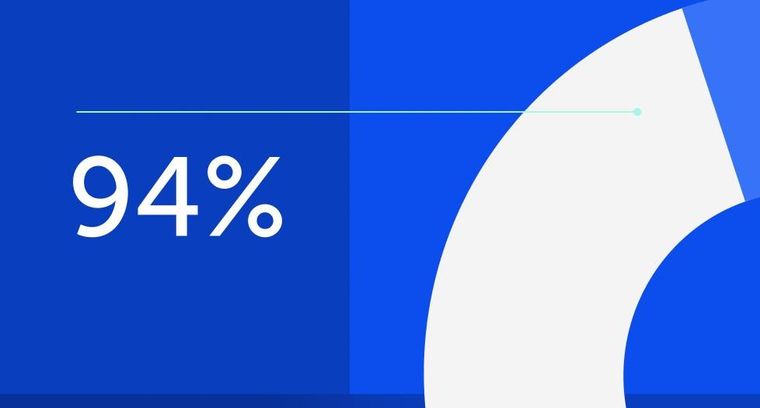
94% of researchers rate our articles as excellent or good
Learn more about the work of our research integrity team to safeguard the quality of each article we publish.
Find out more
REVIEW article
Front. Mol. Biosci., 07 September 2022
Sec. RNA Networks and Biology
Volume 9 - 2022 | https://doi.org/10.3389/fmolb.2022.944774
This article is part of the Research TopicRNAi-Based Biotechnology Approaches Applied to Plant-Pest ControlView all articles
The fall armyworm Spodoptera frugiperda (Lepidoptera: Noctuidae) is among the invasive insect pests that damages maize and sorghum, the high-priority crops in newly colonized agro-ecologies, including African contexts. Owing to the increasing infestation of the pest and the limitations of current conventional methods for its management, there is a call for discovering advanced pest management approaches. RNA interference (RNAi) is an emerging molecular tool showing flexible potential for the management of S. frugiperda. We conducted a search of the recent application of RNAi literature using Google Scholar and Mendeley to find advanced papers on S. frugiperda management using RNAi molecular tools that led to growth inhibition, developmental aberrations, reduced fecundity, and mortality, mainly by disruption of normal biological processes of the pest. Although efforts have been made to accelerate the utility of RNAi, many factors limit the efficiency of RNAi to achieve successful control over S. frugiperda. Owing to RNAi’s potential bioactivity and economic and ecological acceptability, continued research efforts should focus on improving its broad applicability, including field conditions. Screening and identification of key target genes should be a priority task to achieve effective and sustainable management of this insect via RNAi. In addition, a clear understanding of the present status of RNAi utilization in S. frugiperda management is of paramount importance to improve its efficiency. Therefore, in this review, we highlight the biology of S. frugiperda and the RNAi mechanism as a foundation for the molecular management of the pest. Then, we discuss the current knowledge of the RNAi approach in S. frugiperda management and the factors affecting the efficiency of RNAi application. Finally, the prospects for RNAi-based insect pest management are highlighted for future research to achieve effective management of S. frugiperda.
Fall armyworm, Spodoptera frugiperda (J.E. Smith) (Lepidoptera: Noctuidae), a voracious invasive insect pest, is among the key production constraints for several crops, including maize and forage grasses, in areas under invasion, including African countries (Prasanna et al., 2018; Ren et al., 2019; Sisay et al., 2019; Wee et al., 2022). The larvae of S. frugiperda cause significant damage or losses to maize and sorghum and threaten food security in Africa (Day et al., 2017; Assefa and Ayalew, 2019; Gichuhi et al., 2020). In response to pest invasion, synthetic insecticides and cultural and mechanical practices are among the suggested management methods (Prasanna et al., 2018; Sisay et al., 2019; Mehlhorn et al., 2021). However, due to pest aggressiveness or superior biological characteristics, none of the aforementioned tactics achieved the required level of control, and the pest continues to cause substantial losses in most of the colonized areas. According to Gichuhi et al. (2020) and Du-Plessis et al. (2020), the management of S. frugiperda is challenging due to its biological characteristics, including its ability to survive in various environments and a wide range of hosts. On the other hand, synthetic insecticides are still the major approach for controlling insect pests, yet their use is associated with significant hazards to environmental components and human well-being (Prasanna et al., 2018; Xiao-Shuang et al., 2021). Therefore, the investigation of advanced approaches based on molecular systems is mandatory to achieve effective and sustainable management options against S. frugiperda.
Among emerging molecular technologies, RNA interference (RNAi) is a potential biological tool to control target insect species (Olawale et al., 2019; Bai-Zhong et al., 2020; Gurusamy et al., 2020a; Gregoriou et al., 2021; Mehlhorn et al., 2021; Xiao-Shuang et al., 2021). RNAi is a mechanism of gene instruction and an antiviral defense system in a cell, resulting in the sequence-specific degradation of messenger RNA (mRNAs) either by directing inhibitory chromatin modifications or by decreasing the stability or translation potential of the targeted mRNA (Andrew et al., 2001; Liu et al., 2020; Mehlhorn et al., 2021). Double-stranded RNA (dsRNA) generated from an endogenous genomic locus or a foreign source, such as a transgene or virus, is responsible for gene silencing (Liu et al., 2020; Hernández-Soto and Chacón-Cerdas, 2021). The RNAi machinery possesses vital gene “core genes”, which are crucial for its efficiency and strong response in gene silencing (Dowling et al., 2016; Davis-Vogel et al., 2018).
RNAi-based technology shows pronounced potential for use in agriculture, particularly for the management of destructive insect pests, such as S. frugiperda (Davis-Vogel et al., 2018; Bai-Zhong et al., 2020; Gurusamy et al., 2020b; Mehlhorn et al., 2021; Wan et al., 2021). RNAi application in Lepidoptera was first reported in 2001 (Bettencourt et al., 2002). Reports have indicated that different insects have shown a wide range of sensitivity to environmentally introduced RNAs. On the other hand, emphasis has been placed on developing dsRNA application strategies for insect management under field conditions through genetically modified organisms (GMOs) in which transgenic plants produce a dsRNA that silences a target insect gene following ingestion and direct application of dsRNA onto plants (Tabashnik and Carrière, 2017; Rosa et al., 2018; Ren et al., 2019; Shuo et al., 2020; DeSchutter et al., 2021). Recently, Rodriguez et al. (2021) demonstrated a sprayable dsRNA bioinsecticide with a novel mode of action that activates the RNAi pathway in Leptinotarsa decemlineata. Consequently, there was a sufficient level of efficacy of the sprayable dsRNA product over this insect under both field and laboratory conditions. Insecticidal gene silencing using RNAi encompasses a posttranscriptional mechanism with high potential for target insect pest control (Dias et al., 2020). In other words, field trials are confirming the power of spray-induced gene silencing (SIGS) based bioinsecticides and subsequently promoting their transition to the market, although further improvement is needed to boost the field efficacy of SIGS and sustain RNAi technology (R odriguez et al., 2021; DeSchutter et al., 2021). Currently, promising results in gene lockdown or silencing with a recognized side effect in normal biological activities are being reported in S. frugiperda (Rodríguez-Cabrera et al., 2010; Vatanparast and Park, 2022), signifying its potential as a component of pest management. However, the sustainability of these technologies is threatened by increased field resistance in S. frugiperda (Tabashnik, and Carriere, 2017; Wang et al., 2017). In lepidopterans, in addition to their refractory nature, several factors, such as the RNAi machinery, dose or concentration of the dsRNA, insect life stage and tissue used, dsRNA molecule delivered, enzymes/double-stranded ribonucleases (dsRNases); duration of exposure, and measurement points, limit the efficiency of insecticidal RNAi (Ruo-Bing et al., 2018; Peng et al., 2019; Rodríguez-de la Noval et al., 2019; Liu et al., 2020; Chen et al., 2021). Studies on RNAi present both positive implications and the limitations associated with this emerging tool, providing a range of possibilities to improve RNAi efficiency and realize its utilization as a potential ecological approach for the management of S. frugiperda.
In general, understanding differences in insect responses to RNAi is central to the development and proper implementation of RNAi-based insect pest management. Promisingly, different RNAi-based research findings have been reported for the management of S. frugiperda, an invasive insect pest. However, the present knowledge of this technology is not well evaluated with due emphasis on establishing research gaps for further work. On the other hand, searching and evaluating the current information is necessary to obtain knowledge and a better understanding of the present achievement on this pest and prospects to improve the RNAi mechanism and/or to utilize the available technologies. Hence, in this review, the biology of S. frugiperda and the RNAi mechanism are emphasized as a basis for the molecular management of the pest. Then, we discuss the current knowledge of the RNAi approach in S. frugiperda management and summarize the factors influencing the success of RNAi application. Finally, the potential prospects of RNAi-based technologies are identified for future research to achieve effective management against the pest.
A literature search was conducted using Google Scholar and Mendeley (Mendeley-Desktop-1.19.8) with several quest terms. The databases were used because they contained research articles that were available in full text and had undergone peer-review by scientists with advanced search functions. The search terms included the following: (“S. frugiperda” and “biology”), (“RNAi mechanism and lepidopterans”), (“S. frugiperda” and “biological control”), (“S. frugiperda” and “RNAi application”), (“S. frugiperda” and “gene silencing”), (“S. frugiperda” and “RNAi effects”), (“S. frugiperda” and “RNAi delivery methods”), (“S. frugiperda” and “RNAi inefficiency”) and (“S. frugiperda” and “RNAi prospects”) was used to generate a databank of advanced papers or publications that assess RNAi application efforts in a S. frugiperda insect pest management context. The search was limited to the most recent publications that were written in English. Studies that used RNAi-mediated strategies for fall armyworm control, and assessed its effects on biology, physiology and/or behavior were emphasized. Relevant information was extracted from all articles that resulted from the literature search and screening references therein. Accordingly, RNAi application achievements, including target genes, delivery methods, target stage and effects on biological processes (Table 1) and the main factors influencing RNAi-based S. frugiperda management (Table 2), and future lines of research are summarized in this review.
TABLE 2. Some of the factors influencing the efficiency of RNAi application in S. frugiperda management.
A better understanding of the biology of S. frugiperda, an invasive insect pest, is a foundation for its management in noninvasive ways, particularly through molecular options. S. frugiperda is native to America and remained a pest on the continent until its out-break was described in Nigeria, West Africa, in 2016 (Cock et al., 2017; Goergen et al., 2017; Nagoshi et al., 2017; Otim et al., 2018). Presently, its occurrence has been proven on five continents, including Africa (Michael et al., 2021). As an invasive pest, S. frugiperda has a pronounced capacity to occupy wide ecological zones in a short period (Johnson, 1987; Du -Plessis et al., 2020). The rapid dispersal of S. frugiperda is associated with its biological features, notably high reproductive rates, strong migration capacity, and extensive host range (Gichuhi et al., 2020). Interestingly, although it is polyphagous, S. frugiperda prefers to feed on maize, causing substantial yield penalties in invasive ranges, particularly in African countries (Day et al., 2017; Michael et al., 2021).
The insect undergoes holometabolosis, and its life cycle includes eggs (2–3 days), larvae (13–14 days), pupae (7–8 days) and adults (7–21 days) (Jing-Wan et al., 2021; Michael et al., 2021). S. frugiperda has a generation time of approximately 30–40 days during the warm summer months (daily temperature of ∼28°C) and approximately 55 days at cooler temperatures (Prasanna et al., 2018; Sharanabasappa et al., 2018). The individual adult female can lay 1,500–2000 eggs in her life (Johnson, 1987). The eggs are laid on leaves in batches containing 100–200 eggs (Johnson, 1987; FAO, 2019). The eggs hatch in two to 3 days during summer (20–30°C) (Capinera and Smith, 1999; Sharanabasappa et al., 2018). There are six larval instars with a development duration of two to 3 weeks, with the last instar being the most destructive (causing 70% of S. frugiperda damage) (Assefa and Ayalew, 2019). The pest cannot enter diapause (Johnson, 1987); hence, the number of generations produced in invaded areas is determined by the suitability of environmental situations, mainly temperatures coupled with the host plants (Prasanna et al., 2018; Du -Plessis et al., 2020); for instance, in tropical zones, it can have up to eight generations in 1 year in maize fields (Busato et al., 2005).
At present, two strains of S. frugiperda, namely, the “corn” (C strain) and the “rice” (R strain), are known in both the native and invasive ranges (Nagoshi et al., 2019). Accordingly, the corn strain damages corn/maize, sorghum and cotton, but the rice strain damages rice, silk grass and forage grasses. Despite their morphological similarity, however, the two strains differ in genetics, physiology, and behavioral features, such as mating and insecticidal resistance (Pashley, 1988; Nagoshi et al., 2018). S. frugiperda is known as a super pest due to its extensive host range (more than 350 plant species) from 76 plant families (Montezano et al., 2018), its inherent capacity to live in extensive habitats (Jing-Wan et al., 2021), and its remarkable mobility, high fecundity, quick resistance development to insecticidal treatments or external bodies and gluttonous characteristics (Huang et al., 2019; Gichuhi et al., 2020; Gui et al., 2020; Wee et al., 2022). Notably, the distinct inherited biological features of S. frugiperda enhance its invasiveness (Jing-Wan et al., 2021; Wee et al., 2022). In other words, genome research has greatly assisted in understanding the invasive mechanisms of alien species (Wan et al., 2019); mainly, genomic data mining has improved our understanding of the biological and behavioral characteristics of S. frugiperda contributing to invasiveness (Gimenez et al., 2020; Gui et al., 2020; Xiao et al., 2020; Zhang et al., 2020; Jing-Wan et al., 2021) and is suggested to accelerate the ecological management of the pest both in native and newly invaded ranges.
Several proteins, including enzymes, control every biological function in insects, including the respiratory, digestive, muscular, reproductive, circulatory, neurological, and endocrine systems. For instance, the important insect neuropeptides allatotropin and allatostatin prevent the synthesis of juvenile hormones (Teal, 2002; Griebler et al., 2008; Abdel-latief and Hoffmann, 2014). Optimizing RNAi bioassays for insects requires an understanding of their feeding habits. Examples of artificial feeding bioassays for insects with piercing-sucking mouthparts are employed frequently for RNAi, which is a crucial factor to take into account when creating an efficient RNAi control approach against plant sap feeders (Andrade and Hunter 2016).
Furthermore, continuous genomic functional studies should be emphasized to identify the key genes for its susceptibility to molecular management of S. frugiperda, mainly RNAi interference.
RNAi is a gene silencing mechanism by target gene degradation of mRNA triggered by dsRNA (Andrew et al., 2001; Hernández-Soto, and Chacón-Cerdas, 2021). The RNAi strategy was detected in 1998 when the nematode Caenorhabditis elegans was exposed to dsRNA injection-induced gene silencing (Fire et al., 1998). Currently, there are reports on promising achievements in RNAi-based insect pest management. Moreover, understanding the mechanism of RNAi is key to utilizing this technology against target insect species. In general, the RNAi mechanism involves two main events: first, exogenously delivered dsRNAs are internalized and converted by the cellular RNAi machinery into small interfering RNAs (siRNAs), which then activate the degradation of complementary endogenous mRNAs, leading to gene silencing (Andrew et al., 2001; Tomari and Zamore, 2005; Zotti et al., 2018; Maktura et al., 2021).
Briefly, when a dsRNA molecule targeting a specific gene enters the cell, a ribonuclease III enzyme, Dicer-2 (Dcr-2), recognizes it and fragments it into 21–24 bp molecules, siRNA. A dsRNA-binding protein named R2D2 connects Dcr-2-bound siRNA to the RNA-induced silencing complex (RISC) formed by the Argonaute-2 (Ago-2) protein and other proteins (Ye et al., 2019). Ago-2 unwinds the siRNA duplex and keeps the guide strand connected to RISC. Then, this strand becomes a guide for RISC to bind specifically to target mRNA molecules, and Ago-2 severs the complementary mRNA, resulting in posttranscriptional gene silencing (Joga et al., 2016), as illustrated in Figure 1 below. Although the basic RNAi mechanism exists in all insects, there are important differences in the machinery compounds with respect to their sensitivity to systemic RNAi, such as modifications (Davis-Vogel et al., 2018; Xiao-Shuang et al., 2021), duplications or loss of RNAi-related genes (Dowling et al., 2016), which may explain differences in RNAi effects between insect orders or species. In insects, three RNAi pathways have been detected, namely, the dsRNA/siRNA-mediated siRNA pathway, miRNA-mediated miRNA pathway and pian-interacting RNA (piRNA)-mediated piRNA pathway (Zhou et al., 2008; Han et al., 2015; Zhu and Palli, 2020; Maktura et al., 2021). In addition, further inquiry for a better understanding of RNAi mechanisms or pathways is necessary to enhance its application and/or efficiency in devising sustainable insect pest management.
FIGURE 1. RNA-mediated gene silencing pathways, adapted from Andrew et al. (2001)
Most studies using RNAi in S. frugiperda are focused on growth regulating hormones or involving genes to inhibit insect growth or survival. Consequently, remarkable effects of RNAi have been reported to disrupt normal biological processes, particularly growth and development in S. frugiperda (Griebler et al., 2008; Xiao-Shuang et al., 2021; Chen et al., 2021; Mohammad and Youngjin (2022)), and some of these effects are summarized in Table 1. The application strategies, including the cellular mechanism of RNAi-mediated gene silencing to combat the insect pest, are presented in Figure 2. Feeding or injection of S. frugiperda with dsRNA provided efficient gene transcripts in the midgut and brain tissue, posing significant growth inhibition (Griebler et al., 2008; Rodríguez-Cabrera et al., 2010). Griebler et al. (2008) demonstrated silencing of allatostatin and allatotropin production in adult females of S. frugiperda. Additionally, they confirmed that silencing allatostatin led to an increase in peak larval weight while silencing allatotropin resulted in retarded growth and weight, in treated larvae. Hassanien et al. (2014) also indicated that Spofr-AT gene silencing acts as a true allatotropin in larvae and adults of both sexes of S. frugiperda. Likewise, the injection of S. frugiperda adults and larvae with dsRNA silences the production of allatoregulating neuropeptides (Griebler et al., 2008). Efficient silencing by dsRNA by feeding was highly enhanced when larvae were used immediately after the molt and in addition were subjected to starvation for 24 h (Rodríguez-Cabrera et al., 2010), indicating that the degradation of dsRNA in the midgut of larvae was greatly decreased due to starvation and could be an important factor for the increased sensitivity to dsRNA. Juvenile hormone is a multipurpose hormone that contributes to young growth regulation, reproduction, metabolism, and diapause (Sláma, 2013). Allatostatin and allatotropin are produced by the brains of insects to depress or stimulate corpus allatum production of juvenile hormones, respectively (Sláma, 2013). Hence, it could be concluded that improvement in the application of RNAi targeting juvenile hormones (allatostatin and allatotropin) or involving genes could contribute to the effective management of the pest.
FIGURE 2. Application strategies and the basic cellular mechanism of RNAi-mediated plant protection from a lepidopteran pest control standpoint.
On the other hand, following the increasing trends in the improvement of dsRNA/siRNA delivery methods, the efficiency of RNAi in gene silencing has been enhanced, providing a remarkable adverse effect on insect growth and development. Ectopic expression of CeSid1 enabling the passive uptake of dsRNA from the culture medium was first confirmed in Drosophila melanogaster S2 cells (Feinberg and Hunter, 2003), and effective gene silencing was achieved in S. frugiperda Sf9 cells expressing CeSid1 (Mon et al., 2012; Xu et al., 2013; Xiao-Shuang et al., 2021). Feeding S. frugiperda with bacteria expressing dsRNA (Xiao-Shuang et al., 2021) and systemic RNAi defective protein 1 (CeSid1) negatively affected the growth, development and survival of the insect (Chen et al., 2021). According to Xiao-Shuang et al. (2021), feeding larvae with bacteria expressing dsRNAs of target genes or injecting pupae and adults with bacterially synthesized dsRNA induced silencing of target genes (Sf-CHI, Sf-CHSB, Sf-ST and Sf-HEM) and resulted in considerable adverse effects on the development and survival of S. frugiperda. A significant decrease in larval body weight, with reduced rates of 12.96–28.10%, was noted due to the knockdown of these genes (Xiao-Shuang et al., 2021). Likewise, lower survival rates and prolonged developmental duration were observed following RNAi targeting of genes involved in chitin production in S. frugiperda (Pesch et al., 2016; Zhao et al., 2018). The growth and development of insects are firmly dependent on the precise regulation of chitin synthesis and digestion (Pesch et al., 2016; Zhao et al., 2018) and are responsible for chitin synthesis in the midgut and peritrophic membrane of larvae (Zhang et al., 2012; Yang et al., 2019. Interestingly, injection of pupae with dsCHI significantly reduced the enclosion rate of pupae but increased wing malformation in adults (Xiao-Shuang et al., 2021). Chitin is the main substance of insect wings, and the precise regulation of chitin synthesis and digestion may be crucial for the development of wings (Zhang et al., 2012; Pesch et al., 2016; Zhao et al., 2018) and result in adult wing malformation after the knockdown of Sf-CHI in S. frugiperda (Xiao-Shuang et al., 2021). RNAi targeting Sf-GK2 and Sf-GK1 reduces glycerol biosynthesis or content and consequently affects the growth and development of S. frugiperda by knocking down these genes (Shetty et al., 2019; Mohammad and Youngjin (2022)). RNAi of glycerol biosynthesis genes (Sf-GPDH, Sf-GK1 and Sf-GK2) increases the mortality of treated larvae of S. frugiperda (Mohammad and Youngjin, 2022), which suggests that downregulation of the genes involved in the environmental adaptability of the insect has a paramount role in achieving effective management. In other words, the effect of RNAi on the growth, development and survival of S. frugiperda varied based on the target gene, tissue, growth stage, means of dsRNA/siRNA delivery and others (Yang et al., 2019; Xiao-Shuang et al., 2021; Chen et al., 2021; Mohammad and Youngjin, 2022), and some of these factors are presented in Table 1 below. Consequently, further study is needed to identify different factors as well as the involved mechanism of RNAi inefficiency in S. frugiperda to progress its effectiveness against the pest.
Recent studies have shown that systemic RNAi in Caenorhabditis elegans requires systemic RNAi defective protein 1 (CeSid1) to achieve downregulation of genes and adversely affect insect growth or survival. Chen et al. (2021) suggested the tissue-specific enhancement of RNAi efficiency by CeSid1 in S. frugiperda for improving RNAi in insects such as those belonging to the order Lepidoptera where RNAi is variable and inefficient. For example, the expression of CeSid1 enhanced RNAi efficiency in ovarian Sf9 cells but not in midgut Sf17 cells in S. frugiperda. Interestingly, CeSid1-expressing transgenic S. frugiperda revealed a 93.3% reduction in gene expression in tissues such as Verson’s glands compared to wild-type. The reduced accumulation of dsRNA in late endosomes and successful processing of dsRNA to siRNA contribute to enhanced RNAi efficiency in Sf9 cells (Chen et al., 2021). Further examination of CeSid1 and the mechanism through which this dsRNA transporter improves RNAi efficiency in this insect is necessary. Injection of dsRNA into the abdomen of Blattella germanica caused dramatic depletion of essential α-tubulin gene expression in the insect’s midgut and yielded recognized mortality (Lin et al., 2016). Likewise, Laisney et al. (2020) showed that luciferase gene knockdown was more efficient by exposing Sf9 cells to nanoscaled (size <100 nm) compared to microscaled (size >1 µm), providing 58 and 20%, respectively, with a notable side effect on S. frugiperda larvae. These studies signify the need for the development of an effective delivery system in addition to selecting potential candidate tissues or cells to achieve a required level of gene silencing via RNAi. On the other hand, since the insect midgut is in close proximity to the site of dsRNA entry, the potential of midgut genes as targets for RNAi has been investigated, including genes involved in peritrophic membrane (PM) synthesis providing a recognized reduction in the growth and reproduction of S. frugiperda (Rodriguez-Cabrera et al., 2010; Toprak et al., 2013; Kelkenberg et al., 2015; Ni et al., 2017). Rodríguez-de la Noval et al. (2019) and Silva et al. (2017) demonstrated that peritrophin (SfPER) is a new PM CMCMC-type peritrophin A-like protein from S. frugiperda, whose reduction using RNAi decreases pupal weight and adult emergence, two important parameters of insect development and fitness due to alterations in PM functions related to digestion and nutrition (Table 1). Furthermore, the findings suggested that SfPER could be a potential target for novel pest-control strategies in S. frugiperda. It is particularly beneficial to use gene silencing in the field since its effects are independent of additional behavior or supplementary techniques, leading to impaired development and mortality. Once the delivery difficulty is overcome, delays in growth or development will immediately lessen the number of insects and the resulting damages.
In lepidopterans, an alteration of the reproductive process is an alternative for designing RNAi-mediated insect control strategies (Bettencourt et al., 2002; Rajan et al., 2013). RNAi targeting of different genes causes downregulation or silencing and consequently results in disruption of reproduction in S. frugiperda (Griebler et al., 2008; Shu et al., 2011; Xiao-Shuang et al., 2021). Silencing of allatostatin and allatotropin production in adult females of S. frugiperda reduced fecundity by approximately 40% (Griebler et al., 2008). Likewise, a study on the reproductive fitness of S. frugiperda showed that female adults injected with Hemolin (dsHEM) had a significantly lower mating rate than wild-type males and laid fewer eggs with a lower hatching rate (Xiao-Shuang et al., 2021). Researchers have suggested that Sf-HEM has a vital role in the course of egg production, especially disturbing mating behavior or female interest (Ringo, 1996; Wedell, 2005) and embryo development (Xiao-Shuang et al., 2021). However, the mechanism of Hemolin (Sf-HEM) in reproduction remains unclear and requires further investigation. Some of the effects of RNAi on the reproduction system of S. frugiperda are summarized in Table 1. The heritable RNAi effects on the embryos of the subsequent generation were noticed in lepidopterans following injection of dsRNA into pupae, signifying the entrance of the dsRNA into the gonads of the developing pupae (Bettencourt et al., 2002), and the vitellogenin receptor was also detected as a critical component for binding vitellogenin and transporting it into the oocytes in Spodopteran species (Shu et al., 2011; Xiao-Shuang et al., 2021). The successful achievements in gene knockdown or silencing with significant adverse effects on reproduction make RNAi a promising tool against S. frugiperda. Recent studies have shown that the fertility and the total number of eggs laid per adult female of S. frugiperda were decreased by the particular regulation of Sf-PBANr (neuropeptide receptor activating pheromone biosynthesis) by RNA interference (Park and Vatanparast, 2022). Therefore, it is advisable to improve RNAi efficiency by focusing on gene functional studies for the identification of key genes that govern S. frugiperda reproduction and ways of delivery to sustain its applicability.
In lepidopteran species, mostly S. frugiperda, RNAi is used as a tool to establish the functional relevance of cytochrome P450 and esterase genes to susceptibility against insecticides (Griebler et al., 2008; Hu et al., 2014) and Aminopeptidase-N (APN), cadherin, and ATP-binding cassette (ABC) transporter genes in susceptibility to Bt. Proteins (Gordon and Waterhouse, 2006; Guo et al., 2015; Wang et al., 2017). The application of RNAi machinery as a novel mode of action to control S. frugiperda is a weapon identified to halt the increasing difficulty of pest resistance to insecticides and transgenic crops (Jonathan, 2015). Moreover, most of the RNAi studies provided different levels of gene knockdown rather than complete silencing, and these situations are more of a boost to possibilities than a process available for integrated pest management (IPM) implementation (Griebler et al., 2008; Baum and Roberts, 2014). Therefore, this signifies the potential of RNAi to enhance the susceptibility of lepidopterans to products with verified efficiency when used as a component of IPM, especially in the case of S. frugiperda, to halt its superior biological characteristics.
Plant-mediated RNAi by genetically improved crops expressing dsRNA (Figure 2) is one of the most promising pest control strategies (Tabashnik and Carrière, 2017; Ren et al., 2019). Interestingly, RNAi can play an important role in Bt. crops to delay resistance to S. frugiperda, especially when coupled with other management options to achieve the goal of IPM strategies. According to Ren et al. (2019), stacking multiple RNAi expression cassettes against multiple key targets of the pest will successfully achieve useful agronomic traits for crop protection and production. RNAi attacks on several key genes of the midgut may produce synergistic action against S. frugiperda, which is promising for disrupting or blocking the level of resistance alleles. On the other hand, strong synergistic interactions between Vip3 and Cry proteins, including Cry1Ab, Cry2Ab, Cry1Ca and Cry1Ea, have been observed in S. frugiperda (Tabashnik and Carrière, 2017). Moreover, combining Bt. gene pyramiding, in which diverse Bt toxins can fix to various receptors of pests, with plant-mediated RNAi can targeting multiple key genes for the development, detoxification, digestion and defense of pests (Ren et al., 2019).
Advances in the formulation and delivery of dsRNA play a prominent role in enhancing the efficacy of insecticidal RNAi in controlling lepidopteran insects, including S. frugiperda, by limiting their superior or resistance features. The use of potential coformulants for the successful delivery of dsRNA could contribute to improving the effectiveness of gene silencing by designated RNAi. Recently, most studies explained the increments in target cellular uptake following the utilization of lectins and peptides, protein-based carriers (Shen et al., 2017; Parsons et al., 2018; DeSchutter et al., 2021; Martinez et al., 2021). Martinez et al. (2021) reported the high capability of Galanthus nivalis lectin (GNAF) fusion protein for complexing with dsRNA and significantly increasing the rate of dsRNA uptake in lepidopteran midgut CF1 cells. Accordingly, feeding of GNAF: dsV-ATPase by targeting the essential gene V-ATPase-A increased mortality up to 48% in the GNAF-dsRNA treatment compared to only 8.3% and 6.6% in the control treatments with the naked dsRNA and the GNAF, respectively, and impaired development in S. exigua larvae (Martinez et al., 2021). Although the complexation mechanism of GNAF in protecting dsRNA for RNAi efficacy remains unclear, lectin-based carriers could be added to the list of carriers that offer promising prospects for use in the lepidopteran insect pest management context (Shen et al., 2017; Martinez et al., 2021). In other words, further investigation is suggested to clarify the exact uptake mechanism of these lectins into insect midgut cells in addition to validating the evidence for effective management of S. frugiperda. The conjugation of RNA to cell membrane penetrating peptides has been reported to improve the stability of dsRNA, protect dsRNA against nucleolytic degradation and facilitate efficient internalization into the target cells of lepidopteran larvae, mainly the midgut epithelium (DeSchutter et al., 2021). Interestingly, Parsons et al. (2018) proposed the use of synthetic polymer mimics of cell-penetrating peptides to allow efficient internalization into S. frugiperda midgut epithelial cells. Generally, silencing the key genes associated with various functions of gut cells in S. frugiperda through RNAi is the core for pyramiding and silencing several genes and thus could be an effective way of managing this pest. Moreover, the usage of potential candidate co-formulants of dsRNA for efficient delivery as well as deployment of an integrated tactic could provide several opportunities for the investigation of successful and reproducible environmental RNAi in S. frugiperda.
Despite the prominent potential of RNAi in genomic studies and the management of agriculturally important insect pests (Cruz et al., 2018; Davis-Vogel et al., 2018; Kunte et al., 2020), the instability of RNAi effectiveness among insects limits its application in pest management (Davis-Vogel et al., 2018; Chen et al., 2021). Different factors have been noted to determine the efficiency of RNAi in the management of lepidopteran insect pests, including S. frugiperda. An overview of some of the factors affecting RNAi efficiency in S. frugiperda is given below (Table 2). RNAi machinery genes, dsRNA uptake and systemic RNAi, delivery methods (Figure 3 presents the case of feeding and injection), RNAi effect variation related to the target gene, dsRNA persistence in the insect body, resistance to dsRNA and differences among insect populations/lineages are the major factors involved in RNAi efficiency (Cooper et al., 2019; Liu et al., 2020; Xiao-Shuang et al., 2021). Hence, validation of RNAi-based management technology for each insect development stage and core machinery gene is mandatory before its usage in large-scale farms to achieve successful management of S. frugiperda.
FIGURE 3. Variable RNAi response of insects associated with feeding and injection as dsRNA delivery methods, adapted from Song et al. (2018)
RNAi efficiency in lepidoptera, including S. frugiperda, shows high variability, and it is difficult to attain the required level of effectiveness, as in some cases, the increased instability and reduced intracellular transport of dsRNA are challenging factors for RNAi efficacy (Shukla et al., 2016; Peng et al., 2019; Rodríguez-de la Noval et al., 2019; Yang et al., 2019). Similarly, Ruo-Bing et al. (2018) documented a gene called up56, which is upregulated in reaction to dsRNA in lepidoptera. It encodes a nuclease that complements RNAi insensitivity in this group of insects.
By injecting dsRNA into plant vessels or providing it through roots to phytophagous insects (Figure 3), who naturally consume dsRNA through sucking or chewing, dsRNA could be delivered to these insects (Andrade and Hunter, 2016). Furthermore, Hunter et al. (2012) confirmed the translocation of dsRNA supplied exogenously to citrus plants through the vascular system of the plant, which can be taken up by certain xylem/phloem-feeding insect pests of citrus.
While the pH of an insect’s hemolymph ranges from 6.4 to 7.5, the pH of its gut lumen varies greatly (Harrison, 2001). Hindgut pH ranges from neutral to acidic, and crop pH is often acidic (Harrison, 2001). Lepidopterans have extremely alkaline midguts (pH > 8.0), while coleopterans and hemipterans have slightly acidic midguts. Dipterans, hymenopterans, and orthopterans typically have alkaline midguts (Harrison, 2001; Swevers et al., 2013). However, alkaline environments in the guts of dipterans, hymenopterans, and orthopterans may also reduce RNAi efficacy by hydrolyzing dsRNA. The varied alkaline environment of the lepidopteran gut is particularly worrisome for dsRNA stability. Several gut environmental factors influence the effectiveness of oral RNAi in insects by directly impacting dsRNA stability and indirectly influencing the activity of nucleases in the gut (Cooper et al., 2018). This shows that variations in gut pH affect dsRNase activity and may also cause dsRNA hydrolysis, which in turn contributes to low RNAi efficiency (Cooper et al., 2019). In insects with dsRNA stability concerns, additives that change the pH or enzymatic activity in the gut or saliva may also be beneficial for improving RNAi effectiveness.
Similarly, dsRNases clearly define why the RNAi response is limited in Spodopteran species, including S. frugiperda (Guan et al., 2018; Peng et al., 2019; Yao et al., 2022). Degradation of dsRNA by dsRNases is thought to contribute to the variability in RNAi efficiency observed among insects (Peng et al., 2019; Wang et al., 2019). The level of dsRNases expression determines the extent of dsRNA degradation and/or RNAi efficiency in spodopteran species (Guan et al., 2018; Peng et al., 2019). In other words, the extent of dsRNA expression can be influenced by various factors, including the types of dsRNases, insect growth stage, tissues, target genes, feed, and dsRNA delivery methods (Peng et al., 2019; Yao et al., 2022). For instance, the level of expression of the same or different dsRNases varies across the growth stage or larval instars of Spodoptera litura, showing the variable level of dsRNases in response to the changes in the growth stage (larval instars) of insects. Accordingly, the mRNA levels of three midgut-specific dsRNases were amplified during the larval stage, and the maximum dsRNA-degrading action was noticed in third-instar larvae (Peng et al., 2019). Moreover, the authors recognized six genes, namely, dsRNase1, dsRNase2, EndoG, dsRNase3, dsRNase4, and dsRNase5, from S. litura. Recent studies proved that multiple dsRNases encoded by the lepidopteran genome could contribute to the lower and inconsistent RNAi efficiency in this insect group in a broad sense (Ruo-Bing et al., 2018; Peng et al., 2019; Yao et al., 2022). Interestingly, Yao et al. (2022) demonstrated the involvement of multiple dsRNases in exogenous dsRNA degradation in S. frugiperda larvae. This evidence provides a significant implication in the manipulation of dsRNases and/or influencing factors to reduce dsRNA degradation and increase the RNAi efficiency against S. frugiperda. Therefore, further genomic studies should focus examining all aspects of dsRNases, including the mechanism of action in S. frugiperda, to lessen the ongoing challenges in dsRNA degradation in the deployment of RNAi. In addition, the success of RNAi in S. frugiperda differs among host stage, target gene and tissue or organ as well as the amount of dsRNA (Scott et al., 2013; Ren et al., 2019; Xiao-Shuang et al., 2021) (Tables 2).
Efforts have been made to increase the efficiency of RNAi, and a recent study indicated that chitosan nanoparticles and the Cellfectin II (CFII) transfection reagent helped dsRNA escape from endosomes and increased the RNAi response in S. frugiperda (Ana et al., 2020; Gurusamy et al., 2020b). The protamine-lipid-dsRNA nanoparticles, according to a more recent study by Dhandapani et al. (2022), increase RNAi efficiency in the target insect species S. frugiperda by shielding dsRNA from nuclease degradation and thereby significantly increasing the stability of dsRNA in the organism. Similarly, according to McGraw et al. (2022), clathrin-mediated endocytosis and macropinocytosis account for the majority of cellular uptake, and once inside the midgut, transcytosis is involved in moving branched amphipathic peptide nanocapsules (BAPCs) dsRNA from the lumen to the hemolymph of S. frugiperda. RNAi bioinsecticide may not last long in the environment, so extra effort is needed to perform the spraying operation at the right time, which in turn challenges its adoption by growers (Pedigo and Rice, 2009). In other words, the concentration at which the RNAi spray will be effective bears the burden of both cost and field efficacy (Pedigo and Rice, 2009; Baum and Roberts, 2014). In contrast, Baum and Roberts (2014) suggest a tropical application such as nanoparticles to enhance the efficiency of RNAi-based pest management strategies for S. frugiperda. Generally, delivery of dsRNA to this insect (Tables 1 and 2) has been documented by different techniques, including injection, feeding on dsRNA synthesized in vitro or produced by bacteria or transgenic crops, soaking, or produced in viral and bacterial vectors (Christiaens et al., 2018b), and the effectiveness of RNAi in S. frugiperda varies, mainly due to the insect status, delivery method, gene or tissue of interest, and refractoriness to dsRNA (Ruo-Bing et al., 2018; Cooper et al., 2019; Chen et al., 2021; Mohammad and Youngjin, 2022). However, further study is compulsory to identify all the possible limiting factors of the RNAi approach and the underlying mechanisms in this insect, in addition to the assessment of potential safety risks.
Lepidopterans are generally refractory to effective gene silencing by RNAi, especially when using oral delivery of dsRNA (Dias et al., 2020), and this is notably associated with the inherited superior biological features in the case of S. frugiperda, which boost its adaptation against most of the utilized options, especially when they are applied in a single fashion, recognizing its direct and indirect effects in the case of RNAi as an emerging approach. Despite limiting factors, as an efficient tool in gene function, RNAi enhances susceptibility to insect pests (Xiong et al., 2016; Wang et al., 2017; Mehlhorn et al., 2021) and thus could be considered a promising component in the development of integrated management against S. frugiperda. In other words, promising options have been provided to overcome the challenges associated with RNAi applications in lepidopteran S. frugiperda. For example, the use of modified or concatemerized dsRNAs has been suggested as a strategy to improve RNAi efficiency (Christiaens et al., 2018a; Sharath et al., 2018; Vélez and Fishilevich, 2018; Ren et al., 2019; Ana et al., 2020; Gurusamy et al., 2020b; Chen et al., 2021; Xiao-Shuang et al., 2021). Presently, many successes in ds/siRNA delivery systems have been proven to contribute to the improvement of RNAi efficiency in S. frugiperda, inviting further investigations to achieve a required level of management against the pest. Sprayable dsRNA based bioinsecticide is being reported with successful efficiency under field conditions against Leptinotarsa decemlineata (Rodriguez et al., 2021), and this explains great possibilities for use in the case of S. frugiperda. Baum and Roberts (2014) alluded to a tropical application for RNAi-based pest management strategies to protect ds/siRNAs from the environment to control S. frugiperda. Accordingly, nanoparticles of siRNAs would be a likely vector for any tropical application (Ana et al., 2020; Shuo et al., 2020); pheromone bait traps are a sprayable RNAi product (Baum and Roberts, 2014). The establishment of transgenic S. frugiperda expressing Caenorhabditis elegans systemic RNA interference defective protein 1 (CeSid1) provided possibilities for employing CeSid1 to enhance RNAi efficiency in insects that show variable and inefficient RNAi (Chen et al., 2021). Moreover, key genes associated with the development, detoxification, digestion and defense of the midgut have been identified as ideal targets for RNAi in S. frugiperda (Rodríguez-de la Noval et al., 2019), calling for future investigations to focus on these genes to enhance RNAi effectiveness. According to Rodríguez-dela Noval et al. (2019)and Tabashnik and Carrière (2017), the efficacy of RNAi could be significantly improved by pyramiding and expressing dsRNAs of these key genes targeted to the midgut of S. frugiperda. Likewise, the utilization of protein carriers with specific properties such as lectin and peptides, has been confirmed to provide protection for dsRNA and ensure RNAi efficiency (Parsons et al., 2018; DeSchutter et al., 2021). Knockdown of the expression of REase or dsRNases can enhance on RNAi efficiency and thus represents a target for further research of RNAi efficiency in S. frugiperda (Ruo-Bing et al., 2018; Peng et al., 2019). In addition, the study should focus on the appropriate identification of all possible causes of inefficient RNAi in S. frugiperda to enhance its efficiency for sustainable management of the pest.
Application of the RNAi approach in combination with novel biological options that have a different mode of action can provide effective management of S. frugiperda thereby improving the efficacy of RNAi-based technology (Griebler et al., 2008; Baum and Roberts, 2014; Ren et al., 2019). According to Jonathan (2015), RNAi is considered a plausible component of IPM in controlling S. frugiperda. Interestingly, push-pull technology (PPT) and botanical insecticides are among potential candidates to be deployed with RNAi in an integrated fashion. Currently, many studies have shown that the application of bioformulations of botanical materials (Jaoko et al., 2021) and the emission of chemical compounds by PPT (Khan et al., 2018; Midega et al., 2018; Mutyambai et al., 2019) provide promising results against S. frugiperda across diverse agro-ecologies, particularly in the African context. For instance, botanical extracts from Melia volkensii L, Azadirachta indica Juss, Schinnus mol L and Phytolacca dodecandra L. displayed antifeedant activity with larval mortality of more than 90% (Sisay et al., 2019; Jaoko et al., 2021). As a versatile option, PPT improves the aboveground and belowground plant environment to enhance the plant while making it less suitable for S. frugiperda (Khan et al., 2018; Sobhy et al., 2022) and possesses a convincing trait to act with RNAi. In other words, the importance of combining different control strategies is signified to improve the insecticidal activity of bio-options and prevent pest resistance emergence (Wang et al., 2017; Jaoko et al., 2021). Surprisingly, RNAi offers an exceptional mode of action among bioinsecticides through the mechanism of gene suppression and therefore can supplement the present methods used for pest control (Griebler et al., 2008; Tabashnik and Carrière, 2017; Mehlhorn et al., 2021). The deployment of RNAi with these candidate options does not enhance only enhances its efficiency but also provides a great contribution to ensuring the development of the IPM program. However, research evidence is lacking concerning the combined application and/or effect of these biological options to optimize their integration for better bioactivity against S. frugiperda. Hence, research emphasis is needed to determine the optimum combinations of RNAi with these options across diverse ecologies to boost their biological activity against the pest and verify their agronomic suitability.
In general, since endogenously generated and exogenously supplied dsRNAs activate RNAi in a sequence-specific manner, this strategy is a promising avenue for providing low-risk and environmentally safe plant protection (Dias et al., 2018; Hernández-Soto and Chacón-Cerdas, 2021). There is plenty of evidence of its use through host-induced gene silencing techniques to control insects (Shuo et al., 2020; Hernández-Soto and Chacón-Cerdas, 2021). No single protocol can be applied to every gene in every insect and the target tissue (Mehlhorn et al., 2021). Thus, the adaptability of RNAi methodology to control a particular pest must be carefully evaluated before deployment to maximize efficacy and sustainability (Davis-Vogel et al., 2018). The environmental risk assessment of RNAi-based genetically modified plants and new strategies, including direct spraying of dsRNA bioinsecticide should be particularly defined according to the objectives of each RNAi bioassay and experimental setup and are crucial for appropriate insect RNAi research as well as for commercial success (Baum and Roberts, 2014; Christiaens et al., 2018b; Liu et al., 2020; DeSchutter et al., 2021).
RNAi-based technologies have tremendous potential as an effective and noninvasive approach for invasive insect pest management in modern agricultural systems. Most studies have reported promising achievements in RNAi application to control S. frugiperda. Despite increasing trends in RNAi application in S. frugiperda, it is not as straightforward as that observed for other insect species. Generally, lepidopterans, including S. frugiperda, are refractory to effective gene silencing by RNAi, especially when using oral delivery of dsRNA. Various factors could contribute to the efficiency of RNAi technologies to achieve effective management of insect pests. On the other hand, strategies such as the use of modified or concatenated dsRNAs have been used to improve the efficiency of RNAi in S. frugiperda. Moreover, the emergence of alternatives combining RNAi gene silencing with the induction of resistance in crops or multiple silencing is an opportunity to overcome the ongoing challenges in managing S. frugiperda, an invasive insect pest in agriculture. Hence, integration of this technology with any other compatible options is plausible in devising integrated pest management strategies. In addition, further research should be emphasized for a better understanding of RNAi-cellular interactions and insect gene silencing mechanisms as well as other influencing factors to enhance the efficiency of RNAi for the effective management of S. frugiperda. Further studies should also focus on the assessment of risk associated with RNAi-based insect pest management approaches.
TF and MK prepared the review skeleton and drafted it. TF and MK read and approved the final manuscript. All authors have read and approved the manuscript.
The authors declare that the research was conducted in the absence of any commercial or financial relationships that could be construed as a potential conflict of interest.
All claims expressed in this article are solely those of the authors and do not necessarily represent those of their affiliated organizations, or those of the publisher, the editors and the reviewers. Any product that may be evaluated in this article, or claim that may be made by its manufacturer, is not guaranteed or endorsed by the publisher.
Abdel-latief, M. A., and Hoffmann, K. H. (2014). Functional Activity of Allatotropin and Allatostatin in the Pupal Stage of a Holometablous Insect, Tribolium castaneum (Coleoptera, Tenebrionidae). Peptides 53, 172–184. doi:10.1016/j.peptides.2013.10.007
Ali, M. W., Zhang, Z., Xia, S., and Zhang, H. (2017). Biofunctional analysis of Vitellogenin and Vitellogenin receptor in citrus red mites, Panonychus citri by RNA interference. Sci. Rep. 7, 16123. doi:10.1038/s41598-017-16331-3
Ana, T., Yosra, A. H., Gireesh, R., and Andy, M. (2020). Optimizing RNA Interference in Fall Armyworm, Spodoptera Frugiperda Using Improved Nanoparticles. The Ohio State University.
Andrade, C. E., and Hunter, W. B. (2016). “RNA Interference – Natural Gene-Based Technology for Highly Specific Pest Control (HiSPeC),” in RNA Interference. Editor I. Y. Abdurakhmonov (Croatia: InTech), 391–409.
Andrew, F., Ståle, E., Anna, W., and Hee-Chan, S. (2001). RNA Interference: Mechanisms and Applications. Biotechnol. Annu. Rev. 7, 31–57. doi:10.1016/s1387-2656(01)07032-6
Assefa, F., and Ayalew, D. (2019). Status and Control Measures of Fall Armyworm (Spodoptera Frugiperda) Infestations in Maize Fields in Ethiopia: A Review. Cogent Food Agric. 5 (1), 1641902–1641916. doi:10.1080/23311932.2019.1641902
Bai-Zhong, Z., Xu, S., Cong-Ai, Z., Liu-Yang, L., Ya-She, L., Xing, G., et al. (2020). Silencing of Cytochrome P450 in Spodoptera Frugiperda (Lepidoptera: Noctuidae) by RNA Interference Enhances Susceptibility to Chlorantraniliprole. J. Insect Sci. 20 (3), 12. doi:10.1093/jisesa/ieaa047
Baum, J. A., and Roberts, J. K. (2014). Progress toward RNAi-Mediated Insect Pest Management. Adv. Insect Physiology 47, 249–295.
Bettencourt, R., Terenius, O., and Faye, I. (2002). Hemolin Gene Silencing by Ds-RNA Injected into Cecropia Pupae Is Lethal to Next Generation Embryos. Insect Mol. Biol. 11, 267–271. doi:10.1046/j.1365-2583.2002.00334.x
Busato, G. R., Grützmacher, A. D., Garcia, M. S., Giolo, F. P., Zotti, M. J., and Bandeira, J. M. (2005). Exigências térmicas e estimative Do número de gerações dos biótipos “milho” e “arroz” de Spodoptera frugiperda. Pesq. Agropec. Bras. 40, 329–335. doi:10.1590/s0100-204x2005000400003
Capinera, J. L., and Smith, J. E. (1999). “Fall Armyworm, Spodoptera Frugiperda,” in Insecta: Lepidoptera: Noctuidae (Gainesville: University of Florida IFAS extension publication EENY-098).
Chan, G. G., Koch, C. M., and Connors, L. H. (2017). Blood Proteomic Profiling in Inherited (ATTRm) and Acquired (ATTRwt) Forms of Transthyretin-Associated Cardiac Amyloidosis. J. Proteome Res. 16 (4), 1659–1668. doi:10.1021/acs.jproteome.6b00998
Chen, X., Koo, J., Gurusamy, D., Mogilicherla, K., and Reddy Palli, S. (2021). Caenorhabditis elegans Systemic RNA Interference Defective Protein 1 Enhances RNAi Efficiency in a Lepidopteran Insect, the Fall Armyworm, in a Tissue-specific Manner. RNA Biol. 18 (9), 1291–1299. doi:10.1080/15476286.2020.1842632
Christiaens, O., Swevers, L, and Smagghe, G. (2014). DsRNA degradation in the pea aphid (Acyrthosiphon pisum) associated with lack of response in RNAi feeding and injection assay. Peptides 53, 307–314. doi:10.1016/j.peptides.2013.12.014
Christiaens, O., Tardajos, M., Martinez, R., Dash, M., Dubruel, P., and Smagghe, G. (2018a). Increased RNAi Efficacy in Spodoptera Exigua via the Formulation of dsRNA with Guanylated Polymers. Front. Physiol. 9, 316. doi:10.3389/fphys.2018.00316
Christiaens, O., Dzhambazova, T., Kostov, K., Arpaia, S., Joga, M., Urru, I., et al. (2018b). Literature Review of Baseline Information on RNAi to Support the Environmental Risk Assessment of RNAi-Based GM Plants. EFSA Support. Publ. 1424, 1–173. doi:10.2903/sp.efsa.2018.EN-1424
Cock, M. J. W., Beseh, P. K., Buddie, A. G., Cafá, G., and Crozier, J. (2017). Molecular Methods to Detect Spodoptera Frugiperda in Ghana, and Implications for Monitoring the Spread of Invasive Species in Developing Countries. Sci. Rep. 7 (1), 4103. doi:10.1038/s41598-017-04238-y
Cooper, A. M., Silver, K., Zhang, J., Park, Y., and Zhu, K. Y. (2019). Molecular Mechanisms Influencing Efficiency of RNA Interference in Insects. Pest Manag. Sci. 75, 18–28. doi:10.1002/ps.5126
Cruz, C., Tayler, A., and Whyard, S. (2018). RNA Interference-Mediated Knockdown of Male Fertility Genes in the Queensland Fruit Fly Bactrocera Tryoni (Diptera: Tephritidae). Insects 9, 96. doi:10.3390/insects9030096
Davis-Vogel, C., Ortiz, A., Procyk, L., Robeson, J., Kassa, A., Wang, Y., et al. (2018). Knockdown of RNA Interference Pathway Genes Impacts the Fitness of Western Corn Rootworm. Sci. Rep. 8, 7858. doi:10.1038/s41598-018-26129-6
Day, R., Abrahams, P., Bateman, M., Beale, T., Clottey, V., Cock, M., et al. (2017). Fall Armyworm: Impacts and Implications for Africa. outlook. pest Man. 28 (5), 196–201. doi:10.1564/v28_oct_02
DeSchutter, K., Christiaens, O., Taning, C. N. J., and Smagghe, G. (2021). “Boosting dsRNA Delivery in Plant and Insect Cells with Peptide- and Polymer Based Carriers: Case-Based Current Status and Future Perspectives,” in RNAi for Plant Improvement and Protection. Editor B. Mezzettiet al. (Wallingford, CT: CAB International), 102–116. doi:10.1079/9781789248890.0011
Dhandapani, R, K ., Gurusamy, Dh., and Palli, S. R. (2022). Protamine-Lipid-dsRNA Nanoparticles Improve RNAi Efficiency in the Fall Armyworm, Spodoptera Frugiperda. J. Agric. Food Chem. 70 (22), 6634–6643. doi:10.1021/acs.jafc.2c00901
Dias, N., Agliari, D. C., Do-Santos, E. A., Magghe, G., Jurat-Fuentes, J., Mishra, S., et al. (2020). Insecticidal Gene Silencing by RNAi in the Neotropical Region. Neotrop. Entomol. 49, 1–11. doi:10.1007/s13744-019-00722-4
Dowling, D., Pauli, T., Donath, A., Meusemann, K., Podsiadlowski, L., Petersen, M., et al. (2016). Phylogenetic Origin and Diversification of RNAi Pathway Genes in Insects. Genome Biol. Evol. 8 (12), 3784–3793. doi:10.1093/gbe/evw281
Du -Plessis, H., Schlemmer, M. L., and Van den Berg, (2020). J. Te Efect of Temperature on the Development of Spodoptera Frugiperda (Lepidoptera: Noctuidae). Insects 11, 228. doi:10.3390/insects11040228
FAO (2019). Community-based Fall Armyworm Monitoring, Early Warning and Management: Training of Trainers Manual. Available from: http://www.fao.org/3/ca2924en/CA2924EN.pdf.
Feinberg, E. H., and Hunter, C. P. (2003). Transport of dsRNA into Cells by the Transmembrane Protein SID-1. Science 301, 1545–1547. doi:10.1126/science.1087117
Fire, A., Xu, S., Montgomery, M. K., Kostas, S. A., Driver, S. E., and Mello, C. (1998). Potent and Specific Genetic Interference by Double-Stranded RNA in Caenorhabditis elegans. Nature 391, 806–811. doi:10.1038/35888
Gichuhi, J., Sevgan, S., Khamis, F., Van-den Berg, J., Plessis, H., Ekesi, S., et al. (2020). Diversity of Fall Armyworm, Spodoptera Frugiperda and Their Gut Bacterial Community in Kenya. PeerJ 8, e8701. doi:10.7717/peerj.8701
Gimenez, S., Abdelgaffar, H., Goff, L., Hilliou, F., Blanco, C. A., Hanniger, S., et al. (2020). Adaptation by Copy Number Variation Increases Insecticide Resistance in the Fall Armyworm. Commun. Biol. 3, 664. doi:10.1038/s42003-020-01382-6
Goergen, G., Kumar, P. L., Sankung, S. B., Togola, A., and Tamò, M. (2017). First Report of Outbreaks of the Fall Armyworm Spodoptera Frugiperda (J E Smith) (Lepidoptera, Noctuidae), a New Alien Invasive Pest in West and Central Africa. PLoS One 11 (10), e0165632–9. doi:10.1371/journal.pone.0165632
Gordon, K., and Waterhouse, P. (2006). Small RNA Viruses of Insects: Expression in Plants and RNA Silencing. Adv. Virus Res. 68, 459–502. doi:10.1016/S0065-3527(06)68013-5
Gregoriou, M. E., Reczko, M., Kakani, E. G., Tsoumani, K. T., and Mathiopoulos, K. D. (2021). Decoding the Reproductive System of the Olive Fruit Fly, Bactrocera Oleae. Genes. 12, 355. doi:10.3390/genes12030355
Griebler, M., Westerlund, S. A., Hoffmann, K. H., and Meyerring-Vos, M. (2008). RNA Interference with the Allatoregulating Neuropeptide Genes from the Fall Armyworm Spodoptera Frugiperda and its Effects on the JH Titer in the Hemolymph. J. Insect Physiol. 54, 997–1007. doi:10.1016/j.jinsphys.2008.04.019
Guan, R. B., Li, H. C., Fan, Y. J., Hu, S. R., Christiaens, O., Smagghe, G., et al. (2018). A Nuclease Specific to Lepidopteran Insects Suppresses RNAi. J. Biol. Chem. 293 (16), 6011–6021. doi:10.1074/jbc.RA117.001553
Gui, F. R., Lan, T. M., Zhao, Y., Guo, W., Dong, Y., Fang, D. M., et al. (2020). Genomic and Transcriptomic Analysis Unveils Population Evolution and Development of Pesticide Resistance in Fall Armyworm Spodoptera Frugiperda. Protein Cell. 13, 513–531. doi:10.1007/s13238-020-00795-7
Guo, Z., Kang, S., Zhu, X., Xia, J., Wu, Q., Wang, S., et al. (2015). The Novel ABC Transporter ABCH1 Is a Potential Target for RNAi-Based Insect Pest Control and Resistance Management. Sci. Rep. 5, 13728. doi:10.1038/srep13728
Gurusamy, D., Mogilicherla, K., and Palli, S. R. (2020a). Chitosan Nanoparticles Help Double‐stranded RNA Escape from Endosomes and Improve RNA Interference in the Fall Armyworm, Spodoptera Frugiperda. Arch. Insect Biochem. Physiol. 104 (4), e21677. doi:10.1002/arch.21677
Gurusamy, D., Mogilicherla, K., Shukla, J. N., and Palli, S. R. (2020b). Lipids Help Double‐stranded RNA in Endosomal Escape and Improve RNA Interference in the Fall Armyworm, Spodoptera Frugiperda. Arch. Insect Biochem. Physiol. 104 (4), e21678. doi:10.1002/arch.21678
Han, B. W., Wang, W., Li, C., Weng, Z., and Zamore, P. D. (2015). Noncoding RNA. piRNA-Guided Transposon Cleavage Initiates Zucchini-dependent, Phased piRNA Production. Science 348 (6236), 817–821. doi:10.1126/science.aaa1264
Harrison, J. F. (2001). Insect Acid-Base Physiology. Annu. Rev. Entomol. 46, 221–250. doi:10.1146/annurev.ento.46.1.221
Hassanien, I. T. E., Meyering-Vos, M., and Hoffmann, K. H. (2014). RNA Interference Reveals Allatotropin Functioning in Larvae and Adults of Spodoptera Frugiperda (Lepidoptera, Noctuidae). Entomologia 2 (1). doi:10.4081/entomologia.2014.169
Hernández-Soto, A., and Chacón-Cerdas, R. (2021). RNAi Crop Protection Advances. Int. J. Mol. Sci. 22, 12148. doi:10.3390/ijms222212148
Hu, Z., Lin, Q., Chen, H., Li, Z., Yin, F., and Feng, X. (2014). Identification of a novel cytochrome P450 gene, CYP321E1 from the diamondback moth, Plutella xylostella (L.) and RNA interference to evaluate its role in Chlorantraniliprole resistance. Bull. Entomol. Res. 104(6), 716–723. doi:10.1017/s0007485314000510
Huang, C., Li, Y. Z., Yang, N. W., Wu, Q., Xing, L. S., Qian, W. Q., et al. (2019). Progresses in Invasive Insect Genomics. Plant Prot. 45, 112–120.
Hunter, W. B., Glick, E., Paldi, N., and Bextine, B. R. (2012). Advances in RNA Interference: dsRNA Treatment in Trees and Grapevines for Insect Pest Suppression. Southwest. Entomol. 37, 85–87. doi:10.3958/059.037.0110
Huvenne, H., and Smagghe, G. (2010). Mechanisms of dsRNA Uptake in Insects and Potential of RNAi for Pest Control: A Review. J. Insect Physiol. 56, 227–235. doi:10.1016/j.jinsphys.2009.10.004
Jaoko, V., Taning, C., Backx, S., Motti, P., Mulatya, J., Vandenabeele, J., et al. (2021). Laboratory and Greenhouse Evaluation of Melia Volkensii Extracts for Potency Agains T African Sweet Potato Weevil, Cylas Puncticollis, and Fall Armyworm, Spodoptera Frugiperda. Agronomy 11, 1994. doi:10.3390/agronomy11101994
Jing-Wan, C., Cong, H., Chang-you, L., Hong-xu, Z., Yong-lin, R., Zai-yuan, L., et al. (2021). Biology, Invasion and Management of the Agricultural Invader: Fall Armyworm, Spodoptera Frugiperda (Lepidoptera: Noctuidae). J. Integr. Agric. 20 (3), 646–663. doi:10.1016/s2095-3119(20)63367-6
Joga, M. R., Zotti, M. J., Smagghe, G., and Christiaens, O. (2016). RNAi Efficiency, Systemic Properties, and Novel Delivery Methods for Pest Insect Control: What We Know So Far. Front. Physiol. 7, 553. doi:10.3389/fphys.2016.00553
Johnson, S. J. (1987). Migration and the Life History Strategy of the Fall Armyworm, Spodoptera Frugiperda in the Western Hemisphere. Int. J. Trop. Insect Sci. 8 (4-5-6), 543–549. doi:10.1017/S1742758400022591
Jonathan, W. R. (2015). Fall Armyworm, Spodoptera Frugiperda Control by RNAi as a Plausible Component to Integrated Pest Management Practices Plausible Component to Integrated Pest Management Practices. Nebraska – Lincoln: University of Nebraska. https://digitalcommons.unl.edu/entodistmasters.
Kelkenberg, M., Odman-Naresh, J., Muthukrishnan, S., and Merzendorfer, H. (2015). Chitin Is a Necessary Component to Maintain the Barrier Function of the Peritrophic Matrix in the Insect Midgut. Insect biochem. Mol. Biol. 56, 21–28. doi:10.1016/j.ibmb.2014.11.005
Khan, Z., Pittchara, J., Midegaa, C., and Pickettb, J. (2018). Push-pull Farming System Controls Fall Armyworm: Lessons from Africa. outlook. pest Man. 29, 220–224. doi:10.1564/v29_oct_09
Kourti, A., Swevers, L., and Kontogiannatos, D. (2017). “Search of New Methodologies for Efficient Insect Pest Control: “The RNAi “Movement,” in Biological Control of Pest and Vector Insects. Editor V. D. C. Shields (London, United Kingdom: Intech Open), 71–95. doi:10.5772/66633
Kunte, N., McGraw, E., Bell, S., Held, D., and Avila, L. A. (2020). Prospects, Challenges and Current Status of RNAi through Insect Feeding. Pest Manag. Sci. 76 (1), 26–41. doi:10.1002/ps.5588
Laisney, J., Gurusamy, D., Baddar, Z. E., Palli, S. R., and Unrine, J. M. (2020). RNAi in Spodoptera Frugiperda Sf9 Cells via Nanomaterial Mediated Delivery of dsRNA: a Comparison of Poly-L-Arginine Polyplexes and Poly-L-Arginine-Functionalized Au Nanoparticles. ACS Appl. Mat. Interfaces 12 (23), 25645–25657. doi:10.1021/acsami.0c06234
Li, Z., and Blissard, G. W. (2012). Cellular VPS4 Is Required for Efficient Entry and Egress of Budded Virions of Autographa Californica Multiple Nucleopolyhedrovirus. J. Virol. 86 (1), 459–472. doi:10.1128/jvi.06049-11
Lin, Y., Huang, J., Xavier, B., Yun, L., and Leea, H. (2016). Oral Delivery of dsRNA Lipoplexes to German Cockroach Protects dsRNA from Degradation and Induces RNAi Response. Pest Manag. Sci. 73, 960–966. doi:10.1002/ps.4407
Liu, S., Jaouannet, M., Dempsey, D., Imani, J., Coustau, C., and Kogel, K. (2020). RNA-based technologies for insect control in plant production. Biotechnol. Adv. 39, 107463.
Maktura, G. C., Paranhos, B. J., and Marques-Souza, H. (2021). RNAi in Fruit Flies (Diptera: Tephritidae): Successes and Challenges. J. Appl. Entomol. 00, 740–756. doi:10.1111/jen.12905
Martinez, Z., De-Schutter, K., Van-Damme, E., Vogel, E., Wynant, N., Vanden-Broeck, J., et al. (2021). Accelerated Delivery of dsRNA in Lepidopteran Midgut Cells by a Galanthus Nivalis Lectin (GNA)-dsRNA-binding Domain Fusion Protein. Pestic. Biochem. Physiol. 175, 104853. doi:10.1016/j.pestbp.2021.104853
McGraw, E., Roberts, J, D., Nitish, K., Matthew, W., Xavier, S., David, H., et al. (2022). Insight into Cellular Uptake and Transcytosis of Peptide Nanoparticles in Spodoptera Frugiperda Cells and Isolated Midgut. ACS Omega 7 (13), 10933–10943. doi:10.1021/acsomega.1c06638
Mehlhorn, S., Vera, S. H., Sven, G., Ralf, N., and Gregor, B. (2021). Establishing RNAi for Basic Research and Pest Control and Identification of the Most Efficient Target Genes for Pest Control: a Brief Guide. Front. Zool. 18, 60. doi:10.1186/s12983-021-00444-7
Michael, H., Komi, K., Juliet, A., Barnabas, M., Allan, T., Anani, Y., et al. (2021). Managing a Transboundary Pest: The Fall Armyworm on Maize in Africa. London, United Kingdom: Intech Open. doi:10.5772/intechopen.96637
Mohammad, V., and Youngjin, P. (2022). Cold tolerance strategies of the fall armyworm, Spodoptera frugiperda (Smith) (Lepidoptera: Noctuidae). Sci. Rep., 12, 4129. doi:10.1038/s41598-022-08174-4
Mon, H., Kobayashi, I., Ohkubo, S., Tomita, S., Lee, J., Sezutsu, H., et al. (2012). Effective RNA Interference in Cultured Silkworm Cells Mediated by Overexpression of Caenorhabditis elegans SID-1. RNA Biol. 9, 40–46. doi:10.4161/rna.9.1.18084
Montezano, D. G., Sosa-Gomez, D. R., Roque-Specht, V. F., Sousa-Silva, J., Paula-Moraes, S., et al. (2018). Host Plants of Spodoptera frugiperda (Lepidoptera: Noctuidae) in the Americas. Afr. Entomol. 26, 286–300. doi:10.4001/003.026.0286
Mutyambai, D., Bass, E., Luttermoser, T., Poveda, K., Midega, C., Khan, Z., et al. (2019). More Than “Push” and “Pull”? Plant-Soil Feedbacks of Maize Companion Cropping Increase Chemical Plant Defenses against Herbivores. Front. Ecol. Evol. 7, 217. doi:10.3389/fevo.2019.00217
Nagoshi, N., Goergen, G., Tounou, K., Agboka, K., Koffi, D., and Meagher, R. (2018). Analysis of Strain Distribution, Migratory Potential, and Invasion History of Fall Armyworm Populations in Northern Sub-saharan Africa. Sci. Rep. 8, 3710–10. doi:10.1038/s41598-018-21954-1
Nagoshi, R. N., Koffi, D., Agboka, K., Tounou, K. A., Banerjee, R., Jurat-Fuentes, J. L., et al. (2017). Comparative Molecular Analyses of Invasive Fall Armyworm in Togo Reveal Strong Similarities to Populations from the Eastern United States and the Greater Antilles. PLoS One 12 (7), e0181982–15. doi:10.1371/journal.pone.0181982
Nagoshi, R. N., Goergen, G., Du-Plessis, H., Van-Den, B. J., and Meagher, R. (2019). Genetic Comparisons of Fall Armyworm Populations from 11 Countries Spanning Sub-saharan Africa Provide Insights into Strain Composition and Migratory Behaviors. Sci. Rep. 9 (1), 8311. doi:10.1038/s41598-019-44744-9
Ni, M., Wei, M., Xiaofang, W., Meijing, G., Yan, D., Xiaoli, W., et al. (2017). Next-generation Transgenic Cotton: Pyramiding RNAi and Bt Counters Insect Resistance. Plant Biotechnol. J. 15, 1204–1213. doi:10.1111/pbi.12709
Olawale, S. A., Bushra, T., Idrees, A. N., Iqra, Y., Imtiaz, A. S., Khurram, S., et al. (2019). Identifcation and Validation of Potential Reference Gene for Effective dsRNA Knockdown Analysis in Chilo Partellus. Sci. Rep. 9, 13629. doi:10.1038/s41598-019-49810-w
Otim, M. H., Tay, W. T., Walsh, T. K., Kanyesigye, D., Adumo, S., Abongosi, J., et al. (2018). Detection of Sister-Species in Invasive Populations of the Fall Armyworm Spodoptera Frugiperda (Lepidoptera: Noctuidae) from Uganda. PLoS One 13 (4), e0194571–18. doi:10.1371/journal.pone.0194571
Park, Y., and Kim, Y. (2013). RNA Interference of Glycerol Biosynthesis Suppresses Rapid Cold Hardening of the Beet Armyworm, Spodoptera Exigua. J. Exp. Biol. 216, 4196–4203. doi:10.1242/jeb.092031
Park, Y., and Vatanparast, M. (2022). Suppression of PBAN Receptor Expression Reduces Fecundity in the Fall Armyworm, Spodoptera Frugiperda. Arch. Insect Biochem. Physiol. 110, e21897. doi:10.1002/arch.21897
Parsons, K. H., Mondal, M., McCormick, C. L., and Flynt, A. S. (2018). Guanidinium-Functionalized Interpolyelectrolyte Complexes Enabling RNAi in Resistant Insect Pests. Biomacromolecules 19, 1111–1117. doi:10.1021/acs.biomac.7b01717
Pashley, D. P. (1988). Current Status of Fall Armyworm Host Strains. Fla. Entomol. 71 (3), 227. doi:10.2307/3495425
Pedigo, L. P., and Rice, M. E. (2009). Entomology and Pest Management (6th Ed.). Long Grove, IL, USA: Waveland Press, Inc.
Peng, Y., Wang, K., Fu, W., Sheng, C, and Han, Z. (2018). Biochemical comparison of dsRNA degrading nucleases in four different insects. Front. Physiol. 9, 624. doi:10.3389/fphys.2018.00624
Peng, Y., Wang, K., Zhu, G., Han, Q., Chen, J., Elzaki, M. E. A., et al. (2019). Identification and Characterization of Multiple dsRNases from a Lepidopteran Insect, the Tobacco Cutworm, Spodoptera Litura (Lepidoptera: Noctuidae). Pestic. Biochem. Physiol. 162, 86–95. doi:10.1016/j.pestbp.2019.09.011
Pesch, Y. Y., Riedel, D., Patil, K. R., Loch, G., and Behr, M. (2016). Chitinases and imaginal disc growth factors organize the extracellular matrix formation at barrier tissues in insects. Sci. Rep. 6, 18340. doi:10.1038/srep18340
Prasanna, B., Huesing, J., Eddy, R., and Peschke, V. (2018). Fall Armyworm in Africa: A Guide for Integrated Pest Management. Wallingford: CAB International, 1–109.
Rajan, K., Amit, S., Neelam, T., and Larry, L. (2013). RNAi for Insect Control: Current Perspective and Future Challenges. Appl. Biochem. Biotechnol. 171, 847–873. doi:10.1007/s12010-013-0399-4
Ren, D., Cai, Z., Song, J., Wu, Z., and Zhou, S. (2014). dsRNA uptake and persistence account for tissue-dependent susceptibility to RNA interference in the migratory locust, Locusta migratoria, Insect Mol. Biol. 23, 175–184. doi:10.1111/imb.12074
Ren, M., Zafar, M. M., Mo, H., Yang, Z., and Li, F. (2019). Fighting against Fall Armyworm by Using Multiple Genes Pyramiding and Silencing (MGPS) Technology. Sci. China. Life Sci. 62, 1703–1706. doi:10.1007/s11427-019-1586-7
Rodriguez, T., Sridharan, K., Manley, B., Cunningham, D., and Narva, K. (2021). “Development of dsRNA as a sustainable bioinsecticide: from laboratory to field”, in Crop protection Products for Sustainable Agriculture, ACS Symposium Series. 1390 editors. B. M. Rauzan, and B. A. Lorsbach. Washington, DC: ACS Publications, 65–82.
Rodriguez-Cabrera, L., Trujillo-Bacallao, D., Borrás-Hidalgo, O., Wright, D. J., and Ayra-Pardo, C. (2010). RNAi-mediated Knockdown of a Spodoptera Frugiperda Trypsin-like Serine-Protease Gene Reduces Susceptibility to a Bacillus Thuringiensis Cry1Ca1 Protoxin. Environ. Microbiol. 12, 2894–2903. doi:10.1111/j.1462-2920.2010.02259.x
Rodríguez-de la Noval, C., Rodríguez-Cabrera, L., Izquierdo, L., Espinosa, L. A., Hernandez, D., Ponce, M., and Ayra-Pardo, C. (2019). Functional Expression of a Peritrophin A-like SfPER Protein Is Required for Larval Development in Spodoptera Frugiperda (Lepidoptera: Noctuidae). Sci. Rep. 9 (1), 1–14.
Rosa, C., Kuo, Y. W., Wuriyanghan, H., and Falk, B. W. (2018). RNA Interference Mechanisms and Applications in Plant Pathology. Annu. Rev. Phytopathol. 56, 581–610. doi:10.1146/annurev-phyto-080417-050044
Ruo-Bing, G., Hai-Chao, L., Yu-Jie, F., Shao-Ru, H., Olivier, C., Guy, S., et al. (2018). A Nuclease Specific to Lepidopteran Insects Suppresses RNAi. J. Biol. Chem. 293 (16), 6011–6021. doi:10.1074/jbc.RA117.001553
Scott, J. G., Michel, K., Bartholomay, L. C., Siegfried, B. D., Hunter, W. B., Smagghe, G., Zhu, K. Y, and Douglas, A. E. (2013). Towards the elements of successful insect RNAi. J. Insect Physiol. 59 (12), 1212–1221.
Sharanabasappa, M., Kalleshwaraswamy, M., Maruthi, S, and Pavithra, H. (2018). Biology of invasive fall armyworm Spodoptera frugiperda (J.E. Smith) (Lepidoptera: Noctuidae) on Maize. Indian J. Entomol. 80(3), 540–543. doi:10.5958/0974-8172.2018.00238.9
Sharath, C. G., Asokan, R., Manamohan, M., and Krishna, K. N. (2018). Enhancing RNAi by using concatemerized double-stranded RNA. Pest Manag. Sci.. 75(2), 506–514. ddoi:10.1002/ps.5149
Shen, Y., De Schutter, K., Walski, T., Van Damme, E., and Smagghe, G. (2017). Toxicity, Membrane Binding and Uptake of the Sclerotinia sclerotiorum Agglutinin (SSA) in Different Insect Cell Lines. Vitro Cell. Dev. Biol. Anim. 53 (8), 691–698. doi:10.1007/s11626-017-0176-8
Shetty, P., Gitau, M. M., and Maróti, G. (2019). Salinity Stress Responses and Adaptation Mechanisms in Eukaryotic Green Microalgae. Cells 8, 1657. doi:10.3390/cells8121657
Shu, Y. H., Wang, J. W., Lu, K., Zhou, J. L., Zhou, Q., and Zhang, G. R. (2011). The First Vitellogenin Receptor from a Lepidopteran Insect: Molecular Characterization, Expression Patterns and RNA Interference Analysis. Insect Mol. Biol. 20, 61–73. doi:10.1111/j.1365-2583.2010.01054.x
Shukla, J. N., Kalsi, M., Sethi, A., Narva, K. E., Fishilevich, E., Singh, S., et al. (2016). Reduced Stability and Intracellular Transport of dsRNA Contribute to Poor RNAi Response in Lepidopteran Insects. RNA Biol. 13 (7), 656–669. doi:10.1080/15476286.2016.1191728
Shuo, Y., Binyuan, R., Bo, Z., and Jie, S. (2020). Improving RNAi Efficiency for Pest Control in Crop Species. BioTechniques 68, 283–290. doi:10.2144/btn-2019-0171
Silva, D. M., Bueno, A. d. F., Andrade, K., Stecca, C. d. S., Neves, P. M. O. J., and Oliveira, M. C. N. d. (2017). Biology and Nutrition of Spodoptera Frugiperda (Lepidoptera: Noctuidae) Fed on Different Food Sources. Sci. Agric. 74, 18–31. doi:10.1590/1678-992x-2015-0160
Sisay, B., Simiyu, J., Mendesil, E., Likhayo, P., Ayalew, G., Mohamed, S., et al. (2019). Fall Armyworm, Spodoptera Frugiperda Infestations in East Africa: Assessment of Damage and Parasitism. Insects 10, 195. doi:10.3390/insects10070195
Sláma, K. (2013). Insect Hormones: More Than 50-years after the Discovery of Insect Juvenile Hormone Analogues (JHA, Juvenoids). Terr. Arthropod Rev. 6 (4), 257–333. doi:10.1163/18749836-06041073
Sobhy, I., Tamiru, A., Chiriboga, M., Nyagol, D., Cheruiyot, D., Chidawanyika, F., et al. (2022). Bioactive Volatiles from Push-Pull Companion Crops Repel Fall Armyworm and Attract its Parasitoids. Front. Ecol. Evol. 10, 883020. doi:10.3389/fevo.2022.883020
Song, H., Fan, Y., Zhang, J., Cooper, A. M., Silver, K., Li, D., et al. (2018). Contributions of dsRNases to Differential RNAi Efficiencies between the Injection and Oral Delivery of dsRNA in Locusta migratoria. Pest Manag. Sci. 75, 1707–1717. doi:10.1002/ps.5291
Swevers, L., Vanden Broeck, J., and Smagghe, G. (2013). The Possible Impact of Persistent Virus Infection on the Function of the RNAi Machinery in Insects: A Hypothesis. Front. Physiol. 4, 319. doi:10.3389/fphys.2013.00319
Tabashnik, B. E., Brevault, T., and Carriere, Y. (2013). Insect Resistance to Bt Crops: Lessons from the First Billion Acres. Nat. Biotechnol. 31, 510–521. doi:10.1038/nbt.2597
Tabashnik, B. E., and Carrière, Y. (2017). Surge in Insect Resistance to Transgenic Crops and Prospects for Sustainability. Nat. Biotechnol. 35 (10), 926–935. doi:10.1038/nbt.3974
Teal, P. E. (2002). Effects of Allatotropin and Allatostatin on In Vitro Production of Juvenile Hormones by the Corpora Allata of Virgin Females of the Moths of Heliothis virescens and Manduca Sexta. Peptides 23 (4), 663–669. doi:10.1016/s0196-9781(01)00660-x
Terenius, O., Papanicolaou, A., Garbutt, J. S., Eleftherianos, I., Huvenne, H., Kanginakudru, S., et al. (2011). RNA Interference in Lepidoptera: An Overview of Successful and Unsuccessful Studies and Implications for Experimental Design. J. Insect Physiol. 57, 231–245. doi:10.1016/j.jinsphys.2010.11.006
Tomari, Y., and Zamore, P. D. (2005). Perspective: Machines for RNAi. Genes. Dev. 19 (5), 517–529. doi:10.1101/gad.1284105
Toprak, U., Baldwin, D., Erlandson, M., Gillott, C., Harris, S., and Hegedus, D. D. (2013). In Vitro and In Vivo Application of RNA Interference for Targeting Genes Involved in Peritrophic Matrix Synthesis in a Lepidopteran System. Insect Sci. 20, 92–100. doi:10.1111/j.1744-7917.2012.01562.x
Ulvila, J., Parikka, M., Kleino, A., Sormunen, R., Ezekowitz, R. A., Kocks, C., et al. (2006). Double Stranded RNA Is Internalized by Scavenger Receptor-Mediated Endocytosis in Drosophila S2 Cells. J. Biol. Chem. 281, 14370–14375. doi:10.1074/jbc.M513868200
Uryu, O., Kamae, Y., Tomioka, K., and Yoshii, T. (2013). Long-term Effect of Systemic RNA Interference on Circadian Clock Genes in Hemimetabolous Insects. J. Insect Physiol. 59, 494–499. doi:10.1016/j.jinsphys.2013.02.009
Vatanparast, M., and Park, Y. (2022). Cold Tolerance Strategies of the Fall Armyworm, Spodoptera Frugiperda (Smith)(Lepidoptera: Noctuidae). Sci. Rep. 12, 4129. doi:10.1038/s41598-022-08174-4
Vélez, A. M., and Fishilevich, E. (2018). The mysteries of insect RNAi: A focus on dsRNA uptake and transport. Pestic. Biochem. Physiol. 151, 25–31. doi:10.1016/j.pestbp.2018.08.005
Wan, F. H., Yin, C. L., Tang, R., Chen, M. H., Wu, Q., Huang, C., et al. (2019). A Chromosome-Level Genome Assembly of Cydia Pomonella Provides Insights into Chemical Ecology and Insecticide Resistance. Nat. Commun. 10, 4237. doi:10.1038/s41467-019-12175-9
Wang, K., Peng, Y., Fu, W., Shen, Z., and Han, Z. (2019). Key Factors Determining Variations in RNA Interference Efficacy Mediated by Different Double -stranded RNA Lengths in Tribolium castaneum. Insect Mol. Biol. 28, 235–245. doi:10.1111/imb.12546
Wang, L. L., Lu, X. P., Smagghe, G., Meng, L. W., and Wang, J. J. (2017). Functional Characterization of BdB1, a Well-Conserved Carboxylesterase Among Tephritid Fruit Flies Associated with Malathion Resistance in Bactrocera Dorsalis (Hendel). Comp. Biochem. Physiol. C. Toxicol. Pharmacol. 200, 1–8. doi:10.1016/j.cbpc.2017.07.001
Wee, T., Rahul, V., Amanda, P., Tom, W., Samia, E., Sharon, D., et al. (2022). Global Population Genomic Signature of Spodoptera Frugiperda (Fall Armyworm) Supports Complex Introduction Events across the Old World. Commun. Biol. 5, 297. doi:10.1038/s42003-022-03230-1
Wu, H., March, J., and Bentley, W. (2016). “Gene Silencing in Insect Cells Using RNAi,” in Baculovirus and Insect Cell Expression Protocols. Editor D. W. Murhammer (Basel: Springer), 469–476. doi:10.1007/978-1-4939-3043-2_24
Xiao-Shuang, W., Min-Rui, S., Jin, X., Jian-Hong, L., and Hui, Y. (2021). Interference Efficiency and Effects of Bacterium-Mediated RNAi in the Fall Armyworm (Lepidoptera: Noctuidae). J. Insect Sci. 21 (58), 8–12. doi:10.1093/jisesa/ieab073
Xiong, K. C., Wang, J., Li, J. H., Deng, Y. Q., Pu, P., Fan, H., et al. (2016). RNA Interference of a Trehalose-6-Phosphate Synthase Gene Reveals its Roles during Larval-Pupal Metamorphosis in Bactrocera Minax (Diptera: Tephritidae). J. Insect Physiology 91–92, 84–92. doi:10.1016/j.jinsphys.2016.07.003
Xu, J., Nagata, Y., Mon, H., Li, Z., Zhu, L., Iiyama, K., et al. (2013). Soaking RNAi-Mediated Modification of Sf9 Cells for Baculovirus Expression System by Ectopic Expression of Caenorhabditis elegans SID-1. Appl. Microbiol. Biotechnol. 97, 5921–5931. doi:10.1007/s00253-013-4785-1
Yang, X. L., Liu, Y. C., Luo, M. Z., Li, Y., Wang, W. H., Wan, F, and Jiang, H. (2019). Spodoptera frugiperda moved into southwestern China for the first time in Jiangcheng County, Yunnan Province. Yunnan Agricul. 1, 72.
Yao, Y., Lin, D., Cai, X., Wang, R., Hou, Y., Hu, C., et al. (2022). Multiple dsRNases Involved in Exogenous dsRNA Degradation of Fall Armyworm Spodoptera Frugiperda. Front. Physiol. 13, 850022. doi:10.3389/fphys.2022.850022
Ye, C., Jiang, Y., Xin, A., Yang, L., Shang, F., Niu, J, and Wang, J. (2019). Effects of RNAi-based silencing of chitin synthase gene on moulting and fecundity in pea aphids (Acyrthosiphon pisum). Sci. Rep.. 9, 3694. doi:10.1038/s41598-019-39837-4
Yoon, J. S., Gurusamy, D., and Palli, S. R. (2017). Accumulation of dsRNA in Endosomes Contributes to Inefficient RNA Interference in the Fall Armyworm, Spodoptera Frugiperda. Insect biochem. Mol. Biol. 90, 53–60. doi:10.1016/j.ibmb.2017.09.011
Zhang, Y., Wiggins, B. E., Lawrence, C., Petrick, J., Ivashuta, S., and Heck, G. (2012). Analysis of plant-derived miRNAs in animal small RNA datasets. BMC Genomics 13, 381.
Zhang, L., Liu, B., Zheng, W. G., Liu, C. H., Zhang, D. D., Zhao, S. Y., et al. (2020). Genetic Structure and Insecticide Resistance Characteristics of Fall Armyworm Populations Invading China. Mol. Ecol. Resour. 20, 1682–1696. doi:10.1111/1755-0998.13219
Zhao, Y., Sui, X., Xu, L., Liu, G., Lu, L., You, M., and et al., (2018). Plant-mediated RNAi of grain aphid CHS1 gene confers common wheat resistance against aphids. Pest Manag. Sci. 74, 2754–2760. doi:10.1002/ps.5062
Zhou, R., Hotta, I., Denli, A. M., Hong, P., Perrimon, N., and Hannon, G. J. (2008). Comparative Analysis of Argonaute-dependent Small RNA Pathways in Drosophila. Mol. Cell. 32 (4), 592–599. doi:10.1016/j.molcel.2008.10.018
Zhu, J. Q., Liu, S., Ma, Y., Zhang, J. Q., Qi, H. S., Wei, Z-J., et al. (2012). Improvement of Pest Resistance in Transgenic Tobacco Plants Expressing dsRNA of an Insect-Associated Gene EcR. Plos One 7, e38572. doi:10.1371/journal.pone.0038572
Zhu, K. Y., and Palli, S. R. (2020). Mechanisms, Applications, and Challenges of Insect RNA Interference. Annu. Rev. Entomol. 65, 293–311. doi:10.1146/annurev-ento-011019-025224
Keywords: biological option, gene silencing, RNAi, insect pest management, Spodoptera frugiperda
Citation: Kebede M and Fite T (2022) RNA interference (RNAi) applications to the management of fall armyworm, Spodoptera frugiperda (Lepidoptera: Noctuidae): Its current trends and future prospects. Front. Mol. Biosci. 9:944774. doi: 10.3389/fmolb.2022.944774
Received: 03 June 2022; Accepted: 01 August 2022;
Published: 07 September 2022.
Edited by:
Maria Fatima Grossi De Sa, Brazilian Agricultural Research Corporation (EMBRAPA), BrazilReviewed by:
Guy Smagghe, Ghent University, BelgiumCopyright © 2022 Kebede and Fite. This is an open-access article distributed under the terms of the Creative Commons Attribution License (CC BY). The use, distribution or reproduction in other forums is permitted, provided the original author(s) and the copyright owner(s) are credited and that the original publication in this journal is cited, in accordance with accepted academic practice. No use, distribution or reproduction is permitted which does not comply with these terms.
*Correspondence: Tarekegn Fite, dGZkdXJlc3NhQGdtYWlsLmNvbQ==
Disclaimer: All claims expressed in this article are solely those of the authors and do not necessarily represent those of their affiliated organizations, or those of the publisher, the editors and the reviewers. Any product that may be evaluated in this article or claim that may be made by its manufacturer is not guaranteed or endorsed by the publisher.
Research integrity at Frontiers
Learn more about the work of our research integrity team to safeguard the quality of each article we publish.