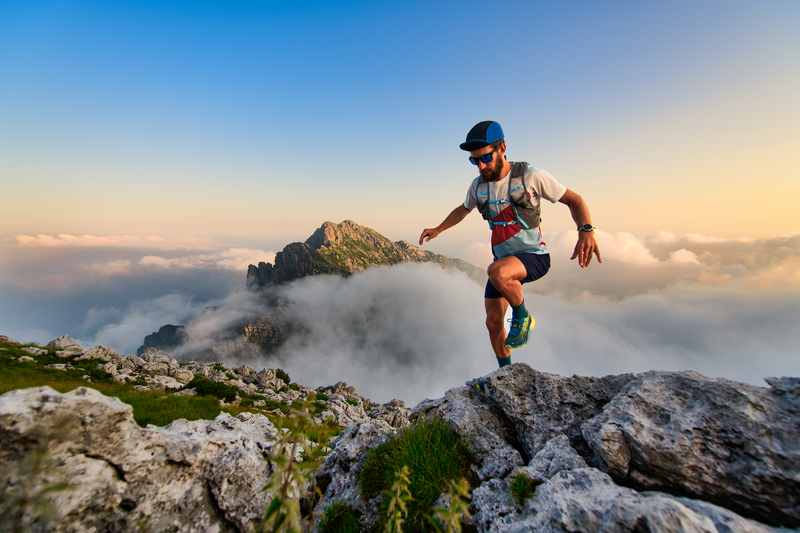
95% of researchers rate our articles as excellent or good
Learn more about the work of our research integrity team to safeguard the quality of each article we publish.
Find out more
REVIEW article
Front. Mol. Biosci. , 24 August 2022
Sec. Molecular Diagnostics and Therapeutics
Volume 9 - 2022 | https://doi.org/10.3389/fmolb.2022.965569
This article is part of the Research Topic Molecular Chaperones and Human Disease View all 11 articles
Plasmodium falciparum is responsible for the most severe and deadliest human malaria infection. The most serious complication of this infection is cerebral malaria. Among the proposed hypotheses that seek to explain the manifestation of the neurological syndrome in cerebral malaria is the vascular occlusion/sequestration/mechanic hypothesis, the cytokine storm or inflammatory theory, or a combination of both. Unfortunately, despite the increasing volume of scientific information on cerebral malaria, our understanding of its pathophysiologic mechanism(s) is still very limited. In a bid to maintain its survival and development, P. falciparum exports a large number of proteins into the cytosol of the infected host red blood cell. Prominent among these are the P. falciparum erythrocytes membrane protein 1 (PfEMP1), P. falciparum histidine-rich protein II (PfHRP2), and P. falciparum heat shock proteins 70-x (PfHsp70-x). Functional activities and interaction of these proteins with one another and with recruited host resident proteins are critical factors in the pathology of malaria in general and cerebral malaria in particular. Furthermore, several neurological impairments, including cognitive, behavioral, and motor dysfunctions, are known to be associated with cerebral malaria. Also, the available evidence has implicated glutamate and glutamatergic pathways, coupled with a resultant alteration in serotonin, dopamine, norepinephrine, and histamine production. While seeking to improve our understanding of the pathophysiology of cerebral malaria, this article seeks to explore the possible links between host/parasite chaperones, and neurotransmitters, in relation to other molecular players in the pathology of cerebral malaria, to explore such links in antimalarial drug discovery.
Malaria remains one of the disturbing public health challenges and a major vector-borne transmitted infection worldwide (Goldberg and Cowman, 2010; Boel et al., 2012; Khanam, 2017; WHO, 2021). The causative pathogen is Plasmodium species, five of which are known to infect human, namely, Plasmodium falciparum, P. vivax, P. ovale, P. malariae, and P. knowlesi, with P. vivax and P. falciparum known to cause the most lethal infection (Schiess et al., 2020; WHO, 2021). Its complex life cycle, spanning two hosts, human, and female Anopheles mosquito, and unabated transmission, has resulted in the high rate of infections, morbidity, and mortality (Nkumama et al., 2016; Khanam, 2017; WHO, 2021). Malaria is most common in rural, indigenous, and impoverished zones of Africa, Asia, and the tropics of America (Monteiro et al., 2014; Talapko et al., 2019). Prior to the advent of the COVID-19 pandemic, the fight against malaria infection appears to have been gaining success with noticeable decreases in the number of reported infections and deaths when compared to the previous year (Daniyan, 2021). However, the disruption in all malaria intervention areas, including prevention, diagnosis, treatment, elimination, and surveillance, occasioned by the pandemic, has led to marked increases in reported cases and associated mortality (Daniyan, 2021; WHO, 2021). The year 2021 world malaria reports estimated 241 million malaria cases and 627,000 deaths, representing 14 million more cases and 69,000 more deaths in 2020, with about 7.5% of these deaths linked to COVID-19 associated disruption (WHO, 2021). Therefore, the dynamics of malaria disease management has been altered by COVID-19 pandemic, and without a more concerted effort, and new strategies to arrest the upsurge, the gains of yesteryears may soon be completely wiped out.
Cerebral malaria (CM) is a deadly complicating manifestation of severe P. falciparum malaria with fast-rising fatal neurological syndromes and a high rate of mortality among children from sub-Saharan Africa (Desruisseaux et al., 2010; Grau and Craig, 2012; Shikani et al., 2012). Cerebral malaria occurs in about 1% of all P. falciparum infections and has a high mortality rate of 15%–25% (Shikani et al., 2012; WHO, 2021) leaving its surviving subjects with acute or long-term physical disability and neurological syndrome even post-treatment of the infection (Kihara et al., 2006; Grau and Craig, 2012; Hora et al., 2016). These manifestations differ in children and adults and vary depending on the onset of severe symptoms, including coma and status epilepticus, which could propel focal sequelae, impaired movement, hyperactivity, and inappropriate speech or vision (MacCormick et al., 2014). For instance, CM is not common in adults in sub-Saharan Africa due to acquired immunity during childhood attributable to high malaria transmission rates. In Southeast Asia on the other hand, where the transmission rate is lower and consequently not enough to lead to the development of immunity, CM is more common in adults and older children (Sahu et al., 2015; Sierro and Grau, 2019a). There are differences in CM-related organ dysfunction between adults and children (Sahu et al., 2015; Sierro and Grau, 2019a). While dysfunction in children is almost exclusively neurological, adults experience other organ dysfunctions, such as renal and respiratory dysfunction, in addition to neurological dysfunction (Sahu et al., 2015). Months or years after CM, neuropsychiatric manifestations and disabilities can set in (Monteiro et al., 2014). Meanwhile, despite the undeniable role of increased parasitemia, the levels of parasitemia are not necessarily the determinant for developing the disease, as there is no clear association between particular parasite features and CM (Storm and Craig, 2014a). However, CM is likely to occur in the absence of adequate antimalarials, and to a greater extent among the non-immune people (Bruneel, 2019), necessitating the need for adequate, affordable and accessible health facilities with approved medicine. Unfortunately, the extremely complex aetiology of CM has not been fully elucidated, and thus limiting our understanding of the pathologic mechanisms of CM (Bruneel, 2019; Sierro and Grau, 2019b). Nevertheless, there is a prevailing asymptomatic parasitaemia and certain non—specific pathological features among CM patients presenting with coma (Hora et al., 2016; Idro et al., 2016). In addition, lack of accurate and early diagnosis may have also contributed greatly to limited specificity and knowledge of the infection. Moreover, the available but limited post-mortem histological studies have not vividly explained the processes in the brain, identified the key players, nor shed light on how to ameliorate the aftermath effect of CM, necessitating the need for new and improve approaches to study CM (Sierro and Grau, 2019b). Therefore, despite increasing volume of scientific information on CM, our understanding of the pathophysiologic mechanism(s) of CM is still very limited.
Meanwhile, in a bid to maintain its survival and development, P. falciparum exports a large number of proteins into the cytosol of the infected host red blood cell (iRBC) (Acharya et al., 2007; Liu and Houry, 2014). Prominent among these are the molecular chaperones of the heat shock proteins family. Functional activities and interaction of these proteins with one another and with recruited host resident proteins are critical factors in the pathology of malaria infection (Banumathy et al., 2002; Pavithra et al., 2007; Daniyan et al., 2019b). Detailed reviews on the activities of these chaperones and their potential as drug targets have been presented (Shonhai, 2010; Daniyan and Blatch, 2017; Daniyan et al., 2019b; Shonhai et al., 2021). Furthermore, several neurological impairments, including cognitive, behavioral, and motor dysfunctions, are known to be associated with CM (Oluwayemi et al., 2013; Monteiro et al., 2014). Available evidence has implicated glutamate and glutamatergic pathways in CM, coupled with a resultant possible alteration in nitric oxide (NO), serotonin (5-HT), dopamine, norepinephrine, and histamine production (Roy et al., 1993; Enwonwu et al., 1999; Rubach et al., 2015; Yeo et al., 2015; Kempaiah et al., 2016; Oliveira et al., 2017). While seeking to improve our understanding of the pathophysiology of CM, this article seeks to explore the possible links or interactions between host/parasite chaperones, and neurotransmitters, in relation to other molecular players in the pathology of CM, with a view to exploring possible functional relationships in antimalarial drug discovery.
There is currently no complete understanding of the pathogenesis of CM. Although some hypotheses, such as that of mechanical obstruction of microvessels (sequestration of parasitized erythrocytes), and that of the release of copious amounts of pro-inflammatory cytokines, have been put forward for the pathogenesis of CM, they do not fully account for disease progression, prognosis, and outcome (van der Heyde et al., 2006; Storm and Craig, 2014a; Dunst et al., 2017; Schiess et al., 2020). The vascular occlusion or sequestration hypothesis is based on the sequestration of iRBCs into the brain capillary endothelia, resulting in microvascular blockage, loss of blood, tissue hypoxia, blood-brain barrier (BBB) compromise, and finally, CM (Storm et al., 2019; Schiess et al., 2020). Sequestration of the parasitized cells is seen as a survival strategy via immune evasion used by the parasites to avoid the removal of the cells by the spleen (Amante et al., 2010; Sierro and Grau, 2019a). Moreover, the somewhat hypoxic environment of venous blood is ideal for the growth of the parasites while protecting them from being destroyed by the spleen (Newton et al., 2000). Human CM post-mortem is characterized by swelling of cerebral capillaries and venules containing parasitized and non-parasitized erythrocytes (Newton et al., 2000; Sierro and Grau, 2019a), and platelets (Sierro and Grau, 2019a). Sequestration occurs to different extents in the various vital organs and the severity and prognosis of the disease have been linked to the size of the sequestered biomass (Newton et al., 2000; Ponsford et al., 2012), which cannot be deduced from peripheral parasite counts (Newton et al., 2000). Meanwhile, the binding of sequestered iRBCs to the endothelium is made possible by the P. falciparum erythrocytes membrane protein I (PfEMP1), ensuring an increased affinity of iRBCs to various receptors, notably, cytokine-activated endothelial protein C receptor (EPCR) and intercellular adhesion molecule-1 (ICAM-1) on the brain endothelial cells (Storm and Craig, 2014b; Nishanth and Schlüter, 2019; Storm et al., 2019). This is followed by the activation of thrombocytes to stimulate adhesion of iRBCs to one another, forming clots and inducing the non-iRBCs rosettes, hence, restraining blood flow, aggravating microvascular obstruction and tissue hypoxia (Storm and Craig, 2014b; Nishanth and Schlüter, 2019; Schiess et al., 2020), and eventual compromise of the BBB integrity (Ponsford et al., 2012; Rosenberg, 2012; Shimizu et al., 2018).
The inflammatory hypothesis is based on the release of toxic products by P. falciparum, causing an imbalance in systemic inflammatory responses, which are exacerbated due to sequestration and cytoadherence of iRBC (Storm and Craig, 2014b; Plewes et al., 2018). The resultant surge in the release of pro-inflammatory cytokines by macrophages, such as tumor necrosis factor-α (TNF-α), interleukin-B1 (IL-B1), and interleukin 10 (IL-10), amplify inflammation and BBB breakage by producing reactive oxygen species (ROS) and nitric oxide (NO) into the circulation (Bruneel, 2019; Schiess et al., 2020), resulting in impaired erythropoiesis and fever (Milner et al., 2014; Milner, 2018; Sierro and Grau, 2019b). On the other hand, these macrophages can as well release interferon-γ which aids the expression of surface proteins such as PfEMP-1, histidine-rich proteins (HRPs), ring erythrocyte surface antigen (RESA) in iRBCs and increase the formulation of adhesion molecules on endothelial cells to aid binding of surface proteins, thereby proliferating vascular permeability in several organs (Sierro and Grau, 2019b). These organs express several adhesion molecules, including cytokine-activated endothelial protein C receptor (EPCR), intercellular adhesion molecule-1 (ICAM-1), thrombospondin, and vascular cell adhesion molecule-1 (V-CAM-1) (Storm and Craig, 2014b; Storm et al., 2019), which further propels the endothelial cell to express several chemokines (Dunst et al., 2017; Nishanth and Schlüter, 2019). Also, P. falciparum-induced platelets are capable of binding to iRBCs, endothelial cells, and rosettes to promote immune activation by binding parasite-derived molecules via their toll-like receptors to further induce cytokines and chemokines (Bruneel, 2019). A detailed review of the roles of cytokines and chemokines in the pathogenesis of CM has been presented (Dunst et al., 2017). Following the multifunctional involvement of platelets in sequestration, humoral response, and endothelial dysfunction in both hypotheses, further investigation of their roles might pave way for more robust drug intervention.
The life cycle of the Plasmodium parasite spans two hosts, mosquito and human. The malaria parasite especially requires a robust adaptation system to cope with the stress of existence in two thermally different hosts, viz: the cold-blooded mosquito vector and warm-blooded humans. This coupled with intermittent febrile events in infected humans predisposes the parasite to experience thermal variations that would be stressful to the organism’s continued survival and growth. To easily adapt to varying physiological changes within these hosts, the malaria parasite expresses a large number of proteins, including molecular chaperones, some of which are exported to aid hosts invasion, facilitate cytoadherence, promote pathogenicity, and establishment of clinical malaria infection (Bozdech et al., 2003; Maier et al., 2008, 2009; Pallavi et al., 2010; Spielmann and Gilberger, 2010; Montagna et al., 2012; Liu and Houry, 2014). Molecular chaperones play critical roles in ensuring that newly synthesized proteins are functional, through proper folding, and where applicable, facilitate their subsequent trafficking to desired destinations and refolded to their native three-dimensional conformations (Hartl, 1996; Smith et al., 1998; Hartl and Hayer-Hartl, 2002; Pallavi et al., 2010). Furthermore, they facilitate the assembly of multi-protein complexes, maintain surveillance on cellular protein quality, and ensure that irreparably damaged proteins are timely handed over for degradation (Hartl, 1996; Hartl and Hayer-Hartl, 2002; Pallavi et al., 2010). They are known to function independently or in association with one another, forming a multi-functional network (Acharya et al., 2007; Pavithra et al., 2007). Generally, molecular chaperones of the heat shock protein (Hsp) superfamily, including Hsp90, Hsp70, Hsp60, Hsp40, and small Hsp, are important in this regard. Among the well-known functional networks involve the functional relationship between Hsp90 and Hsp70 and between these and their counterpart Hsp40 co-chaperones (Chua et al., 2014; Pesce and Blatch, 2014). The functional features of these plasmodial heat shock proteins, functional interplay, and networks of interactions, as well as their potential application in antimalarial drug discoveries, have been presented (Pavithra et al., 2007; Shonhai, 2010; Daniyan and Blatch, 2017; Daniyan et al., 2019b; Shonhai et al., 2021).
Meanwhile, the analysis of potential host-parasite protein-protein interactions has shown that the expression of heat shock proteins is important for efficient PfEMP1 presentation, and thus cytoadherence and sequestration (Rao et al., 2010). The PfEMP1 is a virulence factor encoded by var genes and available evidence has shown that the expression of DC8 and DC13 var genes is associated with cerebral malaria (Avril et al., 2013). Its trafficking to the surface of infected erythrocytes involves a network of proteins complex (Wickert et al., 2003; Acharya et al., 2007; Pavithra et al., 2007; Spycher et al., 2008). The PfEMP1 plays a critical role in malaria pathogenesis, cytoadherence, and immune evasion and has been presented as a potential drug target (Pasternak and Dzikowski, 2009; Hviid and Jensen, 2015; Bull and Abdi, 2016). Another virulence factor of importance to the establishment of CM is P. falciparum histidine-rich protein II (PfHRP2). The PfHRP2 is known to be transported into the cytosol of infected erythrocytes (Howard et al., 1986), compromised the brain endothelial barrier, promote CM pathogenesis (Pal et al., 2016), caused vascular leakage, and exacerbate CM (Pal et al., 2017). PfHRP2 is also involved in the protection of P. falciparum parasite from the toxic effects of heme by aiding neutralization of heme (Huy et al., 2003). Therefore, the combined activities of PfEMP1 and PfHRP2 are critical for the establishment of CM. Also, it has been demonstrated that the ability of human Hsp70 (HSPA1A) to regulate nuclear factor–kappa B (NF-κB) signaling and production of proinflammatory cytokines (such as IL-1β, IL-6, and TNF-α), can be suppressed by intraleukocytic hemozoin (PfHz). Interestingly, this suppression can be reversed in the presence of glutamine, which upregulates human Hsp70, thereby promoting the activation of NF-κB, and attenuation of overexpression of proinflammatory cytokines (Kempaiah et al., 2016). These findings suggest that suppression of functional activities of human Hsp70 is critical for the establishment of cerebral malaria. However, with their ubiquitous nature and multifunctional activities, as well as the involvements of human heat shock proteins, which are often recruited into the cytosol of iRBC (Banumathy et al., 2002), in nervous system activities (including neurotransmission and neuroprotection), and in modulation of the activities of NF-kB (a critical factor in CM pathology) (Stetler et al., 2010; Fusella et al., 2020), these findings show that more are still yet to be unraveled on the importance of host and plasmodial heat shock proteins in the pathogenesis of cerebral malarial.
Neurotransmitters are critical components of central and peripheral nervous systems. They are signaling molecules that are key players in the abilities of nerve cell, or neuron, to efficiently conveys information both electrically and chemically (Rangel-Gomez and Meeter, 2016). Functionally, neurotransmitters can be classified as excitatory and inhibitory neurotransmitters, neuromodulators, and neurohormones. The excitatory neurotransmitters, such as glutamate, acetylcholine, histamine, dopamine, and norepinephrine, induce or motivate target cells to take action by promoting the generation of the action potential. The inhibitory neurotransmitters function to decrease the chances of target cells initiating actions. Examples are dopamine, serotonin, and gamma-Aminobutyric acid (GABA). The neuromodulators, such as dopamine, acetylcholine, serotonin, norepinephrine, and histamine, can send messages to several neurons simultaneously. Finally, neurohormones, such as oxytocin and vasopressin, induce hormonal activities (Hyman, 2005; Rangel-Gomez and Meeter, 2016; Sheffler et al., 2022). Neurotransmitters can also be classified based on their chemical and molecular properties. They are monoamines (dopamine and norepinephrine); peptides (somatostatin and opioids); purines (adenosine triphosphate); and amino acids (glutamate and glycine). Also, some gaseous substances, such as nitric oxide, and endogenous substances, such as tryptamine and phenethylamines, have been shown to function as neurotransmitters (Rangel-Gomez and Meeter, 2016; Sheffler et al., 2022).
The neurodegenerative abnormalities that are consistent with the progression of cerebral malaria are likely to result from the interplay between specific neurotransmitters at various nerve terminals and their receptors. Available reports have shown that these baseline biochemical changes may lead to deteriorations in the histoarchitectural integrity of localized areas of the brain, especially those concerned with the coordination of cognitive, motor, and neurobehavioural activities (Roy et al., 1993; Cha et al., 1998; Suda et al., 2008; Jamwal and Kumar, 2019). In addition, the interplay between neurotransmitters with specific receptor molecules and associated protein expressions has been implicated in the complex cascade mechanisms of cerebral malaria. For instance, dopamine is known to play a central role in modulating cognitive functions, which may be linked to specific dopamine receptor signaling (Cropley et al., 2006). Specifically, the expressions of dopamine D2 receptor within the striatal medium spiny neurons (MSNs) are involved in the flexibility of human cognitive functions (van Holstein et al., 2011), and its defective signaling may alter metabolic activities within the brain while also taking a deregulatory toll on certain neural functions (Bhatia et al., 2022). Interestingly, the progression of cerebral malaria has been shown to trigger overexpression of dopamine D1 and D2 receptors (Kumar and Babu, 2020). The pathological consequence associated with defective signaling of dopamine receptors is the alteration of the structural and functional histoarchitecture of striatal MSNs (Rangel-Barajas et al., 2015). In addition, while excessive dopamine utilization within the prefrontal cortex was found to be consistent with the decline in cognitive functions among experimental animals (Murphy et al., 1996), the neuronal degenerations in the nigrostriatal dopamine signaling pathway can be very detrimental to motor coordination (Krashia et al., 2019). This evidence suggests that dopamine receptors may play an intermediary role between the induction of CM and associated neurodegenerative changes. Therefore, the defective interaction of dopamine with specific dopamine receptors may likely be having a significant implication on the neuropathogenesis of cognitive decline among CM subjects. Moreover, the interference of estrogen with dopaminergic pathways has been used to establish the memory deficits associated with certain neurodegenerative symptoms (Quinlan et al., 2008; Almey et al., 2015; Tozzi et al., 2015; McEwen and Milner, 2017). However, the relationship between sex hormone levels and the molecular pathway of dopamine-dependent neuromodulation in cerebral malaria is unclear. Consequently, the elucidation of these biochemical mechanisms may explain gender-related susceptibility to cerebral malaria. Also, it is possible that the chronic level of oxidative imbalance, occasioned by the presence of highly reactive metabolic by-products of dopamine, such as 3, 4–dihydroxyphenylacetaldehyde, may be involved in the neuroinflammatory pathologic mechanism of cerebral malaria (Miyazaki and Asanuma, 2009; Juárez Olguín et al., 2015; Monzani et al., 2019; Chen et al., 2020).
Furthermore, glutamate signaling is commonly associated with excitatory neurotransmission (Dauvermann et al., 2017). The interaction of glutamate with several receptors at the presynaptic and postsynaptic terminal is known to play important roles in normal brain functioning (Yamaguchi et al., 2011). Of all the L - glutamate receptors, the dense expressions of the N-methyl–D–aspartate (NMDA) receptor within the hippocampus and the cerebral cortex makes it highly critical for mediating learning and memory, or spatial memory activities within the central nervous system (Kumar, 2015). As ionotropic glutamate receptors, the NMDA receptors also confer synaptic plasticity as well as excitotoxic neuronal dysfunctions (Reiner and Levitz, 2018). Apart from the individual functional roles of glutamate and NMDA—glutamate receptors under physiological conditions, their overexpressions have been implicated in the neuropathogenesis of debilitating degenerative conditions (Singh et al., 2019). As a result, potential agents which can function as a specific antagonist of NMDA receptor signaling are currently being explored as ideal drug candidates for the improvement of cognitive decline and other comorbidities associated with cerebral malaria (de Miranda et al., 2017). Also, the metabolic products of glutamate and their baseline interactions with several important biomolecules have been found to play pivotal roles in the initiation and progression of cerebral malaria, and similar to dopamine, metabolic by-products of glutamate oxidation can also worsen the prognosis of cerebral malaria (Kelly and Stanley, 2001; Walker and van der Donk, 2016; Simões et al., 2018). The overstimulation of glutamate release is known to be excitotoxic (Simões et al., 2018), resulting in epileptic seizures commonly manifested in cerebral malaria (Lewerenz and Maher, 2015). Although the exact pathophysiology is unclear, oxidative stress and upregulated mitochondrial dysfunction have been implicated (Atlante et al., 2001). Also, there is an increasing interest in exploring glutamate receptor antagonist as potential neuroprotective agent (de Miranda et al., 2017).
Apart from dopamine and glutamate, available evidence revealed possible alteration in nitric oxide (NO), norepinephrine, histamine, and serotonin (5-HT) (Roy et al., 1993; Enwonwu et al., 1999; Beghdadi et al., 2009a; Miller et al., 2013; Rubach et al., 2015; Yeo et al., 2015; Kempaiah et al., 2016; Oliveira et al., 2017). For instance, the proinflammatory cytokines induce nitric oxide synthase, guanidine triphosphate (GTP) cyclohydrolase I, and indoleamine-pyrrole-2,3-dioxygenase pathways (Busse and Mülsch, 1990; Sakai et al., 1995; Katusic et al., 1998; Dunst et al., 2017). The induction of GTP cyclohydrolase I lead to the generation of tetrahydrobiopterin, a cofactor for nitric oxide synthase and tryptophan hydroxylase (Sakai et al., 1995; Higgins and Gross, 2010), with subsequent generation of nitric oxide and serotonin respectively. Similarly, the induction of indoleamine-pyrrole-2,3-dioxygenase, a regulatory enzyme in the kynurenine pathway, leads to the generation of quinolinic acid, a potent agonist of N-methyl-D-aspartate (NMDA) glutamate receptor (Guillemin et al., 2005; Lugo-Huitrón et al., 2013). Furthermore, enhanced synthesis of histamine has been linked to cerebral malaria-associated pathogenetic processes, including the disruption of BBB, and sequestration of T lymphocytes to cerebral vascular endothelium in mice (Enwonwu et al., 2000; Beghdadi et al., 2009a, 2009b). Also, an increased level of norepinephrine may lead to the potential aggravation of cerebral vasospasm (Zeiler et al., 2014). Therefore, the understanding of the roles of neurotransmitters, and their functional interplay are important not only to better understand pathogenic processes in CM but also for effective drug intervention and drug discovery.
The ability of iRBC to induce NF-kB-regulated inflammatory pathways in human cerebral endothelium has been demonstrated (Tripathi et al., 2009), indicating that NF-kB signaling has an important role to play in the pathology of CM (Figure 1). The NF-κB transcription factors have been implicated in many key physiological and pathophysiological processes, such as regulation of expression of genes needed for inflammation and immune responses, as well as cell proliferation, and apoptosis. In the central nervous system, the NF-κB is involved in diverse functions, including neuroinflammation. It is constitutively expressed in glutamatergic neurons, such as the cerebral cortex and hippocampus, serves neuroprotective roles, and is implicated in learning and memory. Both canonical and non-canonical NF-kB pathways are involved and serve to regulate gene transcription, including those involved in inflammatory processes, such as cytokines, chemokines, adhesion molecules, and proinflammatory enzymes and transcription factors. NF-κB is also found in glial cells, where its induction leads to the regulation of inflammatory processes that exacerbate neurodegenerating diseases, such as Alzheimer’s disease [reviewed here (Kaltschmidt and Kaltschmidt, 2009; Shih et al., 2015)]. These findings underscore the importance of NF-kB in immune responses to malaria infection (Bąska and Norbury, 2022) and may therefore serve as a critical link between the proposed vascular and inflammation hypotheses of CM pathology (Figure 1). Interestingly, chaperones are known to play functional roles in many processes regulated by NF-kB pathways (Fusella et al., 2020). Such roles depend on the regulated processes as well as on whether these chaperones are constitutively expressed or inducible. For instance, several lines of evidence have validated the inhibitory effects of upregulation of human Hsp70 on the induced expression of TNF-α and IL-6, and the activation of NF-κB (Senf et al., 2008; Wang C.-H. et al., 2017; Lyu et al., 2020) (Figure 1). However, the inhibition of Hsc70 by deoxyspergualin and the siRNA knocked down variant of Hsc70, significantly decreased nuclear translocation and neuronal activity of NF-κB p65. This suggests that Hsc70 may likely be involved in the activation, rather than inhibition of NF-κB (Klenke et al., 2013, 65). In addition, beyond the protein folding and chaperoning, available evidence has also implicated chaperones of the heat shock proteins as critical regulator of normal neuronal physiological functions, including neurotransmission. Essentially, chaperones, especially Hsc70 and human Hsp70 (HSPA1A), and their counterpart DNAJ have been implicated in the vesicular storage of neurotransmitters, release into the synaptic cleft, reforming and recycling of the vesicular membrane, postsynaptic interaction, and G-protein signaling [reviewed here (Stetler et al., 2010)]. For instance, recent evidence has shown that in response to dopamine-induced oxidative assaults on other proteins concerned with neurodegeneration, molecular chaperones are commonly expressed as endogenous mechanisms for curtailing protein aggregation (Webster et al., 2019). These chaperones, including Hsp27 and αβ—crystallin (Hayashi et al., 2021), as well as Hsp 40 and Hsp70 (Hu et al., 2021), were able to prevent the aggregation of certain proteins that are associated with the progression of parkinsonism (Hayashi et al., 2021). Interestingly, the induction of Hsp70 molecular chaperone in the dopaminergic neurons has been associated with neurodegeneration (Pastukhov et al., 2013; Smith et al., 2015; Zhang et al., 2018). Nevertheless, the effects of dopamine oxidation on the structural and functional integrities of human and plasmodial Hsp70 and its implication on the pathogenesis of cerebral malaria, remain unclear. However, it is clear that for any CM-mediated neurotransmitter alteration to take place, the malaria parasite needs molecular chaperones for continuous survival and development. Indeed, the presence of Hsp70 in dopaminergic neuron suggest a potential functional relationship. Furthermore, the induction of pro-inflammatory cytokines following cytoadherence and rupturing of pRBC to release malaria toxins (PfHz and glycosylphosphatidylinositol) is linked to the induction of cascade of biochemical pathways that are critical for the establishment of CM (Storm and Craig, 2014b; Storm et al., 2019) (Figure 1). While the induction of nitric oxide synthase and GTP cyclohydrolase I lead to the increased production of serotonin (Katusic et al., 1998), induction of indoleamine-pyrole-2,3-dioxygenase leads to the generation of quinolinic acid, a potent agonist of NMDA receptor (Guillemin et al., 2005; Liu et al., 2015) (Figure 1). Interestingly, nitric oxide is also known to modulate NMDA receptor and may inhibit cytoadherence of pRBC (Hopper et al., 2004). However, the inhibitory effect of PfHz on human Hsp70 can be reversed in the presence of glutamine (Kempaiah et al., 2016) (Figure 1). Therefore, chaperones may be the important link among the many molecular players in the pathophysiology of cerebral malaria (Figure 1). The interrelationship between chaperones and other associated molecular indices (Figure 1) may likely be a promising target for the development of novel drug candidates for the effective treatment of cerebral malaria.
FIGURE 1. Potential chaperones linked neurotransmitter pathways of the inflammation pathophysiologic mechanism of cerebral malaria. PfEMP1 is P. falciparum erythrocyte membrane protein 1; PfHz is hemozoin; PfHRPII is P. falciparum histidine-rich protein II; In red boxes are the pro-inflammatory cytokines inducers; In green boxes are the potentially major players in the inhibition of pro-inflammatory cytokines; In black boxes are downstream biochemical cascade potentially linked to the neurological syndrome in cerebral malaria; - and + signs indicate inhibition and induction respectively; ? With dotted double or single-faced arrows are potential functional relationships that are yet to be or partially investigated respectively; - and + signs with arrows are reported functional relationships with HSPA1A, but not for plasmodial Hsp70 chaperones.
Several reports have shown that heat shock proteins are promising antimalarial drug targets (Pesce et al., 2010; Shonhai, 2010; Shrestha et al., 2016; Daniyan and Blatch, 2017; Daniyan et al., 2019b; Daniyan, 2021). Despite the potential for compensatory upregulation of some heat shock proteins when one is inhibited (e.g., Hsp90 and Hsp70) (Posfai et al., 2018), the essentiality of some members of the heat shock proteins family, their unique functional features, and their ability to form functional networks, not only make their inhibition lethal but that such inhibition could cause a wave of deleterious consequences on the down-stream biochemical processes that these functional networks influence (Pavithra et al., 2007; Daniyan et al., 2019a; Daniyan and Ojo, 2019). Today, small molecule inhibition of plasmodial heat shock proteins has shown promise in reversing P. falciparum - induced drug resistance, while also synergizing with traditional antimalarial drugs (Shahinas et al., 2013b; Cockburn et al., 2014; Posfai et al., 2018). Chalcones, polyphenols, terpenoids, alkaloids, and peptides are among the well-tested classes of small molecule inhibitors of heat shock proteins Table 1 [reviewed here (Anokwuru et al., 2021; Daniyan, 2021)]. While there are evidence that some may help in preventing the breakdown of the blood-brain barrier (BBB) (Kam et al., 2012), their potential usefulness in cerebral malaria is largely unexplored.
TABLE 1. Features of selected small molecule inhibitors of molecular chaperones with antimalarial/antiplasmodial activities.
Chalcones possess several biological activities and have shown promise in antimalarial drug discovery (Singh et al., 2014; Murwih Alidmat et al., 2021; Salehi et al., 2021). The reported potent antimalarial activity of a novel oxygenated chalcone, 2,4-Dimethoxy-4′-Butoxychalcone, against Plasmodium falciparum in vitro, and Plasmodium berghei and Plasmodium yoelii in vivo (Chen et al., 1997), suggest a possible role for chalcone in the management of cerebral malarial. However, an important limitation is their low bioavailability (Mathew et al., 2016; Sinha et al., 2021), necessitating the need for improvement in the design of chalcone derivatives. Polyphenols, on the other hand, can suppress neuroinflammation, as well as promote memory, learning, and cognitive function (Vauzour, 2012; Figueira et al., 2019). They are neuroprotective, possibly via neuronal protection against oxidative stress and inflammatory injury (Filosa et al., 2018). While some polyphenols, such as epigallocatechin, daidzein, genistein, equol, and nobiletin, are reported to show high BBB permeability, others like apigenin, and kaempferol, as well as epicatechin, and curcumin showed medium to no permeability (Figueira et al., 2017, 2019; Shimazu et al., 2021). Interestingly, epigallocatechin-3-gallate, an essential polyphenol in green tea, has demonstrated antiplasmodial activity and inhibited both the chaperone and ATPase activity of Hsp90 and plasmodial Hsp70 (Tran et al., 2010; Moses et al., 2015; Zininga et al., 2017b). These reported dual inhibitory activities on Hsp90 and Hsp70 chaperones, coupled with the ability to cross the BBB, and protect neurons (Youn et al., 2022), suggest that epigallocatechin-3-gallate may be a promising candidate in the management of cerebral malaria. In addition, curcumin, another neuroprotective polyphenol (Chin et al., 2013; Wang X.-S. et al., 2017; Subedi and Gaire, 2021), has severally been shown to have potential as adjuvant therapy in ameliorating neurological syndrome in cerebral malarial despite its poor bioavailability (Jain et al., 2013). However, recent evidence has shown that the use of nano-formulated and liposome-incorporated curcumin has the potential to improve the bioavailability and biological activity of curcumin (Dende et al., 2017; Martí Coma-Cros et al., 2018). Moreover, curcumin modulates NMDA receptors and protects against NMDA and glutamate-induced toxicity (Matteucci et al., 2011; Mallozzi et al., 2018; Simões et al., 2018). Therefore, polyphenols are a promising class of small molecules in the management of cerebral malarial.
Meanwhile, alkaloids remain one of the oldest and most popular classes of compounds whose members have found usefulness for decades as antimalarial agents. The most popular member of this group is quinine (Kaur et al., 2009). Quinine, given within the recommended doses, is safe, and at higher doses with proper monitoring, the benefits often outweigh the exaggerated toxicity (White et al., 1982; Pussard et al., 2003). Moreso, quinine, though an old friend, still has a comparable level of effectiveness with newer artesunate despite the reported superiority of parenteral artesunate (Dondorp et al., 2010; Eltahir et al., 2010; Sinclair et al., 2012; Abdallah et al., 2014). However, though available evidence suggests that quinine does not freely cross the BBB (Silamut et al., 1985), quinine uptake into the brain can be increased by inhibition of P-glycoprotein (Pussard et al., 2007). Thus, inhibition of P-glycoprotein may improve the effectiveness of quinine in cerebral malaria through enhanced uptake into the brain. Also, quinine could modulate the expression of some plasmodial proteins, including enolase (PF3D7_1015900), endoplasmic reticulum-resident calcium-binding protein (PF3D7_1108600), chaperonin (PF3D7_1213500), the host cell invasion protein (PF3D7_1027300) and proteins related to redox processes (PF3D7_0827900) (Segura et al., 2014). In addition, quinine has been shown to decrease serotonin production via competitive inhibition of tryptophan hydroxylase in the presence of tryptophan (Islahudin et al., 2014), suggesting a potential mechanism of quinine action in cerebral malaria. Other members of the alkaloid class of compounds that have shown antimalarial properties and demonstrated Hsp90 inhibitory activities include ganetespib, harmine, PU-H71, geldanamycin (GA), and its analogs, 17-dimethylaminoethylamino-17-demethoxygeldanamycin (17-DMAG) and 17-allyamino-17-demethoxygeldanamycin (Shahinas et al., 2013a, 2013b; Posfai et al., 2018). The available report shows that geldanamycin could prevent focal ischemia in the brain, possibly by stimulating heat shock gene transcription (Lu et al., 2002). The synergistic ability of PU-H71 with chloroquine (Shahinas et al., 2013b), suggests that these compounds can be combined with existing antimalarial agents to improve treatment outcomes and reduce resistance. Also, a marine prenylated alkaloid, malonganenone A was shown to possess the antimalarial activity and selectively inhibit plasmodial Hsp70 (Cockburn et al., 2014).
Furthermore, terpenoids, which are the most abundant and structurally diverse secondary metabolites, possess a wide range of pharmacological activities, including antimalarial effects and heat shock protein inhibitory activities (Wang et al., 2005; Gabriel et al., 2018; Anokwuru et al., 2021). For instance, gedunin, kotschyins D, celastrol, and fusicoccane have been shown to inhibit Plasmodial Hsp90 and/or induced degradation of Hsp90-dependent client proteins (Westerheide et al., 2004; Brandt et al., 2008; Zhang et al., 2008; Piaz et al., 2012; Patwardhan et al., 2013; D’Ambola et al., 2019). Interestingly, celastrol also induces increased expression of human Hsp70 and protects motor neurons from excitotoxicity (Westerheide et al., 2004; Petrović et al., 2019). It is tempting to postulate that celastrol may find usefulness in cerebral malarial as evidence has shown that increased expression of Hsp70 prevents the production of inflammatory mediators via inhibition of NF_kB, (Kempaiah et al., 2016). In addition, a peptide antibiotic, polymyxin B, and an immunosuppressant, deoxyspergualin, have also demonstrated antimalarial activities (Anokwuru et al., 2021). Polymyxin B showed inhibitory activities on PfHsp70-1 (PF3D7_0818900) and PfHsp70-z (PF3D7_0708800) (Zininga et al., 2017a).
Therefore, while there are limitations, such as limited or inability to cross the blood-brain barrier, and limited to lack of information on the effects of these small molecules on brain neurotransmitters, there is more than enough evidence to propel further research into their potential usefulness in the treatment or as adjuvant therapy to mitigate the deleterious effects of the associated neurological syndrome in cerebral malaria.
Plants and other natural sources have been the bedrock of drug discovery over the years (Balunas and Kinghorn, 2005; Ahmad et al., 2006; Gurib-Fakim, 2006; Dzoyem et al., 2013). They are not just serving as sources of drug candidates, they have and still are been used in traditional medicine as worthy alternatives to orthodox drugs (Ahmad et al., 2006; Amoateng et al., 2018). Many of the tested small molecules are from these natural sources [reviewed here (Anokwuru et al., 2021; Daniyan, 2021)]. Many medicinal plant products have shown antimalarial, and/or neuroprotective abilities (Kaur et al., 2009; Mahomoodally, 2013; Amoateng et al., 2018; Erhirhie et al., 2021), and may therefore find usefulness in the management of cerebral malarial. Apart from those already mentioned and discussed, Table 2 provided a list of some medicinal plants’ derived compounds with neuroprotective and/or antimalarial activities which may find usefulness in cerebral malarial.
TABLE 2. Some medicinal plants’ derived compounds with neuroprotective and/or antimalarial properties.
Cerebral malaria is a deadly complication of severe P. falciparum malaria, with many survivors left to cope with long-term neurological deficits. A thorough understanding of the functional interplay among major molecular players, including molecular chaperones, neurotransmitters, and NF-kB signaling, in the pathology of CM, is critical for the development of a new treatment approach, and a new focus on antimalarial drug discovery. With their ubiquitous nature, multi-functional activities in the central nervous systems, and NF-kB signaling pathways, host heat shock protein chaperones, maybe the critical link amidst these molecular players. However, while functional interactions between host and parasite proteins are well established, the functional interplay between plasmodial heat shock proteins, especially the exported chaperones, and other molecular players (neurotransmitters, NF-kB, etc), requires further investigations.
MD: Conceptualization, Validation, and Project Administration. MD, FF, and OA: Contributed equally to writing, reviewing, and editing of the manuscript.
The authors declare that the research was conducted in the absence of any commercial or financial relationships that could be construed as a potential conflict of interest.
All claims expressed in this article are solely those of the authors and do not necessarily represent those of their affiliated organizations, or those of the publisher, the editors and the reviewers. Any product that may be evaluated in this article, or claim that may be made by its manufacturer, is not guaranteed or endorsed by the publisher.
Abdallah, T. M., Elmardi, K. A., Elhassan, A. H., Omer, M. B., Elhag, M. S., Desogi, M. A., et al. (2014). Comparison of artesunate and quinine in the treatment of severe Plasmodium falciparum malaria at Kassala hospital, Sudan. J. Infect. Dev. Ctries. 8, 611–615. doi:10.3855/jidc.3813
Acharya, P., Kumar, R., and Tatu, U. (2007). Chaperoning a cellular upheaval in malaria: Heat shock proteins in Plasmodium falciparum. Mol. Biochem. Parasitol. 153, 85–94. doi:10.1016/j.molbiopara.2007.01.009
Aguiar, S., and Borowski, T. (2013). Neuropharmacological review of the nootropic herb bacopa monnieri. Rejuvenation Res. 16, 313–326. doi:10.1089/rej.2013.1431
Ahmad, I. A., Farrukh, F., and Owais, M. (2006). “Herbal medicines: Prospects and constraints,” in Aqil and M. Owais, modern phytomedicine: Turning medicinal plants into drugs. Editor I., F. Ahmad (Germany: -VCH Verlag GmbH & Co.), 59–78.
Akompong, T., Ghori, N., and Haldar, K. (2000). In vitro activity of riboflavin against the human malaria parasite Plasmodium falciparum. Antimicrob. Agents Chemother. 44, 88–96. doi:10.1128/AAC.44.1.88-96.2000
Alam, Dr. M. (2018). Neuroprotective effects of Zingerone against carbon tetrachloride (CCl4) induced brain mitochondrial toxicity in Swiss albino mice. J. Appl. Nat. Sci. 10, 548–552. doi:10.31018/jans.v10i2.1734
Almey, A., Milner, T. A., and Brake, W. G. (2015). Estrogen receptors in the central nervous system and their implication for dopamine-dependent cognition in females. Horm. Behav. 74, 125–138. doi:10.1016/j.yhbeh.2015.06.010
Alson, S. G., Jansen, O., Cieckiewicz, E., Rakotoarimanana, H., Rafatro, H., Degotte, G., et al. (2018). In-vitro and in-vivo antimalarial activity of caffeic acid and some of its derivatives. J. Pharm. Pharmacol. 70, 1349–1356. doi:10.1111/jphp.12982
Alves, P. e. S., Santos, F. P. da S., Rodrigues, A. P., Dias, L. S., Silva, G. C. da, Araújo, L. da S., et al. (2021). Piper methysticum G. Forst (piperaceae) in the central nervous system: Phytochemistry, pharmacology and mechanism of action. Res. Soc. Dev. 10, e216101220479. doi:10.33448/rsd-v10i12.20479
Amante, F. H., Haque, A., Stanley, A. C., Rivera, F. de L., Randall, L. M., Wilson, Y. A., et al. (2010). Immune-mediated mechanisms of parasite tissue sequestration during experimental cerebral malaria. J. Immunol. 185, 3632–3642. doi:10.4049/jimmunol.1000944
Amoateng, P., Quansah, E., Karikari, T. K., Asase, A., Osei-Safo, D., Kukuia, K. K. E., et al. (2018). Medicinal plants used in the treatment of mental and neurological disorders in Ghana. Evid. Based. Complement. Altern. Med. 2018, 8590381. doi:10.1155/2018/8590381
Anokwuru, C., Makumire, S., and Shonhai, A. (2021). “Bioprospecting for novel heat shock protein modulators: The new frontier for antimalarial drug discovery?” in Heat shock Proteins of malaria advances in experimental medicine and biology. Editors A. Shonhai, D. Picard, and G. L. Blatch (Cham: Springer International Publishing), 187–203. doi:10.1007/978-3-030-78397-6_8
Atlante, A., Calissano, P., Bobba, A., Giannattasio, S., Marra, E., and Passarella, S. (2001). Glutamate neurotoxicity, oxidative stress and mitochondria. FEBS Lett. 497, 1–5. doi:10.1016/S0014-5793(01)02437-1
Avril, M., Brazier, A. J., Melcher, M., Sampath, S., and Smith, J. D. (2013). DC8 and DC13 var genes associated with severe malaria bind avidly to diverse endothelial cells. PLoS Pathog. 9, e1003430. doi:10.1371/journal.ppat.1003430
Balunas, M. J., and Kinghorn, A. D. (2005). Drug discovery from medicinal plants. Life Sci. 78, 431–441. doi:10.1016/j.lfs.2005.09.012
Banumathy, G., Singh, V., and Tatu, U. (2002). Host chaperones are recruited in membrane-bound complexes by Plasmodium falciparum. J. Biol. Chem. 277, 3902–3912. doi:10.1074/jbc.M110513200
Bąska, P., and Norbury, L. J. (2022). The role of nuclear factor kappa B (NF-κB) in the immune response against parasites. Pathogens 11, 310. doi:10.3390/pathogens11030310
Beghdadi, W., Porcherie, A., Schneider, B. S., Dubayle, D., Peronet, R., Huerre, M., et al. (2009a). Role of histamine and histamine receptors in the pathogenesis of malaria. Med. Sci. 25, 377–381. doi:10.1051/medsci/2009254377
Beghdadi, W., Porcherie, A., Schneider, B. S., Morisset, S., Dubayle, D., Peronet, R., et al. (2009b). Histamine H3 receptor-mediated signaling protects mice from cerebral malaria. PLOS ONE 4, e6004. doi:10.1371/journal.pone.0006004
Bhatia, A., Lenchner, J. R., and Saadabadi, A. (2022). StatPearls. Treasure Island (FL): StatPearls Publishing. Available at: http://www.ncbi.nlm.nih.gov/books/NBK538242/ (Accessed May 8, 2022).Biochemistry, dopamine receptors.
Bhatt, D., Kumar, S., Kumar, P., Bisht, S., Kumar, A., Maurya, A. K., et al. (2022). Rutin ameliorates malaria pathogenesis by modulating inflammatory mechanism: An in vitro and in vivo study. Inflammopharmacology 30, 159–171. doi:10.1007/s10787-021-00920-w
Bhatt, P. C., Pandey, P., Panda, B. P., Anwar, F., and Kumar, V. (2017). Commentary: L-3-n-butylphthalide rescues hippocampal synaptic failure and attenuates neuropathology in aged APP/PS1 mouse model of alzheimer’s disease. Front. Aging Neurosci. 9, 4. doi:10.3389/fnagi.2017.00004
Boel, M. E., Rijken, M. J., Brabin, B. J., Nosten, F., and McGready, R. (2012). The epidemiology of postpartum malaria: A systematic review. Malar. J. 11, 114. doi:10.1186/1475-2875-11-114
Bozdech, Z., Llinás, M., Pulliam, B. L., Wong, E. D., Zhu, J., DeRisi, J. L., et al. (2003). The transcriptome of the intraerythrocytic developmental cycle of Plasmodium falciparum. PLoS Biol. 1, 85–100. doi:10.1371/journal.pbio.0000005
Brandt, G. E. L., Schmidt, M. D., Prisinzano, T. E., and Blagg, B. S. J. (2008). Gedunin, a novel hsp90 inhibitor: Semisynthesis of derivatives and preliminary structure-activity relationships. J. Med. Chem. 51, 6495–6502. doi:10.1021/jm8007486
Brodsky, J. L. (1999). Selectivity of the molecular chaperone-specific immunosuppressive agent 15-deoxyspergualin: Modulation of Hsc70 ATPase activity without compromising DnaJ chaperone interactions. Biochem. Pharmacol. 57, 877–880. doi:10.1016/s0006-2952(98)00376-1
Bruneel, F. (2019). Human cerebral malaria: 2019 mini review. Rev. Neurol. Paris. 175, 445–450. doi:10.1016/j.neurol.2019.07.008
Budzynska, B., Faggio, C., Kruk-Slomka, M., Samec, D., Nabavi, S. F., Sureda, A., et al. (2019). Rutin as neuroprotective agent: From bench to bedside. Curr. Med. Chem. 26, 5152–5164. doi:10.2174/0929867324666171003114154
Bull, P. C., and Abdi, A. I. (2016). The role of PfEMP1 as targets of naturally acquired immunity to childhood malaria: Prospects for a vaccine. Parasitology 143, 171–186. doi:10.1017/S0031182015001274
Busse, R., Mülsch, A., and Mulsch, A. (1990). Induction of nitric oxide synthase by cytokines in vascular smooth muscle cells. FEBS Lett. 275, 87–90. doi:10.1016/0014-5793(90)81445-T
Cha, J.-H. J., Kosinski, C. M., Kerner, J. A., Alsdorf, S. A., Mangiarini, L., Davies, S. W., et al. (1998). Altered brain neurotransmitter receptors in transgenic mice expressing a portion of an abnormal human Huntington disease gene. Proc. Natl. Acad. Sci. U. S. A. 95, 6480–6485. doi:10.1073/pnas.95.11.6480
Chang, C.-C., Duann, Y.-F., Yen, T.-L., Chen, Y.-Y., Jayakumar, T., Ong, E.-T., et al. (2014). Andrographolide, a novel NF-κB inhibitor, inhibits vascular smooth muscle cell proliferation and cerebral endothelial cell inflammation. Acta Cardiol. Sin. 30, 308–315.
Chen, H.-H., Lin, S.-C., and Chan, M.-H. (2011). Protective and restorative effects of magnolol on neurotoxicity in mice with 6-hydroxydopamine-induced hemiparkinsonism. Neurodegener. Dis. 8, 364–374. doi:10.1159/000323872
Chen, M., Christensen, S. B., Zhai, L., Rasmussen, M. H., Theander, T. G., Frøkjaer, S., et al. (1997). The novel oxygenated chalcone, 2, 4-dimethoxy-4′-butoxychalcone, exhibits potent activity against human malaria parasite Plasmodium falciparum in vitro and rodent parasites Plasmodium berghei and Plasmodium yoelii in vivo. J. Infect. Dis. 176, 1327–1333. doi:10.1086/514129
Chen, S.-H., Kuo, C.-W., Lin, T.-K., Tsai, M.-H., and Liou, C.-W. (2020). Dopamine therapy and the regulation of oxidative stress and mitochondrial DNA copy number in patients with Parkinson’s disease. Antioxidants 9, 1159. doi:10.3390/antiox9111159
Chin, D., Huebbe, P., Pallauf, K., and Rimbach, G. (2013). Neuroprotective properties of curcumin in Alzheimer’s disease–merits and limitations. Curr. Med. Chem. 20, 3955–3985. doi:10.2174/09298673113209990210
Chua, C.-S., Low, H., and Sim, T.-S. (2014). Co-chaperones of Hsp90 in Plasmodium falciparum and their concerted roles in cellular regulation. Parasitology 141, 1177–1191. doi:10.1017/S0031182013002084
Cockburn, I. L., Boshoff, A., Pesce, E., and Blatch, G. L. (2014). Selective modulation of plasmodial Hsp70s by small molecules with antimalarial activity. Biol. Chem. 395, 1353–1362. doi:10.1515/hsz-2014-0138
Cockburn, I. L. L., Pesce, E. R. R., Pryzborski, J. M. M., Davies-Coleman, M. T. T., Clark, P. G. G., Keyzers, R. A. A., et al. (2011). Screening for small molecule modulators of Hsp70 chaperone activity using protein aggregation suppression assays: Inhibition of the plasmodial chaperone PfHsp70-1. Biol. Chem. 392, 431–438. doi:10.1515/BC.2011.040
Cropley, V. L., Fujita, M., Innis, R. B., and Nathan, P. J. (2006). Molecular imaging of the dopaminergic system and its association with human cognitive function. Biol. Psychiatry 59, 898–907. doi:10.1016/j.biopsych.2006.03.004
D’Ambola, M., Fiengo, L., Chini, M. G., Cotugno, R., Bader, A., Bifulco, G., et al. (2019). Fusicoccane diterpenes from Hypoestes forsskaolii as heat shock protein 90 (Hsp90) modulators. J. Nat. Prod. 82, 539–549. doi:10.1021/acs.jnatprod.8b00924
Daniyan, M. O., and Blatch, G. L. (2017). Plasmodial Hsp40s: New avenues for antimalarial drug discovery. Curr. Pharm. Des. 23, 4555–4570. doi:10.2174/1381612823666170124142439
Daniyan, M. O. (2021). Heat shock proteins as targets for novel antimalarial drug discovery. Adv. Exp. Med. Biol. 1340, 205–236. doi:10.1007/978-3-030-78397-6_9
Daniyan, M. O., and Ojo, O. T. (2019). In silico identification and evaluation of potential interaction of Azadirachta indica phytochemicals with Plasmodium falciparum heat shock protein 90. J. Mol. Graph. Model. 87, 144–164. doi:10.1016/j.jmgm.2018.11.017
Daniyan, M. O., Przyborski, J. M., Shonhai, A., Daniyan, M. O., Przyborski, J. M., and Shonhai, A. (2019b). Partners in mischief: Functional networks of heat shock proteins of Plasmodium falciparum and their influence on parasite virulence. Biomolecules 9, 295. doi:10.3390/biom9070295
Daniyan, M. O., Przyborski, J. M., and Shonhai, A. (2019a). Partners in mischief: Functional networks of heat shock proteins of Plasmodium falciparum and their influence on parasite virulence. Biomolecules 9 (7), 285(1–17). doi:10.3390/biom9070295
Dauvermann, M. R., Lee, G., and Dawson, N. (2017). Glutamatergic regulation of cognition and functional brain connectivity: Insights from pharmacological, genetic and translational schizophrenia research. Br. J. Pharmacol. 174, 3136–3160. doi:10.1111/bph.13919
de Miranda, A. S., Brant, F., Vieira, L. B., Rocha, N. P., Vieira, É. L. M., Rezende, G. H. S., et al. (2017). A neuroprotective effect of the glutamate receptor antagonist MK801 on long-term cognitive and behavioral outcomes secondary to experimental cerebral malaria. Mol. Neurobiol. 54, 7063–7082. doi:10.1007/s12035-016-0226-3
Dende, C., Meena, J., Nagarajan, P., Nagaraj, V. A., Panda, A. K., and Padmanaban, G. (2017). Nanocurcumin is superior to native curcumin in preventing degenerative changes in Experimental Cerebral Malaria. Sci. Rep. 7, 10062. doi:10.1038/s41598-017-10672-9
Desruisseaux, M. S., Machado, F. S., Weiss, L. M., Tanowitz, H. B., and Golightly, L. M. (2010). Cerebral malaria: A vasculopathy. Am. J. Pathol. 176, 1075–1078. doi:10.2353/ajpath.2010.091090
Dondorp, A. M., Fanello, C. I., Hendriksen, I. C., Gomes, E., Seni, A., Chhaganlal, K. D., et al. (2010). Artesunate versus quinine in the treatment of severe falciparum malaria in african children (AQUAMAT): An open-label, randomised trial. Lancet 376, 1647–1657. doi:10.1016/S0140-6736(10)61924-1
Dunst, J., Kamena, F., and Matuschewski, K. (2017). Cytokines and chemokines in cerebral malaria pathogenesis. Front. Cell. Infect. Microbiol. 7, 324(1–16). doi:10.3389/fcimb.2017.00324
Dzoyem, J. P., Tshikalange, E., and Kuete, V. (2013). “Medicinal plants market and industry in Africa,” in Medicinal plant research in Africa. Editor V. Kuete (Oxford: Elsevier), 859–890. doi:10.1016/B978-0-12-405927-6.00024-2
Eltahir, H. G., Omer, A. A., Mohamed, A. A., and Adam, I. (2010). Comparison of artesunate and quinine in the treatment of Sudanese children with severe Plasmodium falciparum malaria. Trans. R. Soc. Trop. Med. Hyg. 104, 684–686. doi:10.1016/j.trstmh.2010.05.009
Enwonwu, C. O., Afolabi, B. M., Salako, L. A., Idigbe, E. O., al-Hassan, H., and Rabiu, R. A. (1999). Hyperphenylalaninaemia in children with falciparum malaria. QJM Mon. J. Assoc. Physicians 92, 495–503. doi:10.1093/qjmed/92.9.495
Enwonwu, C. O., Afolabi, B. M., Salako, L. O., Idigbe, E. O., and Bashirelah, N. (2000). Increased plasma levels of histidine and histamine in falciparum malaria: Relevance to severity of infection. J. Neural Transm. 107, 1273–1287. doi:10.1007/s007020070017
Erhirhie, E. O., Ikegbune, C., Okeke, A. I., Onwuzuligbo, C. C., Madubuogwu, N. U., Chukwudulue, U. M., et al. (2021). Antimalarial herbal drugs: A review of their interactions with conventional antimalarial drugs. Clin. Phytosci. 7, 4–10. doi:10.1186/s40816-020-00242-4
Ezenyi, I. C., Salawu, O. A., Kulkarni, R., and Emeje, M. (2014). Antiplasmodial activity-aided isolation and identification of quercetin-4’-methyl ether in Chromolaena odorata leaf fraction with high activity against chloroquine-resistant Plasmodium falciparum. Parasitol. Res. 113, 4415–4422. doi:10.1007/s00436-014-4119-y
Farombi, E. O., Abolaji, A. O., Adetuyi, B. O., Awosanya, O., and Fabusoro, M. (2018). Neuroprotective role of 6-Gingerol-rich fraction of Zingiber officinale (Ginger) against acrylonitrile-induced neurotoxicity in male Wistar rats. J. Basic Clin. Physiol. Pharmacol. 30 (3), 1–11. doi:10.1515/jbcpp-2018-0114
Farombi, E. O., Awogbindin, I. O., Farombi, T. H., Oladele, J. O., Izomoh, E. R., Aladelokun, O. B., et al. (2019). Neuroprotective role of kolaviron in striatal redo-inflammation associated with rotenone model of Parkinson’s disease. Neurotoxicology 73, 132–141. doi:10.1016/j.neuro.2019.03.005
Figueira, I., Garcia, G., Pimpão, R. C., Terrasso, A. P., Costa, I., Almeida, A. F., et al. (2017). Polyphenols journey through blood-brain barrier towards neuronal protection. Sci. Rep. 7, 11456. doi:10.1038/s41598-017-11512-6
Figueira, I., Tavares, L., Jardim, C., Costa, I., Terrasso, A. P., Almeida, A. F., et al. (2019). Blood-brain barrier transport and neuroprotective potential of blackberry-digested polyphenols: An in vitro study. Eur. J. Nutr. 58, 113–130. doi:10.1007/s00394-017-1576-y
Filosa, S., Di Meo, F., and Crispi, S. (2018). Polyphenols-gut microbiota interplay and brain neuromodulation. Neural Regen. Res. 13, 2055–2059. doi:10.4103/1673-5374.241429
Fu, W., Wang, H., Ren, X., Yu, H., Lei, Y., and Chen, Q. (2017). Neuroprotective effect of three caffeic acid derivatives via ameliorate oxidative stress and enhance PKA/CREB signaling pathway. Behav. Brain Res. 328, 81–86. doi:10.1016/j.bbr.2017.04.012
Fusella, F., Seclì, L., Cannata, C., and Brancaccio, M. (2020). The one thousand and one chaperones of the NF-κB pathway. Cell. Mol. Life Sci. 77, 2275–2288. doi:10.1007/s00018-019-03402-z
Gabriel, H. B., Sussmann, R. A., Kimura, E. A., Rodriguez, A. A. M., Verdaguer, I. B., Leite, G. C. F., et al. (2018). “Terpenes as potential antimalarial drugs,” in Terpenes and Terpenoids. 1st Editors S. Perveen, and A. Al-Taweel (IntechOpen) Chapter 3, 39–57. doi:10.5772/intechopen.75108
Ganesh, D., Fuehrer, H.-P., Starzengrüber, P., Swoboda, P., Khan, W. A., Reismann, J. A. B., et al. (2012). Antiplasmodial activity of flavonol quercetin and its analogues in Plasmodium falciparum: Evidence from clinical isolates in Bangladesh and standardized parasite clones. Parasitol. Res. 110, 2289–2295. doi:10.1007/s00436-011-2763-z
Goldberg, D. E., and Cowman, A. F. (2010). Moving in and renovating: Exporting proteins from Plasmodium into host erythrocytes. Nat. Rev. Microbiol. 8, 617–621. doi:10.1038/nrmicro2420
Grau, G. E. R., and Craig, A. G. (2012). Cerebral malaria pathogenesis: Revisiting parasite and host contributions. Future Microbiol. 7, 291–302. doi:10.2217/fmb.11.155
Guillemin, G. J., Smythe, G., Takikawa, O., and Brew, B. J. (2005). Expression of indoleamine 2, 3-dioxygenase and production of quinolinic acid by human microglia, astrocytes, and neurons. Glia 49, 15–23. doi:10.1002/glia.20090
Gul, A., Bakht, J., and Mehmood, F. (2019). Huperzine-A response to cognitive impairment and task switching deficits in patients with Alzheimer’s disease. J. Chin. Med. Assoc. 82, 40–43. doi:10.1016/j.jcma.2018.07.004
Gurib-Fakim, A. (2006). Medicinal plants: Traditions of yesterday and drugs of tomorrow. Mol. Asp. Med. 27, 1–93. doi:10.1016/j.mam.2005.07.008
Han, H., Chen, Y., Bi, H., Yu, L., Sun, C., Li, S., et al. (2011). In vivo antimalarial activity of ginseng extracts. Pharm. Biol. 49, 283–289. doi:10.3109/13880209.2010.511235
Hartl, F. U., and Hayer-Hartl, M. (2002). Molecular chaperones in the cytosol: From nascent chain to folded protein. Science 295, 1852–1858. doi:10.1126/science.1068408
Hartl, F. U. (1996). Molecular chaperones in cellular protein folding. Nature 381, 571–579. doi:10.1038/381571a0
Hayashi, J., Ton, J., Negi, S., Stephens, D. E. K. M., Pountney, D. L., Preiss, T., et al. (2021). The effect of oxidized dopamine on the structure and molecular chaperone function of the small heat-shock proteins, αb-crystallin and Hsp27. Int. J. Mol. Sci. 22, 3700. doi:10.3390/ijms22073700
Higgins, C. E., and Gross, S. S. (2010). “Chapter 6 - tetrahydrobiopterin: An essential cofactor for nitric oxide synthases and amino acid hydroxylases,” in Nitric oxide. Editor L. J. Ignarro. 2nd Edition (San Diego: Academic Press), 169–209. doi:10.1016/B978-0-12-373866-0.00006-X
Hopper, R., Lancaster, B., and Garthwaite, J. (2004). On the regulation of NMDA receptors by nitric oxide. Eur. J. Neurosci. 19, 1675–1682. doi:10.1111/j.1460-9568.2004.03306.x
Hora, R., Kapoor, P., Thind, K. K., and Mishra, P. C. (2016). Cerebral malaria – clinical manifestations and pathogenesis. Metab. Brain Dis. 31, 225–237. doi:10.1007/s11011-015-9787-5
Howard, R. J., Uni, S., Aikawa, M., Aley, S. B., Leech, J. H., Lew, A. M., et al. (1986). Secretion of a malarial histidine-rich protein (Pf HRP II) from Plasmodium falciparum-infected erythrocytes. J. Cell Biol. 103, 1269–1277. doi:10.1083/jcb.103.4.1269
Hu, S., Tan, J., Qin, L., Lv, L., Yan, W., Zhang, H., et al. (2021). Molecular chaperones and Parkinson’s disease. Neurobiol. Dis. 160, 105527. doi:10.1016/j.nbd.2021.105527
Huang, S.-K., Lu, C.-W., Lin, T.-Y., and Wang, S.-J. (2022). Neuroprotective role of the B vitamins in the modulation of the central glutamatergic neurotransmission. CNS Neurol. Disord. Drug Targets 21, 292–301. doi:10.2174/1871527320666210902165739
Huang, X., Li, N., Pu, Y., Zhang, T., and Wang, B. (2019). Neuroprotective effects of ginseng phytochemicals: Recent perspectives. Molecules 24, 2939. doi:10.3390/molecules24162939
Hung, K.-C., Huang, H.-J., Wang, Y.-T., and Lin, A. M.-Y. (2016). Baicalein attenuates α-synuclein aggregation, inflammasome activation and autophagy in the MPP+-treated nigrostriatal dopaminergic system in vivo. J. Ethnopharmacol. 194, 522–529. doi:10.1016/j.jep.2016.10.040
Hussien, H. M., Abd-Elmegied, A., Ghareeb, D. A., Hafez, H. S., Ahmed, H. E. A., and El-moneam, N. A. (2018). Neuroprotective effect of berberine against environmental heavy metals-induced neurotoxicity and Alzheimer’s-like disease in rats. Food Chem. Toxicol. 111, 432–444. doi:10.1016/j.fct.2017.11.025
Huy, N. T., Serada, S., Thi, D., Trang, X., Takano, R., Kondo, Y., et al. (2003). Neutralization of toxic heme by Plasmodium falciparum histidine- rich protein 2. J. Biochem. (Tokyo) 133, 693–698. doi:10.1093/jb/mvg089
Hviid, L., and Jensen, A. T. R. (2015). “PfEMP1 – a parasite protein family of key importance in Plasmodium falciparum malaria immunity and pathogenesis,” in Advances in parasitology. Editors D. Rollinson,, and J. R. Stothard (San Diego: Academic Press), 51–84. doi:10.1016/bs.apar.2015.02.004
Idro, R., Kakooza-Mwesige, A., Asea, B., Ssebyala, K., Bangirana, P., Opoka, R. O., et al. (2016). Cerebral malaria is associated with long-term mental health disorders: A cross sectional survey of a long-term cohort. Malar. J. 15, 184. doi:10.1186/s12936-016-1233-6
Islahudin, F., Tindall, S. M., Mellor, I. R., Swift, K., Christensen, H. E. M., Fone, K. C. F., et al. (2014). The antimalarial drug quinine interferes with serotonin biosynthesis and action. Sci. Rep. 4, 3618. doi:10.1038/srep03618
Jain, K., Sood, S., and Gowthamarajan, K. (2013). Modulation of cerebral malaria by curcumin as an adjunctive therapy. Braz. J. Infect. Dis. 17, 579–591. doi:10.1016/j.bjid.2013.03.004
Jamwal, S., and Kumar, P. (2019). Insight into the emerging role of striatal neurotransmitters in the pathophysiology of Parkinson’s disease and huntington’s disease: A review. Curr. Neuropharmacol. 17, 165–175. doi:10.2174/1570159X16666180302115032
Juárez Olguín, H., Calderón Guzmán, D., Hernández García, E., and Barragán Mejía, G. (2015). The role of dopamine and its dysfunction as a consequence of oxidative stress. Oxid. Med. Cell. Longev. 2016, e9730467. doi:10.1155/2016/9730467
Kaltschmidt, B., and Kaltschmidt, C. (2009). NF-kappaB in the nervous system. Cold Spring Harb. Perspect. Biol. 1, a001271. doi:10.1101/cshperspect.a001271
Kam, A., Li, K. M., Razmovski-Naumovski, V., Nammi, S., Chan, K., Li, Y., et al. (2012). The protective effects of natural products on blood-brain barrier breakdown. Curr. Med. Chem. 19, 1830–1845. doi:10.2174/092986712800099794
Katusic, Z. S., Stelter, A., and Milstien, S. (1998). Cytokines stimulate GTP cyclohydrolase I gene expression in cultured human umbilical vein endothelial cells. Arterioscler. Thromb. Vasc. Biol. 18, 27–32. doi:10.1161/01.atv.18.1.27
Kaur, K., Jain, M., Kaur, T., and Jain, R. (2009). Antimalarials from nature. Bioorg. Med. Chem. 17, 3229–3256. doi:10.1016/j.bmc.2009.02.050
Kelly, A., and Stanley, C. A. (2001). Disorders of glutamate metabolism. Ment. Retard. Dev. Disabil. Res. Rev. 7, 287–295. doi:10.1002/mrdd.1040
Kempaiah, P., Dokladny, K., Karim, Z., Raballah, E., Ong’echa, J. M., Moseley, P. L., et al. (2016). Reduced Hsp70 and glutamine in pediatric severe malaria anemia: Role of hemozoin in suppressing Hsp70 and NF-κB activation. Mol. Med. 22, 570–584. doi:10.2119/molmed.2016.00130
Khanam, S. (2017). Prevalence and epidemiology of malaria in Nigeria : A review. Int. J. Res. Pharm. Biosci. 4, 10–12.
Kihara, M., Carter, J. A., and Newton, C. R. J. C. (2006). The effect of Plasmodium falciparum on cognition: A systematic review. Trop. Med. Int. Health 11, 386–397. doi:10.1111/j.1365-3156.2006.01579.x
Kim, C.-Y., Seo, Y., Lee, C., Park, G. H., and Jang, J.-H. (2018). Neuroprotective effect and molecular mechanism of [6]-Gingerol against scopolamine-induced amnesia in C57bl/6 mice. Evid. Based. Complement. Altern. Med. 2018, e8941564. doi:10.1155/2018/8941564
Klenke, C., Widera, D., Engelen, T., Müller, J., Noll, T., Niehaus, K., et al. (2013). Hsc70 is a novel interactor of NF-kappaB p65 in living hippocampal neurons. PLoS ONE 8, e65280. doi:10.1371/journal.pone.0065280
Krashia, P., Nobili, A., and D’Amelio, M. (2019). Unifying hypothesis of dopamine neuron loss in neurodegenerative diseases: Focusing on alzheimer’s disease. Front. Mol. Neurosci. 12, 123. doi:10.3389/fnmol.2019.00123
Kumar, A. (2015). NMDA receptor function during senescence: Implication on cognitive performance. Front. Neurosci. 9, 473. doi:10.3389/fnins.2015.00473
Kumar, S. P., and Babu, P. P. (2020). Aberrant dopamine receptor signaling plays critical role in the impairment of striatal neurons in experimental cerebral malaria. Mol. Neurobiol. 57, 5069–5083. doi:10.1007/s12035-020-02076-0
Lewerenz, J., and Maher, P. (2015). Chronic glutamate toxicity in neurodegenerative diseases—what is the evidence? Front. Neurosci. 9, 469. doi:10.3389/fnins.2015.00469
Liao, D., Xiang, D., Dang, R., Xu, P., Wang, J., Han, W., et al. (2018). Neuroprotective effects of dl-3-n-Butylphthalide against doxorubicin-induced neuroinflammation, oxidative stress, endoplasmic reticulum stress, and behavioral changes. Oxid. Med. Cell. Longev. 2018, e9125601. doi:10.1155/2018/9125601
Liu, K., and Houry, W. A. (2014). “Chaperones and proteases of Plasmodium falciparum,” in Heat shock proteins of malaria. Editors A. Shonhai,, and G. L. Blatch (Dordrecht: Springer), 161–187. doi:10.1007/978-94-007-7438-4_9
Liu, Y.-N., Peng, Y.-L., -Liu, L., Wu, T.-Y., Zhang, Y., Lian, Y.-J., et al. (2015). TNFα mediates stress-induced depression by upregulating indoleamine 2, 3-dioxygenase in a mouse model of unpredictable chronic mild stress. Eur. Cytokine Netw. 26, 15–25. doi:10.1684/ecn.2015.0362
Lu, A., Ran, R., Parmentier-Batteur, S., Nee, A., and Sharp, F. R. (2002). Geldanamycin induces heat shock proteins in brain and protects against focal cerebral ischemia. J. Neurochem. 81, 355–364. doi:10.1046/j.1471-4159.2002.00835.x
Lugo-Huitrón, R., Ugalde Muñiz, P., Pineda, B., Pedraza-Chaverrí, J., Ríos, C., and Pérez-de la Cruz, V. (2013). Quinolinic acid: An endogenous neurotoxin with multiple targets. Oxid. Med. Cell. Longev. 2013, e104024. doi:10.1155/2013/104024
Lyu, Q., Wawrzyniuk, M., Rutten, V. P. M. G., van Eden, W., Sijts, A. J. A. M., and Broere, F. (2020). Hsp70 and NF-kB mediated control of innate inflammatory responses in a canine macrophage cell line. Int. J. Mol. Sci. 21, E6464. doi:10.3390/ijms21186464
MacCormick, I. J. C., Beare, N. A. V., Taylor, T. E., Barrera, V., White, V. A., Hiscott, P., et al. (2014). Cerebral malaria in children: Using the retina to study the brain. Brain 137, 2119–2142. doi:10.1093/brain/awu001
Mackinnon, S., Durst, T., Arnason, J. T., Angerhofer, C., Pezzuto, J., Sanchez-Vindas, P. E., et al. (1997). Antimalarial activity of tropical meliaceae extracts and gedunin derivatives. J. Nat. Prod. 3864, 336–341. doi:10.1021/np9605394
Mahomoodally, M. F. (2013). Traditional medicines in Africa: An appraisal of ten potent african medicinal plants. Evid. Based. Complement. Altern. Med. 2013, 617459. doi:10.1155/2013/617459
Maier, A. G., Cooke, B. M., Cowman, A. F., and Tilley, L. (2009). Malaria parasite proteins that remodel the host erythrocyte. Nat. Rev. Microbiol. 7, 341–354. doi:10.1038/nrmicro2110
Maier, A. G., Rug, M., O’Neill, M. T., Brown, M., Chakravorty, S., Szestak, T., et al. (2008). Exported proteins required for virulence and rigidity of Plasmodium falciparum-infected human erythrocytes. Cell 134, 48–61. doi:10.1016/j.cell.2008.04.051
Mallozzi, C., Parravano, M., Gaddini, L., Villa, M., Pricci, F., Malchiodi-Albedi, F., et al. (2018). Curcumin modulates the NMDA receptor subunit composition through a mechanism involving CaMKII and ser/thr protein phosphatases. Cell. Mol. Neurobiol. 38, 1315–1320. doi:10.1007/s10571-018-0595-4
Martí Coma-Cros, E., Biosca, A., Lantero, E., Manca, M. L., Caddeo, C., Gutiérrez, L., et al. (2018). Antimalarial activity of orally administered curcumin incorporated in eudragit®-containing liposomes. Int. J. Mol. Sci. 19, 1361. doi:10.3390/ijms19051361
Mathew, B., Suresh, J., Elizabeth Mathew, G., Haridas, A., Suresh, G., and Sabreena, P. (2016). Synthesis, ADME studies, toxicity estimation, and exploration of molecular recognition of thiophene based chalcones towards monoamine oxidase-A and B. Beni. Suef. Univ. J. Basic Appl. Sci. 5, 396–401. doi:10.1016/j.bjbas.2015.06.003
Matteucci, A., Cammarota, R., Paradisi, S., Varano, M., Balduzzi, M., Leo, L., et al. (2011). Curcumin protects against NMDA-induced toxicity: A possible role for NR2A subunit. Invest. Ophthalmol. Vis. Sci. 52, 1070–1077. doi:10.1167/iovs.10-5966
McEwen, B. S., and Milner, T. A. (2017). Understanding the broad influence of sex hormones and sex differences in the brain. J. Neurosci. Res. 95, 24–39. doi:10.1002/jnr.23809
Midorikawa, Y., and Haque, Q. M. (1997). 15-Deoxyspergualin, an immunosuppressive agent, used in organ transplantation showed suppressive effects on malarial parasites. Chemotherapy 43, 31–35. doi:10.1159/000239532
Miller, A. H., Haroon, E., Raison, C. L., and Felger, J. C. (2013). Cytokine targets in the brain: Impact on neurotransmitters and neurocircuits. Depress. Anxiety 30, 297–306. doi:10.1002/da.22084
Milner, D. A. (2018). Malaria pathogenesis. Cold Spring Harb. Perspect. Med. 8, a025569–12. doi:10.1101/cshperspect.a025569
Milner, D. A., Whitten, R. O., Kamiza, S., Carr, R., Liomba, G., Dzamalala, C., et al. (2014). The systemic pathology of cerebral malaria in African children. Front. Cell. Infect. Microbiol. 4, 104–113. doi:10.3389/fcimb.2014.00104
Mishra, K., Dash, A. P., and Dey, N. (2011). Andrographolide: A novel antimalarial diterpene lactone compound from andrographis paniculata and its interaction with curcumin and artesunate. J. Trop. Med. 2011, 579518. doi:10.1155/2011/579518
Miyazaki, I., and Asanuma, M. (2009). Approaches to prevent dopamine quinone-induced neurotoxicity. Neurochem. Res. 34, 698–706. doi:10.1007/s11064-008-9843-1
Montagna, G. N., Matuschewski, K., and Buscaglia, C. A. (2012). Small heat shock proteins in cellular adhesion and migration: Evidence from Plasmodium genetics. Cell adh. Migr. 6, 78–84. doi:10.4161/cam.20101
Monteiro, M. C., Oliveira, F. R., Oliveira, G. B., Romao, P. R. T., and Maia, C. S. F. (2014). Neurological and behavioral manifestations of cerebral malaria: An update. World J. Transl. Med. 3, 9–16. doi:10.5528/wjtm.v3.i1.9
Monzani, E., Nicolis, S., Dell’Acqua, S., Capucciati, A., Bacchella, C., Zucca, F. A., et al. (2019). Dopamine, oxidative stress and protein–quinone modifications in Parkinson’s and other neurodegenerative diseases. Angew. Chem. Int. Ed. Engl. 58, 6512–6527. doi:10.1002/anie.201811122
Morroni, F., Sita, G., Graziosi, A., Turrini, E., Fimognari, C., Tarozzi, A., et al. (2018). Neuroprotective effect of caffeic acid phenethyl ester in A mouse model of alzheimer’s disease involves Nrf2/HO-1 pathway. Aging Dis. 9, 605–622. doi:10.14336/AD.2017.0903
Moses, M. A., Henry, E. C., Ricke, W. A., and Gasiewicz, T. A. (2015). The heat shock protein 90 inhibitor, (-)-epigallocatechin gallate, has anticancer activity in a novel human prostate cancer progression model. Cancer Prev. Res. phila. Pa.) 8, 249–257. doi:10.1158/1940-6207.CAPR-14-0224
Murphy, B. L., Arnsten, A. F., Goldman-Rakic, P. S., and Roth, R. H. (1996). Increased dopamine turnover in the prefrontal cortex impairs spatial working memory performance in rats and monkeys. Proc. Natl. Acad. Sci. U. S. A. 93, 1325–1329. doi:10.1073/pnas.93.3.1325
Murwih Alidmat, M., Khairuddean, M., Mohammad Norman, N., Mohamed Asri, A. N., Mohd Suhaimi, M. H., and Sharma, G. (2021). Synthesis, characterization, docking study and biological evaluation of new chalcone, pyrazoline, and pyrimidine derivatives as potent antimalarial compounds. Arab. J. Chem. 14, 103304. doi:10.1016/j.arabjc.2021.103304
Nabavi, S. F., Braidy, N., Habtemariam, S., Orhan, I. E., Daglia, M., Manayi, A., et al. (2015). Neuroprotective effects of chrysin: From chemistry to medicine. Neurochem. Int. 90, 224–231. doi:10.1016/j.neuint.2015.09.006
Newton, C. R. J. C., Hien, T. T., and White, N. (2000). Cerebral malaria. J. Neurol. Neurosurg. Psychiatry 69, 433–441. doi:10.1136/jnnp.69.4.433
Nishanth, G., and Schlüter, D. (2019). Blood–brain barrier in cerebral malaria: Pathogenesis and therapeutic intervention. Trends Parasitol. 35, 516–528. doi:10.1016/j.pt.2019.04.010
Nkumama, I. N., Meara, W. P. O., and Osier, F. H. A. (2016). Changes in malaria epidemiology in Africa and new challenges for elimination. Trends Parasitol. 33, 128–140. doi:10.1016/j.pt.2016.11.006
Oliveira, K. R. H. M., Kauffmann, N., Leão, L. K. R., Passos, A. C. F., Rocha, F. A. F., Herculano, A. M., et al. (2017). Cerebral malaria induces electrophysiological and neurochemical impairment in mice retinal tissue: Possible effect on glutathione and glutamatergic system. Malar. J. 16, 440. doi:10.1186/s12936-017-2083-6
Oluwatosin, A., Tolulope, A., Ayokulehin, K., Patricia, O., Aderemi, K., Catherine, F., et al. (2014). Antimalarial potential of kolaviron, a biflavonoid from Garcinia kola seeds, against Plasmodium berghei infection in Swiss albino mice. Asian pac. J. Trop. Med. 7, 97–104. doi:10.1016/S1995-7645(14)60003-1
Oluwayemi, I. O., Brown, B. J., Oyedeji, O. A., and Oluwayemi, M. A. (2013). Neurological sequelae in survivors of cerebral malaria. Pan Afr. Med. J. 15, 88–89. doi:10.11604/pamj.2013.15.88.1897
Ounjaijean, S., and Somsak, V. (2020). Combination of zingerone and dihydroartemisinin presented synergistic antimalarial activity against Plasmodium berghei infection in BALB/c mice as in vivo model. Parasitol. Int. 76, 102088. doi:10.1016/j.parint.2020.102088
Pal, P., Balaban, A. E., Diamond, M. S., Sinnis, P., Klein, R. S., and Goldberg, D. E. (2017). Plasmodium falciparum histidine-rich protein II causes vascular leakage and exacerbates experimental cerebral malaria in mice. PLoS ONE 12, e0177142. doi:10.1371/journal.pone.0177142
Pal, P., Daniels, B. P., Oskman, A., Diamond, M. S., Klein, R. S., and Goldberg, D. E. (2016). Plasmodium falciparum histidine-rich protein II compromises brain endothelial barriers and may promote cerebral malaria pathogenesis. mBio 7, e00617–16. doi:10.1128/mBio.00617-16
Pallavi, R., Acharya, P., Chandran, S., Daily, J. P., and Tatu, U. (2010). Chaperone expression profiles correlate with distinct physiological states of Plasmodium falciparum in malaria patients. Malar. J. 9, 236. doi:10.1186/1475-2875-9-236
Pasternak, N. D., and Dzikowski, R. (2009). PfEMP1: An antigen that plays a key role in the pathogenicity and immune evasion of the malaria parasite Plasmodium falciparum. Int. J. Biochem. Cell Biol. 41, 1463–1466. doi:10.1016/j.biocel.2008.12.012
Pastukhov, Yu. F., Ekimova, I. V., Guzhova, I. V., Romanova, I. V., and Artyukhina, Z. E. (2013). Chaperone Hsp70 content in dopaminergic neurons of the substantia nigra increases in proteasome dysfunction. Neurosci. Behav. Physiol. 43, 380–387. doi:10.1007/s11055-013-9744-x
Patwardhan, C. A., Fauq, A., Peterson, L. B., Miller, C., Blagg, B. S. J., and Chadli, A. (2013). Gedunin inactivates the co-chaperone p23 protein causing cancer cell death by apoptosis. J. Biol. Chem. 288, 7313–7325. doi:10.1074/jbc.M112.427328
Pavithra, S. R., Kumar, R., and Tatu, U. (2007). Systems analysis of chaperone networks in the malarial parasite Plasmodium falciparum. PLoS Comput. Biol. 3, 1701–1715. doi:10.1371/journal.pcbi.0030168
Pesce, E.-R., Cockburn, I. L., Goble, J. L., Stephens, L. L., and Blatch, G. L. (2010). Malaria heat shock proteins: Drug targets that chaperone other drug targets. Infect. Disord. Drug Targets 10, 147–157. doi:10.2174/187152610791163417
Pesce, E., and Blatch, G. L. (2014). Plasmodial Hsp40 and Hsp70 chaperones : Current and future perspectives. Parasitology 141, 1167–1176. doi:10.1017/S003118201300228X
Petrović, A., Kaur, J., Tomljanović, I., Nistri, A., and Mladinic, M. (2019). Pharmacological induction of Heat Shock Protein 70 by celastrol protects motoneurons from excitotoxicity in rat spinal cord in vitro. Eur. J. Neurosci. 49, 215–231. doi:10.1111/ejn.14218
Piaz, F. D., Malafronte, N., Romano, A., Gallotta, D., Belisario, M. A., Bifulco, G., et al. (2012). Structural characterization of tetranortriterpenes from Pseudrocedrela kotschyi and Trichilia emetica and study of their activity towards the chaperone Hsp90. Phytochemistry 75, 78–89. doi:10.1016/j.phytochem.2011.12.002
Plewes, K., Turner, G. D. H., and Dondorp, A. M. (2018). Pathophysiology, clinical presentation, and treatment of coma and acute kidney injury complicating falciparum malaria. Curr. Opin. Infect. Dis. 31, 69–77. doi:10.1097/QCO.0000000000000419
Ponsford, M. J., Medana, I. M., Prapansilp, P., Hien, T. T., Lee, S. J., Dondorp, A. M., et al. (2012). Sequestration and microvascular congestion are associated with coma in human cerebral malaria. J. Infect. Dis. 205, 663–671. doi:10.1093/infdis/jir812
Posfai, D., Eubanks, A. L., Keim, A. I., Lu, K. Y., Wang, G. Z., Hughes, P. F., et al. (2018). Identification of Hsp90 inhibitors with anti-plasmodium activity. Antimicrob. Agents Chemother. 62, e01799–17. doi:10.1128/AAC.01799-17
Pussard, E., Bernier, A., Fouquet, E., and Bouree, P. (2003). Quinine distribution in mice withplasmodium berghei malaria. Eur. J. Drug Metab. Pharmacokinet. 28, 11–20. doi:10.1007/BF03190862
Pussard, E., Merzouk, M., and Barennes, H. (2007). Increased uptake of quinine into the brain by inhibition of P-glycoprotein. Eur. J. Pharm. Sci. 32, 123–127. doi:10.1016/j.ejps.2007.06.007
Quinlan, M. G., Hussain, D., and Brake, W. G. (2008). Use of cognitive strategies in rats: The role of estradiol and its interaction with dopamine. Horm. Behav. 53, 185–191. doi:10.1016/j.yhbeh.2007.09.015
Raghavan, R., Cheriyamundath, S., and Madassery, J. (2012). Andrographolide, a new potential NF-κB inhibitor: Docking simulation and evaluation of drug-likeness. Mol. Simul. 38, 582–588. doi:10.1080/08927022.2011.651138
Ramya, T. N. C., Karmodiya, K., Surolia, A., and Surolia, N. (2007). 15-Deoxyspergualin primarily targets the trafficking of apicoplast proteins in Plasmodium falciparum. J. Biol. Chem. 282, 6388–6397. doi:10.1074/jbc.M610251200
Rangel-Barajas, C., Coronel, I., and Florán, B. (2015). Dopamine receptors and neurodegeneration. Aging Dis. 6, 349–368. doi:10.14336/AD.2015.0330
Rangel-Gomez, M., and Meeter, M. (2016). Neurotransmitters and novelty: A systematic review. J. Psychopharmacol. 30, 3–12. doi:10.1177/0269881115612238
Rao, A., Kumar, M. K., Joseph, T., and Bulusu, G. (2010). Cerebral malaria: Insights from host-parasite protein-protein interactions. Malar. J. 9, 155. doi:10.1186/1475-2875-9-155
Reiner, A., and Levitz, J. (2018). Glutamatergic signaling in the central nervous system: Ionotropic and metabotropic receptors in concert. Neuron 98, 1080–1098. doi:10.1016/j.neuron.2018.05.018
Rosenberg, G. A. (2012). Neurological diseases in relation to the blood – brain barrier. J. Cereb. Blood Flow. Metab. 32, 1139–1151. doi:10.1038/jcbfm.2011.197
Roy, S., Chattopadhyay, R. N., and Maitra, S. K. (1993). Changes in brain neurotransmitters in rodent malaria. Indian J. Malariol. 30, 183–185.
Rubach, M. P., Mukemba, J., Florence, S., Lopansri, B. K., Hyland, K., Volkheimer, A. D., et al. (2015). Impaired systemic tetrahydrobiopterin bioavailability and increased oxidized biopterins in pediatric falciparum malaria: Association with disease severity. PLoS Pathog. 11, e1004655. doi:10.1371/journal.ppat.1004655
Sahu, P. K., Satpathi, S., Behera, P. K., Mishra, S. K., Mohanty, S., and Wassmer, S. C. (2015). Pathogenesis of cerebral malaria: New diagnostic tools, biomarkers, and therapeutic approaches. Front. Cell. Infect. Microbiol. 5, 75. doi:10.3389/fcimb.2015.00075
Sakai, N., Kaufman, S., and Milstien, S. (1995). Parallel induction of nitric oxide and tetrahydrobiopterin synthesis by cytokines in rat glial cells. J. Neurochem. 65, 895–902. doi:10.1046/j.1471-4159.1995.65020895.x
Salehi, B., Quispe, C., Chamkhi, I., El Omari, N., Balahbib, A., Sharifi-Rad, J., et al. (2021). Pharmacological properties of chalcones: A review of preclinical including molecular mechanisms and clinical evidence. Front. Pharmacol. 11, 592654. doi:10.3389/fphar.2020.592654
Santos, J., Quimque, M. T., Liman, R. A., Agbay, J. C., Macabeo, A. P. G., Corpuz, M. J.-A., et al. (2021). Computational and experimental assessments of magnolol as a neuroprotective agent and utilization of UiO-66(Zr) as its drug delivery system. ACS Omega 6, 24382–24396. doi:10.1021/acsomega.1c02555
Schiess, N., Villabona-Rueda, A., Cottier, K. E., Huether, K., Chipeta, J., and Stins, M. F. (2020). Pathophysiology and neurologic sequelae of cerebral malaria. Malar. J. 19, 266(1–12). doi:10.1186/s12936-020-03336-z
Segura, C., Cuesta-Astroz, Y., Nunes-Batista, C., Zalis, M., von Krüger, W. M. de A., and Mascarello Bisch, P. (2014). Partial characterization of Plasmodium falciparum trophozoite proteome under treatment with quinine, mefloquine and the natural antiplasmodial diosgenone. Biomedica 34, 237–249. doi:10.1590/S0120-41572014000200010
Senf, S. M., Dodd, S. L., McClung, J. M., and Judge, A. R. (2008). Hsp70 overexpression inhibits NF-kappaB and Foxo3a transcriptional activities and prevents skeletal muscle atrophy. FASEB J. 22, 3836–3845. doi:10.1096/fj.08-110163
Shahinas, D., Folefoc, A., and Pillai, D. R. (2013a). Targeting Plasmodium falciparum Hsp90: Towards reversing antimalarial resistance. Pathogens 2, 33–54. doi:10.3390/pathogens2010033
Shahinas, D., Folefoc, A., Taldone, T., Chiosis, G., Crandall, I., and Pillai, D. R. (2013b). A purine analog synergizes with chloroquine ( CQ ) by targeting Plasmodium falciparum Hsp90 (PfHsp90). PloS One 8 (1-13), e75446. doi:10.1371/journal.pone.0075446
Sheffler, Z. M., Reddy, V., and Pillarisetty, L. S. (2022). In StatPearls. Treasure Island (FL): StatPearls Publishing, Physiology, neurotransmitters.
Shih, R.-H., Wang, C.-Y., and Yang, C.-M. (2015). NF-kappaB signaling pathways in neurological inflammation: A mini review. Front. Mol. Neurosci. 8, 77. doi:10.3389/fnmol.2015.00077
Shikani, H. J., Freeman, B. D., Lisanti, M. P., Weiss, L. M., Tanowitz, H. B., and Desruisseaux, M. S. (2012). Cerebral malaria: We have come a long way. Am. J. Pathol. 181, 1484–1492. doi:10.1016/j.ajpath.2012.08.010
Shimazu, R., Anada, M., Miyaguchi, A., Nomi, Y., and Matsumoto, H. (2021). Evaluation of blood–brain barrier permeability of polyphenols, anthocyanins, and their metabolites. J. Agric. Food Chem. 69, 11676–11686. doi:10.1021/acs.jafc.1c02898
Shimizu, F., Nishihara, H., and Kanda, T. (2018). Blood – brain barrier dysfunction in immuno- mediated neurological diseases. Immunol. Med. 41, 120–128. doi:10.1080/25785826.2018.1531190
Shonhai, A., Picard, D., and Blatch, G. L. (Editors) (2021). Heat shock proteins of malaria. 2nd Edition (Germany: Springer Nature Switzerland AG).
Shonhai, A. (2010). Plasmodial heat shock proteins: Targets forchemotherapy. FEMS Immunol. Med. Microbiol. 58, 61–74. doi:10.1111/j.1574-695X.2009.00639.x
Shrestha, L., Bolaender, A., Patel, H. J., and Taldone, T. (2016). Heat shock protein (HSP) drug discovery and development: Targeting heat shock proteins in disease. Curr. Top. Med. Chem. 16, 2753–2764. doi:10.2174/1568026616666160413141911
Sierro, F., and Grau, G. E. R. (2019a). The ins and outs of cerebral malaria pathogenesis: Immunopathology, extracellular vesicles, immunometabolism, and trained immunity. Front. Immunol. 10, 830. doi:10.3389/fimmu.2019.00830
Sierro, F., and Grau, G. E. R. (2019b). The ins and outs of cerebral malaria pathogenesis: Immunopathology, extracellular vesicles, immunometabolism, and trained immunity. Front. Immunol. 10, 830(1–11). doi:10.3389/fimmu.2019.00830
Silamut, K., White, N. J., Looareesuwan, S., and Warrell, D. A. (1985). Binding of quinine to plasma proteins in falciparum malaria. Am. J. Trop. Med. Hyg. 34, 681–686. doi:10.4269/ajtmh.1985.34.681
Simões, A. P., Silva, C. G., Marques, J. M., Pochmann, D., Porciúncula, L. O., Ferreira, S., et al. (2018). Glutamate-induced and NMDA receptor-mediated neurodegeneration entails P2Y1 receptor activation. Cell Death Dis. 2012 (6), 1–50. doi:10.1038/s41419-018-0351-1
Sinclair, D., Donegan, S., Isba, R., and Lalloo, D. G. (2012). Artesunate versus quinine for treating severe malaria. Cochrane Database Syst. Rev. 2012, CD005967. doi:10.1002/14651858.CD005967.pub4
Singh, P., Anand, A., and Kumar, V. (2014). Recent developments in biological activities of chalcones: A mini review. Eur. J. Med. Chem. 85, 758–777. doi:10.1016/j.ejmech.2014.08.033
Singh, S., Jamwal, S., and Kumar, P. (2017). Neuroprotective potential of Quercetin in combination with piperine against 1-methyl-4-phenyl-1, 2, 3, 6-tetrahydropyridine-induced neurotoxicity. Neural Regen. Res. 12, 1137–1144. doi:10.4103/1673-5374.211194
Singh, S. K., Dwivedi, H., Gunjan, S., Chauhan, B. S., Pandey, S. K., and Tripathi, R. (2019). Potential role of arteether on N-methyl-D-aspartate (NMDA) receptor expression in experimental cerebral malaria mice and extension of their survival. Parasitology 146, 1571–1577. doi:10.1017/S0031182019000878
Sinha, S., Prakash, A., Medhi, B., Sehgal, A., Batovska, D. I., and Sehgal, R. (2021). Pharmacokinetic evaluation of Chalcone derivatives with antimalarial activity in New Zealand White Rabbits. BMC Res. Notes 14, 264. doi:10.1186/s13104-021-05684-8
Smith, D. F., Whitesell, L., and Katsanis, E. (1998). Molecular chaperones: Biology and prospects for pharmacological intervention. Pharmacol. Rev. 50, 493–514.
Smith, H. L., Li, W., and Cheetham, M. E. (2015). Molecular chaperones and neuronal proteostasis. Semin. Cell Dev. Biol. 40, 142–152. doi:10.1016/j.semcdb.2015.03.003
Sowndhararajan, K., Deepa, P., Kim, M., Park, S. J., and Kim, S. (2018). Neuroprotective and cognitive enhancement potentials of baicalin: A review. Brain Sci. 8, 104. doi:10.3390/brainsci8060104
Spielmann, T., and Gilberger, T.-W. (2010). Protein export in malaria parasites: Do multiple export motifs add up to multiple export pathways? Trends Parasitol. 26, 6–10. doi:10.1016/j.pt.2009.10.001
Spycher, C., Rug, M., Pachlatko, E., Hanssen, E., Ferguson, D., Cowman, A. F., et al. (2008). The Maurer’s cleft protein MAHRP1 is essential for trafficking of PfEMP1 to the surface of Plasmodium falciparum-infected erythrocytes. Mol. Microbiol. 68, 1300–1314. doi:10.1111/j.1365-2958.2008.06235.x
Stetler, R. A., Gan, Y., Zhang, W., Liou, A. K., Gao, Y., Cao, G., et al. (2010). Heat shock proteins: Cellular and molecular mechanisms in the central nervous system. Prog. Neurobiol. 92, 184–211. doi:10.1016/j.pneurobio.2010.05.002
Storm, J., and Craig, A. G. (2014a). Pathogenesis of cerebral malaria--inflammation and cytoadherence. Front. Cell. Infect. Microbiol. 4, 100. doi:10.3389/fcimb.2014.00100
Storm, J., and Craig, A. G. (2014b). Pathogenesis of cerebral malaria—Inflammation and cytoadherence. Front. Cell. Infect. Microbiol. 4, 100. doi:10.3389/fcimb.2014.00100
Storm, J., Jespersen, J. S., Seydel, K. B., Szestak, T., Mbewe, M., Chisala, N. V., et al. (2019). Cerebral malaria is associated with differential cytoadherence to brain endothelial cells. EMBO Mol. Med. 11, e9164–15. doi:10.15252/emmm.201809164
Subedi, L., and Gaire, B. P. (2021). Neuroprotective effects of curcumin in cerebral ischemia: Cellular and molecular mechanisms. ACS Chem. Neurosci. 12, 2562–2572. doi:10.1021/acschemneuro.1c00153
Suda, M., Honma, T., Miyagawa, M., and Wang, R.-S. (2008). Alteration of brain levels of neurotransmitters and amino acids in male F344 rats induced by three-week repeated inhalation exposure to 1-bromopropane. Ind. Health 46, 348–359. doi:10.2486/indhealth.46.348
Talapko, J., Škrlec, I., Alebić, T., Jukić, M., and Včev, A. (2019). Malaria: The past and the present. Microorganisms 7 (6), 179(1–17). doi:10.3390/microorganisms7060179
Tozzi, A., de Iure, A., Tantucci, M., Durante, V., Quiroga-Varela, A., Giampà, C., et al. (2015). Endogenous 17β-estradiol is required for activity-dependent long-term potentiation in the striatum: Interaction with the dopaminergic system. Front. Cell. Neurosci. 9, 192. doi:10.3389/fncel.2015.00192
Tran, P. L. C. H. B., Kim, S. A., Choi, H. S., Yoon, J. H., and Ahn, S. G. (2010). Epigallocatechin-3-gallate suppresses the expression of HSP70 and HSP90 and exhibits anti-tumor activity in vitro and in vivo. BMC Cancer 10, 276. doi:10.1186/1471-2407-10-276
Tripathi, A. K., Dwivedi, A., Pal, M. K., Rastogi, N., Gupta, P., Ali, S., et al. (2014). Attenuated neuroprotective effect of riboflavin under UV-B irradiation via miR-203/c-Jun signaling pathway in vivo and in vitro. J. Biomed. Sci. 21, 39. doi:10.1186/1423-0127-21-39
Tripathi, A. K., Sha, W., Shulaev, V., Stins, M. F., and Sullivan, D. J. (2009). Plasmodium falciparum-infected erythrocytes induce NF-kappaB regulated inflammatory pathways in human cerebral endothelium. Blood 114, 4243–4252. doi:10.1182/blood-2009-06-226415
Tzeng, Y.-M., and Lee, M.-J. (2015). Neuroprotective properties of kavalactones. Neural Regen. Res. 10, 875–877. doi:10.4103/1673-5374.158335
van der Heyde, H. C., Nolan, J., Combes, V., Gramaglia, I., and Grau, G. E. (2006). A unified hypothesis for the Genesis of cerebral malaria: Sequestration, inflammation and hemostasis leading to microcirculatory dysfunction. Trends Parasitol. 22, 503–508. doi:10.1016/j.pt.2006.09.002
van Holstein, M., Aarts, E., van der Schaaf, M. E., Geurts, D. E. M., Verkes, R. J., Franke, B., et al. (2011). Human cognitive flexibility depends on dopamine D2 receptor signaling. Psychopharmacol. (Berl.) 218, 567–578. doi:10.1007/s00213-011-2340-2
Vauzour, D. (2012). Dietary polyphenols as modulators of brain functions: Biological actions and molecular mechanisms underpinning their beneficial effects. Oxid. Med. Cell. Longev. 2012, e914273. doi:10.1155/2012/914273
Velaga, M. K., Basuri, C. K., Robinson Taylor, K. S., Yallapragada, P. R., Rajanna, S., and Rajanna, B. (2014). Ameliorative effects of Bacopa monniera on lead-induced oxidative stress in different regions of rat brain. Drug Chem. Toxicol. 37, 357–364. doi:10.3109/01480545.2013.866137
Vijayan, V., Bhaskar, S., Kavitha, S. K., Ratheesh, M., Kripa, K., and Helen, A. (2010). Betulinic acid isolated from Bacopa monniera (L.) Wettst suppresses lipopolysaccharide stimulated interleukin-6 production through modulation of nuclear factor-kappaB in peripheral blood mononuclear cells. Int. Immunopharmacol. 10, 843–849. doi:10.1016/j.intimp.2010.04.013
Walker, M. C., and van der Donk, W. A. (2016). The many roles of glutamate in metabolism. J. Ind. Microbiol. Biotechnol. 43, 419–430. doi:10.1007/s10295-015-1665-y
Wang, C.-H., Chou, P.-C., Chung, F.-T., Lin, H.-C., Huang, K.-H., and Kuo, H.-P. (2017a). Heat shock protein70 is implicated in modulating NF-κB activation in alveolar macrophages of patients with active pulmonary tuberculosis. Sci. Rep. 7, 1214. doi:10.1038/s41598-017-01405-z
Wang, G., Tang, W., and Bidigare, R. R. (2005). “Terpenoids as therapeutic drugs and pharmaceutical agents,” in Natural products: Drug discovery and therapeutic medicine. Editors L. Zhang,, and A. L. Demain (Totowa, NJ: Humana Press), 197–227. doi:10.1007/978-1-59259-976-9_9
Wang, H.-C., Wang, B.-D., Chen, M.-S., Chen, H., Sun, C.-F., Shen, G., et al. (2018). Neuroprotective effect of berberine against learning and memory deficits in diffuse axonal injury. Exp. Ther. Med. 15, 1129–1135. doi:10.3892/etm.2017.5496
Wang, X.-S., Zhang, Z.-R., Zhang, M.-M., Sun, M.-X., Wang, W.-W., and Xie, C.-L. (2017b). Neuroprotective properties of curcumin in toxin-base animal models of Parkinson’s disease: A systematic experiment literatures review. BMC Complement. Altern. Med. 17, 412. doi:10.1186/s12906-017-1922-x
Webster, J. M., Darling, A. L., Uversky, V. N., and Blair, L. J. (2019). Small heat shock proteins, big impact on protein aggregation in neurodegenerative disease. Front. Pharmacol. 10, 1047. doi:10.3389/fphar.2019.01047
Westerheide, S. D., Bosman, J. D., Mbadugha, B. N. A., Kawahara, T. L. A., Matsumoto, G., Kim, S., et al. (2004). Celastrols as inducers of the heat shock response and cytoprotection. J. Biol. Chem. 279, 56053–56060. doi:10.1074/jbc.M409267200
White, N. J., Looareesuwan, S., Warrell, D. A., Warrell, M. J., Bunnag, D., and Harinasuta, T. (1982). Quinine pharmacokinetics and toxicity in cerebral and uncomplicated falciparum malaria. Am. J. Med. 73, 564–572. doi:10.1016/0002-9343(82)90337-0
Wickert, H., Wissing, F., Andrews, K. T., Stich, A., Krohne, G., and Lanzer, M. (2003). Evidence for trafficking of PfEMP1 to the surface of P. falciparum-infected erythrocytes via a complex membrane network. Eur. J. Cell Biol. 82, 271–284. doi:10.1078/0171-9335-00319
Wu, W. C., Wu, M. H., Chang, Y. C., Hsieh, M. C., Wu, H. J., Cheng, K. C., et al. (2010). Geldanamycin and its analog induce cytotoxicity in cultured human retinal pigment epithelial cells. Exp. Eye Res. 91, 211–219. doi:10.1016/j.exer.2010.05.005
Yamaguchi, T., Wang, H.-L., Li, X., Ng, T. H., and Morales, M. (2011). Mesocorticolimbic glutamatergic pathway. J. Neurosci. 31, 8476–8490. doi:10.1523/JNEUROSCI.1598-11.2011
Ye, X., Rong, Z., Li, Y., Wang, X., Cheng, B., Cheng, Y., et al. (2018). Protective role of L-3-n-butylphthalide in cognitive function and dysthymic disorders in mouse with chronic epilepsy. Front. Pharmacol. 9, 734. doi:10.3389/fphar.2018.00734
Yeo, T. W., Lampah, D. A., Kenangalem, E., Tjitra, E., Price, R. N., Weinberg, J. B., et al. (2015). Impaired systemic tetrahydrobiopterin bioavailability and increased dihydrobiopterin in adult falciparum malaria: Association with disease severity, impaired microvascular function and increased endothelial activation. PLoS Pathog. 11, e1004667. doi:10.1371/journal.ppat.1004667
Youn, K., Ho, C.-T., and Jun, M. (2022). Multifaceted neuroprotective effects of (-)-epigallocatechin-3-gallate (EGCG) in alzheimer’s disease: An overview of pre-clinical studies focused on β-amyloid peptide. Food Sci. Hum. Wellness 11, 483–493. doi:10.1016/j.fshw.2021.12.006
Zeiler, F. A., Silvaggio, J., Kaufmann, A. M., Gillman, L. M., and West, M. (2014). Norepinephrine as a potential aggravator of symptomatic cerebral vasospasm: Two cases and argument for milrinone therapy. Case Rep. Crit. Care 2014, 630970. doi:10.1155/2014/630970
Zhang, H. (2012). New insights into huperzine A for the treatment of Alzheimer’s disease. Acta Pharmacol. Sin. 33, 1170–1175. doi:10.1038/aps.2012.128
Zhang, S., Wang, R., and Wang, G. (2018). Impact of dopamine oxidation on dopaminergic neurodegeneration. ACS Chem. Neurosci. 10, 945–953. doi:10.1021/acschemneuro.8b00454
Zhang, T., Hamza, A., Cao, X., Wang, B., Yu, S., Zhan, C.-G., et al. (2008). A novel Hsp90 inhibitor to disrupt Hsp90/Cdc37 complex against pancreatic cancer cells. Mol. Cancer Ther. 7, 162–170. doi:10.1158/1535-7163.MCT-07-0484
Zininga, T., Pooe, O. J., Makhado, P. B., Ramatsui, L., Prinsloo, E., Achilonu, I., et al. (2017a). Polymyxin B inhibits the chaperone activity of Plasmodium falciparum Hsp70. Cell Stress Chaperones 22, 707–715. doi:10.1007/s12192-017-0797-6
Zininga, T., Ramatsui, L., Makhado, P., Makumire, S., Achilinou, I., Hoppe, H., et al. (2017b). (−)-Epigallocatechin-3-Gallate inhibits the chaperone activity of Plasmodium falciparum Hsp70 chaperones and abrogates their association with functional partners. Molecules 22, 2139. doi:10.3390/molecules22122139
Keywords: neurotransmitters, molecular chaperones, cerebral malaria, P. falciparum, cytoadherence, sequestration, inflammation
Citation: Daniyan MO, Fisusi FA and Adeoye OB (2022) Neurotransmitters and molecular chaperones interactions in cerebral malaria: Is there a missing link?. Front. Mol. Biosci. 9:965569. doi: 10.3389/fmolb.2022.965569
Received: 09 June 2022; Accepted: 28 July 2022;
Published: 24 August 2022.
Edited by:
Stanley Makumire, University of Cape Town, South AfricaReviewed by:
Prakash Chandra Mishra, Guru Nanak Dev University, IndiaCopyright © 2022 Daniyan, Fisusi and Adeoye. This is an open-access article distributed under the terms of the Creative Commons Attribution License (CC BY). The use, distribution or reproduction in other forums is permitted, provided the original author(s) and the copyright owner(s) are credited and that the original publication in this journal is cited, in accordance with accepted academic practice. No use, distribution or reproduction is permitted which does not comply with these terms.
*Correspondence: Michael Oluwatoyin Daniyan, bWRhbml5YW5Ab2F1aWZlLmVkdS5uZw==, dG95aW5waGFybUBnbWFpbC5jb20=
Disclaimer: All claims expressed in this article are solely those of the authors and do not necessarily represent those of their affiliated organizations, or those of the publisher, the editors and the reviewers. Any product that may be evaluated in this article or claim that may be made by its manufacturer is not guaranteed or endorsed by the publisher.
Research integrity at Frontiers
Learn more about the work of our research integrity team to safeguard the quality of each article we publish.