- College of Traditional Chinese medicine, Tianjin University of Traditional Chinese Medicine, Tianjin, China
More than 25% of all malignant tumors are digestive system tumors (DSTs), which mostly include esophageal cancer, gastric cancer, pancreatic cancer, liver cancer, gallbladder cancer and cholangiocarcinoma, and colorectal cancer. DSTs have emerged as one of the prominent reasons of morbidity and death in many nations and areas around the world, posing a serious threat to human life and health. General treatments such as radiotherapy, chemotherapy, and surgical resection can poorly cure the patients and have a bad prognosis. A type of immunotherapy known as oncolytic virus therapy, have recently shown extraordinary anti-tumor effectiveness. One of the viruses that has been the subject of the greatest research in this field, the herpes simplex virus (HSV), has shown excellent potential in DSTs. With a discussion of HSV-1 based on recent studies, we outline the therapeutic effects of HSV on a number of DSTs in this review. Additionally, the critical function of HSV in the detection of cancers is discussed, and some HSV future possibilities are shown.
1 Introduction
Digestive system tumors (DSTs) are the most common cancers worldwide (Sung et al., 2021). The following DSTs have the highest global mortality rates in 2020: esophageal (5.5%), gastric (7.7%), pancreatic (4.7%), liver (8.3%), gallbladder (0.9%), and colorectal (9.4%). Except for gallbladder cancer, the rest of them increased in comparison to 2018 statistics (Bray et al., 2018). It is undeniably a major threat to human health and an urgent issue for both researchers and healthcare workers to address.
Currently, the most effective treatment modalities are still surgical resection, chemotherapy, radiotherapy, and conventional anti-cancer drug therapy, either alone or in combination (Zhao and Liu, 2020; Wei and Hackert, 2021). The prognosis of these treatments, however, has never met expectations, according to numerous reports (Lu et al., 2021; Yang et al., 2021), and patient survival rates after 5 years have not significantly increased. More reversing interventions need to be investigated and promoted in order to change this phenomenon as soon as possible. Many researchers have been successful in developing effective therapeutic targets (Li et al., 2022a; Xu et al., 2022) and microbial studies in recent years, and are moving toward targeted therapies and immunotherapies that can kill tumor cells more precisely (McAllister et al., 2019; Lu et al., 2021). Meanwhile, cancer patients have received immunotherapies like immune checkpoint therapy (ICT), tumor vaccines, immune adoptive therapy, and immunomodulator (Chen et al., 2021). These efforts all contribute to the search for distinctive breakthroughs against digestive tumors.
The oncolytic virus (OV) is a recent cancer treatment method among immunotherapies (Li et al., 2022d). OV selectively targets tumor cells as hosts, activates innate as well as adaptive anti-tumor immunity, and productively lyses tumor cells (Melcher et al., 2021; Li et al., 2022b). On the basis of the above advantages, OV gradually evolves into a potentially promising alternative and curative treatment to traditional cancer therapy. Oncolytic viruses come in a variety of forms, including those involving DNA, single-stranded RNA (ssRNA), and double-stranded RNA (dsRNA) viruses. Reports of associated clinical trials are rapidly rising (Fundora Ramos et al., 2021; Huang et al., 2021; Li et al., 2022c; Li and Sun, 2022). In 1991, herpes simplex virus (HSV) became the first genetically engineered virus to be utilized as an oncolytic virus (Martuza et al., 1991). T-VEC, a type of oncolytic virus that expresses granulocyte-macrophage colony-stimulating factor (GM-CSF), was approved by the FDA 25 years later (Pol et al., 2016). It is undoubtedly a milestone in viro-immunotherapy and believed that to be bloom in the future. Recent progress in viro-immunotherapy has reached this point, and additional growth is anticipated. In this review, we summarize the role of HSV and the diagnostic value that exists in the treatment of DSTs, as well as the clinical value and future of HSV.
2 Oncolytic virus
Tumor cells are granted several “privileges” in order to facilitate malignant proliferation and immune escape, but this comes at the expense of disrupting regulatory pathways and suppressing immune gene expression and inheritance (Ilkow et al., 2014). Oncolytic viruses are typically genetically modified viruses based on natural viruses that exploit this vulnerability to penetrate the barrier, invade cancer cells, and are greatly supported by the cancer cells’ crippled, malignant metabolic base (Hanahan and Weinberg, 2011), which serves as the foundation of an oncolytic virus as a new anti-tumor strategy. OV has been the subject of extensive investigation for more than 70 years. Despite its long-reported efficacy (Moore, 1949a;Moore, 1949b;Nemunaitis, 1999), the FDA just first approved T-VEC in 2015 as an oncolytic agent for the treatment of metastatic melanoma. T-VEC was produced on the basis of HSV-1 with the insertion of the gene called GM-CSF (Andtbacka et al., 2015; Pol et al., 2016).
The anti-tumor mechanism of oncolytic virus has been interpreted as: 1) selective infiltration and replication of tumor cells as hosts, causing direct cell lysis and death; and 2) induction of a systemic anti-tumor immune response, reactivating dormant innate and adaptive immunity (Russell et al., 2012; Engeland, 2020), restoring “control” to established immune escapees (Kaufman et al., 2015). However, we should mention that the oncolytic virus’ rebuilding of the tumor microenvironment (TME) has far-reaching implications for the latter (Zhu et al., 2022b; Su et al., 2022). These mechanisms have been thoroughly investigated, providing us with an insight into the irreplaceable nature of the oncolytic virus (Lichty et al., 2014; Heidbuechel and Engeland, 2021).
3 HSV as the oncolytic virus
3.1 Structure and advantages of HSV
The herpesvirus family is a sizable group of a large amount of double-stranded DNA viruses with envelopes (Scanlan et al., 2022). It was split up into the α, β, and γ subfamilies in 1978 based on the biological characteristics and genetic makeup of the viruses. HSV is a representative species of the α subfamily of herpesviruses, and in contrast to the other two, the α subfamily of herpesviruses is unique in having neuroinvasiveness (Knipe et al., 2013). HSV is split into the HSV-1 and HSV-2 serotypes depending on the antigenicity of each. The two virus types share 50% of their DNA, and they both carry common antigens between them as well as type-specific antigens. The viruses are round in shape and have 11 glycoproteins on their envelope surface that, when changed, have been proven to improve targeting to cancer cells (Sokolowski et al., 2015). Another factor in the choice of HSV as an oncolytic virus vector, in relation to this selective invasive effect, is that patients can still be kept safe with powerful anti-herpesviral medications like ganciclovir (GCV), even in life-threatening situations brought on by dose or self-specific reactions (Hartkopf et al., 2011; Koch et al., 2020).
HSV-1 has a genome of 150 kb and HSV-2 has a genome of 154 kb (Watson et al., 2012), both consisting of covalently linked unique long (UL) and unique short (US) fragments, respectively bordered by inverted repetitions (TR and IR) (Figure 1). Nearly half of the HSV genome is composed of non-essential genes, with a large scope for genetic modification, allowing both easy modification and the simultaneous insertion of large segments of exogenous genes or multiple transgenes into the viral genome (Table 1). This provides a great convenience for HSV to be used as an oncolytic virus. Other viruses only bind to one receptor, while HSV has four cellar receptors. HSV can infect practically all cells thanks to this advantageous characteristic (Watanabe and Goshima, 2018). Because of this, when HSV is used as an oncolytic agent, the problem of treatment resistance is considerably diminished. The aforementioned proof is the main justification for academics’ intense interest in oncolytic HSV.
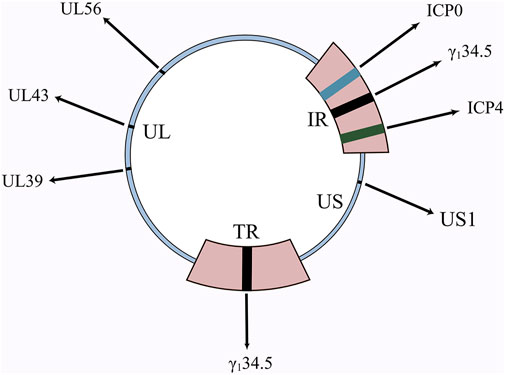
FIGURE 1. Schematic diagram of HSV genome and important genes. γ134.5, ICP0, ICP4, UL39, UL43, UL56 are all important genes in the HSV genome. The deletion of these genes, as well as the insertion of numerous other genes, has resulted in the birth of a diverse family of oncolytic HSVs.
3.2 How does HSV work?
3.2.1 Direct invasion and lysis of cancer cells
Pattern recognition receptors (PRR) consisting of Toll-like receptors (TLR) (Bansode et al., 2021), NOD-like receptors (NLR) (Dutta et al., 2015), and RIG-I-like receptors (RLR) (Unterholzner, 2013) that activate antiviral mechanisms are present on the surface and in the cytoplasm of normal cells. When a viral infection is encountered, PRR performs its vital function of antigen recognition and immune initiation at this moment. Immune signals are transmitted through a variety of different pathways, leading to the synthesis and release of a number of inflammatory factors, such as type I interferon (IFN-I) (Lin and Zheng, 2019; Zhu et al., 2022a; Li et al., 2022e; Gong et al., 2022).
The ingenious fact is that HSV is skilled at finding weaknesses in cancer cells’ faulty antiviral response system. It initially infects cancer cells by identifying and attaching to the proper receptors and then begins reproduction and amplification (Kaufman et al., 2015). For instance, HSV-1 enters the cell with the help of HVEM (herpesvirus entry medium) or envelope glycoproteins (Bommareddy et al., 2017). After DNA is inserted into the nucleus of cellular tumor cells, HSV uses intranuclear RNA polymerase to transcribe its own DNA into mRNA and then uses intracellular organelles to direct the synthesis of some proteins. These proteins, usually DNA replication enzymes and regulatory proteins, are synthesized preferentially at the earliest stage (Rice et al., 1995). This is followed by the replication of viral DNA and finally the synthesis of structural proteins such as capsid proteins and envelope proteins (Cui et al., 2022). The assembly of progeny virions is done in the nucleus (Mohan et al., 2020).
Continuous proliferation depletes tumor cells of resources and triggers its lysis apoptosis. Viral nucleic acids, capsid proteins, and new progeny virions during proliferation (Wang et al., 2022a), are released as the cancer cells are lysed and recognized as pathogen-associated molecular patterns (PAMPS). Cancer cell tumor-associated antigens (TAA) and damage-associated molecular patterns (DAMPS) have also been made public (Kaufman et al., 2015) (Figure 2).
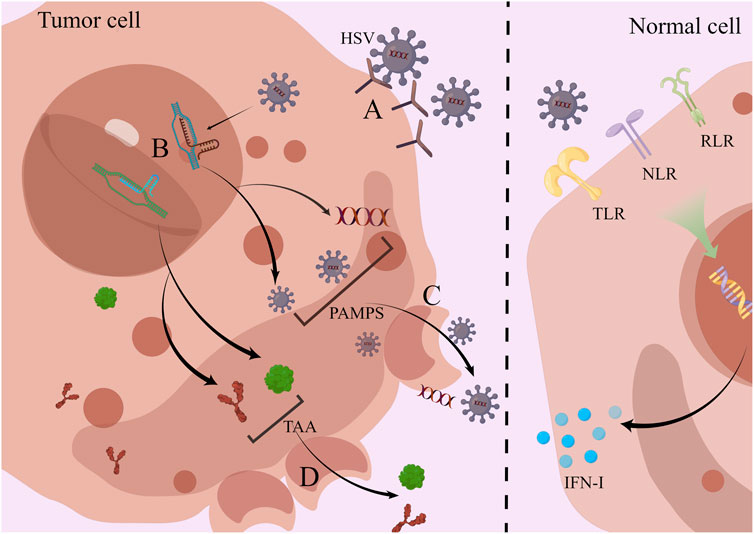
FIGURE 2. HSV directly infects and lyses tumor cells; normal cells activate antiviral effects via PRR. (A) HSV enters cells via HVEM or glycoproteins in the envelope. (B) Inside the nucleus, HSV uses cells for self-replication and value-added, producing large amounts of viral nucleic acids, viral capsid proteins, and progeny viral particles. (C) Viral proliferation leads to cell lysis, and PAMPS are released into the outside of the cell. (D) Along with cell lysis, TAA is also released which is highly expressed in tumor cells. In contrast to tumor cells, normal cells rely on PRRs such as TLR, NLR, and RLR to activate antiviral mechanisms and secrete inflammatory factors such as type I IFN to kill the virus.
3.2.2 Remodeling the tumor microenvironment and inducing systemic immunity
Typically, solid tumors contain a range of cellular and non-cellular components, consisting of immune cells, fibroblasts, and endothelial cells of the vascular system, cancer-associated fibroblasts (CAFs), extracellular matrix (ECM), in addition to cancer cells. These components make up the TME (Belli et al., 2018). Cancer cells and TME interact to create a sophisticated immune evasion network (Hanahan and Coussens, 2012), and this “fortress” has a negative impact on the body’s anti-tumor response (Gujar et al., 2018). It is crucial to recognize that TME creates a barrier or trap for the oncolytic virus (Vähä-Koskela and Hinkkanen, 2014), and the disturbance of capture or rejection is frequently what makes intratumoral injection superior to other delivery methods (Osali et al., 2020).
HSV remodels a “cold tumor” in an immunosuppressed state into a “hot tumor” in an immune-activated state. The distinction between “cold” and “hot” is made from an immunological perspective. T-cell are unable to infiltrate cold tumors, whereas hot tumors exhibit significant molecular characteristics of immune activation and an abundance of infiltrating T-cell (van der Woude et al., 2017). Infiltrating by T-cell, especially CD8+ T-cell, has been shown by several pieces of evidence to correlate with increased cancer cell killing and patient survival (Galon and Bruni, 2019; Newman et al., 2020). The change from cold to hot tumors can lead to an enhanced effect of TME receiving immune recognition, and inflammatory and immune cells are able to infiltrate toxicity into the TME to kill cancer cells (Samson et al., 2018). Moreover, this reversal of tumor induced by HSV has a distal effect and is not only present in certain specific sites or systems. Further evidence suggests that remodeling of TME by HSV with arousal of effector cells is a systemic immune activation response. For instance, in a mouse experiment, both the T-VEC injected area and the contralateral non-injected area showed morphological shrinkage of the tumor, and a significant rise in CD8+ T-cell was observed (Moesta et al., 2017). Against tumors, these T-cell are unquestionably effective. Clinical trial outcomes are also in agreement with this. Two lines of evidence demonstrating that cytotoxic T-cell produced after intra-tumor injection of T-VEC can be found in peripheral blood further support the general consensus that they induce systemic antitumor immunity (Kaufman et al., 2010; Ribas et al., 2017). In addition to CD8+ T-cell, variations of Treg cell levels occupy an essential place in the intricate network of TME. In human and mouse tumors, Treg cells foster tumor immune escape of cancer cells (Hori et al., 2003; Rech et al., 2012). A decrease of its level within the tumor has also been proved to be connected with the intervention of oncolytic viruses. In a mouse melanoma model, injection of oncolytic HSV leads to a decrease of intratumoral Tregs (Bozhanova et al., 2022). However, it has also been indicated that treatment with oncolytic HSV leads to an increase rather than a decrease in intratumoral Tregs levels (Ring et al., 2017). The possible explanation for the opposite result may be that oncolytic HSV treatment alone is not adequate to generate a transformation-mimetic advantage in some tumors prone to Tregs infiltration.
When released into the tumor and the circulation, PAMPS is recognized by PRR of various immune cell subsets, consisting of dendritic cells (DCs), natural killer (NK) cells, antigen presenting cells (APCs), and the corresponding immune cells secrete inflammatory factors such as IL-6, IL-12, and IFN-γ(Yan and Chen, 2012). As more immune cells get enriched around the tumor tissue as a result of these successive events (Oosenbrug et al., 2017), more these important immunoreactive substances will work on both the tumor cells and the HSV progeny viruses themselves, causing immunogenic cell death (ICD) (Kroemer et al., 2013). Usually the ICD involves immunogenic apoptosis, necrosis, pyroptosis, and autophagic cell death (Guo et al., 2014). Each of these cell death patterns can be instrumental in the effect of antitumor immune response (Workenhe et al., 2014). High-mobility group box 1 (HMGB1) is an intracellularly derived DAMPS that serves as a sensitive inflammatory signal and functions as a warning for cell death and microbial invasion (Yanai et al., 2009). Its release was examined in several oncolytic HSVs infestations (Workenhe et al., 2014). In this experiment, the rate of apoptosis was remarkably accelerated in all cases, indicating that ICD associated with HMGB1-mediated is quite beneficial for inducing antitumor immunity. The promotion of the immune process by ICD as resulted from other modalities has been equally reported (Workenhe and Mossman, 2014). Tumor cells struggle to easily escape and are subjected to several anticancer immunoreactive chemicals, whereas TAA is collected by APCs and given to T-cell in a cross-presentation pattern, activating their adaptive immune responses against that antigen (Shi et al., 2020) (Figure 3). The easy entry of HSV into cells and the necessity for the activation of immune cells and the promotion of the release of inflammatory factors are both mediated by signaling pathways associated with viral clearance, such as TLR and protein kinase RNA activation (PKR) pathways, which are restricted in cancer cells (Zhao et al., 2020).
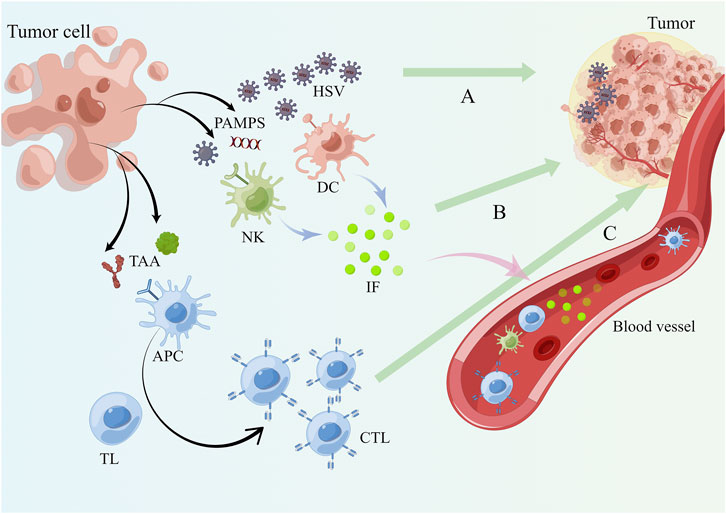
FIGURE 3. HSV remodels the TME and induces systemic anti-tumor immunity. (A) After the tumor cells are lysed, PAMPS are released and the mature HSV virus continues to infect other tumor cells in the TME. (B) PAMPS are recognized by immune cell subsets present in the TME such as DC, NK and APC relying on PRR which secrete immune inflammatory factors like IFN-γ, IL-6 and IL-12. (C) APC phagocytose TAA and PAMPS and present antigens to T lymphocytes, activating them to become cytotoxic T lymphocytes to complete the adaptive immunity against tumor cells. Blood circulation allows mature progeny viruses, immune cells and immune molecules to reach the whole body and a systemic anti-tumor immune response occurs.
4 Diagnosis and treatment of DST by HSV
4.1 Esophageal cancer
According to histopathology, esophageal cancer is classified into two types: squamous cell carcinoma (ESCC) and adenocarcinoma (EAC) (Siewert and Ott, 2007). EAC is more common in North America as well as Western Europe, whereas ESCC is more common in Eastern Europe and Asia (Ajani et al., 2019). However, conventional treatments frequently have poor outcomes, and resistance to chemotherapy and radiotherapy in both cases has resulted in the development of new therapies (Zhao et al., 2019). Oncolytic virus therapy has been a standout among emerging immunotherapies.
The novel telomerase-specific OV (OBP-301) in combination with locally focused irradiation was effective and tolerated in older ESCC patients, according to the findings of a phase I/II study (Tanabe et al., 2015). G47Δ has been identified as a highly expected innovative cancer therapeutic agent as a third-generation lysogenic HSV-1. Its α47 gene was removed when compared to G207 (prior generation HSV-1). G47Δ demonstrated a strong ability for replication and pro-apoptotic effects in each of the eight human esophageal cancer cell lines examined in vitro. This evidence unquestionably highlights the safety of G47Δ because it did not impair mouse survival whether either topically or orally for 2 months (Yajima et al., 2021). The tegument protein VP22, which acts as a heterologous protein transporter across the host cell membrane following HSV-1 infiltration of cancer cells, has been reported to improve the antitumor activity of the ESCC cell line Eca109 in vitro and in vivo by mediating the intercellular delivery of the phosphatase and tensin homolog (PTEN) protein. Surprisingly, VP22 does not inhibit tumor growth or anti-angiogenesis on its own. It must rely on intercellular delivery of PTEN, a tumor suppressor, to increase its concentration before it becomes effective. The gap in HSV treatment of EAC remains unfilled, and we anticipate that the value of HSV will be realized in this field.
4.2 Gastric cancer
Less than 25% of people with gastric cancer survive it for 5 years (Gamboa and Winer, 2019) and is prone to peritoneal metastases (Macrì and Morabito, 2019; Manzanedo et al., 2019), which can lead to peritoneal cancer at diagnosis and following surgery. Due to their link, the two have been the subject of much parallel research. The oncolytic activity of the two oncolytic HSVs, G207 and NV1020, is inconsistent since the different deletion of γ134.5 and ICP6 (ribonucleotide reductase) genes (Ma et al., 2018; Friedman et al., 2021). They quickly infect human gastric cancer cells in vitro and kill them. When given intraperitoneally at large doses, cytotoxicity was also noted in a created animal model of peritoneal disseminated gastric cancer. The outcome, however, does not bode well for intravenous administration methods (Bennett et al., 2002). This highlights the value of localized care. The human gastric cancer cell line OCUM shown sensitivity to NV1066 in both in vitro and in vivo disseminated peritoneal cancer models, with strong cytotoxicity detected. NV1066 is an attenuated HSV-1 that possesses an enhanced green fluorescent protein (EGFP) gene and can be induced to express EGFP when infecting tumor cells. Additionally, EGFP expression was seen in the lesions (Stanziale et al., 2004). This finding lends support to the use of HSV as a means of lesion localization and therapeutic agent for peritoneal or gastric cancer.
Patients with stomach cancer experience symptoms that range from mildly uncomfortable to transient dyspeptic symptoms in the early stages of the disease to severe symptoms such as malignant gastric hemorrhage, perforation, and blockage in the late stages (Wang et al., 2022a). Third-generation oncolytic HSV-1 showed great killing potential in an in vitro cell infection assay, pushing 50% of gastric cancer cells over the survival limit in a short amount of time, according to an earlier investigation of oncolytic HSV killing gastric cancer cells (Wong et al., 2010). The combination of bevacizumab (a tumor angiogenesis inhibitor) and hrR3 (an oncolytic HSV) was only effective in an in vivo gastric cancer model (Deguchi et al., 2012), and the reasons for the lack of efficacy in vitro cell infection assays are still unknown. Platelet response factor-1 (TSP-1) was armed with a third-generation oncolytic HSV-1 vector, and its active expression was increased in vivo and in vitro compared to an unarmed one by viral vector replication (Tsuji et al., 2013). Another study found that arming increased antitumor efficacy (Matsumura et al., 2021), with the difference that the third-generation oncolytic HSV-1 vector was armed with suppressor of cytokine signaling 3 (SOCS3). SOCS3 was found to be silenced in a variety of cancer cells. These positive findings show how valuable HSV is as a “fighter jet carrier” laden with “aircraft.” The third-generation oncolytic HSV-1 G47Δ, which was previously discussed, has a significant therapeutic potential for the treatment of gastric cancer. Viral replication and quick cell death were seen in every of the 9 cell lines of human gastric cancer examined. Additionally, an in vivo G47 inoculation assay revealed a substantial inhibition of advanced gastric cancer and scirrhous-type gastric cancer (Sugawara et al., 2020).
The human telomerase reverse transcriptase promoter (T-hTERT) was regulated in investigations of the fourth-generation HSV carrying the ICP6 gene, resulting in improved expression of this gene, which is crucial for viral replication, and superior tumor suppression compared to the third-generation HSV-1 (Kato et al., 2021). Based on the genetic modification of HSV-2 HG52 that enables the virus to preferentially replicate in tumors and express GM-CSF, a phase I/II study was created to examine the safety and effectiveness of OH2 either alone or in conjunction with the anti-PD-1 antibody HX008 in patients. All the patients have malignant solid tumors, such as gastrointestinal cancers. The trial is currently ongoing, and the outcomes are unknown (NCT03866525). However, we can infer from this information that more and more modified viruses are being researched, which, by releasing more antigens, strengthen the immune response against cancers.
4.3 Pancreatic cancer
A highly aggressive tumor of the digestive system, pancreatic cancer is extremely aggressive and has the potential to spread (Zhang et al., 2022b). Its metastasis is intimately linked to circulating tumor cells (CTCs) and tracking CTCs enable earlier tumor recurrence risk prediction than is possible with traditional imaging techniques. Numerous studies have demonstrated that CTC identification will aid in the monitoring of recurring metastases, assessment of the prognosis of the patient, and direction of postoperative adjuvant therapy (Hodgkinson et al., 2014).
Recent evidence suggests that HSV has a non-negligible ability to act as a diagnostic agent for cancer dissemination status. By using a cell-surface marker-free, HSV-1-based technique, escaping CTCs from lesions that are going to “seed” and “colonize” elsewhere from blood samples can be detected (Zhang et al., 2016). This is related to the HSV’s ability to replicate only in tumor cells at the source. The importance of this research lies in its capacity to render these cancerous tumor cells incapable of hiding, providing medical personnel with an invaluable opportunity to stop and cure them. Oncolytic HSV can act as a “scout” and a highly accurate diagnostic tool for the rapid identification of pancreatic cancer micrometastases (Kelly et al., 2016). It is noteworthy that HSV excels in diagnostic procedures and can enhance a patient’s therapy options for malignancies, including pancreatic cancer.
Surely, we cannot dismiss the capability of HSV itself as an OV to kill tumor cells. The good therapeutic effects of this medication have been demonstrated by pathology reports from 17 patients (8 with pancreatic cancer) in a phase I dose-escalation clinical trial (Kasuya et al., 2014) after treatment with HF10 (a mutation of HSV-1). Two human pancreatic cancer cell lines, BxPC-3 and PANC-1, have shown inconsistent cytotoxicity in response to HF10 treatment either alone or in combination with erlotinib (Yamamura et al., 2014). The former showed the strongest cytotoxicity after combination, but the latter did not exhibit this combination effect, indicating that such medicines have a certain tendency to be selective towards cancer cells.
Except for the medication combination, the combination of hyperthermia and elevation of Hsp72 expression also increased the oncolytic impact of NV1066 (Eisenberg et al., 2010), resulting in a more potent death of pancreatic cancer cells. When HSV was employed in conjunction with chemotherapy and radiotherapy to treat pancreatic cancer, we discovered that the results were reversed. When pancreatic cancer cells were exposed in vitro to the chemotherapeutic agents 5-FU, CPT-11, or MTX, they significantly inhibited HSV-1 replication and cell dissolution (Dai et al., 2010). However, ionizing radiation (IR) exhibited a synergistic oncolytic effect with HSV-1 (Kulu et al., 2013). This suggests that we should not assume that combining two or more therapies will result in stronger tumor lysis, and that more research is needed to confirm the contradiction between the two.
4.4 Liver cancer
There are two types of liver cancer: primary liver cancer and secondary liver cancer. Hepatocellular carcinoma (HCC), intrahepatic cholangiocarcinoma, and mixed carcinoma are the three types of primary liver cancer. Mixed carcinoma is defined as having elements of both hepatocellular carcinoma and cholangiocarcinoma cell carcinoma. Secondary hepatocellular carcinoma develops when tumor cells from other sites spread to the liver via blood and colonize. Liver metastasis from colorectal cancer, liver metastasis from gastric cancer, liver metastasis from lung cancer, and liver metastasis from gynecological tumors like ovarian cancer and uterine cancer are all common. These are all referred to as liver metastases. Immunotherapy has also been adopted to treat liver cancer.
RANTES, B7.1, and GM-CSF are all immunostimulatory proteins. A single agent tumor vaccine secreting GM-CS or a multi-drug combination tumor vaccine secreting RANTES/B7.1/GM-CSF can effectively inhibit cancer cells and exert excellent anti-tumor effects when produced by gene transfer using HSV as an amplicon vector. When comparing single-drug and multi-drug combination vaccinations, hepatectomy would benefit the latter (Delman et al., 2002). This information approaches the development of novel HSV-based immunotherapeutic strategies for the treatment of liver cancer. Cgal-Luc and H6-Luc, two oncolytic viruses created based on HSV-1, were genetically modified from HSV-1 17syn+ and HFEM, respectively. H6-Luc demonstrated unusual oncolytic activity of HCC cells (Argnani et al., 2011). In this research, it was also found that mice given intravenous or intrahepatic injections of both viruses experienced transient transduction, which was found using bioluminescent markers in multiple organs throughout the body. This finding may be related to the systemic immunity that oncolytic HSV exerts.
A liver cancer-specific lysing virus (LCSOV) was created to be used in HCC as a result of the miR-122 being discovered to be silenced in HCC cells. Studies showed that LCSOV is highly selective in its ability to reduce HCC xenografts and shows cytotoxicity against HCC cells (Fu et al., 2012). Another HSV-1-based oncolytic virus, Ld0-GFP, also demonstrated excellent killing capacity against HCC and may have promise for other tumor types (Luo et al., 2019). When aMPD-1 scFv is inserted into HSV-1, it becomes active for expression and allows for a continuous distribution of aMPD-1 scFv in the tumor microenvironment (TME). This improves TME, inhibits PD-1, and lowers tumor load in liver cancer models (Lin et al., 2020). The promising future of HSV as a vector for gene insertion, alteration, and expression to lyse tumor cells is still discernible from this study, in my opinion.
4.5 Gallbladder cancer and cholangiocarcinoma
Unfortunately, no current studies on HSV and gallbladder treatment are available, but studies on cholangiocarcinoma can demonstrate its potential developmental significance. Gallbladder cancer and cholangiocarcinoma are both types of biliary tract cancer; the only difference between the two is their location (Ethun et al., 2017; Banales et al., 2020). Cholangiocarcinoma is a malignant tumor that develops from the epithelial cells of the bile duct, whereas gallbladder cancer develops from the epithelial cells of the gallbladder wall. Although cholangiocarcinoma has a higher 5-year survival rate than gallbladder cancer (Banales et al., 2016; Gamboa and Maithel, 2020), both pose the same health risk.
Three human cholangiocarcinoma cell lines were employed for an experimental NV1023 mixed with radiation (XRT) treatment. NV1023 is an oncolytic HSV defective in γ134.5. The outcomes demonstrated that although NV1023 had varying sensitivity to each of the 3 cells, they all strongly destroyed tumor cells, displaying cytotoxicity, and that the addition of radiation improved this result even further (Jarnagin et al., 2006). It is thought that radiation may enhance the tumor-lytic effect of NV1023 by upregulating growth arrest and DNA damage protein 34 (GADD34) expression since GADD34 is highly similar to γ134.5 and promotes cell cycle arrest and DNA repair. However, various cell types must be separated. A new oncolytic HSV-1 known as VG161 carries genes for a number of anti-tumor immunomodulatory molecules, including IL-12, IL-15, and IL-15 receptor alpha subunit, as well as TF-Fc that prevents PD-1/PD-L1 interactions (Chouljenko et al., 2020). While maintaining patient safety, VG161 can simultaneously mediate the antitumor immune responses of several routes, exerting more cytotoxicity and better lysis. Intrahepatic cholangiocarcinoma (ICC) patient-derived xenograft (PDX) animals appear to exhibit improved antitumor activity in response to both HSV-1 preimmunization and multi-cycle VG161 therapy (Ding et al., 2022). This may provide support for a long-term VG161 treatment plan for individuals in clinical trials who are HSV-1 seropositive. It is suggested that more alluring HSVs be created and employed for the diagnosis or treatment of gallbladder cancer as well as other malignancies.
4.6 Colorectal cancer
According to the International Agency for Research on Cancer, colorectal cancer has become the third most common malignancy in 185 countries worldwide (Sung et al., 2021), and OV therapy research has become increasingly diverse, with the goal of benefiting patients.
HSV oncolytic therapy for colorectal cancer has been studied since before the turn of the century. The hrR3 vector derived from HSV-1, for example, has been shown to be effective in killing colon cancer cells both in vivo and in vitro (Yoon et al., 1998). Notably, hrR3 contains the herpes simplex virus thymidine kinase (HSV-TK) suicide gene, an HSV-1 product that converts GCV into the toxic metabolite triphosphate-GCV (GCV-TP) and increases cytotoxicity (Yi et al., 2018). As a result, using this approach in the field of gene-directed enzyme pre-drug therapy (GDEPT) for the treatment of colorectal cancer and other digestive system tumors is feasible (Zhou et al., 2016; Luo et al., 2018; Sawdon et al., 2021; Zhou et al., 2022). The HSV-TK gene must first be delivered to the site of the lesion, followed by the administration of GCV as a prodrug to malignant cells to generate the toxic activation response described above, which will rapidly kill tumor cells. This two-step approach was replaced by a one-step approach employing polymeric micellar nanocarriers that bind the gene and the prodrug simultaneously for simple and efficient delivery of the suicide gene and the prodrug, resulting in high toxicity in colorectal cancer HT-29 cells in vitro (Sawdon et al., 2021).
In a phase I clinical trial in 2009, groups of 12 patients with intrahepatic metastatic colorectal cancer received varying doses of NV1020 by hepatic arterial injection, which proved to be a reliable method of vascular delivery of HSV-1. With decreased levels of the tumor marker carcinoembryonic antigen (CEA), the phase I trial opened a new pathway for the administration of oncolytic drugs. One patient in the group that received the most injections of tumor lysate experienced a 25% decrease in tumor volume (Fong et al., 2009). Following targeted treatment, CEA levels continued to drop (Fong et al., 2009). However, a prior study found a small number of side effects in participants and the discovery of viruses in serum and saliva samples following NV1020 dosing (Kemeny et al., 2006). Despite the fact that this study’s findings indicated NV1020 can enter the hepatic artery safely, it is important to continue to monitor the situation and to undertake follow-up phase II and III clinical trials.
Not only that, but as we enter the twenty-first century, we see that combination therapy with HSV modification studies is becoming more common and popular, increasing the likelihood of multiple mechanisms of enhanced tumor lysis efficacy. In mouse lateral abdominal tumor models and in vitro colorectal cancer cells, loss of viral ribonucleotide reductase (RR) of HSV-1 mutant G207 in combination with IR resulted in significant tumor lysis enhancement, but no such synergistic effect was observed with combination therapy of parental virus with IR (Stanziale et al., 2002). This undoubtedly demonstrates that IR’s upregulation of RR activity function is the driving force behind this additional oncolytic effect. HSV1716Ing4, a novel variant of HSV1716 found to express growth inhibitor 4 (Ing4), had a oncolytic effect 1000-fold greater than HSV1716 in the study (Conner and Braidwood, 2012). With progeny output, Ing4 may contribute to HSV1716Ing4 infection of colorectal cancer cells. Its safety has also been established, with no replication found in any organ other than tumor tissue. Canerpaturev (C-REV) is the predecessor of HF10, and the combination of C-REV with cetuximab resulted in enhanced antitumor activity, allowing all three human colorectal cancer cell lines expressing different levels of epidermal growth factor receptor (EGFR) to exhibit a corresponding state of decay with the dose and time of C-REV administration. HSV-1 in combination with the chemotherapeutic agent bortezomib improves oncolytic efficiency in colorectal cancer cells, which was demonstrated by upregulating heat shock protein expression while dramatically inducing endoplasmic reticulum stress and activating the cystein-12 apoptotic pathway (El-Sayes et al., 2022). Furthermore, when combined with chemotherapeutic agents such as mitomycin C (mito), immune checkpoint therapy improves in colorectal cancer patients (El-Sayes et al., 2022).
It is obvious that HSV has the ability to treat cancers not only through its oncolytic action but also by opening up a lot of opportunities for the creation of additional treatment modalities. This modified oncolytic HSV can express high levels of IL-12 in infected cells, promote T-cell proliferation, and strongly express IFN-γ, playing a critical role in lysing cancer cells after interleukin 12 (IL-12) and C-X-C motif chemokine ligand 11 (CXCL11) are substituted for the HSV-1 ICP47 and ICP34.5 coding regions, respectively (Zhang et al., 2022a). The findings of a comparable study lend credence to this notion (Haghighi-Najafabadi et al., 2021). Hypoxia is a constant in the TME, which is attributed to the inefficient tumor vascular system’s poor oxygenation (Jing et al., 2019). The cytotoxicity of an HSV-1 (HSV-HMGB1) expressing the HMGB1 protein, which inhibits tumor cell aerobic respiration, was tested in both normoxic and hypoxic conditions against three colorectal cancer cell lines (HCT116, SW480, and HT29) and compared to the parental virus HSV-ble (Shayan et al., 2022). In both states, HSV- HMGB1 was significantly more virulent than its parental virus for HCT116 and SW480. However, under hypoxia, HT29 showed value-added promotion, indicating that HSV-HMGB1 is not applicable to all types of colorectal cancer. In the future, more broad-spectrum and effective HSVs for improving TME should be investigated.
5 Discussion and challenge
Oncolytic viruses are thought to be promising and play a significant part in innovative immunotherapies for the treatment of malignancies. A standout performance among the various new oncolytic viruses is oncolytic HSV. In recent decades, there has been uninterrupted research on oncolytic HSV. Its genomic advantage provides a platform for the insertion of many genes with anti-cancer potential. From this point of view alone, oncolytic HSV is bound to gain more momentum in the future as research continues to advance. In addition, pharmacological immunotherapies are not abandoned. We note that the use of immunotherapeutic agents in combination with oncolytic HSV is supported by wide-ranging clinical data (Bommareddy et al., 2018). The Japanese Ministry of Health approved G47Δ as a treatment for malignant glioma in 2021, in addition to the previously mentioned T-VEC approved by the FDA (Otani et al., 2022). To date, only a limited number of OV drugs have been approved, obviously due to thoughtful safety concerns. Not only the complex mechanism of action between OV and cancer cells matters, but also the mode of OV delivery needs to be carefully considered. Intratumoral injection of oncolytic HSV is probably the most efficient delivery method available (Jahan et al., 2021). We believe that more recent iterations of HSV-based lysing viruses will be used more frequently for the clinical treatment of tumors in the future, even though many of these viruses alter the editable region of HSV genes and are currently in clinical trials or are only in the experimental validation stage (Patel et al., 2016; Suzuki et al., 2021; Wang et al., 2022b; Liu et al., 2022; Tian et al., 2022).
For the treatment of digestive system malignancies, oncolytic HSV-1 is frequently mentioned and exhibits excellent therapeutic potential in a range of digestive system tumors (Hu et al., 2022; Tang et al., 2022). Oncolytic HSV-2, which mostly treats cancers of the non-digestive system, appears to have notably scant data within the same group. The vast majority of lysogenic HSVs are currently derived from lysogenic HSV-1, but one piece of evidence suggests that FusOn-H2 from HSV-2 has a far superior antitumor effect on a subpopulation of cancer cells than Baco-1 from HSV-1 (Fu et al., 2018). This is a source of concern. On the one hand, research on oncolytic viruses with HSV-2 origins is few, making it difficult to assess their benefits and drawbacks; on the other hand, it is possible that we overlooked viral vectors with superior effects.
Additionally, the percentage of HSV studies filled by various tumor forms falling under the general category of digestive system cancers varies greatly. There are not many examples, for instance, of oncolytic virus HSV for gallbladder cancer. This is where we would want to suggest the direction for future investigation because we found it troubling in our collection of reports. Many proactive attempts should be pursued in subsequent studies for the treatment of cancers such as gallbladder cancer, including in vivo and in vitro trials. However, we know that a large part of the reason that oncolytic HSV is effective in treating tumors lies in the ignition of a fire of systemic immune processes. The efficacy of the treatment is closely related to the degree of this burst of immunity. Therefore, we would like to emphasize here that the patient remains in a cheerful mood during the treatment, which can positively influence the immune inflammatory response in the body (Avvenuti et al., 2016) and contribute to the treatment of oncolytic HSV.
Undoubtedly, oncolytic HSV’s anticancer properties are valued. On the other hand, the concept of using HSV as a diagnostic tool based on its preferential replication in cancer cells is also welcomed (Kelly et al., 2016; Zhang et al., 2016). This is due to the fact that it is crucial for detecting the metastasis and spread of many malignancies, may be successful in preventing many secondary cancers, and has broad repercussions for disruption and therapy in the future. Therefore, further research must be done after the discovery of its diagnostic significance. Last but not least, additional clinical trials and analysis are required to confirm the effectiveness and safety of various oncolytic HSVs as tumor lysis and diagnostic agents using data from phase I, II, and III clinical trials. These studies will open up fresh possibilities for effective DST immunotherapy.
Author contributions
SL and QL: writing and editing. YR and JY: reviewing and visualization. JG: validation. XK: supervision. All authors contributed to the article and approved the submitted version.
Funding
This study was supported by the scientific research project of Tianjin Education Commission (2021KJ134).
Acknowledgments
The figures were drawn by Figdraw (www.figdraw.com).
Conflict of interest
The authors declare that the research was conducted in the absence of any commercial or financial relationships that could be construed as a potential conflict of interest.
Publisher’s note
All claims expressed in this article are solely those of the authors and do not necessarily represent those of their affiliated organizations, or those of the publisher, the editors and the reviewers. Any product that may be evaluated in this article, or claim that may be made by its manufacturer, is not guaranteed or endorsed by the publisher.
Supplementary material
The Supplementary Material for this article can be found online at: https://www.frontiersin.org/articles/10.3389/fmolb.2023.1142498/full#supplementary-material
References
Ajani, J. A., D'amico, T. A., Bentrem, D. J., Chao, J., Corvera, C., Das, P., et al. (2019). Esophageal and esophagogastric junction cancers, version 2.2019, NCCN clinical practice guidelines in Oncology. J. Natl. Compr. Canc Netw. 17, 855–883. doi:10.6004/jnccn.2019.0033
Andtbacka, R. H., Kaufman, H. L., Collichio, F., Amatruda, T., Senzer, N., Chesney, J., et al. (2015). Talimogene laherparepvec improves durable response rate in patients with advanced melanoma. J. Clin. Oncol. 33, 2780–2788. doi:10.1200/jco.2014.58.3377
Argnani, R., Marconi, P., Volpi, I., Bolanos, E., Carro, E., Ried, C., et al. (2011). Characterization of herpes simplex virus 1 strains as platforms for the development of oncolytic viruses against liver cancer. Liver Int. 31, 1542–1553. doi:10.1111/j.1478-3231.2011.02628.x
Avvenuti, G., Baiardini, I., and Giardini, A. (2016). Optimism's explicative role for chronic diseases. Front. Psychol. 7, 295. doi:10.3389/fpsyg.2016.00295
Banales, J. M., Cardinale, V., Carpino, G., Marzioni, M., Andersen, J. B., Invernizzi, P., et al. (2016). Expert consensus document: Cholangiocarcinoma: Current knowledge and future perspectives consensus statement from the European network for the study of cholangiocarcinoma (ENS-CCA). Nat. Rev. Gastroenterol. Hepatol. 13, 261–280. doi:10.1038/nrgastro.2016.51
Banales, J. M., Marin, J. J. G., Lamarca, A., Rodrigues, P. M., Khan, S. A., Roberts, L. R., et al. (2020). Cholangiocarcinoma 2020: The next horizon in mechanisms and management. Nat. Rev. Gastroenterol. Hepatol. 17, 557–588. doi:10.1038/s41575-020-0310-z
Bansode, Y. D., Chattopadhyay, D., and Saha, B. (2021). Transcriptomic analysis of interferon response in toll-like receptor 2 ligand-treated and herpes simplex virus 1-infected neurons and astrocytes. Viral Immunol. 34, 256–266. doi:10.1089/vim.2020.0238
Belli, C., Trapani, D., Viale, G., D'amico, P., Duso, B. A., Della Vigna, P., et al. (2018). Targeting the microenvironment in solid tumors. Cancer Treat. Rev. 65, 22–32. doi:10.1016/j.ctrv.2018.02.004
Bennett, J. J., Delman, K. A., Burt, B. M., Mariotti, A., Malhotra, S., Zager, J., et al. (2002). Comparison of safety, delivery, and efficacy of two oncolytic herpes viruses (G207 and NV1020) for peritoneal cancer. Cancer Gene Ther. 9, 935–945. doi:10.1038/sj.cgt.7700510
Bommareddy, P. K., Patel, A., Hossain, S., and Kaufman, H. L. (2017). Talimogene laherparepvec (T-VEC) and other oncolytic viruses for the treatment of melanoma. Am. J. Clin. Dermatol 18, 1–15. doi:10.1007/s40257-016-0238-9
Bommareddy, P. K., Shettigar, M., and Kaufman, H. L. (2018). Integrating oncolytic viruses in combination cancer immunotherapy. Nat. Rev. Immunol. 18, 498–513. doi:10.1038/s41577-018-0014-6
Bozhanova, G., Hassan, J., Appleton, L., Jennings, V., Foo, S., Mclaughlin, M., et al. (2022). CD4 T cell dynamics shape the immune response to combination oncolytic herpes virus and BRAF inhibitor therapy for melanoma. J. Immunother. Cancer 10, e004410. doi:10.1136/jitc-2021-004410
Bray, F., Ferlay, J., Soerjomataram, I., Siegel, R. L., Torre, L. A., and Jemal, A. (2018). Global cancer statistics 2018: GLOBOCAN estimates of incidence and mortality worldwide for 36 cancers in 185 countries. CA Cancer J. Clin. 68, 394–424. doi:10.3322/caac.21492
Chen, X., Lan, H., He, D., Wang, Z., Xu, R., Yuan, J., et al. (2021). Analysis of autophagy-related signatures identified two distinct subtypes for evaluating the tumor immune microenvironment and predicting prognosis in ovarian cancer. Front. Oncol. 11, 616133. doi:10.3389/fonc.2021.616133
Chouljenko, D. V., Ding, J., Lee, I. F., Murad, Y. M., Bu, X., Liu, G., et al. (2020). Induction of durable antitumor response by a novel oncolytic herpesvirus expressing multiple immunomodulatory transgenes. Biomedicines 8, 484. doi:10.3390/biomedicines8110484
Conner, J., and Braidwood, L. (2012). Expression of inhibitor of growth 4 by HSV1716 improves oncolytic potency and enhances efficacy. Cancer Gene Ther. 19, 499–507. doi:10.1038/cgt.2012.24
Cui, Y., Zhang, L., Hu, D., and Yang, Y. (2022). Berberine inhibits herpes simplex virus 1 replication in HEK293T cells. Comput. Math. Methods Med. 2022, 7137401. doi:10.1155/2022/7137401
Dai, M. H., Zamarin, D., Gao, S. P., Chou, T. C., Gonzalez, L., Lin, S. F., et al. (2010). Synergistic action of oncolytic herpes simplex virus and radiotherapy in pancreatic cancer cell lines. Br. J. Surg. 97, 1385–1394. doi:10.1002/bjs.7124
Deguchi, T., Shikano, T., Kasuya, H., Nawa, A., Fujiwara, S., Takeda, S., et al. (2012). Combination of the tumor angiogenesis inhibitor bevacizumab and intratumoral oncolytic herpes virus injections as a treatment strategy for human gastric cancers. Hepatogastroenterology 59, 1844–1850. doi:10.5754/hge11566
Delman, K. A., Zager, J. S., Bennett, J. J., Malhotra, S., Ebright, M. I., Mcauliffe, P. F., et al. (2002). Efficacy of multiagent herpes simplex virus amplicon-mediated immunotherapy as adjuvant treatment for experimental hepatic cancer. Ann. Surg. 236, 337. doi:10.1097/00000658-200209000-00010
Ding, J., Murad, Y. M., Sun, Y., Lee, I. F., Samudio, I., Liu, X., et al. (2022). Pre-existing HSV-1 immunity enhances anticancer efficacy of a novel immune-stimulating oncolytic virus. Viruses 14, 2327. doi:10.3390/v14112327
Dutta, D., Dutta, S., Veettil, M. V., Roy, A., Ansari, M. A., Iqbal, J., et al. (2015). BRCA1 regulates IFI16 mediated nuclear innate sensing of herpes viral DNA and subsequent induction of the innate inflammasome and interferon-β responses. PLoS Pathog. 11, e1005030. doi:10.1371/journal.ppat.1005030
Eisenberg, D. P., Carpenter, S. G., Adusumilli, P. S., Chan, M. K., Hendershott, K. J., Yu, Z., et al. (2010). Hyperthermia potentiates oncolytic herpes viral killing of pancreatic cancer through a heat shock protein pathway. Surgery 148, 325–334. doi:10.1016/j.surg.2010.05.005
El-Sayes, N., Vito, A., Salem, O., Workenhe, S. T., Wan, Y., and Mossman, K. (2022). A combination of chemotherapy and oncolytic virotherapy sensitizes colorectal adenocarcinoma to immune checkpoint inhibitors in a cDC1-dependent manner. Int. J. Mol. Sci. 23, 1754. doi:10.3390/ijms23031754
Ethun, C. G., Le, N., Lopez-Aguiar, A. G., Pawlik, T. M., Poultsides, G., Tran, T., et al. (2017). Pathologic and prognostic implications of incidental versus nonincidental gallbladder cancer: A 10-institution study from the United States extrahepatic biliary malignancy consortium. Am. Surg. 83, 679–686. doi:10.1177/000313481708300721
Fong, Y., Kim, T., Bhargava, A., Schwartz, L., Brown, K., Brody, L., et al. (2009). A herpes oncolytic virus can be delivered via the vasculature to produce biologic changes in human colorectal cancer. Mol. Ther. 17, 389–394. doi:10.1038/mt.2008.240
Friedman, G. K., Johnston, J. M., Bag, A. K., Bernstock, J. D., Li, R., Aban, I., et al. (2021). Oncolytic HSV-1 G207 immunovirotherapy for pediatric high-grade gliomas. N. Engl. J. Med. 384, 1613–1622. doi:10.1056/NEJMoa2024947
Fu, X., Rivera, A., Tao, L., De Geest, B., and Zhang, X. (2012). Construction of an oncolytic herpes simplex virus that precisely targets hepatocellular carcinoma cells. Mol. Ther. 20, 339–346. doi:10.1038/mt.2011.265
Fu, X., Tao, L., Wang, P. Y., Cripe, T. P., and Zhang, X. (2018). Comparison of infectivity and spread between HSV-1 and HSV-2 based oncolytic viruses on tumor cells with different receptor expression profiles. Oncotarget 9, 21348–21358. doi:10.18632/oncotarget.25096
Fundora Ramos, M. I., Maden, L. B., Casanova, F. O., Cruz, F. H., Reyes, C. S., Gato, A. H., et al. (2021). Oncoxin-Viusid(®) may improve quality of life and survival in patients with hormone-refractory prostate cancer undergoing onco-specific treatments. Mol. Clin. Oncol. 14, 5. doi:10.3892/mco.2020.2167
Galon, J., and Bruni, D. (2019). Approaches to treat immune hot, altered and cold tumours with combination immunotherapies. Nat. Rev. Drug Discov. 18, 197–218. doi:10.1038/s41573-018-0007-y
Gamboa, A. C., and Maithel, S. K. (2020). The landmark series: Gallbladder cancer. Ann. Surg. Oncol. 27, 2846–2858. doi:10.1245/s10434-020-08654-9
Gamboa, A. C., and Winer, J. H. (2019). Cytoreductive surgery and hyperthermic intraperitoneal chemotherapy for gastric cancer. Cancers (Basel) 11, 1662. doi:10.3390/cancers11111662
Gong, L., Ou, X., Hu, L., Zhong, J., Li, J., Deng, S., et al. (2022). The molecular mechanism of herpes simplex virus 1 UL31 in antagonizing the activity of IFN-β. Microbiol. Spectr. 10, e0188321. doi:10.1128/spectrum.01883-21
Gujar, S., Pol, J. G., and Kroemer, G. (2018). Heating it up: Oncolytic viruses make tumors 'hot' and suitable for checkpoint blockade immunotherapies. Oncoimmunology 7, e1442169. doi:10.1080/2162402x.2018.1442169
Guo, Z. S., Liu, Z., and Bartlett, D. L. (2014). Oncolytic immunotherapy: Dying the right way is a key to eliciting potent antitumor immunity. Front. Oncol. 4, 74. doi:10.3389/fonc.2014.00074
Haghighi-Najafabadi, N., Roohvand, F., Shams Nosrati, M. S., Teimoori-Toolabi, L., and Azadmanesh, K. (2021). Oncolytic herpes simplex virus type-1 expressing IL-12 efficiently replicates and kills human colorectal cancer cells. Microb. Pathog. 160, 105164. doi:10.1016/j.micpath.2021.105164
Hanahan, D., and Coussens, L. M. (2012). Accessories to the crime: Functions of cells recruited to the tumor microenvironment. Cancer Cell 21, 309–322. doi:10.1016/j.ccr.2012.02.022
Hanahan, D., and Weinberg, R. A. (2011). Hallmarks of cancer: The next generation. Cell 144, 646–674. doi:10.1016/j.cell.2011.02.013
Hartkopf, A. D., Fehm, T., Wallwiener, D., and Lauer, U. (2011). Oncolytic virotherapy of gynecologic malignancies. Gynecol. Oncol. 120, 302–310. doi:10.1016/j.ygyno.2010.10.031
Heidbuechel, J. P. W., and Engeland, C. E. (2021). Oncolytic viruses encoding bispecific T cell engagers: A blueprint for emerging immunovirotherapies. J. Hematol. Oncol. 14, 63. doi:10.1186/s13045-021-01075-5
Hodgkinson, C. L., Morrow, C. J., Li, Y., Metcalf, R. L., Rothwell, D. G., Trapani, F., et al. (2014). Tumorigenicity and genetic profiling of circulating tumor cells in small-cell lung cancer. Nat. Med. 20, 897–903. doi:10.1038/nm.3600
Hori, S., Nomura, T., and Sakaguchi, S. (2003). Control of regulatory T cell development by the transcription factor Foxp3. Science 299, 1057–1061. doi:10.1126/science.1079490
Hu, H., Zhang, S., Cai, L., Duan, H., Li, Y., Yang, J., et al. (2022). A novel cocktail therapy based on quintuplet combination of oncolytic herpes simplex virus-2 vectors armed with interleukin-12, interleukin-15, GM-CSF, PD1v, and IL-7 × CCL19 results in enhanced antitumor efficacy. Virol. J. 19, 74. doi:10.1186/s12985-022-01795-1
Huang, Q., Cai, W. Q., Han, Z. W., Wang, M. Y., Zhou, Y., Cheng, J. T., et al. (2021). Bispecific T cell engagers and their synergistic tumor immunotherapy with oncolytic viruses. Am. J. Cancer Res. 11, 2430–2455.
Ilkow, C. S., Swift, S. L., Bell, J. C., and Diallo, J. S. (2014). From scourge to cure: Tumour-selective viral pathogenesis as a new strategy against cancer. PLoS Pathog. 10, e1003836. doi:10.1371/journal.ppat.1003836
Jahan, N., Ghouse, S. M., Martuza, R. L., and Rabkin, S. D. (2021). Situ cancer vaccination and immunovirotherapy using oncolytic HSV. Viruses 13, 1740. doi:10.3390/v13091740
Jarnagin, W. R., Zager, J. S., Hezel, M., Stanziale, S. F., Adusumilli, P. S., Gonen, M., et al. (2006). Treatment of cholangiocarcinoma with oncolytic herpes simplex virus combined with external beam radiation therapy. Cancer Gene Ther. 13, 326–334. doi:10.1038/sj.cgt.7700890
Jing, X., Yang, F., Shao, C., Wei, K., Xie, M., Shen, H., et al. (2019). Role of hypoxia in cancer therapy by regulating the tumor microenvironment. Mol. Cancer 18, 157. doi:10.1186/s12943-019-1089-9
Kasuya, H., Kodera, Y., Nakao, A., Yamamura, K., Gewen, T., Zhiwen, W., et al. (2014). Phase I dose-escalation clinical trial of HF10 oncolytic herpes virus in 17 Japanese patients with advanced cancer. Hepatogastroenterology 61, 599–605.
Kato, T., Nakamori, M., Matsumura, S., Nakamura, M., Ojima, T., Fukuhara, H., et al. (2021). Oncolytic virotherapy with human telomerase reverse transcriptase promoter regulation enhances cytotoxic effects against gastric cancer. Oncol. Lett. 21, 490. doi:10.3892/ol.2021.12751
Kaufman, H. L., Kim, D. W., Deraffele, G., Mitcham, J., Coffin, R. S., and Kim-Schulze, S. (2010). Local and distant immunity induced by intralesional vaccination with an oncolytic herpes virus encoding GM-CSF in patients with stage IIIc and IV melanoma. Ann. Surg. Oncol. 17, 718–730. doi:10.1245/s10434-009-0809-6
Kaufman, H. L., Kohlhapp, F. J., and Zloza, A. (2015). Oncolytic viruses: A new class of immunotherapy drugs. Nat. Rev. Drug Discov. 14, 642–662. doi:10.1038/nrd4663
Kelly, K. J., Wong, J., Gönen, M., Allen, P., Brennan, M., Coit, D., et al. (2016). Human trial of a genetically modified herpes simplex virus for rapid detection of positive peritoneal cytology in the staging of pancreatic cancer. EBioMedicine 7, 94–99. doi:10.1016/j.ebiom.2016.03.043
Kemeny, N., Brown, K., Covey, A., Kim, T., Bhargava, A., Brody, L., et al. (2006). Phase I, open-label, dose-escalating study of a genetically engineered herpes simplex virus, NV1020, in subjects with metastatic colorectal carcinoma to the liver. Hum. Gene Ther. 17, 1214–1224. doi:10.1089/hum.2006.17.1214
Knipe, D., Howley, P., Griffin, D., Lamb, R., Martin, M., Roizman, B., et al. (2013). Fields Virology. Philadelphia, PA Lippincott Williams & Willkins:
Koch, M. S., Lawler, S. E., and Chiocca, E. A. (2020). HSV-1 oncolytic viruses from bench to bedside: An overview of current clinical trials. Cancers (Basel) 12, 3514. doi:10.3390/cancers12123514
Kroemer, G., Galluzzi, L., Kepp, O., and Zitvogel, L. (2013). Immunogenic cell death in cancer therapy. Annu. Rev. Immunol. 31, 51–72. doi:10.1146/annurev-immunol-032712-100008
Kulu, Y., Kawasaki, H., Donahue, J. M., Kasuya, H., Cusack, J. C., Choi, E. W., et al. (2013). Concurrent chemotherapy inhibits herpes simplex virus-1 replication and oncolysis. Cancer Gene Ther. 20, 133–140. doi:10.1038/cgt.2012.97
Li, C., Zhou, W., Zhu, J., Shen, Q., Wang, G., Chen, L., et al. (2022a). Identification of an immune-related gene signature associated with prognosis and tumor microenvironment in esophageal cancer. Biomed. Res. Int. 2022, 7413535. doi:10.1155/2022/7413535
Li, J., Meng, Q., Zhou, X., Zhao, H., Wang, K., Niu, H., et al. (2022b). Gospel of malignant Glioma: Oncolytic virus therapy. Gene 818, 146217. doi:10.1016/j.gene.2022.146217
Li, K., Zhao, Y., Hu, X., Jiao, J., Wang, W., and Yao, H. (2022c). Advances in the clinical development of oncolytic viruses. Am. J. Transl. Res. 14, 4192–4206.
Li, Q., Oduro, P. K., Guo, R., Li, R., Leng, L., Kong, X., et al. (2022d). Oncolytic viruses: Immunotherapy drugs for gastrointestinal malignant tumors. Front. Cell Infect. Microbiol. 12, 921534. doi:10.3389/fcimb.2022.921534
Li, Q., Tan, F., Wang, Y., Liu, X., Kong, X., Meng, J., et al. (2022e). The gamble between oncolytic virus therapy and IFN. Front. Immunol. 13, 971674. doi:10.3389/fimmu.2022.971674
Li, S. J., and Sun, Z. J. (2022). Fueling immune checkpoint blockade with oncolytic viruses: Current paradigms and challenges ahead. Cancer Lett. 550, 215937. doi:10.1016/j.canlet.2022.215937
Lichty, B. D., Breitbach, C. J., Stojdl, D. F., and Bell, J. C. (2014). Going viral with cancer immunotherapy. Nat. Rev. Cancer 14, 559–567. doi:10.1038/nrc3770
Lin, C., Ren, W., Luo, Y., Li, S., Chang, Y., Li, L., et al. (2020). Intratumoral delivery of a PD-1-blocking scFv encoded in oncolytic HSV-1 promotes antitumor immunity and synergizes with TIGIT blockade. Cancer Immunol. Res. 8, 632–647. doi:10.1158/2326-6066.Cir-19-0628
Lin, Y., and Zheng, C. (2019). A tug of war: DNA-sensing antiviral innate immunity and herpes simplex virus type I infection. Front. Microbiol. 10, 2627. doi:10.3389/fmicb.2019.02627
Liu, Y., Zheng, Y., Deng, T., Huang, Y., Liu, Z., Zhan, B., et al. (2022). Oncolytic herpes simplex virus delivery of dual CAR targets of CD19 and BCMA as well as immunomodulators to enhance therapeutic efficacy in solid tumors combined with CAR T cell therapy. Front. Oncol. 12, 1037934. doi:10.3389/fonc.2022.1037934
Lu, S. Y., Hua, J., Xu, J., Wei, M. Y., Liang, C., Meng, Q. C., et al. (2021). Microorganisms in chemotherapy for pancreatic cancer: An overview of current research and future directions. Int. J. Biol. Sci. 17, 2666–2682. doi:10.7150/ijbs.59117
Luo, J., Zhou, J., Xie, F., Zhu, Y., Zhou, F., Zhang, S., et al. (2018). Combined treatment of cholangiocarcinoma with interventional radiofrequency hyperthermia and heat shock protein promoter-mediated HSV-TK gene therapy. Am. J. Cancer Res. 8, 1595–1603.
Luo, Y., Lin, C., Ren, W., Ju, F., Xu, Z., Liu, H., et al. (2019). Intravenous injections of a rationally selected oncolytic herpes virus as a potent virotherapy for hepatocellular carcinoma. Mol. Ther. Oncolytics 15, 153–165. doi:10.1016/j.omto.2019.09.004
Ma, W., He, H., and Wang, H. (2018). Oncolytic herpes simplex virus and immunotherapy. BMC Immunol. 19, 40. doi:10.1186/s12865-018-0281-9
Macrì, A., and Morabito, F. (2019). The use of intraperitoneal chemotherapy for gastric malignancies. Expert Rev. Anticancer Ther. 19, 879–888. doi:10.1080/14737140.2019.1671189
Manzanedo, I., Pereira, F., Rihuete Caro, C., Pérez-Viejo, E., Serrano, Á., Gutiérrez Calvo, A., et al. (2019). Cytoreductive surgery and hyperthermic intraperitoneal chemotherapy (HIPEC) for gastric cancer with peritoneal carcinomatosis: Multicenter study of Spanish group of peritoneal oncologic surgery (GECOP). Ann. Surg. Oncol. 26, 2615–2621. doi:10.1245/s10434-019-07450-4
Martuza, R. L., Malick, A., Markert, J. M., Ruffner, K. L., and Coen, D. M. (1991). Experimental therapy of human glioma by means of a genetically engineered virus mutant. Science 252, 854–856. doi:10.1126/science.1851332
Matsumura, S., Nakamori, M., Tsuji, T., Kato, T., Nakamura, M., Ojima, T., et al. (2021). Oncolytic virotherapy with SOCS3 enhances viral replicative potency and oncolysis for gastric cancer. Oncotarget 12, 344–354. doi:10.18632/oncotarget.27873
Mcallister, F., Khan, M. A. W., Helmink, B., and Wargo, J. A. (2019). The tumor microbiome in pancreatic cancer: Bacteria and beyond. Cancer Cell 36, 577–579. doi:10.1016/j.ccell.2019.11.004
Melcher, A., Harrington, K., and Vile, R. (2021). Oncolytic virotherapy as immunotherapy. Science 374, 1325–1326. doi:10.1126/science.abk3436
Moesta, A. K., Cooke, K., Piasecki, J., Mitchell, P., Rottman, J. B., Fitzgerald, K., et al. (2017). Local delivery of OncoVEX(mGM-CSF) generates systemic antitumor immune responses enhanced by cytotoxic T-lymphocyte-associated protein blockade. Clin. Cancer Res. 23, 6190–6202. doi:10.1158/1078-0432.Ccr-17-0681
Mohan, S., Elhassan Taha, M. M., Makeen, H. A., Alhazmi, H. A., Al Bratty, M., Sultana, S., et al. (2020). Bioactive natural antivirals: An updated review of the available plants and isolated molecules. Molecules 25, 4878. doi:10.3390/molecules25214878
Moore, A. E. (1949b). Effect of inoculation of the viruses of influenza A and herpes simplex on the growth of transplantable tumors in mice. Cancer 2, 516–524. doi:10.1002/1097-0142(194905)2:3<516::aid-cncr2820020316>3.0.co;2-p
Moore, A. E. (1949a). The destructive effect of the virus of Russian Far East encephalitis on the transplantable mouse sarcoma 180. Cancer 2, 525–534. doi:10.1002/1097-0142(194905)2:3<525::aid-cncr2820020317>3.0.co;2-o
Nemunaitis, J. J. (1999). Cancer treatment involving oncolytic viruses. Clin. Lung Cancer 1, 50. doi:10.3816/clc.1999.n.003
Newman, J. H., Chesson, C. B., Herzog, N. L., Bommareddy, P. K., Aspromonte, S. M., Pepe, R., et al. (2020). Intratumoral injection of the seasonal flu shot converts immunologically cold tumors to hot and serves as an immunotherapy for cancer. Proc. Natl. Acad. Sci. U. S. A. 117, 1119–1128. doi:10.1073/pnas.1904022116
Oosenbrug, T., Van De Graaff, M. J., Ressing, M. E., and Van Kasteren, S. I. (2017). Chemical tools for studying TLR signaling dynamics. Cell Chem. Biol. 24, 801–812. doi:10.1016/j.chembiol.2017.05.022
Osali, A., Zhiani, M., Ghaebi, M., Meymanat, M., and Esmaeilzadeh, A. (2020). Multidirectional strategies for targeted delivery of oncolytic viruses by tumor infiltrating immune cells. Pharmacol. Res. 161, 105094. doi:10.1016/j.phrs.2020.105094
Otani, Y., Yoo, J. Y., Shimizu, T., Kurozumi, K., Date, I., and Kaur, B. (2022). Implications of immune cells in oncolytic herpes simplex virotherapy for glioma. Brain Tumor Pathol. 39, 57–64. doi:10.1007/s10014-022-00431-8
Patel, D. M., Foreman, P. M., Nabors, L. B., Riley, K. O., Gillespie, G. Y., and Markert, J. M. (2016). Design of a phase I clinical trial to evaluate M032, a genetically engineered HSV-1 expressing IL-12, in patients with recurrent/progressive glioblastoma multiforme, anaplastic astrocytoma, or gliosarcoma. Hum. Gene Ther. Clin. Dev. 27, 69–78. doi:10.1089/humc.2016.031
Pol, J., Kroemer, G., and Galluzzi, L. (2016). First oncolytic virus approved for melanoma immunotherapy. Oncoimmunology 5, e1115641. doi:10.1080/2162402x.2015.1115641
Rech, A. J., Mick, R., Martin, S., Recio, A., Aqui, N. A., Powell, D. J., et al. (2012). CD25 blockade depletes and selectively reprograms regulatory T cells in concert with immunotherapy in cancer patients. Sci. Transl. Med. 4, 134ra62. doi:10.1126/scitranslmed.3003330
Ribas, A., Dummer, R., Puzanov, I., Vanderwalde, A., Andtbacka, R. H. I., Michielin, O., et al. (2017). Oncolytic virotherapy promotes intratumoral T cell infiltration and improves anti-PD-1 immunotherapy. Cell 170, 1109–1119.e10. doi:10.1016/j.cell.2017.08.027
Rice, S. A., Long, M. C., Lam, V., Schaffer, P. A., and Spencer, C. A. (1995). Herpes simplex virus immediate-early protein ICP22 is required for viral modification of host RNA polymerase II and establishment of the normal viral transcription program. J. Virol. 69, 5550–5559. doi:10.1128/jvi.69.9.5550-5559.1995
Ring, E. K., Li, R., Moore, B. P., Nan, L., Kelly, V. M., Han, X., et al. (2017). Newly characterized murine undifferentiated sarcoma models sensitive to virotherapy with oncolytic HSV-1 M002. Mol. Ther. Oncolytics 7, 27–36. doi:10.1016/j.omto.2017.09.003
Russell, S. J., Peng, K. W., and Bell, J. C. (2012). Oncolytic virotherapy. Nat. Biotechnol. 30, 658–670. doi:10.1038/nbt.2287
Samson, A., Scott, K. J., Taggart, D., West, E. J., Wilson, E., Nuovo, G. J., et al. (2018). Intravenous delivery of oncolytic reovirus to brain tumor patients immunologically primes for subsequent checkpoint blockade. Sci. Transl. Med. 10, eaam7577. doi:10.1126/scitranslmed.aam7577
Sawdon, A. J., Zhang, J., Peng, S., Alyami, E. M., and Peng, C. A. (2021). Polymeric nanovectors incorporated with ganciclovir and HSV-tk encoding plasmid for gene-directed enzyme prodrug therapy. Molecules 26, 1759. doi:10.3390/molecules26061759
Scanlan, H., Coffman, Z., Bettencourt, J., Shipley, T., and Bramblett, D. E. (2022). Herpes simplex virus 1 as an oncolytic viral therapy for refractory cancers. Front. Oncol. 12, 940019. doi:10.3389/fonc.2022.940019
Shayan, S., Arashkia, A., Bahramali, G., Abdoli, A., Nosrati, M. S. S., and Azadmanesh, K. (2022). Cell type-specific response of colon cancer tumor cell lines to oncolytic HSV-1 virotherapy in hypoxia. Cancer Cell Int. 22, 164. doi:10.1186/s12935-022-02564-4
Shi, T., Song, X., Wang, Y., Liu, F., and Wei, J. (2020). Combining oncolytic viruses with cancer immunotherapy: Establishing a new generation of cancer treatment. Front. Immunol. 11, 683. doi:10.3389/fimmu.2020.00683
Siewert, J. R., and Ott, K. (2007). Are squamous and adenocarcinomas of the esophagus the same disease? Semin. Radiat. Oncol. 17, 38–44. doi:10.1016/j.semradonc.2006.09.007
Sokolowski, N. A., Rizos, H., and Diefenbach, R. J. (2015). Oncolytic virotherapy using herpes simplex virus: How far have we come? Oncolytic Virother 4, 207–219. doi:10.2147/ov.S66086
Stanziale, S. F., Petrowsky, H., Joe, J. K., Roberts, G. D., Zager, J. S., Gusani, N. J., et al. (2002). Ionizing radiation potentiates the antitumor efficacy of oncolytic herpes simplex virus G207 by upregulating ribonucleotide reductase. Surgery 132, 353–359. doi:10.1067/msy.2002.125715
Stanziale, S. F., Stiles, B. M., Bhargava, A., Kerns, S. A., Kalakonda, N., and Fong, Y. (2004). Oncolytic herpes simplex virus-1 mutant expressing green fluorescent protein can detect and treat peritoneal cancer. Hum. Gene Ther. 15, 609–618. doi:10.1089/104303404323142051
Su, Y., Su, C., and Qin, L. (2022). Current landscape and perspective of oncolytic viruses and their combination therapies. Transl. Oncol. 25, 101530. doi:10.1016/j.tranon.2022.101530
Sugawara, K., Iwai, M., Yajima, S., Tanaka, M., Yanagihara, K., Seto, Y., et al. (2020). Efficacy of a third-generation oncolytic herpes virus G47Δ in advanced stage models of human gastric cancer. Mol. Ther. Oncolytics 17, 205–215. doi:10.1016/j.omto.2020.03.022
Sung, H., Ferlay, J., Siegel, R. L., Laversanne, M., Soerjomataram, I., Jemal, A., et al. (2021). Global cancer statistics 2020: GLOBOCAN estimates of incidence and mortality worldwide for 36 cancers in 185 countries. CA Cancer J. Clin. 71, 209–249. doi:10.3322/caac.21660
Suzuki, T., Uchida, H., Shibata, T., Sasaki, Y., Ikeda, H., Hamada-Uematsu, M., et al. (2021). Potent anti-tumor effects of receptor-retargeted syncytial oncolytic herpes simplex virus. Mol. Ther. Oncolytics 22, 265–276. doi:10.1016/j.omto.2021.08.002
Tanabe, S., Tazawa, H., Kagawa, S., Noma, K., Takehara, K., Koujima, T., et al. (2015). Abstract CT123: Phase I/II trial of endoscopic intratumoral administration of OBP-301, a novel telomerase-specific oncolytic virus, with radiation in elderly esophageal cancer patients. Cancer Res. 75, CT123. doi:10.1158/1538-7445.Am2015-ct123
Tang, J., Ma, X., Chen, X., Feng, M., Jin, X., Wang, K., et al. (2022). Strengthening of antitumor effects in breast cancer from a novel B7-H4- and CD3-targeting bispecific antibody by an oncolytic virus. Ann. Transl. Med. 10, 805. doi:10.21037/atm-22-3423
Tian, L., Xu, B., Chen, Y., Li, Z., Wang, J., Zhang, J., et al. (2022). Specific targeting of glioblastoma with an oncolytic virus expressing a cetuximab-CCL5 fusion protein via innate and adaptive immunity. Nat. Cancer 3, 1318–1335. doi:10.1038/s43018-022-00448-0
Tsuji, T., Nakamori, M., Iwahashi, M., Nakamura, M., Ojima, T., Iida, T., et al. (2013). An armed oncolytic herpes simplex virus expressing thrombospondin-1 has an enhanced in vivo antitumor effect against human gastric cancer. Int. J. Cancer 132, 485–494. doi:10.1002/ijc.27681
Unterholzner, L. (2013). The interferon response to intracellular DNA: Why so many receptors? Immunobiology 218, 1312–1321. doi:10.1016/j.imbio.2013.07.007
Vähä-Koskela, M., and Hinkkanen, A. (2014). Tumor restrictions to oncolytic virus. Biomedicines 2, 163–194. doi:10.3390/biomedicines2020163
Van Der Woude, L. L., Gorris, M. A. J., Halilovic, A., Figdor, C. G., and De Vries, I. J. M. (2017). Migrating into the tumor: A roadmap for T cells. Trends Cancer 3, 797–808. doi:10.1016/j.trecan.2017.09.006
Wang, J., Du, L., and Chen, X. (2022a). Oncolytic virus: A catalyst for the treatment of gastric cancer. Front. Oncol. 12, 1017692. doi:10.3389/fonc.2022.1017692
Wang, R., Chen, J., Wang, W., Zhao, Z., Wang, H., Liu, S., et al. (2022b). CD40L-armed oncolytic herpes simplex virus suppresses pancreatic ductal adenocarcinoma by facilitating the tumor microenvironment favorable to cytotoxic T cell response in the syngeneic mouse model. J. Immunother. Cancer 10, e003809. doi:10.1136/jitc-2021-003809
Watanabe, D., and Goshima, F. (2018). Oncolytic virotherapy by HSV. Adv. Exp. Med. Biol. 1045, 63–84. doi:10.1007/978-981-10-7230-7_4
Watson, G., Xu, W., Reed, A., Babra, B., Putman, T., Wick, E., et al. (2012). Sequence and comparative analysis of the genome of HSV-1 strain McKrae. Virology 433, 528–537. doi:10.1016/j.virol.2012.08.043
Wei, K., and Hackert, T. (2021). Surgical treatment of pancreatic ductal adenocarcinoma. Cancers (Basel) 13, 1971. doi:10.3390/cancers13081971
Wong, J., Kelly, K., Mittra, A., Gonzalez, S. J., Song, K. Y., Simpson, G., et al. (2010). A third-generation herpesvirus is effective against gastroesophageal cancer. J. Surg. Res. 163, 214–220. doi:10.1016/j.jss.2010.03.021
Workenhe, S. T., and Mossman, K. L. (2014). Oncolytic virotherapy and immunogenic cancer cell death: Sharpening the sword for improved cancer treatment strategies. Mol. Ther. 22, 251–256. doi:10.1038/mt.2013.220
Workenhe, S. T., Simmons, G., Pol, J. G., Lichty, B. D., Halford, W. P., and Mossman, K. L. (2014). Immunogenic HSV-mediated oncolysis shapes the antitumor immune response and contributes to therapeutic efficacy. Mol. Ther. 22, 123–131. doi:10.1038/mt.2013.238
Xu, R., Hu, Y., Xie, Q., Zhang, C., Zhao, Y., Zhang, H., et al. (2022). LRRC8A is a promising prognostic biomarker and therapeutic target for pancreatic adenocarcinoma. Cancers (Basel) 14, 5526. doi:10.3390/cancers14225526
Yajima, S., Sugawara, K., Iwai, M., Tanaka, M., Seto, Y., and Todo, T. (2021). Efficacy and safety of a third-generation oncolytic herpes virus G47Δ in models of human esophageal carcinoma. Mol. Ther. Oncolytics 23, 402–411. doi:10.1016/j.omto.2021.10.012
Yamamura, K., Kasuya, H., Sahin, T. T., Tan, G., Hotta, Y., Tsurumaru, N., et al. (2014). Combination treatment of human pancreatic cancer xenograft models with the epidermal growth factor receptor tyrosine kinase inhibitor erlotinib and oncolytic herpes simplex virus HF10. Ann. Surg. Oncol. 21, 691–698. doi:10.1245/s10434-013-3329-3
Yan, N., and Chen, Z. J. (2012). Intrinsic antiviral immunity. Nat. Immunol. 13, 214–222. doi:10.1038/ni.2229
Yanai, H., Ban, T., Wang, Z., Choi, M. K., Kawamura, T., Negishi, H., et al. (2009). HMGB proteins function as universal sentinels for nucleic-acid-mediated innate immune responses. Nature 462, 99–103. doi:10.1038/nature08512
Yang, S., Pang, L., Dai, W., Wu, S., Ren, T., Duan, Y., et al. (2021). Role of forkhead box O proteins in hepatocellular carcinoma biology and progression (review). Front. Oncol. 11, 667730. doi:10.3389/fonc.2021.667730
Yi, Q. Y., Bai, Z. S., Cai, B., Chen, N., Chen, L. S., Yuan, T., et al. (2018). HSV-TK/GCV can induce cytotoxicity of retinoblastoma cells through autophagy inhibition by activating MAPK/ERK. Oncol. Rep. 40, 682–692. doi:10.3892/or.2018.6454
Yoon, S. S., Carroll, N. M., Chiocca, E. A., and Tanabe, K. K. (1998). Cancer gene therapy using a replication-competent herpes simplex virus type 1 vector. Ann. Surg. 228, 366–374. doi:10.1097/00000658-199809000-00009
Zhang, B., Huang, J., Tang, J., Hu, S., Luo, S., Luo, Z., et al. (2021). Intratumoral OH2, an oncolytic herpes simplex virus 2, in patients with advanced solid tumors: a multicenter, phase I/II clinical trial. J Immunother Cancer 9. doi:10.1136/jitc-2020-002224
Zhang, N., Li, J., Yu, J., Wan, Y., Zhang, C., Zhang, H., et al. (2022a). Construction of an IL12 and CXCL11 armed oncolytic herpes simplex virus using the CRISPR/Cas9 system for colon cancer treatment. Virus Res. 323, 198979. doi:10.1016/j.virusres.2022.198979
Zhang, W., Bao, L., Yang, S., Qian, Z., Dong, M., Yin, L., et al. (2016). Tumor-selective replication herpes simplex virus-based technology significantly improves clinical detection and prognostication of viable circulating tumor cells. Oncotarget 7, 39768–39783. doi:10.18632/oncotarget.9465
Zhang, Y., He, H., He, L., and Shi, B. (2022b). IL-6 accelerates the proliferation and metastasis of pancreatic cancer cells via the miR-455-5p/IGF-1R Axis. Cancer Biother Radiopharm. doi:10.1089/cbr.2022.0045
Zhao, J., Qin, C., Liu, Y., Rao, Y., and Feng, P. (2020). Herpes simplex virus and pattern recognition receptors: An arms race. Front. Immunol. 11, 613799. doi:10.3389/fimmu.2020.613799
Zhao, Q., Yu, J., and Meng, X. (2019). A good start of immunotherapy in esophageal cancer. Cancer Med. 8, 4519–4526. doi:10.1002/cam4.2336
Zhao, Z., and Liu, W. (2020). Pancreatic cancer: A review of risk factors, diagnosis, and treatment. Technol. Cancer Res. Treat. 19, 1533033820962117. doi:10.1177/1533033820962117
Zhou, H., He, Z., Wang, C., Xie, T., Liu, L., Liu, C., et al. (2016). Intravenous administration is an effective and safe route for cancer gene therapy using the bifidobacterium-mediated recombinant HSV-1 thymidine kinase and ganciclovir. Int. J. Mol. Sci. 17, 891. doi:10.3390/ijms17060891
Zhou, H., Liu, H., Zhang, Y., Xin, Y., Huang, C., Li, M., et al. (2022). PFH/AGM-CBA/HSV-TK/LIPOSOME-Affibody": Novel targeted nano ultrasound contrast agents for ultrasound imaging and inhibited the growth of ErbB2-overexpressing gastric cancer cells. Drug Des. Devel Ther. 16, 1515–1530. doi:10.2147/dddt.S351623
Zhu, H., Zhang, R., Yi, L., Tang, Y. D., and Zheng, C. (2022a). UNC93B1 attenuates the cGAS-STING signaling pathway by targeting STING for autophagy-lysosome degradation. J. Med. Virol. 94, 4490–4501. doi:10.1002/jmv.27860
Keywords: HSV, digestive system tumors, oncolytic virus, immunology, cancer
Citation: Li S, Li Q, Ren Y, Yi J, Guo J and Kong X (2023) HSV: The scout and assault for digestive system tumors. Front. Mol. Biosci. 10:1142498. doi: 10.3389/fmolb.2023.1142498
Received: 11 January 2023; Accepted: 15 February 2023;
Published: 28 February 2023.
Edited by:
Pier Paolo Piccaluga, S. Orsola-Malpighi Hospital, ItalyReviewed by:
Lance Hellman, Nevada State College, United StatesZong Sheng Guo, University at Buffalo, United States
Copyright © 2023 Li, Li, Ren, Yi, Guo and Kong. This is an open-access article distributed under the terms of the Creative Commons Attribution License (CC BY). The use, distribution or reproduction in other forums is permitted, provided the original author(s) and the copyright owner(s) are credited and that the original publication in this journal is cited, in accordance with accepted academic practice. No use, distribution or reproduction is permitted which does not comply with these terms.
*Correspondence: Jinhe Guo, Z3VvamluaGVAdGp1dGNtLmVkdS5jbg==; Xianbin Kong, ODlrb25neGlhbmJpbkB0anV0Y20uZWR1LmNu
†These authors have contributed equally to this work