimination of excitatory synapses and maintaining normal synapse count (Huo et al., 2021). Neuronal sEVs have been shown to influence synaptic pruning by microglia by increasing the microglial expression of complement component 3 (Bahrini et al., 2015). These findings support sEVs as regulators of synaptic pruning, hence influencing synaptic plasticity.
The effect of sEVs on inflammation
There is evidence that sEVs are regulators of neuroinflammation, for example, monocytes activated by interferon-alpha secrete sEVs carrying altered miRNA profiles, subsequently activating the nuclear factor-κB, and increasing the inflammatory response (Dalvi et al., 2017). Likewise, microglia cells can release sEVs that carry IL-1- β, caspase-1, and P2X7 receptors which induce neuroinflammation (Frühbeis et al., 2013). sEV protein cargo from human cerebral microvascular endothelial cell culture is changed in response to the inflammatory cytokine tumor necrosis factor (TNF) (Dozio & Sanchez, 2017). Using serum sEVs from immune-challenged mice, recipient animals showed increased microglial activation with augmented CNS expression of pro-inflammatory cytokine mRNA and associated miRNA (Li et al., 2018). Li and colleagues demonstrated that natural killer (NK) cell derived sEVs carry miR-207 that rescued depressive-like behaviors in mice through the targeting of TLR4-interactor with leucine-rich repeats (Tril) and by decreasing the levels of IL-1β, IL-6, and TNF-α released by astrocytic cells in culture (Li et al., 2020). Several studies have also demonstrated the relationship between sEVs and neuroinflammation in depression (Li et al., 2020; Wang et al., 2021; Duarte-Silva et al., 2022; Xie et al., 2023). Protein profiling of peripherally sourced neural-derived sEVs using ELISA revealed higher levels of IL34/CD81 in MDD compared to the healthy controls (Kuwano et al., 2018).
Gut inflammation has been related to the development of various mental diseases, including anxiety and depression (Clapp et al., 2017). Recently, the microbiome has gained considerable attention for its relationship to neuropsychiatric disorders including depression. For example, Rhee and colleagues, found that MDD patients carry more DNA from specific bacteria genera (Prevotella 2 and Ruminococcaceae UCG-002) in their bacteria-derived sEVs from serum (Rhee et al., 2020).
In a review article by Soares et al. (2021), the researchers propose a captivating hypothesis suggesting the effects of exercise on MDD are mediated through altered concentration and cargo profiles of circulating extracellular vesicles capable of modifying inflammatory signals throughout the body. The authors examine clinical studies focusing only on the relationship between PE and MDD, EVs and MDD, as well as PE and EVs. Additionally, they present a wide range of potential biomarkers, including brain-derived biomarkers (GFAP, α-synuclein, glutamine synthetase), exerkines (myostatin, myonectin), neurotrophic factors (BDNF, nerve growth factor (NGF)), miRNAs, and stress and inflammation signaling mediators (interleukins/cytokines, C reactive protein (CRP)), that hold promise in the field of exercise and depression research (Soares et al., 2021).
The journey of sEVs from peripheral tissues to the brain
sEVs must cross the vascular BBB to functionally communicate between the CNS and the periphery. The exact molecular mechanisms regulating EV passage across the BBB remain unclear.
sEVs follow a complex journey from being secreted by parental cells in the periphery to crossing the blood-brain barrier and infiltrating into the brain (Matsumoto et al., 2017). Understanding EV trafficking is important for elucidating the underlying crosstalk between tissues integral to exercise’s beneficial effects on MDD. Table 1 shows that different aerobic exercise protocols induce a change in EV cargo in the bloodstream, and/or to a lesser extent, the rapid increase in EV concentration (Table 1). In the brain, the concentration of sEVs significantly increases after exercise (Zhang et al., 2021), either due to increased passage through the blood-brain barrier or as a direct consequence of exercise on the brain and local release of EVs. The exact mechanisms involved in transporting sEVs across the BBB are not yet fully understood. However recent research has provided some evidence showing that sEVs can indeed cross the BBB (Fowler, 2019). For example, Alvarez-Erviti et al. demonstrated that intravenously injected sEVs can deliver short interfering RNA to the brain in mice, specifically targeting neurons, microglia, and oligodendrocytes, leading to a specific gene knockdown (Alvarez-Erviti et al., 2011). Different in vitro models have been used to investigate the mechanism of sEV migration through the BBB (Ramos-Zaldívar et al., 2022). Using human brain microvascular endothelial cells (BMECs) transwell assays, Chen et al. (2016) showed that luciferase carrying sEVs have the ability to cross the BMEC layer under inflamed conditions. Morad et al. (2019) demonstrated that cancer-derived EVs can breach the BBB in vivo through transcytosis (across the interior of the cell) via decreasing the expression of rab7 in brain endothelial cells and enhancing their transport.
In vivo and in vitro studies have shown that peripheral sEVs interact with the BMECs of the BBB under stroke-like conditions, and enter the brain via different mechanisms such as: micropinocytosis, receptor-mediated transcytosis, and adsorptive-transcytosis (Chen et al., 2016; Heidarzadeh et al., 2021). Morad et al. (2019) monitored the distribution of brain-seeking EVs (Br-EVs) in a zebrafish model to explore the transcytosis mechanism in the brain via live imaging. Br-EVs were taken up by cells of the brain parenchyma, showing that Br-EVs can cross the BBB. Time-lapse imaging also showed movement of these vesicles towards the plasma membrane where it fused with the membrane, indicative of a transcytosis mechanism (Morad et al., 2019).
Given that, crossing the blood-brain barrier facilitates the connection between the brain and the periphery, sEVs play a critical role in some of the systemic alterations reported in neuropsychiatric disorders (Saeedi et al., 2019). In individuals with MDD, the BBB is disrupted, and neuroinflammation has been proposed as the potential cause of this disruption (Wu et al., 2022) (Figure 3). There is a significant increase in the infiltration of cells into the brain of individuals with MDD as cytokines increase the BBB’s permeability (Cheng et al., 2018). Interestingly, inflammation of the BBB has been demonstrated to increase EV passage by five folds (Chen et al., 2016). However, sEVs are not just a connection between the periphery and the brain in neuropsychiatric and neurological disorders, they also provide information on the changing processes of the CNS through brain-derived EVs present in circulation. For instance, glioblastoma-specific mRNA has been discovered in circulating sEVs and has been proposed as a diagnostic biomarker (Skog et al., 2008). Several studies have found alterations in sEV cargo, particularly miRNAs in individuals with MDD that target neuroinflammation, synaptic plasticity, and neurogenesis. Dysregulation of various miRNAs such as miR-223, miR-451, miR-182, and miR-16 is linked to MDD (Gururajan et al., 2016), supporting the role of sEVs as intercellular communicators, and potential mediators in the pathogenesis of neuropsychiatric disorders such as depression.
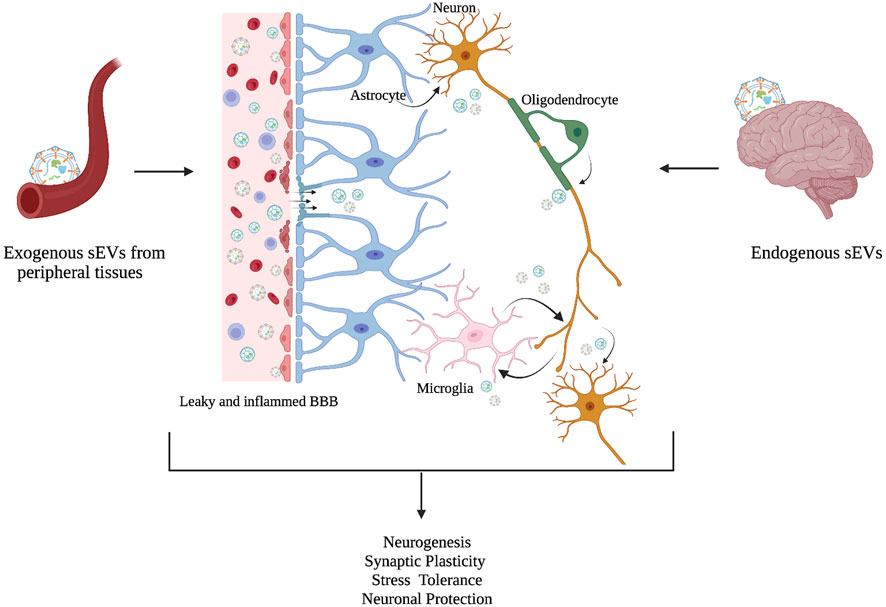
FIGURE 3. sEVs as intercellular mediators between the periphery and the brain. Although the molecular mechanism is not fully understood, an increasing number of studies suggest that exogenous sEVs from peripheral tissues cross BBB, and act as intercellular communicators, along with endogenous sEVs to regulate physiological processes in the brain such as neurogenesis, synaptic plasticity, stress tolerance, and neuronal protection. Changes in these physiological processes have been associated in MDD. A leaky and inflamed BBB is linked with MDD pathology in the brain (Adapted from Saeedi et al., 2019).
More research is needed to fully understand the underlying mechanisms of the bi-directional transfer of sEVs across the BBB, particularly as brain-derived sEVs may reflect physiological changes in psychiatric disorders.
The link between physical exercise, small extracellular vesicles, and major depressive disorder
Exercise has been identified as an effective preventative and treatment strategy for a variety of neuropsychiatric disorders (Smith and Merwin, 2021). However, the use of exercise as a treatment remains elusive due to the paucity of information on the underlying neurobiological processes, and the lack of knowledge on the metabolic crosstalk between exercise-induced peripheral changes and the brain in MDD. Current literature suggests that aerobic exercise can ease symptoms of depression via several neural mechanisms (Xie et al., 2021), as well as modulate circulating sEVs and their RNA and protein content which is important for intercellular communication for exercise-induced adaptations (Nederveen et al., 2021). Exercise might increase plasma sEV levels by releasing them from peripheral tissues such as skeletal muscles or change its cargo signature, principally RNA and protein (Bei et al., 2017; Oliveira et al., 2018). Subsequently, exercise-induced sEVs, circulate to other tissues such as the brain to regulate specific cell responses by increasing nerve cell growth, regulating inflammation (Fuller et al., 2020), and possibly modulating depressive symptoms in individuals with MDD (Figure 4). The cargo of circulating extracellular vesicles post-exercise include different exerkines such as: miRNA (miR-139-5p, miR-206, and miR-207), neurotrophic factors (BDNF, VEGF, TrkA, and TrkB), and cytokines (IL-1β, IL-6, and TNF-α). Identifying and assessing these markers in circulating sEVs may provide a more comprehensive view of the signaling mechanisms necessary for the beneficial effects of exercise on MDD (Table 3). Although there is no research showing the role of exercise-induced sEVs in the antidepressant effects of exercise, the combined evidence presented here indeed supports the possibility of this relationship. Taken together, these studies suggest that the beneficial effects of exercise on MDD can be mediated by sEVs through their capacity to regulate processes such as synaptic plasticity, neuroinflammation, and neurogenesis.
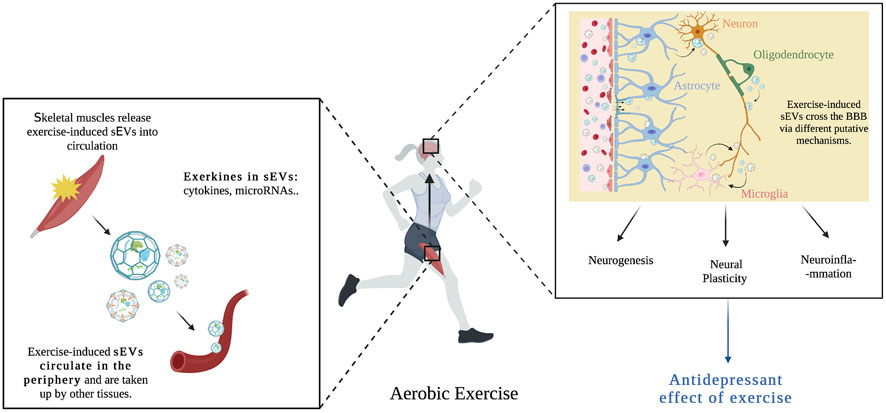
FIGURE 4. Aerobic exercise causes skeletal muscles to release exercise-induced sEVs into blood circulation. These sEVs contain important exerkines (cytokines, microRNAs) and are taken up by other tissues such as the brain. Exercise-induced sEVs cross the BBB via different putative mechanisms (transcytosis, micropinocytosis, and clathrin-dependent endocytosis), and influence neural pathways associated with the etiopathogenesis of major depression, such as neurogenesis, neural plasticity and neuroinflammation, hence mediating the beneficial effects of exercise on MDD.
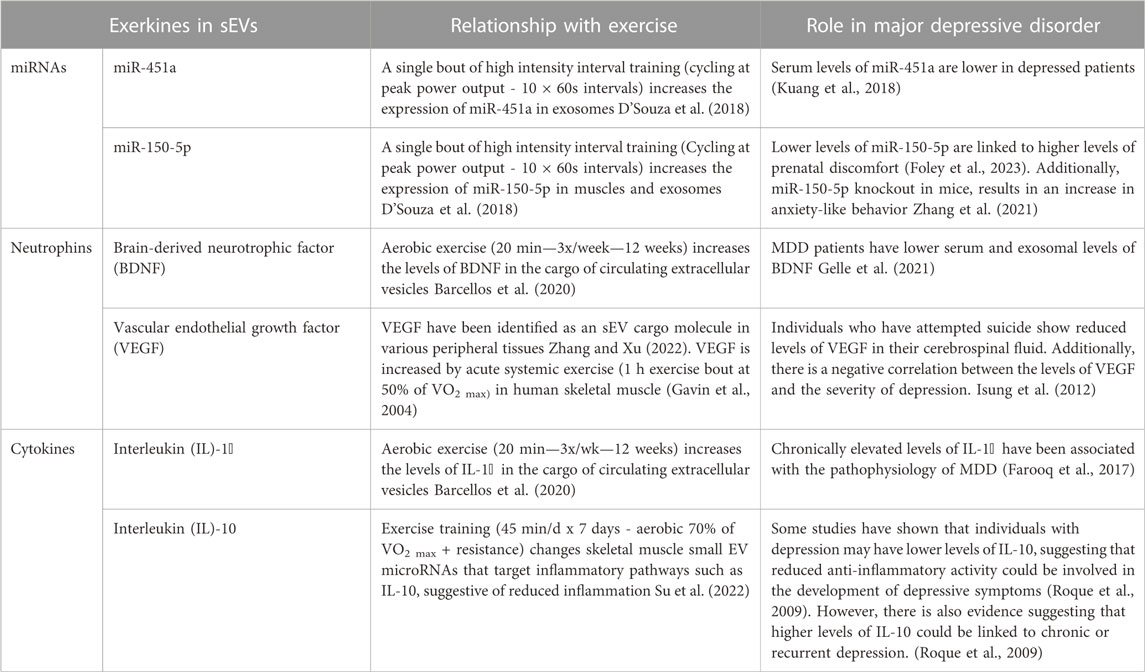
TABLE 3. Potential role of exerkines encapsulated in small EVs and their relationship with major depressive disorder.
Conclusion and future directions
Aerobic exercise is a promising and evidence-based treatment for MDD, and the discovery that exercise-induced sEVs can promote intercellular communication by delivering cargo, acting on neighboring and distant cells, promoting healthier physiological status is an important new advance in the field of exercise. Knowing that both, the effects of exercise and the prevalence of MDD are influenced by sex, sex as a biological variable should be taken into consideration in future studies. The role of sEVs in the context of exercise and depression has yet to be investigated, however, based on the evidence summarized here, we identify common biological features between exercise and depression, exercise and sEVs, and sEVs and depression. We suggest that exercise’s effect on depression is mediated by altered peripheral sEV cargo signatures that influence neural pathways associated with the etiopathogenesis of major depression, such as neural plasticity and neuroinflammation. Future studies, including examining the effects of exercise-induced sEV cargo on depression and better understanding the mechanisms of bi-directional sEV transfer, as well as investigating the extent and type of sEVs released in different types, intensity, duration, and frequency of exercise, would provide important missing links across all three research domains.
Author contributions
RK wrote, revised and edited the original draft of the review article. CN wrote, revised and edited the review article. All authors contributed to the article and approved the submitted version.
Funding
CN is supported by grants from the Canadian Institute of Health Research CIHR (PJT-183904) and the Natural Sciences and Engineering Research Council of Canada NSERC (RGPIN-2022-03979).
Conflict of interest
The authors declare that the research was conducted in the absence of any commercial or financial relationships that could be construed as a potential conflict of interest.
Publisher’s note
All claims expressed in this article are solely those of the authors and do not necessarily represent those of their affiliated organizations, or those of the publisher, the editors and the reviewers. Any product that may be evaluated in this article, or claim that may be made by its manufacturer, is not guaranteed or endorsed by the publisher.
References
Albert, P. R. (2015). Why is depression more prevalent in women? J. Psychiatry & Neurosci. 40 (4), 219–221. doi:10.1503/jpn.150205
Alemi, F., Min, H., Yousefi, M., Becker, L. K., Hane, C. A., Nori, V. S., et al. (2021). Effectiveness of common antidepressants: A post market release study. EClinicalMedicine 41, 101171. doi:10.1016/j.eclinm.2021.101171
Alvarez-Erviti, L., Seow, Y., Yin, H., Betts, C., Lakhal, S., and Wood, M. J. A. (2011). Delivery of siRNA to the mouse brain by systemic injection of targeted exosomes. Nat. Biotechnol. 29 (4), 341–345. doi:10.1038/nbt.1807
Bahrini, I., Song, J., Diez, D., and Hanayama, R. (2015). Neuronal exosomes facilitate synaptic pruning by up-regulating complement factors in microglia. Sci. Rep. 5 (1), 7989. doi:10.1038/srep07989
Baker, L. D., Frank, L. L., Foster-Schubert, K., Green, P. S., Wilkinson, C. W., McTiernan, A., et al. (2010). Effects of aerobic exercise on mild cognitive impairment: A controlled trial. Archives Neurology 67 (1), 71–79. doi:10.1001/archneurol.2009.307
Bangasser, D. A., and Cuarenta, A. (2021). Sex differences in anxiety and depression: Circuits and mechanisms. Nat. Rev. Neurosci. 22 (11), 674–684. doi:10.1038/s41583-021-00513-0
Barcellos, N., Cechinel, L. R., de Meireles, L. C. F., Lovatel, G. A., Bruch, G. E., Carregal, V. M., et al. (2020). Effects of exercise modalities on BDNF and IL-1β content in circulating total extracellular vesicles and particles obtained from aged rats. Exp. Gerontol. 142, 111124. doi:10.1016/j.exger.2020.111124
Barha, C. K., Davis, J. C., Falck, R. S., Nagamatsu, L. S., and Liu-Ambrose, T. (2017). Sex differences in exercise efficacy to improve cognition: A systematic review and meta-analysis of randomized controlled trials in older humans. Front. Neuroendocrinol. 46, 71–85. doi:10.1016/j.yfrne.2017.04.002
Bei, Y., Xu, T., Lv, D., Yu, P., Xu, J., Che, L., et al. (2017). Exercise-induced circulating extracellular vesicles protect against cardiac ischemia–reperfusion injury. Basic Res. Cardiol. 112 (4), 38. doi:10.1007/s00395-017-0628-z
Belvederi Murri, M., Ekkekakis, P., Magagnoli, M., Zampogna, D., Cattedra, S., Capobianco, L., et al. (2019). Physical exercise in major depression: Reducing the mortality gap while improving clinical outcomes. Front. Psychiatry 9, 762. doi:10.3389/fpsyt.2018.00762
Belzeaux, R., Lin, R., and Turecki, G. (2017). Potential use of MicroRNA for monitoring therapeutic response to antidepressants. CNS Drugs 31 (4), 253–262. doi:10.1007/s40263-017-0418-z
Bertoldi, K., Cechinel, L. R., Schallenberger, B., Corssac, G. B., Davies, S., Guerreiro, I. C. K., et al. (2018). Circulating extracellular vesicles in the aging process: Impact of aerobic exercise. Mol. Cell. Biochem. 440 (1), 115–125. doi:10.1007/s11010-017-3160-4
Block, S. G., and Nemeroff, C. B. (2014). Emerging antidepressants to treat major depressive disorder. Asian J. Psychiatry 12, 7–16. doi:10.1016/j.ajp.2014.09.001
Blumenthal, J. A., Babyak, M. A., Doraiswamy, P. M., Watkins, L., Hoffman, B. M., Barbour, K. A., et al. (2007). Exercise and pharmacotherapy in the treatment of major depressive disorder. Psychosom. Med. 69 (7), 587–596. doi:10.1097/PSY.0b013e318148c19a
Boku, S., Nakagawa, S., Toda, H., and Hishimoto, A. (2018). Neural basis of major depressive disorder: Beyond monoamine hypothesis. Psychiatry Clin. Neurosci. 72 (1), 3–12. doi:10.1111/pcn.12604
Bozzo, L., Puyal, J., and Chatton, J.-Y. (2013). Lactate modulates the activity of primary cortical neurons through a receptor-mediated pathway. PloS One 8 (8), e71721. doi:10.1371/journal.pone.0071721
Brahmer, A., Neuberger, E., Esch-Heisser, L., Haller, N., Jorgensen, M. M., Baek, R., et al. (2019). Platelets, endothelial cells and leukocytes contribute to the exercise-triggered release of extracellular vesicles into the circulation. J. Extracell. Vesicles 8 (1), 1615820. doi:10.1080/20013078.2019.1615820
Carter, T., Guo, B., Turner, D., Morres, I., Khalil, E., Brighton, E., et al. (2015). Preferred intensity exercise for adolescents receiving treatment for depression: A pragmatic randomised controlled trial. BMC Psychiatry 15 (1), 247. doi:10.1186/s12888-015-0638-z
Castaño, C., Mirasierra, M., Vallejo, M., Novials, A., and Párrizas, M. (2020). Delivery of muscle-derived exosomal miRNAs induced by HIIT improves insulin sensitivity through down-regulation of hepatic FoxO1 in mice. Proc. Natl. Acad. Sci. U. S. A. 117 (48), 30335–30343. doi:10.1073/pnas.2016112117
CCA (2017). 5 ways aerobic and anaerobic exercises are different, and why you need both. Available at: https://chiropractic.ca/blog/5-ways-aerobic-and-anaerobic-exercises-are-different-and-why-you-need-both/.
Cerqueira, É., Marinho, D. A., Neiva, H. P., and Lourenço, O. (2019). Inflammatory effects of high and moderate intensity exercise-A systematic review. Front. Physiology 10, 1550. doi:10.3389/fphys.2019.01550
Chen, B., Dowlatshahi, D., MacQueen, G. M., Wang, J. F., and Young, L. T. (2001). Increased hippocampal BDNF immunoreactivity in subjects treated with antidepressant medication. Biol. Psychiatry 50 (4), 260–265. doi:10.1016/s0006-3223(01)01083-6
Chen, C. C., Liu, L., Ma, F., Wong, C. W., Guo, X. E., Chacko, J. V., et al. (2016). Elucidation of exosome migration across the blood-brain barrier model in vitro. Cell. Mol. Bioeng. 9 (4), 509–529. doi:10.1007/s12195-016-0458-3
Cheng, Y., Desse, S., Martinez, A., Worthen, R. J., Jope, R. S., and Beurel, E. (2018). TNFα disrupts blood brain barrier integrity to maintain prolonged depressive-like behavior in mice. Brain, Behav. Immun. 69, 556–567. doi:10.1016/j.bbi.2018.02.003
Choi, J. S., Yoon, H. I., Lee, K. S., Choi, Y. C., Yang, S. H., Kim, I.-S., et al. (2016). Exosomes from differentiating human skeletal muscle cells trigger myogenesis of stem cells and provide biochemical cues for skeletal muscle regeneration. J. Control. Release 222, 107–115. doi:10.1016/j.jconrel.2015.12.018
Choi, K. W., Chen, C.-Y., Stein, M. B., Klimentidis, Y. C., Wang, M.-J., Koenen, K. C., et al. (2019). Assessment of bidirectional relationships between physical activity and depression among adults: A 2-sample mendelian randomization study. JAMA Psychiatry 76(4), 399–408. doi:10.1001/jamapsychiatry.2018.4175
Chow, C., Epp, J. R., Lieblich, S. E., Barha, C. K., and Galea, L. A. M. (2013). Sex differences in neurogenesis and activation of new neurons in response to spatial learning and memory. Psychoneuroendocrinology 38 (8), 1236–1250. doi:10.1016/j.psyneuen.2012.11.007
Chow, L. S., Gerszten, R. E., Taylor, J. M., Pedersen, B. K., van Praag, H., Trappe, S., et al. (2022). Exerkines in health, resilience and disease. Nat. Rev. Endocrinol. 18 (5), 273–289. doi:10.1038/s41574-022-00641-2
Clapp, M., Aurora, N., Herrera, L., Bhatia, M., Wilen, E., and Wakefield, S. (2017). Gut microbiota’s effect on mental health: The gut-brain axis. Clin. Pract. 7 (4), 987. doi:10.4081/cp.2017.987
Cooper, C., Moon, H. Y., and van Praag, H. (2018). On the run for hippocampal plasticity. Cold Spring Harb. Perspect. Med. 8 (4), a029736. doi:10.1101/cshperspect.a029736
Cotman, C. W., Berchtold, N. C., and Christie, L.-A. (2007). Exercise builds brain health: Key roles of growth factor cascades and inflammation. Trends Neurosci. 30 (9), 464–472. doi:10.1016/j.tins.2007.06.011
Craft, L. L., and Perna, F. M. (2004). The benefits of exercise for the clinically depressed. Prim. Care Companion J. Clin. Psychiatry 6 (3), 104–111. doi:10.4088/pcc.v06n0301
Dalvi, P., Sun, B., Tang, N., and Pulliam, L. (2017). Immune activated monocyte exosomes alter microRNAs in brain endothelial cells and initiate an inflammatory response through the TLR4/MyD88 pathway. Sci. Rep. 7 (1), 9954. doi:10.1038/s41598-017-10449-0
Dean, J., and Keshavan, M. (2017). The neurobiology of depression: An integrated view. Asian J. Psychiatry 27, 101–111. doi:10.1016/j.ajp.2017.01.025
Dozio, V., and Sanchez, J.-C. (2017). Characterisation of extracellular vesicle-subsets derived from brain endothelial cells and analysis of their protein cargo modulation after TNF exposure. J. Extracell. Vesicles 6 (1), 1302705. doi:10.1080/20013078.2017.1302705
D’Souza, R. F., Woodhead, J. S. T., Zeng, N., Blenkiron, C., Merry, T. L., Cameron-Smith, D., et al. (2018). Circulatory exosomal miRNA following intense exercise is unrelated to muscle and plasma miRNA abundances. Am. J. Physiology. Endocrinol. Metabolism 315 (4), E723–E733. doi:10.1152/ajpendo.00138.2018
Duarte-Silva, E., Oriá, A. C., Mendonça, I. P., de Melo, M. G., Paiva, I. H. R., Maes, M., et al. (2022). Tiny in size, big in impact: Extracellular vesicles as modulators of mood, anxiety and neurodevelopmental disorders. Neurosci. Biobehav. Rev. 135, 104582. doi:10.1016/j.neubiorev.2022.104582
Duman, R. S., and Monteggia, L. M. (2006). A neurotrophic model for stress-related mood disorders. Biol. Psychiatry 59 (12), 1116–1127. doi:10.1016/j.biopsych.2006.02.013
Dwivedi, Y. (2009). Brain-derived neurotrophic factor: Role in depression and suicide. Neuropsychiatric Dis. Treat. 5, 433–449. doi:10.2147/ndt.s5700
El Hayek, L., Khalifeh, M., Zibara, V., Abi Assaad, R., Emmanuel, N., Karnib, N., et al. (2019). Lactate mediates the effects of exercise on learning and memory through SIRT1-dependent activation of hippocampal brain-derived neurotrophic factor (BDNF). J. Neurosci. 39 (13), 2369–2382. doi:10.1523/JNEUROSCI.1661-18.2019
Erickson, K. I., Miller, D. L., and Roecklein, K. A. (2012). The aging hippocampus: Interactions between exercise, depression, and BDNF. Neurosci. A Rev. J. Bringing Neurobiol. Neurology Psychiatry 18 (1), 82–97. doi:10.1177/1073858410397054
Erickson, K. I., Voss, M. W., Prakash, R. S., Basak, C., Szabo, A., Chaddock, L., et al. (2011). Exercise training increases size of hippocampus and improves memory. Proc. Natl. Acad. Sci. U. S. A. 108 (7), 3017–3022. doi:10.1073/pnas.1015950108
Estébanez, B., Jiménez-Pavón, D., Huang, C.-J., Cuevas, M. J., and González-Gallego, J. (2021). Effects of exercise on exosome release and cargo in in vivo and ex vivo models: A systematic review. J. Cell. Physiology 236 (5), 3336–3353. doi:10.1002/jcp.30094
Fakhoury, M., Eid, F., El Ahmad, P., Khoury, R., Mezher, A., El Masri, D., et al. (2022). Exercise and dietary factors mediate neural plasticity through modulation of BDNF signaling. Brain Plast. 8, 121–128. doi:10.3233/BPL-220140
Farooq, R. K., Asghar, K., Kanwal, S., and Zulqernain, A. (2017). Role of inflammatory cytokines in depression: Focus on interleukin-1β. Biomed. Rep. 6 (1), 15–20. doi:10.3892/br.2016.807
Fernandes, J., Vieira, A. S., Kramer-Soares, J. C., Da Silva, E. A., Lee, K. S., Lopes-Cendes, I., et al. (2018). Hippocampal microRNA-mRNA regulatory network is affected by physical exercise. Biochimica Biophysica Acta 1862 (8), 1711–1720. doi:10.1016/j.bbagen.2018.05.004
Foley, H. B., Howe, C. G., Eckel, S. P., Chavez, T., Gevorkian, L., Reyes, E. G., et al. (2023). Depression, perceived stress, and distress during pregnancy and EV-associated miRNA profiles in MADRES. J. Affect. Disord. 323, 799–808. doi:10.1016/j.jad.2022.12.039
Forterre, A., Jalabert, A., Berger, E., Baudet, M., Chikh, K., Errazuriz, E., et al. (2014). Proteomic analysis of C2C12 myoblast and myotube exosome-like vesicles: A new paradigm for myoblast-myotube cross talk? PloS One 9 (1), e84153. doi:10.1371/journal.pone.0084153
Fowler, C. D. (2019). NeuroEVs: Characterizing extracellular vesicles generated in the neural domain. J. Neurosci. 39 (47), 9262–9268. doi:10.1523/JNEUROSCI.0146-18.2019
Franz, S. I., and Hamilton, G. V. (1905). The effects of exercise upon the retardation in conditions of depression. Am. J. Psychiatry 62 (2), 239–256. doi:10.1176/ajp.62.2.239
Frühbeis, C., Fröhlich, D., Kuo, W. P., and Krämer-Albers, E.-M. (2013). Extracellular vesicles as mediators of neuron-glia communication. Front. Cell. Neurosci. 7, 182. doi:10.3389/fncel.2013.00182
Frühbeis, C., Helmig, S., Tug, S., Simon, P., and Krämer-Albers, E.-M. (2015). Physical exercise induces rapid release of small extracellular vesicles into the circulation. J. Extracell. Vesicles 4, 28239. doi:10.3402/jev.v4.28239
Fuller, O. K., Whitham, M., Mathivanan, S., and Febbraio, M. A. (2020). The protective effect of exercise in neurodegenerative diseases: The potential role of extracellular vesicles. Cells 9 (10), 2182. doi:10.3390/cells9102182
Gelle, T., Ra, S., Plansont, B., Bessette, B., Mo, J. M., Lalloué, F., et al. (2021). BDNF and pro-BDNF in serum and exosomes in major depression: Evolution after antidepressant treatment. Prog. Neuro-Psychopharmacology Biol. Psychiatry 109, 110229. doi:10.1016/j.pnpbp.2020.110229
Gheinani, A. H., Vögeli, M., Baumgartner, U., Vassella, E., Draeger, A., Burkhard, F. C., et al. (2018). Improved isolation strategies to increase the yield and purity of human urinary exosomes for biomarker discovery. Sci. Rep. 8, 3945. doi:10.1038/s41598-018-22142-x
Goldie, B. J., Dun, M. D., Lin, M., Smith, N. D., Verrills, N. M., Dayas, C. V., et al. (2014). Activity-associated miRNA are packaged in Map1b-enriched exosomes released from depolarized neurons. Nucleic Acids Res. 42 (14), 9195–9208. doi:10.1093/nar/gku594
Goodwin, R. D. (2003). Association between physical activity and mental disorders among adults in the United States. Prev. Med. 36 (6), 698–703. doi:10.1016/s0091-7435(03)00042-2
Gruhn, K., Siteneski, A., Camargo, A., Freitas, A. E., Olescowicz, G., Brocardo, P. S., et al. (2021). Physical exercise stimulates hippocampal mTORC1 and FNDC5/irisin signaling pathway in mice: Possible implication for its antidepressant effect. Behav. Brain Res. 400, 113040. doi:10.1016/j.bbr.2020.113040
Gruzdev, S. K., Yakovlev, A. A., Druzhkova, T. A., Guekht, A. B., and Gulyaeva, N. V. (2019). The missing link: How exosomes and miRNAs can help in bridging psychiatry and molecular biology in the context of depression, bipolar disorder and schizophrenia. Cell. Mol. Neurobiol. 39 (6), 729–750. doi:10.1007/s10571-019-00684-6
Gujral, S., Aizenstein, H., Reynolds, C. F., Butters, M. A., and Erickson, K. I. (2017). Exercise effects on depression: Possible neural mechanisms. General Hosp. Psychiatry 49, 2–10. doi:10.1016/j.genhosppsych.2017.04.012
Gururajan, A., Naughton, M. E., Scott, K. A., O’Connor, R. M., Moloney, G., Clarke, G., et al. (2016). MicroRNAs as biomarkers for major depression: A role for let-7b and let-7c. Transl. Psychiatry 6 (8), e862. doi:10.1038/tp.2016.131
Handschin, C., and Spiegelman, B. M. (2008). The role of exercise and PGC1alpha in inflammation and chronic disease. Nature 454 (7203), 463–469. doi:10.1038/nature07206
Hariri, A. R., Goldberg, T. E., Mattay, V. S., Kolachana, B. S., Callicott, J. H., Egan, M. F., et al. (2003). Brain-derived neurotrophic factor val66met polymorphism affects human memory-related hippocampal activity and predicts memory performance. J. Neurosci. Official J. Soc. Neurosci. 23 (17), 6690–6694. doi:10.1523/JNEUROSCI.23-17-06690.2003
Harte-Hargrove, L., MacLusky, N. J., and Scharfman, H. E. (2013). Brain-derived neurotrophic factor-estrogen interactions in the hippocampal mossy fiber pathway: Implications for normal brain function and disease. Neuroscience 239, 46–66. doi:10.1016/j.neuroscience.2012.12.029
Heidarzadeh, M., Gürsoy-Özdemir, Y., Kaya, M., Eslami Abriz, A., Zarebkohan, A., Rahbarghazi, R., et al. (2021). Exosomal delivery of therapeutic modulators through the blood–brain barrier; promise and pitfalls. Cell. & Biosci. 11 (1), 142. doi:10.1186/s13578-021-00650-0
Helmig, S., Frühbeis, C., Krämer-Albers, E.-M., Simon, P., and Tug, S. (2015). Release of bulk cell free DNA during physical exercise occurs independent of extracellular vesicles. Eur. J. Appl. Physiology 115 (11), 2271–2280. doi:10.1007/s00421-015-3207-8
Hoque, R., Farooq, A., Ghani, A., Gorelick, F., and Mehal, W. Z. (2014). Lactate reduces liver and pancreatic injury in toll-like receptor– and inflammasome-mediated inflammation via GPR81-mediated suppression of innate immunity. Gastroenterology 146 (7), 1763–1774. doi:10.1053/j.gastro.2014.03.014
Hötting, K., and Röder, B. (2013). Beneficial effects of physical exercise on neuroplasticity and cognition. Neurosci. Biobehav. Rev. 37 (9), 2243–2257. doi:10.1016/j.neubiorev.2013.04.005
Huo, L., Du, X., Li, X., Liu, S., and Xu, Y. (2021). The emerging role of neural cell-derived exosomes in intercellular communication in health and neurodegenerative diseases. Front. Neurosci. 15, 738442. doi:10.3389/fnins.2021.738442
Ieraci, A., Madaio, A. I., Mallei, A., Lee, F. S., and Popoli, M. (2016). Brain-derived neurotrophic factor Val66Met human polymorphism impairs the beneficial exercise-induced neurobiological changes in mice. Neuropsychopharmacology 41 (13), 3070–3079. doi:10.1038/npp.2016.120
Islam, M. R., Luo, R., Valaris, S., Haley, E. B., Takase, H., Chen, Y. I., et al. (2020). Diffusion tensor-MRI detects exercise-induced neuroplasticity in the hippocampal microstructure in mice. Brain Plast. 5 (2), 147–159. doi:10.3233/BPL-190090
Isung, J., Aeinehband, S., Mobarrez, F., Mårtensson, B., Nordström, P., Åsberg, M., et al. (2012). Low vascular endothelial growth factor and interleukin-8 in cerebrospinal fluid of suicide attempters. Transl. Psychiatry 2 (11), e196. doi:10.1038/tp.2012.123
Jaworska, N., Courtright, A. K., De Somma, E., MacQueen, G. M., and MacMaster, F. P. (2019). Aerobic exercise in depressed youth: A feasibility and clinical outcomes pilot. Early Intervention Psychiatry 13 (1), 128–132. doi:10.1111/eip.12537
Kang, H.-J., Kim, S.-Y., Bae, K.-Y., Kim, S.-W., Shin, I.-S., Yoon, J.-S., et al. (2015). Comorbidity of depression with physical disorders: Research and clinical implications. Chonnam Med. J. 51 (1), 8–18. doi:10.4068/cmj.2015.51.1.8
Kano, S.-I., Dohi, E., and Rose, I. V. L. (2019). Extracellular vesicles for research on psychiatric disorders. Schizophr. Bull. 45 (1), 7–16. doi:10.1093/schbul/sby127
Karnib, N., El-Ghandour, R., El Hayek, L., Nasrallah, P., Khalifeh, M., Barmo, N., et al. (2019). Lactate is an antidepressant that mediates resilience to stress by modulating the hippocampal levels and activity of histone deacetylases. Neuropsychopharmacology 44 (6), 1152–1162. doi:10.1038/s41386-019-0313-z
Karvinen, S., Sievänen, T., Karppinen, J. E., Hautasaari, P., Bart, G., Samoylenko, A., et al. (2020). MicroRNAs in extracellular vesicles in sweat change in response to endurance exercise. Front. Physiology 11, 676. doi:10.3389/fphys.2020.00676
Khoury, R., Saad, J., Jabre, V., Ghayad, L. M., Khalifeh, M., Houbeika, R., et al. (2023). Autophagy regulates the release of exercise factors and their beneficial effects on spatial memory recall. Heliyon 9 (4), e14705. doi:10.1016/j.heliyon.2023.e14705
Khrimian, L., Obri, A., Ramos-Brossier, M., Rousseaud, A., Moriceau, S., Nicot, A.-S., et al. (2017). Gpr158 mediates osteocalcin’s regulation of cognition. J. Exp. Med. 214 (10), 2859–2873. doi:10.1084/jem.20171320
Kim, I. B., and Park, S.-C. (2021). Neural circuitry-neurogenesis coupling model of depression. Int. J. Mol. Sci. 22 (5), 2468. doi:10.3390/ijms22052468
Kim, Y.-K., Lee, H.-P., Won, S.-D., Park, E.-Y., Lee, H.-Y., Lee, B.-H., et al. (2007). Low plasma BDNF is associated with suicidal behavior in major depression. Prog. Neuro-Psychopharmacology Biol. Psychiatry 31 (1), 78–85. doi:10.1016/j.pnpbp.2006.06.024
Knubben, K., Reischies, F. M., Adli, M., Schlattmann, P., Bauer, M., and Dimeo, F. (2007). A randomised, controlled study on the effects of a short-term endurance training programme in patients with major depression. Br. J. Sports Med. 41 (1), 29–33. doi:10.1136/bjsm.2006.030130
Kuang, W.-H., Dong, Z.-Q., Tian, L.-T., and Li, J. (2018). MicroRNA-451a, microRNA-34a-5p, and microRNA-221-3p as predictors of response to antidepressant treatment. Braz. J. Med. Biol. Res. = Revista Brasileira De Pesquisas Medicas E Biol. 51 (7), e7212. doi:10.1590/1414-431x20187212
Kuwano, N., Kato, T. A., Mitsuhashi, M., Sato-Kasai, M., Shimokawa, N., Hayakawa, K., et al. (2018). Neuron-related blood inflammatory markers as an objective evaluation tool for major depressive disorder: An exploratory pilot case-control study. J. Affect. Disord. 240, 88–98. doi:10.1016/j.jad.2018.07.040
Kvam, S., Kleppe, C. L., Nordhus, I. H., and Hovland, A. (2016). Exercise as a treatment for depression: A meta-analysis. J. Affect. Disord. 202, 67–86. doi:10.1016/j.jad.2016.03.063
Lafourcade, C., Ramírez, J. P., Luarte, A., Fernández, A., and Wyneken, U. (2016). MiRNAs in astrocyte-derived exosomes as possible mediators of neuronal plasticity. J. Exp. Neurosci. 10 (1), 1–9. doi:10.4137/JEN.S39916
Lansford, K. A., Shill, D. D., Dicks, A. B., Marshburn, M. P., Southern, W. M., and Jenkins, N. T. (2016). Effect of acute exercise on circulating angiogenic cell and microparticle populations. Exp. Physiol. 101 (1), 155–167. doi:10.1113/EP085505
Lauritzen, K. H., Morland, C., Puchades, M., Holm-Hansen, S., Hagelin, E. M., Lauritzen, F., et al. (2014). Lactate receptor sites link neurotransmission, neurovascular coupling, and brain energy metabolism. Cereb. Cortex 24 (10), 2784–2795. doi:10.1093/cercor/bht136
Lee, T. H.-Y., Formolo, D. A., Kong, T., Lau, S. W.-Y., Ho, C. S.-L., Leung, R. Y. H., et al. (2019). Potential exerkines for physical exercise-elicited pro-cognitive effects: Insight from clinical and animal research. Int. Rev. Neurobiol. 147, 361–395. doi:10.1016/bs.irn.2019.06.002
Lee, Y., El Andaloussi, S., and Wood, M. J. A. (2012). Exosomes and microvesicles: Extracellular vesicles for genetic information transfer and gene therapy. Hum. Mol. Genet. 21 (1), R125–R134. doi:10.1093/hmg/dds317
Li, D., Wang, Y., Jin, X., Hu, D., Xia, C., Xu, H., et al. (2020). NK cell-derived exosomes carry miR-207 and alleviate depression-like symptoms in mice. J. Neuroinflammation 17 (1), 126. doi:10.1186/s12974-020-01787-4
Li, J. J., Wang, B., Kodali, M. C., Chen, C., Kim, E., Patters, B. J., et al. (2018). In vivo evidence for the contribution of peripheral circulating inflammatory exosomes to neuroinflammation. J. Neuroinflammation 15 (1), 8. doi:10.1186/s12974-017-1038-8
Liang, J.-Q., Liao, H.-R., Xu, C.-X., Li, X.-L., Wei, Z.-X., Xie, G.-J., et al. (2020). Serum exosome-derived miR-139-5p as a potential biomarker for major depressive disorder. Neuropsychiatric Dis. Treat. 16, 2689–2693. doi:10.2147/NDT.S277392
Lin, T.-W., and Kuo, Y.-M. (2013). Exercise benefits brain function: The monoamine connection. Brain Sci. 3 (1), 39–53. doi:10.3390/brainsci3010039
Liu, T., Zhang, Q., Zhang, J., Li, C., Miao, Y.-R., Lei, Q., et al. (2019). EVmiRNA: A database of miRNA profiling in extracellular vesicles. Nucleic Acids Res. 47 (1), D89–D93. doi:10.1093/nar/gky985
Liu, W., Ge, T., Leng, Y., Pan, Z., Fan, J., Yang, W., et al. (2017). The role of neural plasticity in depression: From Hippocampus to prefrontal cortex. Neural Plast. 2017, 6871089. doi:10.1155/2017/6871089
Lopresti, A. L., Hood, S. D., and Drummond, P. D. (2013). A review of lifestyle factors that contribute to important pathways associated with major depression: Diet, sleep and exercise. J. Affect. Disord. 148 (1), 12–27. doi:10.1016/j.jad.2013.01.014
Luarte, A., Cisternas, P., Caviedes, A., Batiz, L. F., Lafourcade, C., Wyneken, U., et al. (2017). Astrocytes at the hub of the stress response: Potential modulation of neurogenesis by miRNAs in astrocyte-derived exosomes. Stem Cells Int. 2017, 1719050. doi:10.1155/2017/1719050
Ma, C., Wang, J., Liu, H., Chen, Y., Ma, X., Chen, S., et al. (2018). Moderate exercise enhances endothelial progenitor cell exosomes release and function. Med. Sci. Sports Exerc. 50 (10), 2024–2032. doi:10.1249/MSS.0000000000001672
Mandolesi, L., Gelfo, F., Serra, L., Montuori, S., Polverino, A., Curcio, G., et al. (2017). Environmental factors promoting neural plasticity: Insights from animal and human studies. Neural Plast. 2017, 7219461. doi:10.1155/2017/7219461
Mandolesi, L., Polverino, A., Montuori, S., Foti, F., Ferraioli, G., Sorrentino, P., et al. (2018). Effects of physical exercise on cognitive functioning and wellbeing: Biological and psychological benefits. Front. Psychol. 9, 509. doi:10.3389/fpsyg.2018.00509
Matsumoto, J., Stewart, T., Banks, W. A., and Zhang, J. (2017). The transport mechanism of extracellular vesicles at the blood-brain barrier. Curr. Pharm. Des. 23 (40), 6206–6214. doi:10.2174/1381612823666170913164738
Miranda, M., Morici, J. F., Zanoni, M. B., and Bekinschtein, P. (2019). Brain-derived neurotrophic factor: A key molecule for memory in the healthy and the pathological brain. Front. Cell. Neurosci. 13, 363. doi:10.3389/fncel.2019.00363
Morad, G., Carman, C. V., Hagedorn, E. J., Perlin, J. R., Zon, L. I., Mustafaoglu, N., et al. (2019). Tumor-derived extracellular vesicles breach the intact blood-brain barrier via transcytosis. ACS Nano 13 (12), 13853–13865. doi:10.1021/acsnano.9b04397
Morres, I. D., Hatzigeorgiadis, A., Stathi, A., Comoutos, N., Arpin-Cribbie, C., Krommidas, C., et al. (2019). Aerobic exercise for adult patients with major depressive disorder in mental health services: A systematic review and meta-analysis. Depress. Anxiety 36 (1), 39–53. doi:10.1002/da.22842
Mucher, P., Batmyagmar, D., Perkmann, T., Repl, M., Radakovics, A., Ponocny-Seliger, E., et al. (2021). Basal myokine levels are associated with quality of life and depressed mood in older adults. Psychophysiology 58 (5), e13799. doi:10.1111/psyp.13799
Munir, J., Yoon, J. K., and Ryu, S. (2020). Therapeutic miRNA-enriched extracellular vesicles: Current approaches and future prospects. Cells 9 (10), 2271. doi:10.3390/cells9102271
Mustapic, M., Eitan, E., Werner, J. K., Berkowitz, S. T., Lazaropoulos, M. P., Tran, J., et al. (2017). Plasma extracellular vesicles enriched for neuronal origin: A potential window into brain pathologic processes. Front. Neurosci. 11, 278. doi:10.3389/fnins.2017.00278
Nair, V. D., Ge, Y., Li, S., Pincas, H., Jain, N., Seenarine, N., et al. (2020). Sedentary and trained older men have distinct circulating exosomal microRNA profiles at baseline and in response to acute exercise. Front. Physiology 11, 605. doi:10.3389/fphys.2020.00605
Nauer, R. K., Dunne, M. F., Stern, C. E., Storer, T. W., and Schon, K. (2020). Improving fitness increases dentate gyrus/CA3 volume in the hippocampal head and enhances memory in young adults. Hippocampus 30 (5), 488–504. doi:10.1002/hipo.23166
Nederveen, J. P., Warnier, G., Di Carlo, A., Nilsson, M. I., and Tarnopolsky, M. A. (2021). Extracellular vesicles and exosomes: Insights from exercise science. Front. Physiology 11, 604274. doi:10.3389/fphys.2020.604274
Netz, Y. (2017). Is the comparison between exercise and pharmacologic treatment of depression in the clinical practice guideline of the American college of physicians evidence-based? Front. Pharmacol. 8, 257. doi:10.3389/fphar.2017.00257
Nicolini, C., Michalski, B., Toepp, S. L., Turco, C. V., D’Hoine, T., Harasym, D., et al. (2020). A single bout of high-intensity interval exercise increases corticospinal excitability, brain-derived neurotrophic factor, and uncarboxylated osteolcalcin in sedentary, healthy males. Neuroscience 437, 242–255. doi:10.1016/j.neuroscience.2020.03.042
Nobis, A., Zalewski, D., and Waszkiewicz, N. (2020). Peripheral markers of depression. J. Clin. Med. 9 (12), 3793. doi:10.3390/jcm9123793
Notaras, M., and van den Buuse, M. (2020). Neurobiology of BDNF in fear memory, sensitivity to stress, and stress-related disorders. Mol. Psychiatry 25 (10), 2251–2274. doi:10.1038/s41380-019-0639-2
O’Brien, K., Breyne, K., Ughetto, S., Laurent, L. C., and Breakefield, X. O. (2020). RNA delivery by extracellular vesicles in mammalian cells and its applications. Nat. Rev. Mol. Cell. Biol. 21 (10), 585–606. doi:10.1038/s41580-020-0251-y
Oliveira, G. P., Porto, W. F., Palu, C. C., Pereira, L. M., Petriz, B., Almeida, J. A., et al. (2018). Effects of acute aerobic exercise on rats serum extracellular vesicles diameter, concentration and small RNAs content. Front. Physiology 9, 532. doi:10.3389/fphys.2018.00532
Ortega, M. A., Alvarez-Mon, M. A., García-Montero, C., Fraile-Martinez, O., Lahera, G., Monserrat, J., et al. (2021). MicroRNAs as critical biomarkers of major depressive disorder: A comprehensive perspective. Biomedicines 9 (11), 1659. doi:10.3390/biomedicines9111659
Oury, F., Khrimian, L., Denny, C. A., Gardin, A., Chamouni, A., Goeden, N., et al. (2013). Maternal and offspring pools of osteocalcin influence brain development and functions. Cell. 155 (1), 228–241. doi:10.1016/j.cell.2013.08.042
Paffenbarger, R. S., Lee, I. M., and Leung, R. (1994). Physical activity and personal characteristics associated with depression and suicide in American college men. Acta Psychiatr. Scand. Suppl. 377, 16–22. doi:10.1111/j.1600-0447.1994.tb05796.x
Paluska, S. A., and Schwenk, T. L. (2000). Physical activity and mental health: Current concepts. Sports Med. 29 (3), 167–180. doi:10.2165/00007256-200029030-00003
Paolucci, E. M., Loukov, D., Bowdish, D. M. E., and Heisz, J. J. (2018). Exercise reduces depression and inflammation but intensity matters. Biol. Psychol. 133, 79–84. doi:10.1016/j.biopsycho.2018.01.015
Pearce, M., Garcia, L., Abbas, A., Strain, T., Schuch, F. B., Golubic, R., et al. (2022). Association between physical activity and risk of depression: A systematic review and meta-analysis. JAMA Psychiatry 79 (6), 550–559. doi:10.1001/jamapsychiatry.2022.0609
Pedersen, B. K., and Saltin, B. (2015). Exercise as medicine – evidence for prescribing exercise as therapy in 26 different chronic diseases. Scand. J. Med. Sci. Sports 25 (3), 1–72. doi:10.1111/sms.12581
Pierdoná, T. M., Martin, A., Obi, P. O., Seif, S., Bydak, B., Labouta, H. I., et al. (2022). Extracellular vesicles as predictors of individual response to exercise training in youth living with obesity. Front. Bioscience-Landmark 27 (5), 143. doi:10.31083/j.fbl2705143
Proia, P., Di Liegro, C. M., Schiera, G., Fricano, A., and Di Liegro, I. (2016). Lactate as a metabolite and a regulator in the central nervous system. Int. J. Mol. Sci. 17 (9), 1450. doi:10.3390/ijms17091450
Qu, H., Liu, R., Chen, J., Zheng, L., and Chen, R. (2020). Aerobic exercise inhibits CUMS-depressed mice hippocampal inflammatory response via activating hippocampal miR-223/TLR4/MyD88-NF-κB pathway. Int. J. Environ. Res. Public Health 17 (8), E2676. doi:10.3390/ijerph17082676
Raison, C. L., Capuron, L., and Miller, A. H. (2006). Cytokines sing the blues: Inflammation and the pathogenesis of depression. Trends Immunol. 27 (1), 24–31. doi:10.1016/j.it.2005.11.006
Ramos-Zaldívar, H. M., Polakovicova, I., Salas-Huenuleo, E., Corvalán, A. H., Kogan, M. J., Yefi, C. P., et al. (2022). Extracellular vesicles through the blood–brain barrier: A review. Fluids Barriers CNS 19 (1), 60. doi:10.1186/s12987-022-00359-3
Rentz, J., Winberg, J., Swardfager, W., and Mitchell, J. (2020). SAT-293 osteocalcin and exercise improve mood and cognition in female mice with high-fat diet induced type 2 diabetes. J. Endocr. Soc. 4 (1). doi:10.1210/jendso/bvaa046.500
Rethorst, C. D., Toups, M. S., Greer, T. L., Nakonezny, P. A., Carmody, T. J., Grannemann, B. D., et al. (2013). Pro-inflammatory cytokines as predictors of antidepressant effects of exercise in major depressive disorder. Mol. Psychiatry 18 (10), 1119–1124. doi:10.1038/mp.2012.125
Rhee, S. J., Kim, H., Lee, Y., Lee, H. J., Park, C. H. K., Yang, J., et al. (2020). Comparison of serum microbiome composition in bipolar and major depressive disorders. J. Psychiatric Res. 123, 31–38. doi:10.1016/j.jpsychires.2020.01.004
Roque, S., Correia-Neves, M., Mesquita, A. R., Palha, J. A., and Sousa, N. (2009). Interleukin-10: A key cytokine in depression? Cardiovasc. Psychiatry Neurology 2009, 187894. doi:10.1155/2009/187894
Rome, S., Forterre, A., Mizgier, M. L., and Bouzakri, K. (2019). Skeletal muscle-released extracellular vesicles: State of the art. Front. Physiology 10, 929. doi:10.3389/fphys.2019.00929
Ross, R. E., VanDerwerker, C. J., Saladin, M. E., and Gregory, C. M. (2023). The role of exercise in the treatment of depression: Biological underpinnings and clinical outcomes. Mol. Psychiatry 28 (1), 298–328. doi:10.1038/s41380-022-01819-w
Rush, A. J., Trivedi, M. H., Wisniewski, S. R., Nierenberg, A. A., Stewart, J. W., Warden, D., et al. (2006). Acute and longer-term outcomes in depressed outpatients requiring one or several treatment steps: A STAR*D report. Am. J. Psychiatry 163 (11), 1905–1917. doi:10.1176/ajp.2006.163.11.1905
Russo-Neustadt, A. A., Beard, R. C., Huang, Y. M., and Cotman, C. W. (2000). Physical activity and antidepressant treatment potentiate the expression of specific brain-derived neurotrophic factor transcripts in the rat hippocampus. Neuroscience 101 (2), 305–312. doi:10.1016/s0306-4522(00)00349-3
Sadovska, L., Auders, J., Keiša, L., Romanchikova, N., Silamiķele, L., Kreišmane, M., et al. (2022). Exercise-induced extracellular vesicles delay the progression of prostate cancer. Front. Mol. Biosci. 8, 784080. doi:10.3389/fmolb.2021.784080
Saeedi, S., Israel, S., Nagy, C., and Turecki, G. (2019). The emerging role of exosomes in mental disorders. Transl. Psychiatry 9 (1), 122. doi:10.1038/s41398-019-0459-9
Saeedi, S., Nagy, C., Ibrahim, P., Théroux, J.-F., Wakid, M., Fiori, L. M., et al. (2021). Neuron-derived extracellular vesicles enriched from plasma show altered size and miRNA cargo as a function of antidepressant drug response. Mol. Psychiatry 26 (12), 7417–7424. doi:10.1038/s41380-021-01255-2
Safdar, A., Saleem, A., and Tarnopolsky, M. A. (2016). The potential of endurance exercise-derived exosomes to treat metabolic diseases. Nat. Rev. Endocrinol. 12 (9), 504–517. doi:10.1038/nrendo.2016.76
Safdar, A., and Tarnopolsky, M. A. (2018). Exosomes as mediators of the systemic adaptations to endurance exercise. Cold Spring Harb. Perspect. Med. 8 (3), a029827. doi:10.1101/cshperspect.a029827
Salk, R. H., Hyde, J. S., and Abramson, L. Y. (2017). Gender differences in depression in representative national samples: Meta-analyses of diagnoses and symptoms. Psychol. Bull. 143 (8), 783–822. doi:10.1037/bul0000102
Schuch, F. B., Vancampfort, D., Firth, J., Rosenbaum, S., Ward, P. B., Silva, E. S., et al. (2018). Physical activity and incident depression: A meta-analysis of prospective cohort studies. Am. J. Psychiatry 175 (7), 631–648. doi:10.1176/appi.ajp.2018.17111194
Scisciola, L., Fontanella, R. A., SurinaCataldo, V., Paolisso, G., and Barbieri, M. (2021). Sarcopenia and cognitive function: Role of myokines in muscle brain cross-talk. Life 11 (2), 173. doi:10.3390/life11020173
Shi, M., Sheng, L., Stewart, T., Zabetian, C. P., and Zhang, J. (2019). New windows into the brain: Central nervous system-derived extracellular vesicles in blood. Prog. Neurobiol. 175, 96–106. doi:10.1016/j.pneurobio.2019.01.005
Siteneski, A., Olescowicz, G., Pazini, F. L., Camargo, A., Fraga, D. B., Brocardo, P. S., et al. (2020). Antidepressant-like and pro-neurogenic effects of physical exercise: The putative role of FNDC5/irisin pathway. J. Neural Transm. 127(3), 355–370. doi:10.1007/s00702-020-02143-9
Skog, J., Würdinger, T., van Rijn, S., Meijer, D. H., Gainche, L., Curry, W. T., et al. (2008). Glioblastoma microvesicles transport RNA and proteins that promote tumour growth and provide diagnostic biomarkers. Nat. Cell. Biol. 10 (12), 1470–1476. doi:10.1038/ncb1800
Sleiman, S. F., Henry, J., Al-Haddad, R., El Hayek, L., Abou Haidar, E., Stringer, T., et al. (2016). Exercise promotes the expression of brain derived neurotrophic factor (BDNF) through the action of the ketone body β-hydroxybutyrate. ELife 5, e15092. doi:10.7554/eLife.15092
Smalheiser, N. R., Lugli, G., Zhang, H., Rizavi, H., Cook, E. H., and Dwivedi, Y. (2014). Expression of microRNAs and other small RNAs in prefrontal cortex in schizophrenia, bipolar disorder and depressed subjects. PloS One 9 (1), e86469. doi:10.1371/journal.pone.0086469
Smith, P. J., and Merwin, R. M. (2021). The role of exercise in management of mental health disorders: An integrative review. Annu. Rev. Med. 72, 45–62. doi:10.1146/annurev-med-060619-022943
Soares, E., Reis, J., Rodrigues, M., Ribeiro, C. F., and Pereira, F. C. (2021). Circulating extracellular vesicles: The missing link between physical exercise and depression management? Int. J. Mol. Sci. 22 (2), 542. doi:10.3390/ijms22020542
Stanton, R., and Reaburn, P. (2014). Exercise and the treatment of depression: A review of the exercise program variables. J. Sci. Med. Sport 17 (2), 177–182. doi:10.1016/j.jsams.2013.03.010
Stephan, J. S., and Sleiman, S. F. (2021). Exercise factors released by the liver, muscle, and bones have promising therapeutic potential for stroke. Front. Neurology 12, 600365. doi:10.3389/fneur.2021.600365
Ström-Olsen, R. (1937). The therapeutic value in mental illness of physical fitness through exercise. Ment. Welf. 18 (1), 1–10.
Su, B., Cheng, S., Wang, L., and Wang, B. (2022). MicroRNA-139-5p acts as a suppressor gene for depression by targeting nuclear receptor subfamily 3, group C, member 1. Bioengineered 13 (5), 11856–11866. doi:10.1080/21655979.2022.2059937
Takafuji, Y., Tatsumi, K., Ishida, M., Kawao, N., Okada, K., and Kaji, H. (2020). Extracellular vesicles secreted from mouse muscle cells suppress osteoclast formation: Roles of mitochondrial energy metabolism. Bone 134, 115298. doi:10.1016/j.bone.2020.115298
Tian, F., Shen, Y., Chen, Z., Li, R., and Ge, Q. (2017). No significant difference between plasma miRNAs and plasma-derived exosomal miRNAs from healthy people. BioMed Res. Int. 2017, 1304816. doi:10.1155/2017/1304816
Ung, T. H., Madsen, H. J., Hellwinkel, J. E., Lencioni, A. M., and Graner, M. W. (2014). Exosome proteomics reveals transcriptional regulator proteins with potential to mediate downstream pathways. Cancer Sci. 105 (11), 1384–1392. doi:10.1111/cas.12534
Upadhya, R., Madhu, L. N., Attaluri, S., Gitaí, D. L. G., Pinson, M. R., Kodali, M., et al. (2020). Extracellular vesicles from human iPSC-derived neural stem cells: MiRNA and protein signatures, and anti-inflammatory and neurogenic properties. J. Extracell. Vesicles 9 (1), 1809064. doi:10.1080/20013078.2020.1809064
Valenzuela, P. L., Castillo-García, A., Morales, J. S., de la Villa, P., Hampel, H., Emanuele, E., et al. (2020). Exercise benefits on Alzheimer’s disease: State-of-the-science. Ageing Res. Rev. 62, 101108. doi:10.1016/j.arr.2020.101108
van Niel, G., D’Angelo, G., and Raposo, G. (2018). Shedding light on the cell biology of extracellular vesicles. Nat. Rev. Mol. Cell. Biol. 19 (4), 213–228. doi:10.1038/nrm.2017.125
Vaynman, S., Ying, Z., and Gomez-Pinilla, F. (2004). Hippocampal BDNF mediates the efficacy of exercise on synaptic plasticity and cognition. Eur. J. Neurosci. 20 (10), 2580–2590. doi:10.1111/j.1460-9568.2004.03720.x
Vechetti, I. J., Valentino, T., Mobley, C. B., and McCarthy, J. J. (2021). The role of extracellular vesicles in skeletal muscle and systematic adaptation to exercise. J. Physiology 599 (3), 845–861. doi:10.1113/JP278929
Wang, Y., Gao, C., Gao, T., Zhao, L., Zhu, S., and Guo, L. (2021). Plasma exosomes from depression ameliorate inflammation-induced depressive-like behaviors via sigma-1 receptor delivery. Brain, Behav. Immun. 94, 225–234. doi:10.1016/j.bbi.2021.02.004
Wei, Z.-X., Xie, G.-J., Mao, X., Zou, X.-P., Liao, Y.-J., Liu, Q.-S., et al. (2020). Exosomes from patients with major depression cause depressive-like behaviors in mice with involvement of miR-139-5p-regulated neurogenesis. Neuropsychopharmacology 45 (6), 1050–1058. doi:10.1038/s41386-020-0622-2
Whitham, M., Parker, B. L., Friedrichsen, M., Hingst, J. R., Hjorth, M., Hughes, W. E., et al. (2018). Extracellular vesicles provide a means for tissue crosstalk during exercise. Cell. Metab. 27 (1), 237–251. doi:10.1016/j.cmet.2017.12.001
Wrann, C. D., White, J. P., Salogiannnis, J., Laznik-Bogoslavski, D., Wu, J., Ma, D., et al. (2013). Exercise induces hippocampal BDNF through a PGC-1α/FNDC5 pathway. Cell. Metab. 18 (5), 649–659. doi:10.1016/j.cmet.2013.09.008
Wu, S., Yin, Y., and Du, L. (2022). Blood–brain barrier dysfunction in the pathogenesis of major depressive disorder. Cell. Mol. Neurobiol. 42 (8), 2571–2591. doi:10.1007/s10571-021-01153-9
Xie, X., Lai, W., Xu, S., Di Forti, M., Zhang, J., Chen, M., et al. (2023). Hyper-inflammation of astrocytes in patients of major depressive disorder: Evidence from serum astrocyte-derived extracellular vesicles. Brain, Behav. Immun. 109, 51–62. doi:10.1016/j.bbi.2022.12.014
Xie, Y., Wu, Z., Sun, L., Zhou, L., Wang, G., Xiao, L., et al. (2021). The effects and mechanisms of exercise on the treatment of depression. Front. Psychiatry 12, 705559. doi:10.3389/fpsyt.2021.705559
Xu, X., Lai, Y., and Hua, Z.-C. (2019). Apoptosis and apoptotic body: Disease message and therapeutic target potentials. Biosci. Rep. 39 (1), BSR20180992. doi:10.1042/BSR20180992
Xue, X., Liu, B., Hu, J., Bian, X., and Lou, S. (2022). The potential mechanisms of lactate in mediating exercise-enhanced cognitive function: A dual role as an energy supply substrate and a signaling molecule. Nutr. Metabolism 19 (1), 52. doi:10.1186/s12986-022-00687-z
Zhan, G., Huang, N., Li, S., Hua, D., Zhang, J., Fang, X., et al. (2018). PGC-1α-FNDC5-BDNF signaling pathway in skeletal muscle confers resilience to stress in mice subjected to chronic social defeat. Psychopharmacology 235 (11), 3351–3358. doi:10.1007/s00213-018-5041-2
Zhang, R., Liang, X., Tang, S., Song, L., Zhang, J., and Du, Y. (2021). Short-term high-intensity treadmill exercise promotes ceramide-dependent extracellular vesicle secretion in the central nervous system of mice. Int. Med. J. Exp. Clin. Res. 27, e929609. doi:10.12659/MSM.929609
Zhang, Y., and Xu, C. (2022). Effects of exosomes on adult hippocampal neurogenesis and neuropsychiatric disorders. Mol. Biol. Rep. 49 (7), 6763–6777. doi:10.1007/s11033-022-07313-4
Keywords: aerobic exercise, small extracellular vesicles, major depressive disorder, miRNA, epigenetics, sex differences, blood-brain barrier
Citation: Khoury R and Nagy C (2023) Running from stress: a perspective on the potential benefits of exercise-induced small extracellular vesicles for individuals with major depressive disorder. Front. Mol. Biosci. 10:1154872. doi: 10.3389/fmolb.2023.1154872
Received: 31 January 2023; Accepted: 06 June 2023;
Published: 15 June 2023.
Edited by:
Chiara Porro, University of Foggia, ItalyReviewed by:
Hua Liu, Wuhan Sports University, ChinaBharathi Hattiangady, Texas A&M University College of Medicine, United States
Copyright © 2023 Khoury and Nagy. This is an open-access article distributed under the terms of the Creative Commons Attribution License (CC BY). The use, distribution or reproduction in other forums is permitted, provided the original author(s) and the copyright owner(s) are credited and that the original publication in this journal is cited, in accordance with accepted academic practice. No use, distribution or reproduction is permitted which does not comply with these terms.
*Correspondence: Corina Nagy, Y29yaW5hLm5hZ3lAbWNnaWxsLmNh