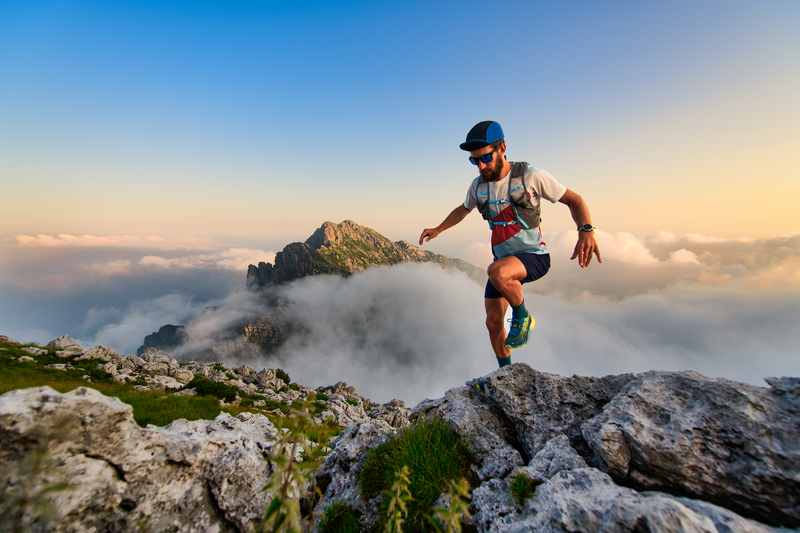
95% of researchers rate our articles as excellent or good
Learn more about the work of our research integrity team to safeguard the quality of each article we publish.
Find out more
REVIEW article
Front. Mol. Biosci. , 06 September 2022
Sec. Molecular Diagnostics and Therapeutics
Volume 9 - 2022 | https://doi.org/10.3389/fmolb.2022.986405
This article is part of the Research Topic Volume II: Fibrotic Tissue Remodeling as a Driver of Disease Pathogenesis View all 6 articles
Renal fibrosis is a common progressive manifestation of chronic kidney disease. This phenomenon of self-repair in response to kidney damage seriously affects the normal filtration function of the kidney. Yet, there are no specific treatments for the condition, which marks fibrosis as an irreversible pathological sequela. As such, there is a pressing need to improve our understanding of how fibrosis develops at the cellular and molecular levels and explore specific targeted therapies for these pathogenic mechanisms. It is now generally accepted that renal fibrosis is a pathological transition mediated by extracellular matrix (ECM) deposition, abnormal activation of myofibroblasts, and epithelial-mesenchymal transition (EMT) of renal tubular epithelial cells under the regulation of TGF-β. Histone deacetylases (HDACs) appear to play an essential role in promoting renal fibrosis through non-histone epigenetic modifications. In this review, we summarize the mechanisms of renal fibrosis and the signaling pathways that might be involved in HDACs in renal fibrosis, and the specific mechanisms of action of various HDAC inhibitors (HDACi) in the anti-fibrotic process to elucidate HDACi as a novel therapeutic tool to slow down the progression of renal fibrosis.
Chronic kidney disease (CKD) is a clinical syndrome characterized by persistent changes in kidney structure and function, which manifests as hypertension, edema, and oliguria, accompanied by abnormally elevated serum creatinine or blood urea nitrogen. The most common pathological manifestation of CKD is renal fibrosis (Romagnani et al., 2017). Renal fibrosis is chronic and progressive and seriously affects the glomerular filtration function. Data show that renal disease and interstitial fibrosis are present in approximately 50% of the over 70-year-old population and up to 10% of the world population. However, no specific anti-fibrotic drugs exist. Fortunately, recent advances have shed light on vital mechanisms of renal fibrosis development at the molecular level. Vorinostat (SAHA) is the first HDAC inhibitor approved by the FDA, mainly for the treatment of cutaneous T-cell lymphoma (Marks and Breslow, 2007). HDACi inhibits the proliferation and differentiation of tumors and can also promote apoptosis (Kim and Bae, 2011). Recent studies have shown that HDACi can also effectively inhibit the progression of renal fibrosis in various animal models such as ureteral ligation, diabetic nephropathy, ischemia-reperfusion, hypertensive nephropathy, and multiple sclerosis. As such, HDACs may be a potential target for antifibrotic effects, and HDACi may provide a clinically effective therapeutic to reverse renal fibrosis.
Renal fibrosis mainly stems from abnormal activation of myofibroblasts and their secretion of large amounts of ECM, such as α-smooth muscle actin (α-SMA), fibronectin (FN), and large deposits of collagen type I (collagen I), causing abnormal expansion of the renal tubular mesenchyme (Figure 1) (Humphreys, 2018). Tracking and localization assays using a genetic mouse model demonstrated the origin of mesenchymal myofibroblasts in the renal fibrosis model; half originated from the proliferative differentiation of resident fibroblasts with a contractile myofibroblast phenotype (Broekema et al., 2007; Meran and Steadman, 2011; LeBleu et al., 2013).
FIGURE 1. The activated TGF-β1/Smad pathway initiates the transcription of pro-fibrotic factors when the kidney damaged. At the same time, myofibroblasts in the renal interstitium are activated and produce large amounts of ECM. In response to TGF-β1, renal tubular epithelial cells undergo phenotypic conversion to fibroblasts (type 2 EMT). Immune cells infiltrate in the renal interstitium, triggering the deposition of ECM. Macrophages convert from type M1 to type M2 in response to TGF-β1, promoting fibrosis. BMP-7 activates the phosphorylation of Smad5, which plays a protective role against the TGF-β1/Smad pathway. Blocked G2/M phase of cell cycle in damaged tubular epithelial cells activates the JNK pathway and promotes the production of TGF-β1. Meanwhile, MAPK kinases activated by TGF-β1, p53, EGF, and Wnt/β-catenin pathways are also involved in the renal fibrosis process.
It is now generally accepted that the initial histological hallmark of renal fibrosis progression is the accumulation of myofibroblasts in the renal interstitium, which is typically characterized by the secretion of α-SMA and its further internalization to form stress fibers and the production of collagen I. The expression of α-SMA by renal tubular epithelial cells during EMT is used to identify EMT by immunostaining, thus demonstrating the existence of this process (Strutz et al., 1995; Iwano et al., 2002; Cheng et al., 2006; Tyler et al., 2006).
When the kidney is damaged, TGF-β1 is secreted into the renal interstitium by a variety of recruited inflammatory cells and almost all renal cells. TGF-β1plays a role in the development of renal fibrosis as a negative regulator of cells and tissue (Miyazono et al., 2001; Shi and Massagué, 2003). TGF-β1 is a crucial regulator of renal fibrosis. TGF-β1 synthesis usually takes the form of precursors and binds specifically to TGF-β binding protein. When the complex precursor is cleaved by proteases such as fibrinolytic enzymes or matrix metalloproteinases (MMP), the TGF-β1 precursor releases a latency-associated peptide (LAP) that is simultaneously separated from the TGF-β1 binding protein, at which point TGF-β1 is activated and binds to its receptor (Annes et al., 2003; Robertson et al., 2015). Inazaki et al. (2004) constructed a Smad3-deficient UUO mouse model and found that it displayed significantly reduced renal fibrosis compared to Smad3+/+ mice; the pathological manifestations were, in fact, comparable to those in wild-type mice, suggesting the importance of Smad3 in the signaling cascade response of TGF-β1. Based on existing work, it has been concluded that TGF-β1 induces the expression of target genes mainly through a Smad-dependent pathway (Smad-dependent) and a Smad-independent pathway (Smad-independent), and ultimately triggers renal fibrosis by activating myofibroblasts or causing excessive deposition of ECM. The classical TGF-β1/Smad pathway mainly binds TGF-β1R1 (ALK5) and TGF-β1R2 (BMPR-II) to phosphorylate further the regulatory R-Smad (Smad2 and Smad3), which forms a complex with the Co-Smad (Smad4) in the cytoplasm to ectopically initiate target gene transcription in the nucleus (Shi and Massagué, 2003; Meng et al., 2016). The independent Smad TGF -β1 pathway is mainly mediated through interaction with the three kinases of the mitogen-activated protein kinase (MAPK) family, p38, c-Jun terminal kinase (JNK), and extracellular signal-regulated kinase (ERK); numerous studies have shown that all three MAPKs are activated in chronic kidney injury (Adhikary et al., 2004; Stambe et al., 2004a; De Borst et al., 2007). These three MAPKs phosphorylate three serine (S) and one threonine (T) residues in the Smad2/Smad3 linkage region, thereby promoting the classical Smad pathway-dependent signaling of TGF-β1 (Kamato et al., 2013). It has been demonstrated in animal models that inhibition of p38, or blockade of JNK/c-Jun signaling, can effectively inhibit the progression of renal fibrosis (Stambe et al., 2004b; Ma et al., 2007; Ma et al., 2009; Müller et al., 2013). This suggests that, in renal fibrosis mediated by TGF-β1 as a central regulator, there exist highly complex interactions involving multiple signaling factors and their expression products. Our current understanding of these signaling mechanisms remains inadequate.
ECM is divided into two main categories: one is mesenchymal connective tissue with extracellular scaffolding function related to physiological activity, and the other is related to the formation of fibrosis, mainly composed of collagen, Laminins, fibronectin, and several Proteoglycans (Bonnans et al., 2014; Raeeszadeh-Sarmazdeh et al., 2020), Recent studies have shown that ECM is mainly produced by three functional areas of the kidney, specifically the glomeruli, tubules, and blood vessels, which participate in the production and degradation of interstitial ECM. Myofibroblasts are the most critical and are activated under pathological conditions, secrete large amounts of ECM, and exacerbate the development of renal fibrosis (Rayego-Mateos et al., 2021).
The deposition of ECM is an essential component of renal fibrosis pathology. The process is a double-edged sword in the development of renal fibrosis. On the one hand, the deposition of ECM accelerates the process of renal fibrosis. On the other hand, ECM interacts with cytokines and growth factors to enhance or inhibit renal fibrosis. ECM production is accompanied by the release of many soluble molecules, which directly or indirectly bind Toll-like receptors (TLR2/4) expressed on immune cells, and further activate the NF-κB signaling pathway to mediate the production of various cytokines and chemokines, forming a pro-fibrosis vicious cycle. Conversely, the soluble core can inhibit the TGF-β1 signaling pathway by inhibiting apoptosis of residual renal tubular epithelial cells by binding to insulin-like growth factor receptor (IGF-1) and increasing fibroblast production of Fibrillin 1. It can also inhibit CTGF-mediated production of collagen III and fibronectin in fibroblasts, thus exerting antifibrotic effects (Nastase et al., 2018).
There is ongoing debate as to whether EMT occurrs during renal fibrosis. Lineage tracing studies have demonstrated that EMT does not occur in vivo. However, type 2 EMT has been suggested to play an important role in fibrosis, with renal tubular epithelial cells acquiring a mesenchymal fibroblast-like phenotype and secreting extracellular matrix such as collagen fibers (Marconi et al., 2021). Zeisberg et al. (Zeisberg and Kalluri, 2004; Zeisberg and Kalluri, 2008) summarized the importance of EMT involvement in renal fibrogenesis. Firstly, TGF-β1 was shown to couple with the type I receptor ALK-5 and type II receptor serine/threonine kinase receptor (BMPR-II) in renal tubular epithelial cells to further phosphorylate and activate Smad2/3. This activated Smad2/3 further bound to Smad4 and translocated into the nucleus, inducing transcriptional effects. At the same time, TGF-β1 can also enhance the Smad pathway through the JNK and RhoA pathways. The TGF-β1-mediated signaling pathway triggers a series of morphological transformations such as the formation of fibrous bundles in the renal tubular epithelium, the reduction or even disappearance of the apical brush border (likely due to TGF-β1-mediated downregulation of E-cadherin), the destruction of the basement membrane, and the disappearance of tight junctions between the epithelium.
During renal tissue repair, multiple immune cells are involved in the inflammatory and fibrotic processes. Macrophage polarization plays an important role in chronic kidney injury, mainly involving the infiltration of M1 macrophages in the early stages of kidney injury in response to inflammation and cellular damage. In the late stages, the infiltration of M2 (M2b, M2C) macrophages occurs as part of the tissue repair process. In chronic kidney disease, macrophages become polarized from the M1 to M2 phenotype by pro-fibrotic factors such as TGF-β1, and their massive infiltration into the renal interstitium promotes the deposition of ECM. At the same time, their secreted cytokines, such as IL-1, MMP (2, 9, 12), platelet-derived growth factor, and other regulatory factors, further promote the proliferation and activation of fibroblasts, which intensify the progression of renal fibrosis (Tang et al., 2019). Zhang et al. reported that various T cell subsets mediate glomerular injury and pro-fibrotic mechanisms during renal inflammation, with tissue-associated γδ T cells playing an important role in the development of renal interstitial fibrosis (Zhang and Zhang, 2020). γδ T cells further activate myofibroblasts and promote ECM deposition by producing IL-17A in the renal interstitium in chronic kidney disease (Peng et al., 2015; Law et al., 2019). CD4+T lymphocytes can produce TGF-β1, thereby modulating myofibroblasts, and can also promote the secretion of PDGF, TGF-β1, CTGF, fibroblast growth factor-2 (FGF-2), and other cytokines and growth factors by activating macrophages or renal tubular epithelial cells to induce the proliferation and activation of fibroblasts, ultimately resulting in the deposition of ECM (Nikolic-Paterson, 2010).
Bone morphogenetic protein-7 (BMP-7), a member of the transforming growth factor TGF -β superfamily, reverses TGF-β1-induced EMT and epithelial cell injury in a mouse model of chronic kidney injury. BMP-7 activates Smad5 by coupling to the type I receptor ALK-3 and the type II receptor (BMPR-II) of renal tubular epithelial cells. The phosphorylated Smad5 further binds to Smad4, causing upregulation of E-cadherin and intercellular tight junction protein (ZO-1) expression, resulting in the reversal of renal fibrosis (Zeisberg et al., 2003; Zeisberg and Kalluri, 2004).
Li et al. (Yang et al., 2010) constructed three models of renal injury, namely ischemia-reperfusion injury (IRI), aristolochic acidosis (AAN), and unilateral ureteral ligation (UUO), and found a significant correlation between the development of renal fibrosis and the arrest of renal tubular epithelial cells in the G2/M cell cycle DNA damage checkpoint phase. When renal tubular epithelial cells are damaged, a large number of renal epithelial cells become arrested in the G2/M phase via the activation of the protein kinases Chk1 and Chk2, which are downstream of the ATM-ATR signaling pathway. At the same time, the G2/M phase arrest activates the JNK signaling pathway, which mediates the production of many pro-fibrotic factors, such as TGF-β and CTGF, thus promoting the development of renal fibrosis.
p53, a tumor suppressor gene, also plays an essential regulatory role in renal fibrosis. TGF-β can activate serine protein kinase (ATM) through phosphorylation of ROS signaling, which allows activation of p53 phosphorylation and promotes the formation of the p53/Smad3 complex, which translocates to the nucleus to induce transcription of fibrogenic factors (Meng et al., 2016).
Renal pathological biopsies from ESRD and various renal diseases show that renal fibrosis is associated with elevated connective tissue growth factor (CTGF) expression, and is associated with elevated TGF-β1. It was found that the CTGF mainly originated from α-SMA-positive myofibroblasts, suggesting that myofibroblasts synthesize CTGF by autocrine or paracrine means following induction by TGF-β1. The elevated CTGF promotes the development of renal interstitial fibrosis by increasing the phosphorylation of R-Smad (Smad2/Smad3) and promoting TGF-β1 signaling (Toda et al., 2018). CTGF may be involved in the G2/M phase arrest of renal tubular epithelial cells, indirectly promoting the development of fibrosis (Yang et al., 2010).
Mammalian target of rapamycin (mTOR), a serine/threonine-protein kinase, can be activated during kidney damage. Current studies suggest that TGF-β helps protect mTOR from degradation by inhibiting Deptor (an inhibitor of mTOR) in a Smad-dependent manner, and also activates mTOR1 and mTOR2 by inducing the PI3K/AKT signaling pathway in renal fibrosis (Lieberthal and Levine, 2009; Lieberthal and Levine, 2012; Das et al., 2013).
The epidermal growth factor receptor (EGFR) is a tyrosine kinase that binds to EGF and can specifically phosphorylate and activate Smad3 (Meng et al., 2016). In cultured renal mesenchymal fibroblasts, EFGR was found to promote EMT formation through activation of STAT3 and the ERK (1/2) pathway (Liu et al., 2012). TGF-β1 can also activate EFGR via tyrosine kinase Src; blocking of c-Src was found to reduce EFGR and TGF-β1 activation and thus renal fibrosis injury in an animal model of UUO (Samarakoon et al., 2013; Yan et al., 2016).
The Wnt/β-catenin signaling pathway can be activated during renal injury and promotes the expression of many genes associated with fibrosis such as Snail 1, Fibrinogen activator inhibitor (PAL-1), and MMP-7, which promote the development of fibrosis (Hao et al., 2011; Tan et al., 2011). The Wnt-specific antagonist Dickkopf-1 significantly inhibits the accumulation of β-catenin and suppresses the expression of pro-fibrotic factors and the activation of myofibroblasts (He et al., 2009). Wnt/β-catenin may potentially interact with TGF-β/Smad in renal fibrosis, however, the specific intrinsic association between the two pathways needs to be elucidated by further experimental studies.
The histone deacetylase family mediates chromatin compression by deacetylating histones inside the cell, ultimately repressing gene transcription. In contrast, histone acetyltransferases (HATs) promote gene expression by acetylating histones and thereby spreading chromatin. However, it has been shown that HDACs can deacetylate and modulate cytokines other than histones to produce various biological effects (Chen et al., 2001; Hubbert et al., 2002; Simonsson et al., 2005).
Histone deacetylases are typically categorized into four classes: class I (HDAC1, 2, 3, and 8), class II containing class IIa (HDAC4, 5, 7, 9) and class IIb (HDAC6, HDAC10), class III (sir-like proteins; Sirt1-7), and class IV is the recently discovered HDAC11. Activating class III HDAC molecules requires nicotinamide adenine dinucleotide (NAD+) as a cofactor. In contrast, the activation of the other three HDAC classes requires Zn+ as a cofactor. Notably, class I HDACs are mainly expressed in the kidney (de Ruijter et al., 2003).
HDACs are important epigenetic regulators that play an essential role in various renal diseases such as polycystic kidney disease, renal cell carcinoma, podocyte injury, and renal fibrosis (Liu, 2021). HDACs may act through different signaling pathways and produce different disease progression mechanisms. We summarize how HDACs promote renal fibrosis according to different HDACs subtypes in Table 1.
Dai et al. treated human mesangial cells (HMC) in vitro with isolated poly IgA1 (P-aIgA1) and found that HDAC1, HDAC2, and HDAC8 upregulated the expression and subsequent activation of TGF-β/Smad2/3 and Jak2/Stat3 signaling pathways, which promoted the proliferation of HMCs and facilitated the production of extracellular matrix deposition, such as collagen I. In addition, activation of the TGF-β1/Smad pathway induced the proliferation and activation of myofibroblasts and promoted the progression of renal interstitial fibrosis (Dai et al., 2016). Utilizing a diabetic mouse model, Ma et al. found that short-chain fatty acids (SCFAs) enhance the autophagic effect by inhibiting HDAC2, deregulating the inhibitory effect of HDAC2 on the autophagy-activated kinase ULK1 promoter, and then upregulating ULK1, thereby reducing renal fibrosis progression (Ma and Wang, 2022), In addition, in Npr1 (coding for receptor guanylyl cyclase-A [GC-A/NPRA]) haplotype male mice, HDAC1 and HDAC2 inhibit STAT1 binding to NF-κB by deacetylating STAT1, which leads to enhanced NF-κB binding activity to pro-inflammatory and pro-fibrotic genes, thereby promoting the progression of renal fibrosis (Kumar et al., 2017). In the 1990s, researchers extracted cDNA encoding HDAC3 from humans. Analysis of the amino acid sequence encoded by the open reading frame (ORF) of the HDAC3 gene revealed that its gene homology with HDAC1 and HDAC2 is around 50% (Yang et al., 1997; Mahlknecht et al., 1999).
Chen et al. (2021) constructed renal fibrosis models by using UUO and AAN. HDAC3 was found to be highly expressed in the mouse model, accompanied by low expression of the anti-aging protein klotho. After knocking down the HDAC3 gene, there was no significant difference in the expression of klotho between the experimental and sham-operated groups. The application of the TGF-β receptor inhibitor SB431542 to UUO mice revealed that it alleviated renal fibrosis. When the Smad3 inhibitor (SIS3) was added, HDAC3 expression was found to be reduced, thus indicating that the TGF-β/Smad3 signaling pathway upregulated HDAC3. Meanwhile, HDAC3 formed a complex by recruiting NCoR and NF-κB, which acted on klotho. In addition, HDAC3 inhibited the expression of klotho by recruiting NCoR and NF-κB to form a complex that acts on the klotho promoter, thereby promoting the progression of renal fibrosis, developing a pro-fibrotic pathway initiated by TGF-β with HDAC3 as an intermediary.
In a mouse model of chronic kidney disease utilizing adenine administration, histone deacetylase 3 (HDAC3) inhibits the acetylation of the klotho protein-associated transcription factor PPARγ, suppresses klotho protein expression and reduces secretory klotho concentrations in the blood, thereby increasing the progression of chronic kidney disease (Lin et al., 2017). In patients with focal segmental glomerulosclerosis (FSGS), there is significantly downregulated expression of the microRNA-30 family, which maintains glomerular homeostasis. In vitro studies revealed that elevated HDAC3, under the regulation of TGF-β, combined with Smad2/3 and NcoR to form an inhibitory complex near the miR-30d promoter. The final negative regulation of downregulated miR-30d, which aggravates the glomerular injury, will further exacerbate the renal injury. TSA (trigonellin A) and RGFP966 can alleviate the complex-mediated downregulation of these miRNA levels that maintain glomerular homeostasis, thus exerting a protective effect on the kidney (Liu et al., 2016).
Some investigators have demonstrated that HDAC3 creates a transcriptional repressor complex with SMRT and NcoR by successfully isolating the respective enzymatically active complex and demonstrating the interaction (Li et al., 2000; Wen et al., 2000). These studies suggest that the HDAC3 complexes may collaborate to negatively regulate organism functions. HDAC3 regulates TIMAP (TGF-β inhibited membrane-associated protein), a protein downstream of TGF-β that is enriched on the cell membrane surface. In renal fibrosis mice, TIMAP expression was shown to be reduced, likely due to the elevated expression of TGF-β, which promoted the high expression of HDAC3 and activated the Smad signaling pathway, which combined with HDAC3 to bind near the TIMAP promoter and inhibit the expression of TIMAP, which could dephosphorylate the light chain of myosin in M2 macrophages, thereby reducing their migration and phagocytosis (Yang et al., 2017). The activation of TGF-β and the M2 macrophage polarization promote the development of renal fibrosis (Mosser and Edwards, 2008; Casalena et al., 2012). This suggests that elevation of TGF-β enhances the activity and phagocytosis of M2 macrophages. However, this may further aggravate tissue damage and the progression of chronic disease. Therefore, inhibition of the TGF-β-HDAC3/Smad-TIMAP pathway may slow down the progression of TGF-β-mediated renal fibrosis.
The effect of HDAC8 on renal fibrosis, another important subtype of the type I HDAC family, has also been investigated. It was found that HDAC8 was highly expressed in the UUO kidney model and that HDAC8 specific selective inhibitor PCI34051 or silencing HDAC8 by siRNA alleviated fibrosis progression and inhibited EMT triggered by TGF-β, and that high HDAC8 expression in UUO kidneys could cause pro-fibrotic factors to be produced. Most importantly, elevated HDAC8 arrests renal tubular epithelial cells in the G2/M phase, which promotes the production of the pro-EMT factor (Snail), which is necessary to initiate EMT. However, the application of HDAC8 selective inhibitors can alleviate the EMT process. HDAC8 inhibitors may also reverse the decrease of BMP-7 and klotho, exerting an anti-fibrotic effect (Figure 2) (Zhang et al., 2020).
FIGURE 2. HDACs promote renal interstitial fibrosis in non-histone epigenetic modalities of regulation. When renal fibrosis begins, TGF-β expression is upregulated, and elevated TGF-β upregulates HADC expression in renal tubular epithelial cells, in a Smad2/3-dependent way. Then, HDACs translocate into the nucleus by recruiting multiple cytokines to form complexes that act near the gene promoter, mediating the expression of α-SMA and collagen I. On the other hand, elevated HDACs inhibit the anti-fibrotic factor BMP-7/Smad5 pathway and deregulate the protective factor of BMP-7, ultimately promoting the development of renal fibrosis.
Xiong et al. (2019) examined the effect of the selective class IIa HDACs inhibitor MC1568 on renal fibrosis with UUO. They found that renal injury was followed by a significant elevation of class IIa HDACs, HDAC4 was most significantly upregulated in renal tubular epithelial cells. Elevated class IIa HDACs enhanced the TGF-β1/Smad pathway and promoted the deposition of collagen I, α-SMA, and fibronectin. Meanwhile, class IIa HDACs enhanced the activation of phosphorylation of inducible transcription factor (NF-κB) and promoted the production of degradative enzymes with ECM degradation function, namely MMP-2 and MMP-9. In addition, they inhibited the down-regulation of two renal fibrosis protective factors (klotho and BMP-7). When the fibrotic mice were treated with MC1568 or siRNA, the above metabolic activities were inhibited and the production of MMP-2 and MMP-9 was enhanced, helping to degrade the extracellular matrix and reducing the progression of renal fibrosis. A study using a mouse model of Alport syndrome, which inhibits metalloproteinase (MMP) at different stages of renal disease, seems to reveal a pattern whereby elevated MMP promotes EMT and the progression of fibrosis in its early stages. In contrast, elevated MMP plays a role in degrading the extracellular matrix in the late stages of renal fibrosis, mitigating renal fibrosis (Zeisberg et al., 2006). Piceatannol, a phenolic compound, inhibits the expression of HDAC4 and HDAC5 in unilateral ureteral obstruction UUO and may also inhibit the p38-MAPK pathway to produce antifibrotic effects (Choi et al., 2016). The mechanism by which HDAC7 promotes fibrosis in renal fibroblasts has not been extensively studied. When TGF-β was applied to human lung fibroblasts, it was found that TGF-β, through the intermediate regulator HDAC7, deacetylates histone H3 near the promoter of the anti-fibrotic gene PGC1α, inhibiting the expression of anti-fibrotic genes such as PGC1α, thereby ultimately promoting the activation and proliferation of fibroblasts and causing massive deposition of ECM such as collagen I and fibronection (Jones et al., 2019). In Hepatic Stellate cell (HSC) cells, cytokines such as IL-6 and TNF promote the binding of HDAC7 to the anti-tumor gene cylindromatosis (CYLD), which recruits HDAC7 to the nucleus near the promoter of the hepatocyte growth factor (HGF). In the absence of CYLD, HGF expression is downregulated, ultimately triggering hepatocyte injury and promoting the progression of liver fibrosis (Pannem et al., 2014). In Peyronie’s disease (PD), the fibroblast expression of HDAC7 was significantly higher than normal. When HDAC7 was knocked down, the TGF-β1/Smad pathway was inhibited and the phosphorylation-activated Smad2/3 complex was unable to enter the nucleus, inhibiting myofibroblast activation, which reduced the deposition of extracellular matrix such as collagen I (Kang et al., 2018). This suggests that HDAC7 plays an important role as an intermediate factor in the progression of fibrosis. The correlation between HDAC9 and renal fibrosis is less studied, however, HDAC9 has been shown to promote vascular endothelial cell injury by acting on the p38 MAPK pathway (Kuang et al., 2021).
In a mouse model of hypertension generated using Angiotensin II (ANGII), Choi et al. (2015) demonstrated that the HDAC6 selective inhibitor (Tubastatin A) combined with a Smad3 knockdown inhibited TGF-β and ANG-induced renal fibrosis. It was shown that HDAC6 promotes the activation of Smad2/3 phosphorylation as well as increases the binding activity of R-Smad to the promoter of pro-fibrotic genes, increasing the expression of ANGII-induced collagen I, collagen III, fibronectin, and other extracellular matrices, as well as the expression and release of inflammatory factors such as monocyte chemotactic protein-1 (MCP-1) and fibrinogen activator inhibitor-1 (PAI-1). TubA or knockdown of HDAC6 inhibits the secretion of these inflammatory factors. At the same time, HDAC6 also enhances the binding activity of histone H4 to the collagen I promoter and promotes the deposition of type I collagen In the renal interstitium. HDAC6 has been found to play an important regulatory role in TGF-β-induced EMT. TGF-β increases the activity of HDAC6, and activated HDAC6 deacetylates the renal tubular epithelial cytoskeletal protein (α-tubulin). The acetylation of α-tubulin can be significantly upregulated, accompanied by a decrease in the degree of EMT. α-tubulin deacetylation modifications promote cytoskeletal reorganization during EMT formation (Shan et al., 2008; Gu et al., 2016). How TGF-β activates HDAC6 is not known. Perhaps the deacetylation modification of α-tubulin by HDAC6 provides the structural basis for EMT. In combination with the genetic reprogramming of renal tubular epithelial cells in response to TGF-β/Smad signaling, it leads to EMT, which may also be present during EMT in renal fibrosis. In UUO mice, HDAC1, 4, 5, 6, 10 upregulated expression and HDAC8 downregulated expression, which may exert pro-fibrotic effects through a TGF-β1/Smad independent pathway (Choi et al., 2016). However, the mechanism of action of class IIb HDACs in the fibrosis process still needs to be further explored.
The class III HDAC family members currently thought to be associated with the development of renal fibrosis mainly include SIRT1 and SIRT2; there is no consensus on the role of SIRT1 in renal fibrosis. SIRT1 is localized in the nucleus, and its activation is dependent on NAD+ as a coenzyme; resveratrol is an activator of SIRT1. Zerr et al. (2016) found in a mouse model of bleomycin-induced skin fibrosis and TGF-β receptor overexpression that SIRT1 was reduced under the regulation of overactivated TGF-β. The activation of SIRT1 enhances TGF-β-mediated extracellular matrix release and exacerbates the extent of the fibrotic response. Specific knockdown of the SIRT1 gene inhibits the TGF-β/Smad signaling pathway, and reduces Collagen release from fibroblasts and the fibrotic response. However, in a UUO mouse model, some investigators found that acetylation of Smad3 under the regulation of TGF-β appeared to mediate the renal fibrotic process, and SIRT1 could deacetylate Smad3, resulting in antifibrotic effects. Resveratrol produced antifibrotic effects by activating SIRT1, reducing the activation of myofibroblasts, the deposition of ECM, and other fibrotic responses (Li et al., 2010). These differences may be attributed to the different roles of SIRT1 in renal fibrosis. SIRT2 is localized in the nucleus. Its expression is significantly increased in the renal interstitium of fibrotic patients and the UUO mouse model. SIRT2 expression is elevated upon induction of TGF-β1 in fibroblasts. It promotes myofibroblast activation, as well as α-SMA collagen III, fibronectin, and other extracellular matrix deposition, a process that is significantly inhibited by applying the SIRT2-specific inhibitor AGK2. However, SIRT2 was not found to affect renal tubular epithelial-to-mesenchymal transition. At the same time, murine double minute2 (MDM2) was also found to be induced by TGF-β1 and may be involved in myofibrillar activation through activation of P53 and inhibition of its transcriptional activity. Inhibition of SIRT2 was followed by downregulation of MDM2. In contrast, inhibition of the SIRT-MDM2 interaction did not alter the TGF-β1-mediated increase in SIRT2 expression, suggesting that SIRT2 may be an upstream regulator of MDM2 and that it exerts a pro-fibrotic effect through MDM2 (He et al., 2018).
Ponnusamy et al. (2014) reported that the addition of SIRT1 and SIRT2 inhibitors (Sirtinol) or knockdown of SIRT1 and SIRT2 by siRNA in UUO mice significantly decreased proliferating cell nuclear antigen (PCNA) and cell cycle proteins (cyclinD1, cyclinE), which in turn inhibited fibroblast activation and proliferation, and inhibited α-SMA, collagen I, fibronectin, and other extracellular matrix deposition. When applied alone, the SIRT1 inhibitor EX527 or the SIRT2 inhibitor AGK2 was found to produce similar antifibrotic effects in cultured NRK-49F rat fibroblasts. In conclusion, there may be a complex regulatory network of SIRT1,2 involved in the development of renal fibrosis. Its pro-fibrotic mechanisms need to be further explored.
SIRT3 is mainly localized in the mitochondrial matrix and is the main regulator of organelle deacetylation. Marina Morigi summarized the regulatory mechanisms of different isoforms of SIRTs in renal physiology. SIRT1 and SIRT3 can exert protective effects against renal fibrosis by inhibiting inflammatory responses and apoptosis, and by regulating energy metabolism (Morigi et al., 2018). In the Ang II-induced hypertension mouse model, SIRT3 knockdown enhances the conversion of pericytes to fibroblasts, while inducing iron overload, decreasing glucose metabolism in cells, and causing ROS production, thus causing kidney tissue damage and aggravating the progression of renal fibrosis (Feng et al., 2020). The role of SIRT3 in deacetylation of pyruvate dehydrogenase E1α (PDHE1α) is closely related to metabolic reprogramming during renal fibrosis (Zhang et al., 2021). In CKD mice, mitochondria-targeted superoxide mimetic (Mito-TEMPO) can protect against renal fibrosis by slowing mitochondrial dysfunction and endoplasmic reticulum stress through the SIRT3-SOD2 pathway (Liu et al., 2018). The regulatory mechanisms of SIRT4 in renal fibrosis have not been fully established. SIRT4 acts as a deacetylase associated with the cell stress response. In metabolic reprogramming, it regulates various factors such as NAD+ and ATP-dependent protein kinase (AMPK), and exerts antioxidant damage and repair effects. SIRT4 can also regulate myofibroblast transdifferentiation and subsequent collagen fiber deposition by regulating glutamine (Gln) metabolism. SIRT4 can also promote fibrosis progression by increasing ROS levels in cardiac myocytes and sensitivity to Ang II responses to promote fibrosis progression (Luo et al., 2017; Mazumder et al., 2020). SIRT5 is also a mitochondria-localized deacetylase but few studies have been published on its regulatory mechanisms associated with chronic kidney disease and renal fibrosis. However, SIRT5 can reduce AKI injury by regulating the balance of mitochondrial and peroxisomal fatty acid oxidation in proximal renal tubular epithelial cells (Chiba et al., 2019). In renal tubular epithelial cells, SIRT6 binds to β-catenin and mediates histone deacetylation near the promoters of fibronectin, MMP7, and snail, exerting a protective effect against renal fibrosis by inhibiting their transcription (Cai et al., 2020; Gewin, 2020). In a caloric restriction (CR) mouse model, SIRT6 slows the development of renal fibrosis by inhibiting NF-κB signaling, which in turn slows the proliferation and senescence of WI38 fibroblasts (Zhang et al., 2016). SIRT7 has been shown to inhibit the TGF-β1/Smad signaling pathway and regulate fibrosis by deacetylating Smad4 and reducing Smad3 levels. Since the Wnt/β-catenin pathway plays a key role in the development of fibrosis, SIRT7 may regulate fibrosis progression by inhibiting the Wnt/β-catenin pathway (Chen et al., 2017; Li et al., 2022). However, our understanding of the specific regulatory mechanisms of SIRT7 in renal fibrosis is limited and this topic calls for significant research attention.
In renal tubular epithelial cells (RTECs), HDAC11 expression was upregulated after administration of Ang II; HDAC11 formed a complex with Activator protein 2 (AP-2α). Kruppel-like factor 15 (KLF15), a regulator of renal fibrosis, may play an anti-fibrotic role by inhibiting ERK/MAPK, TNK/MAPK, Wnt/β-catenin, and other pathways. Since the vicinity of the KLF15 promoter may contain AP-2α binding sites, the formation of the HDAC11- AP-2α complex inhibits KLF15 mRNA and protein levels, and the expression of collagen I, α-SMA, and other genes was increased, thus promoting the progression of renal fibrosis (Mao et al., 2020).
Smith et al. (2019) utilized an in vitro culture of fibroblasts from a UUO rat model, to show that TGF-β can reduce the acetylation of histone H3 in fibroblasts, resulting in the activation of multiple pro-fibrotic genes. This may arise from a reduced expression of histone acetylases (HAT) and elevated levels of histone deacetylases (HDACs), and reduced availability of Acetyl CoA in fibroblasts. The imbalance of H3 acetylation could be corrected via exogenous administration of citrate or the pan-HDAC inhibitor TSA, reducing the activation of myofibroblasts. In a rat fibroblast model (NRK-49F), histone H3 in the vicinity of the promoter of the Fibrogenic gene can be modified by TGF-β-induced deacetylation, a regulatory process that can be inhibited by the class I HDAC inhibitor valproic acid (VPA), thereby reducing myofibroblast activation and proliferation as well as the accumulation of α-SMA, collagen I, and fibronectin (Nguyễn-Thanh et al., 2018).
Previous studies have shown that aberrant acetylation and deacetylation of histones play an essential role in tumor proliferation and differentiation. Johnstone (2002) proposed that HDACi could inhibit the G2 to M cell cycle transition in tumor cells and induce a high expression of major histocompatibility complex (MHC) class I and II molecules, Intercellular Adhesion Molecule 1 (ICAM 1), and activating factor (CD40) in tumor cells, thereby increasing their immunogenicity and thus enhancing the body’s immune response to the tumor cells. The FDA has approved two non-specific HDAC targeting inhibitors, Vorinostat and Romidepsin, primarily for the treatment of cutaneous carcinoma and peripheral T-cell lymphoma (West and Johnstone, 2014). Bush and McKinsey (2010) suggested that the regulation of histone and non-histone deacetylation plays a critical epigenetic role in the pathogenesis of the renal disease. HDAC inhibitors can target a variety of HDAC-mediated transcription-related renal dysfunctions, such as EMT, aberrant activation of fibroblasts, ECM deposition, etc. Liu et al. (Liu and Zhuang, 2015) reported that HDAC inhibitors can delay the progression of renal fibrosis, reduce the formation of polycystic kidney disease, improve the condition of diabetic nephropathy, lupus nephropathy, and aristolochic nephropathy, and even play an important role in the anti-rejection reaction of transplanted kidneys in animal models. Therefore, HDAC inhibitors may be used to inhibit interstitial fibrosis, slow down the progression of fibrosis in kidneys, and slow down renal failure. In Table 2, we summarize published animal model experiments, which were primarily performed utilizing broad-spectrum HDAC inhibitors, and the molecular mechanisms involved in the anti-fibrotic process of HDACi which may eventually help us attenuate the progression of end-stage renal disease (Table 2). We also summarized the inhibitory activity of HDAC inhibitors associated with the slowing of renal fibrosis against different subtypes of HDACs, expressed as half-inhibitory concentrations IC50 values (Table 3) (Novotny-Diermayr et al., 2010; Lauffer et al., 2013; Ho et al., 2020; Bondarev et al., 2021). In the available clinical trials, the application of HDACi can trigger the risk of cardiotoxicity, blood cell suppression, gastrointestinal reactions, and even thrombosis, etc. We summarized the various adverse effects of HDACi (Table 4) (Bondarev et al., 2021; Nanau and Neuman, 2013; Knipstein and Gore, 2011; Smolewski and Robak, 2017; Jo et al., 2022; O'Connor et al., 2015; Siegel et al., 2009; Chu et al., 2015; Van Veggel et al., 2018; Yagishita et al., 2019; Fahey et al., 2019).
TABLE 3. IC50 values of HDAC inhibitors (Novotny-Diermayr et al., 2010; Lauffer et al., 2013; Ho et al., 2020; Bondarev et al., 2021).
In the UUO mouse model, the class I HDACs inhibitor, Valproic acid (VPA), inhibited the aberrant expression of the pro-fibrotic signal TGF-β1 and subsequent phosphorylation of Smad2/3 while upregulating the protective cytokine Smad7 (Nguyễn-Thanh et al., 2018). VPA significantly ameliorated fibrosis in a diabetic rat model by inhibiting TGF-β1 and similarly reduced extracellular matrix deposition of CTGF, α-SMA, collagen I, and fibronectin (Khan et al., 2015). Another class I HDACi, MS-275, also exerted antifibrotic effects in the UUO mouse model by inhibiting TGF-β1 elevation, probably because MS-275 inhibited the expression of TGF-βR1, thereby blocking the activation of phosphorylation of the downstream signal (Smad3) and reducing the deposition of ECM in the renal interstitium (Liu et al., 2013). Yang et al. (2019) found that the application of HDAC1 and HDAC2 inhibitor FK228 slowed down the fibrosis process mediated by the pro-fibrotic factor TGF-β1 in a UUO rat model. This study found that FK228 reduced the expression of ECMs such as α-SMA, collagen I, and fibronectin; this was mediated by TGF-β1. FK228 attenuated the activation and proliferation of renal interstitial fibroblasts and promoted their apoptosis; this may stem from FK228 inhibition of the expression of the cell cycle protein CyclinD1 in fibroblasts and prevention of G1 to S phase transition of fibroblasts during proliferation. FK228 also inhibits the phosphorylation activation of Smad2/3 in the classical Smad pathway and Smad-independent pathways (ERK, p38, PI3K/AKT), which attenuates renal interstitial fibrosis in the rat model. A novel pan-inhibitor of HDACs, CG200745 (CG), exerts antifibrotic effects mainly by inhibiting class I and class II HDACs in various models of renal injury. It was found that CG can attenuate the expression of TGF-β1 mRNA and the phosphorylation of Smad2/3 in UUO mice while downregulating the accumulation of ECMs such as α-SMA, collagen I, and fibronectin (Choi et al., 2018). In a mouse model of Alport Syndrome (AS), CG was found to reverse renal fibrosis by inhibiting the RAS-mediated inflammatory response and subsequent activation of TGF-β1, and by inhibiting the phosphorylation of Smad2/3/4 and activation of myofibroblasts (Suh et al., 2020). CG inhibits the activation of the TGF-β1/Smad pathway and reduces the deposition of α-SMA, fibronectin, and collagen I in the renal interstitium, exerting an anti-fibrotic effect in a hypertensive rat model. It also inhibits the expression of inflammatory cell chemokines MCP-1, ICAM-1, and VCAM-1, ultimately exerting an anti-inflammatory effect. In the presence of CG, TUNEL analysis of DNA damage showed a significant decrease in the number of positive cells, indicating that CG inhibits apoptosis in renal tubular epithelial cells (Bae et al., 2019). In a mouse model of bilateral renal ischemia-reperfusion injury (IRI), the administration of TSA or MS275 was also effective in inhibiting the development of renal fibrosis after IRI, which was closely related to the inhibition of the TGF-β1/Smad pathway by HDACi (Hyndman et al., 2019). SB939 (pracinostat) mainly inhibits class I, II, and IV HDACs and is currently in phase II clinical trials. It was found that the administration of SB939 in a rat renal mesenchymal fibroblast model (NRK-49F) can inhibit phosphorylation of ERK, p38, and PI3K/AKT; as such, SB939 exerts its antifibrotic effects by blocking TGF-β/Smad independent pathways (Kang et al., 2017).
BMP-7, a member of the TGF-β superfamily, protects against the TGF-β/Smad pathway during renal fibrosis. Sulforaphane (SFN), an inhibitor of HDAC2, can reverse renal interstitial fibrosis induced by high glucose (HG/Pal) by inhibiting HDAC2 and reactivating the BMP-7-Smad1/5/8 pathway in the mouse streptozotocin (STZ)-induced diabetes model. This suggests that upregulation of HDAC2 expression in a mouse model of diabetic nephropathy inhibits BMP-7, reduces E-cadherin formation, and promotes renal tubular epithelial-to-mesenchymal transition. In contrast, in DN, the HDAC2 inhibitor SFN reversed this process, which might prevent DN-induced renal fibrosis (Kong et al., 2021).
Yoshikawa et al. (2007) found in in vitro cultured human proximal tubular epithelial cells (RPTEC) that the HDAC inhibitor TSA did not reverse TGF-β1-induced phosphorylation of Smad2/3, but induced upregulation of BMP-7 and inhibitory differentiation factor (id-2). TSA reverses the TGF-β1/Smad signaling-induced down-regulation of E-cadherin and collagen I up-regulation while inhibiting the conversion of RPTEC to mesenchyme. Thereby, it enhances tubular homeostasis and delays the onset and progression of renal fibrosis. A similar study demonstrated that HDAC in a UUO mouse model regulates BMP-7 transcription. The inhibition of TGF-β1-induced Collagen-1, α1 chain (COLIα1), as well as α-SMA by TSA, was significantly reduced when the BMP-7 receptor inhibitor dorsomorphin was administered, suggesting that the HDAC inhibitor TSA counteracted TGF-β1-induced renal fibrosis by upregulating the transcription of BMP-7 (Manson et al., 2014).
It has been shown that TSA inhibits activation of fibroblasts in the rat renal interstitium and attenuates the progression of renal fibrosis in UUO. The mechanism may involve HDAC activation of the transcription factor STAT3, which undergoes nuclear translocation to promote the activation of fibroblasts in the renal interstitium. At the same time, STAT3 can promote the high expression of α-SMA and fibronectin, which promote the development of renal fibrosis in CKD, a process that can be alleviated by the specific STAT3 signaling pathway inhibitors AG490 or TSA (Pang et al., 2009). It has been shown that the All-trans-retinoic acid (ATRA) and HDAC inhibitors Sodium Butyrate (NaBu) can reduce the expression of pro-inflammatory and pro-fibrotic genes downstream of NF-κB by acetylating the transcription factor STAT1, which promotes STAT1 binding to NF-κB to form a complex. This ameliorated renal interstitial fibrosis in a haploid mouse model of Npr1 (Kumar et al., 2017).
Analysis of the renal interstitial macrophage phenotype in TSA-treated UUO mice revealed that TSA reduced the number of inducible nitric oxide synthase (iNOS)-positive M1-type macrophages in renal tissue, as well as C-type lectin domain family seven member A (CLEC7A)-positive M2a-type macrophages. These two macrophage phenotypes promote peritubular inflammation and myofibroblast activation, which further exacerbate inflammation by promoting tubular epithelial apoptosis and exacerbating immunocidal responses. However, at the same time, the number of signaling lymphocytic activation molecule (SLAM)-positive M2c macrophages in the renal interstitium was increased, which should result in anti-inflammatory effects on fibrosis formation. M2c inhibits the activation of myofibroblasts and the release of pro-fibrotic factors by promoting tubular reepithelialization and neovascularization in the damaged kidney. TSA inhibits the progression of inflammation in the injured kidney through the phenotypic transformation of macrophages M1-M2c, thus inhibiting the regression of renal tissue to fibrosis during the repair process (Tseng et al., 2020).
Chronic kidney disease seriously affects renal filtration function in patients. Its most typical pathological manifestation is fibrosis. As renal fibrosis progresses, there is a gradual loss of glomeruli and a gradual creep of blood creatinine, eventually resulting in renal failure. Although patients can benefit significantly from kidney transplantation, there is a shortage of available donors and organ rejection can lead to renal failure; many patients are forced to rely on long-term dialysis treatment. These limited treatment options have a significant impact on the morbidity and prognosis of CKD patients.
Therefore, there is a great need to identify anti-fibrosis targets at the molecular level. We found that the HDAC family may contribute to the development of renal fibrosis through non-histone epigenetic regulation. In animal models of renal fibrosis, HDACs mainly promote the TGF-β1/Smad and inhibit the BMP-7/Smad pathway. As a result, myofibroblast activation, ECM deposition, and EMT are promoted. In animal models, the application of HDACi inhibits the progression of interstitial fibrosis in the mouse kidney. Chidamide, a class I HDACi, under independent development in China, has been approved by the China Food and Drug Administration (CFDA) as a treatment for relapsed or refractory peripheral T cell lymphoma (Shi et al., 2017). We may speculate whether the application of such HDACis could similarly slow the progression of renal fibrosis in clinical patients. Such hypotheses need to be addressed by evidence from future clinical trials. However, clinical data show that the administration of HDACi is often accompanied by complications such as nausea and vomiting due to cytotoxicity (Minucci and Pelicci, 2006; Marks and Breslow, 2007). Cardiac arrhythmias, immune reactions, prolonged QT intervals, myelosuppression/thrombocytopenia, and even neurological damage and teratogenicity are known complications of HDACis (Campas-Moya, 2009; Ornoy, 2009). As such, there is an urgent need for clinical trials to identify the appropriate dose of antifibrotic treatment while screening for HDACis with high specificity and low side effects.
JW, JL, and XZ conceived and designed the manuscript. JW wrote the first draft of the manuscript. JL and XZ collected the data and drew the illustrative figures. JL, XZ, and MZ revised and polished the manuscript. HY and XH conducted this research study and revised the manuscript. All authors have read and approved the final version of this manuscript.
The authors would like to express their gratitude to EditSprings (https://www.editsprings.cn) for the expert linguistic services provided.
The authors declare that the research was conducted in the absence of any commercial or financial relationships that could be construed as a potential conflict of interest.
All claims expressed in this article are solely those of the authors and do not necessarily represent those of their affiliated organizations, or those of the publisher, the editors and the reviewers. Any product that may be evaluated in this article, or claim that may be made by its manufacturer, is not guaranteed or endorsed by the publisher.
Adhikary, L., ChowF., , Nikolic-Paterson, D. J., Stambe, C., Dowling, J., Atkins, R. C., et al. (2004). Abnormal p38 mitogen-activated protein kinase signalling in human and experimental diabetic nephropathy. Diabetologia 47 (7), 1210–1222. doi:10.1007/s00125-004-1437-0
Annes, J. P., Munger, J. S., and Rifkin, D. B. (2003). Making sense of latent TGFbeta activation. J. Cell Sci. 116 (2), 217–224. doi:10.1242/jcs.00229
Bae, E. H., Kim, I. J., Song, J. H., Choi, H. S., Kim, C. S., Eom, G. H., et al. (2019). Renoprotective effect of the histone deacetylase inhibitor CG200745 in DOCA-salt hypertensive rats. Int. J. Mol. Sci. 20 (3), E508. doi:10.3390/ijms20030508
Bondarev, A. D., Attwood, M. M., Jonsson, J., Chubarev, V. N., Tarasov, V. V., and Schioth, H. B. (2021). Recent developments of HDAC inhibitors: Emerging indications and novel molecules. Br. J. Clin. Pharmacol. 87 (12), 4577–4597. doi:10.1111/bcp.14889
Bonnans, C., Chou, J., and Werb, Z. (2014). Remodelling the extracellular matrix in development and disease. Nat. Rev. Mol. Cell Biol. 15 (12), 786–801. doi:10.1038/nrm3904
Broekema, M., Harmsen, M. C., van Luyn, M. J. A., Koerts, J. A., Petersen, A. H., van Kooten, T. G., et al. (2007). Bone marrow-derived myofibroblasts contribute to the renal interstitial myofibroblast population and produce procollagen I after ischemia/reperfusion in rats. J. Am. Soc. Nephrol. 18 (1), 165–175. doi:10.1681/ASN.2005070730
Bush, E. W., and McKinsey, T. A. (2010). Protein acetylation in the cardiorenal axis: The promise of histone deacetylase inhibitors. Circ. Res. 106 (2), 272–284. doi:10.1161/CIRCRESAHA.109.209338
Cai, J., Liu, Z., Huang, X., Shu, S., Hu, X., Zheng, M., et al. (2020). The deacetylase sirtuin 6 protects against kidney fibrosis by epigenetically blocking β-catenin target gene expression. Kidney Int. 97 (1), 106–118. doi:10.1016/j.kint.2019.08.028
Campas-Moya, C. (2009). Romidepsin for the treatment of cutaneous T-cell lymphoma. Drugs Today (Barc) 45 (11), 787–795. doi:10.1358/dot.2009.45.11.1437052
Casalena, G., Daehn, I., and Bottinger, E. (2012). Transforming growth factor-β, bioenergetics, and mitochondria in renal disease. Semin. Nephrol. 32 (3), 295–303. doi:10.1016/j.semnephrol.2012.04.009
Chen, E. E. M., Zhang, W., Ye, C. C. Y., Gao, X., Jiang, L. L. J., Zhao, T. T. F., et al. (2017). Knockdown of SIRT7 enhances the osteogenic differentiation of human bone marrow mesenchymal stem cells partly via activation of the Wnt/β-catenin signaling pathway. Cell Death Dis. 8 (9), e3042. doi:10.1038/cddis.2017.429
Chen, F., Gao, Q., Wei, A., Chen, X., Shi, Y., Wang, H., et al. (2021). Histone deacetylase 3 aberration inhibits Klotho transcription and promotes renal fibrosis. Cell Death Differ. 28 (3), 1001–1012. doi:10.1038/s41418-020-00631-9
Chen, L., Fischle, W., VErdin, E., and Greene, W. C. (2001). Duration of nuclear NF-kappaB action regulated by reversible acetylation. Science 293 (5535), 1653–1657. doi:10.1126/science.1062374
Cheng, O., ThuillieR, R., Sampson, E., Schultz, G., Ruiz, P., Zhang, X., et al. (2006). Connective tissue growth factor is a biomarker and mediator of kidney allograft fibrosis. Am. J. Transpl. 6 (10), 2292–2306. doi:10.1111/j.1600-6143.2006.01493.x
Chiba, T., Peasley, K. D., Cargill, K. R., Maringer, K. V., Bharathi, S. S., Mukherjee, E., et al. (2019). Sirtuin 5 regulates proximal tubule fatty acid oxidation to protect against AKI. J. Am. Soc. Nephrol. 30 (12), 2384–2398. doi:10.1681/ASN.2019020163
Choi, H. S., Song, J. H., Kim, I. J., Joo, S. Y., Eom, G. H., Kim, I., et al. (2018). Histone deacetylase inhibitor, CG200745 attenuates renal fibrosis in obstructive kidney disease. Sci. Rep. 8 (1), 11546. doi:10.1038/s41598-018-30008-5
Choi, S. Y., Piao, Z. H., Jin, L., Kim, J. H., Kim, G. R., Ryu, Y., et al. (2016). Piceatannol attenuates renal fibrosis induced by unilateral ureteral obstruction via downregulation of histone deacetylase 4/5 or p38-MAPK signaling. PLoS One 11 (11), e0167340. doi:10.1371/journal.pone.0167340
Choi, S. Y., Ryu, Y., Kee, H. J., Cho, S. N., Kim, G. R., Cho, J. Y., et al. (2015). Tubastatin A suppresses renal fibrosis via regulation of epigenetic histone modification and Smad3-dependent fibrotic genes. Vasc. Pharmacol. 72, 130–140. doi:10.1016/j.vph.2015.04.006
Chu, Q. S., Nielsen, T. O., Alcindor, T., GuptA, A., EndoM., , GoytAin, A., et al. (2015). A phase II study of SB939, a novel pan-histone deacetylase inhibitor, in patients with translocation-associated recurrent/metastatic sarcomas-NCIC-CTG IND 200†. Ann. Oncol. 26 (5), 973–981. doi:10.1093/annonc/mdv033
Dai, Q., Liu, J., Du, Y. l., Hao, X., Ying, J., Tan, Y., et al. (2016). Histone deacetylase inhibitors attenuate P-aIgA1-induced cell proliferation and extracellular matrix synthesis in human renal mesangial cells in vitro. Acta Pharmacol. Sin. 37 (2), 228–234. doi:10.1038/aps.2015.79
Das, F., Ghosh-Choudhury, N., Bera, A., Dey, N., Abboud, H. E., Kasinath, B. S., et al. (2013). Transforming growth factor β integrates Smad 3 to mechanistic target of rapamycin complexes to arrest deptor abundance for glomerular mesangial cell hypertrophy. J. Biol. Chem. 288 (11), 7756–7768. doi:10.1074/jbc.M113.455782
De Borst, M. H., Prakash, J., Melenhorst, W. B. W. H., van den Heuvel, M. C., Kok, R. J., Navis, G., et al. (2007). Glomerular and tubular induction of the transcription factor c-Jun in human renal disease. J. Pathol. 213 (2), 219–228. doi:10.1002/path.2228
de Ruijter, A. J., van Gennip, A. H., Caron, H. N., Kemp, S., and van Kuilenburg, A. B. P. (2003). Histone deacetylases (HDACs): Characterization of the classical HDAC family. Biochem. J. 370 (3), 737–749. doi:10.1042/BJ20021321
Fahey, J. W., Wade, K. L., Stephenson, K. K., Panjwani, A. A., Liu, H., Cornblatt, G., et al. (2019). Bioavailability of sulforaphane following ingestion of glucoraphanin-rich broccoli sprout and seed extracts with active myrosinase: A pilot study of the effects of proton pump inhibitor administration. Nutrients 11 (7), E1489. doi:10.3390/nu11071489
Feng, X., Su, H., He, X., Chen, J. X., and Zeng, H. (2020). SIRT3 deficiency sensitizes angiotensin-II-induced renal fibrosis. Cells 9 (11), E2510. doi:10.3390/cells9112510
Gewin, L. S. (2020). Sirtuin 6 and renal injury: Another link in the β-catenin chain? Kidney Int. 97 (1), 24–27. doi:10.1016/j.kint.2019.09.022
Gu, S., Liu, Y., Zhu, B., Ding, K., Yao, T. P., Chen, F., et al. (2016). Loss of α-tubulin acetylation is associated with TGF-β-induced epithelial-mesenchymal transition. J. Biol. Chem. 291 (10), 5396–5405. doi:10.1074/jbc.M115.713123
Hao, S., He, W., Li, Y., Ding, H., Hou, Y., Nie, J., et al. (2011). Targeted inhibition of β-catenin/CBP signaling ameliorates renal interstitial fibrosis. J. Am. Soc. Nephrol. 22 (9), 1642–1653. doi:10.1681/ASN.2010101079
He, F. F., You, R. Y., Ye, C., Lei, C. T., Tang, H., Su, H., et al. (2018). Inhibition of SIRT2 alleviates fibroblast activation and renal tubulointerstitial fibrosis via MDM2. Cell. Physiol. biochem. 46 (2), 451–460. doi:10.1159/000488613
He, W., Dai, C., Li, Y., Zeng, G., Monga, S. P., and Liu, Y. (2009). Wnt/beta-catenin signaling promotes renal interstitial fibrosis. J. Am. Soc. Nephrol. 20 (4), 765–776. doi:10.1681/ASN.2008060566
Ho, T. C. S., Chan, A. H. Y., and Ganesan, A. (2020). Thirty years of HDAC inhibitors: 2020 insight and hindsight. J. Med. Chem. 63 (21), 12460–12484. doi:10.1021/acs.jmedchem.0c00830
Hubbert, C., Guardiola, A., Shao, R., Kawaguchi, Y., Ito, A., Nixon, A., et al. (2002). HDAC6 is a microtubule-associated deacetylase. Nature 417 (6887), 455–458. doi:10.1038/417455a
Humphreys, B. D. (2018). Mechanisms of renal fibrosis. Annu. Rev. Physiol. 80, 309–326. doi:10.1146/annurev-physiol-022516-034227
Hyndman, K. A., Kasztan, M., Mendoza, L. D., and Monteiro-Pai, S. (2019). Dynamic changes in histone deacetylases following kidney ischemia-reperfusion injury are critical for promoting proximal tubule proliferation. Am. J. Physiol. Ren. Physiol. 316 (5), F875–F888. doi:10.1152/ajprenal.00499.2018
Inazaki, K., Kanamaru, Y., Kojima, Y., Sueyoshi, N., Okumura, K., Kaneko, K., et al. (2004). Smad3 deficiency attenuates renal fibrosis, inflammation, and apoptosis after unilateral ureteral obstruction. Kidney Int. 66 (2), 597–604. doi:10.1111/j.1523-1755.2004.00779.x
Iwano, M., Plieth, D., Danoff, T. M., Xue, C., Okada, H., and Neilson, E. G. (2002). Evidence that fibroblasts derive from epithelium during tissue fibrosis. J. Clin. Invest. 110 (3), 341–350. doi:10.1172/JCI15518
Jo, J. H., Jung, D. E., Lee, H. S., Park, S. B., Chung, M. J., Park, J. Y., et al. (2022). A phase I/II study of ivaltinostat combined with gemcitabine and erlotinib in patients with untreated locally advanced or metastatic pancreatic adenocarcinoma. Intl. J. Cancer. doi:10.1002/ijc.34144
Johnstone, R. W. (2002). Histone-deacetylase inhibitors: Novel drugs for the treatment of cancer. Nat. Rev. Drug Discov. 1 (4), 287–299. doi:10.1038/nrd772
Jones, D. L., Haak, A. J., Caporarello, N., Choi, K. M., Ye, Z., Yan, H., et al. (2019). TGFβ-induced fibroblast activation requires persistent and targeted HDAC-mediated gene repression. J. Cell Sci. 132 (20), jcs233486. doi:10.1242/jcs.233486
Kamato, D., Burch, M. L., Piva, T. J., Rezaei, H. B., Rostam, M. A., Xu, S., et al. (2013). Transforming growth factor-β signalling: Role and consequences of Smad linker region phosphorylation. Cell. Signal. 25 (10), 2017–2024. doi:10.1016/j.cellsig.2013.06.001
Kang, D. H., Yin, G. N., Choi, M. J., Song, K. M., Ghatak, K., Minh, N. N., et al. (2018). Silencing histone deacetylase 7 alleviates transforming growth factor-β1-induced profibrotic responses in fibroblasts derived from peyronie's plaque. World J. Mens. Health 36 (2), 139–146. doi:10.5534/wjmh.170005
Kang, S. W., Lee, S. M., Kim, J. Y., Kim, S. Y., Kim, Y. H., Kim, T. H., et al. (2017). Therapeutic activity of the histone deacetylase inhibitor SB939 on renal fibrosis. Int. Immunopharmacol. 42, 25–31. doi:10.1016/j.intimp.2016.11.008
Khan, S., Jena, G., and Tikoo, K. (2015). Sodium valproate ameliorates diabetes-induced fibrosis and renal damage by the inhibition of histone deacetylases in diabetic rat. Exp. Mol. Pathol. 98 (2), 230–239. doi:10.1016/j.yexmp.2015.01.003
Kim, H. J., and Bae, S. C. (2011). Histone deacetylase inhibitors: Molecular mechanisms of action and clinical trials as anti-cancer drugs. Am. J. Transl. Res. 3 (2), 166–179.
Knipstein, J., and Gore, L. (2011). Entinostat for treatment of solid tumors and hematologic malignancies. Expert Opin. Investig. Drugs 20 (10), 1455–1467. doi:10.1517/13543784.2011.613822
Kong, L., Wang, H., Li, C., Cheng, H., Cui, Y., Liu, L., et al. (2021). Sulforaphane ameliorates diabetes-induced renal fibrosis through epigenetic up-regulation of BMP-7. Diabetes Metab. J. 45 (6), 909–920. doi:10.4093/dmj.2020.0168
Kuang, X., Chen, S., Lao, J., Chen, Y., Jia, D., Tu, L., et al. (2021). HDAC9 in the injury of vascular endothelial cell mediated by P38 MAPK pathway. J. Interferon Cytokine Res. 41 (12), 439–449. doi:10.1089/jir.2021.0050
Kumar, P., Gogulamudi, V. R., Periasamy, R., Raghavaraju, G., Subramanian, U., and Pandey, K. N. (2017). Inhibition of HDAC enhances STAT acetylation, blocks NF-κB, and suppresses the renal inflammation and fibrosis in Npr1 haplotype male mice. Am. J. Physiol. Ren. Physiol. 313 (3), F781–F795. doi:10.1152/ajprenal.00166.2017
Lauffer, B. E., Mintzer, R., Fong, R., Mukund, S., Tam, C., Zilberleyb, I., et al. (2013). Histone deacetylase (HDAC) inhibitor kinetic rate constants correlate with cellular histone acetylation but not transcription and cell viability. J. Biol. Chem. 288 (37), 26926–26943. doi:10.1074/jbc.M113.490706
Law, B. M., Wilkinson, R., Wang, X., Kildey, K., Lindner, M., Beagley, K., et al. (2019). Effector γδ T cells in human renal fibrosis and chronic kidney disease. Nephrol. Dial. Transpl. 34 (1), 40–48. doi:10.1093/ndt/gfy098
LeBleu, V. S., Taduri, G., O'Connell, J., Teng, Y., Cooke, V. G., Woda, C., et al. (2013). Origin and function of myofibroblasts in kidney fibrosis. Nat. Med. 19 (8), 1047–1053. doi:10.1038/nm.3218
Li, J., Qu, X., Ricardo, S. D., Bertram, J. F., and Nikolic-Paterson, D. J. (2010). Resveratrol inhibits renal fibrosis in the obstructed kidney: Potential role in deacetylation of Smad3. Am. J. Pathol. 177 (3), 1065–1071. doi:10.2353/ajpath.2010.090923
Li, J., Wang, J., Wang, J., NawaZ, Z., Liu, J. M., Qin, J., et al. (2000). Both corepressor proteins SMRT and N-CoR exist in large protein complexes containing HDAC3. Embo J. 19 (16), 4342–4350. doi:10.1093/emboj/19.16.4342
Li, X. T., Zhang, Y. P., Zhang, M. W., Zhang, Z. Z., and Zhong, J. C. (2022). Sirtuin 7 serves as a promising therapeutic target for cardiorenal diseases. Eur. J. Pharmacol. 925, 174977. doi:10.1016/j.ejphar.2022.174977
Lieberthal, W., and Levine, J. S. (2012). Mammalian target of rapamycin and the kidney. II. Pathophysiology and therapeutic implications. Am. J. Physiol. Ren. Physiol. 303 (2), F180–F191. doi:10.1152/ajprenal.00015.2012
Lieberthal, W., and Levine, J. S. (2009). The role of the mammalian target of rapamycin (mTOR) in renal disease. J. Am. Soc. Nephrol. 20 (12), 2493–2502. doi:10.1681/ASN.2008111186
Lin, W., Zhang, Q., Liu, L., Yin, S., Liu, Z., and Cao, W. (2017). Klotho restoration via acetylation of Peroxisome Proliferation-Activated Receptor γ reduces the progression of chronic kidney disease. Kidney Int. 92 (3), 669–679. doi:10.1016/j.kint.2017.02.023
Liu, H. (2021). The roles of histone deacetylases in kidney development and disease. Clin. Exp. Nephrol. 25 (3), 215–223. doi:10.1007/s10157-020-01995-5
Liu, L., Lin, W., Zhang, Q., Cao, W., and Liu, Z. (2016). TGF-β induces miR-30d down-regulation and podocyte injury through Smad2/3 and HDAC3-associated transcriptional repression. J. Mol. Med. 94 (3), 291–300. doi:10.1007/s00109-015-1340-9
Liu, N., Guo, J. K., Pang, M., Tolbert, E., Ponnusamy, M., Gong, R., et al. (2012). Genetic or pharmacologic blockade of EGFR inhibits renal fibrosis. J. Am. Soc. Nephrol. 23 (5), 854–867. doi:10.1681/ASN.2011050493
Liu, N., He, S., Ma, L., Ponnusamy, M., Tang, J., Tolbert, E., et al. (2013). Blocking the class I histone deacetylase ameliorates renal fibrosis and inhibits renal fibroblast activation via modulating TGF-beta and EGFR signaling. PLoS One 8 (1), e54001. doi:10.1371/journal.pone.0054001
Liu, N., and Zhuang, S. (2015). Treatment of chronic kidney diseases with histone deacetylase inhibitors. Front. Physiol. 6, 121. doi:10.3389/fphys.2015.00121
Liu, Y., Wang, Y., Ding, W., and Wang, Y. (2018). Mito-TEMPO alleviates renal fibrosis by reducing inflammation, mitochondrial dysfunction, and endoplasmic reticulum stress. Oxid. Med. Cell Longev. 2018, 5828120. doi:10.1155/2018/5828120
Luo, Y. X., Tang, X., An, X. Z., Xie, X. M., Chen, X. F., Zhao, X., et al. (2017). SIRT4 accelerates Ang II-induced pathological cardiac hypertrophy by inhibiting manganese superoxide dismutase activity. Eur. Heart J. 38 (18), 1389–1398. doi:10.1093/eurheartj/ehw138
Ma, F. Y., Flanc, R. S., Tesch, G. H., Bennett, B. L., Friedman, G. C., and Nikolic-Paterson, D. J. (2009). Blockade of the c-Jun amino terminal kinase prevents crescent formation and halts established anti-GBM glomerulonephritis in the rat. Lab. Invest. 89 (4), 470–484. doi:10.1038/labinvest.2009.2
Ma, F. Y., Flanc, R. S., Tesch, G. H., Han, Y., Atkins, R. C., Bennett, B. L., et al. (2007). A pathogenic role for c-Jun amino-terminal kinase signaling in renal fibrosis and tubular cell apoptosis. J. Am. Soc. Nephrol. 18 (2), 472–484. doi:10.1681/ASN.2006060604
Ma, X., and Wang, Q. (2022). Short-chain fatty acids attenuate renal fibrosis and enhance autophagy of renal tubular cells in diabetic mice through the HDAC2/ULK1 Axis. Endocrinol. Metab. 37 (3), 432–443. doi:10.3803/EnM.2021.1336
Mahlknecht, U., Najfeld, V., Young, S., and VErdin, E. (1999). Genomic organization and chromosomal localization of the human histone deacetylase 3 gene. Genomics 56 (2), 197–202. doi:10.1006/geno.1998.5645
Manson, S. R., Song, J. B., Hruska, K. A., and Austin, P. F. (2014). HDAC dependent transcriptional repression of Bmp-7 potentiates TGF-β mediated renal fibrosis in obstructive uropathy. J. Urol. 191 (1), 242–252. doi:10.1016/j.juro.2013.06.110
Mao, L., Liu, L., Zhang, T., Qin, H., Wu, X., and Xu, Y. (2020). Histone deacetylase 11 contributes to renal fibrosis by repressing KLF15 transcription. Front. Cell Dev. Biol. 8, 235. doi:10.3389/fcell.2020.00235
Marconi, G. D., Fonticoli, L., Rajan, T. S., Pierdomenico, S. D., Trubiani, O., Pizzicannella, J., et al. (2021). Epithelial-mesenchymal transition (EMT): The type-2 EMT in wound healing, tissue regeneration and organ fibrosis. Cells 10 (7), 1587. doi:10.3390/cells10071587
Marks, P. A., and Breslow, R. (2007). Dimethyl sulfoxide to vorinostat: Development of this histone deacetylase inhibitor as an anticancer drug. Nat. Biotechnol. 25 (1), 84–90. doi:10.1038/nbt1272
Mazumder, S., Barman, M., Bandyopadhyay, U., and Bindu, S. (2020). Sirtuins as endogenous regulators of lung fibrosis: A current perspective. Life Sci. 258, 118201. doi:10.1016/j.lfs.2020.118201
Meng, X. M., Nikolic-Paterson, D. J., and Lan, H. Y. (2016). TGF-Β: The master regulator of fibrosis. Nat. Rev. Nephrol. 12 (6), 325–338. doi:10.1038/nrneph.2016.48
Meran, S., and Steadman, R. (2011). Fibroblasts and myofibroblasts in renal fibrosis. Int. J. Exp. Pathol. 92 (3), 158–167. doi:10.1111/j.1365-2613.2011.00764.x
Minucci, S., and Pelicci, P. G. (2006). Histone deacetylase inhibitors and the promise of epigenetic (and more) treatments for cancer. Nat. Rev. Cancer 6 (1), 38–51. doi:10.1038/nrc1779
Miyazono, K., Kusanagi, K., and Inoue, H. (2001). Divergence and convergence of TGF-beta/BMP signaling. J. Cell. Physiol. 187 (3), 265–276. doi:10.1002/jcp.1080
Morigi, M., Perico, L., and Benigni, A. (2018). Sirtuins in renal health and disease. J. Am. Soc. Nephrol. 29 (7), 1799–1809. doi:10.1681/ASN.2017111218
Mosser, D. M., and Edwards, J. P. (2008). Exploring the full spectrum of macrophage activation. Nat. Rev. Immunol. 8 (12), 958–969. doi:10.1038/nri2448
Müller, R., Daniel, C., Hugo, C., Amann, K., Mielenz, D., Endlich, K., et al. (2013). The mitogen-activated protein kinase p38α regulates tubular damage in murine anti-glomerular basement membrane nephritis. PLoS One 8 (2), e56316. doi:10.1371/journal.pone.0056316
Nanau, R. M., and Neuman, M. G. (2013). Adverse drug reactions induced by valproic acid. Clin. Biochem. 46 (15), 1323–1338. doi:10.1016/j.clinbiochem.2013.06.012
Nastase, M. V., Zeng-Brouwers, J., Wygrecka, M., and Schaefer, L. (2018). Targeting renal fibrosis: Mechanisms and drug delivery systems. Adv. Drug Deliv. Rev. 129, 295–307. doi:10.1016/j.addr.2017.12.019
Nguyễn-Thanh, T., Kim, D., Lee, S., Kim, W., Park, S. K., and Kang, K. P. (2018). Inhibition of histone deacetylase 1 ameliorates renal tubulointerstitial fibrosis via modulation of inflammation and extracellular matrix gene transcription in mice. Int. J. Mol. Med. 41 (1), 95–106. doi:10.3892/ijmm.2017.3218
Nikolic-Paterson, D. J. (2010). CD4+ T cells: A potential player in renal fibrosis. Kidney Int. 78 (4), 333–335. doi:10.1038/ki.2010.182
Novotny-Diermayr, V., Sangthongpitag, K., Hu, C. Y., Wu, X., Sausgruber, N., Yeo, P., et al. (2010). SB939, a novel potent and orally active histone deacetylase inhibitor with high tumor exposure and efficacy in mouse models of colorectal cancer. Mol. Cancer Ther. 9 (3), 642–652. doi:10.1158/1535-7163.MCT-09-0689
O'Connor, O. A., Horwitz, S., Masszi, T., Van Hoof, A., Brown, P., Doorduijn, J., et al. (2015). Belinostat in patients with relapsed or refractory peripheral T-cell lymphoma: Results of the pivotal phase II BELIEF (CLN-19) study. J. Clin. Oncol. 33 (23), 2492–2499. doi:10.1200/JCO.2014.59.2782
Ornoy, A. (2009). Valproic acid in pregnancy: How much are we endangering the embryo and fetus? Reprod. Toxicol. 28 (1), 1–10. doi:10.1016/j.reprotox.2009.02.014
Pang, M., Kothapally, J., Mao, H., Tolbert, E., Ponnusamy, M., Chin, Y. E., et al. (2009). Inhibition of histone deacetylase activity attenuates renal fibroblast activation and interstitial fibrosis in obstructive nephropathy. Am. J. Physiol. Ren. Physiol. 297 (4), F996–F1005. doi:10.1152/ajprenal.00282.2009
Pannem, R. R., Dorn, C., Hellerbrand, C., and Massoumi, R. (2014). Cylindromatosis gene CYLD regulates hepatocyte growth factor expression in hepatic stellate cells through interaction with histone deacetylase 7. Hepatology 60 (3), 1066–1081. doi:10.1002/hep.27209
Peng, X., Xiao, Z., Zhang, J., Li, Y., Dong, Y., and Du, J. (2015). IL-17A produced by both γδ T and Th17 cells promotes renal fibrosis via RANTES-mediated leukocyte infiltration after renal obstruction. J. Pathol. 235 (1), 79–89. doi:10.1002/path.4430
Ponnusamy, M., Zhou, X., Yan, Y., Tang, J., Tolbert, E., Zhao, T. C., et al. (2014). Blocking sirtuin 1 and 2 inhibits renal interstitial fibroblast activation and attenuates renal interstitial fibrosis in obstructive nephropathy. J. Pharmacol. Exp. Ther. 350 (2), 243–256. doi:10.1124/jpet.113.212076
Raeeszadeh-Sarmazdeh, M., Do, L. D., and Hritz, B. G. (2020). Metalloproteinases and their inhibitors: Potential for the development of new therapeutics. Cells 9 (5), E1313. doi:10.3390/cells9051313
Rayego-Mateos, S., Campillo, S., Rodrigues-Diez, R. R., Tejera-Munoz, A., Marquez-Exposito, L., Goldschmeding, R., et al. (2021). Interplay between extracellular matrix components and cellular and molecular mechanisms in kidney fibrosis. Clin. Sci. 135 (16), 1999–2029. doi:10.1042/CS20201016
Robertson, I. B., Horiguchi, M., Zilberberg, L., Dabovic, B., Hadjiolova, K., and Rifkin, D. B. (2015). Latent TGF-β-binding proteins. Matrix Biol. 47, 44–53. doi:10.1016/j.matbio.2015.05.005
Romagnani, P., Remuzzi, G., Glassock, R., Levin, A., Jager, K. J., Tonelli, M., et al. (2017). Chronic kidney disease. Nat. Rev. Dis. Prim. 3, 17088. doi:10.1038/nrdp.2017.88
Samarakoon, R., Dobberfuhl, A. D., Cooley, C., Overstreet, J. M., Patel, S., Goldschmeding, R., et al. (2013). Induction of renal fibrotic genes by TGF-β1 requires EGFR activation, p53 and reactive oxygen species. Cell. Signal. 25 (11), 2198–2209. doi:10.1016/j.cellsig.2013.07.007
Shan, B., Yao, T. p., Nguyen, H. T., Zhuo, Y., Levy, D. R., Klingsberg, R. C., et al. (2008). Requirement of HDAC6 for transforming growth factor-beta1-induced epithelial-mesenchymal transition. J. Biol. Chem. 283 (30), 21065–21073. doi:10.1074/jbc.M802786200
Shi, Y., Jia, B., Xu, W., Li, W., Liu, T., Liu, P., et al. (2017). Chidamide in relapsed or refractory peripheral T cell lymphoma: A multicenter real-world study in China. J. Hematol. Oncol. 10 (1), 69. doi:10.1186/s13045-017-0439-6
Shi, Y., and Massagué, J. (2003). Mechanisms of TGF-beta signaling from cell membrane to the nucleus. Cell 113 (6), 685–700. doi:10.1016/s0092-8674(03)00432-x
Siegel, D., Hussein, M., Belani, C., Robert, F., Galanis, E., Richon, V. M., et al. (2009). Vorinostat in solid and hematologic malignancies. J. Hematol. Oncol. 2, 31. doi:10.1186/1756-8722-2-31
Simonsson, M., Heldin, C. H., Ericsson, J., and Gronroos, E. (2005). The balance between acetylation and deacetylation controls Smad7 stability. J. Biol. Chem. 280 (23), 21797–21803. doi:10.1074/jbc.M503134200
Smith, E. R., Wigg, B., Holt, S., and Hewitson, T. D. (2019). TGF-β1 modifies histone acetylation and acetyl-coenzyme A metabolism in renal myofibroblasts. Am. J. Physiol. Ren. Physiol. doi:10.1152/ajprenal.00513.2018
Smolewski, P., and Robak, T. (2017). The discovery and development of romidepsin for the treatment of T-cell lymphoma. Expert Opin. Drug Discov. 12 (8), 859–873. doi:10.1080/17460441.2017.1341487
Stambe, C., Atkins, R. C., Tesch, G. H., Masaki, T., Schreiner, G. F., and Nikolic-Paterson, D. J. (2004b). The role of p38alpha mitogen-activated protein kinase activation in renal fibrosis. J. Am. Soc. Nephrol. 15 (2), 370–379. doi:10.1097/01.asn.0000109669.23650.56
Stambe, C., Nikolic-Paterson, D. J., Hill, P. A., Dowling, J., and Atkins, R. C. (2004a). p38 Mitogen-activated protein kinase activation and cell localization in human glomerulonephritis: correlation with renal injury. J. Am. Soc. Nephrol. 15 (2), 326–336. doi:10.1097/01.asn.0000108520.63445.e0
Strutz, F., Okada, H., Lo, C. W., Danoff, T., Carone, R. L., Tomaszewski, J. E., et al. (1995). Identification and characterization of a fibroblast marker: FSP1. J. Cell Biol. 130 (2), 393–405. doi:10.1083/jcb.130.2.393
Suh, S. H., Choi, H. S., Kim, C. S., Kim, I. J., Cha, H., Cho, J. M., et al. (2020). CG200745, a novel HDAC inhibitor, attenuates kidney fibrosis in a murine model of Alport syndrome. Int. J. Mol. Sci. 21 (4), E1473. doi:10.3390/ijms21041473
Tan, R. J., Zhou, D., Zhou, L., and Liu, Y. (2011). Wnt/β-catenin signaling and kidney fibrosis. Kidney Int. Suppl. 4 (1), 84–90. doi:10.1038/kisup.2014.16
Tang, P. M., Nikolic-Paterson, D. J., and Lan, H. Y. (2019). Macrophages: Versatile players in renal inflammation and fibrosis. Nat. Rev. Nephrol. 15 (3), 144–158. doi:10.1038/s41581-019-0110-2
Toda, N., Mukoyama, M., Yanagita, M., and Yokoi, H. (2018). CTGF in kidney fibrosis and glomerulonephritis. Inflamm. Regen. 38, 14. doi:10.1186/s41232-018-0070-0
Tseng, W. C., Tsai, M. T., Chen, N. J., and Tarng, D. C. (2020). Trichostatin A alleviates renal interstitial fibrosis through modulation of the M2 macrophage subpopulation. Int. J. Mol. Sci. 21 (17), E5966. doi:10.3390/ijms21175966
Tyler, J. R., Robertson, H., Booth, T. A., Burt, A. D., and Kirby, J. A. (2006). Chronic allograft nephropathy: Intraepithelial signals generated by transforming growth factor-beta and bone morphogenetic protein-7. Am. J. Transpl. 6 (6), 1367–1376. doi:10.1111/j.1600-6143.2006.01339.x
Van Veggel, M., Westerman, E., and Hamberg, P. (2018). Clinical pharmacokinetics and pharmacodynamics of panobinostat. Clin. Pharmacokinet. 57 (1), 21–29. doi:10.1007/s40262-017-0565-x
Wen, Y. D., Perissi, V., Staszewski, L. M., Yang, W. M., Krones, A., Glass, C. K., et al. (2000). The histone deacetylase-3 complex contains nuclear receptor corepressors. Proc. Natl. Acad. Sci. U. S. A. 97 (13), 7202–7207. doi:10.1073/pnas.97.13.7202
West, A. C., and Johnstone, R. W. (2014). New and emerging HDAC inhibitors for cancer treatment. J. Clin. Invest. 124 (1), 30–39. doi:10.1172/JCI69738
Xiong, C., Guan, Y., Zhou, X., Liu, L., Zhuang, M. A., Zhang, W., et al. (2019). Selective inhibition of class IIa histone deacetylases alleviates renal fibrosis. Faseb J. 33 (7), 8249–8262. doi:10.1096/fj.201801067RR
Yagishita, Y., Fahey, J. W., Dinkova-Kostova, A. T., and Kensler, T. W. (2019). Broccoli or sulforaphane: Is it the source or dose that matters? Molecules 24 (19), E3593. doi:10.3390/molecules24193593
Yan, Y., Ma, L., Zhou, X., Ponnusamy, M., Tang, J., Zhuang, M. A., et al. (2016). Src inhibition blocks renal interstitial fibroblast activation and ameliorates renal fibrosis. Kidney Int. 89 (1), 68–81. doi:10.1038/ki.2015.293
Yang, J., Yin, S., Bi, F., Liu, L., Qin, T., Wang, H., et al. (2017). TIMAP repression by TGFβ and HDAC3-associated Smad signaling regulates macrophage M2 phenotypic phagocytosis. J. Mol. Med. 95 (3), 273–285. doi:10.1007/s00109-016-1479-z
Yang, L., Besschetnova, T. Y., Brooks, C. R., Shah, J. V., and Bonventre, J. V. (2010). Epithelial cell cycle arrest in G2/M mediates kidney fibrosis after injury. Nat. Med. 16 (5), 535–543. doi:10.1038/nm.2144
Yang, M., Lee, S., Wang, T., Cha, E., Jang, J., Kim, D., et al. (2019). 26-Week repeated dose oral toxicity study of KCHO-1 in sprague-dawley rats. J. Pharmacopuncture 142, 192–199. doi:10.3831/KPI.2019.22.026
Yang, W. M., Yao, Y. L., Sun, J. M., Davie, J. R., and SEto, E. (1997). Isolation and characterization of cDNAs corresponding to an additional member of the human histone deacetylase gene family. J. Biol. Chem. 272 (44), 28001–28007. doi:10.1074/jbc.272.44.28001
Yoshikawa, M., Hishikawa, K., Marumo, T., and Fujita, T. (2007). Inhibition of histone deacetylase activity suppresses epithelial-to-mesenchymal transition induced by TGF-beta1 in human renal epithelial cells. J. Am. Soc. Nephrol. 18 (1), 58–65. doi:10.1681/ASN.2005111187
Zeisberg, M., Hanai, J. i., Sugimoto, H., Mammoto, T., Charytan, D., Strutz, F., et al. (2003). BMP-7 counteracts TGF-beta1-induced epithelial-to-mesenchymal transition and reverses chronic renal injury. Nat. Med. 9 (7), 964–968. doi:10.1038/nm888
Zeisberg, M., and Kalluri, R. (2008). Fibroblasts emerge via epithelial-mesenchymal transition in chronic kidney fibrosis. Front. Biosci. 13, 6991–6998. doi:10.2741/3204
Zeisberg, M., and Kalluri, R. (2004). The role of epithelial-to-mesenchymal transition in renal fibrosis. J. Mol. Med. 82 (3), 175–181. doi:10.1007/s00109-003-0517-9
Zeisberg, M., Khurana, M., Rao, V. H., Cosgrove, D., Rougier, J. P., Werner, M. C., et al. (2006). Stage-specific action of matrix metalloproteinases influences progressive hereditary kidney disease. PLoS Med. 3 (4), e100. doi:10.1371/journal.pmed.0030100
Zerr, P., Palumbo-Zerr, K., Huang, J., Tomcik, M., Sumova, B., Distler, O., et al. (2016). Sirt1 regulates canonical TGF-β signalling to control fibroblast activation and tissue fibrosis. Ann. Rheum. Dis. 75 (1), 226–233. doi:10.1136/annrheumdis-2014-205740
Zhang, M., and Zhang, S. (2020). T cells in fibrosis and fibrotic diseases. Front. Immunol. 11, 1142. doi:10.3389/fimmu.2020.01142
Zhang, N., Li, Z., Mu, W., Li, L., Liang, Y., Lu, M., et al. (2016). Calorie restriction-induced SIRT6 activation delays aging by suppressing NF-κB signaling. Cell Cycle 15 (7), 1009–1018. doi:10.1080/15384101.2016.1152427
Zhang, Y., Wen, P., Luo, J., Ding, H., Cao, H., He, W., et al. (2021). Sirtuin 3 regulates mitochondrial protein acetylation and metabolism in tubular epithelial cells during renal fibrosis. Cell Death Dis. 12 (9), 847. doi:10.1038/s41419-021-04134-4
Keywords: renal fibrosis, CKD, HDACs, HDAC inhibitors, TGF-β, BMP-7, smad, ECM
Citation: Wang J, Li J, Zhang X, Zhang M, Hu X and Yin H (2022) Molecular mechanisms of histone deacetylases and inhibitors in renal fibrosis progression. Front. Mol. Biosci. 9:986405. doi: 10.3389/fmolb.2022.986405
Received: 05 July 2022; Accepted: 17 August 2022;
Published: 06 September 2022.
Edited by:
Sarika Saraswati, Tennessee State University, United StatesReviewed by:
Anqun Chen, Second Xiangya Hospital, Central South University, ChinaCopyright © 2022 Wang, Li, Zhang, Zhang, Hu and Yin. This is an open-access article distributed under the terms of the Creative Commons Attribution License (CC BY). The use, distribution or reproduction in other forums is permitted, provided the original author(s) and the copyright owner(s) are credited and that the original publication in this journal is cited, in accordance with accepted academic practice. No use, distribution or reproduction is permitted which does not comply with these terms.
*Correspondence: Xiaopeng Hu, eGlhb3BlbmdfaHVAc2luYS5jb20=; Hang Yin, ZHIueWluaGFuZ0BnbWFpbC5jb20=
†These authors have contributed equally to this work and share first authorship
Disclaimer: All claims expressed in this article are solely those of the authors and do not necessarily represent those of their affiliated organizations, or those of the publisher, the editors and the reviewers. Any product that may be evaluated in this article or claim that may be made by its manufacturer is not guaranteed or endorsed by the publisher.
Research integrity at Frontiers
Learn more about the work of our research integrity team to safeguard the quality of each article we publish.