- 1Experimental and Translational Immunology Group, Intercollegiate Faculty of Biotechnology of University of Gdansk and Medical University of Gdansk, University of Gdansk, Gdansk, Poland
- 2Laboratory of Protein Metabolism, International Institute of Molecular and Cell Biology in Warsaw, Warsaw, Poland
- 3Centre for Genomic and Experimental Medicine, Institute of Genetics and Cancer, University of Edinburgh, Edinburgh, United Kingdom
Background: Loss of function mutation in FLG is the major genetic risk factor for atopic dermatitis (AD) and other allergic manifestations. Presently, little is known about the cellular turnover and stability of profilaggrin, the protein encoded by FLG. Since ubiquitination directly regulates the cellular fate of numerous proteins, their degradation and trafficking, this process could influence the concentration of filaggrin in the skin.
Objective: To determine the elements mediating the interaction of profilaggrin with the ubiquitin-proteasome system (i.e., degron motifs and ubiquitination sites), the features responsible for its stability, and the effect of nonsense and frameshift mutations on profilaggrin turnover.
Methods: The effect of inhibition of proteasome and deubiquitinases on the level and modifications of profilaggrin and processed products was assessed by immunoblotting. Wild-type profilaggrin sequence and its mutated variants were analysed in silico using the DEGRONOPEDIA and Clustal Omega tool.
Results: Inhibition of proteasome and deubiquitinases stabilizes profilaggrin and its high molecular weight of presumably ubiquitinated derivatives. In silico analysis of the sequence determined that profilaggrin contains 18 known degron motifs as well as multiple canonical and non-canonical ubiquitination-prone residues. FLG mutations generate products with increased stability scores, altered usage of the ubiquitination marks, and the frequent appearance of novel degrons, including those promoting C-terminus-mediated degradation routes.
Conclusion: The proteasome is involved in the turnover of profilaggrin, which contains multiple degrons and ubiquitination-prone residues. FLG mutations alter those key elements, affecting the degradation routes and the mutated products’ stability.
Introduction
Atopic dermatitis (AD) is a disease characterized by chronically relapsing-remitting skin inflammation. The etiology of AD is multifactorial, involving gene-environment interaction with strong hereditability (80%) (Larsen, 1993). Recent studies show that epidermal barrier dysfunction is a central feature in the pathogenesis of AD (Cork et al., 2009; Luger et al., 2021). Genetic studies point towards a region that harbours several genes involved in epidermal barrier maintenance (Cookson et al., 2001; Paternoster et al., 2011), known as the “epidermal differentiation complex” (EDC) (Mischke et al., 1996), spanning 1.9 Mbp within chromosome 1q21 and known to be prone to chromosomal rearrangement (Forus et al., 1998; Itoyama et al., 2002; Chen et al., 2003; Wong et al., 2003). The genes of the EDC can be grouped into S100 calcium binding proteins (Eckert et al., 2004; Marenholz et al., 2004), S100 fused-type protein (SFTP) family (Gan et al., 1990; Lee et al., 1993; Krieg et al., 1997; Contzler et al., 2005; Takaishi et al., 2005; Wu et al., 2011; Kypriotou et al., 2012), cornified envelope precursor family (Backendorf and Hohl, 1992), and small proline-rich proteins (Zhao and Elder, 1997; Marshall et al., 2001; Jackson et al., 2005). Among the 63 genes (59 protein coding genes and four pseudogenes) located within the EDC, null mutations in the gene encoding profilaggrin (FLG), an SFTP gene, was shown to be the major risk factor for AD (Morar et al., 2007).
The first suggestion of the involvement of filaggrin in barrier maintenance was reported by Sybert et al. (Sybert et al., 1985) where reduction of its expression was correlated with ichthyosis vulgaris (IV); follow-up studies confirmed reduction or loss of filaggrin expression with epidermal barrier dysfunction (Fleckman et al., 1987; Peña Penabad et al., 1998). A breakthrough came with the discovery that loss of function FLG mutations (R501X and 2282del4) are highly prevalent in the IV (Smith et al., 2006) and AD patients (Palmer et al., 2006); this was replicated on different genetic backgrounds and ethnicities (Marenholz et al., 2006; Ruether et al., 2006; Sandilands et al., 2006; Weidinger et al., 2006; Barker et al., 2007; Morar et al., 2007; Nomura et al., 2007; Enomoto et al., 2008; Rodríguez et al., 2008; Nemoto-Hasebe et al., 2009; Osawa et al., 2010; Cheng et al., 2012; Pigors et al., 2018; Handa et al., 2019; Koseki et al., 2019; Jurakic Toncic et al., 2020; Smieszek et al., 2020), with hundreds of mutations now identified (Karczewski et al., 2020). Importantly, while filaggrin expression is almost entirely restricted to the epidermis, FLG mutations have been also shown to be linked to additional manifestations of atopic march and allergy, including food (Brown et al., 2011) and contact allergies (Novak et al., 2008), asthma (van den Oord and Sheikh, 2009; Rodríguez et al., 2009), allergic rhinitis (van den Oord and Sheikh, 2009) and eosinophilic esophagitis (Sherrill and Blanchard, 2014).
Composed of approximately 4,061 amino acids (aa), profilaggrin is the largest protein of the SFTP family; the protein is structurally complex and composed of 10–12 filaggrin monomer repeats flanked with truncated filaggrin repeats (Gan et al., 1990) and a S100 domain at the N-terminus (Kypriotou et al., 2012). Unlike any other SFTP, profilaggrin contains a “bipartite” nuclear localization signal (Lu et al., 2021) next to the S100 calcium-binding domain, an indicator of its nuclear function (Presland et al., 1992; Aho et al., 2012). Upon expression in keratinocytes, profilaggrin is phosphorylated and stored within keratohyalin granules (KHGs), mainly present in the stratum granulosum layer (Resing et al., 2002), from where it is released by an AKT1-dependent, actin scaffold-driven mechanism that we have recently described (Gutowska-Owsiak et al., 2018). It is speculated that dephosphorylation makes the protein accessible to the pro-protein convertase-mediated cleavage (Resing et al., 1993). The cleaved N-terminal domain translocates into the nucleus, where it is involved in denucleation (Ishida-Yamamoto et al., 1998; Pearton et al., 2002; Yamamoto-Tanaka et al., 2014) and control of epidermal homeostasis (Aho et al., 2012; Naeem et al., 2015); the remaining part is cleaved by SASPase (Matsui et al., 2011) and KLK5 (Sakabe et al., 2013) proteolytic enzymes. Monomeric filaggrin promotes aggregation and collapse of keratin intermediate filaments (IFs), resulting in the formation of more squamous flattened cells (Steinert et al., 1981; Candi et al., 2005). In parallel, further events occur, such as the conversion of arginine (Arg) residues to citrulline (Nachat et al., 2005) and covalent cross-linking of monomers by transglutaminase, which leads to stabilization of the cornified cell envelope (Takahashi et al., 1996). Finally, the crosslinked filaggrin undergoes extensive proteolytic cleavage by caspase-14 (Denecker et al., 2007), calpain-1 (Yamazaki et al., 1997), bleomycin hydrolase (Kamata et al., 2009), elastase-2 (Bonnart et al., 2010), matripase (List et al., 2003), prostatin (Leyvraz et al., 2005) and other proteases, resulting in a pool of hygroscopic aa and derivatives, i.e., urocanic acid (UCA), a derivative of histidine, highly abundant in the protein, and pyrrolidone carboxylic acid (PCA), a glutamine derivative. These constitute the majority of the so-called “natural moisturizing factor” (NMF) (Rawlings and Harding, 2004), contributing to stratum corneum (SC) hydration, as well as acidic pH, with antimicrobial action (Miajlovic et al., 2010). In addition, trans-UCA may protect cells in deeper layers from UVB-mediated mutagenesis by absorbing UVB (Tabachnick, 1957). FLG mutations significantly reduce the amount of NMF in SC compared to the healthy control (Kezic et al., 2011).
The ubiquitin-proteasome system (UPS) is a major proteolytic pathway that removes damaged and unwanted proteins. The selective turnover is initiated by a covalent attachment of a small ubiquitin (Ub) protein, mainly to the internal lysine (Lys) residues, which is mediated by an enzymatic cascade orchestrated by E1, E2, and E3 enzymes (Hershko and Ciechanover, 1992; Komander and Rape, 2012). However, a single ubiquitination event is usually insufficient to target a protein for degradation; several kinds of polyubiquitin chains are formed between the Lys residues of the Ub subunits and these have specific functions (Hershko and Ciechanover, 2003; Husnjak and Dikic, 2012; Oh et al., 2018). The Lys11 and Lys48 linkages, and their combination drive proteasomal degradation (Chau et al., 1989; Jin et al., 2008; Meyer and Rape, 2014), whereas Lys6 and Lys63 linkages mediate the processes of autophagy (Ordureau et al., 2014), endocytosis-exocytosis (Lauwers et al., 2009), or lysosomal degradation (Duncan et al., 2006). Interestingly, ubiquitination can also occur on the free amino group of the N-terminus of a protein, as well as on serine (Ser), cysteine (Cys), and threonine (Thr) residues, and can lead to proteasomal turnover of the modified proteins (Kravtsova-Ivantsiv and Ciechanover, 2012; McClellan et al., 2019).
E3 ligases, which transfer Ub onto a substrate protein, mainly recognize substrates through their short linear motif called the primary degron, which may be located at the unstructured ends of the proteins, inducing protein elimination via the N- or C-degron pathways or internally. In addition, proteolytic cleavage may lead to the emergence of a degron motif within the novel N- or C-terminus, subsequently initiating degradation of the cleavage products (Guharoy et al., 2016; Varshavsky, 2019; Guharoy et al., 2022). Moreover, post-translational modifications (PTMs) can also modulate the recognition of the primary degrons by E3 ligases (van Roey et al., 2013; Guharoy et al., 2016; Lucas and Ciulli, 2017; Millar et al., 2019; Varshavsky, 2019; Chen and Kashina, 2021; Guharoy et al., 2022). Following interaction with a degron, E3 mediates ubiquitination in the proximal position(s) (secondary degron), often found close to the intrinsically disordered region (IDR) (tertiary degron), which triggers substrate breakdown by successful activation of the proteasome. Mutations affecting degron components can enhance protein stability and disrupt cellular proteostasis, leading to disease (Tokheim et al., 2021; Guharoy et al., 2022; Kampmeyer et al., 2022). Accordingly, adequately controlled profilaggrin turnover is likely crucial for SC functionality since its reduced expression or instability confers vulnerability and impacts several epidermal functions, leading to pathological conditions (Scott et al., 1982; Resing et al., 1984; Moosbrugger-Martinz et al., 2022). However, despite the central role of profilaggrin and filaggrin in skin barrier function, little is known about the regulation of their degradation, the proteolytic mechanisms involved, and the influence of FLG mutations on their turnover and stability.
In this study, we investigated proteasome inhibition’s effect on the intracellular profilaggrin level and its processing products. We also studied the entire profilaggrin sequence for the presence of degrons and examined possible ubiquitination sites throughout. Furthermore, we analysed the effect of recurrent pathogenic and rare family-specific frameshift and nonsense FLG mutations on the introduction of possible ubiquitination sites, the appearance of novel degrons, and protein stability.
Materials and methods
Keratinocyte culture
Human keratinocyte cell line N/TERT1 (Dickson et al., 2000; Smits et al., 2017), a kind gift from J. Rheinwald laboratory (Harvard Medical School, Boston, United States), was cultured in T75 flask or T150 flask in Keratinocyte serum free medium (K-SFM), with L-glutamine, without CaCl2 (Gibco™, Thermo Fisher Scientific, Cat#10725018) and supplemented with 0.2 ng epidermal growth factor (EGF) per ml, 25 μg bovine pituitary extract (BPE) per ml (Gibco™, Thermo Fisher Scientific, Cat#13028014), 100 unit penicillin per ml (Sigma Aldrich, Cat#P4333), 100 μg streptomycin per ml (Sigma Aldrich, Cat#P4333) and 0.4 mM CaCl2 (VVR, Cat#97062822) up to 40% confluency. Cells were plated in 6-well plates at 300,000 cells per well in K-SFM complete medium and incubated at 37°C, 5% CO2 for 48 h.
Calcium switch, proteasome inhibition and deubiquitinase inhibition
After 48 h the medium was replaced with DFK medium composed of 1:1 of calcium-free Dulbecco’s Modified Eagle Medium (DMEM) containing 4.5 mg D-glucose per ml, and Ham’s F12 nutrient mix (Gibco™, Thermo Fisher Scientific, Cat#11765054), supplemented with 0.2 ng EGF per ml, 25 μg BPE per ml, 2 mM L-glutamate (Sigma Aldrich, Cat#G7513-100 ML), 100 unit penicillin, 100 μg streptomycin per ml and 1.5 mM CaCl2 (to trigger calcium induced differentiation; i.e., “calcium switch”). Upon differentiation (>24 h at 1.5 mM CaCl2) mediated by calcium switch, keratinocytes were treated with 10 μM MG132 (Sigma Aldrich, Cat#474790); a potent, reversible proteasome inhibitor or 10 µM PR-619 (Sigma Aldrich, Cat#SML0430-1 MG), a broad range deubiquitinase inhibitor; dissolved in DMSO for 2 h, 4 h, 8 h and 16 h. Protein extraction was performed after 48 h of calcium switch.
Western blot
The cells were lysed with RIPA buffer (Cell Signalling Technology, Cat#9806) supplemented with cOmplete™ protease inhibitor cocktail (Roche, Cat#11836170001). Cell lysates were harvested by centrifugation at 14,000 g for 15 min at 4°C and denatured with 4X Bolt™ LDS sample Buffer (Novex®, Life technology™, Cat#B0007) at 70°C for 10 min. Samples were run on 4%–12% polyacrylamide gradient gel (Invitrogen™, Thermo Fisher Scientific, Cat#NP0321BOX). Protein transfer was carried out onto a nitrocellulose membrane {[iBlot™ 2 Transfer Stacks (Invitrogen™, Thermo Fisher Scientific, Cat#IB23001)]} on the iBlot™ 2 dry blotting system (Invitrogen™, Thermo Fisher Scientific, Cat#IB21001). Membranes were blocked with 5% fat-free milk in phosphate buffer saline (PBS), followed by overnight incubation with 1:200 anti-filaggrin monoclonal antibody (FLG01; raised to recombinant filaggrin) (Invitrogen™, Thermo Fisher Scientific, Cat#MA513440; FLG01 monoclonal antibody was used in this study and was validated for its specificity (Supplementary Figure E1 or 1:5,000 dilution of anti-GAPDH antibody (6C5) (Santa Cruz Biotechnology, Cat#SC32233) at 4°C. Secondary antibody incubation was carried out with 1:25,000 PBS diluted IRDye®800CW donkey anti-mouse (LI-COR®, Cat#92632312) and imaged with Odyssey® CLx Imaging System (LI-COR® Biosciences). Membranes were stripped with the Restore™ fluorescence western blot stripping buffer (Thermo Scientific™, Cat#62300) according to the manufacturers’ instruction and stained with 1:1,000 dilution of anti-ubiquitin antibody (Santa Cruz Biotechnology, Cat#sc-8017) and detected as of the procedure described above. Acquired images were analysed with ImageJ (Schneider et al., 2012) (v.1.53f51) for protein quantification as a means of protein band intensity. The intensity of filaggrin protein bands was expressed as a band intensity ratio compared to the GAPDH band. One-way or two-way ANOVA (Tukey’s multiple comparison test) was performed with GraphPad Prism (v.9.4.1) to compare the variance between different treatment groups.
FLG sequence and AD-relevant mutations
The amino acid sequence of profilaggrin (UniProt ID P20930; NP_002007.1; herein referred as wild-type) was examined for the presence of Lys, Ser, Thr, and Cys residues as possible ubiquitination sites on individual domains. To examine if frameshift mutations alter the number of Lys, Ser, Thr, and Cys residues, all the mutations of FLG identified to date were retrieved from the Genome Aggregation Database (gnomAD, v2.1.1.) (accessed on 9.02.2022) (Karczewski et al., 2020). In addition, we cross-checked for additional frameshift mutations in the Gene4Denove (accessed 26.08.2022) (Zhao et al., 2020) and denovo-db v.1.6.1 (accessed 28.08.2022) (Seattle, 2022) databases. The mutations were introduced to the wild-type nucleotide sequence (NM_002016.2) and translated using the EMBL-EBI nucleotide sequence translation tool EMBOSS transeq (Madeira et al., 2022). Recurrent pathogenic mutations located within the coding sequence NM_002016.2: c.1 to c12183 and rare family-specific mutations located within the coding sequence NM_002016.2: c. 1 to c.5700 were used for the subsequent analyses.
Screening for degron motifs and stability of protein N- and C-termini
In all cases, we used our recently released tool DEGRONOPEDIA (Szulc et al., 2022) (all presented data in this work comply with the DEGRONOPEDIA’s version from 19.09.2022) to screen for the known degron motifs and post-translational modifications (PTMs), simulate proteolysis, calculate the Gravy hydrophobicity index (GHI) of terminal 15 residues (Kyte and Doolittle, 1982) and report experimental or predicted Protein Stability Index (PSI) values (Koren et al., 2018; Timms et al., 2019) for 23 residues at each of the N- and C-termini. Additionally, the DEGRONOPEDIA server provided experimentally validated E3s interacting with profilaggrin based on the BioGRID (Biological General Repository for Interaction Datasets) (Oughtred et al., 2021) and IntAct (Orchard et al., 2014) databases. Since sequences shorter than 50 aa are unsuitable input for the DEGRONOPEDIA, we excluded them from our FLG mutant variants analysis.
Analysis of amino acid sequence conservation
To investigate the conservation of profilaggrin ubiquitin-conjugating amino acids and the degron motifs within SFTPs and S100 proteins, we utilized EMBL-EMBI multiple sequence alignment tool Clustal Omega (Madeira et al., 2022).
Results
Inhibition of the proteasome and deubiquitinases results in the accumulation of profilaggrin and its processed products of high molecular weight
To determine if the intracellular levels of profilaggrin could be, at least partially, controlled by the proteasome-mediated turnover of the nascent protein, we used 2D-grown N/TERT-1 (Dickson et al., 2000; Smits et al., 2017) keratinocytes as our model because the expression of FLG mRNA and protein in these immortalized cells shown to be similar to that of primary human keratinocytes consisting WT FLG (Smits et al., 2017). Treatment with the proteasome inhibitor MG132 had no effect on cell morphology compared to the control (solvent control DMSO), with shorter treatment times and only a slight reduction in culture confluence (Supplementary Figure E1). In contrast, with the late time point (16 h), we noticed a reduction in cell viability and cells losing contact with the substratum. Proteasome inhibition increased the accumulation of ubiquitinated protein as expected (Figure 1A) and this increase was gradual in our time-point experiment, with the highest intensity measured after 16 h of 10 µM MG132 incubation (Figure 1B). In line with our expectations, proteasome inhibition altered the content of profilaggrin and profilaggrin-processed products; specifically, we observed an increase in the overall intensity of profilaggrin and filaggrin-relevant bands (adjusted to GAPDH) in the cells treated with MG132 (Figures 1C, D). This change was observed as an increase in high molecular weight products (Figure 1E) and was apparent after the overnight treatment. Specifically, we noticed an increase in the adjusted intensity of the bands corresponding to the products of 110–130 kDa (likely containing 3-4x filaggrin monomer repeats) and those of over 250 kDa in weight (likely including profilaggrin and a very high molecular weight processed product) (arrows in Figures 1C, E). This increase was pronounced despite harvesting fewer cells due to the reduction in cell viability at the 16 h time point. These results indicate that the proteasome is involved in profilaggrin turnover, and its inhibition results in the stabilization of profilaggrin and high molecular weight derivatives.
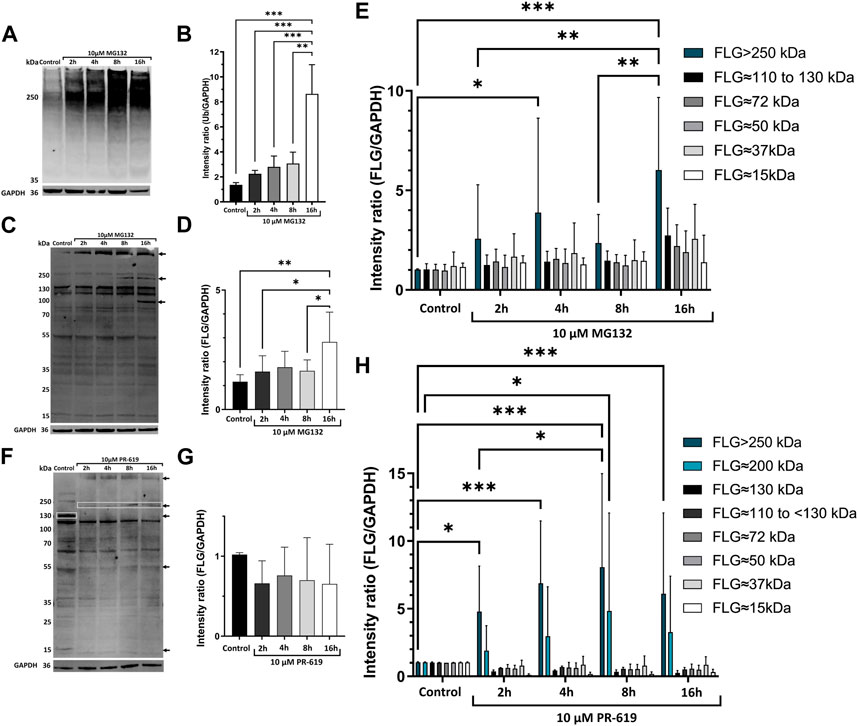
FIGURE 1. UPS is involved in degradation of profilaggrin. (A) Western blot with anti-ubiquitin in the keratinocytes upon treatment with proteasome inhibitor MG132; (B) Intensity ratio of ubiquitinated protein bands after proteasome inhibition for 2 h, 4 h, 8 h and 16 h; one-way ANOVA followed with Tukey’s multiple comparison test. (C) Western blot with anti-filaggrin in keratinocytes upon 2 h, 4 h, 8 h and 16 h treatment of proteasome inhibitor MG132, arrows indicate accumulation of undegraded profilaggrin in proteasome inhibition sample. (D) Intensity ratio of all filaggrin bands detected in different timepoint treatment of proteasome inhibitor MG132; one-way ANOVA followed by Tukey’s multiple comparison test; (E) Intensity ratio of different FLG bands upon MG132 treatment; two-way ANOVA followed by Šidák’s multiple comparison test; (F) Western blot with anti-filaggrin in keratinocytes upon 2 h, 4 h, 8 h and 16 h treatment of deubiquitinase inhibitor PR-619, Top two arrows and top box indicates accumulation of higher molecular weight filaggrin bands in the deubiquitinase inhibited samples whereas, deubiquitinase inhibition leads to depletion or disappearance of filaggrin bands of approximately 130, 50 and 15 kDa pointed with bottom box and arrows. (G) Intensity ratio of all filaggrin bands detected in different timepoint treatment of deubiquitinase inhibitor PR-619; one-way ANOVA followed by Tukey’s multiple comparison test; (H) Intensity ratio of different FLG bands upon PR-619 treatment; two-way ANOVA followed by Šidák’s multiple comparison test; error bar stands for + SD and p-value < 0.001(***); p-value ≤0.002(**); p-value ≤ 0.033(*); (n = 4).
To investigate the possibility of profilagrin undergoing ubiquitination, we utilized a pan-DUB inhibitor, PR-619, as a proof of concept. Our results indicate that the inhibitor had an impact on the ubiquitination of profilagrin. Specifically, we observed accumulation of higher molecular weight products, presumably ubiquitinated (Figure 1F), although the total content of all the profilaggrin/filaggrin-relevant products remained the same in comparison to the solvent control (Figure 1G). Intensity of the bands >250 kDa in size was significantly increased at all time points of the deubiquitinase inhibition, while intensity of the ∼200 kDa band was significantly increased at 8 h time point. At the same time, we could see disappearance of the lower molecular weight bands upon PR-619 treatment (Figures 1F, H). These data, together with the effect of proteasome inhibition, highly suggest that profilaggrin is subjected to significant ubiquitination, which is also likely to be responsible for its turnover.
The profilaggrin sequence contains multiple potential degrons
Having confirmed the proteasome involvement in the turnover of profilaggrin, we next set out to determine degron components in the protein sequence using our DEGRONOPEDIA web server, which enables comprehensive annotation of degron motifs and potentially related PTMs, with a particular focus on ubiquitination and phosphorylation. We noted that native profilaggrin has 18 primary degrons, relatively evenly distributed within the sequence (Figures 2A, B; all found primary degron motifs are summarized in Table 1). Specifically, the N-terminal sequence of profilaggrin contains potential acetylation sites that could direct it to the Ac/N-end rule pathway under specific conditions such as proteotoxic stress or immune response; we also recorded other degron motifs within the profilaggrin sequence, i.e., the destruction box (DBOX), KEN box, ABBA (DiFiore et al., 2015; Davey and Morgan, 2016) and SCFβ−TRCP (Skp1-cullin 1-F-box with β-transducin repeat-containing protein acting as its substrate receptor) motifs. In addition, profilaggrin has eight sequences corresponding to the consensus of motifs recognized by SPOP (Speckle-type POZ—pox virus and zinc finger protein), an adaptor protein for cullin 3 (CRL3)-based E3 ligases (Zhuang et al., 2009). SPOPs typically operate in the nucleus, playing a critical role in regulating apoptosis and cell proliferation. One of these, the ADSST motif located in the 4th filaggrin repeat unit of the FLG (1,689–1,693 aa), is an experimentally confirmed degron through which SPOP controls the level of hybrid protein BCR-ABL1 that governs the expression of several differentiation-related genes (Quintás-Cardama and Cortes, 2009; Liu et al., 2021). In addition, profilaggrin undergoes phosphorylation at this motif, at Ser 1,691, which could modulate SPOP binding to this degron. Molecular interaction databases BioGRID and IntAct also report on the probable binding of profilaggrin by other receptors of the cullin E3 ligases: FBXW7 (Xu et al., 2021), DTL (Huttlin et al., 2021), and VHL (Ewing et al., 2007).
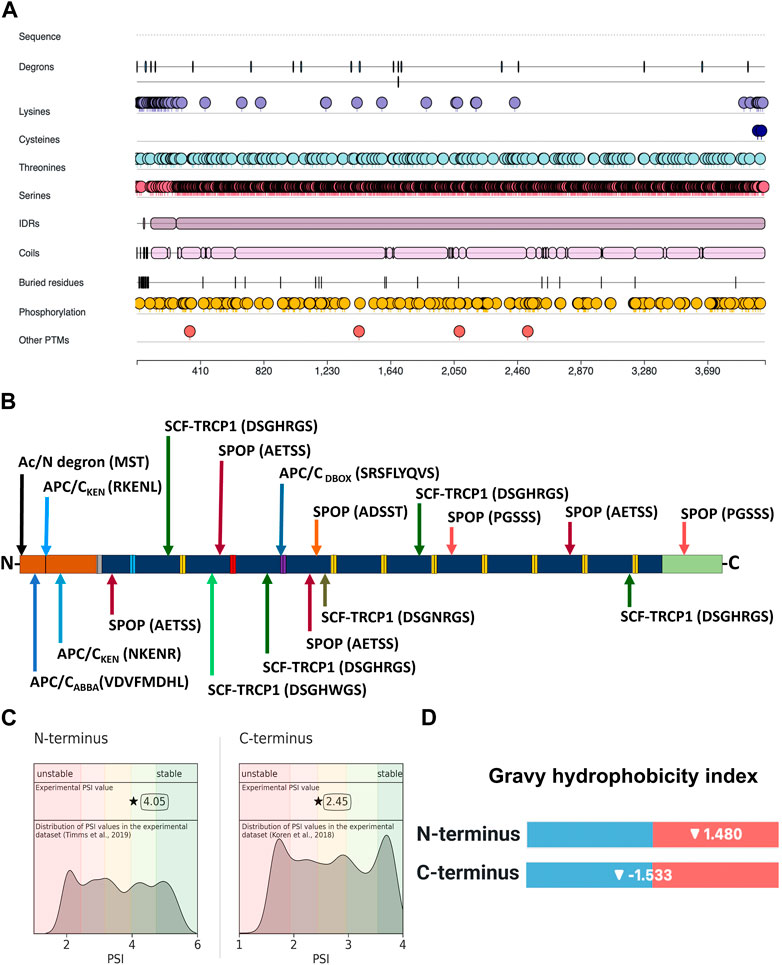
FIGURE 2. Location of degron motifs, ubiquitination-prone residues, and terminal stability of profilaggrin. (A) Illustration showing location of degron, ubiquitin-conjugating amino acid residues, intrinsic disorder region, phosphorylation sites and other post-translational modification sites in the profilaggrin protein. (B) Location of degron motif sequence and degron type in the profilaggrin wild-type sequence (legend as of Figure 3); (C) protein stability index of the N- and C- terminus of the profilaggrin; (D) Gravy hydrophobicity index of profilaggrin N- and C terminus.
Profilaggrin shows differential stability at the C- and N-termini
Based on the experimentally measured Protein Stability Index (PSI), our tool showed that the C-terminus of profilaggrin exhibits a PSI value of 2.45 (the median C-terminal PSI in human proteome is 2.72), whereas the N-terminus is more stable with a PSI value of 4.05 (the reported experimental PSI value is for profilaggrin N-terminal sequence with initiator methionine (Met) cleaved, there is no data on the corresponding variant with initiator Met present; the median N-terminal PSI in human proteome for termini where initiator methionine (Met) undergoes cleavage is 3.49) (Figure 2C). In contrast, the N-terminus has a positive Gravy hydrophobicity index (GHI) value (Kyte and Doolittle, 1982) (Figure 2D), and hydrophobic sequences often determine the specificity for recognition by chaperones and protein quality control E3s (Kats et al., 2018; Stefanovic-Barrett et al., 2018; Hickey et al., 2021; Culver et al., 2022).
The profilaggrin sequence contains multiple potential ubiquitination sites
We identified numerous Lys residues after examining the profilaggrin sequences for the secondary degrons—potential ubiquitination sites. Intriguingly, these appear to be dispersed unevenly throughout the profilaggrin sequence, with the majority accumulating either in the N-terminal domain (Figure 3A) or at the C-terminus (Figure 3B). In contrast, Lys residues are comparatively rare within the filaggrin monomeric repeats: 1st, 2nd 4th and 7th repeat each contain only one Lys residue; 6th repeat contains two and 3rd and 5th repeat harbor three Lys residues (Figures 3B, C). Apart from Lys, ubiquitin conjugation can also occur at the Ser, Thr, and Cys residues (the non-canonical ubiquitination events). In contrast to the Lys residues, which are localized mainly on the terminal ends, the majority of Ser and Thr residues are located within the filaggrin repeat units (Figures 3A–C); i.a., each filaggrin repeat unit harbors 80 to 85 Ser and 10 to 15 Thr residues. The N-terminal truncated filaggrin contains 47 Ser and 7 Thr, and C-terminal truncated filaggrin contains 48 Ser and 7 Thr residues (Figures 3A–C). Interestingly, profilaggrin contains only two Cys residues, both located at the C-terminus (Figure 3B). Altogether, despite the enrichment in the canonical signals (Lys) at the N- and C-terminus of the sequence, which could be potentially ubiquitinated, filaggrin monomer repeats contain almost five times more potential non-canonical ubiquitination sites. The distribution of Lys residues mainly in the vicinity of the profilaggrin ends and the presence of a vast inner region devoid of those (2,460–3,800 aa) may suggest that profilaggrin stability is regulated by N- and C-terminus-dependent pathways.
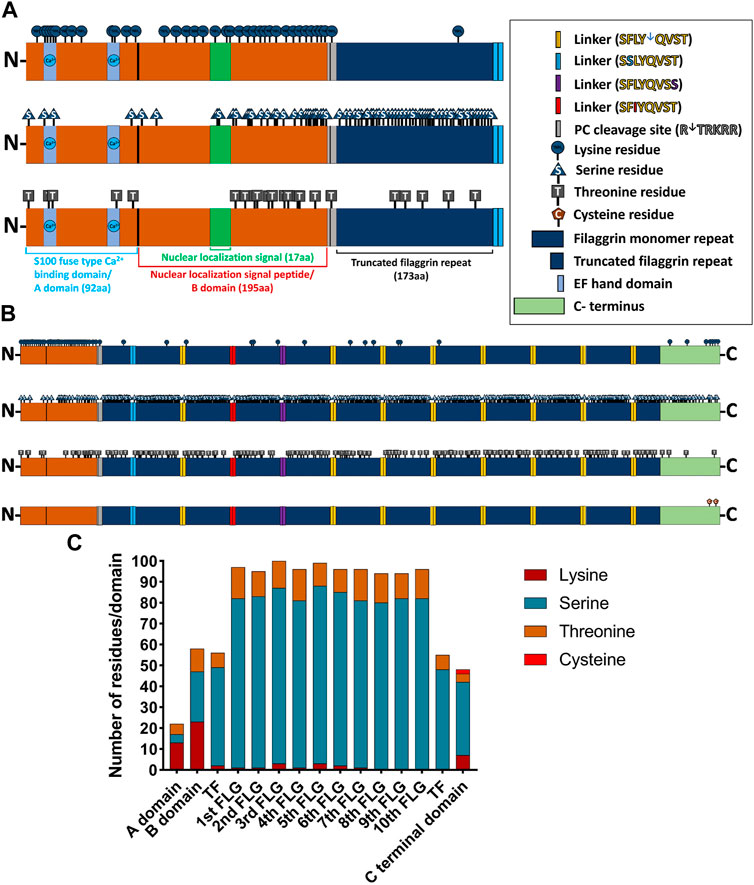
FIGURE 3. Distribution of ubiquitin (Ub)-conjugating residues in the profilaggrin wildtype sequence. (A) Illustration of N terminus of FLG. The N terminal of profilaggrin consists of a S100 fused type Ca++ Binding domain (92 aa) also known as A domain followed by nuclear localization signal peptide (195 aa) also known as B domain and a truncated filaggrin repeat (173 aa). Truncated filaggrin repeat is flanked with a PC cleavage site which links it with B domain and SASPase cleavage site which links it with rest of the filaggrin. Like all the other S100 domain filaggrin S100 domain consists of the Ca++ binding domain followed by a unique nuclear localization signal. (B) Illustration of the wild-type profilaggrin protein. (C) Number of Ub-conjugating amino acids in different domains of wild type profilaggrin.
Protease action generates filaggrin monomers with reduced degron potential at their N-termini
Profilaggrin is cleaved into 10–12 filaggrin monomers by several endoproteases, including the skin-specific retroviral aspartic protease (SASPase) and enzymes of the precursor converting enzyme (PC) family of serine proteases. Using DEGRONOPEDIA, we simulated cleavage of the profilaggrin sequence by PC and SASPase (Figure 4A) to analyse the stability and hydrophobicity of the resulting products as well as to uncover degron motifs in the newly formed termini that may have physiological significance. PC processing results in an N-terminus with residues that can be acetylated and targeted via the Ac/N-degron pathway (Supplementary Table E1). On the other hand, upon processing by SASPase, most of the resulting filaggrin monomer repeats feature an N-terminus containing positively charged (His, Lys, and Arg) and large hydrophobic residues [tryptophane (Trp), isoleucine (Ile), phenylalanine (Phe), leucine (Leu), and tyrosine (Tyr)], which can be recognized by UBR1, UBR2, UBR4, and UER5 E3 ligases or non-E3 autophagy receptor p62/SQSTM, and targeted for degradation via the Arg/N-degron pathway (Supplementary Table E2) (Dissmeyer et al., 2018; Yoo et al., 2018; Varshavsky, 2019). Despite the lower PSI value (indicating lower stability) of the N-terminus of these monomer repeats (Figure 4B), their hydrophobicity index (one of the components used to predict PSI) is also lower overall than that of the entire profilaggrin (Figure 4C). This may imply that their potential turnover based on various protein quality control E3 ligases, such as the C-terminus of HSC70-interacting protein (CHIP) or March6, which rely on hydrophobic degrons to mediate the destruction of proteins, will be impeded (Stefanovic-Barrett et al., 2018; Wang et al., 2020). Therefore, while the predicted degradation routes for the intact wild-type profilaggrin are shown in Figure 4D, we speculate that the monomer repeats generated via PC/SASP cleavage will be regulated via internal degrons or C-degron pathways (Figure 4E). Accordingly, the relatively low hydrophobicity of cleavage products at their C-termini can facilitate their interaction with cullin-RING ligases, which preferentially act upon non-hydrophobic C-terminal degrons (Hickey et al., 2021).
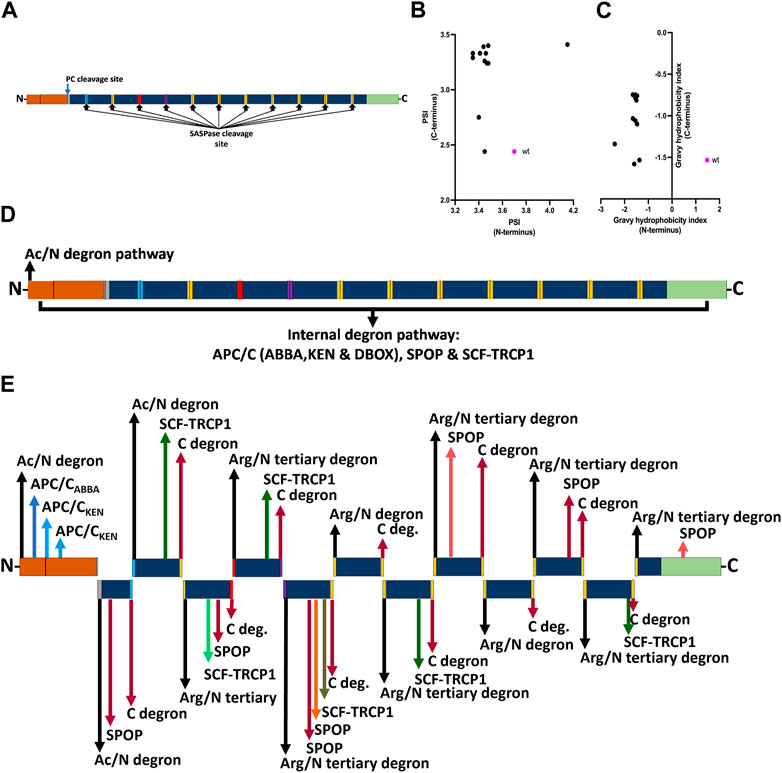
FIGURE 4. Effect of profilaggrin processing on the stability and degron content. (A) PC and SASPase clevage sites in the profilaggrin sequence. (B) terminal protein stability index of newly generated SASPase cleaved FLG products in comparison to the wild-type FLG terminus. (C) terminal Gravy hydrophobicity index of newly generated SASPase cleaved FLG products in comparison to the wild-type FLG terminus. (D) Proposed degradation routes of wild-type profilaggrin. (E) Proposed degradation routes of PC- and SASPase-cleaved profilaggrin products (legends as of Figure 3).
Degrons and ubiquitination sites are conserved within the S100 fused-type protein (SFTP) family, including filaggrin. We examined whether the SFTP have similar degron motifs and residue pattern of Lys, Ser, Thr, and Cys. Enrichment of Lys residues at the N-termini is conserved within the S100 domain (aa 1 to 100; Supplementary Figure E3). Additionally, we analysed the conservation of Lys residues among all S100 calcium-binding proteins and the S100 domain of SFTP (Supplementary Figure E4). Interestingly, the filaggrin S100 domain contains the highest number of Lys (14 residues) compared to the S100 proteins and the S100 domains of the SFTP (Supplementary Figure E5; Supplementary Table E5). In contrast, Ser residues are well conserved between filaggrin, hornerin, and filaggrin 2 in the filaggrin repeat units. Degrons also exhibit a degree of conservation within the family (Supplementary Table E6); e.g., cornulin, trichohyalin, and trichohyalin-like protein 1 contain DBOX motifs that can be recognized by the APC/C E3 complex. Trichohyalin, in addition, contains the KEN motif, which can also interact with APC/C. All the SFTPs have N-termini that can function through the Ac/N-degron pathway. Moreover, hornerin contains a sequence that can bind to E3 SPOP, and filaggrin-2 contains a degron recognized by the SCF-TRCP1. These properties imply that multiple proteins in the SFTP family may be subject to degradation by similar UPS pathways.
Clinically relevant FLG null mutations affect the number of predicted ubiquitination sites
Given the likely importance of ubiquitination affecting (pro) filaggrin turnover in the skin, we also investigated if known genetic predisposition for AD, i.e., FLG null mutations, may impact this process. To this end, we searched for any additions or removals of Lys, Ser, Thr, and Cys residues from the protein sequence resulting from FLG frameshift mutations and compared the number of those residues in the frameshift product with the same length span in the wild-type sequence. We retrieved all the mutations of FLG registered to date in the Genome Aggregation Database (gnomAD, v2.1.1.). We found that out of 23 recurrent and pathogenic frameshifts analysed, 13 (57%) generated products with a greater number of Lys residues compared to the corresponding wild-type amino acid span (Supplementary Figure E5). The highest Lys residue enrichment of eight residues was encountered in p.Ser1235HisfsTer211. Overall, these mutations introduce an additional one to eight Lys residues in the frameshift product. Lys residues remain consistent for the remaining 10 pathogenic frameshift mutations. Six of those (p.His3951ProfsTer4, p.Gln2423ValfsTer2, p.Ser2317Ter, p.Ser417ValfsTer2 or c.1248dupG, p.Gly221GlufsTer3 and p.Asn186LysfsTer4) terminate immediately, yielding products shorter than three amino acids in length, without the introduction of any Lys residues. Similarly, frameshift mutations p.Ser1171GlnfsTer15 or c.3510delG, p.Gly1109GlufsTer13 or c.3321delA, p.Gln1084ValfsTer21 or c.3250_3251delCA and p.Asp433HisfsTer43 or c.1297_1298delGA do not introduce any Lys residues despite generating a moderate length of frameshift products (Supplementary Figure E6; Supplementary Table E4).
A similar trend was observed for Thr and Cys residues—out of the 23 mutations, 14 (61%) generate a frameshift product with an increased number of Thr residues (1–23 additional Thr residues). Only one mutation (p.Asn186LysfsTer4 or c.557dupA) reduces the number of Thr (reduction of one residue) in the frameshift product (Supplementary Figure E7), whereas six of the pathogenic frameshift mutations introduce additional Cys (1–3 residues) in the frameshift products (Supplementary Figure E7). In contrast, out of the 23 pathogenic frameshift mutations, 17 (74%) reduce the number of Ser residues in the frameshift products compared to the wild-type of the same length span; the range of reduction found is by 1–30 residues (Supplementary Figure E9). We have summarized these findings in Figure 5A, showing that 17 out of 23 mutations increase the content of the potential ubiquitination sites (compared to the wild-type of the same length span) and highlights substantial differences between different mutations.
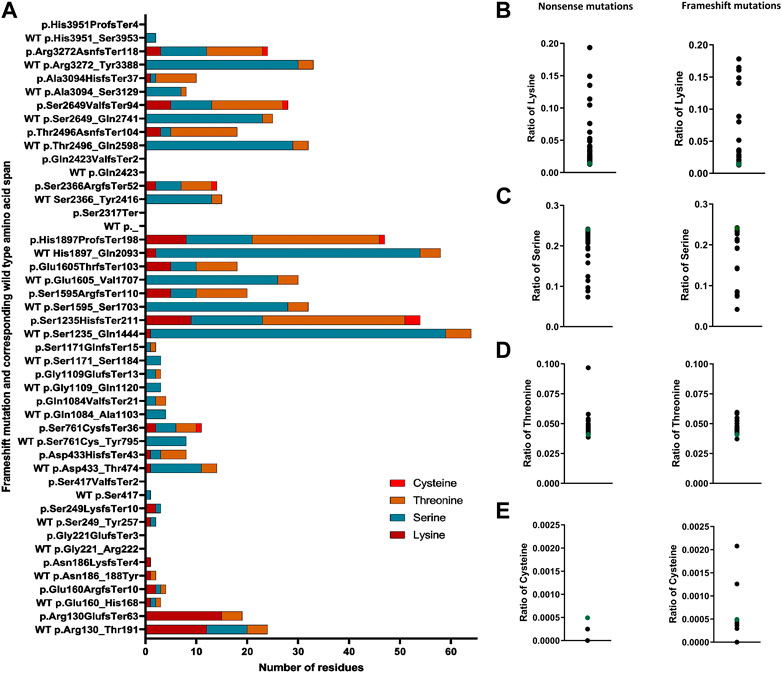
FIGURE 5. Altered content of ubiquitin-conjugating residues in FLG mutation products. (A) Number of ubiquitin-conjugating residues in the frameshift products in comparison to the corresponding wild-type same length span. (B) Ratio of lysine in the nonsense and frameshift mutant protein. (C) Ratio of serine (Ser) in the nonsense and frameshift mutant protein. (D) Ratio of threonine (Thr) in the nonsense and frameshift mutant protein. (E) Ratio of cysteine in the nonsense and frameshift mutant protein.
Because none of the recurrent frameshift mutations’ products reduced the number of Lys residues, and the vast majority led to the introduction of Thr and/or Cys and a reduction of Ser residues, we wanted to check if this is also the case for the non-recurrent rare family-specific frameshifts. To this end, we selected all the frameshifts located within the coding DNA reference sequence NM_002016.2: c. 1 to c.5700. Out of 101 rare frameshift mutations analysed, 70 (70%) introduced additional Lys residues in the frameshift products, introducing 1 to 8 additional Lys residues compared to the same length span in the wild-type sequence; the number of Lys remained the same in 28 frameshifts. In contrast, we only encountered a reduction in the number of Lys residues on three occasions (3%), namely, in the frameshift mutation p.Ser798ArgfsTer19 or c.2394delC, p.Lys801SerfsTer15 or c.2402_2405delAACA and p.Lys255IlefsTer2 or c.762_766delCAAAA; in all those cases, one Lys residue was lost compared to the same length wild-type sequence span (Supplementary Table E3). Out of the 101 rare frameshift mutations analysed, 66 introduced additional Thr residues (ranging from 1 to 25) in the frameshift product, whereas seven reduced the number of Thr residues in the frameshift product (reduction range 1–3 residues). Similarly, 45 of those introduced Cys (one to three residues) in the frameshift product. In contrast, 82 generated a frameshift product with a reduction of Ser (ranging from 1 to 57 residues). Only two frameshifts introduced additional Ser in the frameshift product (one to two additional residues) (Supplementary Table E3). Regardless of their position, all frameshifts translate into products shorter than the wild-type, with their product length varying between 1 and 260 aa.
A higher number of ubiquitination-prone residues in a protein sequence may promote its ubiquitination (Mattiroli and Sixma, 2014). Thus, we also determined the ratio of those to the full product length, nonsense, and frameshift FLG mutations. We found that irrespective of the kind of mutation, all have an increased ratio of Lys residues vs. the product length compared to the respective ratio calculated for the wild-type profilaggrin (Figure 5B), probably due to a high density of Lys residues in the N-terminal domain. As for the residues that may undergo non-canonical ubiquitination, a decreased ratio of Ser residues (Figure 5C) and an increased ratio of Thr residues (Figure 5D) was found as a general rule, while the relative content of the Cys residues was different for the two types of FLG mutations; with a reduction of the ratio for the nonsense mutations and a spectrum of the ratio values for the frameshift mutations, spanning the value obtained for the wild-type (Figure 5E).
Filaggrin mutations change C-terminal stability and introduce degron motifs
Some pathogenic mutations in FLG lead to changes in the aa sequence and the appearance of an altered C-terminus. Interestingly, we found that almost all these mutations are predicted to lead to increased C-terminus stability compared to the wild-type (Figure 6A), and this was similar for nonsense and frameshift mutations (Figures 6B, C); albeit the PSI scores were significantly lower for the latter (Figure 6D). Here, we noted that the common nonsense mutation R501X (p.Arg501Ter or c.1501C>T) had higher PSI in comparison to the common frameshift mutation 2282del4 (p.Ser761CysfsTer36), despite generating mutant proteins of relatively similar lengths. Interestingly, we found the median GHI for all the mutations analysed to be in a similar range to the wild-type protein (−1.547 vs. −1.533, respectively) (Figure 6E), and their negative value points out that their nature is hydrophilic and potentially less prone to regulation by the C-degron pathway. However, we observed a difference in the median GHI between the nonsense and frameshift mutations; again, we observed a discrepancy between the most common European variants (Figures 6F–H). Several pathogenic frameshift mutations additionally introduce degron motifs absent in the profilaggrin (Supplementary Table E4), which may have a reducing impact on the stability of the mutant product, including R501X (Figure 6I). E.g., p.Thr2496AsnfsTer104, which is the only variant with a hydrophobic C-terminus and predicted as destabilizing (Figure 6J), yields two novel primary degron sites. The APC/C (DBOX) motif occurs internally at 2,580–2,588, while the C-terminus carries a sequence corresponding to two known C-degron motifs, both carrying Arg at the antepenultimate position. Importantly, over 25% of the proteins carrying these C-degron motifs are substrates of the cullin-RING E3 ligases (Koren et al., 2018). These results point to the potentially destabilizing character of this frameshift variant, whereas other frameshift mutations moderately improve the stability of their C-termini. Data summarizing predicted C-terminal PSI, GHI, and found degron motifs in the analysed pathogenic frameshift and nonsense variants are available in Supplementary Table E4, and the predicted degradation pathways are shown in Tables 2, 3.
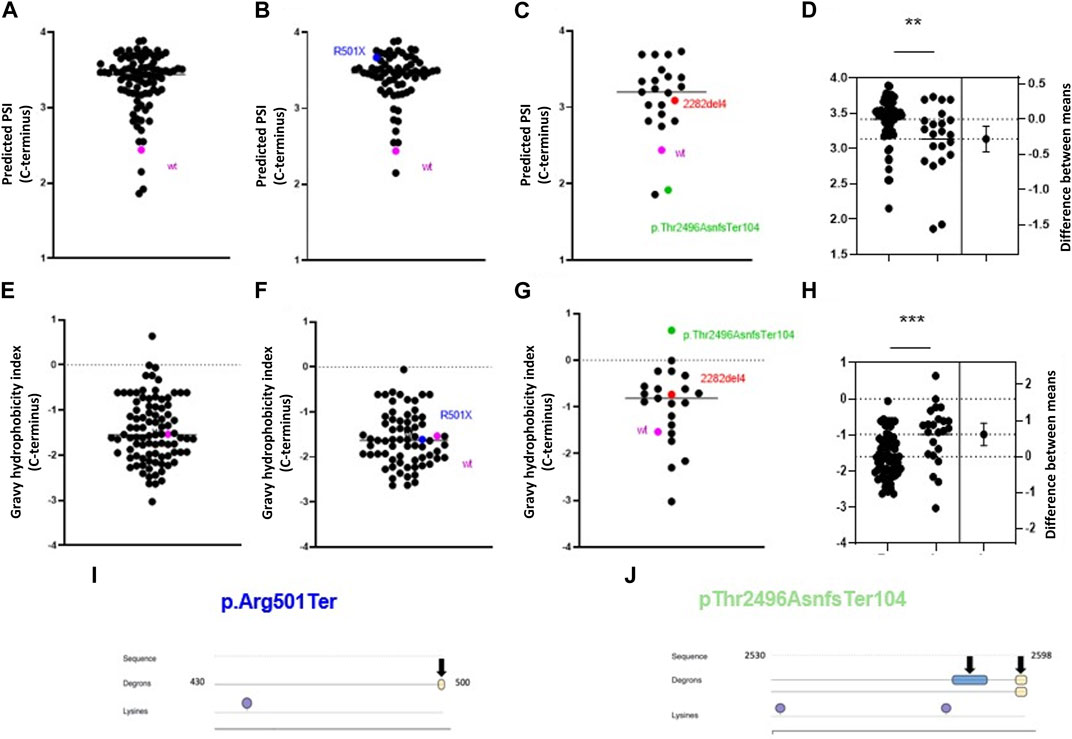
FIGURE 6. Alterations in profilaggrin stability in FLG null mutation carriers. (A–D) C-terminal PSI of recurrent pathogenic frameshift (C), nonsense mutation (B) and combined (A) here wild type is shown as pink and two most common European mutations 2282del4 and R500X are marked in red and blue; (D) estimation plot and comparison between PSI scores; Student’s t-test; p < 0.01 (**); (E–H) C terminal Gravy hydrophobicity index of recurrent pathogenic frameshift (G), nonsense mutation (F) and combined (E) here wild type is shown as pink and two most renowned mutation 2282del4 and R500X are marked in red and blue; (H) estimation plot and comparison between PSI scores; Student’s t-test; p < 0.001 (***); (I) Location of C-terminal degron in p.Arg501Ter and Lysine residue. (J) Location of C terminal degrons and lysine residues in the p.Thr2496AsnfsTer104.
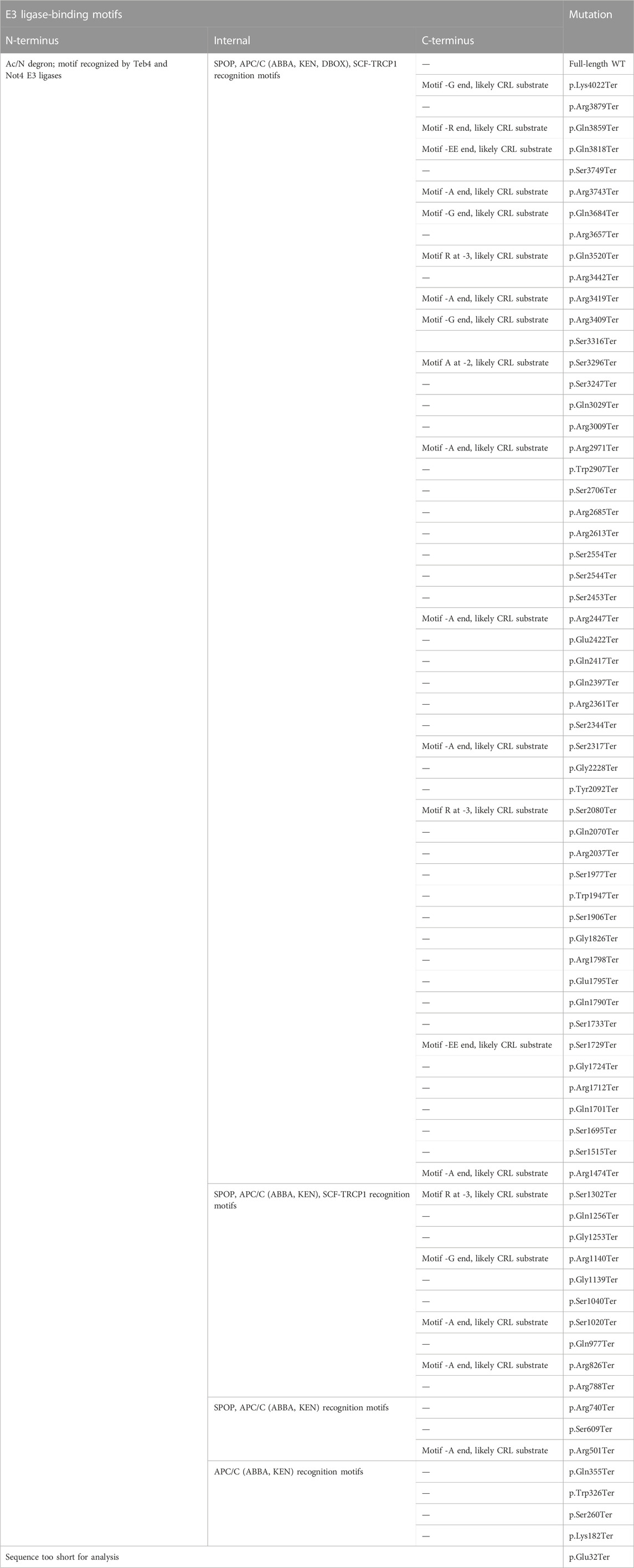
TABLE 2. List of recurrent pathogenic nonsense mutation and their degrons in comparison to the wild-type profilaggrin.
Discussion
Expression of profilaggrin and its processing into filaggrin monomer units, as well as their further breakdown into the components of the NMF, is essential for the functionality of the epidermal barrier; disturbances at any point of this intricate process are detrimental and lead to pathology. Given their role in the collapse of the keratin-based cytoskeleton and the striking effect on nuclear integrity, it is apparent why the accumulation of free filaggrin monomers into the cytosol of a keratinocyte is toxic to the cell and initiates its programmed death (Dale et al., 1997; Kuechle et al., 2000; Presland et al., 2001). Hence, both the released filaggrin monomers and any free profilaggrin molecules that could be processed must be under rigorous control in the cytosol to prevent premature cell death and allow for keratinocyte differentiation and stratification, critical for the formation of the functional skin barrier. Such control may be executed by different means, including protein sequestration, removal of the excess from the cytosol, and re-direction for turnover by the UPS.
We have previously described two separate mechanisms that control intracellular filaggrin levels during keratinocyte differentiation; the actin-based Akt-1/HspB1-dependent mechanism governing profilaggrin sequestration in KHGs (Gutowska-Owsiak et al., 2018) as well as the small extracellular vesicle (sEV)-mediated removal of excess free-floating profilaggrin/filaggrin from the cytosol (Gutowska-Owsiak, 2022). As for the latter, we also determined that Staphylococcus aureus, a skin pathogen with high prevalence and significant contribution to the pathology in AD patients, enhances filaggrin loading into the sEV cargo, facilitating its removal from the skin. Modeling protein networks indicated that the link between TLR2 signaling, profilaggrin processing, and cargo loading into the sEVS might include proteins involved in ubiquitination, pointing to protein degradation and intracellular trafficking. Indeed, the importance of protein turnover has been shown previously to be critical for controlling cellular protein abundance (Yen et al., 2008; Cambridge et al., 2011), and we envisage that both subcellular localization and propensity for degradation are key for homeostasis within the profilaggrin/filaggrin system. Ubiquitination is important for protein trafficking to diverse cellular localizations, including the compartments of the endocytic system, as well as nuclear localization aiding gene regulation. These pathways are also exploited by pathogenic bacteria and viruses for their successful transmission and immune evasion (Millar et al., 2019; Tokheim et al., 2021; Kampmeyer et al., 2022; Moosbrugger-Martinz et al., 2022).
The importance of the proteasome in profilaggrin turnover and control of its intracellular fate can be speculated already based on the appearance of greatly enlarged KHGs (hypergranulosis) in the skin of patients with an autosomal recessive epidermal abnormality known as keratosis linearis with ichthyosis congenita and sclerosing keratoderma (KLICK) syndrome (Vahlquist et al., 1997), (Dahlqvist et al., 2010), (Takeichi and Akiyama, 2020). Those patients have a deletion of a single nucleotide at the 5’ untranslated region of the proteasomal maturation protein (POMP), a chaperon that mediates stabilization of the proteasome complex; thus, loss of function leads to insufficiency of the proteasome in differentiating keratinocytes (Dahlqvist et al., 2010; Morice-Picard et al., 2017; Onnis et al., 2018). KLICK patients also demonstrate thickened SC and aberrant filaggrin staining with an antibody directed against filaggrin monomer (Dahlqvist et al., 2010).
In this study we were able to confirm the involvement of the UPS in profilaggrin degradation using a proteasome inhibitor MG132 and deubiquitinases inhibitor PR-619. Treatment with these compounds allowed us to observe the accumulation of profilaggrin and high molecular weight processed products in the lysates, suggesting reduced profilaggrin turnover likely underlying hypergranulosis in the KLICK syndrome. At the same time, the reduction in keratinocyte viability after the overnight treatment could result from the accumulation of the free unsequestered/uncontrolled monomer filaggrin units (Presland et al., 2001). Unfortunately, dead cells where this could be potentially detectable were not evaluated in this study.
Our findings are in a sharp contrast to just published short communication by Briot et al. who failed to observe a difference upon proteasome inhibition and speculated that filaggrin monomer is not degraded via proteasome (Briot et al., 2023). We believe that this discrepancy results from the use of different models. Specifically, we used cells growing in monolayers, where many cells present with low, but detectable profilaggrin expression in relatively undifferentiated cells (Gutowska-Owsiak, 2022). This is where the greatest control over free profilaggrin must be exerted and where it could undergo the UPS-mediated turnover. With increased profilaggrin expression in the 3D epidermal equivalent used by Briot et al., the vast majority of the protein is already sequestered within KGHs, therefore the risk of keratin aggregation by free cytosolic filaggrin is reduced. Importantly, such containment of the protein within KHGs prevents its access to the proteasome, which is not able to sample from those insoluble organelles. The importance of UPS-mediated control over profilaggrin is also supported by the biological pattern of the proteasome expression, which functions primarily in the undifferentiated keratinocytes, corresponding to basal and suprabasal epidermal layers (Zieba et al., 2017); proteasome gets disassembled in the cells at the late stages of differentiation, which results from regulation of POMP expression.
To get a global picture of features important from the perspective of regulation by the UPS, it is important to consider multiple factors, i.e., internal degron motifs, different properties of the N-/C-termini, such as their stability and hydrophobicity, structural features of the protein with regards to the solvent accessibility and IDRs, and residues that may undergo ubiquitination. Here, we determined sequence characteristics by identifying the degron sequences with the DEGRONOPEDIA web server, complemented with the detailed analysis of PTMs, i.e., ubiquitination and phosphorylation with the potential to govern profilaggrin/filaggrin degradation and cellular trafficking, allowing us to propose likely degradation pathways. Protein degradation via the UPS often requires the formation of the Lys-linked polyubiquitin chains, while the nuclear re-directing depends on the Lys monoubiquitination (Trotman et al., 2007). Apart from the Lys residues, the profilaggrin sequence contains multiple Cys, Ser, and Thr residues; such residues have been shown to undergo ubiquitination at these marks. Furthermore, it has been shown that such non-canonical ubiquitination is less thermodynamically stable (McDowell and Philpott, 2013; McClellan et al., 2019; Squair and Virdee, 2022) than the canonical Lys ubiquitination and predominantly drives proteasomal degradation over protein sorting (McClellan et al., 2019). The high enrichment of the canonical ubiquitination sites in the N-terminal domains of profilaggrin and the accumulation of the non-canonical ubiquitination sites within the monomer repeats are interesting observations and suggest potential differences in the degradation pathways between the domains. The N-terminal domain is involved in nuclear signaling events that initiate a positive feedback loop for profilaggrin expression and keratinocyte differentiation. Proteasome activity is considered high in the nucleus, thus, increased predicted stability values could potentially indicate relative resistance of the profilaggrin N-terminal domain.
Furthermore, we found that the entire profilaggrin sequence contains 18 putative primary degron sites. Our screening suggests that the unprocessed profilaggrin protein is likely degraded upon recognition of the Ac/N-degron motif as the second N-terminal residue is Ser and Nα-terminal acetylation (Nt-acetylation) of nascent proteins, whose N-terminus contains Met or a small uncharged residue alanine, Cys, Val, Ser or Thr as these become N-terminal after co-translational removal of Met by Met-aminopeptidase), is one of the most significant and common protein modifications occurring on ∼80% of all human proteins (van Damme et al., 2012; Aksnes et al., 2016). Nt-acetylation can serve as a degron motif for the two E3 ligases: March6/TEB4, a Really Interesting New Gene (RING)-type E3 ligase located in the endoplasmic reticulum membrane, and NOT4, a component of the CCR4-NOT multi-subunit complex, which induces substrate protein degradation via the Ac/N-degron pathway (Varshavsky, 2011; Shemorry et al., 2013; Park et al., 2015; Lee et al., 2016).
The presence of 17 internal degrons in wild-type profilaggrin indicates the possibility of its N-/C-terminus-independent degradation. Among these degrons, the destruction box (DBOX), KEN box, and ABBA motifs are found and substrates containing one or more of these sequences typically undergo polyubiquitylation by the anaphase-promoting complex (APC/C) (Brown et al., 2014). APC/C is a large, multi-subunit E3 ligase that regulates cell cycle progression in eukaryotes. Importantly, it is also involved in proliferation and differentiation regulation in human primary keratinocytes (Quek et al., 2018). Hence, it is probable that APC/C could influence the keratinocyte life cycle and contribute to the disruption in epidermal homeostasis, such as that seen in AD, at least partly through its effect on profilaggrin degradation.
SCFβ-TRCP E3 ligase complex regulates proteasome-dependent degradation of various substrates, including early mitotic inhibitor 1 (EMI1) (Margottin-Goguet et al., 2003), cell cycle homolog 25 (CDC25A) (Busino et al., 2003; Jin et al., 2006) and vascular endothelial growth factor receptor 2 (VEGFR2) (Shaik et al., 2012). Profilaggrin also contains the consensus degron sequence (D(S)Gx{2,3}([ST]) of SCFβ−TRCP where Ser/Thr residues should be phosphorylated for proper motif recognition (x—any aa) (Winston et al., 1999; Guharoy et al., 2016). Interestingly, this motif was shown to be phosphorylated in rat profilaggrin (Resing et al., 1995) but neither the iPTMNet (Huang et al., 2018) nor PhoshoSitePlus (Hornbeck et al., 2015) databases, incorporated into the DEGRONOPEDIA, record any phosphorylation within them.
Profilaggrin has also been proposed as a binding partner of receptors of the cullin E3 ligases: FBXW7 (Xu et al., 2021), DTL (Huttlin et al., 2021), and VHL (Ewing et al., 2007). Present in proliferating cells, FBXW7 (F-Box and WD Repeat Domain Containing 7) is a member of the F-box family of proteins and functions as a substrate recognition element of the SCF E3 ligase. FBXW7 isoforms recognize their substrates through the CDC4 phosphodegron (CPD) motif so that they can be ubiquitinated and targeted for degradation by the proteasome along with the substrate. Substrates of FBXW7 containing CPD variants include MYC, Cyclin E/cyclin-dependent kinase CDK2, JUN, Myeloid cell leukemia-1 (MCL-1), mammalian target of rapamycin (mTOR), and NOTCH1 (Koepp et al., 2001; Wei et al., 2005; Mo et al., 2007; Mao et al., 2008; Inuzuka et al., 2011; King et al., 2013; Yeh et al., 2018). Analysis of the profilaggrin sequence did not reveal the occurrence of CPD motifs, which may suggest a profilaggrin-specific degron/phosphodegron that requires further identification. DTL is another receptor likely to bind to profilaggrin; it associates with CRL4A (cullin 4A-RING ubiquitin ligase) and regulates DNA replication and the cell cycle by regulating proteins such as CDT1, PR-Set7/Set8/KMT5A and p21 (Jin et al., 2006; Abbas and Dutta, 2009; Centore et al., 2010; Shibata et al., 2011; Cui et al., 2019). Since DTL prevents DNA damage after UV irradiation (Ishii et al., 2010), we hypothesize its involvement in skin function, possibly through modulation of profilaggrin or filaggrin monomer levels. In addition, profilaggrin was detected as an interactor of cullin-2 (CUL2)-based E3 ligases (Bennett et al., 2010) and VHL (von Hippel-Lindau). CUL2 is a platform for the Elongin B and Elongin C adaptor protein complex, which interact with various substrate receptors, such as the VHL tumor suppressor protein (Gossage et al., 2014; Cai and Yang, 2016). Since VHL plays a role in skin inflammation, CUL2 and VHL may regulate profilaggrin in psoriasis (Martínez-Torres et al., 2022).
Our analysis indicated that the monomers generated by SASPase cleavage are relatively stable, given their minor decrease in the N-terminal PSI, but a significant increase in the C-terminal PSI and a drop in GHI value; this likely permits their long-lasting functionality required for cross-linking of the proteins within the cornified envelope, and aggregation and collapse of the IF-based cytoskeleton. Intriguingly, the UPS system has been previously implicated in the degradation and turnover of keratins (Yamazaki et al., 2012); increased keratin turnover is observed in a subtype of epidermolysis bullosa simplex (EBS) patients, resulting in shortened lifespan, replicative senescence, and decreased cellular resistance in keratinocytes (Logli et al., 2022).
It is important to note that the UPS-mediated degradation is a pathway separate from the pathway of profilaggrin processing to monomers and subsequently into the compounds of the NMF, which occurs predominantly in the SC containing no living cells. UPS components primarily function in the intracellular environment; however, both the ubiquitinating enzymes and proteasome are also present and active in the extracellular spaces and body fluids (Takada et al., 1997; Sixt and Dahlmann, 2008; Majetschak, 2011; Ben-Nissan et al., 2022), potentially also within the SC. However, the difference in pH could affect the efficiency of the degradation process, given the impact of the acidic environment. All three hydrolytic activities (chymotrypsin-like, trypsin-like, and caspase-like) of the 26S proteasome decreased when the pH was lowered from 7.5 to 7.0 (Ugai et al., 1993; Klinkradt et al., 1997). At the same time, the 20S proteasome from human platelets displayed chymotrypsin-like activity with an optimum pH of 5.0–5.5 (Ostrowska et al., 2009). It can be speculated that similar 20S activity may be maintained within the SC, enabling partial degradation of targeted proteins. Changes in pH may also affect the ubiquitination process, e.g., pH lower than 7.5 destabilized the APC/C complex (Passmore et al., 2005), and we identified potential APC/C degrons in profilaggrin. These observations may suggest that profilaggrin and its derivatives do not undergo intense turnover in the SC.
For this study, we assumed that profilaggrin processing may coincide with the UPS-mediated degradation, at least to some extent. On the other hand, while the majority of profilaggrin processing to monomers can be localized to the SC, i.e., after keratinocyte death, it is unclear how much processing may take place in the live epidermal layers. However, small but detectable amounts of the filaggrin monomer have been observed by Western blot in keratinocytes grown in 2D cultures which were not stratified (Dang et al., 2016), so we could envisage that the process likely begins much earlier, albeit probably at a reduced rate, since the highest expression of the processing enzymes was demonstrated for the top epidermal layers (Miyachi et al., 1986; Pearton et al., 2001; Bernard et al., 2005).
Here we also determined that the common FLG mutations predisposing to AD result in the generation of new products with a different propensity for degradation, supported by changes in stability and ubiquitination-prone residues as well as the emergence of novel degrons. This may suggest that both the filaggrin turnover and organellar distribution in the skin of the patients are affected, and the generated products may undergo altered cellular fates. Indeed, in the case of several mutations, we noted the accumulation of all those pro-degradation motifs. At the same time, while introducing several new ubiquitination-prone residues compared to the wild-type of the same length, one of the most common pathological FLG mutations, 2282del4, does not introduce any novel known degrons. However, it is important to note that it is not clear to what extent profilaggrin could undergo modification at all these potentially ubiquitinated residues; since the protein is known to undergo many different modifications at Lys residues.
FLG mutations resulting in the loss of the C-terminal domain led to practically nonfunctional monomers generated from this allele (Presland et al., 2000; Sandilands et al., 2007); hence, it has been long assumed that the profilaggrin C-terminal domain is critical for processing of the protein. However, it is not clear whether this is of crucial importance, as no experimental data on the C-terminal domain’s involvement in the processing has yet been published. Here, we determined that the C-terminal sequence of the wild-type filaggrin has a stability score in the range that is typical for most human proteins, therefore not allowing us to consider this to be a highly stabilizing part for the entire profilaggrin molecule.
Interestingly, we found the C-terminal PSI scores to be increased for nearly all the mutated products, complicating the picture further. It could be speculated that the increased stability of the mutated product would result in the accumulation of such protein intracellularly, albeit likely not in the form of granules (Sybert et al., 1985). However, there is no evidence in the samples from the patients, and certainly, no evidence indicating increased processing of the protein as this would result in high amounts of NMF being generated and barrier function maintenance. It is possible that in this scenario, the remaining routes (proteasomal degradation, vesicular export) would be enhanced to control the profilaggrin/filaggrin levels in the cytosol. Indeed, we have previously made a very unexpected observation of the increased amount of the profilaggrin/filaggrin protein cargo in the sEVs fractions isolated from the blood of AD patients compared to the healthy controls (Gutowska-Owsiak, 2022), and this would perfectly align with such a scenario. Thus, it is conceivable that keratinocytes could expel the excess profilaggrin/filaggrin-related products to prevent the toxic consequences in the event of a diminished capacity to generate KHGs (Sybert et al., 1985). It would require additional experimental work beyond the scope of this study to determine if the mutated products can indeed be detected in the sEVs found in the blood of the patients.
In summary, we determined that proteasome-mediated profilaggrin degradation is one of the means of controlling intracellular levels of the protein in keratinocytes, and we identified critical components regulating UPS-mediated processing (ubiquitination-prone residues and degrons) within its sequence. Furthermore, we also described how FLG mutations might affect the stability of mutant proteins and potential degradation routes in the cell.
Data availability statement
The original contributions presented in the study are included in the article/Supplementary Material, further inquiries can be directed to the corresponding authors.
Author contributions
AP performed analysis and experiments, wrote sections of the manuscript, prepared figures. NS performed analysis and experiments, wrote sections of the manuscript, prepared figures. SB contributed to conception and design of the study, contributed to manuscript writing. AK performed initial analysis and performed experiments; WP contributed to conception and design of the study, performed analysis, wrote sections of the manuscript, contributed to manuscript writing and provided funding; DG-O, contributed to conception and design of the study, performed analysis, wrote the first and subsequent drafts of the manuscript and provided funding. All authors read and approved the submitted version.
Funding
This research was supported by the National Science Centre, Poland, grant PRELUDIUM number 2021/41/N/NZ1/03473 to NS, National Science Centre, Poland, grant SONATA BIS number 2019/34/E/NZ6/00354 to DG-O, as well as POIR.04.04.00-00-21FA/16–00 grant, carried out within the First TEAM programme of the Foundation for Polish Science co-financed by the European Union under the European Regional Development Fund (awarded to DG-O). WP was supported by the National Science Centre, Poland, grant SONATA-BIS number 2021/42/E/NZ1/00190. SB is supported by a Wellcome Trust Senior Research Fellowship (220875/Z/20/Z).
Acknowledgments
We would like to thank Prof. Rheinwald for the generous gift of the N/TERT-1 cell line.
Conflict of interest
The authors declare that the research was conducted in the absence of any commercial or financial relationships that could be construed as a potential conflict of interest.
Publisher’s note
All claims expressed in this article are solely those of the authors and do not necessarily represent those of their affiliated organizations, or those of the publisher, the editors and the reviewers. Any product that may be evaluated in this article, or claim that may be made by its manufacturer, is not guaranteed or endorsed by the publisher.
Supplementary material
The Supplementary Material for this article can be found online at: https://www.frontiersin.org/articles/10.3389/fmolb.2023.1105678/full#supplementary-material
References
Abbas, T., and Dutta, A. (2009). P21 in cancer: Intricate networks and multiple activities. Nat. Rev. Cancer 9, 400–414. doi:10.1038/nrc2657
Aho, S., Harding, C. R., Lee, J. M., Meldrum, H., and Bosko, C. A. (2012). Regulatory role for the profilaggrin N-terminal domain in epidermal homeostasis. J. Investigative Dermatology 132, 2376–2385. doi:10.1038/jid.2012.174
Aksnes, H., Drazic, A., Marie, M., and Arnesen, T. (2016). First things first: Vital protein marks by N-terminal acetyltransferases. Trends Biochem. Sci. 41, 746–760. doi:10.1016/j.tibs.2016.07.005
Backendorf, C., and Hohl, D. (1992). A common origin for cornified envelope proteins? Nat. Genet. 2, 91. doi:10.1038/ng1092-91
Barker, J. N. W. N., Palmer, C. N. A., Zhao, Y., Liao, H., Hull, P. R., Lee, S. P., et al. (2007). Null mutations in the filaggrin gene (FLG) determine major susceptibility to early-onset atopic dermatitis that persists into adulthood. J. Invest. Dermatol 127, 564–567. doi:10.1038/sj.jid.5700587
Ben-Nissan, G., Katzir, N., Füzesi-Levi, M. G., and Sharon, M. (2022). Biology of the extracellular proteasome. Biomolecules 12, 619. doi:10.3390/biom12050619
Bennett, E. J., Rush, J., Gygi, S. P., and Harper, J. W. (2010). Dynamics of cullin-RING ubiquitin ligase network revealed by systematic quantitative proteomics. Cell 143, 951–965. doi:10.1016/j.cell.2010.11.017
Bernard, D., Méhul, B., Thomas-Collignon, A., Delattre, C., Donovan, M., and Schmidt, R. (2005). Identification and characterization of a novel retroviral-like aspartic protease specifically expressed in human epidermis. J. Gen. Intern Med. 20, 278–287. doi:10.1111/j.0022-202X.2005.23816.x
Bonnart, C., Deraison, C., Lacroix, M., Uchida, Y., Besson, C., Robin, A., et al. (2010). Elastase 2 is expressed in human and mouse epidermis and impairs skin barrier function in Netherton syndrome through filaggrin and lipid misprocessing. J. Clin. Invest. 120, 871–882. doi:10.1172/JCI41440
Briot, J., Arbey, E., Goudounèche, D., Bernard, D., Simon, M., and Méchin, M. C. (2023). Human filaggrin monomer does not seem to be a proteasome target. Exp. Dermatol. doi:10.1111/EXD.14772
Brown, N. G., Watson, E. R., Weissmann, F., Jarvis, M. A., VanderLinden, R., Grace, C. R. R., et al. (2014). Mechanism of polyubiquitination by human anaphase-promoting complex: RING repurposing for ubiquitin chain assembly. Mol. Cell 56, 246–260. doi:10.1016/j.molcel.2014.09.009
Brown, S. J., Asai, Y., Cordell, H. J., Campbell, L. E., Zhao, Y., Liao, H., et al. (2011). Loss-of-function variants in the filaggrin gene are a significant risk factor for peanut allergy. J. Allergy Clin. Immunol. 127, 661–667. doi:10.1016/j.jaci.2011.01.031
Busino, L., Donzelli, M., Chiesa, M., Guardavaccaro, D., Ganoth, D., Dorrello, N. V., et al. (2003). Degradation of Cdc25A by β-TrCP during S phase and in response to DNA damage. Nature 426, 87–91. doi:10.1038/nature02082
Cai, W., and Yang, H. (2016). The structure and regulation of Cullin 2 based E3 ubiquitin ligases and their biological functions. Cell Div. 11, 7–11. doi:10.1186/s13008-016-0020-7
Cambridge, S. B., Gnad, F., Nguyen, C., Bermejo, J. L., Krüger, M., and Mann, M. (2011). Systems-wide proteomic analysis in mammalian cells reveals conserved, functional protein turnover. J. Proteome Res. 10, 5275–5284. doi:10.1021/pr101183k
Candi, E., Schmidt, R., and Melino, G. (2005). The cornified envelope: A model of cell death in the skin. Nat. Rev. Mol. Cell Biol. 6, 328–340. doi:10.1038/nrm1619
Centore, R. C., Havens, C. G., Manning, A. L., Li, J. M., Flynn, R. L., Tse, A., et al. (2010). CRL4Cdt2-mediated destruction of the histone methyltransferase Set8 prevents premature chromatin compaction in S phase. Mol. Cell 40, 22–33. doi:10.1016/j.molcel.2010.09.015
Chau, V., Tobias, J. W., Bachmair, A., Marriott, D., Ecker, D. J., Gonda, D. K., et al. (1989). A multiubiquitin chain is confined to specific lysine in a targeted short-lived protein. Sci. (1979) 243, 1576–1583. doi:10.1126/science.2538923
Chen, L., and Kashina, A. (2021). Post-translational modifications of the protein termini. Front. Cell Dev. Biol. 9, 719590. doi:10.3389/fcell.2021.719590
Chen, Y., Vortmeyer, A., Zhuang, Z., Huang, S., and Jensen, R. T. (2003). Loss of heterozygosity of chromosome 1q in gastrinomas: Occurrence and prognostic significance. AACR 63, 817–823.
Cheng, R., Li, M., Zhang, H., Guo, Y., Chen, X., Tao, J., et al. (2012). Common FLG mutation K4671X not associated with atopic dermatitis in han Chinese in a family association study. PLoS One 7, e49158. doi:10.1371/journal.pone.0049158
Contzler, R., Favre, B., Huber, M., and Hohl, D. (2005). Cornulin, a new member of the “fused gene” family, is expressed during epidermal differentiation. J. Investigative Dermatology 124, 990–997. doi:10.1111/j.0022-202X.2005.23694.x
Cookson, W. O. C. M., Ubhi, B., Lawrence, R., Abecasis, G. R., Walley, A. J., Cox, H. E., et al. (2001). Genetic linkage of childhood atopic dermatitis to psoriasis susceptibility loci. Nat. Genet. 27, 372–373. doi:10.1038/86867
Cork, M. J., Danby, S. G., Vasilopoulos, Y., Hadgraft, J., Lane, M. E., Moustafa, M., et al. (2009). Epidermal barrier dysfunction in atopic dermatitis. J. Invest. Dermatol 129, 1892–1908. doi:10.1038/jid.2009.133
Cui, H., Wang, Q., Lei, Z., Feng, M., Zhao, Z., Wang, Y., et al. (2019). DTL promotes cancer progression by PDCD4 ubiquitin-dependent degradation. J. Exp. Clin. Cancer Res. 38, 350. doi:10.1186/s13046-019-1358-x
Culver, J. A., Li, X., Jordan, M., and Mariappan, M. (2022). A second chance for protein targeting/folding: Ubiquitination and deubiquitination of nascent proteins. BioEssays 44, 2200014. doi:10.1002/bies.202200014
Dahlqvist, J., Klar, J., Tiwari, N., Schuster, J., Törmä, H., Badhai, J., et al. (2010). A single-nucleotide deletion in the POMP 5′ UTR causes a transcriptional switch and altered epidermal proteasome distribution in KLICK genodermatosis. Am. J. Hum. Genet. 86, 596–603. doi:10.1016/j.ajhg.2010.02.018
Dale, B. A., Presland, R. B., Lewis, S. P., Underwood, R. A., and Fleckman, P. (1997). Transient expression of epidermal filaggrin in cultured cells causes collapse of intermediate filament networks with alteration of cell shape and nuclear integrity. J. Invest. Dermatol 108, 179–187. doi:10.1111/1523-1747.ep12334205
Dang, N., Ma, X., Meng, X., An, L., and Pang, S. (2016). Dysregulated function of normal human epidermal keratinocytes in the absence of filaggrin. Mol. Med. Rep. 14, 2566–2572. doi:10.3892/mmr.2016.5539
Davey, N. E., and Morgan, D. O. (2016). Building a regulatory network with short linear sequence motifs: Lessons from the degrons of the anaphase-promoting complex. Mol. Cell 64, 12–23. doi:10.1016/j.molcel.2016.09.006
Denecker, G., Hoste, E., Gilbert, B., Hochepied, T., Ovaere, P., Lippens, S., et al. (2007). Caspase-14 protects against epidermal UVB photodamage and water loss. Nat. Cell Biol. 9, 666–674. doi:10.1038/ncb1597
Dickson, M. A., Hahn, W. C., Ino, Y., Ronfard, V., Wu, J. Y., Weinberg, R. A., et al. (2000). Human keratinocytes that express hTERT and also bypass a p16 INK4a -enforced mechanism that limits life span become immortal yet retain normal growth and differentiation characteristics. Mol. Cell Biol. 20, 1436–1447. doi:10.1128/mcb.20.4.1436-1447.2000
DiFiore, B., Davey, N. E., Hagting, A., Izawa, D., Mansfeld, J., Gibson, T. J., et al. (2015). The ABBA motif binds APC/C activators and is shared by APC/C substrates and regulators. Dev. Cell 32, 358–372. doi:10.1016/j.devcel.2015.01.003
Dissmeyer, N., Rivas, S., and Graciet, E. (2018). Life and death of proteins after protease cleavage: Protein degradation by the N-end rule pathway. New Phytol. 218, 929–935. doi:10.1111/nph.14619
Duncan, L. M., Piper, S., Dodd, R. B., Saville, M. K., Sanderson, C. M., Luzio, J. P., et al. (2006). Lysine-63-linked ubiquitination is required for endolysosomal degradation of class I molecules. EMBO J. 25, 1635–1645. doi:10.1038/sj.emboj.7601056
Eckert, R. L., Broome, A. M., Ruse, M., Robinson, N., Ryan, D., and Lee, K. (2004). S100 proteins in the epidermis. J. Invest. Dermatol 123, 23–33. doi:10.1111/j.0022-202X.2004.22719.x
Enomoto, H., Hirata, K., Otsuka, K., Kawai, T., Takahashi, T., Hirota, T., et al. (2008). Filaggrin null mutations are associated with atopic dermatitis and elevated levels of IgE in the Japanese population: A family and case–control study. J. Hum. Genet. 53, 615–621. doi:10.1007/s10038-008-0293-z
Ewing, R. M., Chu, P., Elisma, F., Li, H., Taylor, P., Climie, S., et al. (2007). Large-scale mapping of human protein-protein interactions by mass spectrometry. Mol. Syst. Biol. 3, 89. doi:10.1038/msb4100134
Fleckman, P., Holbrook, K. A., Dale, B. A., and Sybert, V. P. (1987). Keratinocytes cultured from subjects with ichthyosis vulgaris are phenotypically abnormal. J. Investigative Dermatology 88, 640–645. doi:10.1111/1523-1747.ep12470251
Forus, A., Berner, J. M., Meza-Zepeda, L. A., Saeter, G., Mischke, D., Fodstad, O., et al. (1998). Molecular characterization of a novel amplicon at 1q21-q22 frequently observed in human sarcomas. Br. J. Cancer 78, 495–503. doi:10.1038/bjc.1998.521
Gan, S. Q., McBride, O. W., Idler, W. W., Markova, N., and Steinert, P. M. (1990). Organization, structure, and polymorphisms of the human profilaggrin gene. Biochemistry 29, 9432–9440. doi:10.1021/bi00492a018
Gossage, L., Eisen, T., and Maher, E. R. V. H. L. (2014). VHL, the story of a tumour suppressor gene. Nat. Rev. Cancer 15, 55–64. doi:10.1038/nrc3844
Guharoy, M., Bhowmick, P., Sallam, M., and Tompa, P. (2016). Tripartite degrons confer diversity and specificity on regulated protein degradation in the ubiquitin-proteasome system. Nat. Commun. 7, 10239. doi:10.1038/ncomms10239
Guharoy, M., Lazar, T., Macossay-Castillo, M., and Tompa, P. (2022). Degron masking outlines degronons, co-degrading functional modules in the proteome. Commun. Biol. 5, 445. doi:10.1038/s42003-022-03391-z
Gutowska-Owsiak, D., de La Serna, J. B., Fritzsche, M., Naeem, A., Podobas, E. I., Leeming, M., et al. (2018). Orchestrated control of filaggrin–actin scaffolds underpins cornification. Cell Death Dis. 9, 412–418. doi:10.1038/s41419-018-0407-2
Gutowska-Owsiak, D. (2022). “Excess filaggrin in keratinocytes is removed by extracellular vesicles to prevent premature death and this mechanism can be hijacked by Staphylococcus aureus in a TLR2-dependent fashion,”. CC BY 4.0.
Handa, S., Khullar, G., Pal, A., Kamboj, P., and De, D. (2019). Filaggrin gene mutations in hand eczema patients in the Indian subcontinent: A prospective case-control study. Contact Dermat. 80, 359–364. doi:10.1111/cod.13233
Hershko, A., and Ciechanover, A. (2003). The ubiquitin system. Annu. Rev. Biochem. 67, 425–479. doi:10.1146/annurev.biochem.67.1.425
Hershko, A., and Ciechanover, A. (1992). The ubiquitin system for protein degradation. Annu. Rev. Biochem. 61, 761–807. doi:10.1146/annurev.bi.61.070192.003553
Hickey, C. M., Breckel, C., Zhang, M., Theune, W. C., and Hochstrasser, M. (2021). Protein quality control degron-containing substrates are differentially targeted in the cytoplasm and nucleus by ubiquitin ligases. Genetics 217, 1–19. doi:10.1093/genetics/iyaa031
Hornbeck, P. v., Zhang, B., Murray, B., Kornhauser, J. M., Latham, V., and Skrzypek, E. (2015). PhosphoSitePlus, 2014: Mutations, PTMs and recalibrations. Nucleic Acids Res. 43, D512–D520. doi:10.1093/nar/gku1267
Huang, H., Arighi, C. N., Ross, K. E., Ren, J., Li, G., Chen, S. C., et al. (2018). iPTMnet: an integrated resource for protein post-translational modification network discovery. Nucleic Acids Res. 46, D542–D550. doi:10.1093/nar/gkx1104
Husnjak, K., and Dikic, I. (2012). Ubiquitin-binding proteins: Decoders of ubiquitin-mediated cellular functions. Annu. Rev. Biochem. 81, 291–322. doi:10.1146/annurev-biochem-051810-094654
Huttlin, E. L., Bruckner, R. J., Navarrete-Perea, J., Cannon, J. R., Baltier, K., Gebreab, F., et al. (2021). Dual proteome-scale networks reveal cell-specific remodeling of the human interactome. Cell 184, 3022–3040.e28. doi:10.1016/j.cell.2021.04.011
Inuzuka, H., Shaik, S., Onoyama, I., Gao, D., Tseng, A., Maser, R. S., et al. (2011). SCFFBW7 regulates cellular apoptosis by targeting MCL1 for ubiquitylation and destruction. Nature 471, 104–109. doi:10.1038/nature09732
Ishida-Yamamoto, A., Takahashi, H., Presland, R. B., Dale, B. A., and Iizuka, H. (1998). Translocation of profilaggrin N-terminal domain into keratinocyte nuclei with fragmented DNA in normal human skin and loricrin keratoderma. Lab. Invest. 78, 1245–1253.
Ishii, T., Shiomi, Y., Takami, T., Murakami, Y., Ohnishi, N., and Nishitani, H. (2010). Proliferating cell nuclear antigen-dependent rapid recruitment of Cdt1 and CRL4Cdt2 at DNA-damaged sites after UV irradiation in HeLa cells. J. Biol. Chem. 285, 41993–42000. doi:10.1074/jbc.M110.161661
Itoyama, T., Nanjungud, G., Chen, W., Dyomin, V. G., Teruya-Feldstein, J., Jhanwar, S. C., et al. (2002). Molecular cytogenetic analysis of genomic instability at the 1q12-22 chromosomal site in B-cell non-Hodgkin lymphoma. Genes Chromosom. Cancer 35, 318–328. doi:10.1002/gcc.10120
Jackson, B., Tilli, C. M. L. J., Hardman, M. J., Avilion, A. A., MacLeod, M. C., Ashcroft, G. S., et al. (2005). Late cornified envelope family in differentiating epithelia—response to calcium and ultraviolet irradiation. J. Investigative Dermatology 124, 1062–1070. doi:10.1111/j.0022-202X.2005.23699.x
Jin, J., Arias, E. E., Chen, J., Harper, J. W., and Walter, J. C. (2006). A family of diverse cul4-ddb1-interacting proteins includes Cdt2, which is required for S phase destruction of the replication factor Cdt1. Mol. Cell 23, 709–721. doi:10.1016/j.molcel.2006.08.010
Jin, L., Williamson, A., Banerjee, S., Philipp, I., and Rape, M. (2008). Mechanism of ubiquitin-chain formation by the human anaphase-promoting complex. Cell 133, 653–665. doi:10.1016/j.cell.2008.04.012
Jurakic Toncic, R., Kezic, S., Jakasa, I., Ljubojevic Hadzavdic, S., Balic, A., Petkovic, M., et al. (2020). Filaggrin loss-of-function mutations and levels of filaggrin degradation products in adult patients with atopic dermatitis in Croatia. J. Eur. Acad. Dermatol Venereol. 34, 1789–1794. doi:10.1111/jdv.16232
Kamata, Y., Taniguchi, A., Yamamoto, M., Nomura, J., Ishihara, K., Takahara, H., et al. (2009). Neutral cysteine protease bleomycin hydrolase is essential for the breakdown of deiminated filaggrin into amino acids. J. Biol. Chem. 284, 12829–12836. doi:10.1074/jbc.M807908200
Kampmeyer, C., Larsen-Ledet, S., Wagnkilde, M. R., Michelsen, M., Iversen, H. K. M., Nielsen, S. V., et al. (2022). Disease-linked mutations cause exposure of a protein quality control degron. Structure 30, 1245–1253.e5. doi:10.1016/j.str.2022.05.016
Karczewski, K. J., Francioli, L. C., Tiao, G., Cummings, B. B., Alföldi, J., Wang, Q., et al. (2020). The mutational constraint spectrum quantified from variation in 141,456 humans. Nature 581 (581), 434–443. doi:10.1038/s41586-020-2308-7
Kats, I., Khmelinskii, A., Kschonsak, M., Huber, F., Knieß, R. A., Bartosik, A., et al. (2018). Mapping degradation signals and pathways in a eukaryotic N-terminome. Mol. Cell 70, 488–501. doi:10.1016/j.molcel.2018.03.033
Kezic, S., O'Regan, G. M., Yau, N., Sandilands, A., Chen, H., Campbell, L. E., et al. (2011). Levels of filaggrin degradation products are influenced by both filaggrin genotype and atopic dermatitis severity. Allergy 66, 934–940. doi:10.1111/j.1398-9995.2010.02540.x
King, B., Trimarchi, T., Reavie, L., Xu, L., Mullenders, J., Ntziachristos, P., et al. (2013). The ubiquitin ligase FBXW7 modulates leukemia-initiating cell activity by regulating MYC stability. Cell 153, 1552–1566. doi:10.1016/j.cell.2013.05.041
Klinkradt, S., Naudé, R. J., Muramoto, K., and Oelofsen, W. (1997). Purification and characterization of proteasome from ostrich liver. Int. J. Biochem. Cell Biol. 29, 611–622. doi:10.1016/s1357-2725(96)00143-4
Koepp, D. M., Schaefer, L. K., Ye, X., Keyomarsi, K., Chu, C., Harper, J. W., et al. (2001). Phosphorylation-dependent ubiquitination of cyclin E by the SCFFbw7 ubiquitin ligase. Sci. (1979) 294, 173–177. doi:10.1126/science.1065203
Komander, D., and Rape, M. (2012). The ubiquitin code. Annu. Rev. Biochem. 81, 203–229. doi:10.1146/annurev-biochem-060310-170328
Koren, I., Timms, R. T., Kula, T., Xu, Q., Li, M. Z., and Elledge, S. J. (2018). The eukaryotic proteome is shaped by E3 ubiquitin ligases targeting C-terminal degrons. Cell 173, 1622–1635. doi:10.1016/j.cell.2018.04.028
Koseki, R., Morii, W., Noguchi, E., Ishikawa, M., Yang, L., Yamamoto-Hanada, K., et al. (2019). Effect of filaggrin loss-of-function mutations on atopic dermatitis in young age: A longitudinal birth cohort study. J. Hum. Genet. 64, 911–917. doi:10.1038/s10038-019-0628-y
Kravtsova-Ivantsiv, Y., and Ciechanover, A. (2012). Non-canonical ubiquitin-based signals for proteasomal degradation. J. Cell Sci. 125, 539–548. doi:10.1242/jcs.093567
Krieg, P., Schuppler, M., Koesters, R., Mincheva, A., Lichter, P., and Marks, F. (1997). Repetin (rptn), a new member of the “fused gene” subgroup within the S100 gene family encoding a murine epidermal differentiation protein. Genomics 43, 339–348. doi:10.1006/geno.1997.4818
Kuechle, M. K., Presland, R. B., Lewis, S. P., Fleckman, P., and Dale, B. A. (2000). Inducible expression of filaggrin increases keratinocyte susceptibility to apoptotic cell death. Cell Death Differ. 7, 566–573. doi:10.1038/sj.cdd.4400687
Kypriotou, M., Huber, M., and Hohl, D. (2012). The human epidermal differentiation complex: Cornified envelope precursors, S100 proteins and the ‘fused genes’ family. Exp. Dermatol 21, 643–649. doi:10.1111/j.1600-0625.2012.01472.x
Kyte, J., and Doolittle, R. F. (1982). A simple method for displaying the hydropathic character of a protein. J. Mol. Biol. 157, 105–132. doi:10.1016/0022-2836(82)90515-0
Larsen, F. S. (1993). Atopic dermatitis: A genetic-epidemiologic study in a population-based twin sample. J. Am. Acad. Dermatol 28, 719–723. doi:10.1016/0190-9622(93)70099-f
Lauwers, E., Jacob, C., and Andre, B. (2009). K63-linked ubiquitin chains as a specific signal for protein sorting into the multivesicular body pathway. J. Cell Biol. 185, 493–502. doi:10.1083/jcb.200810114
Lee, K. E., Heo, J. E., Kim, J. M., and Hwang, C. S. (2016). N-terminal acetylation-targeted N-end rule proteolytic system: The Ac/N-end rule pathway. Mol. Cells 39, 169–178. doi:10.14348/molcells.2016.2329
Lee, S. C., Wang, M., McBride, O. W., O'Keefe, E. J., Kim, I. G., and Steinert, P. M. (1993). Human trichohyalin gene is clustered with the genes for other epidermal structural proteins and calcium-binding proteins at chromosomal locus 1q21. J. Investigative Dermatology 100, 65–68. doi:10.1111/1523-1747.ep12354504
Leyvraz, C., Charles, R. P., Rubera, I., Guitard, M., Rotman, S., Breiden, B., et al. (2005). The epidermal barrier function is dependent on the serine protease CAP1/Prss8. J. Cell Biol. 170, 487–496. doi:10.1083/jcb.200501038
List, K., Szabo, R., Wertz, P. W., Segre, J., Haudenschild, C. C., Kim, S. Y., et al. (2003). Loss of proteolytically processed filaggrin caused by epidermal deletion of Matriptase/MT-SP1. J. Cell Biol. 163, 901–910. doi:10.1083/jcb.200304161
Liu, J., Tokheim, C., Lee, J. D., Gan, W., North, B. J., Liu, X. S., et al. (2021). Genetic fusions favor tumorigenesis through degron loss in oncogenes. Nat. Commun. 12, 6704–6715. doi:10.1038/s41467-021-26871-y
Logli, E., Marzuolo, E., D'Agostino, M., Conti, L. A., Lena, A. M., Diociaiuti, A., et al. (2022). Proteasome-mediated degradation of keratins 7, 8, 17 and 18 by mutant KLHL24 in a foetal keratinocyte model: Novel insight in congenital skin defects and fragility of epidermolysis bullosa simplex with cardiomyopathy. Hum. Mol. Genet. 31, 1308–1324. doi:10.1093/hmg/ddab318
Lu, J., Wu, T., Zhang, B., Liu, S., Song, W., Qiao, J., et al. (2021). Types of nuclear localization signals and mechanisms of protein import into the nucleus. Cell Commun. Signal. 19, 60–10. doi:10.1186/s12964-021-00741-y
Lucas, X., and Ciulli, A. (2017). Recognition of substrate degrons by E3 ubiquitin ligases and modulation by small-molecule mimicry strategies. Curr. Opin. Struct. Biol. 44, 101–110. doi:10.1016/j.sbi.2016.12.015
Luger, T., Amagai, M., Dreno, B., Dagnelie, M. A., Liao, W., Kabashima, K., et al. (2021). Atopic dermatitis: Role of the skin barrier, environment, microbiome, and therapeutic agents. J. Dermatol Sci. 102, 142–157. doi:10.1016/j.jdermsci.2021.04.007
Madeira, F., Pearce, M., Tivey, A. R. N., Basutkar, P., Lee, J., Edbali, O., et al. (2022). Search and sequence analysis tools services from EMBL-EBI in 2022. Nucleic Acids Res. 50, W276–W279. doi:10.1093/nar/gkac240
Majetschak, M. (2011). Extracellular ubiquitin: Immune modulator and endogenous opponent of damage-associated molecular pattern molecules. J. Leukoc. Biol. 89, 205–219. doi:10.1189/jlb.0510316
Mao, J. H., Kim, I. J., Wu, D., Climent, J., Kang, H. C., DelRosario, R., et al. (2008). FBXW7 targets mTOR for degradation and cooperates with PTEN in tumor suppression. Sci. (1979) 321, 1499–1502. doi:10.1126/science.1162981
Marenholz, I., Heizmann, C. W., and Fritz, G. (2004). S100 proteins in mouse and man: From evolution to function and pathology (including an update of the nomenclature). Biochem. Biophys. Res. Commun. 322, 1111–1122. doi:10.1016/j.bbrc.2004.07.096
Marenholz, I., Nickel, R., Rüschendorf, F., Schulz, F., Esparza-Gordillo, J., Kerscher, T., et al. (2006). Filaggrin loss-of-function mutations predispose to phenotypes involved in the atopic march. J. Allergy Clin. Immunol. 118, 866–871. doi:10.1016/j.jaci.2006.07.026
Margottin-Goguet, F., Hsu, J. Y., Loktev, A., Hsieh, H. M., Reimann, J. D. R., and Jackson, P. K. (2003). Prophase destruction of Emi1 by the SCF(betaTrCP/Slimb) ubiquitin ligase activates the anaphase promoting complex to allow progression beyond prometaphase. Dev. Cell 4, 813–826. doi:10.1016/s1534-5807(03)00153-9
Marshall, D., Hardman, M. J., Nield, K. M., and Byrne, C. (2001). Differentially expressed late constituents of the epidermal cornified envelope. Proc. Natl. Acad. Sci. U. S. A. 98, 13031–13036. doi:10.1073/pnas.231489198
Martínez-Torres, I., Tepale-Segura, A., Castro-Escamilla, O., Cancino-Diaz, J. C., Rodríguez-Martínez, S., Perez-Tapia, S. M., et al. (2022). The protective role of pVHL in imiquimod-induced psoriasis-like skin inflammation. Int. J. Mol. Sci. 23, 5226. doi:10.3390/ijms23095226
Matsui, T., Miyamoto, K., Kubo, A., Kawasaki, H., Ebihara, T., Hata, K., et al. (2011). SASPase regulates stratum corneum hydration through profilaggrin-to-filaggrin processing. EMBO Mol. Med. 3, 320–333. doi:10.1002/emmm.201100140
Mattiroli, F., and Sixma, T. K. (2014). Lysine-targeting specificity in ubiquitin and ubiquitin-like modification pathways. Nat. Struct. Mol. Biol. 21, 308–316. doi:10.1038/nsmb.2792
McClellan, A. J., Laugesen, S. H., and Ellgaard, L. (2019). Cellular functions and molecular mechanisms of non-lysine ubiquitination. Open Biol. 9, 190147. doi:10.1098/rsob.190147
McDowell, G. S., and Philpott, A. (2013). Non-canonical ubiquitylation: Mechanisms and consequences. Int. J. Biochem. Cell Biol. 45, 1833–1842. doi:10.1016/j.biocel.2013.05.026
Meyer, H. J., and Rape, M. (2014). Enhanced protein degradation by branched ubiquitin chains. Cell 157, 910–921. doi:10.1016/j.cell.2014.03.037
Miajlovic, H., Fallon, P. G., Irvine, A. D., and Foster, T. J. (2010). Effect of filaggrin breakdown products on growth of and protein expression by Staphylococcus aureus. J. Allergy Clin. Immunol. 126, 1184–1190. doi:10.1016/j.jaci.2010.09.015
Millar, A. H., Heazlewood, J. L., Giglione, C., Holdsworth, M. J., Bachmair, A., and Schulze, W. X. (2019). The scope, functions, and dynamics of posttranslational protein modifications. Annu. Rev. Biochem. 70, 119. doi:10.1146/annurev-arplant-050718-100211
Mischke, D., Korge, B. P., Marenholz, I., Volz, A., and Ziegler, A. (1996). Genes encoding structural proteins of epidermal cornification and S100 calcium-binding proteins form a gene complex (‘epidermal differentiation complex’) on human chromosome 1q21. J. Invest. Dermatol 106, 989–992. doi:10.1111/1523-1747.ep12338501
Miyachi, Y., Yoshimura, N., Suzuki, S., Hamakubo, T., Kannagi, R., Imamura, S., et al. (1986). Biochemical demonstration and immunohistochemical localization of calpain in human skin. J. Investigative Dermatology 86, 346–349. doi:10.1111/1523-1747.ep12285556
Mo, J.-S., Kim, M. Y., Han, S. O., Kim, I. S., Ann, E. J., Lee, K. S., et al. (2007). Integrin-linked kinase controls Notch1 signaling by down-regulation of protein stability through Fbw7 ubiquitin ligase. Mol. Cell Biol. 27, 5565–5574. doi:10.1128/MCB.02372-06
Moosbrugger-Martinz, V., Leprince, C., Méchin, M. C., Simon, M., Blunder, S., Gruber, R., et al. (2022). Revisiting the roles of filaggrin in atopic dermatitis. Int. J. Mol. Sci. 23, 5318. doi:10.3390/ijms23105318
Morar, N., Cookson, W. O. C. M., Harper, J. I., and Moffatt, M. F. (2007). Filaggrin mutations in children with severe atopic dermatitis. J. Invest. Dermatol 127, 1667–1672. doi:10.1038/sj.jid.5700739
Morice-Picard, F., Jonca, N., Pichery, M., Mermin, D., Leauté-Labrèze, C., Taïeb, A., et al. (2017). KLICK syndrome: Recognizable phenotype and hot-spot POMP mutation. J. Eur. Acad. Dermatology Venereol. 31, e154–e156. doi:10.1111/jdv.13898
Nachat, R., Méchin, M. C., Takahara, H., Chavanas, S., Charveron, M., Serre, G., et al. (2005). Peptidylarginine deiminase isoforms 1–3 are expressed in the epidermis and involved in the deimination of K1 and filaggrin. J. Investigative Dermatology 124, 384–393. doi:10.1111/j.0022-202X.2004.23568.x
Naeem, A. S., Zhu, Y., Di, W. L., Marmiroli, S., and O’Shaughnessy, R. F. L. (2015). AKT1-mediated Lamin A/C degradation is required for nuclear degradation and normal epidermal terminal differentiation. Cell Death Differ. 22, 2123–2132. doi:10.1038/cdd.2015.62
Nemoto-Hasebe, I., Akiyama, M., Nomura, T., Sandilands, A., McLean, W. H. I., and Shimizu, H. (2009). FLG mutation p.Lys4021X in the C-terminal imperfect filaggrin repeat in Japanese patients with atopic eczema. Br. J. Dermatology 161, 1387–1390. doi:10.1111/j.1365-2133.2009.09406.x
Nomura, T., Sandilands, A., Akiyama, M., Liao, H., Evans, A. T., Sakai, K., et al. (2007). Unique mutations in the filaggrin gene in Japanese patients with ichthyosis vulgaris and atopic dermatitis. J. Allergy Clin. Immunol. 119, 434–440. doi:10.1016/j.jaci.2006.12.646
Novak, N., Baurecht, H., Schäfer, T., Rodriguez, E., Wagenpfeil, S., Klopp, N., et al. (2008). Loss-of-function mutations in the filaggrin gene and allergic contact sensitization to nickel. J. Invest. Dermatol 128, 1430–1435. doi:10.1038/sj.jid.5701190
Oh, E., Akopian, D., and Rape, M. (2018). Principles of ubiquitin-dependent signaling. Annu. Rev. Cell Dev. Biol. 34, 137–162. doi:10.1146/annurev-cellbio-100617-062802
Onnis, G., Bourrat, E., Jonca, N., Dreyfus, I., Severino-Freire, M., Pichery, M., et al. (2018). KLICK syndrome: An unusual phenotype. Br. J. Dermatology 178, 1445–1446. doi:10.1111/bjd.16318
Orchard, S., Ammari, M., Aranda, B., Breuza, L., Briganti, L., Broackes-Carter, F., et al. (2014). The MIntAct project—IntAct as a common curation platform for 11 molecular interaction databases. Nucleic Acids Res. 42, D358–D363. doi:10.1093/nar/gkt1115
Ordureau, A., Sarraf, S. A., Duda, D. M., Heo, J. M., Jedrychowski, M. P., Sviderskiy, V. O., et al. (2014). Quantitative proteomics reveal a feedforward mechanism for mitochondrial PARKIN translocation and ubiquitin chain synthesis. Mol. Cell 56, 360–375. doi:10.1016/j.molcel.2014.09.007
Osawa, R., Konno, S., Akiyama, M., Nemoto-Hasebe, I., Nomura, T., Nomura, Y., et al. (2010). Japanese-specific filaggrin gene mutations in Japanese patients suffering from atopic eczema and asthma. J. Invest. Dermatol 130, 2834–2836. doi:10.1038/jid.2010.218
Ostrowska, H., Ostrowska, J. K., Worowski, K., and Radziwon, P. (2009). Human platelet 20S proteasome: Inhibition of its chymotrypsin-like activity and identification of the proteasome activator PA28. A Prelim. Rep. 14, 151–157. doi:10.1080/0953710031000092802
Oughtred, R., Rust, J., Chang, C., Breitkreutz, B. J., Stark, C., Willems, A., et al. (2021). The BioGRID database: A comprehensive biomedical resource of curated protein, genetic, and chemical interactions. Protein Sci. 30, 187–200. doi:10.1002/pro.3978
Palmer, C. N. A., Irvine, A. D., Terron-Kwiatkowski, A., Zhao, Y., Liao, H., Lee, S. P., et al. (2006). Common loss-of-function variants of the epidermal barrier protein filaggrin are a major predisposing factor for atopic dermatitis. Nat. Genet. 38, 441–446. doi:10.1038/ng1767
Park, S. E., Kim, J. M., Seok, O. H., Cho, H., Wadas, B., Kim, S. Y., et al. (2015). Control of mammalian g protein signaling by N-terminal acetylation and the N-end rule pathway. Sci. (1979) 347, 1249–1252. doi:10.1126/science.aaa3844
Passmore, L. A., Barford, D., and Harper, J. W. (2005). Purification and assay of the budding yeast anaphase-promoting complex. Methods Enzymol. 398, 195–219. doi:10.1016/S0076-6879(05)98017-8
Paternoster, L., Standl, M., Chen, C. M., Ramasamy, A., Bønnelykke, K., Duijts, L., et al. (2011). Meta-analysis of genome-wide association studies identifies three new risk loci for atopic dermatitis. Nat. Genet. 44, 187–192. doi:10.1038/ng.1017
Pearton, D. J., Dale, B. A., and Presland, R. B. (2002). Functional analysis of the profilaggrin N-terminal peptide: Identification of domains that regulate nuclear and cytoplasmic distribution. J. Invest. Dermatol 119, 661–669. doi:10.1046/j.1523-1747.2002.01831.x
Pearton, D. J., Nirunsuksiri, W., Rehemtulla, A., Lewis, S. P., Presland, R. B., and Dale, B. A. (2001). Proprotein convertase expression and localization in epidermis: Evidence for multiple roles and substrates. Exp. Dermatol 10, 193–203. doi:10.1034/j.1600-0625.2001.010003193.x
Peña Penabad, C., Pérez Arellano, J. L., Becker, E., Gutierrez de Diego, J., García Salgado, M. J., Valle, F. J., et al. (1998). Differential patterns of filaggrin expression in lamellar ichthyosis. Br. J. Dermatol 139, 958–964. doi:10.1046/j.1365-2133.1998.02549.x
Pigors, M., Common, J. E. A., Wong, X. F. C. C., Malik, S., Scott, C. A., Tabarra, N., et al. (2018). Exome sequencing and rare variant analysis reveals multiple filaggrin mutations in Bangladeshi families with atopic eczema and additional risk genes. J. Investigative Dermatology 138, 2674–2677. doi:10.1016/j.jid.2018.05.013
Presland, R. B., Boggess, D., Lewis, S. P., Hull, C., Fleckman, P., and Sundberg, J. P. (2000). Loss of normal profilaggrin and filaggrin in flaky tail (ft/ft) mice: An animal model for the filaggrin-deficient skin disease ichthyosis vulgaris. J. Invest. Dermatol 115, 1072–1081. doi:10.1046/j.1523-1747.2000.00178.x
Presland, R. B., Haydock, P. V., Fleckman, P., Nirunsuksiri, W., and Dale, B. A. (1992). Characterization of the human epidermal profilaggrin gene. Genomic organization and identification of an S-100-like calcium binding domain at the amino terminus. J. Biol. Chem. 267, 23772–23781. doi:10.1016/s0021-9258(18)35905-2
Presland, R. B., Kuechle, M. K., Lewis, S. P., Fleckman, P., and Dale, B. A. (2001). Regulated expression of human filaggrin in keratinocytes results in cytoskeletal disruption, loss of cell-cell adhesion, and cell cycle arrest. Exp. Cell Res. 270, 199–213. doi:10.1006/excr.2001.5348
Quek, L. S., Grasset, N., Jasmen, J. B., Robinson, K. S., and Bellanger, S. (2018). Dual role of the anaphase promoting complex/cyclosome in regulating stemness and differentiation in human primary keratinocytes. J. Investigative Dermatology 138, 1851–1861. doi:10.1016/j.jid.2018.02.033
Quintás-Cardama, A., and Cortes, J. (2009). Molecular biology of bcr-abl1–positive chronic myeloid leukemia. Blood 113, 1619–1630. doi:10.1182/blood-2008-03-144790
Rawlings, A. v., and Harding, C. R. (2004). Moisturization and skin barrier function. Dermatol Ther. 17, 43–48. doi:10.1111/j.1396-0296.2004.04s1005.x
Resing, K. A., Dale, B. A., and Walsh, K. A. (2002). Multiple copies of phosphorylated filaggrin in epidermal profilaggrin demonstrated by analysis of tryptic peptides. Biochemistry 24, 4167–4175. doi:10.1021/bi00336a053
Resing, K. A., Johnson, R. S., and Walsh, K. A. (1993). Characterization of protease processing sites during conversion of rat profilaggrin to filaggrin. Biochemistry 32, 10036–10045. doi:10.1021/bi00089a020
Resing, K. A., Johnson, R. S., and Walsh, K. A. (1995). Mass spectrometric analysis of 21 phosphorylation sites in the internal repeat of rat profilaggrin, precursor of an intermediate filament associated protein. Biochemistry 34, 9477–9487. doi:10.1021/bi00029a024
Resing, K. A., Walsh, K. A., and Dale, B. A. (1984). Identification of two intermediates during processing of profilaggrin to filaggrin in neonatal mouse epidermis. J. Cell Biol. 99, 1372–1378. doi:10.1083/jcb.99.4.1372
Rodríguez, E., Baurecht, H., Herberich, E., Wagenpfeil, S., Brown, S. J., Cordell, H. J., et al. (2009). Meta-analysis of filaggrin polymorphisms in eczema and asthma: Robust risk factors in atopic disease. J. Allergy Clin. Immunol. 123, 1361–1370. doi:10.1016/j.jaci.2009.03.036
Rodríguez, E., Illig, T., and Weidinger, S. (2008). Filaggrin loss-of-function mutations and association with allergic diseases. Pharmacogenomics 9, 399–413. doi:10.2217/14622416.9.4.399
Ruether, A., Stoll, M., Schwarz, T., Schreiber, S., and Fölster-Holst, R. (2006). Filaggrin loss-of-function variant contributes to atopic dermatitis risk in the population of Northern Germany. Br. J. Dermatol 155, 1093–1094. doi:10.1111/j.1365-2133.2006.07500.x
Sakabe, J. I., Yamamoto, M., Hirakawa, S., Motoyama, A., Ohta, I., Tatsuno, K., et al. (2013). Kallikrein-related peptidase 5 functions in proteolytic processing of profilaggrin in cultured human keratinocytes. J. Biol. Chem. 288, 17179–17189. doi:10.1074/jbc.M113.476820
Sandilands, A., O'Regan, G. M., Liao, H., Zhao, Y., Terron-Kwiatkowski, A., Watson, R. M., et al. (2006). Prevalent and rare mutations in the gene encoding filaggrin cause ichthyosis vulgaris and predispose individuals to atopic dermatitis. J. Invest. Dermatol 126, 1770–1775. doi:10.1038/sj.jid.5700459
Sandilands, A., Terron-Kwiatkowski, A., Hull, P. R., O'Regan, G. M., Clayton, T. H., Watson, R. M., et al. (2007). Comprehensive analysis of the gene encoding filaggrin uncovers prevalent and rare mutations in ichthyosis vulgaris and atopic eczema. Nat. Genet. 39, 650–654. doi:10.1038/ng2020
Schneider, C. A., Rasband, W. S., and Eliceiri, K. W. (2012). NIH image to ImageJ: 25 years of image analysis. Nat. Methods 9, 671–675. doi:10.1038/nmeth.2089
Scott, I. R., Harding, C. R., and Barrett, J. G. (1982). Histidine-rich protein of the keratohyalin granules. Source of the free amino acids, urocanic acid and pyrrolidone carboxylic acid in the stratum corneum. Biochim. Biophys. Acta 719, 110–117. doi:10.1016/0304-4165(82)90314-2
Seattle (2022). denovo-db, Seattle, WA. https://denovo-db.gs.washington.edu (accessed 08 26, 2022).
Shaik, S., Nucera, C., Inuzuka, H., Gao, D., Garnaas, M., Frechette, G., et al. (2012). SCFβ-TRCP suppresses angiogenesis and thyroid cancer cell migration by promoting ubiquitination and destruction of VEGF receptor 2. J. Exp. Med. 209, 1289–1307. doi:10.1084/jem.20112446
Shemorry, A., Hwang, C. S., and Varshavsky, A. (2013). Control of protein quality and stoichiometries by N-terminal acetylation and the N-end rule pathway. Mol. Cell 50, 540–551. doi:10.1016/j.molcel.2013.03.018
Sherrill, J. D., and Blanchard, C. (2014). Genetics of eosinophilic esophagitis. Dig. Dis. 32, 22–29. doi:10.1159/000357005
Shibata, E., Abbas, T., Huang, X., Wohlschlegel, J. A., and Dutta, A. (2011). Selective ubiquitylation of p21 and cdt1 by UBCH8 and UBE2G ubiquitin-conjugating enzymes via the CRL4 Cdt2 ubiquitin ligase complex. Mol. Cell Biol. 31, 3136–3145. doi:10.1128/MCB.05496-11
Sixt, S. U., and Dahlmann, B. (2008). Extracellular, circulating proteasomes and ubiquitin — incidence and relevance. Biochimica Biophysica Acta (BBA) - Mol. Basis Dis. 1782, 817–823. doi:10.1016/j.bbadis.2008.06.005
Smieszek, S. P., Welsh, S., Xiao, C., Wang, J., Polymeropoulos, C., Birznieks, G., et al. (2020). Correlation of age-of-onset of Atopic Dermatitis with Filaggrin loss-of-function variant status. Sci. Rep. 10, 1–11. doi:10.1038/s41598-020-59627-7
Smith, F. J. D., Irvine, A. D., Terron-Kwiatkowski, A., Sandilands, A., Campbell, L. E., Zhao, Y., et al. (2006). Loss-of-function mutations in the gene encoding filaggrin cause ichthyosis vulgaris. Nat. Genet. 38, 337–342. doi:10.1038/ng1743
Smits, J. P. H., Niehues, H., Rikken, G., van Vlijmen-Willems, I. M. J. J., van de Zande, G. W. H. J. F., Zeeuwen, P. L. J. M., et al. (2017). Immortalized N/TERT keratinocytes as an alternative cell source in 3D human epidermal models. Sci. Rep. 7, 1–14. doi:10.1038/s41598-017-12041-y
Squair, D. R., and Virdee, S. (2022). A new dawn beyond lysine ubiquitination. Nat. Chem. Biol. 18, 802–811. doi:10.1038/s41589-022-01088-2
Stefanovic-Barrett, S., Dickson, A. S., Burr, S. P., Williamson, J. C., Lobb, I. T., van den Boomen, D. J., et al. (2018). MARCH6 and TRC8 facilitate the quality control of cytosolic and tail-anchored proteins. EMBO Rep. 19, e45603. doi:10.15252/embr.201745603
Steinert, P. M., Cantieri, J. S., Teller, D. C., Lonsdale-Eccles, J. D., and Dale, B. A. (1981). Characterization of a class of cationic proteins that specifically interact with intermediate filaments. Proc. Natl. Acad. Sci. U. S. A. 78, 4097–4101. doi:10.1073/pnas.78.7.4097
Sybert, V. P., Dale, B. A., and Holbrook, K. A. (1985). Ichthyosis vulgaris: Identification of a defect in synthesis of filaggrin correlated with an absence of keratohyaline granules. J. Investigative Dermatology 84, 191–194. doi:10.1111/1523-1747.ep12264813
Szulc, N. A., Stefaniak, F., Piechota, M., Cappannini, A., Bujnicki, J. M., and Pokrzywa, W. (2022). Degronopedia - a web server for proteome-wide inspection of degrons. bioRxiv 05, 492622. doi:10.1101/2022.05.19.492622
Tabachnick, J. (1957). Urocanic acid, the major acid-soluble, ultraviolet-absorbing compound in Guinea pig epidermis. Arch. Biochem. Biophys. 70, 295–298. doi:10.1016/0003-9861(57)90107-8
Takada, K., Nasu, H., Hibi, N., Tsukada, Y., Shibasaki, T., Fujise, K., et al. (1997). Serum concentrations of free ubiquitin and multiubiquitin chains. Clin. Chem. 43, 1188–1195. doi:10.1093/clinchem/43.7.1188
Takahashi, M., Tezuka, T., and Katunuma, N. (1996). Filaggrin linker segment peptide and cystatin alpha are parts of a complex of the cornified envelope of epidermis. Arch. Biochem. Biophys. 329, 123–126. doi:10.1006/abbi.1996.0199
Takaishi, M., Makino, T., Morohashi, M., and Huh, N. H. (2005). Identification of human hornerin and its expression in regenerating and psoriatic skin. J. Biol. Chem. 280, 4696–4703. doi:10.1074/jbc.M409026200
Takeichi, T., and Akiyama, M. (2020). KLICK syndrome linked to a POMP mutation has features suggestive of an autoinflammatory keratinization disease. Front. Immunol. 11, 641. doi:10.3389/fimmu.2020.00641
Timms, R. T., Zhang, Z., Rhee, D. Y., Harper, J. W., Koren, I., and Elledge, S. J. (2019). A glycine-specific N-degron pathway mediates the quality control of protein N-myristoylation. Sci. (1979) 364. doi:10.1126/science.aaw4912
Tokheim, C., Wang, X., Timms, R. T., Zhang, B., Mena, E. L., Wang, B., et al. (2021). Systematic characterization of mutations altering protein degradation in human cancers. Mol. Cell 81, 1292–1308.e11. doi:10.1016/j.molcel.2021.01.020
Trotman, L. C., Wang, X., Alimonti, A., Chen, Z., Teruya-Feldstein, J., Yang, H., et al. (2007). Ubiquitination regulates PTEN nuclear import and tumor suppression. Cell 128, 141–156. doi:10.1016/j.cell.2006.11.040
Ugai, S. I., Tamura, T., Tanahashi, N., Takai, S., Komi, N., Chung, C. H., et al. (1993). Purification and characterization of the 26S proteasome complex catalyzing ATP-dependent breakdown of ubiquitin-ligated proteins from rat liver. J. Biochem. 113, 754–768. doi:10.1093/oxfordjournals.jbchem.a124116
Vahlquist, A., Pontén, F., and Pettersson, A. (1997). Keratosis linearis with ichthyosis congenita and sclerosing keratoderma (KLICK-syndrome): A rare, autosomal recessive disorder of keratohyaline formation? Acta Derm. Venereol. 77, 225–227. doi:10.2340/0001555577225227
van Damme, P., Lasa, M., Polevoda, B., Gazquez, C., Elosegui-Artola, A., Kim, D. S., et al. (2012). N-terminal acetylome analyses and functional insights of the N-terminal acetyltransferase NatB. Proc. Natl. Acad. Sci. U. S. A. 109, 12449–12454. doi:10.1073/pnas.1210303109
van den Oord, R. A. H. M., and Sheikh, A. (2009). Filaggrin gene defects and risk of developing allergic sensitisation and allergic disorders: Systematic review and meta-analysis. BMJ 339, b2433–b2488. doi:10.1136/bmj.b2433
van Roey, K., Dinkel, H., Weatheritt, R. J., Gibson, T. J., and Davey, N. E. (2013). The switches.ELM resource: A compendium of conditional regulatory interaction interfaces. Sci. Signal 6, rs7. doi:10.1126/scisignal.2003345
Varshavsky, A. (2019). N-degron and C-degron pathways of protein degradation. Proc. Natl. Acad. Sci. U. S. A. 116, 358–366. doi:10.1073/pnas.1816596116
Varshavsky, A. (2011). The N-end rule pathway and regulation by proteolysis. Protein Sci. 20, 1298–1345. doi:10.1002/pro.666
Wang, T., Wang, W., Wang, Q., Xie, R., Landay, A., and Chen, D. (2020). The E3 ubiquitin ligase CHIP in normal cell function and in disease conditions. Ann. N. Y. Acad. Sci. 1460, 3–10. doi:10.1111/nyas.14206
Wei, W., Jin, J., Schlisio, S., Harper, J. W., and Kaelin, W. G. (2005). The v-Jun point mutation allows c-Jun to escape GSK3-dependent recognition and destruction by the Fbw7 ubiquitin ligase. Cancer Cell 8, 25–33. doi:10.1016/j.ccr.2005.06.005
Weidinger, S., Illig, T., Baurecht, H., Irvine, A. D., Rodriguez, E., Diaz-Lacava, A., et al. (2006). Loss-of-function variations within the filaggrin gene predispose for atopic dermatitis with allergic sensitizations. J. Allergy Clin. Immunol. 118, 214–219. doi:10.1016/j.jaci.2006.05.004
Winston, J. T., Strack, P., Beer-Romero, P., Chu, C. Y., Elledge, S. J., and Harper, J. W. (1999). The SCFbeta-TRCP-ubiquitin ligase complex associates specifically with phosphorylated destruction motifs in IkappaBalpha and beta-catenin and stimulates IkappaBalpha ubiquitination in vitro. genesdev.cshlp.Org. 13, 270–283. doi:10.1101/gad.13.3.270
Wong, N., Chan, A., Lee, S. W., Lam, E., To, K. F., Lai, P. B. S., et al. (2003). Positional mapping for amplified DNA sequences on 1q21-q22 in hepatocellular carcinoma indicates candidate genes over-expression. J. Hepatol. 38, 298–306. doi:10.1016/s0168-8278(02)00412-9
Wu, Z., Latendorf, T., Meyer-Hoffert, U., and Schröder, J.-M. (2011). Identification of trichohyalin-like 1, an s100 fused-type protein selectively expressed in hair follicles. Sch. Arch. Org. 131, 1761–1763. doi:10.1038/jid.2011.71
Xu, F., Li, J., Ni, M., Cheng, J., Zhao, H., Wang, S., et al. (2021). FBW7 suppresses ovarian cancer development by targeting the N 6-methyladenosine binding protein YTHDF2. Mol. Cancer 20, 45. doi:10.1186/s12943-021-01340-8
Yamamoto-Tanaka, M., Makino, T., Motoyama, A., Miyai, M., Tsuboi, R., and Hibino, T. (2014). Multiple pathways are involved in DNA degradation during keratinocyte terminal differentiation. Cell Death Dis. 5, e1181. doi:10.1038/cddis.2014.145
Yamazaki, M., Ishidoh, K., Suga, Y., Saido, T. C., Kawashima, S., Suzuki, K., et al. (1997). Cytoplasmic processing of human profilaggrin by active μ-calpain. Biochem. Biophys. Res. Commun. 235, 652–656. doi:10.1006/bbrc.1997.6809
Yamazaki, S., Uchiumi, A., and Katagata, Y. (2012). Hsp40 regulates the amount of keratin proteins via ubiquitin-proteasome pathway in cultured human cells. Int. J. Mol. Med. 29, 165–168. doi:10.3892/ijmm.2011.826
Yeh, C. H., Bellon, M., and Nicot, C. (2018). FBXW7: A critical tumor suppressor of human cancers. Mol. Cancer 17, 115–119. doi:10.1186/s12943-018-0857-2
Yen, H. C. S., Xu, Q., Chou, D. M., Zhao, Z., and Elledge, S. J. (2008). Global protein stability profiling in mammalian cells. Sci. (1979) 322, 918–923. doi:10.1126/science.1160489
Yoo, Y. D., Mun, S. R., Ji, C. H., Sung, K. W., Kang, K. Y., Heo, A. J., et al. (2018). N-terminal arginylation generates a bimodal degron that modulates autophagic proteolysis. Proc. Natl. Acad. Sci. U. S. A. 115, E2716–E2724. doi:10.1073/pnas.1719110115
Zhao, G., Li, K., Li, B., Wang, Z., Fang, Z., Wang, X., et al. (2020). Gene4Denovo: An integrated database and analytic platform for de novo mutations in humans. Nucleic Acids Res. 48, D913–D926. doi:10.1093/nar/gkz923
Zhao, X. P., and Elder, J. T. (1997). Positional cloning of novel skin-specific genes from the human epidermal differentiation complex. Genomics 45, 250–258. doi:10.1006/geno.1997.4952
Zhuang, M., Calabrese, M. F., Liu, J., Waddell, M. B., Nourse, A., Hammel, M., et al. (2009). Structures of SPOP-substrate complexes: Insights into molecular architectures of BTB-cul3 ubiquitin ligases. Mol. Cell 36, 39–50. doi:10.1016/j.molcel.2009.09.022
Zieba, B. A., Henry, L., Lacroix, M., Jemaà, M., Lavabre-Bertrand, T., Meunier, L., et al. (2017). The proteasome maturation protein POMP increases proteasome assembly and activity in psoriatic lesional skin. J. Dermatol Sci. 88, 10–19. doi:10.1016/j.jdermsci.2017.04.009
Glossary
Keywords: atopic dermatitis, filaggrin, proteasome, degron, ubiquitination
Citation: Paul AA, Szulc NA, Kobiela A, Brown SJ, Pokrzywa W and Gutowska-Owsiak D (2023) In silico analysis of the profilaggrin sequence indicates alterations in the stability, degradation route, and intracellular protein fate in filaggrin null mutation carriers. Front. Mol. Biosci. 10:1105678. doi: 10.3389/fmolb.2023.1105678
Received: 22 November 2022; Accepted: 19 April 2023;
Published: 02 May 2023.
Edited by:
Sumera Zaib, University of Central Punjab, PakistanReviewed by:
Bertrand Fabre, UMR5546 Laboratoire de Recherche en Sciences Vegetales (LRSV), FranceLaura Lynne Eggink, Susavion Biosciences, Inc., United States
John Kenneth Hoober, Susavion Biosciences, Inc., United States
Copyright © 2023 Paul, Szulc, Kobiela, Brown, Pokrzywa and Gutowska-Owsiak. This is an open-access article distributed under the terms of the Creative Commons Attribution License (CC BY). The use, distribution or reproduction in other forums is permitted, provided the original author(s) and the copyright owner(s) are credited and that the original publication in this journal is cited, in accordance with accepted academic practice. No use, distribution or reproduction is permitted which does not comply with these terms.
*Correspondence: Wojciech Pokrzywa, d3Bva3J6eXdhQGlpbWNiLmdvdi5wbA==; Danuta Gutowska-Owsiak, ZGFudXRhLmd1dG93c2thLW93c2lha0B1Zy5lZHUucGw=
†ORCID: Argho Aninda Paul, orcid.org/0000-0002-6229-0598; Natalia A. Szulc, orcid.org/0000-0002-2991-3634; Adrian Kobiela, orcid.org/0000-0002-8957-5366; Sara J. Brown, orcid.org/0000-0002-3232-5251; Wojciech Pokrzywa, orcid.org/0000-0002-5110-4462; Danuta Gutowska-Owsiak, orcid.org/0000-0003-4503-2279
‡These authors share first authorship