- 1Institute of Chemical Science, School of Engineering and Physical Sciences, Heriot-Watt University, Edinburgh, United Kingdom
- 2Institute of Quantitative Biology, Biochemistry and Biotechnology, School of Biological Sciences, University of Edinburgh, Edinburgh, United Kingdom
- 3Division of Nanotechnology and Functional Materials, Department of Material Sciences and Engineering, Uppsala University, Uppsala, Sweden
- 4School of Chemistry, University of Glasgow, Glasgow, United Kingdom
- 5Institute of Biological Chemistry, Biophysics and Bioengineering, School of Engineering and Physical Sciences, Heriot-Watt University, Edinburgh, United Kingdom
Nanomedicine plays a crucial role in the development of next-generation therapies. The use of nanoparticles as drug delivery platforms has become a major area of research in nanotechnology. To be effective, these nanoparticles must interact with desired drug molecules and release them at targeted sites. The design of these “nanoplatforms” typically includes a functional core, an organic coating with functional groups for drug binding, and the drugs or bioactive molecules themselves. However, by exploiting the coordination chemistry between organic molecules and transition metal centers, the self-assembly of drugs onto the nanoplatform surfaces can bypass the need for an organic coating, simplifying the materials synthesis process. In this perspective, we use gold-iron oxide nanoplatforms as examples and outline the prospects and challenges of using self-assembly to prepare drug-nanoparticle constructs. Through a case study on the binding of insulin on Au-dotted Fe3O4 nanoparticles, we demonstrate how a self-assembly system can be developed. This method can also be adapted to other combinations of transition metals, with the potential for scaling up. Furthermore, the self-assembly method can also be considered as a greener alternative to traditional methods, reducing the use of chemicals and solvents. In light of the current climate of environmental awareness, this shift towards sustainability in the pharmaceutical industry would be welcomed.
1 Introduction
Nanoparticles (10–100 nm in diameter) are suitable candidates as vehicles for the delivery of bioactive molecules (McBain et al., 2008). Their small size allows circulation inside the blood vessels while the high surface area offers a high loading capacity for bioactive cargos. Recent advancements in using nanoparticles for delivery include targeted delivery (Eskandani et al., 2022; Wu et al., 2022) and co-delivery of bioactive molecules (Hopkins et al., 2022; Li et al., 2022), aided by the development of multifunctional nanoparticles. These are particularly encouraging as they may improve both the delivery and therapeutic efficiency (Elhassan et al., 2022; Xu et al., 2022). In general, the design of a nanoparticle platform for delivery consists of a number of key features: 1) the nanoparticle core, 2) the surface functional groups, and 3) the bioactive molecules (Yiu, 2011). The core forms the main solid-state foundation for functionalities to build on. It can also possess selected key physical properties to enhance the overall properties of the nanoplatform. These properties can be magnetism (e.g., Fe3O4) (Pucci et al., 2022), fluorescence (e.g., ZnS quantum dots) (Wu and Yan, 2013), or plasmonic (Au) (Ou et al., 2021). We may also need to consider if the core needs to be (bio)degradable, depending on the desired applications (Ye et al., 2014; Qin et al., 2020). The surface functional groups, usually organic, are required to interact with the targeted cargos (e.g., drugs). It is necessary to consider what types of surface-to-cargo binding to be used. Electrostatic binding between surface functional groups and cargo is widely used because it is versatile and cargo can be released by disrupting the electrostatic bonds in presence of increased salt (or ions) content. However, more permanent binding such as covalent may also be used if the cargo needs to be retained for a longer period or going through a milieu with a high ion concentration. Most importantly, all these components must be non-toxic and safe to use in vivo.
There are several strategies to functionalize the nanoparticle surface with organic or inorganic methods. For inorganic nanoparticles, silanization using functional silanes such as 3-aminopropyltriethoxysilane (APTES) is commonly used to functionalize Fe3O4 and SiO2 nanoparticles with amine (-NH2) groups (Yiu et al., 2013; Kumar and Paik, 2021). Another method is to graft organic groups onto the protective coating (e.g., dextran) (Yousefvand et al., 2021; Xiong et al., 2022). However, these strategies may lead to several issues in both the synthesis and the final product characteristics/properties themselves. First of all, the added synthesis steps can only add complication to the production of the nanoparticles. These extra steps, with additional chemicals being used, may also potentially cause adverse effects in toxicity or biocompatibility, as well as further burden on sustainability in production. For example, use of solvents (e.g., toluene in a standard silanization protocol) may leave residual solvents that cause unwanted side effects on the applications. Another strategy is to use self-assembly binding between bioactive molecules and the unfunctionalized nanoparticle surface without second-step organic functionalization, circumventing the aforementioned complications.
2 Binding strategies via self-assembly
Transition metals are known to form coordination bonds with many electron donating ligands via their unoccupied orbitals. Indeed, this coordination chemistry has been well-developed and transition metal complexes have found wide applications in important areas such as catalysis (Crawley et al., 2022; Yadav et al., 2022) and sensing (Li et al., 2020; Ma et al., 2021). In nature, transition metal complexes are widely found in enzymes and metalloproteins (Bewley et al., 2013). For example, hemoglobin, an iron-containing metalloprotein, is probably the most well-known and abundant metalloprotein in the human body due to its vital role in the transportation of oxygen. In fact, several amino acid units, with the coordinating side chains of -NH2 (lysine), -COOH (glutamic acid and aspartic acid), -SH (cysteine), guanidino (arginine), and imidazole (histidine) groups, can act as ligands. Other non-amino acids bioactive molecules that can form coordination bonds with a transition metal center include dopamine and folic acid.
The formation of transition metal-ligand coordination bonds can be carried out at room temperature in an aqueous medium due to the strong affinity. This is a distinct advantage because many bioactive molecules such as proteins are solvent- and temperature-sensitive. Reactions at an elevated temperature or in an organic solvent could denature the protein molecules of interest, leading to deactivation. Regarding the transition metal center, although most transition metals can serve as the metal center for coordinating these bioactive ligands, only a few transition metal candidates can be used in vivo biomedical applications due to their associated toxicity. For example, it has been known for decades that Ni and Co are toxic and carcinogenic (Magaye et al., 2012). Very few safe choices of transition metals are available for scientists to develop nanoplatforms. These comprise of iron, gold, platinum, zinc, and titanium.
2.1 Au-thiol and Au-cysteine binding
Thiolated compounds (-SH) are known to bind to noble metal surfaces (e.g., Au, Ag, Pt), due to the π-electron conjugation between the thiol group and the metal atoms (Figure 1AI) (Heimel et al., 2006). There is much published research in the literature studying this thiol-to-metal interaction, in particular on Au (Ulman, 2001). Au is the most popular choice of metal for this purpose because of its safety (e.g., vs. Ag), stability, and its cost (e.g., vs. Pt). There are many bioactive compounds that include thiol groups in their structure, even without modification. One important example is the cysteine unit in peptide and protein molecules, which can bind to the Au surface without modification providing that the cysteine unit(s) is accessible externally. Using protein engineering, Cys-tags can also be attached to bioactive protein molecules, enabling them for effective binding onto an Au surface (van der Meer et al., 2021). Research on using Au nanoparticles for the delivery of bioactive molecules or drugs has been widely available in the literature (Ghosh et al., 2008; Pissuwan et al., 2011). Many of these works were based on thiol- or cysteine-to-gold interactions. Recent examples include the delivery of the anticancer p53 peptides, which were bound directly to the Au nanoparticle surface via cysteine-to-gold interaction (Maraming and Kah, 2021).
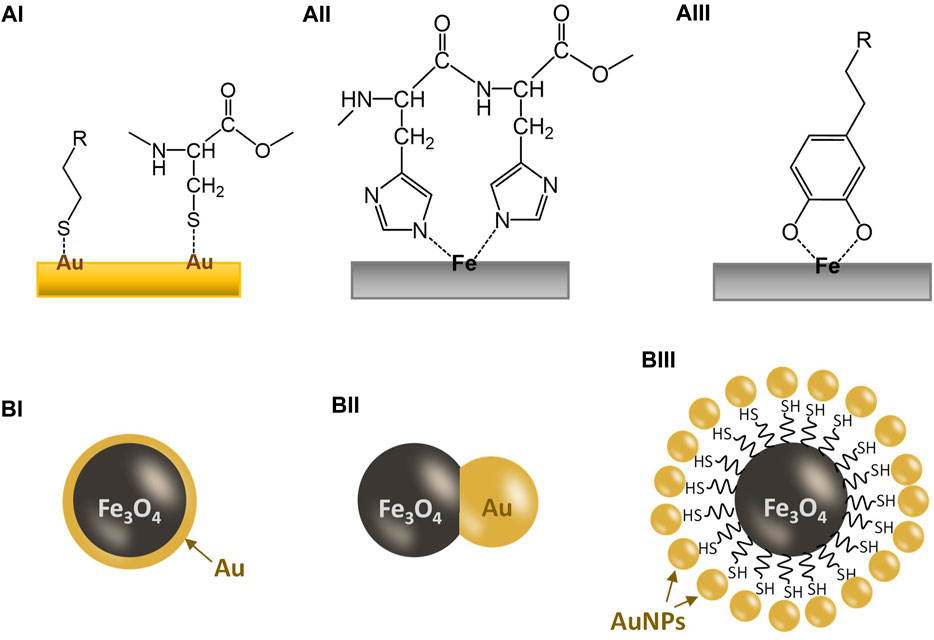
FIGURE 1. Illustration for the coordination bonding between a metal surface and a ligand and the basic designs for common Au/Fe3O4 nanoparticles. (AI) Interaction between a gold surface and thiol groups; (AII) interaction between an iron surface and a dihistidine group; (AIII) interaction between an iron surface and a catechol group; (BI) a gold-coated Fe3O4 nanoparticle; (BII) a Au/Fe3O4 Janus nanoparticle; (BIII) a gold-dotted Fe3O4 nanoparticle assembled via a thiol-functionalized core.
2.2 Iron-dihistidine binding
Iron is another element that is widely used in biomedical research due to its safety and cost. Moreover, the magnetic properties (ferromagnetic or superparamagnetic) of some iron oxides, notably magnetite (Fe3O4) and maghemite (γ-Fe2O3), are also added advantages, including MR-contrasting (Li and Zhang, 2019), enhanced separation (Wan Ibrahim et al., 2015), targeted delivery (Palanisamy and Wang, 2019; Sharifianjazi et al., 2021), and potential for hyperthermia (Zhao et al., 2020; Pinakidou et al., 2022). Similar to many transition metals, the empty orbitals in the iron atom allow the formation of strong coordination bonds with electron-donating ligands, many of which are oxygen- or nitrogen-containing groups. One notable amino acid is histidine, which has an imidazole group on the structure, allowing coordination to transition metals (e.g., Fe, Co, Ni, Cu) (See Figure 1AII) (Kruppa and König, 2006). The histidine units in a metalloprotein can serve as a ligand to bind the metal center (Zastrow and Pecoraro, 2013; Liu et al., 2014). Indeed, the histidine-to-metal affinity has already been exploited in biotechnology, such as protein engineering, by synthetically tethering His-tags comprising six or seven consecutive histidine residues (His-6 or His-7) to maximize the binding to transition metal sites (You and Piehler, 2014). The ultimate purpose of this strategy is to facilitate the laborious protein purification process.
Despite the iron-to-histidine interaction being studied in the literature, examples of using iron oxide nanoparticles for binding His-tagged proteins are few and far between. Most scientists in this area still prefer using Ni as the transition metal for forming a chelate with His-tags due to their higher selectivity. However, as mentioned previously, nickel is considered to be toxic and unsuitable for in vivo biomedical applications. Currently, using unfunctionalized iron oxide nanoparticles to deliver his-tagged proteins with bioactivity is underdeveloped, but not impossible. For example, Schwaminger et al. (2019) demonstrated that unfunctionalized bare iron oxide (magnetite Fe3O4) of 12 nm can bind his-tagged GFP (green fluorescence protein) directly from the cell lysates, with 91% purity. However, in a separate study, iron (III) oxide nanoparticles were found to have the lowest affinity towards his-tagged GFP among the ferrites (Cu, Ni, Co) being tested, with CuFe2O4 showing the highest affinity (Park et al., 2018). As discussed earlier, nanoparticles with Cu, Ni and Co may not be suitable for in vivo applications due to their toxicity.
2.3 Iron-catechol binding
Iron can also form complexes with catechol ligands, notably siderophores but also including derivatives such as adrenaline and dopamine. Dopamine is an essential neuromodulator in the human body and a dysfunctional dopamine system is to be linked to the development of Parkinson’s disease (Speranza et al., 2021). Indeed, dopamine has an intrinsic affinity to iron, a relationship that is used in regulating the dopaminergic neurotransmission (Arreguin et al., 2009; Pino et al., 2017). Such affinity is so strong that dopamine can penetrate stable iron chelates for coordination to form a catechol-iron complex. Scientists have been exploiting such a naturally occurring binding phenomenon for functionalization of iron oxide nanoparticles (Xu et al., 2004), as illustrated in Figure 1AIII. Coordination of dopamine onto iron oxide nanoparticles can generate an amine surface, which is positively charged and allows further modification via organic reactions. However, it was also found that dopamine coordination can facilitate the degradation of iron oxide nanoparticles (Shultz et al., 2007). This degradation may also be seen as positive as a possible excretion mechanism for the spent nanoparticles.
Due to such strong bonding, catechols have also been used to stabilize iron oxide nanoparticles. For example, Amstad et al. (2009) demonstrated the binding of several catechol-modified PEG (5 kDa) onto iron oxide nanoparticles for enhancing colloidal stabilization (Zvarec et al., 2013). Similarly, a catechol-modified chitosan has also been used to stabilize iron oxide nanoparticles. However, in cases of using catechols for nanoparticle stabilization, the bioactive molecules to be delivered will need to be attached onto the stabilizing agents (e.g., PEG or chitosan) via further functionalization.
3 Gold-iron oxide (Au/Fe3O4) nanoparticles
Among the aforementioned transition metal nanoplatforms, gold and iron oxide nanoparticles are by far the most popular choices in research for in vivo biomedical applications. Both are considered to be of low cytotoxicity. While Au can enhance CT-imaging (Bao et al., 2022) and ultrasound imaging (Li et al., 2018), iron(II,III) oxide, or magnetite, nanoparticles had been widely used for MR contrasting (Olariu et al., 2011; Yiu et al., 2012; Yue et al., 2022) due to their superparamagnetic property. In addition, magnetic iron oxide nanoparticles (including maghemite, γ-Fe2O3) have also been tested for targeted drug delivery (Kim et al., 2020) and hyperthermia (Wang et al., 2022), using an external magnetic field. Therefore, nano-composite hybrids of these two components would lead to the development of “multi-functional” nanoplatforms (Leung et al., 2012).
3.1 Gold-coated iron oxide nanoparticles
Gold-coated iron oxide magnetic nanoparticles have been reported as early as in 2001 (Lin et al., 2001; Zhou et al., 2001). Initially, the gold coating served as a protective coating for the magnetic core, as well as an active layer for attaching bioactive molecules, as illustrated in Figure 1BI. However, even to date, not many reports on Au-coated iron oxide nanoparticles for biomedical applications have provided conclusive evidence, e.g., TEM images, that the magnetic cores were completely encapsulated in a non-porous gold coating (e.g., Hoang et al., 2022). Indeed, such a continuous but thin gold coating is difficult to achieve. This is because the deposition mechanism of gold is mostly linked to the precipitation from [AuCl4]− salt by increasing the pH (using a base such as NH3 or NaOH) of the suspension of magnetic iron oxide nanoparticles. This tends to form gold clusters at a nanoscale on the iron oxide nanoparticle surface rather than a thin layer of coating. Another difficulty arises as these gold nanoclusters cannot always be easily identified using conventional analytical techniques such as XRD and TEM if the dimension of the Au coating is less than a few nanometers. Small non-crystalline clusters showed very broad peaks with low intensity in XRD while the EDX (elemental mapping) function of TEM does not have such a high resolution at a few nanometers scale. Therefore, many of these reported “gold-coated magnetic iron oxide” nanoparticles reported in the literature are essentially composite with gold deposited on an iron oxide host as nanoclusters. Non-etheless, these composites do possess the critical chemical and physical properties of both gold and iron oxide nanoparticles, allowing these nanoplatforms to perform specific tasks that they were designed for. Li et al. (2014) showed a detailed analysis of an Au-coated Fe3O4 nanoparticle sample at a high resolution and demonstrated its use in NIR-triggered drug delivery for cancer therapy. However, in order to adapt to the NIR-responsiveness, a complex coating is required for drug release.
3.2 Gold-iron oxide Janus nanoparticles
Janus nanoparticles are dumbbell-like particles composed of two-halves of different components, in this case, gold and iron oxide, as shown in Figure 1BII. They are usually formed by growing the second component (usually gold) on the surface of a particle of the first component (iron oxide) under a controlled manner. However, controlling such growth so that a true Janus nanoparticle is to form can be difficult. There are dozens of reports on gold-iron oxide Janus nanoparticles since the early 2010s but only a few reported particles are of verified dumbbell morphology (Song et al., 2017; Liu et al., 2019; Efremova et al., 2021).
Moreover, most of these reports on gold-iron oxide Janus nanoparticles are prepared for multimodal imaging with enhanced contrasting properties (e.g., via plasmonic resonance) (Chen et al., 2017; Liu et al., 2019). Applications of these Janus nanoparticles as nanoplatforms for the delivery of bioactive molecules are not widely reported. One possible reason is that the morphology of a Janus object does not give a high surface area-to-volume ratio of gold. Therefore, it does not help to improve their binding capacity. If the gold components are dispersed on the iron oxide surface as small clusters, the amount of gold on surface is maximized, leading to a higher binding capacity of the bioactive molecules.
3.3 Gold-dotted iron oxide nanoparticles and other examples
To maximize the gold surface for binding thiolated or cysteine species, gold-dotted iron oxide nanoparticles are developed. There are two main designs; gold nanoparticles impregnated directly onto the iron oxide surface and gold nanoparticles bound onto a thiolated surface of a thiol-functionalized iron oxide nanoparticle (as seen in Figure 1BII). The resultant materials have little difference in terms of ability to bind thiolated compounds but direct impregnation is a simpler procedure to prepare these gold-dotted iron oxide nanoparticles. Zhao et al. demonstrated the synthesis of “strawberry-like” Au-dotted magnetic nanoparticles for CT-MR dual-contrast imaging via a thiolated magnetic nanoparticle as the core (Zhao et al., 2015). The nanoparticles were also embedded with a fluorescence property, allowing a powerful diagnosis for liver diseases. Indeed, biomedical imaging and diagnosis using Au/Fe3O4 nanoparticles is a well-established area of research. For example, Hu et al. (2016) reported an Au/Fe3O4 “nanostar” structure for multimodal imaging while Wang et al. (2016) used a “nanocage” Au/Fe3O4 structure to perform T1-T2 dual MRI diagnosis for tumours. In all these examples, the Au/Fe3O4 nanostructures were shown to be biocompatible.
4 Case study
Previously we have reported gold-dotted iron oxide (Au/Fe3O4) nanoparticles that possessed MR-CT-ultrasound trimodal contrasting capacity (Kuhn et al., 2020). More importantly, these Au/Fe3O4 nanoparticles were found to have low toxicity in vitro to MCF-7 breast cancer cell lines, paving way for being developed as a delivery nanoplatform for small molecules in vivo. Figure 2 shows the key characterizations for an Au/Fe3O4 nanoparticle (10% w/w Au) sample. In short, the core Fe3O4 nanoparticle is around 30 nm in diameter with gold “dots” of around 2 nm dispersed on the surface (Figures 2A, B). The XRD result (Figure 2C) is consistent with a typical Fe3O4 diffraction pattern (JCPDS Card No. 019-0629). The SQUID magnetometry in Figure 2D shows a superparamagnetic character with a magnetization in saturation of 27 emu.g−1 at 15 kOe (See Supplementary Information for experimental procedures).
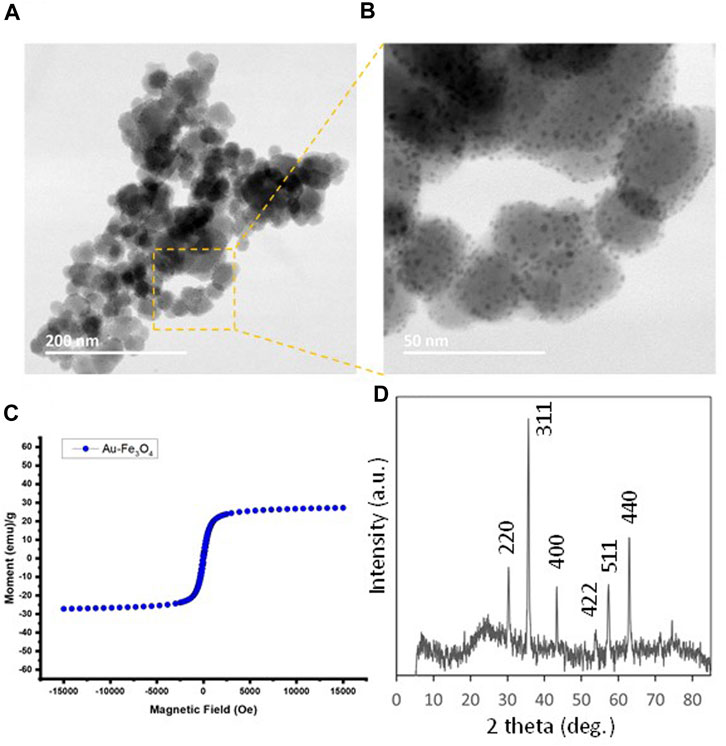
FIGURE 2. (A, B) TEM images for Au-dotted Fe3O4 (10%) nanoparticles prepared by impregnation of gold. (C) Magnetization curve (M vs. H plot) of Au-dotted Fe3O4 (10%) nanoparticles at 298K from a SQUID measurement. (D) XRD powder diffraction pattern for Au-dotted Fe3O4 (10%) nanoparticles, showing predominantly Fe3O4 peaks.
We have chosen two small peptide molecules, insulin and oxytocin, as model biomolecules, both of which are peptides having cysteine residues and, hence, suitable for direct binding to the Au component on Au/Fe3O4 surface. It was found that the binding capacities were 0.47 mg g−1 for insulin and 0.91 mg g−1 for oxytocin, which are comparable values to many nanoplatform systems in the literature.
We also carried out further investigations on the surface binding of insulin using zeta potential measurements. Au/Fe3O4 (10%) suspended in PBS showed a strong negatively charged surface (−26.7 mV) due to the surface-bound phosphate ions. When insulin was bound onto the surface, the zeta potential reduced to −12.3 mV, showing that insulin replaced the weakly bound phosphates. We also used dopamine to deactivate the Fe3O4 surface on the Au/Fe3O4 sample, reducing the zeta potential to −21.0 mV. Using this dopamine-Au/Fe3O4 sample for insulin binding, a zeta potential of −17.0 mV was obtained, suggesting that the phosphate bound on the Au surface was being replaced by insulin. This also suggested that insulin can also bind on the Fe3O4 surface, which is consistent with Schwaminger’s work (Schwaminger et al., 2015).
In order to ensure the safe use of nanoparticles and their sustainability in production, benign chemicals should be considered. For example, in this synthesis, urea was chosen as a mild base for Au deposition. This method is adapted from the preparation of metal-on-oxide supported catalysts. Therefore, scaling up is possible (up to several g per batch in laboratories), making such delivery platforms more suitable for use in the public health sector, compared with some systems reported in the literature where batch size can be limited to below 100 mg. The use of sonication allows an even dispersion of Au nanoparticles on the iron oxide surface, with a narrow particle size distribution. Also because of the nature of catalyst preparation, this method can also be adapted tois also adaptable for preparering a widehile range of metal/-support combinations, e.g., Pt/ZnO, when other properties of nanoparticles are required for specific applications.
However, there are only a small number of metal-to-organic interactions that have been exploited for self-assembly for biomedical applications. As mentioned previously, one reason is that the potential toxicity of components limits the choice of transition metals and organic groups that can be used. Modification of the small bioactive molecules should also be carefully considered as it could deactivate these molecules, causing them to lose their primary functionalities.
Compared with small bioactive peptides, binding and delivery of larger bioactive peptides may be more straightforward. First, as the peptide molecules get larger, there are a larger number of amino acid units on the surface of the peptide that may be active for binding onto the nanoparticle surface. Moreover, the orientation of these large peptides on the nanoparticle surface can also be manipulated by protein engineering, including the addition of N-terminal or C-terminal His-tags or Cys-tags, similar to the strategy that is commonly used for protein/enzyme purification. As such the exposure of active sites on the peptide can be maximized. However, adding His-tags or Cys-tags to small peptide molecules may not always be desirable as these tags may alter the fundamental characteristics significantly to a small peptide with a much shorter amino acid sequence.
5 Conclusion
Nanoplatforms for the delivery of bioactive molecules could be a breakthrough for nanomedicine and much advancement can be seen in the past two decades. Whilst many reports have focused on the (multi-) functionalization of the nanoparticle surface via organic reactions, self-assembly of bioactive molecules on purely inorganic nanoplatforms allows for simpler materials preparation, enhancing process sustainability of manufacturing, as additional organic reactions will increase the use of organic chemicals and solvents. The self-assembly method is based on the formation of coordinating bonds between the bioactive molecules and the transition metal sites on the surface of the nanoparticles. Notable candidate pairings include Au-thiol, Fe-dihistidine, and Fe-catechols. As such, an Au/Fe nanoplatform can be developed for dual delivery, if the cargos carry suitable functionalities for self-assembly, and that is where the challenges lie. Large protein molecules can carry a Cys-tag or His-tag through protein engineering, delivery of smaller peptide molecules using this method may be more restrictive. The addition of Cys-tag or His-tag could alter the primary functions of these molecules, hence, careful evaluation of peptide modifications and peptide-carrier combinations will be necessary steps in development. Using insulin and oxytocin as model molecules, we also demonstrate that Au/Fe3O4 nanoparticles can carry 0.47 and 0.91 mg g−1 of these bioactive peptides respectively via the self-assembly mechanism. In conclusion, this self-assembly route is worth exploring if the cargo molecules carry groups that can form coordination bonds with the nanoplatforms.
Data availability statement
The original contributions presented in the study are included in the article/Supplementary Material, further inquiries can be directed to the corresponding author.
Author contributions
EL, DS, and HY: conceptualization, writing, original draft preparation, reviewing, and editing. EL, MÅ, OC, and AG: conducting the experiments. All authors provided editorial feedback, revisions, and approved the manuscript.
Funding
EL was funded by the EPSRC, United Kingdom through the CRITICAT CDT program (EP/L016419/1), and the Cross-disciplinary Research for Discovery Science programme from the NERC, United Kingdom.
Acknowledgments
We acknowledge the assistance from Colin How (Kelvin Nanocharacterisation Centre, University of Glasgow, United Kingdom) for TEM measurements.
Conflict of interest
The authors declare that the research was conducted in the absence of any commercial or financial relationships that could be construed as a potential conflict of interest.
Publisher’s note
All claims expressed in this article are solely those of the authors and do not necessarily represent those of their affiliated organizations, or those of the publisher, the editors and the reviewers. Any product that may be evaluated in this article, or claim that may be made by its manufacturer, is not guaranteed or endorsed by the publisher.
Supplementary material
The Supplementary Material for this article can be found online at: https://www.frontiersin.org/articles/10.3389/fmolb.2023.1143190/full#supplementary-material
References
Amstad, E., Gillich, T., Bilecka, I., Textor, M., and Reimhult, E. (2009). Ultrastable iron oxide nanoparticle colloidal suspensions using dispersants with catechol-derived anchor groups. Nano Lett. 9, 4042–4048. doi:10.1021/nl902212q
Arreguin, S., Nelson, P., Padway, S., Shirazi, M., and Pierpont, C. (2009). Dopamine complexes of iron in the etiology and pathogenesis of Parkinson's disease. J. Inorg. Biochem. 103, 87–93. doi:10.1016/j.jinorgbio.2008.09.007
Bao, H., Cheng, S., Li, X., Li, Y., Yu, C., Huang, J., et al. (2022). Functional Au nanoparticles for engineering and long-term CT imaging tracking of mesenchymal stem cells in idiopathic pulmonary fibrosis treatment. Biomaterials 288, 121731. doi:10.1016/j.biomaterials.2022.121731
Bewley, K. D., Ellis, K. E., Firer-Sherwood, M. A., and Elliott, S. J. (2013). Multi-heme proteins: nature's electronic multi-purpose tool. Biochim. Biophys. Acta 1827, 938–948. doi:10.1016/j.bbabio.2013.03.010
Chen, X., Li, G., Han, Q., Li, X., Li, L., Wang, T., et al. (2017). Rational design of branched Au-Fe3O4 Janus nanoparticles for simultaneous trimodal imaging and photothermal therapy of cancer cells. Chem. Eur. J. 23, 17204–17208. doi:10.1002/chem.201704514
Crawley, J. W. M., Gow, I. E., Lawes, N., Kowalec, I., Kabalan, L., Catlow, C. R. A., et al. (2022). Heterogeneous trimetallic nanoparticles as catalysts. Chem. Rev. 122, 6795–6849. doi:10.1021/acs.chemrev.1c00493
Efremova, M. V., Spasova, M., Heidelmann, M., Grebennikov, I. S., Li, Z. A., Garanina, A. S., et al. (2021). Room temperature synthesized solid solution AuFe nanoparticles and their transformation into Au/Fe Janus nanocrystals. Nanoscale 13, 10402–10413. doi:10.1039/d1nr00383f
Elhassan, E., Devnarain, N., Mohammed, M., Govender, T., and Omolo, C. A. (2022). Engineering hybrid nanosystems for efficient and targeted delivery against bacterial infections. J. Control. Release 351, 598–622. doi:10.1016/j.jconrel.2022.09.052
Eskandani, R., Kazempour, M., Farahzadi, R., Sanaat, Z., Eskandani, M., Adibkia, K., et al. (2022). Engineered nanoparticles as emerging gene/drug delivery systems targeting the nuclear factor-κB protein and related signaling pathways in cancer. Biomed. Pharmacother. 156, 113932. doi:10.1016/j.biopha.2022.113932
Ghosh, P., Han, G., De, M., Kim, C. K., and Rotello, V. M. (2008). Gold nanoparticles in delivery applications. Adv. Drug Deliv. Rev. 60, 1307–1315. doi:10.1016/j.addr.2008.03.016
Heimel, G., Romaner, L., Brédas, J.-L., and Zojer, E. (2006). Interface energetics and level alignment at covalent metal-molecule junctions: π-Conjugated thiols on gold. Phys. Rev. Lett. 96, 196806. doi:10.1103/PhysRevLett.96.196806
Hoang, K. N. L., Wheeler, K. E., and Murphy, C. J. (2022). Isolation methods influence the protein corona composition on gold-coated iron oxide nanoparticles. Anal. Chem. 94, 4737–4746. doi:10.1021/acs.analchem.1c05243
Hopkins, C., Javius-Jones, K., Wang, Y., Hong, H., Hu, Q., and Hong, S. (2022). Combinations of chemo-immuno-and gene therapies using nanocarriers as a multifunctional drug platform. Expert Opin. Drug Deliv. 19, 1337–1349. doi:10.1080/17425247.2022.2112569
Hu, Y., Wang, R., Wang, S., Ding, L., Li, J., Luo, Y., et al. (2016). Multifunctional Fe3O4@Au core/shell nanostars: A unique platform for multimode imaging and photothermal therapy of tumors. Sci. Rep. 6, 28325. doi:10.1038/srep28325
Kim, D. H., Kim, D. W., Jang, J. Y., Lee, N., Ko, Y. J., Lee, S. M., et al. (2020). Fe3O4@Void@Microporous organic polymer-based multifunctional drug delivery systems: Targeting, imaging, and magneto-thermal behaviors. ACS Appl. Mater. Interface 12, 37628–37636. doi:10.1021/acsami.0c12237
Kruppa, M., and König, B. (2006). Reversible coordinative bonds in molecular recognition. Chem. Rev. 106, 3520–3560. doi:10.1021/cr010206y
Kuhn, J., Papanastasiou, G., Tai, C. W., Moran, C. M., Jansen, M. A., Tavares, A. A., et al. (2020). Tri-modal imaging of gold-dotted magnetic nanoparticles for magnetic resonance imaging, computed tomography and intravascular ultrasound: An in vitro study. Nanomedicine 15, 2433–2445. doi:10.2217/nnm-2020-0236
Kumar, K., and Paik, P. (2021). Protein immobilization on heterogeneous (SiO2/ZnO) hollow-mesoporous nanocapsules prepared by imprinting CPMV: Drug delivery and possibility of immunological applications. ACS Biomater. Sci. Eng. 7, 4847–4858. doi:10.1021/acsbiomaterials.1c01043
Leung, K. C., Xuan, S., Zhu, X., Wang, D., Chak, C. P., Lee, S. F., et al. (2012). Gold and iron oxide hybrid nanocomposite materials. Chem. Soc. Rev. 41, 1911–1928. doi:10.1039/c1cs15213k
Li, Y., and Zhang, H. (2019). Fe3O4-based nanotheranostics for magnetic resonance imaging-synergized multifunctional cancer management. Nanomedicine 14, 1493–1512. doi:10.2217/nnm-2018-0346
Li, W.-P., Liao, P.-Y., Su, C.-H., and Yeh, C.-S. (2014). Formation of oligonucleotide-gated silica shell-coated Fe3O4-Au core-shell nanotrisoctahedra for magnetically targeted and near-infrared light-responsive theranostic platform. J. Am. Chem. Soc. 136, 10062–10075. doi:10.1021/ja504118q
Li, X. Y., Xu, L., Li, H. L., Du, J., Liu, X. S., and Li, F. H. (2018). Au-poly(lactic-co-glycolic) acid complex nanoparticles as ultrasound contrast agents: Preparation, characterization and in vitro study. Mol. Med. Rep. 17, 3763–3768. doi:10.3892/mmr.2017.8351
Li, K., Luo, Y., Gao, L., Li, T., and Duan, G. (2020). Au-decorated ZnFe2O4 yolk-shell spheres for trace sensing of chlorobenzene. ACS Appl. Mater. Interface 12, 16792–16804. doi:10.1021/acsami.0c00525
Li, B., Shao, H., Gao, L., Li, H., Sheng, H., and Zhu, L. (2022). Nano-drug co-delivery system of natural active ingredients and chemotherapy drugs for cancer treatment: A review. Drug Deliv. 29, 2130–2161. doi:10.1080/10717544.2022.2094498
Lin, J., Zhou, W., Kumbhar, A., Wiemann, J., Fang, J., Carpenter, E. E., et al. (2001). Gold-coated iron (Fe@Au) nanoparticles: Synthesis, characterization, and magnetic field-induced self-assembly. J. Solid State Chem. 159 (1), 26–31. doi:10.1006/jssc.2001.9117
Liu, J., Chakraborty, S., Hosseinzadeh, P., Yu, Y., Tian, S., Petrik, I., et al. (2014). Metalloproteins containing cytochrome, iron-sulfur, or copper redox centers. Chem. Rev. 114 (8), 4366–4469. doi:10.1021/cr400479b
Liu, F., Goyal, S., Forrester, M., Ma, T., Miller, K., Mansoorieh, Y., et al. (2019). Self-assembly of Janus dumbbell nanocrystals and their enhanced surface plasmon resonance. Nano Lett. 19, 1587–1594. doi:10.1021/acs.nanolett.8b04464
Ma, A., Baek, S. Y., Seo, J. H., Abbas, S. A., Kwon, J.-H., Ahn, S. J., et al. (2021). Photodeposition of Pt nanoparticles on Co3O4 nanocubes for detection of acetone at part-per-billion levels. ACS Appl. Nano Mater. 4, 2752–2759. doi:10.1021/acsanm.0c03393
Magaye, R., Zhao, J., Bowman, L., and Ding, M. (2012). Genotoxicity and carcinogenicity of cobalt-nickel- and copper-based nanoparticles. Exp. Ther. Med. 4, 551–561. doi:10.3892/etm.2012.656
Maraming, P., and Kah, J. C. Y. (2021). Conjugation of peptides to gold nanoparticles. Methods Mol. Biol. 2355, 9–16. doi:10.1007/978-1-0716-1617-8_2
McBain, S.C., Yiu, H.H.P., and Dobson, J. (2008). Magnetic nanoparticles for gene and drug delivery. Int. J. Nanomedicine. 3, 169–180.
Olariu, C., Yiu, H. H. P., Bouffier, L., Nedjadi, T., Costello, E., Williams, S., et al. (2011). Multifunctional Fe3O4 nanoparticles for targeted bi-modal imaging of pancreatic cancer. J. Mater. Chem. 21, 12650–12659. doi:10.1039/c1jm11370d
Ou, X., Liu, Y., Zhang, M., Hua, L., and Zhan, S. (2021). Plasmonic gold nanostructures for biosensing and bioimaging. Microchim. Acta 188, 304. doi:10.1007/s00604-021-04964-1
Palanisamy, S., and Wang, Y.-M. (2019). Superparamagnetic iron oxide nanoparticulate system: Synthesis, targeting, drug delivery and therapy in cancer. Dalton Trans. 48, 9490–9515. doi:10.1039/c9dt00459a
Park, S. J., Kim, S., Kim, S. H., Park, K. M., and Hwang, B. H. (2018). His-tagged protein immobilization on cationic ferrite magnetic nanoparticles. Korean J. Chem. Eng. 35, 1297–1302. doi:10.1007/s11814-018-0043-7
Pinakidou, F., Simeonidis, K., Myrovali, E., Brzhezinskaya, M., Paloura, E. C., Angelakeris, M., et al. (2022). Addressing the effect of magnetic particle hyperthermia application on the composition and spatial distribution of iron oxide nanoparticles using X-ray spectroscopic techniques. J. Phys. Chem. C 126, 10101–10109. doi:10.1021/acs.jpcc.2c01248
Pino, J. M. V., da Luz, M. H. M., Antunes, H. K. M., Giampá, S. Q. C., Martins, V. R., and Lee, K. S. (2017). Iron-restricted diet affects brain ferritin levels, dopamine metabolism and cellular prion protein in a region-specific manner. Manner. Front. Mol. Neurosci. 10, 145. doi:10.3389/fnmol.2017.00145
Pissuwan, D., Niidome, T., and Cortie, M. B. (2011). The forthcoming applications of gold nanoparticles in drug and gene delivery systems. J. Control Release 149, 65–71. doi:10.1016/j.jconrel.2009.12.006
Pucci, C., Degl'Innocenti, A., Belenli Gümüş, M., and Ciofani, G. (2022). Superparamagnetic iron oxide nanoparticles for magnetic hyperthermia: Recent advancements, molecular effects, and future directions in the omics era. Biomater. Sci. 10, 2103–2121. doi:10.1039/d1bm01963e
Qin, Y. T., Feng, Y. S., Ma, Y. J., He, X. W., Li, W. Y., and Zhang, Y. K. (2020). Tumor-sensitive biodegradable nanoparticles of molecularly imprinted polymer-stabilized fluorescent zeolitic imidazolate framework-8 for targeted imaging and drug delivery. ACS Appl. Mater. Interface 12, 24585–24598. doi:10.1021/acsami.0c05154
Schwaminger, S. P., García, P. F., Merck, G. K., Bodensteiner, F. A., Heissler, S., Günther, S., et al. (2015). Nature of interactions of amino acids with bare magnetite nanoparticles. J. Phys. Chem. C 119, 23032–23041. doi:10.1021/acs.jpcc.5b07195
Schwaminger, S. P., Fraga-García, P., Blank-Shim, S. A., Straub, T., Haslbeck, M., Muraca, F., et al. (2019). Magnetic one-step purification of his-tagged protein by bare iron oxide nanoparticles. ACS Omega 4, 3790–3799. doi:10.1021/acsomega.8b03348
Sharifianjazi, F., Irani, M., Esmaeilkhanian, A., Bazli, L., Asl, M. S., Jang, H. W., et al. (2021). Polymer incorporated magnetic nanoparticles: Applications for magnetoresponsive targeted drug delivery. Mater. Sci. Eng. B 272, 115358. doi:10.1016/j.mseb.2021.115358
Shultz, M. D., Reveles, J. U., Khanna, S. N., and Carpenter, E. E. (2007). Reactive nature of dopamine as a surface functionalization agent in iron oxide nanoparticles. J. Am. Chem. Soc. 129, 2482–2487. doi:10.1021/ja0651963
Song, J., Wu, B., Zhou, Z., Zhu, G., Liu, Y., Yang, Z., et al. (2017). Double-layered plasmonic-magnetic vesicles by self-assembly of Janus amphiphilic gold-iron(II,III) oxide nanoparticles. Angew. Chem. Int. Ed. 56, 8110–8114. doi:10.1002/anie.201702572
Speranza, L., Di Porzio, U., Viggiano, D., de Donato, A., and Volpicelli, F. (2021). Dopamine: The neuromodulator of long-term synaptic plasticity, reward and movement control. Cells 10, 735. doi:10.3390/cells10040735
Ulman, A. (2001). Self-assembled monolayers of 4-mercaptobiphenyls. Acc. Chem. Res. 34, 855–863. doi:10.1021/ar0001564
van der Meer, S. B., Seiler, T., Buchmann, C., Partalidou, G., Boden, S., Loza, K., et al. (2021). Controlling the surface functionalization of ultrasmall gold nanoparticles by sequence-defined macromolecules. Chem. Eur. J. 27, 1451–1464. doi:10.1002/chem.202003804
Wan Ibrahim, W. A., Nodeh, H. R., Aboul-Enein, H. Y., and Sanagi, M. M. (2015). Magnetic solid-phase extraction based on modified ferum oxides for enrichment, preconcentration, and isolation of pesticides and selected pollutants. Crit. Rev. Anal. Chem. 45, 270–287. doi:10.1080/10408347.2014.938148
Wang, G., Gao, W., Zhang, X., and Mei, X. (2016). Au nanocage functionalized with ultra-small Fe3O4 nanoparticles for targeting T1-T2Dual MRI and CT imaging of tumor. Sci. Rep. 6, 28258. doi:10.1038/srep28258
Wang, Q., Cheng, Y., Wang, W., Tang, X., and Yang, Y. (2022). Polyetherimide- and folic acid-modified Fe3 O4 nanospheres for enhanced magnetic hyperthermia performance. J. Biomed. Mater. Res. B Appl. Biomater. 111, 795–804. Advance Article. doi:10.1002/jbm.b.35190
Wu, P., and Yan, X. P. (2013). Doped quantum dots for chemo/biosensing and bioimaging. Chem. Soc. Rev. 42, 5489–5521. doi:10.1039/c3cs60017c
Wu, L., Wang, C., and Li, Y. (2022). Iron oxide nanoparticle targeting mechanism and its application in tumor magnetic resonance imaging and therapy. Nanomed. Adv. Artic. 17, 1567–1583. doi:10.2217/nnm-2022-0246
Xiong, Y., Su, L., Peng, Y., Zhao, S., and Ye, F. (2022). Dextran-coated Gd-based ultrasmall nanoparticles as phosphatase-like nanozyme to increase ethanol yield via reduction of yeast intracellular ATP level. J. Colloid Interface Sci. 627, 405–414. doi:10.1016/j.jcis.2022.07.036
Xu, C., Xu, K., Gu, H., Zheng, R., Liu, H., Zhang, X., et al. (2004). Dopamine as a robust anchor to immobilize functional molecules on the iron oxide shell of magnetic nanoparticles. J. Am. Chem. Soc. 126, 9938–9939. doi:10.1021/ja0464802
Xu, W., Ye, C., Qing, X., Liu, S., Lv, X., Wang, W., et al. (2022). Multi-target tyrosine kinase inhibitor nanoparticle delivery systems for cancer therapy. Mater. Today. Bio 16, 100358. doi:10.1016/j.mtbio.2022.100358
Yadav, G. D., Mewada, R. K., Wagh, D. P., and Manyar, H. G. (2022). Advances and future trends in selective oxidation catalysis: A critical review. Catal. Sci. Technol. 12, 7245–7269. Advance Article. doi:10.1039/D2CY01322C
Ye, F., Barrefelt, A., Asem, H., Abedi-Valugerdi, M., El-Serafi, I., Saghafian, M., et al. (2014). Biodegradable polymeric vesicles containing magnetic nanoparticles, quantum dots and anticancer drugs for drug delivery and imaging. Biomaterials 35, 3885–3894. doi:10.1016/j.biomaterials.2014.01.041
Yiu, H. H. P., Pickard, M. R., Olariu, C. I., Williams, S. R., Chari, D. M., and Rosseinsky, M. J. (2012). Fe3O4-PEI-RITC magnetic nanoparticles with imaging and gene transfer capability: Development of a tool for neural cell transplantation therapies. Pharm. Res. 29, 1328–1343. doi:10.1007/s11095-011-0632-1
Yiu, H. H. P., Bouffier, L., Boldrin, P., Long, J., Claridge, J. B., and Rosseinsky, M. J. (2013). Comprehensive study of DNA binding on iron (II,III) oxide nanoparticles with a positively charged polyamine three-dimensional coating. Langmuir 29, 11354–11365. doi:10.1021/la400848r
Yiu, H. H. P. (2011). Engineering the multifunctional surface on magnetic nanoparticles for targeted biomedical applications: A chemical approach. Nanomedicine 6, 1429–1446. doi:10.2217/nnm.11.132
You, C., and Piehler, J. (2014). Multivalent chelators for spatially and temporally controlled protein functionalization. Anal. Bioanal. Chem. 406, 3345–3357. doi:10.1007/s00216-014-7803-y
Yousefvand, M., Mohammadi, Z., Ghorbani, F., Irajirad, R., Abedi, H., Seyedi, S., et al. (2021). Investigation of specific targeting of triptorelin-conjugated dextran-coated magnetite nanoparticles as a targeted probe in GnRH+ cancer cells in MRI. Contrast Media Mol. Imaging 2021, 5534848. doi:10.1155/2021/5534848
Yue, S., Zhang, X., Xu, Y., Zhu, L., Cheng, J., Qiao, Y., et al. (2022). The influence of surface charge on the tumor-targeting behavior of Fe3O4 nanoparticles for MRI. J. Mater. Chem. B 10, 646–655. doi:10.1039/d1tb02349g
Zastrow, M. L., and Pecoraro, V. L. (2013). Designing functional metalloproteins: From structural to catalytic metal sites. Coord. Chem. Rev. 257, 2565–2588. doi:10.1016/j.ccr.2013.02.007
Zhao, H. Y., Liu, S., He, J., Pan, C. C., Li, H., Zhou, Z. Y., et al. (2015). Synthesis and application of strawberry-like Fe3O4-Au nanoparticles as CT-MR dual-modality contrast agents in accurate detection of the progressive liver disease. Biomaterials 51, 194–207. doi:10.1016/j.biomaterials.2015.02.019
Zhao, S., Yu, X., Qian, Y., Chen, W., and Shen, J. (2020). Multifunctional magnetic iron oxide nanoparticles: An advanced platform for cancer theranostics. Theranostics 10, 6278–6309. doi:10.7150/thno.42564
Zhou, W. L., Carpenter, E. E., Lin, J., Kumbhar, A., Sims, J., and O'Connor, C. J. (2001). Nanostructures of gold coated iron core-shell nanoparticles and the nanobands assembled under magnetic field. Eur. Phys. J. D. 8, 289–292. doi:10.1007/s100530170112
Keywords: iron oxide, gold, magnetite nanoparticle, insulin, cysteine, dopamine, Cys-tag, drug delivery
Citation: Lau ECHT, Åhlén M, Cheung O, Ganin AY, Smith DGE and Yiu HHP (2023) Gold-iron oxide (Au/Fe3O4) magnetic nanoparticles as the nanoplatform for binding of bioactive molecules through self-assembly. Front. Mol. Biosci. 10:1143190. doi: 10.3389/fmolb.2023.1143190
Received: 12 January 2023; Accepted: 15 March 2023;
Published: 27 March 2023.
Edited by:
Sebastian Esperante, Infant Foundation, ArgentinaReviewed by:
Atul Dev, University of California, Davis, United StatesSaumya Nigam, Michigan State University, United States
Copyright © 2023 Lau, Åhlén, Cheung, Ganin, Smith and Yiu. This is an open-access article distributed under the terms of the Creative Commons Attribution License (CC BY). The use, distribution or reproduction in other forums is permitted, provided the original author(s) and the copyright owner(s) are credited and that the original publication in this journal is cited, in accordance with accepted academic practice. No use, distribution or reproduction is permitted which does not comply with these terms.
*Correspondence: Humphrey H. P. Yiu, aC5oLnlpdUBody5hYy51aw==