- Institute of Biological Sciences, The John Paul II Catholic University of Lublin, Lublin, Poland
Casein kinases are involved in a variety of signaling pathways, and also in inflammation, cancer, and neurological diseases. Therefore, they are regarded as potential therapeutic targets for drug design. Recent studies have highlighted the importance of the casein kinase 1 superfamily as well as protein kinase CK2 in the development of several neurodegenerative pathologies, such as Alzheimer’s disease, Parkinson’s disease, Huntington’s disease, and amyotrophic lateral sclerosis. CK1 kinases and their closely related tau tubulin kinases as well as CK2 are found to be overexpressed in the mammalian brain. Numerous substrates have been detected which play crucial roles in neuronal and synaptic network functions and activities. The development of new substances for the treatment of these pathologies is in high demand. The impact of these kinases in the progress of neurodegenerative disorders, their bona fide substrates, and numerous natural and synthetic compounds which are able to inhibit CK1, TTBK, and CK2 are discussed in this review.
Introduction
Due to the fact that the world population is getting progressively older, the risk of neurodegenerative disorders (NDDs) is increasing. Those disorders occur when neurons lose their structure and function which finally leads to their death. Noteworthy, by 2000 it was estimated that the number of patients suffering from dementia in developed countries reached 13.5 million and will rise up to 36.7 million in 2050 (Zheng and Chen, 2022). Alzheimer’s disease (AD) and Parkinson’s disease (PD) are the most prevalent NDDs worldwide.
NDDs are classified according to their major clinical symptoms, altered proteins, and cellular/subcellular pathology. On the molecular level, typical features include protein misfolding and the formation of protein aggregates. The exact mechanisms for that are still unknown. These aggregates are the result of protein modification, like phosphorylation, sumoylation, and ubiquitination (Kovacs et al., 2010).
Typical characteristics of AD are senile plaques of amyloid-beta (Aβ) peptide precipitated in the space between neurons and the neurofibrillary tangles (NFTs) of fibrillar hyperphosphorylated tau protein (Glenner and Wong, 1984; Grundke-Iqbal et al., 1986). The Aβ peptide is the product of the proteolytic cleavage by β- and γ-secretases of the amyloid precursor protein (APP) (Vassar et al., 1999). In AD patients typical clinical features are the progressive loss of mental abilities, e.g., increasing forgetfulness, changes in personality, and cognitive difficulties. Additionally, as result of an immune response in the brain, nerve cells lose their function and die.
Huntington’s disease (HD) is caused by an autosomal dominant genetic defect. Mutations in the huntingtin gene (HTT) encoding huntingtin are responsible for the onset of HD. Typical features of this disease are movement disorders, like chorea and loss of coordination, as well as cognitive decline. Furthermore, common psychiatric symptoms are psychosis, depression, and obsessive-compulsive disorder (Rosenblatt, 2007). In HD patients a degeneration of the striatum and general shrinkage of the brain can be observed (Reiner et al., 1988). Loss of cortical mass is regionally selective and proceeds from posterior to anterior cortical regions during HD progression. Other symptoms are weight loss, cardiac failure, and skeletal-muscle wasting (Arenas et al., 1998; Aziz et al., 2008).
PD is a neurodegenerative disease characterized by progressive loss of neuromelanin containing dopaminergic neurons in the substantia nigra pars compacta. There is evidence for the relation between a small volume of this brain region and the weaker or less controlled motor movements of PD patients resulting in the often observed tremors (Menke et al., 2010). The pathological picture of PD includes motor impairments, like resting tremors, bradykinesia, postural instability, and rigidity. Due to the loss of norepinephrine non-movement related symptoms, like psychiatric problems, low blood pressure, and constipation, are seen. The cause of PD might be a combination of environmental and genetic factors.
Amyotrophic lateral sclerosis (ALS) is a fast progressive NDD affecting lower and upper neurons in the brain stem, spinal cord, and the motor cortex (Robberecht and Philips, 2013). It is the most common motor neuron disease in adults and the third most NDD worldwide (Renton et al., 2013). Typical features of ALS are atrophy and paralysis of skeletal muscles resulting from neuron loss and lack of communication between voluntary muscles of the body and the nervous system. Besides these symptoms, in most cases also cognitive and behavioral dysfunctions are present. ALS patients generally die within 3–5 years after first symptoms (Regal et al., 2006). The great majority of cases are classified as sporadic ALS, whereas only 10% are familial. Abnormal aggregations of transactive response DNA-binding protein 43 (TDP-43) are detected in almost all ALS cases (Neumann et al., 2006; Mackenzie et al., 2010). Mutations in the TARDBP gene encoding TDP-43 are associated with ALS (Sreedharan et al., 2008).
Protein kinases are encoded by about 2% of all human genes and are capable of phosphorylating up to 20% of all proteins. The counterplay of protein kinases and phosphatases regulates many processes in living cells through modification of serine, threonine and tyrosine residues (Hunter, 1995, 2012; Cohen, 2002; Schwartz and Murray, 2011; Nishi et al., 2014). As a result of phosphorylation protein activities, their stability, localization and interaction with other proteins are controlled. This posttranslational modification is capable of changing protein functions either by allosteric interaction or binding to regulatory domains (Jin and Pawson, 2012; Ardito et al., 2017). It has an important impact on processes, such as DNA replication, transcription and translation, cell metabolism, apoptosis, as well as stress and immunological response (Hunter, 1995; Cohen, 2002; Tarrant and Cole, 2009; Karve and Cheema, 2011). Proteins mainly undergo phosphorylation in the cytosol or in the nucleus (Duan and Walther, 2015).
Currently, there are no drugs available which cure or prevent NDDs, only acute disorders and symptoms are treated.
Numerous protein kinases have been described to play an important role in NDDs (Benn and Dawson, 2020). Unfortunately, most kinase inhibitors are not able to cross the blood-brain-barrier and are, therefore, only suitable for non central nervous disorders. During last decades, there is an increasing interest in the field to develop brain penetrant kinase inhibitors using the approaches from cancer research.
CK2 and protein kinases of the CK1 superfamily
Human protein kinases have been divided into 10 groups, 9 of them contain an eukaryotic kinase domain (ePK) and the last group is classified as atypical kinases. The majority of protein kinases phosphorylate serine and threonine residues (Ser/Thr kinases), others phosphorylate tyrosine (Tyr kinases). Few kinases are able to modify all three amino acids (dual-specificity kinases). Eukaryotic protein kinases share similarities in the primary sequences and structural features (Hanks and Hunter, 1995; Taylor et al., 1995; Taylor and Kornev, 2011).
CK1 isoforms together with the closely related vaccinia-related kinases (VRKs) and tau tubulin kinases (TTBKs) are classified in a separated group within the Ser/Thr kinase superfamily, whereas CK2 isoforms constitute a subclass of the CMGC group (Knippschild et al., 2005; Perez et al., 2011; Venerando et al., 2014; Fulcher and Sapkota, 2020). Protein kinases of the CK1 group and CK2 completely differ in their structure. At the beginning, they were named according to the phosphorylated in vitro protein substrate, casein. They were purified for the first time from soluble extracts of lactating bovine mammary (Waddy and Mackinlay, 1971). Natively isolated enzymes were purified on DEAE-cellulose and called casein kinase 1 and 2 depending on their elution profile (Hathaway and Traugh, 1979). G-CK or Fam20C shows high similarity to the casein kinase found in lactating mammary glands. It has been found in rat liver and brain and phosphorylates casein in the Golgi bodies (Bingham and Farrel, 1974; Lasa et al., 1997). Fam20C is characterized as a secretory kinase phosphorylating secreted proteins, from milk to bone proteins. This is important in the process of biomineralization of bones and teeth (Tagliabracci et al., 2015).
The role of CK1 in NDDs
CK1 is an evolutionarily conserved and ubiquitously expressed protein kinase. It belongs to second-messenger-independent and constitutively active kinases. CK1 exists in monomeric form with seven isoforms (α, β, γ1, γ2, γ3, δ, and ε) and their alternative splicing forms, which are encoded by different genes (Fulcher and Sapkota, 2020). The CK1 isoforms differ in their kinase activities, functions, subcellular localization, and biochemical properties (Zhang et al., 1996; Burzio et al., 2002; Takano et al., 2004; Xu et al., 2019). They vary in their molecular weights between 37 and 51 kDa with CK1α being the smallest and CK1γ3 the largest protein. The analysis of the substrate specificity of CK1 isoforms initially determined pS/pT-X1-2-S/T as their consensus sequence. This led to the assumption that phosphorylation by CK1 depends on the prior phosphorylation of position −2 or −3 (Meggio et al., 1991; Meggio et al., 1992). Further studies have shown that this hypothesis did not hold true when proteins were efficiently phosphorylated without prephosphorylated residues (Flotow and Roach, 1991; Marin et al., 1994; Pulgar et al., 1999). Later, novel substrates were described containing a non-canonical motif (S-L-S) with acidic residues downstream of the phosphorylation site. Over 140 substrates are described for CK1. Most of them are involved in various cell processes, e.g., membrane transport and trafficking, microtubule-associated dynamics, apoptosis, and cell cycle progression (Yang et al., 2017; Xu et al., 2019). In diverse studies the important role of CK1 in NDDs was shown, emphasizing on tauopathies, such as AD. A distribution study of all CK1 isoforms comparing AD and control brains revealed that CK1 can be found in fibrillar lesions and, additionally, within the matrix of granulovacuolar degeneration bodies (Ghoshal et al., 1999; Kannanayakal et al., 2006). Contrary to CK1α which is linked to fibrillar lesions, CK1δ is linked to granulovacuolar degeneration bodies (Kannanayakal et al., 2006). It is possible that alteration of CK1δ function is aligned with dysregulation of circadian rhythms in AD. Elevated expression levels of CK1δ (33-fold) and CK1ε (9-fold) has been described in AD post-mortem brain tissue and ALS (Ghoshal et al., 1999; Yasojima et al., 2000; Salado et al., 2014; Palomo et al., 2020; Carter et al., 2021). Many proteins related to neurological disorders are modified by CK1α, e.g., β-secretase (Walter et al., 2001), α-synuclein (Mbefo et al., 2015), and parkin (Yamamoto et al., 2005). Similarly, it has been discovered that CK1δ phosphorylates several proteins that are associated with different NDDs in vitro. CK1δ phosphorylates the tau protein leading to its aggregation and finally the formation of neurofibrillary tangles (Li et al., 2004). Increased CK1 activity is associated with tau aggregation (Schwab et al., 2000). CK1δ-dependent phosphorylation has been also shown for other proteins connected with neurodegenerative diseases, e.g., presenilin-2, β-secretase, parkin, TDP43, α-synuclein, LRRK2, and tau (Okochi et al., 2000; Walter, 2000; Rubio de la Torre et al., 2009; Alquezar et al., 2016; Nonaka et al., 2016; Morales-Garcia et al., 2017; De Wit et al., 2018). Therefore, inhibition of CK1δ/ε has been described to possess favorable effects on ALS, frontotemporal dementia (FTD), and PD phenotypes in vivo (Perez et al., 2011; Salado et al., 2014; Alquezar et al., 2016; Cozza and Pinna, 2016; Jiang et al., 2018; Palomo et al., 2020).
The role of TTBK in NDDs
Within the CK1 superfamily a small family of brain-specific kinases phosphorylating microtubule-associated proteins tau and tubulin is classified (Ikezu and Ikezu, 2014). TTBK is a Ser/Thr and Tyr dual-kinase conducting multiple functions inside the cell. It comprises two isoforms: TTBK1 and TTBK2. TTBK1 was characterized as a neuron-specific kinase phosphorylating tau which leads to its aggregation, while TTBK2 was purified from the bovine brain (Takahashi et al., 1995; Sato et al., 2006). Both isoforms are encoded by distinctive genes, and furthermore, their localization in tissues is diverse (Nozal and Martinez, 2019). The sequence of TTBK1, consisting of 1321 amino acids, can be divided into kinase domain (residues 34-297), and a regulatory domain, which contains a characteristic 39 amino acids poly-Glu motif (Ikezu and Ikezu, 2014). The comparison of the TTBK1 and CK1δ kinase domain sequences revealed a 38% identity and 52% similarity (Sato et al., 2006). TTBK1 is primarily expressed in the human brain, notably in the adult brain cortex, cerebellum, and fetal brain. When mouse brain was analysed, TTBK1 was also detected in the frontal cortical layers, the hippocampus, and the granular layer of the cerebellum. Studies using antibodies confirmed the colocalization with tubulin in neurons (Sato et al., 2006). TTBK1 is upregulated in AD cases (Takashima et al., 1993).
TTBK2 consists of 1244 amino acids, and contrary to TTBK1, is ubiquitously expressed in the whole body. Highest TTBK2 mRNA expression levels are monitored in cerebellum Purkinje cells, granular cell layer, hippocampus, midbrain, and substantia nigra, whereas lower levels were found in the cortex of human, rat, and mouse brains (Houlden et al., 2007). In analyses of the protein expression in the brain and testis higher amounts of TTBK2 were found which correlates with higher activities of TTBK2 in these tissues (Bouskila et al., 2011). Mutations in the TTBK2 gene are responsible for the onset of spinocerebellar ataxia type 11, an NDD characterized by progressive ataxia and atrophy of the cerebellum and brainstem.
Both isoforms contain highly similar catalytic domains (88% identity and 96% similarity), but diverse C-terminal domains of 43% identity and 58% similarity (Ikezu and Ikezu, 2014; Nozal and Martinez, 2019). TTBK1/2 were described as kinases which show higher phosphorylation activity in case of a prephosphorylated substrate at position -3 (S/T-X-X-S/T/Y). On the surface of TTBK1 two positive sequences have been identified, which might act as putative binding sites for the prephosphorylated substrate (Xue et al., 2013; Kosten et al., 2014). Phosphorylation of tau was shown at Y197, S198, S199, S202, and S422, the critical sites in paired helical filaments (Grundke-Iqbal et al., 1986; Sato et al., 2006; Tomizawa et al., 2001). Interestingly, both isoforms differ in the aa sites which they phosphorylate. Due to tau phosphorylation in neurons at S422, TTBK1 is responsible for neurofibrillary pretangle formation and subsequent tau aggregation (Sato et al., 2008; Vazquez-Higuera et al., 2011; Yu et al., 2011; Lund et al., 2013). In knock-down experiments it has been demonstrated that TTBK1, not TTBK2, is the main isoform responsible for tau phosphorylation at S422 (Bao et al., 2021). Overexpression of TTBK1 in mice resulted in increased phosphorylation and oligomerization of tau in the brain (Xu et al., 2010).
Besides the phosphorylation of tau and tubulin by TTBK2, further substrates include centrosomal proteins CEP164 and CEP97, SV2A as well as neurodegeneration-associated protein TDP-43 (Takahashi et al., 1995; Tomizawa et al., 2001; Ikezu and Ikezu, 2014; Liachko et al., 2014; Liao et al., 2015; Zhang et al., 2015). Additionally, TTBK2 is crucial for the regulation of the growth of axonemal microtubules in ciliogenesis (Liao et al., 2015).
Especially TTBK1 is a promising candidate as target for NDDs treatment. It is mainly expressed in brain tissue, and therefore, possesses limited off-pathway roles. The phosphorylation of tau and TDP-43 makes it an ideal kinase in the case of these two proteinopathies.
The role of CK2 in NDDs
Together with CK1, CK2 was identified as phosphotranferase using casein as protein substrate for enzymes able to catalyse phosphate transfer from ATP to proteins (Burnett and Kennedy, 1954). Native protein kinase CK2 exists as a heterotetrameric holoenzyme consisting of two catalytic subunits, α and α′, and a dimer of regulatory subunits β (Hathaway and Traugh, 1979). The two isoforms of the CK2 catalytic subunit are highly homologous, but they are products of two different genes (Wirkner et al., 1994; Yang-Feng et al., 1994; Ackermann et al., 2005). CK2 subunits may build different active holoenzymes in three different conformations (αα′, α2, or α′2) or exist as free catalytic subunits. Each CK2 isoform possesses characteristics common for CK2, but differences in their substrate specificity and sensitivity to inhibitors have been described. They may also regulate different cellular processes (Pinna, 2002; Domańska et al., 2005; Janeczko et al., 2012).
CK2 is a constitutively active protein kinase, independent from second messengers, and is able to use both, ATP and GTP, as phosphoryl donors (Litchfield, 2003). Analysis of the eukaryotic phosphoproteome revealed that CK2 is responsible for the phosphorylation of almost one-quarter of phosphoproteins (Meggio and Pinna, 2003; Salvi et al., 2009; Franchin et al., 2015).
The minimal consensus sequence of CK2 was estimated as S/T-X-X-D/E/pS/pY, which is present in numerous proteins (Meggio et al., 1994; Venerando et al., 2014).
After the detection of the critical role in various disease states, like cancers and neurodegenerative disorders research groups worldwide focussed their attention on CK2 as a potential therapeutic target (Blanquet, 2000; Perez et al., 2011; Castello et al., 2017; Borgo et al., 2021a).
Several CK2 targets in NDDs were described. α-synuclein is phosphorylated at S129 that leads to aggregates which are the main component of Lewy bodies. In 90% of PD samples this phosphorylation is found, whereas in only 4% of normal tissue. As shown, this site is affected by several kinases and dependent on which one the biological effects might differ (Oueslati, 2016).
In different reports the diverse role of CK2 in AD is described. CK2 phosphorylates presenilin-2 at S7 and S9 in vitro while not altering APP cleavage by γ-secretase (Walter et al., 1996; Sannerud et al., 2016). Additionally, it was shown that CK2 phosphorylates SET, a phosphatase PP2A inhibitor, at position S9 which leads to its translocation to the cytoplasm (Zhang et al., 2018).
Numerous reports reveal that CK2 possesses a protective role in HD. The phosphorylation of huntingtin at S13 and S16 alters its location. Phospho-huntingtin is found in the nucleus which reduces its cellular toxicity (Atwal et al., 2011). Similarly, TDP-43 phosphorylation may prevent protein aggregation of truncated forms (Li et al., 2011).
Proteins involved in neurodegenerative diseases
A lot of NDD-associated proteins playing an important role in the onset of these disorders were identified (Kovacs et al., 2010): (1) the tubulin-associated unit (tau) protein; (2) amyloid-β (Aβ), peptides which result from cleavage of a large transmembrane precursor protein (Aβ-precursor protein or APP); (3) α-synuclein; (4) prion protein; (5) TDP-43 (Ou et al., 1995); (6) fused in sarcoma protein, Ewing’s sarcoma RNA-binding protein 1, and TATA-binding protein-associated factor 15, also known as FET proteins (Neumann et al., 2011). Other proteins are associated with neurological disorders caused by mutations leading to trinucleotide repeats (e.g., huntingtin, ataxins, atrophin-1).
Neurodegenerative proteinopathies can be classified according to the major protein involved in the disease: tauopathies, α-synucleinopathies, TDP-43 proteinopathies, FUS/FET proteinopathies, prion diseases, trinucleotide repeat diseases, neuroserpinopathy, ferritinopathy, and cerebral amyloidoses.
Several of these proteins were identified as substrates for CK1, TTBK, and CK2 and are further described below. Table 1 summarizes information about these proteins phosphorylated by CK1, TTBK, and CK2 and their respective phosphorylation sites.
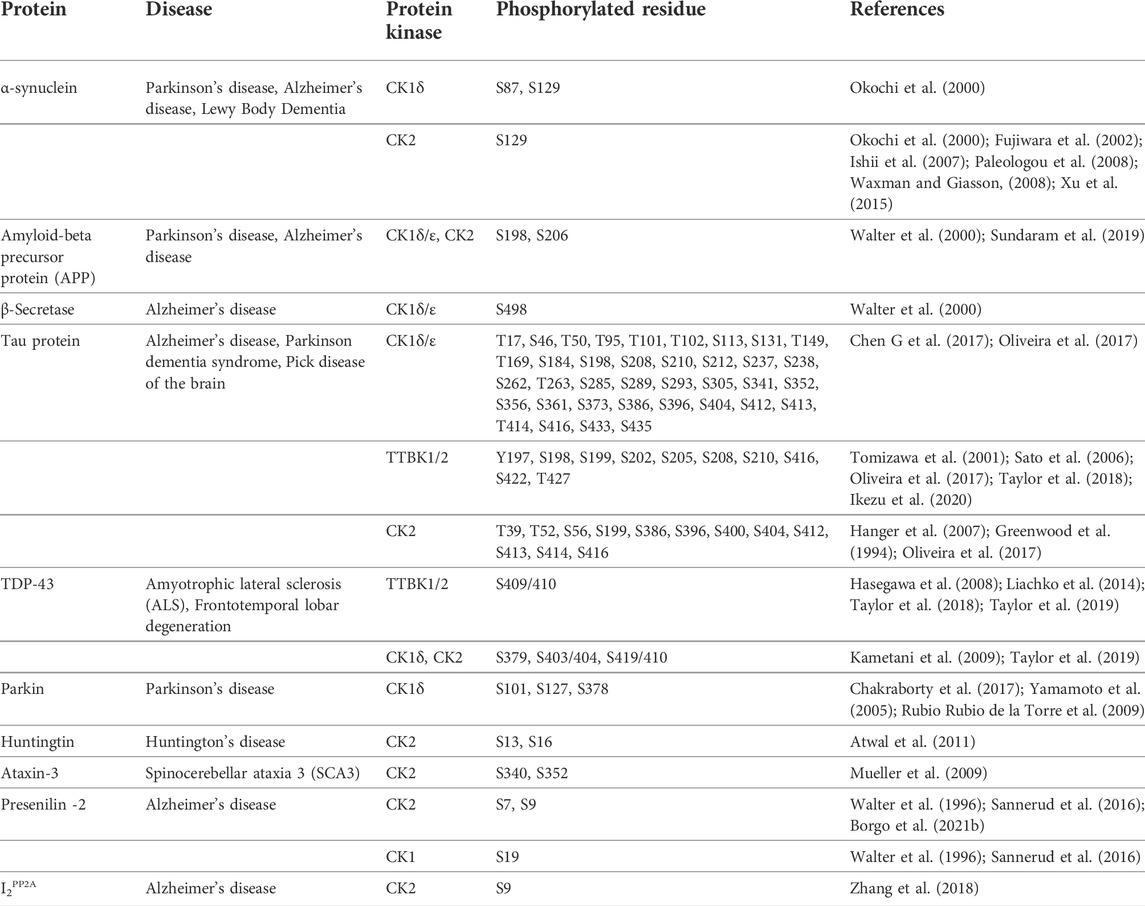
TABLE 1. Selected proteins involved in neurodegenerative diseases and their phosphomodifications by casein kinases.
α-synuclein
The name of this protein is derived from synaptic vesicles (syn-) and the nuclear envelope (-nuclein), both places where α-synuclein was first identified (Maroteaux et al., 1988). As we know now, this was probably the effect of a contaminated antibody that was used in this study. It is involved in PD, dementia with Lewy bodies, and multiple system atrophy (Spillantini et al., 1997; Wakabayashi et al., 1997; Gai et al., 1998). α-synuclein is a ubiquitously expressed protein with the highest levels in neurons, especially in presynaptic terminals. Other localizations for α-synuclein have been also identified, mainly based on overexpression experiments, but its function there remains unclear.
α-synuclein is a protein built of 140 amino acids that undergo posttranslational modification, especially at its C-terminus, e.g., phosphorylation, oxidation, ubiquitination, acetylation, and glycosylation. The major phosphorylation sites are S87 and S129 (Okochi et al., 2000; Fujiwara et al., 2002; Ishii et al., 2007). It was also shown that tyrosine residues are phosphorylated: Y125, Y133, and Y136 (Ellis et al., 2001; Nakamura et al., 2001; Negro et al., 2002). In pathological states, α-synuclein adopts a β-sheet conformation, which consequently leads to α-synuclein aggregation, fibril formation, and deposition into Lewy bodies (Conway et al., 1998; El-Agnaf et al., 1998; Narhi et al., 1999; Uversky, 2007; Yonetani et al., 2009). In PD, Lewy bodies, point mutations, and duplications/triplication of α-synuclein gene are the main pathological hallmark (Burré et al., 2018). Lewy bodies containing α-synuclein were also deteced in samples from familial AD patients (Lippa et al., 1998). Furthermore, in senil plaques in AD a short fragment of α-synuclein (aa residues 61-95) was found and termed non-Aβ-amyloid component, a region that is necessary for α-synuclein aggregation and fibrillogenesis (Ueda et al., 1993). Phosphorylation prevents or at least decreases the aggregation and toxicity of α-synuclein (Waxman and Giasson, 2008). Within all identified phosphorylation sites in vivo and in vitro only S87 lies in the non-Aβ-amyloid component (El-Agnaf et al., 1998).
Amyloid-beta precursor protein
APP was isolated and purified from cerebral Aβ deposits in 1984 (Glenner and Wong, 1984). It is found in different tissues, particularly in the brain, as a type I transmembrane protein located predominantly in the endoplasmic reticulum (Zheng and Koo, 2006). In 1992, Hardy and Higgins presented the amyloid cascade hypothesis as their theory of AD pathophysiology (Hardy and Higgins, 1992). Proteases from the secretase family (β-secretase and γ-secretase) cleave APP into Aβ peptides of different lengths, mainly Aβ38, Aβ40, and Aβ42, whereas α- and γ-secretases produce P3 peptides (LaFerla et al., 2007; O’Brien and Wong, 2011). Although the most abundant form is Aβ40 (80%–90%), Aβ42 is mainly responsible for protein aggregations and the formation of oligomers, amyloid fibrils, and amyloid plaques (Chen C et al., 2017). Those amyloid plaques are the cause of neurotoxicity in AD progression (Citron et al., 1996; Murphy and LeVine, 2010). Effects of Aβ40/Aβ42 aggregation, especially Aβ oligomers, are calcium dishomeostasis, disturbance of ion channels, alteration of glucose regulation and oxidative damage (Tiwari et al., 2019). Furthermore, it was described that Aβ aggregation promotes tau phosphorylation and aggregation. Out of 30 mutations described in the APP gene, 25 are involved in the deposition of insoluble Aβ, like KM670/671NL (Swedish), V717I (London), V717F (Indiana).
Tau protein
The tau protein was firstly discovered in porcine brain and isolated as heat-stable protein. The function of tau is the stabilization of internal microtubules (Weingarten et al., 1975). It is particularly highly expressed in axons of neuronal cells of the central nervous system (Binder et al., 1985). Studies have shown that tau is a phosphoprotein that then negatively influences the microtubule assembly by changes of the molecule shape (Jameson et al., 1980; Lindwall and Cole, 1984). Phosphorylation of tau is often accompanied by other posttranslational modifications, e.g., O-glycosylation, ubiquitination, and methylation. Tau inclusions occur in AD, Pick’s disease, progressive supranuclear palsy, corticobasal degeneration, argyrophilic grain disease, Parkinsonism-dementia complex of Guam, and FTD (Lee et al., 2001).
Tau primary transcript generates six isoforms by alternative splicing resulting in proteins of 352-441 amino acids and MW of 45–65 kDa (Boyarko and Hook, 2021). Tau protein possesses 80 S/T and 5 Y residues of which at least 46 have been found to be phosphorylated in AD (Hanger et al., 2009). The total phosphorylation level of tau in AD and other tauopathies is several times higher than in control samples (Gong and Iqbal, 2008). The ability of tau to polymerize tubulin and to promote microtubule assembly is reduced through hyperphosphorylation (Yoshida and Ihara, 1993). A correlation between the level of hyperphosphorylation at multiple sites and the severity of NFT pathology was found which also correlates with the degree of neuronal loss and cognitive deficit (Grundke-Iqbal et al., 1986; Braak and Braak, 1991; Augustinack et al., 2002).
TDP-43
TDP-43 is a ubiquitous protein belonging to the ribonucleoprotein family and is normally localized in the nucleus where it takes part in RNA regulation (Nakielny and Dreyfuss, 1997; Geuens et al., 2016). Firstly, it was identified as a transcrptional repressor of HIV-1 transactivator response (TAR) long terminal repeats (Ou et al., 1995). Later, it was demonstrated that it is the major component of ubiquitinated inclusions in ALS and frontotemporal lobar degeneration (FTLD). Posttranslational modifications, such as cleavage, hyperphosphorylation, and ubiquitination lead to cytoplasmic accumulation and aggregation of TDP-43 (Arai et al., 2006; Neumann et al., 2006; Hasegawa et al., 2008). The sequence of TDP-43 is divided into three parts: an N-terminal domain (residues 1–103), two RNA recognition motifs (residues 104–200 and 191–262), and a C-terminal domain (residues 274–413). TDP-43 possesses 64 potential phosphorylation sites. Phosphorylation at S403/404 and S409/410 at the C-terminus results in pathological inclusions (Hasegawa et al., 2008; Inukai et al., 2008; Zhang et al., 2009). The N-terminus contains a nuclear localization sequence that is prone to mutations leading to cytoplasmic localization of TDP-43 and aggregation, whereas the C-terminus is necessary for solubility and cellular localization (Ayala et al., 2008; Barmada et al., 2010).
Parkin
Parkin possesses an activity of an E3 ubiquitin ligase (Shimura et al., 2000). Insoluble parkin, resulting from point mutations, plays a major role in the inactivation of the protein in PD (Cookson et al., 2003; Sriram et al., 2005; Hampe et al., 2006). Phosphorylation of S101, S127, and S378 was identified using CK1 in vitro and in vivo with HEK293T cells transiently transfected with parkin (Yamamoto et al., 2005; Rubio de la Torre et al., 2009). Treatment of those cells with IC261, a selective CK1 inhibitor, significantly decreased the phosphorylation level of parkin (Mashhoon et al., 2000; Bain et al., 2007; Rubio de la Torre et al., 2009). Another potent CK1 inhibitor, D4476, was used, which confirmed the hypothesis that S101 and S378 are phosphorylated in vivo. Besides CK1, parkin is phosphorylated by CDK5 at S121 (Avraham et al., 2007). Experiments in vivo and in vitro have shown that the interplay of CK1 and CDK5 is necessary for efficient phosphorylation by both kinases. A phospho-mimetic mutant on the phosphorylation site of one kinase increased the phosphorylation level by the second kinase. Supporting evidence is the finding that roscovitine, a selective CDK5 inhibitor, reduced the phosphorylation of parkin by CK1 resulting from inhibition of S121 phosphorylation (Meijer et al., 1997; Rubio de la Torre et al., 2009). In further experiments, the influence of parkin phosphorylation on its activity and its effect on the formation of inclusions was examined. The results indicate that the phospho-mimetic mutant for compound phosphorylation possesses slightly enhanced enzymatic activity and showed a significantly higher tendency for aggregation (Rubio de la Torre et al., 2009).
Huntingtin
Huntingtin is a ubiquitously expressed protein with a molecular weight of 350 kDa. It possesses a poly-Glu sequence at the N-terminus containing up to 35 CAG repeats in wild-type, whereas HD patients carry 36 or more repeats (Rubinsztein et al., 1996). There is evidence for an inverse correlation between the age of onset of symptoms and the number of CAG repeats (Andrew et al., 1993). In 65%–71% of cases, larger CAG repeats led to earlier ages of onset. Genetic and environmental factors are also playing an important role in the age of onset. Huntingtin is localized in the cytoplasm, partially in the nucleus. Its nuclear localization sequence is also found in the N-terminus and in the C-terminus a nuclear export sequence is found (Xia et al., 2003; Desmond et al., 2012). The prolongated poly-Glu sequence in HD patients inhibits the interaction between the N-terminus with the nuclear pore protein translocated promotor region which is involved in nuclear export. As a result, huntingtin is accumulated in the nucleus (Cornett et al., 2005). Noteworthy, toxic fragments of huntingtin present in the nucleus are mainly from the mutated protein due to their higher concentration in the nucleus than in the cytoplasm (Hackam et al., 1998; Lunkes et al., 2002). One therapeutic strategy is the inhibition of the formation of these fragments by modification of huntingtin (e.g., phosphorylation) to prevent the cleavage of the protein (Atwal et al., 2011).
Ataxin-3
Ataxin-3 is a ubiquitin protease involved in transcriptional regulation and the disease protein in spinocerebellar ataxia type 3 (Burnett et al., 2003; Evert et al., 2006). It possesses nuclear and cytoplasmic functions. Its subcellular distribution is regulated through phosphorylation. As in the case of huntingtin, the nuclear presence of ataxin-3 represents a key element in the accumulation of toxic fragments (Bichelmeier et al., 2007). Analysis of 15 putative serine phosphorylation site mutants revealed that S236 in the first ubiquitin-interacting motif (UIM), S256 and S260/261 in the second UIM, as well as S340 and S352 in the third UIM, are the major phosphorylation sites of CK2 (Mueller et al., 2009). Phosphorylation of those serines determines the subcellular location of ataxin-3. Modulation of S340, S352, and S236 increases the nuclear presence of ataxin-3, while phosphorylation of S256 and S260/261 provides preferential cytoplasmic localization (Mueller et al., 2009). Apart from the influence on the cellular distribution of ataxin-3, phosphorylation plays also an important role in the solubility of the protein. As shown in vivo experiments phospho-mimetic mutants formed aggregates in the nucleus (Mueller et al., 2009). The effect of two selective CK2 inhibitors (DMAT and TBB) on the localization and presence of inclusions was examined in cell culture (Pagano et al., 2008). Inhibition of CK2 resulted in lower nuclear localization of ataxin-3 and less formation of protein aggregates (Mueller et al., 2009).
Presenilin-2
PSEN1 and PSEN2 genes, containing 10 exons, encode presenilin-1 and presenilin-2, respectively, which play important roles in AD pathogenesis. Presenilin is a part of the γ-secretase complex responsible for the cleavage of APP to generate Aβ peptides. Mutations in PSEN1/2 and deletions leading to alternative transcripts are associated with AD and FTD (Raux et al., 2000; Evin et al., 2002; Marcon et al., 2009). Incorrect transcripts, like PS2V lacking exon 6, are related to different diseases, e.g., AD (Braggin et al., 2019). PS2V produces truncated presenilin-2 containing 124 amino acids and only 1 of 9 transmembrane domains. This isoform was identified in the brain of AD patients with elevated levels leading to an increased amount of Aβ peptide (Sato et al., 1999; Smith et al., 2004). Two phosphorylation sites for CK2 (S7 and S9) and one for CK1 (S19) were identified (Walter et al., 1996). S19 phosphorylation elevated the binding of AP-1 protein to presenilin-2, whereas S7 and S9 phosphorylation did not show any change in the binding of activator protein 1 (Sannerud et al., 2016). Further experiments are necessary to examine the role of phosphorylation in the case of presenilin-2.
Inhibitor-2 PP2A
The phosphorylation of tau is regulated by phosphoseryl/phosphothreonyl protein phosphatase PP2A and its activity is decreased in AD brain. PP2A is regulated by two endogenous inhibitory proteins called I1PP2A and I2PP2A (Gong et al., 1993; Li et al., 1996). Typically, I2PP2A is mainly located in the nucleus regulating DNA replication, gene transcription, cell-cycle progression, DNA repair and migration as well as chromatin remodeling. In AD patients, I2PP2A is overexpressed and translocated from the nucleus into the cytoplasm, where PP2A and significantly hyperphosphorylated tau is localized forming the NFTs in the neuronal cytoplasm (Tanimukai et al., 2005). In PC12 cells, stably transfected with tau and transiently transfected with human I2PP2A, accumulation of the inhibitor in the cytoplasm was observed (Chohan et al., 2006). CK2 was identified as one of two kinases responsible for the phosphorylation of S9 (Vera et al., 2007). This phosphorylation affects the ability of I2PP2A to bind to importin proteins (importin-α and importin-β). Phosphorylated I2PP2A does not form a complex with importin, therefore, it is localized in the cytoplasm instead to be transported into the nucleus (Yu et al., 2013).
Kinase inhibitors used in NDD pathway interrogation
In the literature synthetic and natural substances are described inhibiting protein kinases CK1, TTBK, and CK2. Table 2 gives an overview of several inhibitors with in vitro and/or in vivo activities on these kinase targets. Many of them do not selectively inhibit kinases, but might be a good starting point for drug design. Many research results on cancers and cancer cell lines involving protein kinase inhibitors were published, but only a few reports towards NDDs, especially in the case of CK2. Cancer and NDDs are both characterized by the dysregulation of the same signalling pathways, but with opposite effects. In cancers the cell survival and proliferation is increased, whereas, in NDDs those alterations lead to cell death and apoptosis. The most altered signal pathways in cancer, e.g., Nrf2 pathway and Wnt/β-catenin pathway, are also implicated in NDDs, like AD and PD (Varela and Garcia-Rendueles, 2022).
The development of specific CK1 inhibitors capable to cross the blood-brain-barrier is a promising target for the treatment of TDP-43 proteinopathies, e.g., ALS. Small brain-penetrating molecules were described which block the neurotoxicity of TDP-43 in cell culture experiments through inhibition of its phosphorylation (Perez et al., 2011; Salado et al., 2014; Morales-Garcia et al., 2017). Up to now, few CK1-specific compounds have been synthesised and a small part of them has been also examined in animal models. Kinetic studies of these compounds revealed an ATP-competitive mode of action in the case of almost all molecules.
In several studies, it has been proven that CK1 activity is necessary for molecular pacemaking. It was shown that CK1δ is the main regulatory element of the clock period: inhibition of CK1δ remarkably prolongated the circadian rhythms in locomotor activity in vivo and molecular oscillations in the suprachiasmatic nucleus (SCN) and peripheral tissue slices in vitro. Additionally, cumulation of PER2 protein in the nucleus was observed, in vitro and in vivo (Meng et al., 2010).
Cell proliferation is increased and colony formation is promoted through overexpression of CK1α. Effects of CK1α inhibition include the increase of the sensitivity to radiotherapy and reduction of the production and secretion of pro-inflammatory factors (Liu et al., 2021).
Several benzimidazole-based inhibitors displayed significant inhibition of CK1δ, e.g., Bischof-5 and -6. Other potent ATP-competitive and selective CK1δ/ε inhibitors are represented by difluoro-dioxolo-benzimidazole derivatives, compounds 1 and 2 (Richter et al., 2014). Substances derived from inhibitors of Wnt production (IWP) are structurally similar to benzimidazoles. Such inhibitors have been characterized as ATP-competitive and CK1 specific.
Another potent inhibitor, compound 1h, which is highly selective and CK1 isoform-specific was identified from a high-throughput screen of the Amgen compound library (Hua et al., 2012).
Many modulators of CK1 presently under investigation are isolated from natural environment or are derivatives of natural products. Nowadays, compounds from marine organisms are getting more attention and are now being investigated in clinical tests, essentially against cancer, inflammation, chronic pain, and NDDs. Among the promising drug candidates is the family of lamellarins, which are marine alkaloids with fused 14-phenyl-6H-[1]benzopyrano[4′,3′:4,5]pyrrolo[2,1-a]isoquinoline or non-fused 3,4-diarylpyrrole-2-carboxylate ring systems (Baunbæk et al., 2008; Bailly, 2015; Fukuda et al., 2020). So far, over 50 lamellarins have been purified from different marine organisms, e.g., mollusks, tunicates, and sponges. In 2008, protein kinases have been identified as new molecular targets of anticancer lamellarins (Baunbæk et al., 2008). 22 lamellarins were screened for cancer- and Alzheimer’s disease-relevant protein kinases.
Until now, only a few protein kinases were described, which are associated with abnormal TDP-43 hyperphosphorylation, including both TTBK isoforms (Versluys et al., 2022). Both are known to colocalize with TDP-43 inclusions in spinal cords of ALS patients. TTBK2 is involved in crucial cellular mechanisms, e.g., ciliogenesis, microtubule dynamics, and neurotransmitter trafficking. Thus, its reduced activty may have negative effects for the patients (Jackson, 2012; Bowie and Goetz, 2020). Only a small number of TTBK1/2 inhibitors have been described, but unfortunately they do not show selectivity for one isoform (Xue et al., 2013; Kiefer et al., 2014).
A set of TTBK1 azaindazole inhibitors has been examined (Halkina et al., 2021). Two of them are characterized by high potency, selectivity and they are brain penetrant: compound 8 (4-(1-(2-Aminopyrimidin-4-yl)-1H-pyrazolo[4,3-c]pyridin-6-yl)-2- methylbut-3-yn-2-ol) with an IC50 of 60 nM and compound 31 ((S)-1-(1-(2-Amino-6-methoxypyrimidin-4-yl)-1H-pyrrolo[3,2-c]-pyridin-6-yl)-3-methylpent-1-yn-3-ol) with an IC50 of 2.7 nM.
Lately, receptor-based pharmacophore models were developed applying three TTBK1 protein structures. The combination of integrated e-pharmacophore based virtual screening and molecular dynamics simulation resulted in four hits: ZINC14644839 (5,6,4′-Trihydroxy-7,3′-Dimethoxyflavone, 5-TDMF), ZINC00012956 (3-phenyl-2-(9H-purin-6-ylamino)propan-1-ol), ZINC91332506 (1-[3-(6-aminopurin-9-yl)propyl]-3-methyl-pyridin-2-one), and ZINC69775110 (N-[(4-ethoxy-3-fluoro-phenyl)methyl]-7H-purin-6-amine). AMG-28 (4-(2-amino-5,6,7,8-tetrahydropyrimido[4′,5′:3,4]cyclohepta[1,2-b]indol-11-yl)-2-methylbut-3-yn-2-ol) was originally designed as an inhibitor of a Ser/Thr protein kinase essential for the activation of the NF-κB pathway (NIK) (Li et al., 2013). A co-crystal structure of this inhibitor with the kinase domain of human TTBK1 showed binding of the aminopyrimidine ring with the hinge region of protein kinase. On the basis of AMG-28 11 new indolyl pyriminidine compounds were synthetized. New analogs are more potent inhibitors of TTBK2 than TTBK1 (Potjewyd et al., 2022).
Compounds that may inhibit CK2 activities where described in numerous publications. Since research results on the role of CK2 in NDD are diverse, there are only a few reports about CK2 inhibitors on NDDs available. The observation that CK2 is either overactive or overexpressed in patient brains supports kinase inhibition as a therapeutic approach for multiple neurodegenerative diseases. A large group of CK2 inhibitors, known for more than 20 years, are benzimidazoles, e.g., TBB and DMAT (Szyszka et al., 1995).
Data propose the influence of CK2 on astrocytes in the neuroinflammatory response in AD. In astrocytes in the hippocampus and temporal cortex of AD patients levels of CK2α/α’ are increased. Those astrocytes are linked to amyloid deposits in the AD brain. In human primary astrocytes and U373 astrocytoma cells, the IL-1β/TNF-α induced secretion of the inflammatory cytokines MCP-1 and IL-6 is potently inhibited by CX-4945 dependent on the dose (Rosenberger et al., 2016). CX-4945 is the first described orally bioavailable CK2 inhibitor that advanced into clinical trials (Pierre et al., 2011; Cozza et al., 2012).
Quite recently SRPIN803-rev (6-(4-hydroxy-3-metoxybenzylidene)-5-imino-2-(trifluoromethyl)-5H-[1,3,4]thiadiazolo[3,2-a]pyrimidin-7(6H)-one), a new dual inhibitor of CK2 and serine-arginine protein kinase 1, was identified (Dalle Vedove et al., 2020). SRPIN803-rev and its new synthesized derivatives bind to the open conformation of the hinge/αD region within the ATP-binding pocket of CK2α.
The MLL/COMPASS stability is regulated by taspase1 cleavage and might be a possible target for clinical therapy of leukemia. Destabilized MLL and unprocessed version MLL1 associated with chromatin results in the displacement of MLL chimeras from chromatin in leukemic cells. The CK2 phosphorylation site is next to the taspase1 cleavage site, and enables its cleavage. Inhibition of CK2 by specific inhibitors (CX-4945 or TTP22) decrease taspase1-dependent MLL1 processing, which leads to higher MLL1 stability, and finally displace the MLL chimeras from chromatin. In a MLL-AF9 leukemia mouse model the suppression of CK2 retard the leukemic progression (Zhao et al., 2018).
Naturally occurring compounds might act as antioxidant, anti-inflammatory, antiviral, antimicrobial, and anticancer agents (Baier and Szyszka, 2020). They have shown neuroprotective effects in many clinical trials (Ullah et al., 2020; Akter et al., 2021; Kim and Park, 2021; Wang et al., 2021).
It has been proven that flavonoids are most effective in the treatment of NDDs, including AD. First analyses with flavonoids against CK2 were reported by Li et al. (2009), Lolli et al. (2012). As we described in our own publications, flavonoids naturally occurring in plants are highly potent CK2 inhibitors. A set of more than 20 compounds (e.g., apigenin, pedalitin, and chrysoeriol) was tested for their inhibitory effect on four human CK2 isoforms. The results reveal that CK2α’ was most sensitive to the examined compounds (Baier et al., 2017; Baier et al., 2018).
Quercetin (3,5,7,3′,4′–pentahydroxyflavone) belongs to the polyphenolic compounds with powerful antioxidant and anti-inflammatory activities. Polyphenolic compounds are often applied in the treatment and protection against severe diseases, like diabetes, cancer, neurodegenerative and cardiovascular diseases.
In time-course experiments it was shown that CK2 is crucial at early time points just after the induction of cell differentiation (Schwind et al., 2017).
In several studies it was observed that Nrf2 signaling is involved in PD pathogenesis (Dheen et al., 2005). The increase of Nrf2 induced dopamine (DA) neuroprotection and, at the same time, the decrease of Nrf2 altered DA neurons to get sensitive to oxidative stress damage (Lastres-Becker et al., 2016). It was shown that the progress of PD is linked to an incomplete activation of Nrf2 (Vargas et al., 2006).
Conclusion
During past decades many research groups provided new information to better understand the molecular aspects of cancerogenesis and neurodegenerative diseases. Protein kinases play an important role in the regulation of the activity of a huge amount of proteins involved in the control of different cell functions. Nevertheless, in many cases of NDDs, protein aggregation often caused by (hyper-)phosphorylation is observed. Therefore, the inhibition of these reactions is a promising therapeutic target. Unfortunately, whereas for the treatment of cancers several compounds were successfully developed, there does not exist a therapy for NDDs being a kinase inhibitor. Until September 2021, 73 small molecule kinase inhibitors were approved by FDA but only a small amount of them are for noncancer-related diseases (Ayala-Aguilera et al., 2022). The main obstacle in the design of substances targeting the CNS is the effective crossing of the blood-brain-barrier which is necessary in the treatment of NDDs, but also in the case of oncology. CK1 superfamily and CK2 play essential roles in the regulation of cell processes, like in signaling pathways. With respect to this fact, it is not surprising that their deregulation might be associated to numerous disorders, e.g., inflammations, cancer, and NDDs. The starting point for CK1 inhibitors could be described as poorly selective and weakly potent molecules necessary to be improved for application in pharmacological treatment. Subsequently, compounds were developed, which show significant preference between the functionally different CK1 isoforms. Noteworthy, Pfizer designed two ATP-competitive compounds (PF-4800567 and PF-0670462), which possess selectivity towards the CK1δ and CK1ε isoforms. TG Therapeutics discovered umbralisib (UKONIQ™), an orally available dual inhibitor for PI3Kδ and CK1ε applied in the treatment of adults with relapsed or refractory marginal zone lymphoma, which received its FDA approval in 2021 (Burris et al., 2018). Despite this, there are no CK1 inhibitors reaching the clinical stage in neurodegenerative disorders. Those first successes raise the hope for the design of more selective and potent inhibitors of CK1 isoforms to improve the therapeutic opportunities.
In the case of TTBK1/2, up to date, only a small amount of molecules are known, which show potent inhibitory activity towards TTBK1/2. The undisputable advantage of TTBK1 over other kinases is its specific expression in neurons, and therefore, it seems to be a favorable target for NDDs.
Many kinds of CK2 inhibitors have been reported by using different methods, e.g., computer-aided drug design or structure-based reconstitution. Most of them lack cell permeability, high selectivity, but possess off-target potential. The latter might be explained by the fact that this kinase phosphorylates a huge amount of protein substrates. The principle characteristics for a satisfactory molecule are, furthermore, metabolic stability and a good pharmacokinetic profile. Even the best compound CX-4945, already in clinical use, is not devoid of unspecific effects. Nevertheless, the number of newly developed inhibitors (GO289, SGC-CK2-1, and the SRPIN803-rev derivatives), may increase the chance of developing highly selective and CNS penetrating molecules for CK2 in the near future.
Author contributions
AB and RS conceived, wrote, and submitted this manuscript.
Conflict of interest
The authors declare that the research was conducted in the absence of any commercial or financial relationships that could be construed as a potential conflict of interest.
Publisher’s note
All claims expressed in this article are solely those of the authors and do not necessarily represent those of their affiliated organizations, or those of the publisher, the editors and the reviewers. Any product that may be evaluated in this article, or claim that may be made by its manufacturer, is not guaranteed or endorsed by the publisher.
References
Ackermann, K., Neidhart, T., Gerber, J., Waxmann, A., and Pyerin, W. (2005). The catalytic subunit α′ gene of human protein kinase CK2 (CSNK2A2): Genomic organization, promoter identification and determination of Ets1 as a key regulator. Mol. Cell. Biochem. 274, 91–101. doi:10.1007/s11010-005-3076-2
Adler, P., Mayne, J., Walker, K., Ning, Z., and Figeys, D. (2019). Therapeutic targeting of casein kinase 1δ/ε in an Alzheimer’s disease mouse model. J. Proteome Res. 18, 3383–3393. doi:10.1021/acs.jproteome.9b00312
Ahmed, T., Javed, S., Javed, S., Tariq, A., Šamec, D., Tejada, S., et al. (2017). Resveratrol and Alzheimer’s disease: Mechanistic insights. Mol. Neurobiol. 54, 2622–2635. doi:10.1007/s12035-016-9839-9
Akter, R., Rahman, H., Behl, T., Chowdhury, M. A. R., Manirujjaman, M., Bulbul, I. J., et al. (2021). Prospective role of polyphenolic compounds in the treatment of neurodegenerative diseases. CNS Neurol. Disord. Drug Targets 20, 430–450. doi:10.2174/1871527320666210218084444
Alquezar, C., Salado, I. G., de la Encarnación, A., Pérez, D. I., Moreno, F., Gil, C., et al. (2016). Targeting TDP-43 phosphorylation by casein kinase-1δ inhibitors: A novel strategy for the treatment of frontotemporal dementia. Mol. Neurodegener. 11, 36. doi:10.1186/s13024-016-0102-7
Andrew, S. E., Goldberg, Y. P., Kremer, B., Telenius, H., Theilmann, J., Adam, S., et al. (1993). The relationship between trinucleotide (CAG) repeat length and clinical features of Huntington’s disease. Nat. Genet. 4, 398–403. doi:10.1038/ng0893-398
Arai, T., Hasegawa, M., Akiyama, H., Ikeda, K., Nonaka, T., Mori, H., et al. (2006). TDP-43 is a component of ubiquitin-positive tau-negative inclusions in frontotemporal lobar degeneration and amyotrophic lateral sclerosis. Biochem. Biophys. Res. Commun. 351, 602–611. doi:10.1016/j.bbrc.2006.10.093
Ardito, F., Giuliani, M., Perrone, D., Troiano, G., and Muzio, L. L. (2017). The crucial role of protein phosphorylation in cell signaling and its use as targeted therapy (Review). Int. J. Mol. Med. 40, 271–280. doi:10.3892/ijmm.2017.3036
Arenas, J., Campos, Y., Ribacoba, R., Martín, M. A., Rubio, J. C., Ablanedo, P., et al. (1998). Complex I defect in muscle from patients with Huntington’s disease. Ann. Neurol. 43, 397–400. doi:10.1002/ana.410430321
Atwal, R. S., Desmond, C. R., Caron, N., Maiuri, T., Xia, J., Sipione, S., et al. (2011). Kinase inhibitors modulate huntingtin cell localization and toxicity. Nat. Chem. Biol. 7, 453–460. doi:10.1038/nchembio.582
Augustinack, J. C., Schneider, A., Mandelkow, E. M., and Hyman, B. T. (2002). Specific tau phosphorylation sites correlate with severity of neuronal cytopathology in Alzheimer’s disease. Acta Neuropathol. 103, 26–35. doi:10.1007/s004010100423
Avraham, E., Rott, R., Liani, E., Szargel, R., and Engelender, S. (2007). Phosphorylation of parkin by the cyclin-dependent kinase 5 at the linker region modulates its ubiquitin-ligase activity and aggregation. J. Biol. Chem. 282, 12842–12850. doi:10.1074/jbc.M608243200
Ayala, Y. M., Zago, P., D’Ambrogio, A., Xu, Y.-F., Petrucelli, L., Buratti, E., et al. (2008). Structural determinants of the cellular localization and shuttling of TDP-43. J. Cell Sci. 121, 3778–3785. doi:10.1242/jcs.038950
Ayala-Aguilera, C. C., Valero, T., lvaro Lorente-Macías, Á., Baillache, D. J., Croke, S., and Unciti-Broceta, A. (2022). Small molecule kinase inhibitor drugs (1995–2021): Medical indication, pharmacology, and synthesis. J. Med. Chem. 65, 1047–1131. doi:10.1021/acs.jmedchem.1c00963
Aziz, N. A., van der Burg, J. M. M., Landwehrmeyer, G. B., Brundin, P., and Stijnen, T.EHDI Study Groupet al. (2008). Weight loss in Huntington disease increases with higher CAG repeat number. Neurology 71, 1506–1513. doi:10.1212/01.wnl.0000334276.09729.0e
Badura, L., Swanson, T., Adamowicz, W., Adams, J., Cianfrogna, J., Fisher, K., et al. (2007). An inhibitor of casein kinase I induces phase delays in circadian rhythms under free-running and entrained conditions. J. Pharmacol. Exp. Ther. 322, 730–738. doi:10.1124/jpet.107.122846
Baier, A., Galicka, A., Nazaruk, J., and Szyszka, R. (2017). Selected flavonoid compounds as promising inhibitors of protein kinase CK2α and CK2α’, the catalytic subunits of CK2. Phytochemistry 136, 39–45. doi:10.1016/j.phytochem.2016.12.018
Baier, A., Nazaruk, J., Galicka, A., and Szyszka, R. (2018). Inhibitory influence of natural flavonoids on human protein kinase CK2 isoforms: Effect of the regulatory subunit. Mol. Cell. Biochem. 444, 35–42. doi:10.1007/s11010-017-3228-1
Baier, A., and Szyszka, R. (2020). Compounds from natural sources as protein kinase inhibitors. Biomolecules 10 (11), 1546. doi:10.3390/biom10111546
Bailly, C. (2015). Anticancer properties of lamellarins. Mar. Drugs 13, 1105–1123. doi:10.3390/md13031105
Bain, J., Plater, L., Elliott, M., Shpiro, N., Hastie, C. J., McLauchlan, H., et al. (2007). The selectivity of protein kinase inhibitors: A further update. Biochem. J. 408, 297–315. doi:10.1042/BJ20070797
Ball, B. J., Stein, A. S., Borthakur, G., Murray, C., Kook, K., Chan, K. W. H., et al. (2020). Trial in progress: A phase I trial of BTX-A51 in patients with relapsed or refractory aml or high-risk mds. Blood 136, 18–19. doi:10.1182/blood-2020-142557
Baluchnejadmojarad, T., Rabiee, N., Zabihnejad, S., and Roghani, M. (2017). Ellagic acid exerts protective effect in intrastriatal 6-hydroxydopamine rat model of Parkinson’s disease: Possible involvement of ERβ/Nrf2/HO-1 signaling. Brain Res. 1662, 23–30. doi:10.1016/j.brainres.2017.02.021
Bao, C., Bajrami, B., Marcotte, D. J., Chodaparambil, J. V., Kerns, H. M., Henderson, J., et al. (2021). Mechanisms of regulation and diverse activities of tau-tubulin kinase (TTBK) isoforms. Cell. Mol. Neurobiol. 41, 669–685. doi:10.1007/s10571-020-00875-6
Barmada, S. J., Skibinski, G., Korb, E., Rao, E. J., Wu, J. Y., and Finkbeiner, S. (2010). Cytoplasmic mislocalization of TDP-43 is toxic to neurons and enhanced by a mutation associated with familial amyotrophic lateral sclerosis. J. Neurosci. 30, 639–649. doi:10.1523/jneurosci.4988-09.2010
Baunbæk, D., Trinkler, N., Ferandin, Y., Lozach, O., Ploypradith, P., Rucirawat, S., et al. (2008). Anticancer alkaloid lamellarins inhibit protein kinases. Mar. Drugs 6, 514–527. doi:10.3390/md20080026
Benn, C. L., and Dawson, L. A. (2020). Clinically precedented protein kinases: Rationale for their use in neurodegenerative disease. Front. Aging Neurosci. 12, 242. doi:10.3389/fnagi.2020.00242
Bibian, M., Rahaim, R. J., Choi, J. Y., Noguchi, Y., Schürer, S., Chen, W., et al. (2013). Development of highly selective casein kinase 1δ/1ε (CK1δ/ε) inhibitors with potent antiproliferative properties. Bioorg. Med. Chem. Lett. 23, 4374–4380. doi:10.1016/j.bmcl.2013.05.075
Bichelmeier, U., Schmidt, T., Hübener, J., Boy, J., Rüttiger, L., Häbig, K., et al. (2007). Nuclear localization of ataxin-3 is required for the manifestation of symptoms in SCA3: In vivo evidence. J. Neurosci. 27, 7418–7428. doi:10.1523/JNEUROSCI.4540-06.2007
Binder, L. I., Frankfurter, A., and Rebhun, L. I. (1985). The distribution of tau in the mammalian central nervous system. J. Cell Biol. 101, 1371–1378. doi:10.1083/jcb.101.4.1371
Bingham, E. W., and Farrel, H. M. (1974). Casein kinase from the Golgi apparatus of lactating mam mary gland. J. Biol. Chem. 249, 3647–3651. doi:10.1016/S0021-9258(19)42622-7
Bischof, J., Leban, J., Zaja, M., Grothey, A., Radunsky, B., Othersen, O., et al. (2012). 2-Benzamido-N-(1H-benzo[d]imidazol-2-yl)thiazole-4-carboxamide derivatives as potent ihnhibitors CK1δ/ε. Amino Acids 43, 1577–1591. doi:10.1007/s00726-012-1234-x
Blanquet, P. R. (2000). Casein kinase 2 as a potentially important enzyme in the nervous system. Prog. Neurobiol. 60, 211–246. doi:10.1016/s0301-0082(99)00026-x
Borgo, C., Cesaro, L., Hirota, T., Kuwata, K., D’Amore, C., Ruppert, T., et al. (2021a). Comparing the efficacy and selectivity of CK2 inhibitors. A phosphoproteomics approach. Eur. J. Med. Chem. 214, 113217. doi:10.1016/j.ejmech.2021.113217
Borgo, C., D’Amore, C., Sarno, S., Salvi, M., and Ruzzene, M. (2021b). Protein kinase CK2: A potential therapeutic target for diverse human diseases. Signal Transduct. Target. Ther. 6, 183. doi:10.1038/s41392-021-00567-7
Bouskila, M., Esoof, N., Gay, L., Fang, E. H., Deak, M., Begley, M. J., et al. (2011). TTBK2 kinase substrate specificity and the impact of spinocerebellar-ataxia-causing mutations on expression, activity, localization and development. Biochem. J. 437, 157–167. doi:10.1042/bj20110276
Bowie, E., and Goetz, S. C. (2020). TTBK2 and primary cilia are essential for the connectivity and survival of cerebellar Purkinje neurons. Elife 9, e51166. doi:10.7554/eLife.51166
Boyarko, B., and Hook, V. (2021). Human tau isoforms and proteolysis for production of toxic tau fragments in neurodegeneration. Front. Neurosci. 15, 702788. doi:10.3389/fnins.2021.702788
Braak, H., and Braak, E. (1991). Neuropathological stageing of Alzheimer-related changes. Acta Neuropathol. 82, 239–259. doi:10.1007/BF00308809
Braggin, J. E., Bucks, S. A., Course, M. M., Smith, C. L., Sopher, B., Osnis, L., et al. (2019). Alternative splicing in a presenilin 2 variant associated with Alzheimer disease. Ann. Clin. Transl. Neurol. 6, 762–777. doi:10.1002/acn3.755
Buontempo, F., Orsini, E., Lonetti, A., Capellini, A., Chiarini, F., Evangelisti, C., et al. (2016). Synergistic cytotoxic effects of bortezomib and CK2 inhibitor CX-4945 in acute lymphoblastic leukemia: Turning off the prosurvival ER chaperone BIP/Grp78 and turning on the pro-apoptotic NF-κB. Oncotarget 7, 1323–1340. doi:10.18632/oncotarget.6361
Burnett, B., Li, F., and Pittman, R. N. (2003). The polyglutamine neurodegenerative protein ataxin-3 binds polyubiquitylated proteins and has ubiquitin protease activity. Hum. Mol. Genet. 12, 3195–3205. doi:10.1093/hmg/ddg344
Burnett, G., and Kennedy, E. P. (1954). The enzymatic phosphorylation of proteins. J. Biol. Chem. 211, 969–980. doi:10.1016/S0021-9258(18)71184-8
Burré, J., Sharma, M., and Südhof, T. C. (2018). Cell biology and pathophysiology of α-synuclein. Cold Spring Harb. Perspect. Med. 8, a024091. doi:10.1101/cshperspect.a024091
Burris, H. A., Flinn, I. W., Patel, M. R., Fenske, T. S., Deng, C., Brander, D. M., et al. (2018). Umbralisib, a novel PI3Kδ and casein kinase-1ε inhibitor, in relapsed or refractory chronic lymphocytic leukaemia and lymphoma: An open-label, phase 1, dose-escalation, first-in-human study. Lancet. Oncol. 19, 486–496. doi:10.1016/s1470-2045(18)30082-2
Burzio, V., Antonelli, M., Allende, C. C., and Allende, J. E. (2002). Biochemical and cellular characteristics of the four splice variants of protein kinase CK1alpha from zebrafish (Danio rerio). J. Cell. Biochem. 86, 805–814. doi:10.1002/jcb.10263
Caltagirone, C., Cisari, C., Schievano, C., Di Paola, R., Cordaro, M., Bruschetta, G., et al. (2015). Co-Ultramicronized palmitoylethanolamide/luteolin in the treatment of cerebral ischemia: From rodent to man. Transl. Stroke Res. 7, 54–69. doi:10.1007/s12975-015-0440-8
Carter, B., Justin, H. S., Gulick, D., and Gamsby, J. J. (2021). The molecular clock and neurodegenerative disease: A stressful time. Front. Mol. Biosci. 8, 644747. doi:10.3389/fmolb.2021.644747
Castello, J., Ragnauth, A., Friedman, E., and Rebholz, H. (2017). CK2—an emerging target for neurological and psychiatric disorders. Pharmaceuticals 10, 7. doi:10.3390/ph10010007
Chakraborty, J., Basso, V., and Ziviani, E. (2017). Post translational modification of Parkin. Biol. Direct 12, 6. doi:10.1186/s13062-017-0176-3
Chen, C., Gu, J., Basurto-Islas, G., Jin, N., Wu, F., Gong, C.-X., et al. (2017). Up-regulation of casein kinase 1ε is involved in tau pathogenesis in Alzheimer’s disease. Sci. Rep. 7, 13478. doi:10.1038/s41598-017-13791-5
Chen, G.-F., Xu, T.-H., Yan, Y., Zhou, Y.-R., Jiang, Y., Melcher, K., et al. (2017). Amyloid beta: Structure, biology and structure-based therapeutic development. Acta Pharmacol. Sin. 38, 1205–1235. doi:10.1038/aps.2017.28
Cheong, J. K., Hung, N. T., Wang, H., Tan, P., Voorhoeve, P. M., Lee, S. H., et al. (2011). IC261 induces cell cycle arrest and apoptosis of human cancer cells via CK1δ/ɛ and Wnt/β-catenin independent inhibition of mitotic spindle formation. Oncogene 30, 2558–2569. doi:10.1038/onc.2010.627
Chohan, M. O., Khatoon, S., Iqbal, I.-G., and Iqbal, K. (2006). Involvement of I2PP2A in the abnormal hyperphosphorylation of tau and its reversal by Memantine. FEBS Lett. 580, 3973–3979. doi:10.1016/j.febslet.2006.06.021
Chon, H. J., Bae, K. J., Lee, Y., and Kim, J. (2015). The casein kinase 2 inhibitor, CX-4945, as an anti-cancer drug in treatment of human hematological malignancies. Front. Pharmacol. 6, 70. doi:10.3389/fphar.2015.00070
Citron, M., Diehl, T. S., Gordon, G., Biere, A. L., Seubert, P., and Selkoe, D. J. (1996). Evidence that the 42- and 40-amino acid forms of amyloid β protein are generated from the β-amyloid precursor protein by different protease activities. Proc. Natl. Acad. Sci. U. S. A. 93, 13170–13175. doi:10.1073/pnas.93.23.13170
Cohen, P. (2002). The origins of protein phosphorylation. Nat. Cell Biol. 4, E127–E130. doi:10.1038/ncb0502-e127
Conway, K. A., Harper, J. D., and Lansbury, P. T. (1998). Accelerated in vitro fibril formation by a mutant alpha-synuclein linked to early-onset Parkinson disease. Nat. Med. 4, 1318–1320. doi:10.1038/3311
Cookson, M. R., Lockhart, P. J., McLendon, C., O’Farrell, C., Schlossmacher, M., and Farrer, M. J. (2003). RING finger 1 mutations in parkin produce altered localization of the protein. Hum. Mol. Genet. 12, 2957–2965. doi:10.1093/hmg/ddg328
Cornett, J., Cao, F., Wang, C. E., Ross, C. A., Bates, G. P., Li, S. H., et al. (2005). Polyglutamine expansion of huntingtin impairs its nuclear export. Nat. Genet. 37, 198–204. doi:10.1038/ng1503
Costa, P. S. D., Ramos, P. S., Fereira, C., Silva, J. L., El-Baha, T., and Fialho, E. (2021). Pro-oxidant efeect of resveratrol on human breast cancer MCF-7 cells is associated with CK2 inhibition. Nutr. Cancer 14, 1–10. doi:10.1080/016355581.2021.1977834
Cozza, G., Bonvini, P., Zorzi, E., Poletto, G., Pagano, M. A., Sarno, S., et al. (2006). Identification of ellagic acid as potent inhibitor of protein kinase CK2: A successful example of a virtual screening application. J. Med. Chem. 49, 2363–2366. doi:10.1021/jm060112m
Cozza, G., Gianoncelli, A., Montopoli, M., Caparrotta, L., Venerando, A., Meggio, F., et al. (2008). Identification of novel protein kinase CK1 delta (CK1delta) inhibitors through structure-based virtual screening. Bioorg. Med. Chem. Lett. 18, 5672–5675. doi:10.1016/j.bmcl.2008.08.072
Cozza, G., Mazzorana, M., Papinutto, E., Bain, J., Elliott, M., di Maira, G., et al. (2009). Quinalizarin as a potent, selective and cell-permeable inhibitor of protein kinase CK2. Biochem. J. 421, 387–395. doi:10.1042/bj20090069
Cozza, G., and Pinna, L. A. (2016). Casein kinases as potential therapeutic targets. Expert Opin. Ther. Targets 20, 319–340. doi:10.1517/14728222.2016.1091883
Cozza, G., Pinna, L. A., and Moro, S. (2012). Protein kinase CK2 inhibitors: A patent review. Expert Opin. Ther. Pat. 22, 1081–1097. doi:10.1517/13543776.2012.717615
Cozza, G., Venerando, A., Sarno, S., and Pinna, L. A. (2015). The selectivity of CK2 inhibitor quinalizarin: A reevaluation. Biomed. Res. Int. 2015, 734127. doi:10.1155/2015/734127
Cunningham, P. S., Ahern, S. A., Smith, L. C., da Silva Santos, C. S., Wager, T. T., and Bechtold, D. A. (2016). Targeting of the circadian clock via CK1δ/ε to improve glucose homeostasis in obesity. Sci. Rep. 6, 29983. doi:10.1038/srep29983
Daily, J. W., Kang, S., and Park, S. (2021). Protection against Alzheimer's disease by luteolin: Role of brain glucose regulation, anti-inflammatory activity, and the gut microbiota-liver-brain axis. Biofactors 47, 218–231. doi:10.1002/biof.1703
Dalle Vedove, A., Zonta, F., Zanforlin, E., Demitri, N., Ribaudo, G., Cazzanelli, G., et al. (2020). A novel class of selective CK2 inhibitors targeting its open hinge conformation. Eur. J. Med. Chem. 195, 112267. doi:10.1016/j.ejmech.2020.112267
De Wit, T., Baekelandt, V., and Lobbestael, E. (2018). Inhibition of LRRK2 or casein kinase 1 results in LRRK2 protein destabilization. Mol. Neurobiol. 56, 5273–5286. doi:10.1007/s12035-018-1449-2
Desmond, C. R., Atwal, R. S., Xia, J., and Truant, R. (2012). Identification of a karyopherin β1/β2 proline-tyrosine nuclear localization signal in huntingtin protein. J. Biol. Chem. 287, 39626–39633. doi:10.1074/jbc.M112.412379
Dheen, S. T., Jun, Y., Yan, Z., Tay, S. S. W., and Ang Ling, E. (2005). Retinoic acid inhibits expression of TNF-α and iNOS in activated rat microglia. Glia 50, 21–31. doi:10.1002/glia.20153
Dillon, G. M., Henderson, J. L., Bao, C., Joyce, J. A., Calhoun, M., Amaral, B., et al. (2020). Acute inhibition of the CNS-specific kinase TTBK1 significantly lowers tau phosphorylation at several disease relevant sites. PLOS ONE 15 (4), e0228771. doi:10.1371/journal.pone.0228771
Domańska, K., Zieliński, R., Kubiński, K., Sajnaga, E., Masłyk, M., Bretner, M., et al. (2005). Different properties of four molecular forms of protein kinase CK2 from Saccharomyces cerevisiae. Acta Biochim. Pol. 52, 947–952. doi:10.18388/abp.2005_3413
Duan, G., and Walther, D. (2015). The roles of post-translational modifications in the context of protein interaction networks. PLoS Comput. Biol. 11, e1004049. doi:10.1371/journal.pcbi.1004049
Ebrahimi, R., Sepand, M. R., Seyednejad, S. A., Omidi, A., Akbariani, M., Gholami, M., et al. (2019). Ellagic acid reduces methotrexate-induced apoptosis and mitochondrial dysfunction via up-regulating Nrf2 expression and inhibiting the IĸBα/NFĸB in rats. DARU 27, 721–733. doi:10.1007/s40199-019-00309-9
El-Agnaf, O. M., Jakes, R., Curran, M. D., and Wallace, A. (1998). Effects of the mutations Ala30 to Pro and Ala53 to Thr on the physical and morphological properties of α-synuclein protein implicated in Parkinson’s disease. FEBS Lett. 440, 67–70. doi:10.1016/s0014-5793(98)01419-7
Ellis, C. E., Schwartzberg, P. L., Grider, T. L., Fink, D. W., and Nussbaum, R. L. (2001). alpha-synuclein is phosphorylated by members of the Src family of protein-tyrosine kinases. J. Biol. Chem. 276, 3879–3884. doi:10.1074/jbc.M010316200
Evert, B. O., Araujo, J., Vieira-Saecker, A. M., de Vos, R. A., Harendza, S., Klockgether, T., et al. (2006). Ataxin-3 represses transcription via chromatin binding, interaction with histone deacetylase 3, and histone deacetylation. J. Neurosci. 26, 11474–11486. doi:10.1523/JNEUROSCI.2053-06.2006
Evin, G., Smith, M. J., Tziotis, A., McLean, C., Canterford, L., Sharples, R. A., et al. (2002). Alternative transcripts of presenilin-1 associated with frontotemporal dementia. Neuroreport 13, 917–921. doi:10.1097/00001756-200205070-00036
Farkhondeh, T., Folgado, S. L., Pourbagher-Shahri, A. M., Ashrafizadeh, M., and Samarghandian, S. (2020). The therapeutic effect of resveratrol: Focusing on the Nrf2 signaling pathway. Biomed. Pharmacother. 127, 110234. doi:10.1016/j.biopha.2020.110234
Flajolet, M., He, G., Heiman, M., Lin, A., Nairn, A. C., and Greengard, P. (2007). Regulation of Alzheimer’s disease amyloid-beta formation by casein kinase I. Proc. Natl. Acad. Sci. U. S. A. 104, 4159–4164. doi:10.1073/pnas.0611236104
Flotow, H., and Roach, P. J. (1991). Role of acidic residues as substrate determinants for casein kinase I. J. Biol. Chem. 266, 3724–3727. doi:10.1016/S0021-9258(19)67854-3
Franchin, C., Cesaro, L., Salvi, M., Millioni, R., Iori, E., Cifani, P., et al. (2015). Quantitative analysis of a phosphoproteome readily altered by the protein kinase CK2 inhibitor quinalizarin in HEK-293T cells. Biochim. Biophys. Acta 1854, 609–623. doi:10.1016/j.bbapap.2014.09.017
Fujiwara, H., Hasegawa, M., Dohmae, N., Kawashima, A., Masliah, E., Goldberg, M. S., et al. (2002). Alpha-Synuclein is phosphorylated in synucleinopathy lesions. Nat. Cell Biol. 4, 160–164. doi:10.1038/ncb748
Fukuda, T., Ishibashi, F., and Iwao, M. (2020). Lamellarin alkaloids: Isolation, synthesis, and biological activity. Alkaloids. Chem. Biol. 83, 1–112. doi:10.1016/bs.alkal.2019.10.001
Fulcher, L. J., and Sapkota, G. P. (2020). Functions and regulation of the serine/threonine protein kinase CK1 family: Moving beyond promiscuity. Biochem. J. 477, 4603–4621. doi:10.1042/BCJ20200506
Gai, W. P., Power, J. H., Blumbergs, P. C., and Blessing, W. W. (1998). Multiple-system atrophy: A new a-synuclein disease? Lancet 352, 547–548. doi:10.1016/s0140-6736(05)79256-4
García-Reyes, B., Witt, L., Jansen, B., Karasu, E., Gehring, T., Leban, J., et al. (2018). Discovery of inhibitor of Wnt production 2 (IWP-2) and related compounds as selective ATP-competitive inhibitors of casein kinase 1 (CK1) δ/ε. J. Med. Chem. 61, 4087–4102. doi:10.1021/acs.jmedchem.8b00095
Geuens, T., Bouhy, D., and Timmerman, V. (2016). The hnRNP family: Insights into their role in health and disease. Hum. Genet. 135, 851–867. doi:10.1007/s00439-016-1683-5
Ghoshal, N., Smiley, J. F., DeMaggio, A. J., Hoekstra, M. F., Cochran, E. J., Binder, L. I., et al. (1999). A new molecular link between the fibrillar and granulovacuolar lesions of Alzheimer’s disease. Am. J. Pathol. 155, 1163–1172. doi:10.1016/s0002-9440(10)65219-4
Glenner, G. G., and Wong, C. W. (1984). Alzheimer’s disease: Initial report of the purification and characterization of a novel cerebrovascular amyloid protein. Biochem. Biophys. Res. Commun. 120, 885–890. doi:10.1016/s0006-291x(84)80190-4
Golub, A. G., Bdzhola, V. G., Briukhovetska, N. V., Balanda, A. O., Kukharenko, O. P., Kotey, I. M., et al. (2011). Synthesis and biological evaluation of substituted (thieno[2, 3-d]pyrimidin-4-ylthio)carboxylic acids as inhibitors of human protein kinase CK2. Eur. J. Med. Chem. 46, 870–876. doi:10.1016/j.ejmech.2010.12.025
Gomes, B. A. Q., Silva, J. P. B., Romeiro, C. F. R., dos Santos, S. M., Rodrigues, C. A., Gonçalves, P. R., et al. (2018). Neuroprotective mechanisms of resveratrol in Alzheimer’s disease: Role of SIRT1. Oxid. Med. Cell. Longev. 2018, 8152373. doi:10.1155/2018/8152373
Gong, C.-X., and Iqbal, K. (2008). Hyperphosphorylation of microtubule-associated protein tau: A promising therapeutic target for alzheimer disease. Curr. Med. Chem. 15, 2321–2328. doi:10.2174/092986708785909111
Gong, C.-X., Singh, T. J., Grundke-Iqbal, I., and Iqbal, K. (1993). Phosphoprotein phosphatase activities in Alzheimer disease brain. J. Neurochem. 61, 921–927. doi:10.1111/j.1471-4159.1993.tb03603.x
Greenwood, J. A., Scott, C. W., Spreen, R. C., Caputo, C. B., and Johnson, G. V. (1994). Casein kinase II preferentially phosphorylates human tau isoforms containing an amino-terminal insert. Identification of threonine 39 as the primary phosphate acceptor. J. Biol. Chem. 269, 4373–4380. doi:10.1016/S0021-9258(17)41790-x
Grundke-Iqbal, I., Iqbal, K., Tung, Y. C., Quinlan, M., Wisniewski, H. M., and Binder, L. I. (1986). Abnormal phosphorylation of the microtubule-associated protein tau (tau) in Alzheimer cytoskeletal pathology. Proc. Natl. Acad. Sci. U. S. A. 83, 4913–4917. doi:10.1073/pnas.83.13.4913
Guo, D.-J., Li, F., Yu, P. H.-F., and Chan, S.-W. (2013). Neuroprotective effects of luteolin against apoptosis induced by 6-hydroxydopamine on rat pheochromocytoma PC12 cells. Pharm. Biol. 51, 190–196. doi:10.3109/13880209.2012.716852
Hackam, A. S., Singaraja, R., Wellington, C. L., Metzler, M., McCutcheon, K., Zhang, T., et al. (1998). The influence of huntingtin protein size on nuclear localization and cellular toxicity. J. Cell Biol. 141, 1097–1105. doi:10.1083/jcb.141.5.1097
Haidar, S., Aichele, D., Birus, R., Hielscher, J., Laitinen, T., Poso, A., et al. (2019). In vitro and in silico evaluation of bikaverin as a potent inhibitor of human protein kinase CK2. Molecules 24, 1380. doi:10.3390/molecules24071380
Halekotte, J., Witt, L., Ianes, C., Krüger, M., Bührmann, M., Rauh, D., et al. (2017). Optimized 4, 5-diarylimidazoles as potent/selective inhibitors of protein kinase CK1δ and their structural relation to p38α MAPK. Molecules 22, 522. doi:10.3390/molecules22040522
Halkina, T., Henderson, J. L., Lin, E. Y., Himmelbauer, M. K., Jones, J. H., Nevalainen, M., et al. (2021). Discovery of potent and brain-penetrant tau tubulin kinase 1 (TTBK1) inhibitors that lower tau phosphorylation in vivo. J. Med. Chem. 64, 6358–6380. doi:10.1021/acs.jmedchem.1c00382
Hampe, C., Ardila-Osorio, H., Fournier, M., Brice, A., and Corti, O. (2006). Biochemical analysis of Parkinson’s disease-causing variants of parkin, an E3 ubiquitin-protein ligase with monoubiquitylation capacity. Hum. Mol. Genet. 15, 2059–2075. doi:10.1093/hmg/ddl131
Hanger, D. P., Anderton, B. H., and Noble, W. (2009). Tau phosphorylation: The therapeutic challenge for neurodegenerative disease. Trends Mol. Med. 15, 112–119. doi:10.1016/j.molmed.2009.01.003
Hanger, D. P., Byers, H. L., Wray, S., Leung, K.-Y., Saxton, M. J., Seereeram, A., et al. (2007). Novel phosphorylation sites in tau from alzheimer brain support a role for casein kinase 1 in disease pathogenesis. J. Biol. Chem. 282, 23645–23654. doi:10.1074/jbcM703269200
Hanks, S. K., and Hunter, T. (1995). The eukaryotic protein kinase superfamily: Kinase (catalytic) domain structure and classification 1. FASEB J. 9, 576–596. doi:10.1096/fasebj.9.8.7768349
Hardy, J. A., and Higgins, G. A. (1992). Alzheimer’s disease: The amyloid cascade hypothesis. Science 1256, 184–185. doi:10.1126/science.1566067
Hasegawa, M., Arai, T., Nonaka, T., Kametani, F., Yoshida, M., Hashizume, Y., et al. (2008). Phosphorylated TDP-43 in frontotemporal lobar degeneration and amyotrophic lateral sclerosis. Ann. Neurol. 64, 60–70. doi:10.1002/Ana.21425
Hathaway, G. M., and Traugh, J. A. (1979). Cyclic nucleotide-independent protein kinases from rabbit reticulocytes. Purification of casein kinases. J. Biol. Chem. 254, 762–768. doi:10.1016/S0021-9258(17)37871-7
Hinojosa-Ventura, G., Puebla-Pérez, A. M., Gallegos-Arreola, M. P., Chávez-Parga, M.-C., Romero-Estrada, A., and Delgado-Saucedo, J. I. (2019). Cytotoxic and antitumoral effects of bikaverin from Gibberella fujikuroi on L5178Y lymphoma murine model. J. Mex. Chem. Soc. 63, 115–122. doi:10.29356/jmcs.v63i4.729
Houlden, H., Johnson, J., Gardner-Thorpe, C., Lashley, T., Hernandez, D., Worth, P., et al. (2007). Mutations in TTBK2, encoding a kinase implicated in tau phosphorylation, segregate with spinocerebellar ataxia type 11. Nat. Genet. 39, 1434–1436. doi:10.1038/ng.2007.43
Hua, Z., Huang, X., Bregman, H., Chakka, N., DiMauro, E. F., Doherty, E. M., et al. (2012). 2-Phenylamino-6-cyano-1H-benzimidazole-based isoform selective casein kinase 1 gamma (CK1γ) inhibitors. Bioorg. Med. Chem. Lett. 22, 5392–5395. doi:10.1016/j.bmcl.2012.07.046
Hunter, T. (1995). Protein kinases and phosphatases: The yin and yang of protein phosphorylation and signaling. Cell 80, 225–236. doi:10.1016/0092-8674(95)90405-0
Hunter, T. (2012). Why nature chose phosphate to modify proteins. Philos. Trans. R. Soc. Lond. B Biol. Sci. 367, 2513–2516. doi:10.1098/rstb.2012.0013
Ikezu, S., and Ikezu, T. (2014). Tau-tubulin kinase. Front. Mol. Neurosci. 7, 33. doi:10.3389/fnmol.2014.00033
Ikezu, S., Ingraham Dixie, K. L., Koro, L., Watanabe, T., Kaibuchi, K., and Ikezu, T. (2020). Tau-tubulin kinase 1 and amyloid-β peptide induce phosphorylation of collapsin response mediator protein-2 and enhance neurite degeneration in Alzheimer disease mouse models. Acta Neuropathol. Commun. 8, 12. doi:10.1186/s40478-020-0890-4
Inukai, Y., Nonaka, T., Arai, T., Yoshida, M., Hashizume, Y., Beach, T. G., et al. (2008). Abnormal phosphorylation of ser409/410 of TDP-43 in FTLD-U and ALS. FEBS Lett. 582, 2899–2904. doi:10.1016/j.febslet.2008.07.027
Ishii, A., Nonaka, T., Taniguchi, S., Saito, T., Arai, T., Mann, D., et al. (2007). Casein kinase 2 is the major enzyme in brain that phosphorylates Ser129 of human alpha-synuclein: Implication for alpha-synucleinopathies. FEBS Lett. 581, 4711–4717. doi:10.1016/j.febslet.2007.08.067
Iwao, M., Fukuda, T., and Ishibashi, F. (2011). Synthesis and biological activity of lamellarin alkaloids: An overview. HETEROCYCLES 83, 491. doi:10.3987/rev-10-686
Jackson, P. K. (2012). TTBK2 kinase: Linking primary cilia and cerebellar ataxias. Cell 151, 697–699. doi:10.1016/j.cell.2012.10.027
Jameson, L., Frey, T., Zeeberg, B., Dalldorf, F., and Caplow, M. (1980). Inhibition of microtubule assembly by phosphorylation of microtubule-associated proteins. Biochemistry 19, 2472–2479. doi:10.1021/bi00552a027
Jana, S., and Singh, S. K. (2020). Identification of human tau-tubulin kinase 1inhibitors: An integrated e-pharmacophore based virtual screening and molecular dynamics simulation. J. Biomol. Struct. Dyn. 38, 886–900. doi:10.1080/07391102.2019.1590242
Janeczko, M., Orzeszko, A., Kazimierczuk, Z., Szyszka, R., and Baier, A. (2012). CK2α and CK2α′ subunits differ in their sensitivity to 4, 5, 6, 7-tetrabromo- and 4, 5, 6, 7-tetraiodo-1H-benzimidazole derivatives. Eur. J. Med. Chem. 47, 345–350. doi:10.1016/j.ejmech.2011.11.002
Järås, M., Miller, P. G., Chu, L. P., Puram, R. V., Fink, E. C., Schneider, R. K., et al. (2014). Csnk1a1 inhibition has p53-dependent therapeutic efficacy in acute myeloid leukemia. J. Exp. Med. 211, 605–612. doi:10.1084/jem.20131033
Jiang, S., Zhang, M., Sun, J., and Yang, X. (2018). Casein kinase 1α: Biological mechanisms and theranostic potential. Cell Commun. Signal. 16, 23. doi:10.1186/s12964-018-0236-z
Jin, J., and Pawson, T. (2012). Modular evolution of phosphorylation-based signalling systems. Philos. Trans. R. Soc. Lond. B Biol. Sci. 367, 2540–2555. doi:10.1098/rstb.2012.0106
Kametani, F., Nonaka, T., Suzuki, T., Arai, T., Dohmae, N., Akiyama, H., et al. (2009). Identification of casein kinase-1 phosphorylation sites on TDP-43. Biochem. Biophys. Res. Commun. 382, 405–409. doi:10.1016/j.bbrc.2009.03.038
Kang, S. S., Lee, J. Y., Choi, Y. K., Kim, G. S., and Han, B. H. (2004). Neuroprotective effects of flavones on hydrogen peroxide-induced apoptosis in SH-SY5Y neuroblostoma cells. Bioorg. Med. Chem. Lett. 14, 2261–2264. doi:10.1016/j.bmcl.2004.02.003
Kannanayakal, T. J., Tao, H., Vandre, D. D., and Kuret, J. (2006). Casein kinase-1 isoforms differentially associate with neurofibrillary and granulovacuolar degeneration lesions. Acta Neuropathol. 111, 413–421. doi:10.1007/s00401-006-0049-9
Karve, T. M., and Cheema, A. K. (2011). Small changes huge impact: The role of protein posttranslational modifications in cellular homeostasis and disease. J. Amino Acids 2011, 207691. doi:10.4061/2011/207691
Kiefer, S. E., Chang, C. J., Kimura, S. R., Gao, M., Xie, D., Zhang, Y., et al. (2014). The structure of human tau-tubulin kinase 1 both in the apo form and in complex with an inhibitor. Acta Crystallogr. F. Struct. Biol. Commun. 70, 173–181. doi:10.1107/S2053230X14000144
Kim, E. N., Lim, J. H., Kim, M. Y., Ban, T. H., Jang, I., Yoon, H. E., et al. (2018). Resveratrol, an Nrf2 activator, ameliorates aging-related progressive renal injury. Aging (Albany NY) 10, 83–99. doi:10.18632/aging.101361
Kim, H., Choi, K., Kang, H., Lee, S.-Y., Chi, S.-W., Lee, M.-S., et al. (2014). Identification of a novel function of CX-4945 as a splicing regulator. PLoS One 9, e94978. doi:10.1371/journal.pone.0094978
Kim, J. K., and Park, S. U. (2021). Flavonoids for treatment of Alzheimer’s disease: An up todate review. EXCLI J. 20, 495–502. doi:10.17179/excli2021-3492
Knippschild, U., Gocht, A., Wolff, S., Huber, N., Löhler, J., and Stöter, M. (2005). The casein kinase 1 family: Participation in multiple cellular processes in eukaryotes. Cell. Signal. 17, 675–689. doi:10.1016/j.cellsig.2004.12.011
Komorowska, J., Wątroba, M., and Szukiewicz, D. (2020). Review of beneficial effects of resveratrol in neurodegenerative diseases such as Alzheimer’s disease. Adv. Med. Sci. 65, 415–423. doi:10.1016/j.advms.2020.08.002
Kosten, J., Binolfi, A., Stuiver, M., Verzini, S., Theillet, F.-X., Bekei, B., et al. (2014). Efficient modification of alpha-synuclein serine 129 by protein kinase CK1 requires phosphorylation of tyrosine 125 as a priming event. ACS Chem. Neurosci. 5, 1203–1208. doi:10.1021/cn5002254
Kotanidou, A., Xagorari, A., Bagli, E., Kitsanta, P., Fotsis, T., Papapetropoulos, A., et al. (2002). Luteolin reduces lipopolysaccharide-induced lethal toxicity and expression of proinflammatory molecules in mice. Am. J. Respir. Crit. Care Med. 165, 818–823. doi:10.1164/ajrccm.165.6.2101049
Kovacs, G. G., Botond, G., and Budka, H. (2010). Protein coding of neurodegenerative dementias: The neuropathological basis of biomarker diagnostics. Acta Neuropathol. 119, 389–408. doi:10.1007/s00401-010-0658-1
Kumar, A., Singh, C. K., LaVoie, H. A., DiPette, D. J., and Singh, U. S. (2011). Resveratrol Restores Nrf2 Level and Prevents Ethanol-Induced Toxic Effects in the Cerebellum of a Rodent Model of Fetal Alcohol Spectrum Disorders. Mol. Pharmacol. 80, 446–457. doi:10.1124/mol.111.071126
Kwon, Y. (2017). Luteolin as a potential preventive and therapeutic candidate for Alzheimer’s disease. Exp. Gerontol. 95, 39–43. doi:10.1016/j.exger.2017.05.014
LaFerla, F. M., Green, K. N., and Oddo, S. (2007). Intracellular amyloid-β in Alzheimer’s disease. Nat. Rev. Neurosci. 8, 499–509. doi:10.1038/nrn2168
Lasa, M., Marin, O., and Pinna, L. A. (1997). Rat liver Golgi apparatus contains a protein kinase similar to the casein kinase of lactating mam mary gland. Eur. J. Biochem. 243, 719–725. doi:10.1111/j.1432-1033.1997.00719.x
Lastres-Becker, I., García-Yagüe, A. J., Scannevin, R. H., Casarejos, M. J., Kügler, S., Rábano, A., et al. (2016). Repurposing the NRF2 activator dimethyl fumarate as therapy against synucleinopathy in Parkinson’s disease. Antioxid. Redox Signal. 25, 61–77. doi:10.1089/ars.2015.6549
Ławnicka, H., Kowalewicz-Kulbat, M., Sicińska, P., Kazimierczuk, Z., Grieb, P., and Stępień, P. (2010). Anti-neoplastic effect of protein kinase CK2 inhibitor, 2-dimethylamino-4, 5, 6, 7-tetrabromobenzimidazole (DMAT), on growth and hormonal activity of human adrenocortical carcinoma cell line (H295R) in vitro. Cell Tissue Res. 340, 371–379. doi:10.1007/s00441-010-0960-1
Lee, J. W., Hirota, T., Peters, E. C., Garcia, M., Gonzalez, R., Cho, C. Y., et al. (2011). A small molecule modulates circadian rhythms through phosphorylation of the period protein. Angew. Chem. Int. Ed. Engl. 50, 10608–10611. doi:10.1002/anie.201103915
Lee, J. Y., Yun, J.-S., Kim, W.-K., Chun, H.-S., Jin, H., Cho, S., et al. (2019). Structural basis for the selective inhibition of cdc2-like kinases by CX-4945. Biomed. Res. Int. 2019, 6125068. doi:10.1155/2019/6125068
Lee, V. M., Goedert, M., and Trojanowski, J. Q. (2001). Neurodegenerative tauopathies. Annu. Rev. Neurosci. 24, 1121–1159. doi:10.1146/annurev.neuro.24.1.1121
Li, C., Liu, X., Lin, X., and Chen, X. (2009). Structure-activity relationship of 7 flavonoids on recombinant human protein kinase CK2 holoenzyme. Zhong Nan Da Xue Xue Bao Yi Xue Ban. 34, 20–26.
Li, G., Yin, H., and Kurent, J. (2004). Casein kinase 1 delta phosphorylates tau and disrupts its binding to microtubules. J. Biol. Chem. 279, 15938–15945. doi:10.1074/jbc.M314116200
Li, H.-Y., Yeh, P.-A., Chiu, H.-C., Tang, C.-Y., and Tu, B. P.-h. (2011). Hyperphosphorylation as a defense mechanism to reduce TDP-43 aggregation. PLoS One 6, e23075. doi:10.1371/journal.pone.0023075
Li, K., McGee, L. R., Fisher, B., Sudom, A., Liu, J., Rubenstein, S. M., et al. (2013). Inhibiting NF-κB-inducing kinase (NIK): Discovery, structure-based design, synthesis, structure–activity relationship, and co-crystal structures. Bioorg. Med. Chem. Lett. 23, 1238–1244. doi:10.1016/j.bmcl.2013.01.012
Li, M., Makkinje, A., and Damuni, Z. (1996). Molecular identification of I1PP2A, a novel potent heat-stable inhibitor protein of protein phosphatase 2A. Biochemistry 35, 6998–7002. doi:10.1021/bi960581y
Liachko, N. F., McMillan, P. J., Strovas, T. J., Loomis, E., Greenup, L., Murrell, J. R., et al. (2014). The tau tubulin kinases TTBK1/2 promote accumulation of pathological TDP-43. PLoS Genet. 10, e1004803. doi:10.1371/journal.pgen.1004803
Liao, J.-C., Yang, T. T., Weng, R. R., Kuo, C.-T., and Chang, C.-W. (2015). TTBK2: A tau protein kinase beyond tau phosphorylation. Biomed. Res. Int. 2015, 575170. doi:10.1155/2015/575170
Lindwall, G., and Cole, R. D. (1984). Phosphorylation affects the ability of tau protein to promote microtubule assembly. J. Biol. Chem. 259, 5301–5305. doi:10.1016/S0021-9258(17)42989-9
Lippa, C. F., Fujiwara, H., Mann, D. M., Giasson, B., Baba, M., Schmidt, M. L., et al. (1998). Lewy bodies contain altered alpha-synuclein in brains of many familial Alzheimer's disease patients with mutations in presenilin and amyloid precursor protein genes. Am. J. Pathol. 153, 1365–1370. doi:10.1016/s0002-9440(10)65722-7
Litchfield, D. W. (2003). Protein kinase CK2: Structure, regulation and role in cellular decisions of life and death. Biochem. J. 369, 1–15. doi:10.1042/bj20021469
Liu, G., Li, H., Zhang, W., Yu, J., Zhang, X., Wu, R., et al. (2021). Csnk1a1 inhibition modulates the inflammatory secretome and enhances response to radiotherapy in glioma. J. Cell. Mol. Med. 25, 7395–7406. doi:10.1111/jcmm.16767
Liu, J., Yi, L., Xiang, Z., Zhong, J., Zhang, H., and Sun, T. (2015). Resveratrol attenuates spinal cord injury-induced inflammatory damage in rat lungs. Int. J. Clin. Exp. Pathol. 8, 1237–1246.
Liu, Q.-S., Deng, R., Li, S., Li, X., Li, K., Kebaituli, G., et al. (2017). Ellagic acid protects against neuron damage in ischemic stroke through regulating the ratio of Bcl-2/Bax expression. Appl. Physiol. Nutr. Metab. 42, 855–860. doi:10.1139/apnm-2016-0651
Lolli, G., Cozza, G., Mazzorana, M., Tibaldi, E., Cesaro, L., Donella-Deana, A., et al. (2012). Inhibition of protein kinase CK2 by flavonoids and tyrphostins. A structural insight. Biochemistry 51, 6097–6107. doi:10.1021/bi300531c
Lopez-Lazaro, M. (2009). Distribution and biological activities of the flavonoid luteolin. Mini Rev. Med. Chem. 9, 31–59. doi:10.2174/138955709787001712
Lund, H., Cowburn, R. F., Gustafsson, E., Strömberg, K., Svensson, A., Dahllund, L., et al. (2013). Tau-tubulin kinase 1 expression, phosphorylation and Co-localization with phospho-ser422 tau in the Alzheimer’s disease brain. Brain Pathol. 23, 378–389. doi:10.1111/bpa.12001
Lunkes, A., Lindenberg, K. S., Ben-Haїem, L., Weber, C., Devys, D., Landwehrmeyer, G. B., et al. (2002). Proteases acting on mutant huntingtin generate cleaved products that differentially build up cytoplasmic and nuclear inclusions. Mol. Cell 10, 259–269. doi:10.1016/s1097-2765(02)00602-0
Mackenzie, I. R., Rademakers, R., and Neumann, M. (2010). TDP-43 and FUS in amyotrophic lateral sclerosis and frontotemporal dementia. Lancet. Neurol. 9, 995–1007. doi:10.1016/S1474-4422(10)70195-2
Maher, P., Dargusch, R., Bodai, L., Gerard, P. E., Purcell, J. M., and Marsh, J. L. (2010). ERK activation by the polyphenols fisetin and resveratrol provides neuroprotection in multiple models of Huntington’s disease. Hum. Mol. Genet. 20, 261–270. doi:10.1093/hmg/ddq460
Marcon, G., Di Fede, G., Giaccone, G., Rossi, G., Giovagnoli, A. R., Maccagnano, E., et al. (2009). A novel Italian presenilin 2 gene mutation with prevalent behavioral phenotype. J. Alzheimers Dis. 16, 509–511. doi:10.3233/JAD-2009-0986
Marin, O., Meggio, F., Sarno, S., Andretta, M., and Pinna, L. A. (1994). Phosphorylation of synthetic fragments of inhibitor-2 of protein phosphatase-1 by casein kinase-1 and -2. Evidence that phosphorylated residues are not strictly required for efficient targeting by casein kinase-1. Eur. J. Biochem. 223, 647–653. doi:10.1111/j.1432-1033.1994.tb19037.x
Maroteaux, L., Campanelli, J. T., and Scheller, R. H. (1988). Synuclein: A neuron-specific protein localized to the nucleus and presynaptic nerve terminal. J. Neurosci. 8, 2804–2815. doi:10.1523/JNEUROSCI.08-08-02804.1988
Martínez-González, L., Rodríguez-Cueto, C., Cabezudo, D., Bartolomé, F., Andrés-Benito, P., Ferrer, I., et al. (2020). Motor neuron preservation and decrease of in vivo TDP-43 phosphorylation by protein CK-1δ kinase inhibitor treatment. Sci. Rep. 10, 4449. doi:10.1038/s41598-020-61265-y
Mashhoon, N., De Maggio, A. J., Tereshko, V., Bergmeier, S. C., Egli, M., Hoekstra, M. F., et al. (2000). Crystal structure of a conformation-selective casein kinase-1 inhibitor. J. Biol. Chem. 275, 20052–20060. doi:10.1074/jbcM001713200
Mbefo, M. K., Fares, M.-B., Paleologou, K., Oueslati, A., Yin, G., Tenreiro, S., et al. (2015). Parkinson disease mutant E46K enhances α-synuclein phosphorylation in mammalian cell lines, in yeast, and in vivo. J. Biol. Chem. 290, 9412–9427. doi:10.1074/jbc.m114.610774
Meggio, F., Marin, O., and Pinna, L. A. (1994). Substrate specificity of protein kinase CK2. Cell. Mol. Biol. Res. 40, 401–409.
Meggio, F., Perich, J. W., Marin, O., and Pinna, L. A. (1992). The comparative efficiencies of the Ser(P)-Thr(P)- and Tyr(P)-residues as specificity determinants for casein kinase-1. Biochem. Biophys. Res. Commun. 182, 1460–1465. doi:10.1016/0006-291x(92)91898-z
Meggio, F., Perich, J. W., Reynolds, E. C., and Pinna, L. A. (1991). A synthetic β-casein phosphopeptide and analogues as model substrates for casein kinase-1, a ubiquitous, phosphate directed protein kinase. FEBS Lett. 283, 303–306. doi:10.1016/0014-5793(91)80614-9
Meggio, F., and Pinna, L. A. (2003). One-thousand-and-one substrates of protein kinase CK2? FASEB J. 17, 349–368. doi:10.1096/fj.02-0473rev
Meijer, L., Borgne, A., Mulner, O., Chong, J. P., Blow, J. J., Inagaki, N., et al. (1997). Biochemical and cellular effects of roscovitine, a potent and selective inhibitor of the cyclin-dependent kinases cdc2, cdk2 and cdk5. Eur. J. Biochem. 243, 527–536. doi:10.1111/j.1432-1033.1997.t01-2-00527.x
Meijer, L., Thunnissen, A.-M., White, A., Garnier, M., Nikolic, M., Tsai, L.-H., et al. (2000). Inhibition of cyclin-dependent kinases, GSK-3beta and CK1 by hymenialdisine, a marine sponge constituent. Chem. Biol. 7, 51–63. doi:10.1016/s1074-5521(00)00063-6
Meng, Q.-J., Maywood, E. S., Bechtold, D. A., Lu, W.-Q., Li, J., Gibbs, J. E., et al. (2010). Entrainment of disrupted circadian behavior through inhibition of casein kinase 1 (CK1) enzymes. Proc. Natl. Acad. Sci. U. S. A. 107, 15240–15245. doi:10.1073/pnas.1005101107
Menke, R. A., Jbabdi, S., Miller, K. L., Matthews, P. M., and Zarei, M. (2010). Connectivity-based segmentation of the substantia nigra in human and its implications in Parkinson's disease. Neuroimage 52, 1175–1180. doi:10.1016/j.neuroimage.2010.05.086
Mishra, S., Kinoshita, Ch., Axtman, A. D., and Young, J. E. (2022). Evaluation of a selective chemical probe validates that CK2 mediates neuroinflammation in a human induced pluripotent stem cell-derived mircroglial model. Front. Mol. Neurosci. 15, 824956. doi:10.3389/fnmol.2022.824956
Morales-Garcia, J. A., Salado, I. G., Sanz-San Cristobal, M., Gil, C., Pérez-Castillo, A., Martínez, A., et al. (2017). Biological and pharmacological characterization of benzothiazole-based CK-1δ inhibitors in models of Parkinson’s disease. ACS Omega 2, 5215–5220. doi:10.1021/acsomega.7b00869
Morooka, S., Hoshina, M., Kii, I., Okabe, T., Kojima, H., Inoue, N., et al. (2015). Identification of a dual inhibitor of SRPK1 and CK2 that attenuates pathological angiogenesis of macular degeneration in mice. Mol. Pharmacol. 88, 316–325. doi:10.1124/mol.114.097345
Mueller, T., Breuer, P., Schmitt, I., Walter, J., Evert, B. O., and Wüllner, U. (2009). CK2-dependent phosphorylation determines cellular localization and stability of ataxin-3. Hum. Mol. Genet. 18, 3334–3343. doi:10.1093/hmg/ddp274
Murphy, M. P., and LeVine, H. (2010). Alzheimer’s disease and the amyloid-β peptide. J. Alzheimer’s Dis. 19, 311–323. doi:10.3233/jad-2010-1221
Nakagawa, T., and Ohta, K. (2019). Quercetin regulates the integrated stress response to improve memory. Int. J. Mol. Sci. 20, 2761. doi:10.3390/ijms20112761
Nakamura, T., Yamashita, H., Takahashi, T., and Nakamura, S. (2001). Activated Fyn phosphorylates alpha-synuclein at tyrosine residue 125. Biochem. Biophys. Res. Commun. 280, 1085–1092. doi:10.1006/bbrc.2000.4253
Nakielny, S., and Dreyfuss, G. (1997). Nuclear export of proteins and RNAs. Curr. Opin. Cell Biol. 9, 420–429. doi:10.1016/s0955-0674(97)80016-6
Narhi, L., Wood, S. J., Steavenson, S., Jiang, Y., Wu, G. M., Anafi, D., et al. (1999). Both familial Parkinson’s disease mutations accelerate alpha-synuclein aggregation. J. Biol. Chem. 274, 9843–9846. doi:10.1074/jbc.274.14.9843
Negro, A., Brunati, A. M., Donella-Deana, A., Massimino, M. L., and Pinna, L. A. (2002). Multiple phosphorylation of alpha-synuclein by protein tyrosine kinase Syk prevents eosin-induced aggregation. FASEB J. 16, 210–212. doi:10.1096/fj.01-0517fje
Neumann, M., Bentmann, E., Dormann, D., Jawaid, A., DeJesus-Hernandez, M., Ansorge, O., et al. (2011). FET proteins TAF15 and EWS are selective markers that distinguish FTLD with FUS pathology from amyotrophic lateral sclerosis with FUS mutations. Brain 134, 2595–2609. doi:10.1093/brain/awr201
Neumann, M., Sampathu, D. M., Kwong, L. K., Truax, A. C., Micsenyi, M. C., Chou, T. T., et al. (2006). Ubiquitinated TDP-43 in frontotemporal lobar degeneration and amyotrophic lateral sclerosis. Science 314, 130–133. doi:10.1126/science.1134108
Nirmaladevi, D., Venkataramana, M., Chandranayaka, S., Ramesha, A., Jameel, N. M., and Srinivas, C. (2014). Neuroprotective effects of bikaverin on H2O2-induced oxidative stress mediated neuronal damage in SH-SY5Y cell line. Cell. Mol. Neurobiol. 34, 973–985. doi:10.1007/s10571-014-0073-6
Nishi, H., Shaytan, A., and Panchenko, A. R. (2014). Physicochemical mechanisms of protein regulation by phosphorylation. Front. Genet. 5, 270. doi:10.3389/fgene.2014.00270
Nonaka, T., Suzuki, G., Tanaka, Y., Kametani, F., Hirai, S., Okado, H., et al. (2016). Phosphorylation of TAR DNA-binding protein of 43 kDa (TDP-43) by truncated casein kinase 1 delta triggers mislocalization and accumulation of TDP-43. J. Biol. Chem. 291, 5473–5483. doi:10.1074/jbc.M115.695379
Nozal, V., and Martinez, A. (2019). Tau Tubulin Kinase 1 (TTBK1), a new player in the fight against neurodegenerative diseases. Eur. J. Med. Chem. 161, 39–47. doi:10.1016/j.ejmech.2018.10.030
Nozal, V., Martínez-González, L., Gomez-Almeria, M., Gonzalo-Consuegra, C., Santana, P., Chaikuad, A., et al. (2022). TDP-43 modulation by tau-tubulin kinase 1 inhibitors: A new avenue for future amyotrophic lateral sclerosis therapy. J. Med. Chem. 65, 1585–1607. doi:10.1021/acs.jmedchem.1c01942
O’Brien, R. J., and Wong, P. C. (2011). Amyloid precursor protein processing and Alzheimer’s disease. Annu. Rev. Neurosci. 34, 185–204. doi:10.1146/annurev-neuro-061010-113613
Okochi, M., Walter, J., Koyama, A., Nakajo, S., Baba, M., Iwatsubo, T., et al. (2000). Constitutive phosphorylation of the Parkinson’s disease associated alpha-synuclein. J. Biol. Chem. 275, 390–397. doi:10.1074/jbc.275.1.390
Oliveira, J., Costa, M., de Almeida, M. S. C., da Cruz e Silva, O. A. B., and Henriques, A. G. (2017). Protein phosphorylation is a key mechanism in Alzheimer’s disease. J. Alzheimer’s Dis. 58, 953–978. doi:10.3233/jad-170176
Oshima, T., Niwa, Y., Kuwata, K., Srivastava, A., Hyoda, T., Tsuchiya, Y., et al. (2019). Cell-based screen identifies a new potent and highly selective CK2 inhibitor for modulation of circadian rhythms and cancer cell growth. Sci. Adv. 5, eaau9060. doi:10.1126/sciadv.aau9060
Ou, S. H., Wu, F., Harrich, D., Garcia-Martinez, L. F., and Gaynor, R. B. (1995). Cloning and characterization of a novel cellular protein, TDP-43, that binds to human immunodeficiency virus type 1 TAR DNA sequence motifs. J. Virol. 69, 3584–3596. doi:10.1128/JVI.69.6.3584-3596.1995
Oueslati, A. (2016). Implication of alpha-synuclein phosphorylation at S129 in synucleinopathies: What have we learned in the last decade? J. Park. Dis. 6, 39–51. doi:10.3233/JPD-160779
Pagano, M. A., Bain, J., Kazimierczuk, Z., Sarno, S., Ruzzene, M., Di Maira, G., et al. (2008). The selectivity of inhibitors of protein kinase CK2: An update. Biochem. J. 415, 353–365. doi:10.1042/bj20080309
Paleologou, K. E., Schmid, A. W., Rospigliosi, C. C., Kim, H.-Y., Lamberto, G. R., Fredenburg, R. A., et al. (2008). Phosphorylation at ser-129 but not the phosphomimics S129E/D inhibits the fibrillation of α-synuclein. J. Biol. Chem. 283, 16895–16905. doi:10.1074/jbc.m800747200
Palomo, V., Nozal, V., Rojas-Prats, E., Gil, C., and Martinez, A. (2020). Protein kinase inhibitors for amyotrophic lateral sclerosis therapy. Br. J. Pharmacol. 178, 1316–1335. doi:10.1111/bph.15221
Perez, D. I., Gil, C., and Martinez, A. (2011). Protein kinases CK1 and CK2 as new targets for neurodegenerative diseases. Med. Res. Rev. 31, 924–954. doi:10.1002/med.20207
Pierre, F., Chua, P. C., O’Brien, S. E., Siddiqui-Jain, A., Bourbon, P., Haddach, M., et al. (2011). Pre-clinical characterization of CX-4945, a potent and selective small molecule inhibitor of CK2 for the treatment of cancer. Mol. Cell. Biochem. 356, 37–43. doi:10.1007/s11010-011-0956-5
Pinna, L. A. (2002). Protein kinase CK2: A challenge to canons. J. Cell Sci. 115, 3873–3878. doi:10.1242/jcs.00074
Plisson, F., Prasad, P., Xiao, X., Piggott, A. M., Huang, X., Khalil, Z., et al. (2014). Callyspongisines A–D: Bromopyrrole alkaloids from an Australian marine sponge, callyspongia sp. Org. Biomol. Chem. 12, 1579–1584. doi:10.1039/c4ob00091a
Posa, D., Martinez-Gonzalez, L., Bartolome, F., Nagaraj, S., Porras, G., Martinez, A., et al. (2019). Recapitulation of pathological TDP-43 features in immortalized lymphocytes from sporadic ALS patients. Mol. Neurobiol. 56, 2424–2432. doi:10.1007/s12035-018-1249-8
Potjewyd, F. M., Marquez, A. B., Chaikuad, A., Howel, S., Dunn, A. S., Beltran, A. A., et al. (2022). Modulation of tau tubulin kinases (TTBK1 and TTBK2) impacts ciliogenesis. bioRxiv 2022, 06490937. doi:10.1101/2022.05.06.490937
Pulgar, V., Marin, O., Meggio, F., Allende, C. C., Allende, J. E., and Pinna, L. A. (1999). Optimal sequences for non-phosphate-directed phosphorylation by protein kinase CK1 (casein kinase-1)--a re-evaluation. Eur. J. Biochem. 260, 520–526. doi:10.1046/j.1432-1327.1999.00195.x
Raux, G., Gantier, R., Martin, C., Pothin, Y., Brice, A., Frebourg, T., et al. (2000). A novel presenilin 1 missense mutation (L153V) segregating with early-onset autosomal dominant Alzheimer’s disease. Hum. Mutat. 16, 95. doi:10.1002/1098-1004(200007)16:1<95:AID-HUMU28>3.0.CO;2-H
Regal, L., Vanopdenbosch, L., Tilkin, P., Van den Bosch, L., Thijs, V., Sciot, R., et al. (2006). The G93C mutation in superoxide dismutase 1: Clinicopathologic phenotype and prognosis. Arch. Neurol. 63, 262–267. doi:10.1001/archneur.63.2.262
Reiner, A., Albin, R. L., Anderson, K. D., D’Amato, C. J., Penney, J. B., and Young, A. B. (1988). Differential loss of striatal projection neurons in Huntington disease. Proc. Natl. Acad. Sci. U. S. A. 85, 5733–5737. doi:10.1073/pnas.85.15.5733
Rena, G., Bain, J., Elliott, M., and Cohen, P. (2004). D4476, a cell-permeant inhibitor of CK1, suppresses the site-specific phosphorylation and nuclear exclusion of FOXO1a. EMBO Rep. 5, 60–65. doi:10.1038/sj.embor.7400048
Renton, A. E., Chiò, A., and Traynor, B. J. (2013). State of play in amyotrophic lateral sclerosis genetics. Nat. Neurosci. 17, 17–23. doi:10.1038/nn.3584
Rezai-Zadeh, K., Shytle, R. D., Bai, Y., Tian, J., Hou, H., Mori, T., et al. (2009). Flavonoid-mediated presenilin-1 phosphorylation reduces Alzheimer's disease beta-amyloid production. J. Cell. Mol. Med. 13, 574–588. doi:10.1111/j.1582-4934.2008.00344.x
Richter, J., Bischof, J., Zaja, M., Kohlhof, H., Othersen, O., Vitt, D., et al. (2014). Difluoro-dioxolo-benzoimidazol-benzamides as potent inhibitors of CK1δ and ε with nanomolar inhibitory activity on cancer cell proliferation. J. Med. Chem. 57, 7933–7946. doi:10.1021/jm500600b
Ríos, J.-L., Giner, R., Marín, M., and Recio, M. (2018). A pharmacological update of ellagic acid. Planta Med. 84, 1068–1093. doi:10.1055/a-0633-9492
Robberecht, W., and Philips, T. (2013). The changing scene of amyotrophic lateral sclerosis. Nat. Rev. Neurosci. 14, 248–264. doi:10.1038/nrn3430
Rosenberger, A. F. N., Morrema, T. H. J., Gerritsen, W. H., van Haastert, E. S., Snkhchyan, H., Hilhorst, R., et al. (2016). Increased occurrence of protein kinase CK2 in astrocytes in Alzheimer’s disease pathology. J. Neuroinflammation 13, 4. doi:10.1186/s12974-015-0470-x
Rosenblatt, A. (2007). Neuropsychiatry of Huntington’s disease. Dialogues Clin. Neurosci. 9, 191–197. doi:10.31887/DCNS.2007.9.2/arosenblatt
Rubinsztein, D. C., Leggo, J., Coles, R., Almqvist, E., Biancalana, V., Cassiman, J. J., et al. (1996). Phenotypic characterization of individuals with 30-40 CAG repeats in the Huntington disease (HD) gene reveals HD cases with 36 repeats and apparently normal elderly individuals with 36-39 repeats. Am. J. Hum. Genet. 59, 16–22.
Rubio de la Torre, E., Luzon-Toro, B., Forte-Lago, I., Minguez-Castellanos, A., Ferrer, I., and Hilfiker, S. (2009). Combined kinase inhibition modulates Parkin inactivation. Hum. Mol. Genet. 18, 809–823. doi:10.1093/hmg/ddn407
Salado, I. G., Redondo, M., Bello, M. L., Perez, C., Liachko, N. F., Kraemer, B. C., et al. (2014). Protein kinase CK-1 inhibitors as new potential drugs for amyotrophic lateral sclerosis. J. Med. Chem. 57, 2755–2772. doi:10.1021/jm500065f
Salvi, M., Sarno, S., Cesaro, L., Nakamura, H., and Pinna, L. A. (2009). Extraordinary pleiotropy of protein kinase CK2 revealed by weblogo phosphoproteome analysis. Biochim. Biophys. Acta 1793, 847–859. doi:10.1016/j.bbamcr.2009.01.013
Sannerud, R., Esselens, C., Ejsmont, P., Mattera, R., Rochin, L., Tharkeshwar, A. K., et al. (2016). Restricted location of PSEN2/γ-secretase determines substrate specificity and generates an intracellular Aβ pool. Cell 166, 193–208. doi:10.1016/j.cell.2016.05.020
Sarno, S., Reddy, H., Meggio, F., Ruzzene, M., Davies, S. P., Donella-Deana, A., et al. (2001). Selectivity of 4, 5, 6, 7-tetrabromobenzotriazole, an ATP site-directed inhibitor of protein kinase CK2 (“casein kinase-2”). FEBS Lett. 496, 44–48. doi:10.1016/s0014-5793(01)02404-8
Sato, N., Hori, O., Yamaguchi, A., Lambert, J. C., Chartier-Harlin, M. C., Robinson, P. A., et al. (1999). A novel presenilin-2 splice variant in human Alzheimer’s disease brain tissue. J. Neurochem. 72, 2498–2505. doi:10.1046/j.1471-4159.1999.0722498.x
Sato, S., Cerny, R. L., Buescher, J. L., and Ikezu, T. (2006). Tau-tubulin kinase 1 (TTBK1), a neuron-specific tau kinase candidate, is involved in tau phosphorylation and aggregation. J. Neurochem. 98, 1573–1584. doi:10.1111/j.1471-4159.2006.04059.x
Sato, S., Xu, J., Okuyama, S., Martinez, L. B., Walsh, S. M., Jacobsen, M. T., et al. (2008). Spatial learning impairment, enhanced CDK5/p35 activity, and downregulation of NMDA receptor expression in transgenic mice expressing tau-tubulin kinase 1. J. Neurosci. 28, 14511–14521. doi:10.1523/jneurosci.3417-08.2008
Schwab, C., DeMaggio, A. J., Ghoshal, N., Binder, L. I., Kuret, J., and McGeer, P. L. (2000). Casein kinase 1 delta is associated with pathological accumulation of tau in several neurodegenerative diseases. Neurobiol. Aging 21, 503–510. doi:10.1016/s0197-4580(00)00110-x
Schwartz, P. A., and Murray, B. W. (2011). Protein kinase biochemistry and drug discovery. Bioorg. Chem. 39, 192–210. doi:10.1016/j.bioorg.2011.07.004
Schwind, L., Nalbach, L., Zimmer, A. D., Kostelnik, K. B., Menegatti, J., Grässer, F., et al. (2017). Quinalizarin inhibits adipogenesis through down-regulation of transcription factors and microRNA modulation. Biochim. Biophys. Acta. Gen. Subj. 1861, 3272–3281. doi:10.1016/j.bbagen.2017.09.018
Selvakumar, K., Bavithra, S., Suganthi, M., Benson, C. S., Elumalai, P., Arunkumar, R., et al. (2012). Protective role of quercetin on PCBs-induced oxidative stress and apoptosis in hippocampus of adult rats. Neurochem. Res. 37, 708–721. doi:10.1007/s11064-011-0661-5
Shao, G., Chen, S., Sun, Y., Xu, H., and Ge, F. (2021). Chrysoeriol promotes functional neurological recovery in a rat model of cerebral ischemia. Phcog. Mag. 17, 802–810. doi:10.4103/pm.pm_329_21
Sharma, V., Mishra, M., Ghosh, S., Tewari, R., Basu, A., Seth, P., et al. (2007). Modulation of interleukin-1beta mediated inflammatory response in human astrocytes by flavonoids: Implications in neuroprotection. Brain Res. Bull. 73, 55–63. doi:10.1016/j.brainresbull.2007.01.016
Shimura, H., Hattori, N., Kubo, S., Mizuno, Y., Asakawa, S., Minoshima, S., et al. (2000). Familial Parkinson disease gene product, parkin, is a ubiquitin-protein ligase. Nat. Genet. 25, 302–305. doi:10.1038/77060
Siddiqui-Jain, A., Drygin, D., Streiner, N., Chua, P., Pierre, F., O'Brien, S. E., et al. (2010). CX-4945, an orally bioavailable selective inhibitor of protein kinase CK2, inhibits prosurvival and angiogenic signaling and exhibits antitumor efficacy. Cancer Res. 70, 10288–10298. doi:10.1158/0008-5472.CAN-10-1893
Smith, M. J., Sharples, R. A., Evin, G., McLean, C. A., Dean, B., Pavey, G., et al. (2004). Expression of truncated presenilin 2 splice variant in Alzheimer’s disease, bipolar disorder, and schizophrenia brain cortex. Brain Res. Mol. Brain Res. 127, 128–135. doi:10.1016/j.molbrainres.2004.05.019
Spillantini, M. G., Schmidt, M. L., Lee, V. M., Trojanowski, J. Q., Jakes, R., and Goedert, M. (1997). Alpha-synuclein in Lewy bodies. Nature 388, 839–840. doi:10.1038/42166
Sreedharan, J., Blair, I. P., Tripathi, V. B., Hu, X., Vance, C., Rogelj, B., et al. (2008). TDP-43 mutations in familial and sporadic amyotrophic lateral sclerosis. Science 319, 1668–1672. doi:10.1126/science.1154584
Sriram, S. R., Li, X., Ko, H. S., Chung, K. K., Wong, E., Lim, K. L., et al. (2005). Familial-associated mutations differentially disrupt the solubility, localization, binding and ubiquitination properties of parkin. Hum. Mol. Genet. 14, 2571–2586. doi:10.1093/hmg/ddi292
Su, C.-F., Jiang, L., Zhang, X. W., Iyaswamy, A., and Li, M. (2021). Resveratrol in rodent models of Parkinson’s disease: A systematic review of experimental studies. Front. Pharmacol. 12, 644219. doi:10.3389/fphar.2021.644219
Su, Z., Song, J., Wang, Z., Zhou, L., Xia, Y., Yu, S., et al. (2018). Tumor promoter TPA activates Wnt/β-catenin signaling in a casein kinase 1-dependent manner. Proc. Natl. Acad. Sci. U. S. A. 115, E7522–E7531. doi:10.1073/pnas.1802422115
Sundaram, S., Nagaraj, S., Mahoney, H., Portugues, A., Li, W., Millsaps, K., et al. (2019). Inhibition of casein kinase 1δ/ε improves cognitive-affective behavior and reduces amyloid load in the APP-PS1 mouse model of Alzheimer’s disease. Sci. Rep. 9, 13743. doi:10.1038/s41598-019-50197-x
Szyszka, R., Grankowski, N., Felczak, K., and Shugar, D. (1995). Halogenated benzimidazoles and benzotriazoles as selective inhibitors of protein kinases CK-I and CK-ii from Saccharomyces cerevisiae and other sources. Biochem. Biophys. Res. Commun. 208, 418–424. doi:10.1006/bbrc.1995.1354
Tagliabracci, V. S., Wiley, S. E., Guo, X., Kinch, L. N., Durrant, E., Wen, J., et al. (2015). A single kinase generates the majority of the secreted phosphoproteome. Cell 161, 1619–1632. doi:10.1016/j.cell.2015.05.028
Takahashi, M., Tomizawa, K., Sato, K., Ohtake, A., and Omori, A. (1995). A novel tau-tubulin kinase from bovine brain. FEBS Lett. 372, 59–64. doi:10.1016/0014-5793(95)00955-9
Takano, A., Hoe, H.-S., Isojima, Y., and Nagai, K. (2004). Analysis of the expression, localization and activity of rat casein kinase 1epsilon-3. Neuroreport 15, 1461–1464. doi:10.1097/01.wnr.0000133297.77278.81
Takashima, A., Noguchi, K., Sato, K., Hoshomoto, T., and Imahori, K. (1993). Tau protein kinase I is essential for amyloid beta-protein-induced neurotoxicity. Proc. Natl. Acad. Sci. U. S. A. 90, 7789–7793. doi:10.1073/pnas.90.16.7789
Tanimukai, H., Grundke-Iqbal, I., and Iqbal, K. (2005). Up-regulation of inhibitors of protein phosphatase-2A in Alzheimer’s disease. Am. J. Pathol. 66, 1761–1771. doi:10.1016/S0002-9440(10)62486-8
Tarrant, M. K., and Cole, P. A. (2009). The chemical biology of protein phosphorylation. Annu. Rev. Biochem. 78, 797–825. doi:10.1146/annurev.biochem.78.070907.103047
Taylor, L. M., McMillan, P. J., Kraemer, B. C., and Liachko, N. F. (2019). Tau tubulin kinases in proteinopathy. FEBS J. 286, 2434–2446. doi:10.1111/febs.14866
Taylor, L. M., McMillan, P. J., Liachko, N. F., Strovas, T. J., Ghetti, B., Bird, T. D., et al. (2018). Pathological phosphorylation of tau and TDP-43 by TTBK1 and TTBK2 drives neurodegeneration. Mol. Neurodegener. 13, 7. doi:10.1186/s13024-018-0237-9
Taylor, S. S., and Kornev, A. P. (2011). Protein kinases: Evolution of dynamic regulatory proteins. Trends biochem. Sci. 36, 65–77. doi:10.1016/j.tibs.2010.09.006
Taylor, S. S., Radzio-Andzelm, E., and Hunter, T. (1995). How do protein kinases discriminate between serine/threonine and tyrosine? Structural insights from the insulin receptor protein-tyrosine kinase. FASEB J. 9, 1255–1266. doi:10.1096/fasebj.9.13.7557015
Tian, Y., Wang, Y., Jablonski, A. M., Hu, Y., Sugam, J. A., Koglin, M., et al. (2021). Tau-tubulin kinase 1 phosphorylates TDP-43 at disease-relevant sites and exacerbates TDP-43 pathology. Neurobiol. Dis. 161, 105548. doi:10.1016/j.nbd.2021.105548
Tiwari, S., Atluri, V., Kaushik, A., Yndar, T. A., and Nair, M. (2019). Alzheimer’s disease: Pathogenesis, diagnostics, and therapeutics. Int. J. Nanomedicine 14, 5541–5554. doi:10.2147/IJN.S200490
Tomizawa, K., Omori, A., Ohtake, A., Sato, K., and Takahashi, M. (2001). Tau-tubulin kinase phosphorylates tau at Ser-208 and Ser-210, sites found in paired helical filament-tau. FEBS Lett. 492, 221–227. doi:10.1016/s0014-5793(01)02256-6
Turner, R. S., Thomas, R. G., Craft, S., van Dyck, C. H., Mintzer, J., Reynolds, B. A., et al. (2015). A randomized, double-blind, placebo-controlled trial of resveratrol for Alzheimer disease. Neurology 85, 1383–1391. doi:10.1212/wnl.0000000000002035
Ueda, K., Fukushima, H., Masliah, E., Xia, Y., Iwai, A., Yoshimoto, M., et al. (1993). Molecular cloning of cDNA encoding an unrecognized component of amyloid in Alzheimer disease. Proc. Natl. Acad. Sci. U. S. A. 90, 11282–11286. doi:10.1073/pnas.90.23.11282
Ullah, A., Munir, S., Badshah, S. L., Khan, N., Ghani, L., Poulson, B. G., et al. (2020). Important flavonoids and their role as a therapeutic agent. Molecules 25, 5243. doi:10.3390/molecules25225243
Uversky, V. N. (2007). Neuropathology, biochemistry, and biophysics of alpha-synuclein aggregation. J. Neurochem. 103, 17–37. doi:10.1111/j.1471-4159.2007.04764.x
Varela, L., and Garcia-Rendueles, M. E. R. (2022). Oncogenic pathways in neurodegenerative diseases. Int. J. Mol. Sci. 23, 3223. doi:10.3390/ijms23063223
Vargas, M. R., Pehar, M., Cassina, P., Beckman, J. S., and Barbeito, L. (2006). Increased glutathione biosynthesis by Nrf2 activation in astrocytes prevents p75NTR-dependent motor neuron apoptosis. J. Neurochem. 97, 687–696. doi:10.1111/j.1471-4159.2006.03742.x
Vassar, R., Bennett, B. D., Babu-Kahn, S., Kahn, S., Mendiaz, E. A., Denis, P., et al. (1999). Beta-secretase cleavage of Alzheimer's amyloid precursor protein by the transmembrane aspartic protease BACE. Science 286, 735–741. doi:10.1126/science.286.5440.735
Vazquez-Higuera, J. L., Martinez-Garcia, A., Sanchez-Juan, P., Rodriguez-Rodriguez, E., Mateo, I., Pozueta, A., et al. (2011). Genetic variations in tau-tubulin kinase-1 are linked to Alzheimer’s disease in a Spanish case-control cohort. Neurobiol. Aging 32, e5–9. doi:10.1016/j.neurobiolaging.2009.12.021
Venerando, A., Ruzzene, M., and Pinna, L. A. (2014). Casein kinase: The triple meaning of a misnomer. Biochem. J. 460, 141–156. doi:10.1042/bj20140178
Vera, J., Estanyol, J. M., Canela, N., Llorens, F., Agell, N., Itarte, E., et al. (2007). Proteomic analysis of SET-binding proteins. Proteomics 7, 578–587. doi:10.1002/pmic.200600458
Versluys, L., Pereira, P. E., Schuermans, N., De Paepe, B., De Bleecker, J. L., Bogaert, E., et al. (2022). Expanding the TDP-43 proteinopathy pathway from neurons to muscle: Physiological and pathophysiological functions. Front. Neurosci. 16, 815765. doi:10.3389/fnins.2022.815765
Waddy, C. T., and Mackinlay, A. G. (1971). Protein kinase activity from lactating bovine mammary gland. Biochim. Biophys. Acta 250, 491–500. doi:10.1016/0005-2744(71)90249-x
Wager, T. T., Chandrasekaran, R. Y., Bradley, J., Rubitski, D., Berke, H., Mente, S., et al. (2014). Casein kinase 1δ/ε inhibitor PF-5006739 attenuates opioid drug-seeking behavior. ACS Chem. Neurosci. 5, 1253–1265. doi:10.1021/cn500201x
Wakabayashi, K., Matsumoto, K., Takayama, K., Yoshimoto, M., and Takahashi, H. (1997). NACP, a presynaptic protein, immunoreactivity in Lewy bodies in Parkinson’s disease. Neurosci. Lett. 239, 45–48. doi:10.1016/s0304-3940(97)00891-4
Walter, J., Capell, A., Grünberg, J., Pesold, B., Schindzielorz, A., Prior, R., et al. (1996). The Alzheimer's disease-associated presenilins are differentially phosphorylated proteins located predominantly within the endoplasmic reticulum. Mol. Med. 2, 673–691. doi:10.1007/BF03401652
Walter, J., Fluhrer, R., Hartung, B., Willem, M., Kaether, C., Capell, A., et al. (2001). Phosphorylation regulates intracellular trafficking of β-secretase. J. Biol. Chem. 276, 14634–14641. doi:10.1074/jbc.m011116200
Walter, J., Schindzielorz, A., Hartung, B., and Haass, C. (2000). Phosphorylation of the beta-amyloid precursor protein at the cell surface by ectocasein kinases 1 and 2. J. Biol. Chem. 275, 23523–23529. doi:10.1074/jbc.M002850200
Walter, J. (2000). The phosphorylation of presenilin proteins. Methods Mol. Med. 32, 317–331. doi:10.1385/1-59259-195-7:317
Walton, K. M., Fisher, K., Rubitski, D., Marconi, M., Meng, Q.-J., Sladek, M., et al. (2009). Selective inhibition of casein kinase 1 minimally alters circadian clock period. J. Pharmacol. Exp. Ther. 330, 430–439. doi:10.1124/jpet.109.151415
Wan, Y., Hur, W., Cho, C. Y., Liu, Y., Adrian, F. J., Lozach, O., et al. (2004). Synthesis and target identification of hymenialdisine analogs. Chem. Biol. 11, 247–259. doi:10.1016/j.chembiol.2004.01.015
Wang, B., Lu, Z., and Polya, G. (1998). Inhibition of eukaryote serine/threonine-specific protein kinases by piceatannol. Planta Med. 64, 195–199. doi:10.1055/s-2006-957407
Wang, Q., Dong, X., Zhang, R., and Zhao, Ch. (2021). Flavonoids with potential anti-amyloidogenic effects as therapeutic drugs for treating alzheimer's disease. J. Alzheimers Dis. 84, 505–533. doi:10.3233/JAD-210735
Wang, S.-H., Liang, C.-H., Liang, F.-P., Ding, H.-Y., Lin, S.-P., Huang, G.-J., et al. (2016). The inhibitory mechanisms study of 5, 6, 4′-trihydroxy-7, 3′-dimethoxyflavone against the LPS-induced macrophage inflammatory responses through the antioxidant ability. Molecules 21, 136. doi:10.3390/molecules21020136
Waxman, E. A., and Giasson, B. I. (2008). Specificity and regulation of casein kinase/mediated phosphorylation of α-synuclein. J. Neuropathol. Exp. Neurol. 67, 402–416. doi:10.1097/NEN.0b013e31816fc995
Wei, Y., Zhu, G., Zheng, C., Li, J., Sheng, S., Li, D., et al. (2020). Ellagic acid protects dopamine neurons from rotenone-induced neurotoxicity via activation of Nrf2 signalling. J. Cell. Mol. Med. 24, 9446–9456. doi:10.1111/jcmm.15616
Weingarten, M. D., Lockwood, A. H., Hwo, S. Y., and Kirschner, M. W. (1975). A protein factor essential for microtubule assembly. Proc. Natl. Acad. Sci. U. S. A. 72, 1858–1862. doi:10.1073/pnas.72.5.1858
Wells, C. I., Drewry, D. H., Pickett, J. E., Tjaden, A., Krämer, A., Müller, S., et al. (2021). Development of a potent and selective chemical probe for the pleiotropic kinase CK2. Cell Chem. Biol. 28, 546–558. e10. doi:10.1016/j.chembiol.2020.12.013
Wirkner, U., Voss, H., Lichter, P., Ansorge, W., and Pyerin, W. (1994). The human gene (CSNK2A1) coding for the casein kinase II subunit alpha is located on chromosome 20 and contains tandemly arranged Alu repeats. Genomics 19, 257–265. doi:10.1006/geno.1994.1056
Xia, J., Lee, D. H., Taylor, J., Vandelft, M., and Truant, R. (2003). Huntingtin contains a highly conserved nuclear export signal. Hum. Mol. Genet. 12, 1393–1403. doi:10.1093/hmg/ddg156
Xu, J., Sato, S., Okuyama, S., Swan, R. J., Jacobsen, M. T., Strunk, E., et al. (2010). Tau-tubulin kinase 1 enhances prefibrillar tau aggregation and motor neuron degeneration in P301L FTDP-17 tau-mutant mice. FASEB J. 24, 2904–2915. doi:10.1096/fj.09-150144
Xu, P., Ianes, C., Gärtner, F., Liu, C., Burster, T., Bakulev, V., et al. (2019). Structure, regulation, and (patho-)physiological functions of the stress-induced protein kinase CK1 delta (CSNK1D). Gene 715, 144005. doi:10.1016/j.gene.2019.144005
Xu, Y., Deng, Y., and Qing, H. (2015). The phosphorylation of α-synuclein: Development and implication for the mechanism and therapy of the Parkinson's disease. J. Neurochem. 135, 4–18. doi:10.1111/jnc.13234
Xue, Y., Wan, P. T., Hillertz, P., Schweikart, F., Zhao, Y., Wissler, L., et al. (2013). X-ray structural analysis of tau-tubulin kinase 1 and its interactions with small molecular inhibitors. ChemMedChem 8, 1846–1854. doi:10.1002/cmdc.201300274
Yadikar, H., Torres, I., Aiello, G., Kurup, M., Yang, Z., Lin, F., et al. (2020). Screening of tau protein kinase inhibitors in a tauopathy-relevant cell-based model of tau hyperphosphorylation and oligomerization. PLOS ONE 15, e0224952. doi:10.1371/journal.pone.0224952
Yamamoto, A., Friedlein, A., Imai, Y., Takahashi, R., Kahle, P. J., and Haass, Ch. (2005). Parkin phosphorylation and modulation of its E3 ubiquitin ligase activity. J. Biol. Chem. 280, 3390–3399. doi:10.1074/jbc.M407724200
Yang, A. J. T., Bagit, A., and MacPherson, R. E. K. (2021). Resveratrol, metabolic dysregulation, and Alzheimer’s disease: Considerations for neurogenerative disease. Int. J. Mol. Sci. 22, 4628. doi:10.3390/ijms22094628
Yang, Y., Xu, T., Zhang, Y., and Qin, X. (2017). Molecular basis for the regulation of the circadian clock kinases CK1δ and CK1ε. Cell. Signal. 31, 58–65. doi:10.1016/j.cellsig.2016.12.010
Yang-Feng, T. L., Naiman, T., Kopatz, I., Eli, D., Dafni, N., and Canaani, D. (1994). Assignment of the human casein kinase II alpha' subunit gene (CSNK2A1) to chromosome 16p13.2-p13.3. Genomics 19, 173. doi:10.1006/geno.1994.1032
Yasojima, K., Schwab, C., McGeer, E. G., and McGeer, P. L. (2000). Human neurons generate C-reactive protein and amyloid P: Upregulation in Alzheimer’s disease. Brain Res. 887, 80–89. doi:10.1016/s0006-8993(00)02970-x
Yde, C. W., Frogne, T., Lykkesfeldt, A. E., Fitchtner, I., Issinger, O.-G., and Stenvang, J. (2007). Induction of cell death in antiestrogen resistant human breast cancer cells by the protein kinase CK2 inhibitor DMAT. Cancer Lett. 256, 229–237. doi:10.1016/j.canlet.2007.06.010
Yonetani, M., Nonaka, T., Masuda, M., Inukai, Y., Oikawa, T., Hisanaga, S., et al. (2009). Conversion of wildtype alpha-synuclein into mutant-type fibrils and its propagation in the presence of A30P mutant. J. Biol. Chem. 284, 7940–7950. doi:10.1074/jbc.M807482200
Yoshida, H., and Ihara, Y. (1993). Tau in paired helical filaments is functionally distinct from fetal tau: Assembly incompetence of paired helical filament-tau. J. Neurochem. 61, 1183–1186. doi:10.1111/j.1471-4159.1993.tb03642.x
Yu, G., Yan, T., Feng, Y., Liu, X., Xia, Y., Luo, H., et al. (2013). Ser9 phosphorylation causes cytoplasmic detention of I2PP2A/SET in Alzheimer disease. Neurobiol. Aging 34, 1748–1758. doi:10.1016/j.neurobiolaging.2012.12.025
Yu, N.-N., Yu, J.-T., Xiao, J.-T., Zhang, H.-W., Lu, R.-C., Jiang, H., et al. (2011). Tau-tubulin kinase-1 gene variants are associated with Alzheimer’s disease in Han Chinese. Neurosci. Lett. 491, 83–86. doi:10.1016/j.neulet.2011.01.011
Yu, P., Wang, L., Tang, F., Zeng, L., Zhou, L., Song, X., et al. (2016). Resveratrol pretreatment decreases ischemic injury and improves neurological function via sonic hedgehog signaling after stroke in rats. Mol. Neurobiol. 54, 212–226. doi:10.1007/s12035-015-9639-7
Zaplatic, E., Bule, M., Shah, S. Z. A., Uddin, M. S., and Niaz, K. (2019). Molecular mechanisms underlying protective role of quercetin in attenuating Alzheimer’s disease. Life Sci. 224, 109–119. doi:10.1016/j.lfs.2019.03.055
Zhang, H., Khalil, Z., Conte, M. M., Plisson, F., and Capon, R. J. (2012). A search for kinase inhibitors and antibacterial agents: Bromopyrrolo-2-aminoimidazoles from a deep-water Great Australian Bight sponge Axinella sp. Tetrahedron Lett. 53, 3784–3787. doi:10.1016/j.tetlet.2012.05.051
Zhang, J., Gross, S. D., Schroeder, M. D., and Anderson, R. A. (1996). Casein kinase I α and αL: Alternative splicing-generated kinases exhibit different catalytic properties. Biochemistry 35, 16319–16327. doi:10.1021/bi9614444
Zhang, N., Gordon, S. L., Fritsch, M. J., Esoof, N., Campbell, D. G., Gourlay, R., et al. (2015). Phosphorylation of synaptic vesicle protein 2A at Thr84 by casein kinase 1 family kinases controls the specific retrieval of synaptotagmin-1. J. Neurosci. 35, 2492–2507. doi:10.1523/JNEUROSCI.4248-14.2015
Zhang, Q., Xia, Y., Wang, Y., Shentu, Y., Zeng, K., Mahaman, Y. A. R., et al. (2018). CK2 phosphorylating I2PP2A/SET mediates tau pathology and cognitive impairment. Front. Mol. Neurosci. 11, 146. doi:10.3389/fnmol.2018.00146
Zhang, Y.-J., Xu, Y.-F., Cook, C., Gendron, T. F., Roettges, P., Link, C. D., et al. (2009). Aberrant cleavage of TDP-43 enhances aggregation and cellular toxicity. Proc. Natl. Acad. Sci. U. S. A. 106, 7607–7612. doi:10.1073/pnas.0900688106
Zhao, H., Mei, X., Yang, D., and Tu, G. (2021). Resveratrol inhibits inflammation after spinal cord injury via SIRT-1/NF-κB signaling pathway. Neurosci. Lett. 762, 136151. doi:10.1016/j.neulet.2021.136151
Zhao, Z., Wang, L., Volk, A. G., Birch, N. W., Stoltz, K. L., Bartom, E. T., et al. (2018). Regulation of MLL/COMPASS stability through its proteolytic cleavage by taspase1 as a possible approach for clinical therapy of leukemia. Genes Dev. 33, 61–74. doi:10.1101/gad.319830.118
Zheng, H., and Koo, E. H. (2006). The amyloid precursor protein: Beyond amyloid. Mol. Neurodegener. 1, 5. doi:10.1186/1750-1326-1-5
Zheng, J. C., and Chen, S. (2022). Translational Neurodegeneration in the era of fast growing international brain research. Transl. Neurodegener. 11, 1. doi:10.1186/s40035-021-00276-9
Keywords: neurodegenerative diseases, CK1, CK2, phosphorylation, TTBK, inhibitors
Citation: Baier A and Szyszka R (2022) CK2 and protein kinases of the CK1 superfamily as targets for neurodegenerative disorders. Front. Mol. Biosci. 9:916063. doi: 10.3389/fmolb.2022.916063
Received: 08 April 2022; Accepted: 02 August 2022;
Published: 06 October 2022.
Edited by:
Andrea Venerando, University of Padua, ItalyReviewed by:
Alison Axtman, University of North Carolina at Chapel Hill, United StatesChandrika Gowda, College of Medicine, Pennsylvania State University, United States
Copyright © 2022 Baier and Szyszka. This is an open-access article distributed under the terms of the Creative Commons Attribution License (CC BY). The use, distribution or reproduction in other forums is permitted, provided the original author(s) and the copyright owner(s) are credited and that the original publication in this journal is cited, in accordance with accepted academic practice. No use, distribution or reproduction is permitted which does not comply with these terms.
*Correspondence: Andrea Baier, YW5kcmVhLmJhaWVyQGt1bC5wbA==; Ryszard Szyszka, c3p5c3prYXJAa3VsLnBs