- Department of Cell, Developmental and Integrative Biology, University of Alabama at Birmingham, Birmingham, AL, United States
The Golgi-sialyltransferase ST6Gal1 (βgalactosidase α2,6 sialyltransferase 1), adds the negatively charged sugar, sialic acid, to the terminal galactose of N-glycosylated proteins. Upregulation of ST6Gal1 is observed in many malignancies, and a large body of research has determined that ST6Gal1-mediated α2,6 sialylation impacts cancer hallmarks. ST6Gal1 affects oncogenic behaviors including sustained proliferation, enhanced self-renewal, epithelial-to-mesenchymal transition, invasion, and chemoresistance. However, there are relatively few ST6GaL1 related signaling pathways that are well-established to mediate these biologies: greater delineation of specific targets and signaling mechanisms that are orchestrated by ST6Gal1 is needed. The aim of this review is to provide a summary of our current understanding of select oncogenic signaling pathways and targets affected by ST6Gal1.
Introduction
Since abnormal glycosylation in malignancy was first described in 1969, it has been established to affect cancer hallmarks (Meezan et al., 1969; Pinho and Reis, 2015; Munkley and Elliott, 2016; Vajaria and Patel, 2017). Interestingly, a large proportion of cancer biomarkers approved by the FDA are glycosylated proteins, further suggesting the importance of glycobiology in cancer (Moss et al., 2005; Gilgunn et al., 2013; Thomas et al., 2021). One of the glycan changes consistently observed in cancer is hyper-sialylation (Bull et al., 2014; Rodriguez et al., 2018; Li and Ding, 2019; Xu et al., 2021). Sialylation is the process in which sialic acid is added to the terminal end of glycoproteins and glycolipids by enzymes called sialyltransferases. Amongst more than 20 sialyltransferases found in the human body (Harduin-Lepers et al., 2001; Hugonnet et al., 2021), growing evidence has illustrated elevated expression of the Golgi β-Galactoside α-2,6-Sialyltransferase 1 (ST6Gal1) in various malignancies including, but not limited to, breast cancer (Lu et al., 2014), cervical cancer (Wang et al., 2003), ovarian cancer (Wichert et al., 2018), prostate cancer (Wei et al., 2016), pancreatic cancer (Schultz et al., 2016), colon cancer (Swindall and Bellis, 2011), gastric cancer (Gretschel et al., 2003), leukemia (Mondal et al., 2010; Zhang et al., 2022) hepatocellular carcinoma (Chen et al., 2000) and melanoma (Agrawal et al., 2017). This elevation of ST6Gal1 in cancers is often attributed to gene amplification (Dorsett et al., 2021). However, ST6Gal1 is regulated in neoplastic development through multiple mechanisms that include transcription factors (HNF1, Sox2, SP1) (Svensson et al., 1992; Taniguchi et al., 1998; Xu et al., 2003; Milflores-Flores et al., 2012; Dorsett et al., 2019), epigenetic factors (gene methylation, miR9, miR-213-3p. mir-200) (Schliekelman et al., 2011; Minami et al., 2013; Antony et al., 2014; Fleischer et al., 2014; Kroes and Moskal, 2016; Vojta et al., 2016; Han et al., 2018; Tao et al., 2019) as well as post-transcriptional and posttranslational modifications like cleavage by BACE1-β secretase (Chen et al., 2000; Kitazume et al., 2001; Lee et al., 2012; Isaji et al., 2014; Bhide and Colley, 2017; Welch and Munro, 2019; Krick et al., 2021).
ST6Gal1 adds sialic acid to the terminal galactose of N-glycoproteins in an α2,6 bond in the trans-Golgi (Figure 1). This α2,6 sialylation by ST6Gal1 is not only a prognostic marker for select cancers but also a driver of malignant progression (Munkley and Elliott, 2016). Mounting reports have implicated ST6Gal1 in eliciting tumorigenic processes like sustained proliferative signaling (Zhao et al., 2014; Wichert et al., 2018), evasion of growth suppressors (Garnham et al., 2019), resistance to cell death (Peter et al., 1995; Meesmann et al., 2010; Liu et al., 2011a; Suzuki et al., 2015), enabling replicative immortality (Garnham et al., 2019), activation of invasion (metastasis) (Wang et al., 2003; Lu et al., 2014; Zhao et al., 2014; Wei et al., 2016), promoting angiogenesis (Croci et al., 2014a; Meng et al., 2015), deregulating cellular energetics (Hsieh et al., 2017) and immune evasion (Hennet et al., 1998; Engdahl et al., 2018) (Figure 2). While there is much to be investigated about phenotypic effects of ST6Gal1 in cancers, even more information is needed on the ST6Gal1-mediated signals that lead to protumorigenic cellular behaviors. Nonetheless, some critical oncogenic pathways involving PI3K/AKT, Wnt/β-catenin and targets for ST6Gal1-mediated sialylation like Epidermal Growth Factor Receptor (EGFR), Platelet and Endothelial Cell Adhesion Molecule (PECAM), Tumor Necrosis Factor Receptor (TNFR), and Vascular Endothelial Growth Factor Receptor (VEGFR) have been identified (Figure 2; Table 1). The purpose of this review is to provide succinct insight into the currently elucidated major targets of, and molecular mechanisms mediated by, ST6Gal1 that contribute to cancer progression.
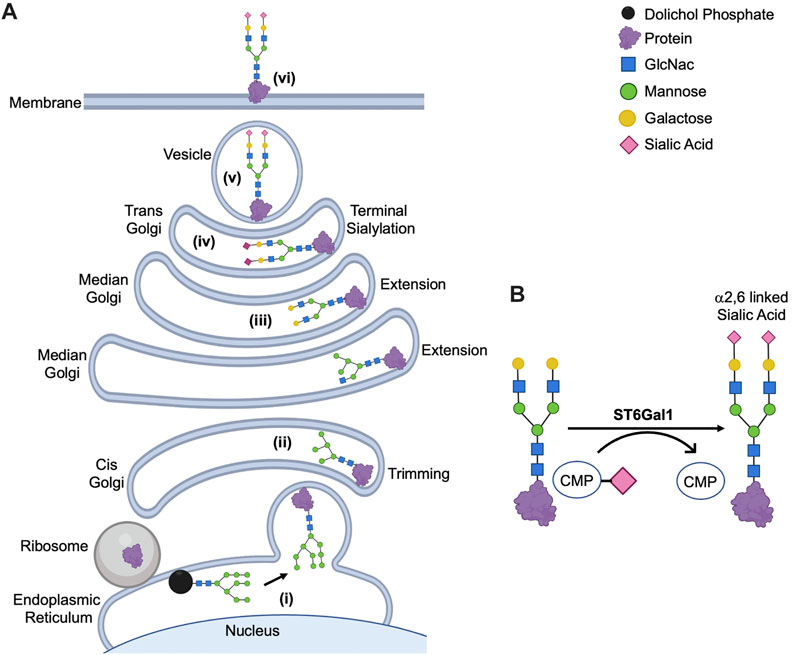
FIGURE 1. Posttranslational Modification of N-Glycoproteins in Golgi. (A) Mature glycan linked to dolichol phosphate is added to the protein synthesized in rough endoplasmic reticulum (i). The glycoproteins are modified via trimming in cis-Golgi (ii), extension in median Golgi (iii) and terminal sialylation by ST6Gal1 among other sialyltransferases in trans-Golgi (iv). The mature proteins are transported in vesicles (v) to the membrane (vi). (B) ST6Gal1 adds α2,6 linked terminal sialic acid to the N-linked glycoproteins using CMP-Sialic acid as a donor. The schematic diagram was created using Biorender (https://biorender.com).
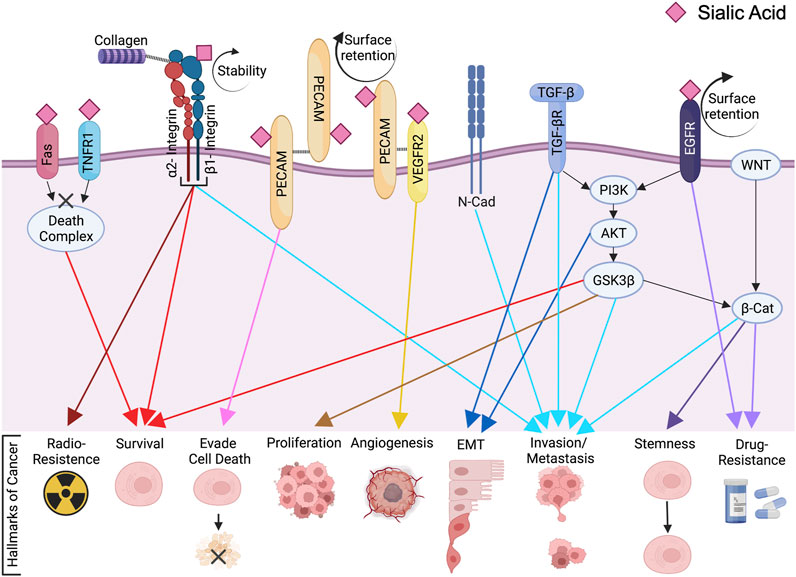
FIGURE 2. ST6Gal1-mediated Signaling Cascades in Cancer. The membrane bound N-glycoproteins synthesized and processed in secretory pathways (including Fas, TNFR1, β1-integrin, PECAM, VEGFR2 and EGFR among others) are α2,6 sialylated by ST6Gal1. This can affect protein cell surface retention, stability, clustering and/or activation. Through direct and indirect signaling pathways, ST6Gal1 promotes oncogenic characteristics in many cancers. The pink diamond represents α2,6 linked sialic acid, although the full glycan structure is not shown for simplification. The figure was created using Biorender (https://biorender.com).
ST6Gal1 and neoplastic pathways
RAS signaling
Activating mutations in the family of RAS proteins (KRAS, NRAS, and HRAS) have been well described to cause oncogenic transformation (Hobbs et al., 2016; Gimple and Wang, 2019). The RAS/RAF/MEK/ERK signaling cascade is highly implicated in oncogenic transcription leading to cell cycle progression, and the RAS/PI3K/AKT pathway induces malignant characteristics like cell survival, growth, and metabolic shifts (Gimple and Wang, 2019). More than 3 decades ago, ST6Gal1 was found to be elevated with overexpression of N-Ras in NIH 3T3 fibroblasts and c-HA-Ras in FR3T3 fibroblasts (Easton et al., 1991; Le Marer et al., 1992; Vandamme et al., 1992). Seales et al. (2003), later verified these findings in the HD3 colon epithelial cell model (Seales et al., 2003). Further, in 2004, Dalziel et al., reported that NIH3T3 expression of K-Ras or H-Ras led to a 10-fold increase in ST6Gal1, although there were no significant changes in the expression of other sialyltransferases (Dalziel et al., 2004). This study further determined that ST6Gal1 elevation during fibroblast transformation by mutant H-RasV12G37 principally occurred via the RalGEF signaling axis. Later, Seales et al. reported that during the differentiation of myeloid cells to monocytes/macrophages, ST6Gal1 expression was suppressed via the protein kinase C/Ras/ERK signaling cascade, indicating that the role of RAS in ST6Gal1 regulation is cell and context dependent (Seales et al., 2005a). Additional roles for downstream mediators of RAS signaling were demonstrated in melanoma, where BRAF mutations conferring constitutive activation are often present. Using 63 melanoma cell lines, ST6Gal1 was identified by Johansson et al. as a gene that was consistently upregulated with activating BRAF mutation (Johansson et al., 2007). Furthermore, in a Genetically Engineered Mouse Model (GEMM) of pancreatic cancer with RAS activation via expression of K-RasG12D under the control of p48Cre (Qian et al., 2009), the loss of ST6Gal1 resulted in normal acinar area and decreased fibrosis (Kurz et al., 2021). This led to delayed disease development and progression (Kurz et al., 2021). Corroborating these findings, in pancreatic organoids developed from mice expressing K-RasG12D under the control of Pdx1-Cre, knockdown of ST6Gal1 inhibited organoid growth (Chakraborty et al., 2022). Considering RAS mutations are observed in up to 93% of pancreatic cancer patients, the results of these studies strongly suggest ST6Gal1 as a downstream mediator of, and potential therapeutic target in, RAS-mediated pancreatic oncogenesis. These studies highlight the importance of ST6Gal1 in cancers with RAS activation, but the mechanisms through which RAS-mediated alterations in ST6Gal1 expression to promote malignant features still remain outstanding.
PI3K/AKT signaling
The phosphatidylinositol 3-kinase (PI3K)/protein kinase B (AKT) signaling cascade, a known RAS effector cascade, influences many biologies important in cancer, including cell cycle regulation, proliferation and apoptosis (Jiang et al., 2020). This pathway is activated in a multitude of cancers and, in turn, leads to uncontrolled cell growth, migration, invasion and therapeutic resistance (Castellano and Downward, 2011; Jiang et al., 2020). While the impact of sialylation and/or ST6Gal1 on the PI3K/AKT pathway remain to be fully explored, recent data suggest PI3K/AKT signaling can be activated via elevation of ST6Gal1. The overexpression of ST6Gal1 in the colon carcinoma cell line SW480 increased adhesion to fibronectin and led to cell survival via activation of AKT as measured by levels of phospho-AKT (Lee et al., 2010). In the same study, targeting ST6Gal1 with siRNA in SW480 cells increased sensitivity to radiation induced cell death through a mechanism associated with decreased phospho-AKT. When the colon cancer cell line HCT116 was treated with nano-diamino-tetrac (NDAT), an antiproliferative/angiogenic agent, the protein expression of ST6Gal1 along with phospho-PI3K was diminished (Chang et al., 2018). However, another study in HCT116 cells reported that knockdown of ST6Gal1 had no effect on expression of AKT or phospho-AKT, and there were no changes in cell adhesion or proliferation (Meezan et al., 1969; Qian et al., 2009). In this report, decreased expression of ST6Gal1 reduced motility through decreased c-Met/STAT3. This hints towards a more nuanced function of ST6Gal1-AKT axis in colon cancer even though a pattern of ST6Gal1-mediated increases in PI3K/AKT signaling was often observed.
ST6Gal1 was also associated with changes in PI3K/AKT signaling in hepatocellular carcinoma (HCC). The overexpression of ST6Gal1 in the less invasive HCC cell line MHCC97L, increased invasion along with expression of PI3K p110α (the catalytic subunit of PI3K) and phospho-AKT (Zhao et al., 2014). In the same study, converse experiments demonstrated that targeting ST6Gal1 in the metastatic HCC cell line MHCC97H decreased invasion as well as expression of PI3K p110α and phospho-AKT (Zhao et al., 2014). In a tissue microarray of HCC, ST6Gal1 expression correlated with worse prognosis and caveolin-1 expression. When caveolin-1 expression was silenced in MHCC97H cells, the expression of ST6Gal1, phospho-PI3K, phospho-AKT and phospho-mTOR also diminished simultaneously (Chen et al., 2021). Consistent with the previous studies, HepG2 HCC cells treated with exosomes isolated from MHCC97H cells had elevated phospho-AKT which promoted an invasive and proliferative phenotype (Wang et al., 2021). In these studies, phospho-GSK3β was also increased, and the PI3K/AKT/GSK3β signaling axis is established to promote cell survival (Romorini et al., 2016). In converse experiments using exosomes isolated from ST6Gal1 KD MHCC97H cells, the expressions of phospho-AKT and phospho-GSK3β were suppressed. In addition to ST6Gal1-mediated regulation of PI3K/AKT signaling, additional data suggests the potential for PI3K/AKT signaling to also regulate ST6Gal1. When the HCC line, Huh7, was treated with the AKT inhibitor MK2206 and the PI3K inhibitor LY294002, protein expression of ST6Gal1 was deceased. Overall, these data demonstrate the importance of ST6Gal1 in the regulation of PI3K/AKT signaling while also suggesting a possible feedback loop in HCC.
Beyond colon and liver cancers, ST6Gal1-mediated activation of the PI3K/AKT axis has also been reported in fibroblasts as well as other cancer types. For example, ST6Gal1 enhanced PI3K/AKT signaling in a monkey kidney fibroblast cell line, Cos7, to increase invasion and proliferation (Rao et al., 2022). This study linked the activation of PI3K/AKT signaling to ST6Gal1-mediated sialylation of EGFR (Rao et al., 2022). Considering the importance of amplifications of EGFR in cancer, this mechanism of ST6Gal1 action may also contribute to oncogenesis and/or tumor progression. In ovarian (Isaji et al., 2014) and breast cancer (Lu et al., 2014), ST6Gal1 mediated activation of the PI3K/AKT pathway was reported to promote invasion and EMT, respectively. Furthermore, in the prostate cancer cell lines, PC-3 and DU145, cell proliferation along with PI3K/ALK signaling was reduced when the ST6Gal1 gene was silenced (Wei et al., 2016). In gastric cancer, ST6Gal1 elevated phosphorylation of AKT in association with the promotion of resistance against trastuzumab (Liu et al., 2018). In this study, the activation of PI3K/AKT was due to ST6Gal1-mediated α2,6 sialylation of Human Epidermal Growth Factor Receptor 2 (HER2) (Liu et al., 2018), a breast cancer biomarker. In the BxPC3 pancreatic cancer line and the ovarian cancer cell line OV4, ST6Gal1 was also identified to provide protection against serum starvation via AKT signaling (Britain et al., 2017). As these data demonstrate, there are clear indications that PI3K/AKT signaling enrichment due to ST6Gal1 impacts a plethora of malignant phototypes like proliferation, growth, invasion and metastasis; however, the precise means by which these systemic changes are conveyed remain poorly understood with only a few receptors that increase AKT signaling identified as targets for ST6Gal1-mediated α2,6 sialylation.
WNT signaling
The Wnt pathway regulates development and stem cell maintenance through canonical (dependent on β-catenin stabilization and translocation into the nucleus for downstream signal transduction) and non-canonical signals (β-catenin independent) (Zhan et al., 2017; Wen et al., 2020). Among a handful of investigations on the impact of ST6Gal1 on WNT signaling, most have focused on the canonical pathway. Elevated Wnt signaling, including via increased expression of regulators like WNT3A and β-catenin, was identified in RNA-sequencing analysis of metastatic subclones of pancreatic cancer cell lines S2-LM7AA and S2-013 that expressed high levels of ST6Gal1 (Britain et al., 2021). This finding was recently corroborated with RNA-sequencing analysis of the pancreas from GEMM models with pancreas-specific ST6Gal1 overexpression (Chakraborty et al., 2022): these data showed elevated stem cell-related pathways including Wnt (Chakraborty et al., 2022). Furthermore, elevated levels of ST6Gal1 in the colorectal cancer cell lines Caco-2 and SW48 were associated with increased expression of WNT3a and β-catenin as well as fluorouracil (5-FU) resistance (Cui et al., 2018). As mentioned earlier, decreased expression of ST6Gal1 in prostate cancer models results in diminished activation of a PI3K/AKT/GSK-3β signaling axis, leading to diminished β-catenin (Wei et al., 2016). In addition to these data suggesting ST6Gal1-mediated regulation of WNT signaling, additional information indicates that Wnt regulates ST6Gal1 expression. In organoids derived from gastric cancer, ST6Gal1 expression depended upon stem cell maintenance factors with an important role for Wnt (Alexander et al., 2020). Modulation of cancer stem cell maintenance by the Wnt pathway is well recognized (Espada et al., 2009; Duchartre et al., 2017; Zhan et al., 2017; Patel et al., 2019), as is the promotion of stem cell maintenance by ST6Gal1 (Christie et al., 2008; Zhuo and Bellis, 2011; Schultz et al., 2013; Swindall et al., 2013; Wang et al., 2015; Chen et al., 2016; Schultz et al., 2016; Wei et al., 2016; Zhang et al., 2016; Britain et al., 2018; Chakraborty et al., 2018; Cui et al., 2018; Dorsett et al., 2019; Alexander et al., 2020; Verge et al., 2020) in myriad of neoplasms. Given the evidence of differential regulation of the Wnt pathway in ST6Gal1-modulated systems, and the importance of both Wnt and ST6Gal1 in cancer stem cell maintenance, it is imperative to understand the upstream and downstream mechanisms though which this signaling axis acts.
TGF-β signaling
Transforming Growth Factor-Beta (TGF-β) is a cytokine known to play a dual role in cancer; it often acts as a tumor suppressor in the earlier stages of disease due to its ability to inhibit cell proliferation while later promoting tumor progression in association with increased angiogenesis, EMT, immunosuppression and invasion (Bierie and Moses, 2006; Du et al., 2015; Hao et al., 2019). Therefore, reports of TGF-β signaling in relation to ST6Gal1 reflect these dynamic functions. For example, a study by Du et al. showed that TGF-β-induced EMT is regulated by sialylation, including that mediated by ST6Gal1, in HaCaT, MDCK and A549 cells. After a short 4-h treatment with TGF-β, ST6Gal1 and other sialyltransferases were downregulated, followed by gradual recovery of ST6Gal1 over time and upregulation at 24 h post treatment (Du et al., 2015). Additional studies confirmed that ST6Gal1 expression was elevated by TGF-β stimulation in other cell types, including the mouse epithelial cell line GE11 where transcriptional upregulation of ST6Gal1 involved SP1 (Lu et al., 2014). In this study that also included MDA-MB-231 breast cancer cells, knockdown of ST6Gal1 resulted in decreased EMT characteristics in association with PI3K/AKT signaling (rather than Smads) and preservation of E-cadherin (Lu et al., 2014). ST6Gal1 was also increased by TGF-β in THESCs endometrial fibroblasts, 12Z immortalized endometrial cells and Ishikawa endometrial cells (Choi et al., 2018). As early-stage endometriosis is defined by focal adhesion of endometrial tissue to the peritoneum, it was interesting to note that knockdown of ST6Gal1 in the Ishikawa endometrial adenocarcinoma cell line reduced TGF-β1-induced adhesion (Choi et al., 2018). While these data suggest an overall trend of TGF-β-mediated upregulation of ST6Gal1, it is important to recognize that not all cells have been reported to increase ST6Gal1 levels in response to TGF-β treatment: in HCV29 bladder epithelial cells, TGF-β decreased expression of ST6Gal1 (Guo et al., 2014). Whether this difference in TGF-β-mediated regulation of ST6Gal1 is cell type and/or time course dependent needs further investigation.
In addition to direct roles in neoplastic cells, ST6Gal1 and TGF-β signaling interactions can impact the tumor microenvironment. TGF-β1 is a well-established immunosuppressive cytokine (Yoshimura and Muto, 2011; Sanjabi et al., 2017; Batlle and Massague, 2019), and ST6Gal1 may enhance TGF-β1 secretion. In a study of HCC probing immune escape of cancer cells via modulation of T cell function (Wang et al., 2019), cells exhibiting upregulated ST6Gal1 were co-cultured with cytotoxic CD8+ T-cells resulting in increased secretion of TGF-β1 by the T-cells. These analyses indicate that, along with cell and context dependent functions in cancer EMT and metastasis, ST6Gal1-modulated expression of TGF-β has potential roles in immunosuppression.
Integrins, cadherins, Ig-CAMs, and selectins and roles in cell adhesion
In cancer, interactions between cells as well as cell to matrix interactions play critical roles in malignant processes such as stem cell maintenance, cell fate and differentiation, inflammatory response, angiogenesis, migration, EMT, cancer progression and metastasis (Cavallaro and Dejana, 2011; Windisch et al., 2019; Janiszewska et al., 2020). These functions are orchestrated by cell adhesion molecules spanning four families of 1) Integrins (α2β1, α5/β1, αL/β2); 2) Cadherins (E-cad, P-cad, N-cad); 3) Ig-CAMs (VCAM, NCAM, ICAM, Nectins, Necl); and 4) Selectins (E-selectin, P-selectin, L-selectin). Roles of ST6Gal1 in promoting EMT and metastasis via differential expression of several adhesion molecules have been established. Recently, an OMICS network analysis investigating adhesion proteins revealed that sialylation of adhesion molecules, specifically by ST6Gal1, plays a vital role in cancer cell EMT, migration and invasion (Bauer et al., 2020). Indeed, the importance of integrin sialylation by ST6Gal1 in imparting the aforementioned neoplastic characteristics is one of the most well described ST6Gal1-regulated pathways.
The β1 subunit of integrin combines with α2/α4/α5 subunits (among others) to form connections with the extracellular matrix that affect the survival, migration, and metastasis of cancer cells (Pan et al., 2018). There is ample evidence that underscores ST6Gal1-mediated expression and α2,6 sialylation of β1-integrin in mammary cancer (Hedlund et al., 2008; Lu et al., 2014), HCC (Han et al., 2018), gliomas (Kroes and Moskal, 2016), ovarian cancer (Christie et al., 2008; Choi et al., 2018), CRC (Seales et al., 2005b) and even in adipogenesis (Kaburagi et al., 2017). α2,6 sialylation of the β1 complex was also reported to affect binding to receptors: α5β1 to fibronectin (Pretzlaff et al., 2000; Semel et al., 2002; Seales et al., 2005a), α4β1 to VCAM-1 (Woodard-Grice et al., 2008), α3β1 to laminin (Pochec et al., 2003) and α1β1 (Seales et al., 2003) as well as α2β1 to collagen (Shaikh et al., 2008). In the colon adenocarcinoma cell line SW480, Lee et al. employed both overexpression and knockdown models to further establish that ST6Gal1 enhances the stability of β1-integrin (Lee et al., 2008). In the same cancer model, ST6Gal1 activated β1-integrin, further enhancing attachment to collagen and laminin: this resulted in heightened motility and highlighted the importance of ST6Gal1-regulated β1 integrin in disease progression and migration (Seales et al., 2005b; Chiricolo et al., 2006). A similar pro-migratory role was determined in HD3 colon epithelial cells in which Ras regulates ST6Gal1 expression and thereby addition of α2 sialic acid in β1, but not in β3 or β5, integrins: removal of α2 sialic acid from β1 integrin inhibited collagen binding and decreased migration and invasion (Seales et al., 2003; Shaikh et al., 2008). An additional report using SW480 cells confirmed overexpression of ST6Gal1 increased sialylation of β1 integrin and increased migration, while also suggesting β1 integrin-independent roles for soluble ST6Gal1 in migration (Lee et al., 2012). In a study with colon adenocarcinoma cells lacking α2,6 sialylation (SW48), ST6Gal1 expression was found to decrease adhesion to galectin-3 coated plates: interestingly, unsialylated β1 integrin bound to galectin-3 and promoted apoptosis, but ST6Gal1 expression and α2,6 sialylation of β1 integrin protected cells from galectin-3 induced apoptosis (Zhuo et al., 2008). ST6Gal1-mediated hypersialylation of β1 integrin in colon cancer cells also resulted in enhanced fibronectin binding that promoted survival via activation of a downstream cascade involving paxillin and AKT (Lee et al., 2010). Together, these data suggest sialylation of β1 integrin is critical for modulating extracellular matrix associations that mediate pro-tumorigenic colon cancer biologies including attachment, survival, and migration. Similar to these studies in colon cancer models, cell adhesion in the HCC cell line H22 via α5β1 integrin required α2,6 sialylation (Yu et al., 2013) and ST6Gal1-mediated hypersialylation of integrin β1 resulting in increased attachment to collagen I was determined in ovarian carcinoma (Christie et al., 2008). In the ovarian cancer models, the ST6Gal1/integrin-mediated signals imparted an invasive phenotype. While a pro-migratory and invasive phenotype of ST6Gal1 mediated by β1 sialylation is often determined, this is not always the case: ST6Gal1 knockdown increased metastasis as well as expression of integrin α3β1 in the metastatic CRC cell line SW620 (Jung et al., 2016).
In addition to roles in attachment and invasion, ST6Gal1/integrin-mediated signals have been associated with changes in cell fate impacting survival and differentiation. ST6Gal1 sialylation of β1 integrin in colon cancer also enhanced radio-resistance (Lee et al., 2008). Furthermore, in the ovarian cancer line OVCAR4, ST6Gal1-mediated α2,6 sialylation was essential for integrin α2-dependent cancer cell survival (Huang et al., 2021). In an in vivo model of mice mammary carcinoma, decreased cancer differentiation was observed with elevated ST6Gal1 and β1-integrin (Hedlund et al., 2008).
There are additional roles for ST6Gal1-mediated regulation of β1-integrin and cadherins in oncogenic transformation from epithelial (low oncogenic potential) to mesenchymal (high oncogenic potential) phenotypes. To indicate epithelial to mesenchymal transition (EMT), elevation of N-cadherin and downregulation E-cadherin are commonly used biomarkers. In the MG-63 osteosarcoma cell line, targeting ST6Gal1 decreased expression of N-cadherin, while increasing the expression of E-cadherin (Meng et al., 2015). As mentioned earlier, Lu et al. reported that during TGF-β-mediated EMT in breast cancer, expression of ST6Gal1 was elevated and, in turn, was inversely proportional to E-cadherin and directly proportional β1-Integrin levels (Lu et al., 2014). In a human lung adenocarcinoma model with cisplatin resistance, migratory capacity increased with ST6Gal1 and N-cadherin expression while E-cadherin expression was reduced (da Fonseca et al., 2022). A similar, elevated expression of N-cadherin with high ST6Gal1 was associated with invasive characteristics in pancreatic cancer (Britain et al., 2021) and HCC (Chen et al., 2021). In ovarian cancer, regulated by the P120 canonical pathway, adhesion of cancer cells to the peritoneal mesothelium stimulates ST6Gal1 expression facilitating hypersialylation of β1-integrin and elevation of P-cadherin (Britain et al., 2021). A comparable investigation in ovarian cancer showed that elevation of ST6Gal1 led to P-cadherin enrichment that resulted in upregulation of β1-integrin and drove metastasis via p70 S6 kinase activity (Britain et al., 2021). Together, these data link ST6Gal1, integrins, and cadherin to EMT and invasion, with β1-integrin being a direct target for ST6Gal-mediated sialylation.
ST6Gal1 also modulates angiogenesis via regulation of integrins and PECAM. PECAM is an important endothelial adhesion molecule with roles in cell survival and signal mechanotransduction (Kitazume et al., 2010). PECAM is a direct substrate of ST6Gal1 in mouse endothelial cells and α2,6 sialylation is crucial for its cell surface retention, PECAM-PECAM interaction (clustering), downstream signaling and anti-apoptotic function (Kitazume et al., 2010; Kitazume et al., 2014; Lee et al., 2014). In node-negative breast cancer patients, higher ST6Gal1 expression was associated with high E-selectin expression and lower survival (Hebbar et al., 2003). In terms of classical functions in angiogenesis, VEGFR2 and integrin β3 are both α2,6 sialylated and interact with PECAM. When ST6Gal1 knockout mice were injected with lung carcinoma cells, there was reduced PECAM surface stabilization and the PECAM-VEGFR2 interaction was compromised: this led to apoptosis in endothelial cells and inhibition of tumor angiogenesis (Imamaki et al., 2018). Another essential cell adhesion molecule, ICAM, is known to be downregulated in metastatic CRC (Maeda et al., 2002; Tachimori et al., 2005). In CRC, ST6Gal1 expression is higher in tumor compared to normal tissue and interestingly, lower expression is observed in metastatic (stage III and IV) tumors compared to the non-metastatic ones (stage I and II) (Zhang et al., 2017). A study in CRC using SW480 and SW620 lines showed that stabilization of ICAM by ST6Gal1 led to decreased metastasis (Zhou et al., 2019).
Although, there is still much to be explored, there have been some reports on the effects of ST6Gal1 on adhesion molecules in the context of the immune system. In U937 and THP-1 myeloid cell lines during differentiation with phorbol 12-myristate 13-acetate, ST6Gal1 expression decreased and, consequently, β1 integrin was hyposialylated: there was enhanced binding to fibronectin (Semel et al., 2002) that was regulated by the PKC/Ras/ERK pathway (Seales et al., 2005a). Another investigation of cell junctions in monocytes revealed that treatment with the proinflammatory cytokine TNF-α resulted in a concomitant decrease of ST6Gal1 expression and VE-cadherin α2,6 sialylation (Deng et al., 2017). Vascular Cell Adhesion Molecule (VCAM) interacts with α4β1 integrin to mediate leukocyte adhesion. In flow conditions, a decrease in VCAM1-mediated adhesion was reported in association with reduced ST6Gal1. ST6Gal1 was also reported to remove α4β1-dependent VCAM1 binding in monocytes (Woodard-Grice et al., 2008). However, the immunomodulatory impacts of ST6Gal1 in general and in the context of cancer remain to be further explored: the importance of these studies will only increase with expanded use of immunotherapies.
As evident from these data, ST6Gal1 function is crucial for the stability, signal transduction and function of adhesion molecules. Even though, among the adhesion molecules, the relationship of ST6Gal1 to integrins has received the most attention, we are still far from understanding the entirety of the upstream regulators and downstream signaling cascades of ST6Gal1-integrin association. Even fewer studies have been conducted on cadherins, which are important in cell-to-cell adhesion, or on selectins, which are important for blood cell-to-endothelial cell adhesion. Only a handful of studies have focused on Ig-CAMs, including one pertaining to Necl in lung adenocarcinoma, where ST6Gal1 was identified as a target of mir-199a leading to reduction of Necl-2 sialylation (Minami et al., 2013). Thus, there are many aspects of ST6Gal1 and sialylation-mediated regulation of adhesion molecule signaling that remain to be fully elucidated.
TNF family of death receptors and cytokines
The Tumor Necrosis Factor (TNF) superfamily is a group of ligands and receptors that include death receptors like TNFR1, FAS (CD95/APO-1), DR3 (TRAMP, APO-3), DR4 (TRAIL-R1), DR5 (TRAIL-R2/APO-2/KILLER), and DR6. While ligand stimulation of TNFR1 by TNF often results in gene activation, stimulation of FAS by FasL, or DR4 and DR5 by TRAIL, often leads to apoptotic signaling cascades. The TNFR family of DRs have been implicated in neoplastic processes by impacting inflammation and tumor survival (Takeda et al., 2007; Walczak, 2013; Dostert et al., 2019). Contrary to its well established pro-apoptotic function, FAS also imparts cell survival and pro-proliferative characteristics in a multitude of models (O'Connell et al., 1998; Lamboley et al., 2002; Natoli et al., 1995; Landowski et al., 1997; Baldwin et al., 1999; Chen et al., 2012; Yuan et al., 2011; Nijkamp et al., 2010; Lai et al., 2010; Ametller et al., 2010; Peter et al., 2007). Interestingly, Lee et al., reported that while apoptosis induction was mediated by receptor internalization, Fas localized in plasma membrane imparted pro-survival signals (Lee et al., 2006).
Sialylation of Fas has been shown to mask its apoptotic function (Peter et al., 1995; Suzuki, et al, 2003; Keppler et al., 1999). More specifically, sialylation by ST6Gal1 of Fas hindered subsequent death complex formation and prevented internalization of Fas receptor, abrogating Fas-mediated apoptosis in colon cancer cells (Swindall and Bellis, 2011; Swindall et al., 2013). Similarly, ST6Gal1 is reported to directly α2,6 sialylate TNFR1 and block the TNFα-induced apoptotic pathway in macrophages (Liu et al., 2011a), rectal cancer (Smithson et al., 2022), and pancreatic and ovarian cancer (Holdbrooks et al., 2018). This phenotype of apoptosis inhibition was caused by prevention of TNFR1 internalization with α2,6 sialylation (Holdbrooks et al., 2018). Intriguingly, while TNFR1 sialylation by ST6Gal1 inhibited the apoptotic arm of this signaling cascade, TNF-induced signaling via NF-κB and AKT pathways was enhanced. Furthermore, these findings were corroborated in a gastric organoid model, where overexpression of ST6Gal1 led to increases in surface TNFR1 expression: this increase protected cells against apoptosis as a result of TNFR1 α2,6 sialylation, which led to reduced receptor internalization and degradation (Alexander et al., 2020). Studies in rectal cancer (Smithson et al., 2022) and pancreatic ductal adenocarcinoma models have also reported that ST6Gal1 mediates chemoresistance via evading apoptosis through a mechanism that potentially involves TNFR1 sialylation (Chakraborty et al., 2018). Thus, signaling of the TNF superfamily in cancer is often modulated by ST6Gal1-mediated sialylation to avoid cell death and thereby promote tumor growth.
EGFR
Epidermal Growth Factor Receptor (EGFR) is a receptor tyrosine kinase activated by EGF ligand that is highly implicated in neoplastic mechanisms including invasion, metastasis, therapy resistance and angiogenesis (Henson and Gibson, 2006; De Luca et al., 2008; Chong and Janne, 2013; Sasaki et al., 2013; Sigismund et al., 2018; Uribe et al., 2021). Liu et al., determined that sialylation of EGFR affects its dimerization and potentially downstream signaling (Liu et al., 2011b). Since then, ST6Gal1-mediated activation of EGFR has been identified to influence survival, proliferation, apoptosis evasion, chemotherapy resistance, invasion and metastasis in a host of cancers including colorectal cancer (Liu et al., 2011b; Chang et al., 2018; Rodrigues et al., 2021) ovarian cancer (Schultz et al., 2013; Britain et al., 2018; Rao et al., 2022), and pancreatic ductal adenocarcinoma (Chakraborty et al., 2018). Using the CRC line SW480, Park et al. reported that EGF-induced EGFR activation and downstream pro-growth and proliferation signaling was enhanced with ST6Gal1 expression. Further, ST6Gal1-mediated resistance to the EGFR kinase inhibitor, gefitinib, was confirmed: these data suggested a major impact of ST6Gal1 in EGFR regulation (Park et al., 2012). When gefitinib-resistant CRC was treated with the antiproliferative agent nano-diamino-tetrac (NDAT), proliferation was abrogated via inhibition of ST6Gal1 (Chang et al., 2018). These findings in drug resistant cells were augmented by a recent study concluding that EGFR is indeed α2,6 sialylated by ST6Gal1 in a glycosite specific fashion: this modification of EGFR was, in part, responsible for resistance to cetuximab induced cytotoxicity in CRC (Rodrigues et al., 2021). These reports were corroborated in an ovarian cancer model (OV4 and SKOV3 cell lines) which showed a direct correlation between EGFR activation and ST6Gal1 expression, further confirmed EGFR as substrate of ST6Gal1, and demonstrated that ST6Gal1-mediated sialylation of EGFR leads to gefitinib resistance (Britain et al., 2018). ST6Gal1-mediated EGFR regulation was recently shown to foster elevated integrin forces, inferring a role in migration (Rao et al., 2022). The same study revealed that elevated expression of ST6Gal1 led to sustained membrane retention of EGFR. In support of this notion, elevated expression of ST6Gal1 concurrently activated EGFR in the pancreatic cancer cell line Suit2, exhibiting higher invasion and elevated levels of mesenchymal markers (Britain et al., 2021). These findings emphasize the importance of ST6Gal1-mediated α2,6 sialylation of EGFR in its turnover, clustering, activation and downstream signaling to effectively dictate its impact in cancer phenotypes.
VEGF
The binding of Vascular Endothelial Growth Factor (VEGF) to its receptors, VEGFR1 and VEGFR2, regulates angiogenesis. In tumors, VEGF and its receptors are known to promote the tumor vasculature and, in turn, increase cancer growth (Carmeliet, 2005; Hicklin and Ellis, 2005; Ellis and Hicklin, 2008; Goel and Mercurio, 2013; Apte et al., 2019). A plethora of reports have established the importance of glycosylation in neoplastic angiogenesis: in particular, VEGFR2 sialylation was critical for angiogenesis mediated by VEGF (Lynch et al., 2012; Croci et al., 2014a; Croci et al., 2014b; Croci and Rabinovich, 2014; Chandler et al., 2017; Chiodelli et al., 2017; Cheng and Oon, 2018). As mentioned earlier, in a mouse Lewis lung carcinoma, while α2,6-sialylated PECAM interacted with VEGFR2, loss of ST6Gal1 inhibited the interaction: this inhibition resulted in apoptosis and prevention of angiogenesis (Imamaki et al., 2018). Similarly, in an osteosarcoma model, loss of ST6Gal1 led to decreased VEGF expression (Meng et al., 2015). Utilizing ST6Gal1 null mice inoculated with B16-F0 melanoma tumors, it was revealed that α2,6 sialylation was high in tumors sensitive to anti-VEGF monoclonal antibody treatment, and ST6Gal1 knockout also led to protection against anti-VEGF treatment (Croci et al., 2014a). The relationship of ST6Gal1 to VEGF was also determined in non-small cell lung cancer cells in which ST6Gal1 downregulation caused Notch1 pathway disruption and subsequently decreased protein expression of VEGF along with MMP-2, MMP-7 and MMP9: this resulted in reduced proliferation, migration and invasion (Sartakhti et al, 2017). One of the factors capable of upregulating VEGF expression, leading to increased angiogenesis, is Hypoxia Inducible factor 1 (HIF1) (de Palma et al., 2017; Zhang et al., 2018a; Zhang et al., 2018b). A recent study in ovarian and pancreatic cancer demonstrated that cells propagated in hypoxia exhibited an upregulation in ST6Gal1 (Jones et al., 2018). Furthermore, overexpression of ST6Gal1 also elevated accumulation of HIF1. As hypoxia is a major driver of VEGF mediated angiogenesis, this suggests a potential signaling axis of ST6Gal1/HIF1/VEGF that contributes to tumor growth via angiogenesis.
Discussion
For pathophysiology of cancer, the perturbations in post-translational modifications like glycosylation are as important as the changes in genetic or protein content. However, compared to other facets, our understanding of the mechanistic basis through which sialylation influences molecular cascades in neoplastic transformation is limited. How a particular sialyltransferase, like the pro-oncogenic protein ST6Gal1, impacts molecular signals to regulate pro-tumorigenic cellular behaviors also remains to be fully determined. While ST6Gal1 has received some attention recently, important questions still remain unresolved to distinguish ST6Gal1 as a cancer biomarker. These include:
1) What are the upstream regulators of ST6Gal1 in different cancers at different stages?
2) What are the direct targets and interactors of ST6Gal1?
3) What are the changes to α2,6 sialylated proteins (confirmation, clustering, turnover) elicited by ST6Gal1?
4) What kind of effect (activating, deactivating) results from α2,6 sialylation of specific proteins?
5) Can we fully elucidate downstream mechanisms and signaling cross talk mediated by ST6Gal1 to develop novel anti-cancer therapeutic strategies?
6) Can we fully understand the specific targets altered by ST6Gal1 that lead to particular hallmarks of cancer to develop novel biomarkers?
It is apparent that ST6Gal1 plays a central role in cancer pathobiology, and thus holds a great potential for anti-cancer therapeutics. Further elucidation of these mechanistic cellular cascades will assist in the process of developing effective therapeutic mechanisms and prognostic guidelines for cancer.
Author contributions
SG performed initial literature review and summations of the literature. AH and SB provided revisions. SG, SB, and AH approved the final version.
Funding
This work was financially supported by awards from the National Institutes of Health via R03 NS125506 to the Hjelmeland laboratory. This work was also supported by the O’Neal Invests Award from the O’Neal Comprehensive Cancer Center which is supported by National Institutes of Health P30 CA013148.
Conflict of interest
The authors declare that the research was conducted in the absence of any commercial or financial relationships that could be construed as a potential conflict of interest.
Publisher’s note
All claims expressed in this article are solely those of the authors and do not necessarily represent those of their affiliated organizations, or those of the publisher, the editors and the reviewers. Any product that may be evaluated in this article, or claim that may be made by its manufacturer, is not guaranteed or endorsed by the publisher.
References
Agrawal, P., Fontanals-Cirera, B., Sokolova, E., Jacob, S., Vaiana, C. A., Argibay, D., et al. (2017). A systems biology approach identifies FUT8 as a driver of melanoma metastasis. Cancer Cell 31, 804–819. doi:10.1016/j.ccell.2017.05.007
Alexander, K. L., Serrano, C. A., Chakraborty, A., Nearing, M., Council, L. N., Riquelme, A., et al. (2020). Modulation of glycosyltransferase ST6Gal-I in gastric cancer-derived organoids disrupts homeostatic epithelial cell turnover. J. Biol. Chem. 295, 14153–14163. doi:10.1074/jbc.RA120.014887
Ametller, E., Garcia-Recio, S., Costamagna, D., Mayordomo, C., Fernandez-Nogueira, P., Carbo, N., et al. (2010). Tumor promoting effects of CD95 signaling in chemoresistant cells. Mol. Cancer 9, 161. doi:10.1186/1476-4598-9-161
Antony, P., Rose, M., Heidenreich, A., Knuchel, R., Gaisa, N. T., Dahl, E., et al. (2014). Epigenetic inactivation of ST6GAL1 in human bladder cancer. Bmc Cancer 14, 901. doi:10.1186/1471-2407-14-901
Apte, R. S., Chen, D. S., and Ferrara, N. (2019). VEGF in signaling and disease: Beyond discovery and development. Cell 176, 1248–1264. doi:10.1016/j.cell.2019.01.021
Baldwin, R. L., Tran, H., and Karlan, B. Y. (1999). Primary ovarian cancer cultures are resistant to Fas-mediated apoptosis. Gynecol. Oncol. 74, 265–271. doi:10.1006/gyno.1999.5448
Batlle, E., and Massague, J. (2019). Transforming growth factor-beta signaling in immunity and cancer. Immunity 50, 924–940. doi:10.1016/j.immuni.2019.03.024
Bauer, T. J., Gombocz, E., Wehland, M., Bauer, J., Infanger, M., Grimm, D., et al. (2020). Insight in adhesion protein sialylation and microgravity dependent cell adhesion-an omics network approach. Int. J. Mol. Sci. 21, E1749. doi:10.3390/ijms21051749
Bhide, G. P., and Colley, K. J. (2017). Sialylation of N-glycans: Mechanism, cellular compartmentalization and function. Histochem. Cell Biol. 147, 149–174. doi:10.1007/s00418-016-1520-x
Bierie, B., and Moses, H. L. (2006). TGF-beta and cancer. Cytokine Growth Factor Rev. 17, 29–40. doi:10.1016/j.cytogfr.2005.09.006
Britain, C. M., Bhalerao, N., Silva, A. D., Chakraborty, A., Buchsbaum, D. J., Crowley, M. R., et al. (2021). Glycosyltransferase ST6Gal-I promotes the epithelial to mesenchymal transition in pancreatic cancer cells. J. Biol. Chem. 296, 100034. doi:10.1074/jbc.RA120.014126
Britain, C. M., Dorsett, K. A., and Bellis, S. L. (2017). The glycosyltransferase ST6Gal-I protects tumor cells against serum growth factor withdrawal by enhancing survival signaling and proliferative potential. J. Biol. Chem. 292, 4663–4673. doi:10.1074/jbc.M116.763862
Britain, C. M., Holdbrooks, A. T., Anderson, J. C., Willey, C. D., and Bellis, S. L. (2018). Sialylation of EGFR by the ST6Gal-I sialyltransferase promotes EGFR activation and resistance to gefitinib-mediated cell death. J. Ovarian Res. 11, 12. doi:10.1186/s13048-018-0385-0
Bull, C., Stoel, M. A., den Brok, M. H., and Adema, G. J. (2014). Sialic acids sweeten a tumor's life. Cancer Res. 74, 3199–3204. doi:10.1158/0008-5472.CAN-14-0728
Carmeliet, P. (2005). VEGF as a key mediator of angiogenesis in cancer. Oncology 69, 4–10. doi:10.1159/000088478
Castellano, E., and Downward, J. (2011). RAS interaction with PI3K: More than just another effector pathway. Genes Cancer 2, 261–274. doi:10.1177/1947601911408079
Cavallaro, U., and Dejana, E. (2011). Adhesion molecule signalling: Not always a sticky business. Nat. Rev. Mol. Cell Biol. 12, 189–197. doi:10.1038/nrm3068
Chakraborty, A., Bhalerao, N., Marciel, M., Hwang, J., Britain, C. M., Eltoum, I. E., et al. (2022). ST6GAL1 sialyltransferase promotes acinar to ductal metaplasia and pancreatic cancer progression. bioRxiv.
Chakraborty, A., Dorsett, K. A., Trummell, H. Q., Yang, E. S., Oliver, P. G., Bonner, J. A., et al. (2018). ST6Gal-I sialyltransferase promotes chemoresistance in pancreatic ductal adenocarcinoma by abrogating gemcitabine-mediated DNA damage. J. Biol. Chem. 293, 984–994. doi:10.1074/jbc.M117.808584
Chandler, K. B., Leon, D. R., Meyer, R. D., Rahimi, N., and Costello, C. E. (2017). Site-specific N-glycosylation of endothelial cell receptor tyrosine kinase VEGFR-2. J. Proteome Res. 16, 677–688. doi:10.1021/acs.jproteome.6b00738
Chang, T. C., Chin, Y. T., Nana, A. W., Wang, S. H., Liao, Y. M., Chen, Y. R., et al. (2018). Enhancement by nano-diamino-tetrac of antiproliferative action of gefitinib on colorectal cancer cells: Mediation by EGFR sialylation and PI3K activation. Horm. Cancer 9, 420–432. doi:10.1007/s12672-018-0341-x
Chen, C., Ma, J. Y., Lazic, A., Backovic, M., and Colley, K. J. (2000). Formation of insoluble oligomers correlates with ST6Gal I stable localization in the Golgi. J. Biol. Chem. 275, 13819–13826. doi:10.1074/jbc.275.18.13819
Chen, L. N., Park, S. M., Tumanov, A. V., Hau, A., Sawada, K., Feig, C., et al. (2012). CD95 promotes tumour growth (vol 465, pg 492, 2010). Nature, 491.
Chen, X., Wang, L., Yu, X., Wang, S., and Zhang, J. (2021). Caveolin-1 facilitates cell migration by upregulating nuclear receptor 4A2/retinoid X receptor α-mediated β-galactoside α2, 6-sialyltransferase I expression in human hepatocarcinoma cells. Int. J. Biochem. Cell Biol. 137, 106027. doi:10.1016/j.biocel.2021.106027
Chen, X. X., Wang, L. P., Zhao, Y. J., Yuan, S. Q., Wu, Q., Zhu, X. L., et al. (2016). ST6Gal-I modulates docetaxel sensitivity in human hepatocarcinoma cells via the p38 MAPK/caspase pathway. Oncotarget 7, 51955–51964. doi:10.18632/oncotarget.10192
Cheng, W. K., and Oon, C. E. (2018). How glycosylation aids tumor angiogenesis: An updated review. Biomed. Pharmacother. 103, 1246–1252. doi:10.1016/j.biopha.2018.04.119
Chiodelli, P., Rezzola, S., Urbinati, C., Signori, F. F., Monti, E., Ronca, R., et al. (2017). Contribution of vascular endothelial growth factor receptor-2 sialylation to the process of angiogenesis. Oncogene 36, 6531–6541. doi:10.1038/onc.2017.243
Chiricolo, M., Malagolini, N., Bonfiglioli, S., and Dall'Olio, F. (2006). Phenotypic changes induced by expression of beta-galactoside alpha2, 6 sialyltransferase I in the human colon cancer cell line SW948. Glycobiology 16, 146–154. doi:10.1093/glycob/cwj045
Choi, H. J., Chung, T. W., Choi, H. J., Han, J. H., Choi, J. H., Kim, C. H., et al. (2018). Increased α2-6 sialylation of endometrial cells contributes to the development of endometriosis. Exp. Mol. Med. 50, 1–12. doi:10.1038/s12276-018-0167-1
Chong, C. R., and Janne, P. A. (2013). The quest to overcome resistance to EGFR-targeted therapies in cancer. Nat. Med. 19, 1389–1400. doi:10.1038/nm.3388
Christie, D. R., Shaikh, F. M., Lucas, J. A., Lucas, J. A., and Bellis, S. L. (2008). ST6Gal-I expression in ovarian cancer cells promotes an invasive phenotype by altering integrin glycosylation and function. J. Ovarian Res. 1, 3. doi:10.1186/1757-2215-1-3
Croci, D. O., Cerliani, J. P., Dalotto-Moreno, T., Mendez-Huergo, S. P., Mascanfroni, I. D., Dergan-Dylon, S., et al. (2014). Glycosylation-dependent lectin-receptor interactions preserve angiogenesis in anti-VEGF refractory tumors. Cell 156, 744–758. doi:10.1016/j.cell.2014.01.043
Croci, D. O., Cerliani, J. P., Pinto, N. A., Morosi, L. G., and Rabinovich, G. A. (2014). Regulatory role of glycans in the control of hypoxia-driven angiogenesis and sensitivity to anti-angiogenic treatment. Glycobiology 24, 1283–1290. doi:10.1093/glycob/cwu083
Croci, D. O., and Rabinovich, G. A. (2014). Linking tumor hypoxia with VEGFR2 signaling and compensatory angiogenesis Glycans make the difference. Oncoimmunology 3, e29380. doi:10.4161/onci.29380
Cui, H., Yang, S., Jiang, Y., Li, C., Zhao, Y., Shi, Y., et al. (2018). The glycosyltransferase ST6Gal-I is enriched in cancer stem-like cells in colorectal carcinoma and contributes to their chemo-resistance. Clin. Transl. Oncol. 20, 1175–1184. doi:10.1007/s12094-018-1840-5
da Fonseca, L. M., da Silva, V. A., da Costa, K. M., Dos Reis, J. S., Previato, J. O., Previato, L. M., et al. (2022). Resistance to cisplatin in human lung adenocarcinoma cells: Effects on the glycophenotype and epithelial to mesenchymal transition markers. Glycoconj. J. 39, 247–259. doi:10.1007/s10719-022-10042-2
Dalziel, M., Dall'Olio, F., Mungul, A., Piller, V., and Piller, F. (2004). Ras oncogene induces beta-galactoside alpha2, 6-sialyltransferase (ST6Gal I) via a RalGEF-mediated signal to its housekeeping promoter. Eur. J. Biochem. 271, 3623–3634. doi:10.1111/j.1432-1033.2004.04284.x
De Luca, A., Carotenuto, A., Rachiglio, A., Gallo, M., Maiello, M. R., Aldinucci, D., et al. (2008). The role of the EGFR signaling in tumor microenvironment. J. Cell. Physiol. 214, 559–567. doi:10.1002/jcp.21260
de Palma, M., Biziato, D., and Petrova, T. V. (2017). Microenvironmental regulation of tumour angiogenesis. Nat. Rev. Cancer 17, 457–474. doi:10.1038/nrc.2017.51
Deng, X., Zhang, J., Liu, Y., Chen, L. M., and Yu, C. (2017). TNF-alpha regulates the proteolytic degradation of ST6Gal-1 and endothelial cell-cell junctions through upregulating expression of BACE1. Sci. Rep. 7, 40256. doi:10.1038/srep40256
Dorsett, K. A., Jones, R. B., Ankenbauer, K. E., Hjelmeland, A. B., and Bellis, S. L. (2019). Sox2 promotes expression of the ST6Gal-I glycosyltransferase in ovarian cancer cells. J. Ovarian Res. 12, 93. doi:10.1186/s13048-019-0574-5
Dorsett, K. A., Marciel, M. P., Hwang, J., Ankenbauer, K. E., Bhalerao, N., Bellis, S. L., et al. (2021). Regulation of ST6GAL1 sialyltransferase expression in cancer cells. Glycobiology 31, 530–539. doi:10.1093/glycob/cwaa110
Dostert, C., Grusdat, M., Letellier, E., and Brenner, D. (2019). The TNF family of ligands and receptors: Communication modules in the immune system and beyond. Physiol. Rev. 99, 115–160. doi:10.1152/physrev.00045.2017
Du, J., Hong, S., Dong, L., Cheng, B., Lin, L., Zhao, B., et al. (2015). Dynamic sialylation in transforming growth factor-beta (TGF-beta)-induced epithelial to mesenchymal transition. J. Biol. Chem. 290, 12000–12013. doi:10.1074/jbc.M115.636969
Duchartre, Y., Kim, Y. M., and Kahn, M. (2017). Pharmacologic manipulation of Wnt signaling and cancer stem cells. Methods Mol. Biol. 1613, 463–478. doi:10.1007/978-1-4939-7027-8_18
Easton, E. W., Bolscher, J. G., and van den Eijnden, D. H. (1991). Enzymatic amplification involving glycosyltransferases forms the basis for the increased size of asparagine-linked glycans at the surface of NIH 3T3 cells expressing the N-ras proto-oncogene. J. Biol. Chem. 266, 21674–21680. doi:10.1016/s0021-9258(18)54689-5
Ellis, L. M., and Hicklin, D. J. (2008). VEGF-Targeted therapy: Mechanisms of anti-tumour activity. Nat. Rev. Cancer 8, 579–591. doi:10.1038/nrc2403
Engdahl, C., Bondt, A., Harre, U., Raufer, J., Pfeifle, R., Camponeschi, A., et al. (2018). Estrogen induces St6gal1 expression and increases IgG sialylation in mice and patients with rheumatoid arthritis: A potential explanation for the increased risk of rheumatoid arthritis in postmenopausal women. Arthritis Res. Ther. 20, 84. doi:10.1186/s13075-018-1586-z
Espada, J., Calvo, M. B., Diaz-Prado, S., and Medina, V. (2009). Wnt signalling and cancer stem cells. Clin. Transl. Oncol. 11, 411–427. doi:10.1007/s12094-009-0380-4
Fleischer, T., Edvardsen, H., Solvang, H. K., Daviaud, C., Naume, B., Borresen-Dale, A. L., et al. (2014). Integrated analysis of high- resolution DNA methylation profiles, gene expression, germline genotypes and clinical end points in breast cancer patients. Int. J. Cancer 134, 2615–2625. doi:10.1002/ijc.28606
Garnham, R., Scott, E., Livermore, K. E., and Munkley, J. (2019). ST6GAL1: A key player in cancer. Oncol. Lett. 18, 983–989. doi:10.3892/ol.2019.10458
Gilgunn, S., Conroy, P. J., Saldova, R., Rudd, P. M., and O'Kennedy, R. J. (2013). Aberrant PSA glycosylation--a sweet predictor of prostate cancer. Nat. Rev. Urol. 10, 99–107. doi:10.1038/nrurol.2012.258
Gimple, R. C., and Wang, X. X. (2019). Ras: Striking at the core of the oncogenic circuitry. Front. Oncol. 9, 965. doi:10.3389/fonc.2019.00965
Goel, H. L., and Mercurio, A. M. (2013). VEGF targets the tumour cell. Nat. Rev. Cancer 13, 871–882. doi:10.1038/nrc3627
Gretschel, S., Haensch, W., Schlag, P. M., and Kemmner, W. (2003). Clinical relevance of sialyltransferases ST6GAL-I and ST3GAL-III in gastric cancer. Oncology 65, 139–145. doi:10.1159/000072339
Guo, J., Li, X., Tan, Z. Q., Lu, W., Yang, G. L., Guan, F., et al. (2014). Alteration of N-glycans and expression of their related glycogenes in the epithelial-mesenchymal transition of HCV29 bladder epithelial cells. Molecules 19, 20073–20090. doi:10.3390/molecules191220073
Han, Y., Liu, Y. B., Fu, X. R., Zhang, Q., Huang, H., Zhang, C., et al. (2018). miR-9 inhibits the metastatic ability of hepatocellular carcinoma via targeting beta galactoside alpha-2, 6-sialyltransferase 1. J. Physiol. Biochem. 74, 491–501. doi:10.1007/s13105-018-0642-0
Hao, Y., Baker, D., and Ten Dijke, P. (2019). TGF-beta-Mediated epithelial-mesenchymal transition and cancer metastasis. Int. J. Mol. Sci. 20, E2767. doi:10.3390/ijms20112767
Harduin-Lepers, A., Vallejo-Ruiz, V., Krzewinski-Recchi, M. A., Samyn-Petit, B., Julien, S., and Delannoy, P. (2001). The human sialyltransferase family. Biochimie 83, 727–737. doi:10.1016/s0300-9084(01)01301-3
Hebbar, M., Krzewinski-Recchi, M. A., Hornez, L., Verdiere, A., Harduin-Lepers, A., Bonneterre, J., et al. (2003). Prognostic value of tumoral sialyltransferase expression and circulating E-selectin concentrations in node-negative breast cancer patients. Int. J. Biol. Markers 18, 116–122. doi:10.5301/jbm.2008.3241
Hedlund, M., Ng, E., Varki, A., and Varki, N. M. (2008). Alpha 2-6-linked sialic acids on N-glycans modulate carcinoma differentiation in vivo. Cancer Res. 68, 388–394. doi:10.1158/0008-5472.CAN-07-1340
Hennet, T., Chui, D., Paulson, J. C., and Marth, J. D. (1998). Immune regulation by the ST6Gal sialyltransferase. Proc. Natl. Acad. Sci. U. S. A. 95, 4504–4509. doi:10.1073/pnas.95.8.4504
Henson, E. S., and Gibson, S. B. (2006). Surviving cell death through epidermal growth factor (EGF) signal transduction pathways: Implications for cancer therapy. Cell. Signal. 18, 2089–2097. doi:10.1016/j.cellsig.2006.05.015
Hicklin, D. J., and Ellis, L. M. (2005). Role of the vascular endothelial growth factor pathway in tumor growth and angiogenesis. J. Clin. Oncol. 23, 1011–1027. doi:10.1200/JCO.2005.06.081
Hobbs, G. A., Der, C. J., and Rossman, K. L. (2016). RAS isoforms and mutations in cancer at a glance. J. Cell Sci. 129, 1287–1292. doi:10.1242/jcs.182873
Holdbrooks, A. T., Britain, C. M., and Bellis, S. L. (2018). ST6Gal-I sialyltransferase promotes tumor necrosis factor (TNF)-mediated cancer cell survival via sialylation of the TNF receptor 1 (TNFR1) death receptor. J. Biol. Chem. 293, 1610–1622. doi:10.1074/jbc.M117.801480
Hsieh, C. C., Shyr, Y. M., Liao, W. Y., Chen, T. H., Wang, S. E., Lu, P. C., et al. (2017). Elevation of β-galactoside α2, 6-sialyltransferase 1 in a fructoseresponsive manner promotes pancreatic cancer metastasis. Oncotarget 8, 7691–7709. doi:10.18632/oncotarget.13845
Huang, Y. L., Liang, C. Y., Labitzky, V., Ritz, D., Oliveira, T., Cumin, C., et al. (2021). Site-specific N-glycosylation of integrin α2 mediates collagen-dependent cell survival. iScience 24, 103168. doi:10.1016/j.isci.2021.103168
Hugonnet, M., Singh, P., Haas, Q., and von Gunten, S. (2021). The distinct roles of sialyltransferases in cancer biology and onco-immunology. Front. Immunol. 12, 799861. doi:10.3389/fimmu.2021.799861
Imamaki, R., Ogawa, K., Kizuka, Y., Komi, Y., Kojima, S., Kotani, N., et al. (2018). Glycosylation controls cooperative PECAM-VEGFR2-beta 3 integrin functions at the endothelial surface for tumor angiogenesis. Oncogene 37, 4287–4299. doi:10.1038/s41388-018-0271-7
Isaji, T., Im, S., Gu, W., Wang, Y. Q., Hang, Q. L., Lu, J. S., et al. (2014). An oncogenic protein Golgi phosphoprotein 3 up-regulates cell migration via sialylation. J. Biol. Chem. 289, 20694–20705. doi:10.1074/jbc.M113.542688
Janiszewska, M., Primi, M. C., and Izard, T. (2020). Cell adhesion in cancer: Beyond the migration of single cells. J. Biol. Chem. 295, 2495–2505. doi:10.1074/jbc.REV119.007759
Jiang, N., Dai, Q., Su, X., Fu, J., Feng, X., Peng, J., et al. (2020). Role of PI3K/AKT pathway in cancer: The framework of malignant behavior. Mol. Biol. Rep. 47, 4587–4629. doi:10.1007/s11033-020-05435-1
Johansson, P., Pavey, S., and Hayward, N. (2007). Confirmation of a BRAF mutation-associated gene expression signature in melanoma. Pigment. Cell Res. 20, 216–221. doi:10.1111/j.1600-0749.2007.00375.x
Jones, R. B., Dorsett, K. A., Hjelmeland, A. B., and Bellis, S. L. (2018). The ST6Gal-I sialyltransferase protects tumor cells against hypoxia by enhancing HIF-1α signaling. J. Biol. Chem. 293, 5659–5667. doi:10.1074/jbc.RA117.001194
Jung, Y. R., Park, J. J., Jin, Y. B., Cao, Y. J., Park, M. J., Kim, E. J., et al. (2016). Silencing of ST6Gal I enhances colorectal cancer metastasis by down-regulating KAI1 via exosome-mediated exportation and thereby rescues integrin signaling. Carcinogenesis 37, 1089–1097. doi:10.1093/carcin/bgw091
Kaburagi, T., Kizuka, Y., Kitazume, S., and Taniguchi, N. (2017). The inhibitory role of α2, 6-sialylation in adipogenesis. J. Biol. Chem. 292, 2278–2286. doi:10.1074/jbc.M116.747667
Keppler, O. T., Peter, M. E., Hinderlich, S., Moldenhauer, G., Stehling, P., Schmitz, I., et al. (1999). Differential sialylation of cell surface glycoconjugates in a human B lymphoma cell line regulates susceptibility for CD95 (APO-1/Fas)-mediated apoptosis and for infection by a lymphotropic virus. Glycobiology 9, 557–569. doi:10.1093/glycob/9.6.557
Kitazume, S., Imamaki, R., Kurimoto, A., Ogawa, K., Kato, M., Yamaguchi, Y., et al. (2014). Interaction of platelet endothelial cell adhesion molecule (PECAM) with alpha 2, 6-sialylated glycan regulates its cell surface residency and anti-apoptotic role. J. Biol. Chem. 289, 27604–27613. doi:10.1074/jbc.M114.563585
Kitazume, S., Imamaki, R., Ogawa, K., Komi, Y., Futakawa, S., Kojima, S., et al. (2010). Alpha 2, 6-sialic acid on platelet endothelial cell adhesion molecule (PECAM) regulates its homophilic interactions and downstream antiapoptotic signaling. J. Biol. Chem. 285, 6515–6521. doi:10.1074/jbc.M109.073106
Kitazume, S., Tachida, Y., Oka, R., Shirotani, K., Saido, T. C., Hashimoto, Y., et al. (2001). Alzheimer's beta-secretase, beta-site amyloid precursor protein-cleaving enzyme, is responsible for cleavage secretion of a Golgi-resident sialyltransferase. Proc. Natl. Acad. Sci. U. S. A. 98, 13554–13559. doi:10.1073/pnas.241509198
Krick, S., Helton, E. S., Easter, M., Bollenbecker, S., Denson, R., Zaharias, R., et al. (2021). ST6GAL1 and alpha 2-6 sialylation regulates IL-6 expression and secretion in chronic obstructive pulmonary disease. Front. Immunol. 12, 693149. doi:10.3389/fimmu.2021.693149
Kroes, R. A., and Moskal, J. R. (2016). The role of DNA methylation in ST6Gal1 expression in gliomas. Glycobiology 26, 1271–1283. doi:10.1093/glycob/cww058
Kurz, E., Chen, S., Vucic, E., Baptiste, G., Loomis, C., Agrawal, P., et al. (2021). Integrated systems analysis of the murine and human pancreatic cancer glycomes reveals a tumor-promoting role for ST6GAL1. Mol. Cell. Proteomics. 20, 100160. doi:10.1016/j.mcpro.2021.100160
Lai, Y. J., Lin, V. T. G., Zheng, Y., Benveniste, E. N., and Lin, F. T. (2010). The adaptor protein TRIP6 antagonizes fas-induced apoptosis but promotes its effect on cell migration. Mol. Cell. Biol. 30, 5582–5596. doi:10.1128/MCB.00134-10
Lamboley, C., Bringuier, A. F., Camus, E., Lardeux, B., Groyer, A., Feldmann, G., et al. (2002). Overexpression of the mouse Fas gene in human Hep3B hepatoma cells overcomes their resistance to Fas-mediated apoptosis. J. Hepatol. 36, 385–394. doi:10.1016/s0168-8278(01)00284-7
Landowski, T. H., GleasonGuzman, M. C., and Dalton, W. S. (1997). Selection for drug resistance results in resistance to Fas-mediated apoptosis. Blood 89, 1854–1861. doi:10.1182/blood.v89.6.1854
Le Marer, N., Laudet, V., Svensson, E. C., Cazlaris, H., Van Hille, B., Lagrou, C., et al. (1992). The c-Ha-ras oncogene induces increased expression of beta-galactoside alpha-2, 6-sialyltransferase in rat fibroblast (FR3T3) cells. Glycobiology 2, 49–56. doi:10.1093/glycob/2.1.49
Lee, C., Liu, A., Miranda-Ribera, A., Hyun, S. W., Lillehoj, E. P., Cross, A. S., et al. (2014). NEU1 sialidase regulates the sialylation state of CD31 and disrupts CD31-driven capillary-like tube formation in human lung microvascular endothelia. J. Biol. Chem. 289, 9121–9135. doi:10.1074/jbc.M114.555888
Lee, K. H., Feig, C., Tchikov, V., Schickel, R., Hallas, C., Schutze, S., et al. (2006). The role of receptor internalization in CD95 signaling. Embo J. 25, 1009–1023. doi:10.1038/sj.emboj.7601016
Lee, M., Lee, H. J., Bae, S., and Lee, Y. S. (2008). Protein sialylation by sialyltransferase involves radiation resistance. Mol. Cancer Res. 6, 1316–1325. doi:10.1158/1541-7786.MCR-07-2209
Lee, M., Park, J. J., Ko, Y. G., and Lee, Y. S. (2012). Cleavage of ST6Gal I by radiation-induced BACE1 inhibits golgi-anchored ST6Gal I-mediated sialylation of integrin beta 1 and migration in colon cancer cells. Radiat. Oncol. 7, 47. doi:10.1186/1748-717X-7-47
Lee, M., Park, J. J., and Lee, Y. S. (2010). Adhesion of ST6Gal I-mediated human colon cancer cells to fibronectin contributes to cell survival by integrin beta1-mediated paxillin and AKT activation. Oncol. Rep. 23, 757–761.
Li, F. J., and Ding, J. J. (2019). Sialylation is involved in cell fate decision during development, reprogramming and cancer progression. Protein Cell 10, 550–565. doi:10.1007/s13238-018-0597-5
Liu, N., Zhu, M., Linhai, Y., Song, Y., Gui, X., Tan, G., et al. (2018). Increasing HER2 α2, 6 sialylation facilitates gastric cancer progression and resistance via the Akt and ERK pathways. Oncol. Rep. 40, 2997–3005. doi:10.3892/or.2018.6680
Liu, Y. C., Yen, H. Y., Chen, C. Y., Chen, C. H., Cheng, P. F., Juan, Y. H., et al. (2011). Sialylation and fucosylation of epidermal growth factor receptor suppress its dimerization and activation in lung cancer cells. Proc. Natl. Acad. Sci. U. S. A. 108, 11332–11337. doi:10.1073/pnas.1107385108
Liu, Z., Swindall, A. F., Kesterson, R. A., Schoeb, T. R., Bullard, D. C., Bellis, S. L., et al. (2011). ST6Gal-I regulates macrophage apoptosis via α2-6 sialylation of the TNFR1 death receptor. J. Biol. Chem. 286, 39654–39662. doi:10.1074/jbc.M111.276063
Lu, J., Isaji, T., Im, S., Fukuda, T., Hashii, N., Takakura, D., et al. (2014). β-Galactoside α2, 6-sialyltranferase 1 promotes transforming growth factor-β-mediated epithelial-mesenchymal transition. J. Biol. Chem. 289, 34627–34641. doi:10.1074/jbc.M114.593392
Lynch, T. P., Ferrer, C. M., Jackson, S. R., Shahriari, K. S., Vosseller, K., Reginato, M. J., et al. (2012). Critical role of O-linked beta-N-acetylglucosamine transferase in prostate cancer invasion, angiogenesis, and metastasis. J. Biol. Chem. 287, 11070–11081. doi:10.1074/jbc.M111.302547
Maeda, K., Kang, S. M., Sawada, T., Nishiguchi, Y., Yashiro, M., Ogawa, Y., et al. (2002). Expression of intercellular adhesion molecule-1 and prognosis in colorectal cancer. Oncol. Rep. 9, 511–514.
Meesmann, H. M., Fehr, E. M., Kierschke, S., Herrmann, M., Bilyy, R., Heyder, P., et al. (2010). Decrease of sialic acid residues as an eat-me signal on the surface of apoptotic lymphocytes. J. Cell Sci. 123, 3347–3356. doi:10.1242/jcs.066696
Meezan, E., Wu, H. C., Black, P. H., and Robbins, P. W. (1969). Comparative studies on the carbohydrate-containing membrane components of normal and virus-transformed mouse fibroblasts. II. Separation of glycoproteins and glycopeptides by sephadex chromatography. Biochemistry 8, 2518–2524. doi:10.1021/bi00834a039
Meng, Q. T., Ren, C. L., Wang, L. P., Zhao, Y. J., and Wang, S. J. (2015). Knockdown of ST6Gal-I inhibits the growth and invasion of osteosarcoma MG-63 cells. Biomed. Pharmacother. 72, 172–178. doi:10.1016/j.biopha.2015.04.020
Milflores-Flores, L., Millan-Perez, L., Santos-Lopez, G., Reyes-Leyva, J., and Vallejo-Ruiz, V. (2012). Characterization of P1 promoter activity of the beta-galactoside alpha2, 6-sialyltransferase I gene (siat 1) in cervical and hepatic cancer cell lines. J. Biosci. 37, 259–267. doi:10.1007/s12038-012-9194-6
Minami, A., Shimono, Y., Mizutani, K., Nobutani, K., Momose, K., Azuma, T., et al. (2013). Reduction of the ST6 beta-galactosamide alpha-2, 6-sialyltransferase 1 (ST6GAL1)-catalyzed sialylation of nectin-like molecule 2/cell adhesion molecule 1 and enhancement of ErbB2/ErbB3 signaling by MicroRNA-199a. J. Biol. Chem. 288, 11845–11853. doi:10.1074/jbc.M112.405993
Mondal, S., Chandra, S., and Mandal, C. (2010). Elevated mRNA level of hST6Gal I and hST3Gal V positively correlates with the high risk of pediatric acute leukemia. Leuk. Res. 34, 463–470. doi:10.1016/j.leukres.2009.07.042
Moss, E. L., Hollingworth, J., and Reynolds, T. M. (2005). The role of CA125 in clinical practice. J. Clin. Pathol. 58, 308–312. doi:10.1136/jcp.2004.018077
Munkley, J., and Elliott, D. J. (2016). Hallmarks of glycosylation in cancer. Oncotarget 7, 35478–35489. doi:10.18632/oncotarget.8155
Natoli, G., Ianni, A., Costanzo, A., Depetrillo, G., Ilari, I., Chirillo, P., et al. (1995). Resistance to fas-mediated apoptosis in human hepatoma-cells. Oncogene 11, 1157–1164.
Nijkamp, M. W., Hoogwater, F. J. H., Steller, E. J. A., Westendorp, B. F., van der Meulen, T. A., Leenders, M. W. H., et al. (2010). CD95 is a key mediator of invasion and accelerated outgrowth of mouse colorectal liver metastases following radiofrequency ablation. J. Hepatol. 53, 1069–1077. doi:10.1016/j.jhep.2010.04.040
O'Connell, J., Bennett, M. W., O'Sullivan, G. C., Roche, D., Kelly, J., Collins, J. K., et al. (1998). Fas ligand expression in primary colon adenocarcinomas: Evidence that the Fas counterattack is a prevalent mechanism of immune evasion in human colon cancer. J. Pathol. 186, 240–246. doi:10.1002/(SICI)1096-9896(199811)186:3<240:AID-PATH173>3.0.CO;2-L
Ou, L., He, X., Liu, N., Song, Y., Li, J., Gao, L., et al. (2020). Sialylation of FGFR1 by ST6GalI overexpression contributes to ovarian cancer cell migration and chemoresistance. Mol. Med. Rep. 21, 1449–1460. doi:10.3892/mmr.2020.10951
Pan, B., Guo, J., Liao, Q., and Zhao, Y. (2018). β1 and β3 integrins in breast, prostate and pancreatic cancer: A novel implication. Oncol. Lett. 15, 5412–5416. doi:10.3892/ol.2018.8076
Park, J. J., Yi, J. Y., Jin, Y. B., Lee, Y. J., Lee, J. S., Lee, Y. S., et al. (2012). Sialylation of epidermal growth factor receptor regulates receptor activity and chemosensitivity to gefitinib in colon cancer cells. Biochem. Pharmacol. 83, 849–857. doi:10.1016/j.bcp.2012.01.007
Patel, S., Alam, A., Pant, R., and Chattopadhyay, S. (2019). Wnt signaling and its significance within the tumor microenvironment: Novel therapeutic insights. Front. Immunol. 10, 2872. doi:10.3389/fimmu.2019.02872
Peter, M. E., Budd, R. C., Desbarats, J., Hedrick, S. M., Hueber, A. O., Newell, M. K., et al. (2007). The CD95 receptor: Apoptosis revisited. Cell 129, 447–450. doi:10.1016/j.cell.2007.04.031
Peter, M. E., Hellbardt, S., Schwartzalbiez, R., Westendorp, M. O., Walczak, H., Moldenhauer, G., et al. (1995). Cell-surface sialylation plays a role in modulating sensitivity towards apo-1-mediated apoptotic cell-death. Cell Death Differ. 2, 163–171.
Pinho, S. S., and Reis, C. A. (2015). Glycosylation in cancer: Mechanisms and clinical implications. Nat. Rev. Cancer 15, 540–555. doi:10.1038/nrc3982
Pochec, E., Litynska, A., Amoresano, A., and Casbarra, A. (2003). Glycosylation profile of integrin alpha 3 beta 1 changes with melanoma progression. Biochim. Biophys. Acta 1643, 113–123. doi:10.1016/j.bbamcr.2003.10.004
Pretzlaff, R. K., Xue, V. W., and Rowin, M. E. (2000). Sialidase treatment exposes the beta1-integrin active ligand binding site on HL60 cells and increases binding to fibronectin. Cell Adhes. Commun. 7, 491–500. doi:10.3109/15419060009040306
Qian, J., Zhu, C. H., Tang, S., Shen, A. J., Ai, J., Li, J., et al. (2009). alpha2, 6-hyposialylation of c-Met abolishes cell motility of ST6Gal-I-knockdown HCT116 cells. Acta Pharmacol. Sin. 30, 1039–1045. doi:10.1038/aps.2009.84
Rao, T. C., Beggs, R. R., Ankenbauer, K. E., Hwang, J., Ma, V. P., Salaita, K., et al. (2022). ST6Gal-I-mediated sialylation of the epidermal growth factor receptor modulates cell mechanics and enhances invasion. J. Biol. Chem. 298, 101726. doi:10.1016/j.jbc.2022.101726
Rodrigues, J. G., Duarte, H. O., Gomes, C., Balmana, M., Martins, A. M., Hensbergen, P. J., et al. (2021). Terminal alpha 2, 6-sialylation of epidermal growth factor receptor modulates antibody therapy response of colorectal cancer cells. Cell. Oncol. 44, 835–850. doi:10.1007/s13402-021-00606-z
Rodriguez, E., Schetters, S. T. T., and van Kooyk, Y. (2018). The tumour glyco-code as a novel immune checkpoint for immunotherapy. Nat. Rev. Immunol. 18, 204–211. doi:10.1038/nri.2018.3
Romorini, L., Garate, X., Neiman, G., Luzzani, C., Furmento, V. A., Guberman, A. S., et al. (2016). AKT/GSK3 beta signaling pathway is critically involved in human pluripotent stem cell survival. Sci. Rep. 6, 35660. doi:10.1038/srep35660
Sanjabi, S., Oh, S. A., and Li, M. O. (2017). Regulation of the immune response by TGF-β: From conception to autoimmunity and infection. Cold Spring Harb. Perspect. Biol. 9, a022236. doi:10.1101/cshperspect.a022236
Sartakhti, J. S., Manshaei, M. H., and Sadeghi, M. (2017). MMP-TIMP interactions in cancer invasion: An evolutionary game-theoretical framework. J. Theor. Biol. 412, 17–26. doi:10.1016/j.jtbi.2016.09.019
Sasaki, T., Hiroki, K., and Yamashita, Y. (2013). The role of epidermal growth factor receptor in cancer metastasis and microenvironment. Biomed. Res. Int. 2013, 546318. doi:10.1155/2013/546318
Schliekelman, M. J., Gibbons, D. L., Faca, V. M., Creighton, C. J., Rizvi, Z. H., Zhang, Q., et al. (2011). Targets of the tumor suppressor miR-200 in regulation of the epithelial-mesenchymal transition in cancer. Cancer Res. 71, 7670–7682. doi:10.1158/0008-5472.CAN-11-0964
Schultz, M. J., Holdbrooks, A. T., Chakraborty, A., Grizzle, W. E., Landen, C. N., Buchsbaum, D. J., et al. (2016). The tumor-associated glycosyltransferase ST6Gal-I regulates stem cell transcription factors and confers a cancer stem cell phenotype. Cancer Res. 76, 3978–3988. doi:10.1158/0008-5472.CAN-15-2834
Schultz, M. J., Swindall, A. F., Wright, J. W., Sztul, E. S., Landen, C. N., Bellis, S. L., et al. (2013). ST6Gal-I sialyltransferase confers cisplatin resistance in ovarian tumor cells. J. Ovarian Res. 6, 25. doi:10.1186/1757-2215-6-25
Seales, E. C., Jurado, G. A., Brunson, B. A., Wakefield, J. K., Frost, A. R., Beilis, S. L., et al. (2005). Hypersialylation of beta(1) integrins, observed in colon adenocarcinoma, may contribute to cancer progression by up-regulating cell motility. Cancer Res. 65, 4645–4652. doi:10.1158/0008-5472.CAN-04-3117
Seales, E. C., Jurado, G. A., Singhal, A., and Bellis, S. L. (2003). Ras oncogene directs expression of a differentially sialylated, functionally altered beta1 integrin. Oncogene 22, 7137–7145. doi:10.1038/sj.onc.1206834
Seales, E. C., Shaikh, F. M., Woodard-Grice, A. V., Aggarwal, P., McBrayer, A. C., Hennessy, K. M., et al. (2005). A protein kinase C/Ras/ERK signaling pathway activates myeloid fibronectin receptors by altering beta1 integrin sialylation. J. Biol. Chem. 280, 37610–37615. doi:10.1074/jbc.M508476200
Semel, A. C., Seales, E. C., Singhal, A., Eklund, E. A., Colley, K. J., Bellis, S. L., et al. (2002). Hyposialylation of integrins stimulates the activity of myeloid fibronectin receptors. J. Biol. Chem. 277, 32830–32836. doi:10.1074/jbc.M202493200
Shaikh, F. M., Seales, E. C., Clem, W. C., Hennessy, K. M., Zhuo, Y., Bellis, S. L., et al. (2008). Tumor cell migration and invasion are regulated by expression of variant integrin glycoforms. Exp. Cell Res. 314, 2941–2950. doi:10.1016/j.yexcr.2008.07.021
Sigismund, S., Avanzato, D., and Lanzetti, L. (2018). Emerging functions of the EGFR in cancer. Mol. Oncol. 12, 3–20. doi:10.1002/1878-0261.12155
Smithson, M., Irwin, R., Williams, G., Alexander, K. L., Smythies, L. E., Nearing, M., et al. (2022). Sialyltransferase ST6GAL-1 mediates resistance to chemoradiation in rectal cancer. J. Biol. Chem. 298, 101594. doi:10.1016/j.jbc.2022.101594
Suzuki, O., Abe, M., and Hashimoto, Y. (2015). Caspase-dependent drug-induced apoptosis is regulated by cell surface sialylation in human B-cell lymphoma. Oncol. Lett. 10, 687–690. doi:10.3892/ol.2015.3320
Suzuki, O., Nozawa, Y., and Abe, M. (2003). Sialic acids linked to glycoconjugates of Fas regulate the caspase-9-dependent and mitochondria-mediated pathway of Fas-induced apoptosis in Jurkat T cell lymphoma. Int. J. Oncol. 23, 769–774.
Svensson, E. C., Conley, P. B., and Paulson, J. C. (1992). Regulated expression of alpha 2, 6-sialyltransferase by the liver-enriched transcription factors HNF-1, DBP, and LAP. J. Biol. Chem. 267, 3466–3472. doi:10.1016/s0021-9258(19)50754-2
Swindall, A. F., and Bellis, S. L. (2011). Sialylation of the Fas death receptor by ST6Gal-I provides protection against fas-mediated apoptosis in colon carcinoma cells. J. Biol. Chem. 286, 22982–22990. doi:10.1074/jbc.M110.211375
Swindall, A. F., Londono-Joshi, A. I., Schultz, M. J., Fineberg, N., Buchsbaum, D. J., Bellis, S. L., et al. (2013). ST6Gal-I protein expression is upregulated in human epithelial tumors and correlates with stem cell markers in normal tissues and colon cancer cell lines. Cancer Res. 73, 2368–2378. doi:10.1158/0008-5472.CAN-12-3424
Tachimori, A., Yamada, N., Sakate, Y., Yashiro, M., Maeda, K., Ohira, M., et al. (2005). Up regulation of ICAM-1 gene expression inhibits tumour growth and liver metastasis in colorectal carcinoma. Eur. J. Cancer 41, 1802–1810. doi:10.1016/j.ejca.2005.04.036
Takeda, K., Stagg, J., Yagita, H., Okumura, K., and Smyth, M. J. (2007). Targeting death-inducing receptors in cancer therapy. Oncogene 26, 3745–3757. doi:10.1038/sj.onc.1210374
Taniguchi, A., Higai, K., Hasegawa, Y., Utsumi, K., and Matsumoto, K. (1998). Differentiation elicits negative regulation of human beta-galactoside alpha2, 6-sialyltransferase at the mRNA level in the HL-60 cell line. FEBS Lett. 441, 191–194. doi:10.1016/s0014-5793(98)01548-8
Tao, Y., Zhao, Z. J., Ma, J. F., Dong, L. Y., Liang, Y., Li, S. Q., et al. (2019). MiR-214-3p regulates the viability, invasion, migration and EMT of TNBC cells by targeting ST6GAL1. Cytotechnology 71, 1155–1165. doi:10.1007/s10616-019-00352-z
Thomas, D., Rathinavel, A. K., and Radhakrishnan, P. (2021). Altered glycosylation in cancer: A promising target for biomarkers and therapeutics. Biochim. Biophys. Acta. Rev. Cancer 1875, 188464. doi:10.1016/j.bbcan.2020.188464
Uribe, M. L., Marrocco, I., and Yarden, Y. (2021). EGFR in cancer: Signaling mechanisms, drugs, and acquired resistance. Cancers 13, 2748. doi:10.3390/cancers13112748
Vajaria, B. N., and Patel, P. S. (2017). Glycosylation: A hallmark of cancer? Glycoconj. J. 34, 147–156. doi:10.1007/s10719-016-9755-2
Vandamme, V., Cazlaris, H., Le Marer, N., Laudet, V., Lagrou, C., Verbert, A., et al. (1992). Comparison of sialyl- and alpha-1, 3-galactosyltransferase activity in NIH3T3 cells transformed with ras oncogene: Increased beta-galactoside alpha-2, 6-sialyltransferase. Biochimie 74, 89–99. doi:10.1016/0300-9084(92)90188-k
Verge, C., Bouchatal, A., Chirat, F., Guerardel, Y., Maftah, A., Petit, J. M., et al. (2020). Involvement of ST6Gal I-mediated alpha 2, 6 sialylation in myoblast proliferation and differentiation. Febs Open Bio 10, 56–69. doi:10.1002/2211-5463.12745
Vojta, A., Samarzija, I., Bockor, L., and Zoldos, V. (2016). Glyco-genes change expression in cancer through aberrant methylation. Biochim. Biophys. Acta 1860, 1776–1785. doi:10.1016/j.bbagen.2016.01.002
Walczak, H. (2013). Death receptor-ligand systems in cancer, cell death, and inflammation. Cold Spring Harb. Perspect. Biol. 5, a008698. doi:10.1101/cshperspect.a008698
Wang, L., Li, S., Yu, X., Han, Y., Wu, Y., Wang, S., et al. (2019). α2, 6-Sialylation promotes immune escape in hepatocarcinoma cells by regulating T cell functions and CD147/MMP signaling. J. Physiol. Biochem. 75, 199–207. doi:10.1007/s13105-019-00674-8
Wang, L. P., Chen, X. X., Wang, L. Y., Wang, S. J., Li, W. L., Liu, Y. B., et al. (2021). Knockdown of ST6Gal-I expression in human hepatocellular carcinoma cells inhibits their exosome-mediated proliferation- and migration-promoting effects. Iubmb Life 73, 1378–1391. doi:10.1002/iub.2562
Wang, P. H., Lee, W. L., Lee, Y. R., Juang, C. M., Chen, Y. J., Chao, H. T., et al. (2003). Enhanced expression of alpha 2, 6-sialyltransferase ST6Gal I in cervical squamous cell carcinoma. Gynecol. Oncol. 89, 395–401. doi:10.1016/s0090-8258(03)00127-6
Wang, Y. C., Stein, J. W., Lynch, C. L., Tran, H. T., Lee, C. Y., Coleman, R., et al. (2015). Glycosyltransferase ST6GAL1 contributes to the regulation of pluripotency in human pluripotent stem cells. Sci. Rep. 5, 13317. doi:10.1038/srep13317
Wei, A., Fan, B., Zhao, Y., Zhang, H., Wang, L., Yu, X., et al. (2016). ST6Gal-I overexpression facilitates prostate cancer progression via the PI3K/Akt/GSK-3β/β-catenin signaling pathway. Oncotarget 7, 65374–65388. doi:10.18632/oncotarget.11699
Welch, L. G., and Munro, S. (2019). A tale of short tails, through thick and thin: Investigating the sorting mechanisms of Golgi enzymes. FEBS Lett. 593, 2452–2465. doi:10.1002/1873-3468.13553
Wen, X., Wu, Y., Awadasseid, A., Tanaka, Y., and Zhang, W. (2020). New advances in canonical wnt/β-catenin signaling in cancer. Cancer Manag. Res. 12, 6987–6998. doi:10.2147/CMAR.S258645
Wichert, B., Milde-Langosch, K., Galatenko, V., Schmalfeldt, B., and Oliveira-Ferrer, L. (2018). Prognostic role of the sialyltransferase ST6GAL1 in ovarian cancer. Glycobiology 28, 898–903. doi:10.1093/glycob/cwy065
Windisch, R., Pirschtat, N., Kellner, C., Chen-Wichmann, L., Lausen, J., Humpe, A., et al. (2019). Oncogenic deregulation of cell adhesion molecules in leukemia. Cancers (Basel) 11, E311. doi:10.3390/cancers11030311
Woodard-Grice, A. V., McBrayer, A. C., Wakefield, J. K., Zhuo, Y., and Bellis, S. L. (2008). Proteolytic shedding of ST6Gal-I by BACE1 regulates the glycosylation and function of alpha4beta1 integrins. J. Biol. Chem. 283, 26364–26373. doi:10.1074/jbc.M800836200
Xu, C., Wang, S., Wu, Y., Sun, X., Yang, D., Wang, S., et al. (2021). Recent advances in understanding the roles of sialyltransferases in tumor angiogenesis and metastasis. Glycoconj. J. 38, 119–127. doi:10.1007/s10719-020-09967-3
Xu, L., Kurusu, Y., Takizawa, K., Tanaka, J., Matsumoto, K., Taniguchi, A., et al. (2003). Transcriptional regulation of human beta-galactoside alpha2, 6-sialyltransferase (hST6Gal I) gene in colon adenocarcinoma cell line. Biochem. Biophys. Res. Commun. 307, 1070–1074. doi:10.1016/s0006-291x(03)01314-7
Yoshimura, A., and Muto, G. (2011). TGF-Beta function in immune suppression. Curr. Top. Microbiol. Immunol. 350, 127–147. doi:10.1007/82_2010_87
Yu, S., Fan, J., Liu, L., Zhang, L., Wang, S., Zhang, J., et al. (2013). Caveolin-1 up-regulates integrin α2, 6-sialylation to promote integrin α5β1-dependent hepatocarcinoma cell adhesion. FEBS Lett. 587, 782–787. doi:10.1016/j.febslet.2013.02.002
Yuan, K. Y., Jing, G., Chen, J. F., Liu, H., Zhang, K., Li, Y. B., et al. (2011). Calmodulin mediates fas-induced FADD-independent survival signaling in pancreatic cancer cells via activation of src-extracellular signal-regulated kinase (ERK). J. Biol. Chem. 286, 24776–24784. doi:10.1074/jbc.M110.202804
Yuan, Q., Chen, X., Han, Y., Lei, T., Wu, Q., Yu, X., et al. (2018). Modification of α2, 6-sialylation mediates the invasiveness and tumorigenicity of non-small cell lung cancer cells in vitro and in vivo via Notch1/Hes1/MMPs pathway. Int. J. Cancer 143, 2319–2330. doi:10.1002/ijc.31737
Zhan, T., Rindtorff, N., and Boutros, M. (2017). Wnt signaling in cancer. Oncogene 36, 1461–1473. doi:10.1038/onc.2016.304
Zhang, J., Liu, Y., Deng, X., Chen, L. M., Yang, X., Yu, C., et al. (2018a). ST6GAL1 negatively regulates monocyte transendothelial migration and atherosclerosis development. Biochem. Biophys. Res. Commun. 500, 249–255. doi:10.1016/j.bbrc.2018.04.053
Zhang, M., Qi, T., Yang, L., Kolarich, D., and Heisterkamp, N. (2022). Multi-faceted effects of ST6Gal1 expression on precursor B-lineage acute lymphoblastic leukemia. Front. Oncol. 12, 828041. doi:10.3389/fonc.2022.828041
Zhang, S., Lu, J., Xu, Z., Zou, X., Sun, X., Xu, Y., et al. (2017). Differential expression of ST6GAL1 in the tumor progression of colorectal cancer. Biochem. Biophys. Res. Commun. 486, 1090–1096. doi:10.1016/j.bbrc.2017.03.167
Zhang, W. Q., Xiong, Z. G., Wei, T. Q., Li, Q. M., Tan, Y., Ling, L., et al. (2018b). Nuclear factor 90 promotes angiogenesis by regulating HIF-1α/VEGF-A expression through the PI3K/Akt signaling pathway in human cervical cancer. Cell Death Dis. 9, 276. doi:10.1038/s41419-018-0334-2
Zhang, X. P., Pan, C. C., Zhou, L., Cai, Z. G., Zhao, S. F., Yu, D. H., et al. (2016). Knockdown of ST6Gal-I increases cisplatin sensitivity in cervical cancer cells. Bmc Cancer 16, 949. doi:10.1186/s12885-016-2981-y
Zhao, Y., Li, Y., Ma, H., Dong, W., Zhou, H., Song, X., et al. (2014). Modification of sialylation mediates the invasive properties and chemosensitivity of human hepatocellular carcinoma. Mol. Cell. Proteomics 13, 520–536. doi:10.1074/mcp.M113.034025
Zhou, L., Zhang, S., Zou, X., Lu, J., Yang, X., Xu, Z., et al. (2019). The β-galactoside α2, 6-sialyltranferase 1 (ST6GAL1) inhibits the colorectal cancer metastasis by stabilizing intercellular adhesion molecule-1 via sialylation. Cancer Manag. Res. 11, 6185–6199. doi:10.2147/CMAR.S208631
Zhuo, Y., and Bellis, S. L. (2011). Emerging role of alpha2, 6-sialic acid as a negative regulator of galectin binding and function. J. Biol. Chem. 286, 5935–5941. doi:10.1074/jbc.R110.191429
Keywords: ST6GAL1, cancer, pathways affecting cancer, targets, sialyltransferase, sialylation
Citation: Sajina GC, Bellis SL and Hjelmeland AB (2022) ST6Gal1: Oncogenic signaling pathways and targets. Front. Mol. Biosci. 9:962908. doi: 10.3389/fmolb.2022.962908
Received: 06 June 2022; Accepted: 11 July 2022;
Published: 29 August 2022.
Edited by:
M. Florencia Haurat, United States Food and Drug Administration, United StatesReviewed by:
Jennifer Munkley, Newcastle University, United KingdomCopyright © 2022 Sajina, Bellis and Hjelmeland. This is an open-access article distributed under the terms of the Creative Commons Attribution License (CC BY). The use, distribution or reproduction in other forums is permitted, provided the original author(s) and the copyright owner(s) are credited and that the original publication in this journal is cited, in accordance with accepted academic practice. No use, distribution or reproduction is permitted which does not comply with these terms.
*Correspondence: Anita B. Hjelmeland, aGplbG1lYUB1YWIuZWR1