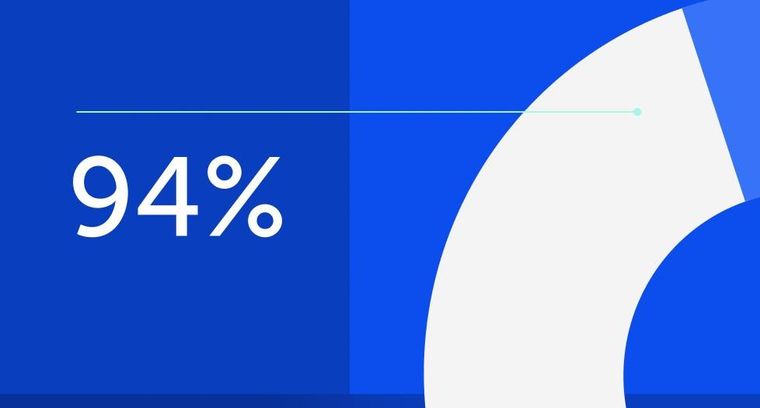
94% of researchers rate our articles as excellent or good
Learn more about the work of our research integrity team to safeguard the quality of each article we publish.
Find out more
MINI REVIEW article
Front. Mol. Biosci., 16 February 2023
Sec. Molecular Diagnostics and Therapeutics
Volume 10 - 2023 | https://doi.org/10.3389/fmolb.2023.1126055
This article is part of the Research TopicMolecular Mechanisms Underlying Polycystic Kidney Disease: From the Smallest Bricks to the Big ScenarioView all 10 articles
Autosomal Dominant Polycystic Kidney Disease (ADPKD) leads to end stage kidney disease (ESKD) through the development and expansion of multiple cysts throughout the kidney parenchyma. An increase in cyclic adenosine monophosphate (cAMP) plays an important role in generating and maintaining fluid-filled cysts because cAMP activates protein kinase A (PKA) and stimulates epithelial chloride secretion through the cystic fibrosis transmembrane conductance regulator (CFTR). A vasopressin V2 receptor antagonist, Tolvaptan, was recently approved for the treatment of ADPKD patients at high risk of progression. However additional treatments are urgently needed due to the poor tolerability, the unfavorable safety profile, and the high cost of Tolvaptan. In ADPKD kidneys, alterations of multiple metabolic pathways termed metabolic reprogramming has been consistently reported to support the growth of rapidly proliferating cystic cells. Published data suggest that upregulated mTOR and c-Myc repress oxidative metabolism while enhancing glycolytic flux and lactic acid production. mTOR and c-Myc are activated by PKA/MEK/ERK signaling so it is possible that cAMPK/PKA signaling will be upstream regulators of metabolic reprogramming. Novel therapeutics opportunities targeting metabolic reprogramming may avoid or minimize the side effects that are dose limiting in the clinic and improve on the efficacy observed in human ADPKD with Tolvaptan.
Autosomal dominant polycystic kidney disease (ADPKD) is the most common inherited cause of kidney disease with an estimated prevalence between 1:400 and 1:1,000 (Torres et al., 2007; Chow and Ong, 2009; Harris and Torres, 2009). In ADPKD, enlarging fluid-filled cysts develop in both kidneys, eventually leading to kidney failure. Besides kidney cysts that can be very painful, ADPKD can present with extra-renal manifestations such as development of cysts in the liver, pancreas, spleen and epididymis, abnormal heart valves and brain aneurysm (Perrone et al., 2015). Common features of ADPKD are flank and abdominal pain, urinary tract infections, hypertension, and kidney stones (Gabow, 1990; Torres et al., 2007). ADPKD is predominantly caused by mutations in either PKD1 or PKD2 genes encoding for two ciliary proteins, Polycystin 1 (PC1) and Polycystin 2 (PC2) (Harris and Torres, 2009; Takiar and Caplan, 2011). These mutations within epithelial cells of the kidney interfere with multiple pathways located within the cilia and promote proliferation, de-differentiation and fluid secretion resulting in growth of these cells into cysts. Due to the slow progression and the intrafamilial difference in disease severity, it has been suggested that defective clearance of precipitated microcrystals may promote cyst formation and drive kidney injury when Pkd1 or Pkd2 are mutated (Torres et al., 2019a). An increase in cyclic adenosine monophosphate (cAMP) and a simultaneous dysregulation in intracellular calcium in the cystic epithelium seems to play a key role in generating and maintaining fluid-filled cysts (Yamaguchi et al., 2003; Belibi et al., 2004; Di Mise et al., 2018). cAMP activates protein kinase A (PKA) and stimulates epithelial chloride secretion through the cystic fibrosis transmembrane conductance regulator (CFTR) (Sullivan et al., 1998). A vasopressin V2 receptor (V2R) antagonist, tolvaptan, was recently approved to preserve kidney function in ADPKD by lowering vasopressin-mediated cAMP increase (Chebib and Torres 2021). However, considering the potential drawbacks of Tolvaptan i.e., side effects, poor tolerability, and high cost it is important to identify additional pathways and novel therapeutic interventions. Recently, alterations of metabolic pathways (metabolic reprogramming) in ADPKD have shown that the abnormal cystic growth utilize aerobic glycolysis, glutaminolysis and reducing oxidative phosphorylation (OXPHOS) (Padovano et al., 2018; Podrini et al., 2020). This review will review and discuss potential therapeutic approaches targeting metabolism-based pathways in ADPKD.
Tolvaptan is a vasopressin-2-receptor (V2R) antagonist approved to slow kidney function decline in adult patients with rapidly progressive ADPKD by reducing cAMP levels (Chebib and Torres 2021, Figure 1). Tolvaptan reduces TKV (Total kidney volume TKV, prognostic biomarker for risk assessment in ADPKD, Fick-Brosnahan et al., 2002) and renal function decline. However, its clinical use is limited by poor tolerability due to aquaretic symptoms, potential liver failure and high cost (Chebib and Torres 2021; Müller et al., 2022). Recently Lixivaptan, a selective vasopressin V2 receptor antagonist which was predicted to have a lower risk of hepatotoxicity compared to tolvaptan was discontinued (https://investors.centessa.com/news-releases/news-release-details/centessa-pharmaceuticals-makes-strategic-decision-discontinue). In the kidney, the V2 receptor is mainly expressed in the distal nephron potentially limiting the area of action of V2R antagonists (Mutig et al., 2007; Sparapani et al., 2021). Tolvaptan has been shown to exhibit a partial agonist activity on β-arrestin recruitment whose expression is increased in human ADPKD kidneys (Xu et al., 2018). These data suggest that there is space for safer and tolerated best in class cAMP lowering approaches in ADPKD either targeting additional pathways regulating cAMP (PKA inhibition, PDE activation), biased V2R antagonists or combination with targets that may provide an additive or synergistic effect such as the calcium-sensing receptor (Di Mise et al., 2021; Zhou and Torres, 2022).
FIGURE 1. Metabolic Pathways in ADPKD. Increased cAMP due to vasopressin (Chebib et al., 2015) but likely also to decreased phosphodiesterases (PDEs) (Pinto et al., 2016) play a key role in generating fluid-filled cysts. Defects in PC1 and PC2 mediated calcium ion influx in the primary cilia and/or in the endoplasmic reticulum (ER) (Nauli et al., 2003; Padhy et al., 2022). Decreased intracellular calcium seems to convert the antiproliferative to proliferative effect of cAMP (Yamaguchi et al., 2003) causing activation of MEK-ERK and increased cell proliferation. cAMP activates PKA and stimulates chloride secretion through the cystic fibrosis transmembrane conductance regulator (CFTR) (Sullivan et al., 1998). Tolvaptan, a vasopressin V2 receptor antagonist, was approved to preserve kidney function by targeting cAMP (Chebib and Torres, 2021). Several somatostatin analogues (SST) are being investigated to lower cAMP with Ocreotide-LAR being approved in ADPKD in Italy (Capuano et al., 2022a). Mammalian target of rapamycin (mTOR) and c-Myc are upregulated in ADPKD and suppress oxidative metabolism while enhancing glycolytic flux, lactate production and export (LDH-A and MCT4) (Rowe et al., 2013; Podrini et al., 2020).
Octreotide long-acting release (octreotide-LAR) is a somatostatin analogue that lowers the annual slope of TKV increase with no effect on renal function worsening. It was approved in Italy for the treatment of adult ADPKD patients at high risk of progression with eGFR ranging from 15 to 30 ml/min/1.73 m2 based on less frequent doubling of serum creatinine or ESKD compared to placebo. Side effects reported for somatostatin analogues include cholelithiasis and risk of cholecystitis, alopecia and increases in blood glucose (Perico et al., 2019; Griffiths et al., 2020). Hepatic cyst infections were also reported in patients treated with Lanreotide which required hospital admission and antibiotics. It has been suggested that the small size of the trials involving somatostatin analogues can explain the inconclusive renoprotective effects (Meijer et al., 2018; Capuano et al., 2022b). While awaiting publication of the results of Lanreotide in ADPKD (LIPS, NCT02127437) a plausible explanation for the apparent different effects across analogues is the affinity for the five somatostatin receptors (SSTR1 to 5) (Suwabe et al., 2021; Bais et al., 2022; Ruggenenti et al., 2022).
The idea of metabolic reprogramming first came from the Warburg effect in cancer cells where OXPHOS is inhibited and cells tend to utilize aerobic glycolysis to produce energy (Koppenol et al., 2011). Metabolic reprogramming does not include only the Warburg effect, but also other metabolic changes. Rowe first suggested that mutations in Pkd1 result in a defective glucose metabolism with decreased gluconeogenesis and increased aerobic glycolysis to supply energy and promote proliferation (Rowe et al., 2013, Figure 1). 2-deoxyglucose (2DG), which is transported into the cells but cannot undergo glycolysis, inhibited the proliferation of Pkd1−/− cells and prevented disease progression in ADKPD models (Rowe et al., 2013; Chiaravalli et al., 2016; Riwanto et al., 2016; Lian et al., 2019). Recently, Soomro provided evidence that alteration in glutamine metabolism play a role in cyst growth (Soomro et al., 2020). During glutaminolysis the enzyme glutaminase (GLS, Figure 1) converts glutamine to glutamate then converted to a TCA cycle intermediate, alpha-ketoglutarate to generate ATP for cyst growth (Soomro et al., 2020). Podrini confirmed the defective glucose metabolism and characterized other altered metabolic pathways in mouse kidney without Pkd1 such as increased pentose phosphate pathway (PPP), glutamine uptake and decreased TCA cycle and fatty acid oxidation (FAO) (Podrini et al., 2020). The authors also generated data supporting targeting asparagine synthetase to interfere with glutaminolysis in conjunction with glycolysis to slow PKD1−/− cell growth and survival (Podrini et al., 2020). Decreased FAO also appears to contribute to disease exacerbation as increased c-MYC upregulates miR-17 in mouse cystic kidneys inhibiting PPARα and leading to FAO inhibition to support proliferation of ADPKD cells. Anti-miR-17 restored PPARα and improved FAO, ameliorating ADPKD (Lee et al., 2019, Figure 1). Considering that a single miRNA specie can regulate hundreds of targets, it is unclear if the beneficial effect is mediated by PPARα (Mohr and Mott, 2015). Nevertheless, the PPARα agonist fenofibrate showed increased FAO and reduced cystic volume in preclinical ADPKD (Lakhia et al., 2018). An anti-miR17 oligonucleotide is in Phase 1b in ADPKD patients to de-repress multiple miR-17 mRNA targets including Pkd1 and Pkd2 (Lakhia et al., 2022; https://www.prnewswire.com/news-releases/regulus-therapeutics-announces-first-patient-dosed-in-phase-1b-multiple-ascending-dose-mad-clinical-trial-of-rgls8429-for-the-treatment-of-autosomal-dominant-polycystic-kidney-disease-adpkd-301665896.html).
The mechanisms that account for elevated mTOR activity in ADPKD are not fully understood but it appears that cAMP/PKA/ERK and AKT are upstream regulators (Distefano et al., 2009; Rowe et al., 2013; Margaria et al., 2020). Animal studies demonstrate that mTOR inhibition improves cystic disease and kidney function (Pema et al., 2016; Su et al., 2022), however, metanalysis of cIinical data with ADPKD patients receiving rapamycin, sirolimus, or everolimus did not support a significant influence on renal progression (Lin et al., 2019). In these trials, it is not clear if mTOR inhibition was achieved in the kidney or whether mTORC1 inhibition triggers a compensatory activation of mTORC2 limiting the beneficial effects of mTORC1 (Canaud et al., 2010). Recently Janssen announced the acquisition of Anakuria Therapeutics and its first-in-class ADPKD candidate, AT-20494, a small molecule inhibitor of mTORC1 (https://www.fdanews.com/articles/206455-janssen-acquires-anakuria-therapeutics-nets-early-phase-polycystic-kidney-disease-candidate).
AMPK is activated under conditions of metabolic and other cellular stresses (Steinberg and Hardie, 2022). AMPK activation during low energy states leads to upregulation of energy generating processes and inhibition of energy-intensive processes involved in cyst expansion such as indirect inactivation of mTORC1 (Inoki et al., 2003; Gwinn et al., 2008) and inhibition of CFTR chloride channel, thus suppressing epithelial fluid and electrolyte secretion (Caplan, 2022).
Metformin, a drug approved for T2D and polycystic ovary syndrome, may serve as a therapy for ADPKD. Treatment of kidney epithelial cells leads to stimulation of AMPK and subsequent inhibition of both mTOR and CFTR (Takiar et al., 2011). However, mixed results in animal models of PKD (Takiar et al., 2011; Leonhard et al., 2019; Lian et al., 2019; Chang et al., 2022; Pastor-Soler et al., 2022) and increased plasma lactate levels observed in Pkd1 miRNA transgenic mice, call for a careful examination of the risk benefit of metformin especially in patients with later stage of ADPKD (Chang et al., 2022). The Trial of administration of Metformin in PKD (TAME PKD, NCT02656017; Seliger et al., 2020) in 97 non-diabetic ADPKD adults with eGFR>50 ml/min per 1.73 m2 (Ong and Gansevoort, 2021; Perrone et al., 2021) suggests that metformin is safe in patients in the early stages of ADPKD (although only 35% completed the study at the maximal dose resulting in dose reductions). Results of the exploratory secondary endpoints were, however, inconclusive, with non-significant trends for eGFR slope and htTKV. A definitive answer should come from the IMPEDE PKD trial where a slow-release formulation of 2000 mg/d Metformin will be tested in 1164 patients with rapid progressive ADPKD over 2 years with estimated completion in 2026 (NCT04939935).
While metformin inhibits renal cyst growth in mouse models, it remains unclear whether its metabolic effects are related to its capacity to activate AMPK (Takiar et al., 2011; Chang A. R. et al., 2017), and it may have tolerability issues. Hence potent selective AMPK activation may be required in ADPKD. Recently, the AMPK activator PF-06409577 demonstrated inhibition of mTOR pathway-mediated proliferation of cyst-lining epithelial cells and reduced CFTR-regulated cystic fluid secretion (Su et al., 2022). Given the potential for cardiac hypertrophy of AMPK following chronic administration it will be important to define the precise isoform selectivity required (Myers et al., 2017). Recently the FDA granted Orphan Drug Designation to Poxel’s AMPK activator PXL770 for the treatment of patients with ADPKD (https://www.poxelpharma.com/en_us/news-media/press-releases/detail/224/poxel-announces-pxl770-granted-orphan-drug-designation-from).
Statins (HMG-CoA reductase inhibitors) are widely prescribed to lower cholesterol in humans (Ginsberg and Tuck, 2001). Among the additional effects that make statins attractive for use in ADPKD (Belibi and Edelstein, 2010) is activation of AMPK (Sun et al., 2006) and cAMP lowering (Kou et al., 2012). Statins have been shown to improve early-onset ADPKD (TKV improvement) in children and young adults (Cadnapaphornchai et al., 2014). Recently, Baliga conducted targeted metabolomics in plasma samples from a phase III trial designed to test the efficacy of pravastatin on ADPKD progression in children and young adults on the ACE inhibitor (Baliga et al., 2021). The authors demonstrated changes in metabolites involved in metabolic reprogramming however statin treatment for 36 months had limited effect on disease progression (Baliga et al., 2021). While these results are overall encouraging, a larger randomized trial in young people with ADPKD is required. In the absence of such data, no consensus was reached on the use of statins in this population (Gimpel et al., 2019). An ongoing trial evaluating 2 years treatment with pravastatin in 150 adults with early stage ADPKD (NCT03273413) should also clarify the s inconclusive results in adult ADPKD (van Dijk et al., 2001; Fassett et al., 2010; Brosnahan et al., 2017; Xue et al., 2020).
Bempedoic acid (BA) antagonizes the ATP citrate-lyase (ACLY) enzyme upstream of HMGCoA reductase and is approved as an adjunct to diet and statin therapy in familial hypercholesterolemic patients who require additional lowering of LDL-C (Huynh, 2019; Ruscica et al., 2022). In animal models BA also activates AMPK (Pinkosky et al., 2016; Hallows et al., 2022) and reduces cystic growth, TKW and BUN (Hallows et al., 2022). BA seems to have a reduced risk of muscle-related side effects reported with statins (Ruscica et al., 2022) although a recent meta-analysis concluded that statins cause a small risk of muscle symptoms that are outweighed by the known cardiovascular benefits of statins (Cholesterol Treatment Trialists' Collaboration, 2022). No major safety concerns were identified for BA in a randomized controlled phase III trial during the intervention period when added to statin therapy, but the incidence of AEs leading to discontinuation was higher in the BA group, as was the incidence of gout (Ray et al., 2019). Bempedoic acid was generally well-tolerated following a single oral dose in subjects with renal impairment (Amore et al., 2022). Because ACLY has been reported to inhibit the AMPK-β1 subunit (Lee et al., 2015), future studies should conclusively demonstrate that the beneficial effects of BA in ADPKD are mediated by AMPK activation and clarify the AMPK subunit involved.
Similar to the general population, the prevalence of overweight and obese ADPKD patients is increasing. In rodent models of ADPKD, caloric restriction has shown to slow kidney growth and improve kidney function (Kipp et al., 2016; Warner et al., 2016). It has been suggested that these improvements involve mTOR signaling inhibition, AMPK activation, and a reduction in IGF-I supporting restoration of metabolic reprogramming. Accordingly, a clinical trial evaluating the effect of weekly caloric reduction achieved with either caloric restriction or intermittent fasting in 29 overweight/obese individuals with ADPKD was recently completed (Hopp et al., 2021; https://clinicaltrials.gov/ct2/show/NCT03342742). The trial was designed as a weight loss intervention based on the prior epidemiological observation that ADPKD progression is faster with higher BMI (Nowak et al., 2018). The investigators demonstrated the feasibility of 1-year daily caloric restriction (DCR) and intermittent fasting (IMF) in a cohort of overweight or obese patients with ADPKD. Weight loss occurred with both DCR and IMF, however, weight loss was greater, and adherence and tolerability were better with caloric restriction (Hopp et al., 2021). The study was a pilot and feasibility study, so the sample size was small, and a control group was not included but, according to the investigators, similar annual kidney growth in both groups was observed that was qualitatively low compared to historical controls. Cessation of kidney growth was observed in participants who achieved clinically meaningful weight loss (Nowak et al., 2018; Nowak et al., 2021). A larger 2-year phase 2 trial with a direct comparison of caloric restriction to a control group, powered for a primary endpoint of change in htTKV (NCT04907799) is currently recruiting with an expected completion in 2026 (https://clinicaltrials.gov/ct2/show/NCT04907799).
Ketosis improves the phenotype of animal models of ADPKD (Torres et al., 2019b). From a mechanism of action perspective, it is possible that Ketone bodies may promote metabolic reprogramming by decreasing glucose availability and increasing fatty acids (Hall et al., 2016). Initial data from clinical trials are becoming available. A self-enrolled survey of ADPKD patients who have self-administered ketogenic diet for at least 6 months reported weight loss and blood pressure lowering together with improvement in PKD symptoms and eGFR in a subgroup of patients. Caution should be applied to the interpretation of this retrospective study since only half of the patients were able to comply with the diet and the side effects reported suggest potential long-term tolerability and safety issues (kidney stones, increased cholesterol) (Strubl et al., 2021). In a follow-up study, Oehm demonstrated the feasibility of a short-term ketogenic intervention in 10 ADPKD patients (RESET-PKD 72h fast or 14 days of a KD) where TLV was decreased while no changes in TKV were observed (Oehm et al., 2022a; Oehm et al., 2022b). Despite the challenges identified, large-scale trials such as the ongoing KETO-ADPKD (NCT04680780) study will address the feasibility and the therapeutic potential of longer-term ketogenesis interventions in ADPKD (Ong and Torra, 2022). Adherence (ketone concentrations), feasibility and secondary outcomes including TKV, and BMI will be evaluated. Based on these findings, Bruen and collaborators have designed a plant-focused ketogenic diet (Ren.Nu diet) for ADPKD based on the theory that a diet high in carbohydrate and animal protein might accelerate disease progression (Bruen et al., 2022). A preliminary beta test was conducted for 12 weeks in 24 ADPKD patients and with the obvious limitations of the study (no control, selection bias, self-reporting). Preliminary data suggest reasonable adherence and feasibility (Bruen et al., 2022).
Bariatric surgery is an effective option to achieve sustained weight loss and improving hypertension and diabetes. Gastric bypass and sleeve gastrectomy result in 20%–30% weight loss (Brajcich and Hungness, 2020) and is expected to impact metabolic reprogramming. Therefore, it is important to understand the benefits and risks of bariatric surgery in ADPKD patients. While evidence suggests important trends for bariatric surgery and overall kidney related outcomes in patients with CKD, there exist several renal risks, including acute kidney injury, and risks of nephrolithiasis, oxalate nephropathy that will need to be considered in a comprehensive risk benefit assessment profile in ADPKD patients (Chang M. Y. et al., 2017).
Several GLP-1 receptor agonists have been approved for the treatment of T2D and obesity and are being considered for liver and kidney complications (Müller et al., 2019; Brown et al., 2021; Newsome et al., 2021). One attractive feature of new generation GLP-1 analogues is the propound weight loss (>10%) achieved in obese and diabetic patients (Frías et al., 2021). Importantly, GLP-1 exerts its effects by binding to GLP-1R and activating adenylate cyclase, which leads to the generation of cAMP, so it will be important to assess the expression of GLP-1R on the cystic epithelium and the potential impact of GLP-1 agonism on cAMP (Körner et al., 2007; Müller et al., 2019).
Dual agonism at the GLP-1 and glucagon receptors has shown superior weight lowering effect to selective GLP-1 agonism (Pocai et al., 2009; Day et al., 2012). Because glucagon lowers mTORC1 and stimulates AMPK (Baum et al., 2009; Welles et al., 2020) and ketogenesis (Pocai, 2012; Torres et al., 2019a), the simultaneous agonism of GLP-1R and glucagon receptors constitute a potential approach for ADPKD. A recent observational study in ADPKD patients with higher endogenous glucagon did not provide evidence for a protective role of glucagon in ADPKD (Knol et al., 2021). Mechanistic studies are needed to determine the relationship between glucagon and ADPKD and evaluate the expression of the receptor in kidney cysts. Future studies will clarify if the body weight lowering effect together with the other reported desirable actions of GLP-1 agonists have potential in ADPKD.
TZD are Peroxisome Proliferator Activator Receptor gamma (PPARγ) agonists approved for T2D. Preclinical studies have tested TZD and found reduced progression of cystic disease (Blazer-Yost et al., 2010; Flaig et al., 2016). A small phase 1b clinical trial was designed to investigate safety and tolerability of low-dose (15 mg) pioglitazone (Blazer-Yost et al., 2021). Concerns about fluid retention, bone loss and weight gain have reduced their use in the clinic and need to be considered in an appropriate risk/benefit assessment in ADPKD patients (Lebovitz, 2019).
SGLT2 inhibitors (SGLT2i) prevent the reabsorption of filtered glucose from the tubular lumen resulting in glucose lowering and additional benefits of weight loss and blood pressure reduction (DeFronzo et al., 2021). While the mechanisms contributing to these beneficial effects are unknown, SGLT2i switch metabolism to a ketotic state and increase plasma glucagon potentially regulating PKA and mTOR pathways (Saponaro et al., 2018). ADPKD patients are more prone to urinary tract infections and were excluded from renal studies as genitourinary infection is a potential risk of SGLT2i (Afsar et al., 2022). In two PKD animal models, SGLT2 inhibition did not reduce cyst growth (Kapoor et al., 2015; Rodriguez et al., 2015). However, because of the beneficial effects of SGLT2 inhibition on kidney function, vascular function, and mortality (DeFronzo et al., 2021), a clinical trial is ongoing in ADPKD patients (NCT05510115).
ADPKD is the leading genetic cause of ESKD. Recent advances in understanding the mechanisms leading to cyst formation and progression has led to the approval of tolvaptan (Torres et al., 2017). Treatment of ADPKD still represents a challenge due to the poor tolerability and the unfavorable safety profile of Tolvaptan. Thanks to new scientific discovery and preclinical models, new targets are being investigated. Metabolic defect in ADPKD support cell proliferation of rapid growing tissues leads to cystic epithelial proliferation and growth. Upregulated mTOR and c-Myc play a major role in repressing oxidative metabolism and FAO while enhancing glycolytic flux, lactic acid production, PPP and glutaminolysis downstream of cAMP/PKA (Rowe et al., 2013; Podrini et al., 2020). It will be important to expand upon the role of dysregulated metabolism as metabolic defects in cells within the kidney microenvironment may also contribute to ADPKD progression. A better understanding of the human pathways regulated by approved therapies (Tolvaptan and Octreotide-LAR) and initial results from ongoing trials with metabolic drugs should provide valuable human data and help expedite the development of new ADPKD therapeutics. Recently, the trial in ADPKD with Venglustat (Glucosylceramide synthase inhibitor; Natoli et al., 2010) was discontinued as it did not reduce TKV growth rate (https://www.sanofi.com/en/media-room/press-releases/2021/2021-06-01-05-00-00-2239122). Trial testing metformin in ADPKD should be completed by 2026 and provide valuable information (Testa and Magistroni, 2020). Weight loss targeting metabolic alterations has the potential to be a disease modifying intervention in ADPKD, but behavioral dietary interventions are limited by long-term adherence (Quiroga and Torra, 2022) so it will be important to explore the potential of bariatric surgery and pharmacological approaches. Therefore, it is tempting to speculate that interventions targeting downstream events such as metabolic reprogramming may retain and improve on the tolerability and efficacy reported with Tolvaptan offering potential new therapeutic opportunities. Ongoing trials may help in answering some of these questions.
IB: writing original draft. AP: writing original draft, reviewing and editing.
The authors declare that the research was conducted in the absence of any commercial or financial relationships that could be construed as a potential conflict of interest.
All claims expressed in this article are solely those of the authors and do not necessarily represent those of their affiliated organizations, or those of the publisher, the editors and the reviewers. Any product that may be evaluated in this article, or claim that may be made by its manufacturer, is not guaranteed or endorsed by the publisher.
Afsar, B., Afsar, R. E., Demiray, A., Altay, S., Korkmaz, H., Yildiz, A., et al. (2022). Sodium-glucose cotransporter inhibition in polycystic kidney disease: Fact or fiction. Clin. Kidney J. 15 (7), 1275–1283. doi:10.1093/ckj/sfac029
Amore, B. M., Sasiela, W. J., Ries, D. K., Tresh, P., and Emery, M. G. (2022). Pharmacokinetics of bempedoic acid in patients with renal impairment. Clin. Transl. Sci. 15 (3), 789–798. doi:10.1111/cts.13202
Bais, T., Gansevoort, R. T., and Meijer, E. (2022). Drugs in clinical development to treat autosomal dominant polycystic kidney disease. Drugs 82, 1095–1115. doi:10.1007/s40265-022-01745-9
Baliga, M. M., Klawitter, J., Christians, U., Hopp, K., Chonchol, M., Gitomer, B. Y., et al. (2021). Metabolic profiling in children and young adults with autosomal dominant polycystic kidney disease. Sci. Rep. 11 (1), 6629. doi:10.1038/s41598-021-84609-8
Baum, J. I., Kimball, S. R., and Jefferson, L. S. (2009). Glucagon acts in a dominant manner to repress insulin-induced mammalian target of rapamycin complex 1 signaling in perfused rat liver. Am. J. Physiol. Endocrinol. Metab. 297 (2), E410–E415. doi:10.1152/ajpendo.00042.2009
Belibi, F. A., and Edelstein, C. L. (2010). Novel targets for the treatment of autosomal dominant polycystic kidney disease. Expert. Opin. Investig. Drugs. 19 (3), 315–328. doi:10.1517/13543781003588491
Belibi, F. A., Reif, G., Wallace, D. P., Yamaguchi, T., Olsen, L., Li, H., et al. (2004). Cyclic AMP promotes growth and secretion in human polycystic kidney epithelial cells. Kidney Int. 66 (3), 964–973. doi:10.1111/j.1523-1755.2004.00843.x
Blazer-Yost, B. L., Bacallao, R. L., Erickson, B. J., LaPradd, M. L., Edwards, M. E., Sheth, N., et al. (2021). A randomized phase 1b cross-over study of the safety of low-dose pioglitazone for treatment of autosomal dominant polycystic kidney disease. Clin. Kidney J. 14 (7), 1738–1746. doi:10.1093/ckj/sfaa232
Blazer-Yost, B. L., Haydon, J., Eggleston-Gulyas, T., Chen, J. H., Wang, X., Gattone, V., et al. (2010). Pioglitazone attenuates cystic burden in the PCK rodent model of polycystic kidney disease. PPAR Res. 2010, 274376. doi:10.1155/2010/274376
Brajcich, B. C., and Hungness, E. S. (2020). Sleeve gastrectomy. JAMA. 324 (9), 908. doi:10.1001/jama.2020.14775
Brosnahan, G. M., Abebe, K. Z., Rahbari-Oskoui, F. F., Patterson, C. G., Bae, K. T., Schrier, R. W., et al. (2017). Effect of statin therapy on the progression of autosomal dominant polycystic kidney disease. A secondary analysis of the halt PKD trials. Curr. Hypertens. Rev. 13 (2), 109–120. doi:10.2174/1573402113666170427142815
Brown, E., Heerspink, H. J. L., Cuthbertson, D. J., and Wilding, J. P. H. (2021). SGLT2 inhibitors and GLP-1 receptor agonists: Established and emerging indications. Lancet 398 (10296), 262–276. doi:10.1016/S0140-6736(21)00536-5
Bruen, D. M., Kingaard, J. J., Munits, M., Paimanta, C. S., Torres, J. A., Saville, J., et al. (2022). Ren.Nu, a dietary program for individuals with autosomal-dominant polycystic kidney disease implementing a sustainable, plant-focused, kidney-safe, ketogenic approach with avoidance of renal stressors. Kidney Dial. 2, 183–203. doi:10.3390/kidneydial2020020
Cadnapaphornchai, M. A., George, D. M., McFann, K., Wang, W., Gitomer, B., Strain, J. D., et al. (2014). Effect of pravastatin on total kidney volume, left ventricular mass index, and microalbuminuria in pediatric autosomal dominant polycystic kidney disease. Clin. J. Am. Soc. Nephrol. 9 (5), 889–896. doi:10.2215/CJN.08350813
Canaud, G., Knebelmann, B., Harris, P. C., Vrtovsnik, F., Correas, J. M., Pallet, N., et al. (2010). Therapeutic mTOR inhibition in autosomal dominant polycystic kidney disease: What is the appropriate serum level? Am. J. Transpl. 10 (7), 1701–1706. doi:10.1111/j.1600-6143.2010.03152.x
Caplan, M. J. (2022). AMPK and polycystic kidney disease drug development: An interesting off-target target. Front. Med. 9, 753418. doi:10.3389/fmed.2022.753418
Capuano, I., Buonanno, P., Riccio, E., Amicone, M., and Pisani, A. (2022a). Therapeutic advances in ADPKD: The future awaits. J. Nephrol. 35 (2), 397–415. doi:10.1007/s40620-021-01062-6
Capuano, I., Buonanno, P., Riccio, E., Rizzo, M., and Pisani, A. (2022b). Tolvaptan vs. somatostatin in the treatment of ADPKD: A review of the literature. Clin. Nephrol. 97 (3), 131–140. doi:10.5414/CN110510
Chang, A. R., Grams, M. E., and Navaneethan, S. D. (2017). Bariatric surgery and kidney-related outcomes. Kidney Int. Rep. 2 (2), 261–270. doi:10.1016/j.ekir.2017.01.010
Chang, M. Y., Ma, T. L., Hung, C. C., Tian, Y. C., Chen, Y. C., Yang, C. W., et al. (2017). Metformin inhibits cyst formation in a zebrafish model of polycystin-2 deficiency. Sci. Rep. 7 (1), 7161. doi:10.1038/s41598-017-07300-x
Chang, M. Y., Tsai, C. Y., Chou, L. F., Hsu, S. H., Yang, H. Y., Hung, C. C., et al. (2022). Metformin induces lactate accumulation and accelerates renal cyst progression in Pkd1-deficient mice. Hum. Mol. Genet. 31 (10), 1560–1573. doi:10.1093/hmg/ddab340
Chebib, F. T., Sussman, C. R., Wang, X., Harris, P. C., and Torres, V. E. (2015). Vasopressin and disruption of calcium signalling in polycystic kidney disease. Nat. Rev. Nephrol. 11 (8), 451–464. doi:10.1038/nrneph.2015.39
Chebib, F. T., and Torres, V. E. (2021). Assessing risk of rapid progression in autosomal dominant polycystic kidney disease and special considerations for disease-modifying therapy. Am. J. Kidney Dis. 78 (2), 282–292. doi:10.1053/j.ajkd.2020.12.020
Chiaravalli, M., Rowe, I., Mannella, V., Quilici, G., Canu, T., Bianchi, V., et al. (2016). 2-Deoxy-d-Glucose ameliorates PKD progression. J. Am. Soc. Nephrol. 27 (7), 1958–1969. doi:10.1681/ASN.2015030231
Cholesterol Treatment Trialists' Collaboration (2022). Effect of statin therapy on muscle symptoms: An individual participant data meta-analysis of large-scale, randomised, double-blind trials. Lancet 400 (10355), 832–845. doi:10.1016/S0140-6736(22)01545-8
Chow, L. C., and Ong, A. C. (2009). Autosomal dominant polycystic kidney disease. Clin. Med. 9 (3), 278–283. doi:10.7861/clinmedicine.9-3-278
Day, J. W., Gelfanov, V., Smiley, D., Carrington, P. E., Eiermann, G., Chicchi, G., et al. (2012). Optimization of co-agonism at GLP-1 and glucagon receptors to safely maximize weight reduction in DIO-rodents. Biopolymers 98 (5), 443–450. doi:10.1002/bip.22072
DeFronzo, R. A., Reeves, W. B., and Awad, A. S. (2021). Pathophysiology of diabetic kidney disease: Impact of SGLT2 inhibitors. Nat. Rev. Nephrol. 17 (5), 319–334. doi:10.1038/s41581-021-00393-8
Di Mise, A., Tamma, G., Ranieri, M., Centrone, M., van den Heuvel, L., Mekahli, D., et al. (2018). Activation of Calcium-Sensing Receptor increases intracellular calcium and decreases cAMP and mTOR in PKD1 deficient cells. Sci. Rep. 8 (1), 5704. doi:10.1038/s41598-018-23732-5
Di Mise, A., Wang, X., Ye, H., Pellegrini, L., Torres, V. E., and Valenti, G. (2021). Pre-clinical evaluation of dual targeting of the GPCRs CaSR and V2R as therapeutic strategy for autosomal dominant polycystic kidney disease. FASEB J. 35 (10), e21874. doi:10.1096/fj.202100774R
Distefano, G., Boca, M., Rowe, I., Wodarczyk, C., Ma, L., Piontek, K. B., et al. (2009). Polycystin-1 regulates extracellular signal-regulated kinase-dependent phosphorylation of tuberin to control cell size through mTOR and its downstream effectors S6K and 4EBP1. Mol. Cell. Biol. 29 (9), 2359–2371. doi:10.1128/MCB.01259-08
Fassett, R. G., Coombes, J. S., Packham, D., Fairley, K. F., and Kincaid-Smith, P. (2010). Effect of pravastatin on kidney function and urinary protein excretion in autosomal dominant polycystic kidney disease. Scand. J. Urology Nephrol. 44 (1), 56–61. doi:10.3109/00365590903359908
Fick-Brosnahan, G. M., Belz, M. M., McFann, K. K., Johnson, A. M., and Schrier, R. W. (2002). Relationship between renal volume growth and renal function in autosomal dominant polycystic kidney disease: A longitudinal study. Am. J. Kidney Dis. 39 (6), 1127–1134. doi:10.1053/ajkd.2002.33379
Flaig, S. M., Gattone, V. H., and Blazer-Yost, B. L. (2016). Inhibition of cyst growth in PCK and Wpk rat models of polycystic kidney disease with low doses of peroxisome proliferator-activated receptor γ agonists. J. Transl. Int. Med. 4 (3), 118–126. doi:10.1515/jtim-2016-0028
Frías, J. P., Davies, M. J., Rosenstock, J., Pérez Manghi, F. C., Fernández Landó, L., Bergman, B. K., et al. (2021). Tirzepatide versus semaglutide once weekly in patients with type 2 diabetes. N. Engl. J. Med. 385 (6), 503–515. doi:10.1056/NEJMoa2107519
Gabow, P. A. (1990). Autosomal dominant polycystic kidney disease--more than a renal disease. Am. J. Kidney Dis. 16 (5), 403–413. doi:10.1016/s0272-6386(12)80051-5
Gimpel, C., Bergmann, C., Bockenhauer, D., Breysem, L., Cadnapaphornchai, M. A., Cetiner, M., et al. (2019). International consensus statement on the diagnosis and management of autosomal dominant polycystic kidney disease in children and young people. Nat. Rev. Nephrol. 15 (11), 713–726. doi:10.1038/s41581-019-0155-2
Griffiths, J., Mills, M. T., and Ong, A. C. (2020). Long-acting somatostatin analogue treatments in autosomal dominant polycystic kidney disease and polycystic liver disease: A systematic review and meta-analysis. B.M.J. Open. 10 (1), e032620. doi:10.1136/bmjopen-2019-032620
Gwinn, D. M., Shackelford, D. B., Egan, D. F., Mihaylova, M. M., Mery, A., Vasquez, D. S., et al. (2008). AMPK phosphorylation of raptor mediates a metabolic checkpoint. Mol. Cell. 30 (2), 214–226. doi:10.1016/j.molcel.2008.03.003
Hall, K. D., Chen, K. Y., Guo, J., Lam, Y. Y., Leibel, R. L., Mayer, L. E., et al. (2016). Energy expenditure and body composition changes after an isocaloric ketogenic diet in overweight and obese men. Am. J. Clin. Nutr. 104 (2), 324–333. doi:10.3945/ajcn.116.133561
Hallows, K. R., Li, H., Saitta, B., Sepehr, S., Huang, P., Pham, J., et al. (2022). Beneficial effects of bempedoic acid treatment in polycystic kidney disease cells and mice. Front. Mol. Biosci. 9, 1001941. doi:10.3389/fmolb.2022.1001941
Harris, P. C., and Torres, V. E. (2009). Polycystic kidney disease. Annu. Rev. Med. 60, 321–337. doi:10.1146/annurev.med.60.101707.125712
Hopp, K., Catenacci, V. A., Dwivedi, N., Kline, T. L., Wang, W., You, Z., et al. (2021). Weight loss and cystic disease progression in autosomal dominant polycystic kidney disease. iScience 25 (1), 103697. doi:10.1016/j.isci.2021.103697
Huynh, K. (2019). Targeting ATP citrate lyase to reduce LDL cholesterol. Nat. Rev. Cardiol. 16 (7), 385. doi:10.1038/s41569-019-0201-6
Inoki, K., Zhu, T., and Guan, K. L. (2003). TSC2 mediates cellular energy response to control cell growth and survival. Cell 115 (5), 577–590. doi:10.1016/s0092-8674(03)00929-2
Kapoor, S., Rodriguez, D., Riwanto, M., Edenhofer, I., Segerer, S., Mitchell, K., et al. (2015). Effect of sodium-glucose cotransport inhibition on polycystic kidney disease progression in PCK rats. PLoS One 10 (4), e0125603. doi:10.1371/journal.pone.0125603
Kipp, K. R., Rezaei, M., Lin, L., Dewey, E. C., and Weimbs, T. (2016). A mild reduction of food intake slows disease progression in an orthologous mouse model of polycystic kidney disease. Am. J. Physiol. Ren. Physiol. 310 (8), F726–F731. doi:10.1152/ajprenal.00551.2015
Knol, M. G. E., Kramers, B. J., Gansevoort, R. T., and van Gastel, M. D. A. (2021). The association of glucagon with disease severity and progression in patients with autosomal dominant polycystic kidney disease: An observational cohort study. Clin. Kidney J. 14 (12), 2582–2590. doi:10.1093/ckj/sfab112
Koppenol, W., Bounds, P., and Dang, C. (2011). Otto Warburg's contributions to current concepts of cancer metabolism. Nat. Rev. Cancer. 11, 325–337. doi:10.1038/nrc3038
Körner, M., Stöckli, M., Waser, B., and Reubi, J. C. (2007). GLP-1 receptor expression in human tumors and human normal tissues: Potential for in vivo targeting. J. Nucl. Med. 48 (5), 736–743. doi:10.2967/jnumed.106.038679
Kou, R., Shiroto, T., Sartoretto, J. L., and Michel, T. (2012). Suppression of Gαs synthesis by simvastatin treatment of vascular endothelial cells. J. Biol. Chem. 287 (4), 2643–2651. doi:10.1074/jbc.M111.303594
Lakhia, R., Ramalingam, H., Chang, C. M., Cobo-Stark, P., Biggers, L., Flaten, A., et al. (2022). PKD1 and PKD2 mRNA cis-inhibition drives polycystic kidney disease progression. Nat. Commun. 13 (1), 4765. doi:10.1038/s41467-022-32543-2
Lakhia, R., Yheskel, M., Flaten, A., Quittner-Strom, E. B., Holland, W. L., and Patel, V. (2018). PPARα agonist fenofibrate enhances fatty acid β-oxidation and attenuates polycystic kidney and liver disease in mice. Am. J. Physiol. Ren. Physiol. 314 (1), F122–F131. doi:10.1152/ajprenal.00352.2017
Lebovitz, H. E. (2019). Thiazolidinediones: The forgotten diabetes medications. Curr. Diab. Rep. 19 (12), 151. doi:10.1007/s11892-019-1270-y
Lee, E. C., Valencia, T., Allerson, C., Schairer, A., Flaten, A., Yheskel, M., et al. (2019). Discovery and preclinical evaluation of anti-miR-17 oligonucleotide RGLS4326 for the treatment of polycystic kidney disease. Nat. Commun. 10 (1), 4148. doi:10.1038/s41467-019-11918-y
Lee, J. H., Jang, H., Lee, S. M., Lee, J. E., Choi, J., Kim, T. W., et al. (2015). ATP-citrate lyase regulates cellular senescence via an AMPK- and p53-dependent pathway. Febs. J. 282 (2), 361–371. doi:10.1111/febs.13139
Leonhard, W. N., Song, X., Kanhai, A. A., Iliuta, I. A., Bozovic, A., Steinberg, G. R., et al. (2019). Salsalate, but not metformin or canagliflozin, slows kidney cyst growth in an adult-onset mouse model of polycystic kidney disease. EBioMedicine 47, 436–445. doi:10.1016/j.ebiom.2019.08.041
Lian, X., Wu, X., Li, Z., Zhang, Y., Song, K., Cai, G., et al. (2019). The combination of metformin and 2-deoxyglucose significantly inhibits cyst formation in miniature pigs with polycystic kidney disease. Br. J. Pharmacol. 176 (5), 711–724. doi:10.1111/bph.14558
Lin, C. H., Chao, C. T., Wu, M. Y., Lo, W. C., Lin, T. C., and Wu, M. S. (2019). Use of mammalian target of rapamycin inhibitors in patient with autosomal dominant polycystic kidney disease: An updated meta-analysis. Int. Urol. Nephrol. 51 (11), 2015–2025. doi:10.1007/s11255-019-02292-1
Margaria, J. P., Campa, C. C., De Santis, M. C., Hirsch, E., and Franco, I. (2020). The PI3K/Akt/mTOR pathway in polycystic kidney disease: A complex interaction with polycystins and primary cilium. Cell Signal 66, 109468. doi:10.1016/j.cellsig.2019.109468
Meijer, E., Visser, F. W., van Aerts, R. M. M., Blijdorp, C. J., Casteleijn, N. F., D'Agnolo, H. M. A., et al. (2018). Effect of Lanreotide on kidney function in patients with autosomal dominant polycystic kidney disease: The DIPAK 1 randomized clinical trial. JAMA 320 (19), 2010–2019. doi:10.1001/jama.2018.15870
Mohr, A. M., and Mott, J. L. (2015). Overview of microRNA biology. Semin. Liver Dis. 35 (1), 3–11. doi:10.1055/s-0034-1397344
Müller, R. U., Messchendorp, A. L., Birn, H., Capasso, G., Cornec-Le Gall, E., Devuyst, O., et al. (2022). An update on the use of tolvaptan for autosomal dominant polycystic kidney disease: Consensus statement on behalf of the ERA working group on inherited kidney disorders, the European rare kidney disease reference network and polycystic kidney disease international. Nephrol. Dial. Transpl. 37 (5), 825–839. doi:10.1093/ndt/gfab312
Müller, T. D., Finan, B., Bloom, S. R., D'Alessio, D., Drucker, D. J., Flatt, P. R., et al. (2019). Glucagon-like peptide 1 (GLP-1). Mol. Metab. 30, 72–130. doi:10.1016/j.molmet.2019.09.010
Mutig, K., Paliege, A., Kahl, T., Jons, T., Muller-Esterl, W., and Bachmann, S. (2007). Vasopressin V2 receptor expression along rat, mouse, and human renal epithelia with focus on TAL. Am. J. Physiol. Ren. Physiol. 293 (4), F1166–F1177. doi:10.1152/ajprenal.00196.2007
Myers, R. W., Guan, H. P., Ehrhart, J., Petrov, A., Prahalada, S., Tozzo, E., et al. (2017). Systemic pan-AMPK activator MK-8722 improves glucose homeostasis but induces cardiac hypertrophy. Science 357 (6350), 507–511. doi:10.1126/science.aah5582
Natoli, T. A., Smith, L. A., Rogers, K. A., Wang, B., Komarnitsky, S., Budman, Y., et al. (2010). Inhibition of glucosylceramide accumulation results in effective blockade of polycystic kidney disease in mouse models. Nat. Med. 16 (7), 788–792. doi:10.1038/nm.2171
Nauli, S. M., Alenghat, F. J., Luo, Y., Williams, E., Vassilev, P., Li, X., et al. (2003). Polycystins 1 and 2 mediate mechanosensation in the primary cilium of kidney cells. Nat. Genet. 33 (2), 129–137. doi:10.1038/ng1076
Newsome, P. N., Buchholtz, K., Cusi, K., Linder, M., Okanoue, T., Ratziu, V., et al. (2021). A placebo-controlled trial of subcutaneous semaglutide in nonalcoholic steatohepatitis. N. Engl. J. Med. 384 (12), 1113–1124. doi:10.1056/NEJMoa2028395
Nowak, K. L., Steele, C., Gitomer, B., Wang, W., Ouyang, J., and Chonchol, M. B. (2021). Overweight and obesity and progression of ADPKD. Clin. J. Am. Soc. Nephrol. 16 (6), 908–915. doi:10.2215/CJN.16871020
Nowak, K. L., You, Z., Gitomer, B., Brosnahan, G., Torres, V. E., Chapman, A. B., et al. (2018). Overweight and obesity are predictors of Pro.gression in early autosomal dominant polycystic kidney disease. J. Am. Soc. Nephrol. 29 (2), 571–578. doi:10.1681/ASN.2017070819
Oehm, S., Konstantin, S., Arjune, S., Todorova, P., Lindemann, C., Woestmann, F., et al. (2022a). MO016: Feasibility and effectiveness of short-term ketogenic interventions in autosomal dominant polycystic kidney disease (ADPKD): Results from the reset-pkd study. Nephrol. Dial. Transpl. 37, 3. gfac061.011. doi:10.1093/ndt/gfac061.011
Oehm, S., Steinke, K., Schmidt, J., Arjune, S., Todorova, P., Lindemann, C. H., et al. (2022b). RESET-PKD: A pilot trial on short-term ketogenic interventions in autosomal dominant polycystic kidney disease. Nephrol. Dial. Transplant 0, 1–13. doi:10.1093/ndt/gfac311
Ong, A. C. M., and Gansevoort, R. T. (2021). TAMEing ADPKD with metformin: Safe and effective? Kidney Int. 100 (3), 513–515. doi:10.1016/j.kint.2021.07.021
Ong, A. C. M., and Torra, R. (2022). Can ketogenic dietary interventions slow disease progression in ADPKD: What we know and what we don't. Clin. Kidney J. 15 (6), 1034–1036. doi:10.1093/ckj/sfac103
Padhy, B., Xie, J., Wang, R., Lin, F., and Huang, C. L. (2022). Channel function of polycystin-2 in the endoplasmic reticulum protects against autosomal dominant polycystic kidney disease. J. Am. Soc. Nephrol. 33 (8), 1501–1516. doi:10.1681/ASN.2022010053
Padovano, V., Podrini, C., Boletta, A., and Caplan, M. J. (2018). Metabolism and mitochondria in polycystic kidney disease research and therapy. Nat. Rev. Nephrol. 14 (11), 678–687. doi:10.1038/s41581-018-0051-1
Pastor-Soler, N. M., Li, H., Pham, J., Rivera, D., Ho, P. Y., Mancino, V., et al. (2022). Metformin improves relevant disease parameters in an autosomal dominant polycystic kidney disease mouse model. Am. J. Physiol. Ren. Physiol. 322 (1), F27–F41. doi:10.1152/ajprenal.00298.2021
Pema, M., Drusian, L., Chiaravalli, M., Castelli, M., Yao, Q., Ricciardi, S., et al. (2016). mTORC1-mediated inhibition of polycystin-1 expression drives renal cyst formation in tuberous sclerosis complex. Nat. Commun. 7, 10786. doi:10.1038/ncomms10786
Perico, N., Ruggenenti, P., Perna, A., Caroli, A., Trillini, M., Sironi, S., et al. (2019). Octreotide-LAR in later-stage autosomal dominant polycystic kidney disease (aladin 2): A randomized, double-blind, placebo-controlled, multicenter trial. PLoS Med. 16 (4), e1002777. doi:10.1371/journal.pmed.1002777
Perrone, R. D., Abebe, K. Z., Watnick, T. J., Althouse, A. D., Hallows, K. R., Lalama, C. M., et al. (2021). Primary results of the randomized trial of metformin administration in polycystic kidney disease (TAME PKD). Kidney Int. 100 (3), 684–696. doi:10.1016/j.kint.2021.06.013
Perrone, R. D., Malek, A. M., and Watnick, T. (2015). Vascular complications in autosomal dominant polycystic kidney disease. Nat. Rev. Nephrol. 11 (10), 589–598. doi:10.1038/nrneph.2015.128
Pinkosky, S. L., Newton, R. S., Day, E. A., Ford, R. J., Lhotak, S., Austin, R. C., et al. (2016). Liver-specific ATP-citrate lyase inhibition by bempedoic acid decreases LDL-C and attenuates atherosclerosis. Nat. Commun. 7, 13457. doi:10.1038/ncomms13457
Pinto, C. S., Raman, A., Reif, G. A., Magenheimer, B. S., White, C., Calvet, J. P., et al. (2016). Phosphodiesterase isoform regulation of cell proliferation and fluid secretion in autosomal dominant polycystic kidney disease. J. Am. Soc. Nephrol. 27 (4), 1124–1134. doi:10.1681/ASN.2015010047
Pocai, A., Carrington, P. E., Adams, J. R., Wright, M., Eiermann, G., Zhu, L., et al. (2009). Glucagon-like peptide 1/glucagon receptor dual agonism reverses obesity in mice. Diabetes 58 (10), 2258–2266. doi:10.2337/db09-0278
Pocai, A. (2012). Unraveling oxyntomodulin, GLP1's enigmatic brother. J. Endocrinol. 215 (3), 335–346. doi:10.1530/JOE-12-0368
Podrini, C., Cassina, L., and Boletta, A. (2020). Metabolic reprogramming and the role of mitochondria in polycystic kidney disease. Cell Signal 67, 109495. doi:10.1016/j.cellsig.2019.109495
Quiroga, B., and Torra, R. (2022). Dietary aspects and drug-related side effects in autosomal dominant polycystic kidney disease progression. Nutrients 14 (21), 4651. doi:10.3390/nu14214651
Ray, K. K., Bays, H. E., Catapano, A. L., Lalwani, N. D., Bloedon, L. T., Sterling, L. R., et al. (2019). Safety and efficacy of bempedoic acid to reduce LDL cholesterol. N. Engl. J. Med. 380 (11), 1022–1032. doi:10.1056/NEJMoa1803917
Riwanto, M., Kapoor, S., Rodriguez, D., Edenhofer, I., Segerer, S., and Wüthrich, R. P. (2016). Inhibition of aerobic glycolysis attenuates disease progression in polycystic kidney disease. PLoS One 11 (1), e0146654. doi:10.1371/journal.pone.0146654
Rodriguez, D., Kapoor, S., Edenhofer, I., Segerer, S., Riwanto, M., Kipar, A., et al. (2015). Inhibition of sodium-glucose cotransporter 2 with dapagliflozin in han: SPRD rats with polycystic kidney disease. Kidney Blood Press. Res. 40 (6), 638–647. doi:10.1159/000368540
Rowe, I., Chiaravalli, M., Mannella, V., Ulisse, V., Quilici, G., Pema, M., et al. (2013). Defective glucose metabolism in polycystic kidney disease identifies a new therapeutic strategy. Nat. Med. 19 (4), 488–493. doi:10.1038/nm.3092
Ruggenenti, P., Perna, A., Caroli, A., Perico, N., Trillini, M., Pisani, A., et al. (2022). Octreotide lar in patients with autosomal dominant polycystic kidney disease: From bench to a novel perspective of therapy. Med. Res. Arch. 10 (11). doi:10.18103/mra.v10i12.3232
Ruscica, M., Sirtori, C. R., Carugo, S., Banach, M., and Corsini, A. (2022). Bempedoic acid: For whom and when. Curr. Atheroscler. Rep. 24 (10), 791–801. doi:10.1007/s11883-022-01054-2
Saponaro, C., Pattou, F., and Bonner, C. (2018). SGLT2 inhibition and glucagon secretion in humans. Diabetes Metab. 44 (5), 383–385. doi:10.1016/j.diabet.2018.06.005
Seliger, S. L., Watnick, T., Althouse, A. D., Perrone, R. D., Abebe, K. Z., Hallows, K. R., et al. (2020). Baseline characteristics and patient-reported outcomes of ADPKD patients in the multicenter TAME-PKD clinical trial. Kidney360. 1 (12), 1363–1372. doi:10.34067/KID.0004002020
Soomro, I., Sun, Y., Li, Z., Diggs, L., Hatzivassiliou, G., Thomas, A. G., et al. (2020). Glutamine metabolism via glutaminase 1 in autosomal-dominant polycystic kidney disease. Nephrol. Dial. Transpl. 35 (10), 1824. doi:10.1093/ndt/gfz109
Sparapani, S., Millet-Boureima, C., Oliver, J., Mu, K., Hadavi, P., Kalostian, T., et al. (2021). The biology of vasopressin. Biomedicines 9 (1), 89. doi:10.3390/biomedicines9010089
Steinberg, G. R., and Hardie, D. G. (2022). New insights into activation and function of the AMPK. Nat. Rev. Mol. Cell. Biol. doi:10.1038/s41580-022-00547-x
Strubl, S., Oehm, S., Torres, J. A., Grundmann, F., Haratani, J., Decker, M., et al. (2021). Ketogenic dietary interventions in autosomal dominant polycystic kidney disease-a retrospective case series study: First insights into feasibility, safety and effects. Clin. Kidney J. 15 (6), 1079–1092. doi:10.1093/ckj/sfab162
Su, L., Yuan, H., Zhang, H., Wang, R., Fu, K., Yin, L., et al. (2022). PF-06409577 inhibits renal cyst progression by concurrently inhibiting the mTOR pathway and CFTR channel activity. FEBS Open Bio 12 (10), 1761–1770. doi:10.1002/2211-5463.13459
Sullivan, L. P., Wallace, D. P., and Grantham, J. J. (1998). Chloride and fluid secretion in polycystic kidney disease. J. Am. Soc. Nephrol. 9 (5), 903–916. doi:10.1681/ASN.V95903
Sun, W., Lee, T. S., Zhu, M., Gu, C., Wang, Y., Zhu, Y., et al. (2006). Statins activate AMP-activated protein kinase in vitro and in vivo. Circulation 114 (24), 2655–2662. doi:10.1161/CIRCULATIONAHA.106.630194
Suwabe, T., Barrera, F. J., Rodriguez-Gutierrez, R., Ubara, Y., and Hogan, M. C. (2021). Somatostatin analog therapy effectiveness on the progression of polycystic kidney and liver disease: A systematic review and meta-analysis of randomized clinical trials. PLoS One 16 (9), e0257606. doi:10.1371/journal.pone.0257606
Takiar, V., and Caplan, M. J. (2011). Polycystic kidney disease: Pathogenesis and potential therapies. Biochim. Biophys. Acta. 1812 (10), 1337–1343. doi:10.1016/j.bbadis.2010.11.014
Takiar, V., Nishio, S., Seo-Mayer, P., King, J. D., Li, H., Zhang, L., et al. (2011). Activating AMP-activated protein kinase (AMPK) slows renal cystogenesis. Proc. Natl. Acad. Sci. U. S. A. 108 (6), 2462–2467. doi:10.1073/pnas.1011498108
Testa, F., and Magistroni, R. (2020). ADPKD current management and ongoing trials. J. Nephrol. 33 (2), 223–237. doi:10.1007/s40620-019-00679-y
Torres, J. A., Kruger, S. L., Broderick, C., Amarlkhagva, T., Agrawal, S., Dodam, J. R., et al. (2019a). Ketosis ameliorates renal cyst growth in polycystic kidney disease. Cell. Metab. 30 (6), 1007–1023.e5. doi:10.1016/j.cmet.2019.09.012
Torres, J. A., Rezaei, M., Broderick, C., Lin, L., Wang, X., Hoppe, B., et al. (2019b). Crystal deposition triggers tubule dilation that accelerates cystogenesis in polycystic kidney disease. J. Clin. Invest. 129 (10), 4506–4522. doi:10.1172/JCI128503
Torres, V. E., Chapman, A. B., Devuyst, O., Gansevoort, R. T., Perrone, R. D., Koch, G., et al. (2017). Tolvaptan in later-stage autosomal dominant polycystic kidney disease. N. Engl. J. Med. 377 (20), 1930–1942. doi:10.1056/NEJMoa1710030
Torres, V. E., Harris, P. C., and Pirson, Y. (2007). Autosomal dominant polycystic kidney disease. Lancet 369 (9569), 1287–1301. doi:10.1016/S0140-6736(07)60601-1
van Dijk, M. A., Kamper, A. M., van Veen, S., Souverijn, J. H., and Blauw, G. J. (2001). Effect of simvastatin on renal function in autosomal dominant polycystic kidney disease. Nephrol. Dial. Transpl. 16 (11), 2152–2157. doi:10.1093/ndt/16.11.2152
Warner, G., Hein, K. Z., Nin, V., Edwards, M., Chini, C. C., Hopp, K., et al. (2016). Food restriction ameliorates the development of polycystic kidney disease. J. Am. Soc. Nephrol. 27 (5), 1437–1447. doi:10.1681/ASN.2015020132
Welles, J. E., Dennis, M. D., Jefferson, L. S., and Kimball, S. R. (2020). Glucagon-Dependent suppression of mTORC1 is associated with upregulation of hepatic FGF21 mRNA translation. Am. J. Physiol. Endocrinol. Metab. 319 (1), E26–E33. doi:10.1152/ajpendo.00555.2019
Xu, H., Li, Q., Liu, J., Zhu, J., Li, L., Wang, Z., et al. (2018). β-Arrestin-1 deficiency ameliorates renal interstitial fibrosis by blocking Wnt1/β-catenin signaling in mice. J. Mol. Med. 96 (1), 97–109. doi:10.1007/s00109-017-1606-5
Xue, C., Zhang, L. M., Zhou, C., Mei, C. L., and Yu, S. Q. (2020). Effect of statins on renal function and total kidney volume in autosomal dominant polycystic kidney disease. Kidney Dis. (Basel) 6 (6), 407–413. doi:10.1159/000509087
Yamaguchi, T., Nagao, S., Wallace, D. P., Belibi, F. A., Cowley, B. D., Pelling, J. C., et al. (2003). Cyclic AMP activates B-Raf and ERK in cyst epithelial cells from autosomal-dominant polycystic kidneys. Kidney Int. 63 (6), 1983–1994. doi:10.1046/j.1523-1755.2003.00023.x
Keywords: ADPKD (autosomal dominant polycystic kidney disease), tolvaptan, metabolic reprograming, therapeutic approaches, metabolism & obesity, GLP-1, glucagon
Citation: Bakaj I and Pocai A (2023) Metabolism-based approaches for autosomal dominant polycystic kidney disease. Front. Mol. Biosci. 10:1126055. doi: 10.3389/fmolb.2023.1126055
Received: 17 December 2022; Accepted: 06 February 2023;
Published: 16 February 2023.
Edited by:
Annarita Di Mise, University of Bari Aldo Moro, ItalyReviewed by:
Nuria M. Pastor-Soler, University of Southern California, United StatesCopyright © 2023 Bakaj and Pocai. This is an open-access article distributed under the terms of the Creative Commons Attribution License (CC BY). The use, distribution or reproduction in other forums is permitted, provided the original author(s) and the copyright owner(s) are credited and that the original publication in this journal is cited, in accordance with accepted academic practice. No use, distribution or reproduction is permitted which does not comply with these terms.
*Correspondence: Alessandro Pocai, QWxlc3NhbmRyb19wb2NhaUB5YWhvby5jb20=
Disclaimer: All claims expressed in this article are solely those of the authors and do not necessarily represent those of their affiliated organizations, or those of the publisher, the editors and the reviewers. Any product that may be evaluated in this article or claim that may be made by its manufacturer is not guaranteed or endorsed by the publisher.
Research integrity at Frontiers
Learn more about the work of our research integrity team to safeguard the quality of each article we publish.