- 1Department of Bioproducts and Biosystems, Aalto University, Espoo, Finland
- 2Kemistintie 1, Aalto University, Otakaari, Finland
Yeasts are widely used and established production hosts for biopharmaceuticals. Despite of tremendous advances on creating human-type N-glycosylation, N-glycosylated biopharmaceuticals manufactured with yeasts are missing on the market. The N-linked glycans fulfill several purposes. They are essential for the properties of the final protein product for example modulating half-lives or interactions with cellular components. Still, while the protein is being formed in the endoplasmic reticulum, specific glycan intermediates play crucial roles in the folding of or disposal of proteins which failed to fold. Despite of this intricate interplay between glycan intermediates and the cellular machinery, many of the glycoengineering approaches are based on modifications of the N-glycan processing steps in the endoplasmic reticulum (ER). These N-glycans deviate from the canonical structures required for interactions with the lectins of the ER quality control system. In this review we provide a concise overview on the N-glycan biosynthesis, glycan-dependent protein folding and quality control systems and the wide array glycoengineering approaches. Furthermore, we discuss how the current glycoengineering approaches partially or fully by-pass glycan-dependent protein folding mechanisms or create structures that mimic the glycan epitope required for ER associated protein degradation.
Introduction
Yeasts are widely used and established production hosts for biopharmaceuticals. Saccharomyces cerevisiae is by far the most utilized yeast species for therapeutic proteins and has been approved as a production host for 35 different products. Following by a large margin, Pichia pastoris is used for the production of three, while Hansenula polymorpha is utilized for producing two therapeutic proteins. The yeast-based biopharmaceuticals currently in market include various insulin products and vaccines, but also other peptide hormones, a blood clotting factor subunit and a recombinant urate oxidase (Walsh, 2018). So far, N-glycosylated biopharmaceuticals manufactured by yeasts are currently missing on the market.
The intricacy of the N-glycan biosynthesis pathways and the wide range of end products of this modification renders the engineering of glycosylation process very demanding. Despite the heterogeneity of N-glycan structures, the formation of a lipid-linked oligosaccharide (LLO), the transfer of the glycan to a protein, and the trimming of the N-glycan during protein folding are highly conserved processes and thus N-glycan maturation in the Golgi apparatus starts from the same structure.
The N-glycans fulfill several purposes, they modulate the properties of the protein product, support protein folding through binding to calnexin and export of folded glycoproteins and provide the necessary cues to the endoplasmic reticulum associated protein degradation (ERAD) machinery to initiate protein turnover. The currently applied glycoengineering approaches affect the N-glycan processing in the endoplasmic reticulum (ER) in various ways and the different engineering approaches have the potential to partially or fully sidestep glycan-dependent protein folding and disposal machineries. In this review, we summarize the current knowledge on N-glycan biosynthesis and glycan-dependent quality control systems in the context of glycoengineering in yeasts.
The Endoplasmic Reticulum Protein N-Glycosylation System
The LLO assembly that is initiated on the cytoplasmic side of the ER leads in a stepwise process to the formation of the Glc3Man9GlcNAc2 oligosaccharide (Figure 1A). First, a phosphorylated N-acetylglucosamine is transferred to a dolichol phosphate by Alg7p (Barnes et al., 1984; Kukuruzinska and Robbins, 1987). A second N-acetylglucosamine is then added via a β1-4 bond by an UDP-GlcNAc transferase complex consisting of Alg13p and Alg14p (Bickel et al., 2005; Chantret et al., 2005). The formed Dol-P-P-GlcNAc2 structure receives three mannose residues by Alg1p and Alg2p leading to the formation of the branched core glycan structure comprising terminal α1-3 linked and α1-6 linked mannoses (Huffaker and Robbins, 1982; Couto et al., 1984; O’Reilly et al., 2006). Then, Alg11p adds two α1-2 linked mannose residues to the α1-3 arm of the LLO initiating the A branch (Cipollo et al., 2001; O’Reilly et al., 2006). The resulting Man5GlcNAc2-P-P-Dol is translocated to the luminal side of the ER. Efforts to identify the flippase and elucidate the mechanism of the translocation reaction through in vivo and in vitro experiments produced conflicting results and the identity of the flippase and the specific role of Rft1p remains unresolved (Helenius et al., 2002; Frank et al., 2008; Sanyal et al., 2008; Rush et al., 2009; Verchère et al., 2021).
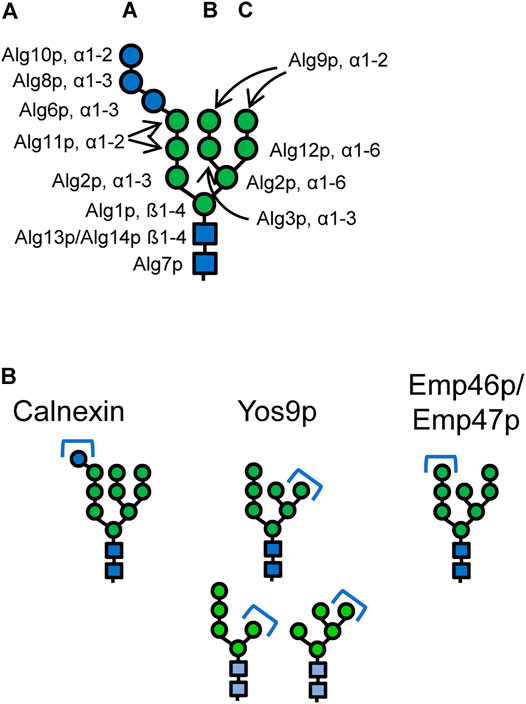
FIGURE 1. (A) Structure of the eukaryotic dolichol-linked oligosaccharide. Linkages and the names of the enzymes catalysing the respective steps are given. Deletion of ALG11 gene prevents formation of the A-branch. Deletion of ALG3 gene prevents formation the B- and C-branch mainly generating a Man5GlcNAc2 structure. Glucosylation of the A-branch is impaired in Δalg3 mutants. (B) Canonical N-glycan structures that mediate binding to the lectins calnexin/calreticulin, the ERAD lectin Yos9p, and the ER export receptors Emp46p/Emp47p, respectively. Alternative Yos9p substrates are marked in lighter hues. Blue circles: glucose, green circles: mannose, blue squares: N-acetylglucosamine.
On the luminal side of the ER, the LLO precursor is further branched and elongated. The α1-6 linked arm of the LLO receives an α1-3 linked mannose by Alg3p (Huffaker and Robbins, 1983; Aebi et al., 1996b; Sharma et al., 2001) forming the B branch which is capped with an α1-2 linked mannose by Alg9p (Burda et al., 1996; Frank and Aebi, 2005). A third branch, C branch, is formed as Alg12p transfers an α1-6 linked mannose to the α1-6 arm of the core glycan (Burda et al., 1999). Again Alg9p catalyzes the addition of a terminal mannose (Frank and Aebi, 2005). The final steps of the LLO biosynthesis include the addition of three glucose residues to the A branch. The first two glucoses are added via α1-3 linkages by Alg6p and Alg8p and the third via an α1-2 linkage by Alg10p, respectively (Runge and Robbins, 1986; Stagljar et al., 1994; Reiss et al., 1996; Burda and Aebi, 1998).
The complete Glc3Man9GlcNAc2 oligosaccharide is transferred en bloc via an N-glycosidic bond to an asparagine residue in the acceptor sequon Asn-X-Ser/Thr by the oligosaccharyltransferase (OST) complex. To ensure that only completely assembled LLOs are transferred, the OST in most eukaryotes has a strong preference for the Glc3Man9GlcNAc2 LLO, and the outermost glucose together with the chitobiose stem (GlcNAc2) of the LLO are important determinants for the substrate recognition (Burda et al., 1999).
N-Glycan Trimming Reactions Involved in Protein Folding and Degradation
The following glycan trimming reactions play important roles in protein folding and marking of misfolded proteins for ERAD. First, two glucose residues are very rapidly removed from the Glc3Man9GlcNAc2 glycan (Jakob et al., 1998). The resulting Glc1Man9GlcNAc2 glycan is specifically recognized by the lectin calnexin (Cne1p) (Parlati et al., 1995; Xu et al., 2004b) (Figure 1B). Removal of the last glucose residue of the N-glycan by the glucosidase heterodimer Gls2p/Gtb1p releases the protein from calnexin (Trombetta et al., 1996; Wilkinson et al., 2006). In some yeasts, an α1-3 linked glucose residue can be re-transferred to the Man9GlcNAc2 glycans by a UDP-glucose:glycoprotein glucosyltransferase (UGGT) enabling rebinding to calnexin and extending the time to correctly fold. However for example, S. cerevisiae lacks UGGT (Fernández et al., 1994), making the removal of the last glucose residue an irreversible process. After the removal of the glucose residues, one mannose residue is removed from the B branch of the N-glycan by the α1-2 mannosidase Mns1p (Camirand et al., 1991). Mns1p is a relatively slowly acting enzyme (Jakob et al., 1998), and this trimming step is thought to act as a timer mechanism for glycoprotein folding, giving the protein a sufficient time window for obtaining its native conformation (Jakob et al., 1998; Helenius and Aebi, 2004). Once the protein has reached its native conformation, the Man8GlcNAc2 glycan acts as a determinant for cargo recruitment into COPII vesicles by the mannose lectins Emp47p and Emp46p (Sato and Nakano, 2002) (Figure 1B). Based on structural similarities to related human proteins and extensive testing of their glycan specificities, the α1-2 linked mannose of the A-branch was suggested to serve as binding motif for interaction with these mannose lectins (Satoh et al., 2006; Kamiya et al., 2008).
However, after trimming by Mns1p, a second mannose residue can be cleaved from the C branch by the α1-2 mannosidase activity of the Htm1p-Pdi1p complex (Pfeiffer et al., 2016). As a result of this reaction, Man7GlcNAc2 glycans containing an exposed terminal α1-6 linked mannose residue are formed (Clerc et al., 2009; Gauss et al., 2011; Pfeiffer et al., 2016). The Htm1p-Pdi1p complex preferentially demannosylates Man8GlcNAc2 glycans of partially unstructured polypeptides (Liu et al., 2016; Pfeiffer et al., 2016).
Recognition of Endoplasmic Reticulum Associated Protein Degradation Substrates and Retrotranslocation
From three different ERAD systems, ERAD-L is responsible for eliminating ER luminal proteins which comprise also any secreted recombinant protein (Carvalho et al., 2006). The presence of a terminal α1-6 linked mannose residue of the Man7GlcNAc2 glycan is an important determinant for ERAD targeting of misfolded proteins (Quan et al., 2008; Kniss et al., 2018). However, also other glycans displaying a terminal α1-6 linked mannose, for example such forms as generated through ALG3 deletion are recognized by Yos9p (Szathmary et al., 2005; Clerc et al., 2009) (Figure 1B).When this residue is displayed, it can be recognized by the lectin domain of Yos9p (Friedmann et al., 2002; Buschhorn et al., 2004). In addition, Yos9p has affinity for unstructured or hydrophobic regions of misfolded proteins (Smith et al., 2014). Thus, a bipartite signal requiring both aberrant protein structures and the α1-6 linked mannose signal is utilized for targeting of a glycoprotein to ERAD (Xie et al., 2009).
The HRD1 complex consists of the transmembrane proteins Hrd1p, Hrd3p, Usa1p, Ubx2p and Der1p and the cytosolic components Cdc48p, Npl4p and Ufd1p and mediates retrotranslocation into the cytosol and ubiquitinylation (Carvalho et al., 2006). Recognition by Yos9p leads to targeting of the misfolded protein towards the HRD1 complex (Martinez Benitez et al., 2011). It was suggested that Hrd3p recognizes sequences downstream of the glycosylation site that are not in their native state and Yos9p makes this more specific by only targeting misfolded glycoproteins to it (Gauss et al., 2006; Mehnert et al., 2015; Wu et al., 2020). The misfolded protein then associates with the close by Der1p, and it was proposed that this association leads to the unfolding and insertion of the substrate into substrate channel of the translocon complex that is formed by Hrd1p and Der1 (Carvalho et al., 2010; Mehnert et al., 2013; Vasic et al., 2020; Wu et al., 2020).
When the substrate emerges on the cytosolic side of the HRD1 complex, the ubiquitination step is performed by Hrd1p and this polyubiquitination prevents the slipping back of the protein into the ER lumen (Hiller et al., 1996; Friedlander et al., 2000; Bays et al., 2001; Metzger et al., 2013). When ubiquitinated, the substrate polypeptide is pulled away from the ER membrane by a complex of Cdc48p, Ufd1p and Npl4p (Wolf and Stolz, 2012) and it is directed to degradation by the 26S proteasome after removal of the N-glycans by Png1p (Suzuki et al., 2000; Hirsch et al., 2003).
Glycoengineering Strategies in Yeasts
The humanization of the yeast N-glycans consists of two main objectives, first, to create suitable N-glycan structures that can act as substrates for the subsequent glycan maturation steps and, second, to introduce the required mammalian mannosidase and glycosyltransferase activities. Here, we focus on the steps and approaches leading to the formation of the simplest complex-type N-glycan GlcNAc2Man3GlcNAc2. Undesired and not well documented side effects of the glycoengineering are often growth defects that can stem from the approach chosen for generation of the glycan acceptor and from the introduction of the required glycan processing enzymes into the Golgi apparatus (Jacobs et al., 2009; Song et al., 2010; Parsaie Nasab et al., 2013).
In mammals, the first glycan processing steps taking place in the Golgi apparatus are the trimming of the remaining α1-2 linked mannoses from Man8GlcNAc2 by α-1,2-mannosidases IA, IB and IC (Bause et al., 1993; Tremblay et al., 1998; Tremblay and Herscovics, 2000). After mannosidase I trimming, the Man5GlcNAc2 glycan receives a β1-2 linked GlcNAc residue from GlcNAc transferase I (GnTI), forming a hybrid-type GlcNAcMan5GlcNAc2 glycan (Oppenheimer and Hill, 1981). The pathway towards complex-type glycans proceeds by trimming of the two remaining mannose residues from the B and C branches by α-mannosidases II and IIx (Tabas and Kornfeld, 1978; Misago et al., 1995; Shah et al., 2008). The α1-6 linked mannose of the GlcNAcMan3GlcNAc2 glycan receives a β1-2 linked GlcNAc residue from GlcNAc transferase II (GnTII), forming the complex-type GlcNAc2Man3GlcNAc2 glycan (Bendiak and Schachter, 1987). Depending on the sequence of these processing steps, Man8GlcNAc2, and Man5GlcNAc2 glycans can be selected as starting point for humanization of N-glycosylation pathway in the Golgi apparatus. Furthermore, from in vitro experiments it is known that GnTI can also act on Man3GlcNAc2 glycan substrates (Vella et al., 1984) and thus Man3GlcNAc2 glycans can serve as third road towards the formation of complex-type N-glycans.
Utilizing the Man8GlcNAc2 as the starting structure, the mammalian N-glycosylation pathway must be reproduced introducing mannosidase activities (ManI and ManII) and glycosyltransferases (GnTI and GnTII) into the Golgi apparatus (Figure 2, route A). By applying an extensive screening approach appropriate genetic constructs for optimal enzymatic activities were identified that led to a strain producing proteins with complex-type GlcNAc2Man3GlcNAc2 of very high homogeneity in P. pastoris (Hamilton et al., 2003).
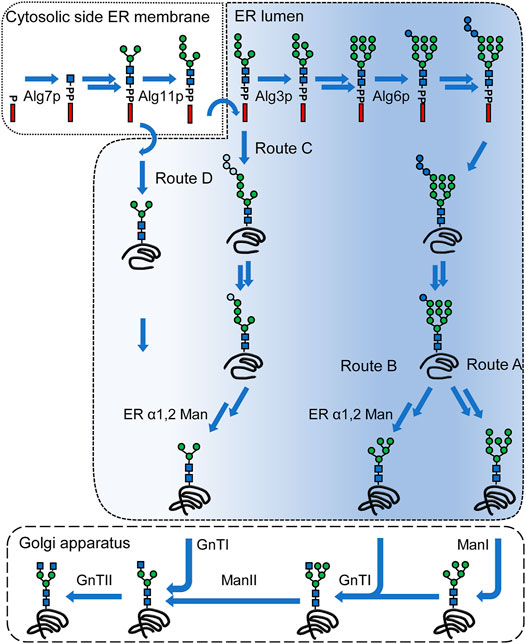
FIGURE 2. N-linked glycosylation pathways leading to complex-type N-glycans. A simplified lipid-linked oligosaccharide (LLO) biosynthesis pathway is depicted in the top of the figure. LLO synthesis is initiated on the cytoplasmic side of the ER membrane by Alg7p. A Man5GlcNAc2 structure is assembled on the cytoplasmic side by the consecutive action of the mannosyltransferases Alg13/14p, Alg1p, Alg2p and Alg11p. This structure is flipped into the ER lumen, where the modification of the LLO is continued by Alg3p. The B-branch is completed by the action of Alg9p after which the C-branch is started by Alg12p and completed by Alg9p. The triglucosyl cap is added by Alg6p, Alg8p and Alg10p generating a Glc3Man9GlcNAc2. This structure is transferred onto a protein by the oligosaccharyltransferase complex. Two glucose residues are removed and Glc1Man9GlcNAc2 mediates binding to calnexin. After release of the protein from calnexin and folding of the protein, the terminal mannose of the B-branch is removed (route A). N-glycans of glycoproteins undergo trimming and transfer reactions in the Golgi apparatus. First, α1,2 mannosidase activities (ManI) are trimming the α1,2 mannose residues, after which first GlcNAc residue is added by GnTI. The remaining mannose residues are trimmed off by ManII, which create the substrate for GnTII that adds a second GlcNAc residue. Route B) includes an α1,2 mannosidase activity in the ER that gives rise to the Man5GlcNAc2 glycan structure in the ER. This structure is modified in the Golgi apparatus by GnTI and route A and B converge. By deletion of ALG3 gene, LLO biosynthesis is abrogated creating a Man5GlcNAc2 that can be glucosylated to varying extent and is transferred onto a protein (route C). After removal of glucose residues and possible interactions with calnexin, an α1,2 mannosidase activity converts this structure into a Man3GlcNAc2 structure. Alternatively, the Man3GlcNAc2 can be generated biosynthetically by deletion of ALG11 and ALG3 genes and this glycan is transferred onto a protein (route D). The Man3GlcNAc2 glycan structure directly serves as a substrate for GnTI and GnTII. The color gradient indicates the presence or absence of canonical glycan features involved in interaction with calnexin/calreticulin and Yos9p, respectively. Blue circles: glucose, green circles: mannose, blue squares: N-acetylglucosamine. Light blue circles indicate reduced glucosylation of the A-branch. Arrows without names: multiple steps; arrows with names: specific enzymes are marked.
Man5GlcNAc2 glycans formed in the ER can serve as the substrate for GnTI. This approach starts from native ER glycan forms but includes a heterologous α-1,2 mannosidase activity for the trimming of all α1-2 linked mannoses (Figure 2, route B). This strategy was first implemented in S. cerevisiae, where a HDEL-tagged α1-2 mannosidase from Aspergillus saitoi was expressed leading to formation of Man5GlcNAc2 structures and later adapted in P. pastoris, where the expression of an HDEL-tagged α-1,2 mannosidase from Trichoderma reesei eliminated over 85% of the α1-2 linked mannoses (Chiba et al., 1998; Callewaert et al., 2001). Using this strategy ManII, GnTI and GnTII activities are required in the Golgi apparatus and an increasing N-glycan heterogeneity was observed with each additional glycan processing step introduced (Jacobs et al., 2009).
In S. cerevisiae and P. pastoris, using the Man8GlcNAc2 and Man5GlcNAc2 structures as starting point required deletions of genes encoding interfering mannosyltransferases including Och1p, and in S. cerevisiae additionally the mannosyltransferase Mnn1p and phosphomannosyltransferase Mnn4p in order to increase homogeneity of the glycan structure (Chiba et al., 1998; Hamilton et al., 2003; Vervecken et al., 2004).
Alternatively, a Man3GlcNAc2 glycan can be generated in the ER. This can be achieved through deletion of ALG3 gene and expression of a heterologous α1-2 mannosidase activity in the ER (Figure 2, route C). In this approach the α-1,6 linked mannose which serves as acceptor for GnTII is formed during LLO biosynthesis. In order to remove the A-branch, expression of HDEL-tagged versions of α-1,2 mannosidase from A. saitoi or of α-1,2 mannosidase from T. reesei were used and yielded glycoproteins predominantly containing the Man3GlcNAc2 glycan. This approach was implemented in P. pastoris, H. polymorpha, Y. lipolytica, A. niger and A. nidulans, respectively, however, was not effective in K. marxiani (Bobrowicz et al., 2004; Kainz et al., 2008; Oh et al., 2008; De Pourcq et al., 2012b; Lee et al., 2020; Anyaogu et al., 2021). Using this strategy, GnTI and GnTII activities are required in the Golgi apparatus.
Incomplete glucosylation of the A-branch, and thus hypoglycosylation, and inefficient glucosidase I and II trimming are associated with ALG3 deletion in various yeasts (Verostek et al., 1993; Aebi et al., 1996a; Bobrowicz et al., 2004; De Pourcq et al., 2012a; Anyaogu et al., 2021). The low glycosylation site occupancy was tackled by the overexpression of glucosyltransferase ALG6, which was earlier shown to partially restore the glucosylation deficiency in S. cerevisiae (Burda et al., 1999; De Pourcq et al., 2012a), while the interfering glucose residues were eliminated by the overexpression of α-glucosidase II activities (De Pourcq et al., 2012a; Anyaogu et al., 2021). Alternatively, in P. pastoris, the low glycosylation site occupancy was compensated by overexpression of single-subunit OST from Leishmania major (Choi et al., 2012).
Direct biosynthetic generation of Man3GlcNAc2 glycan can be achieved by the deletion of ALG3 and ALG11 genes terminating the LLO biosynthesis at a very early stage. The Man3GlcNAc2 glycan contains a terminal α-1,3 linked and α-1,6 linked mannose residue, respectively, that serve as acceptor for GnTI and GnTII (Figure 2, route D). This strategy has been utilized in H. polymorpha and S. cerevisiae (Song et al., 2010; Parsaie Nasab et al., 2013; Piirainen et al., 2021).
Due to the strong modification of the LLO biosynthesis, measures to compensate for the resulting hypoglycosylation phenotypes are required. In both organisms this was achieved by improving the flipping of the LLO into the ER lumen and in S. cerevisiae by additionally overexpressing a single-subunit OST (Song et al., 2010; Parsaie Nasab et al., 2013). Furthermore, a number of mannosyltransferases compete with GnTI and GnTII for the Man3GlcNAc2 glycan in the Golgi apparatus leading to glycan structures with additional mannose residues that precluded the complete processing into complex type N-glycans and reduce glycan homogeneity (Song et al., 2010; Parsaie Nasab et al., 2013; Piirainen et al., 2016, 2021).
Discussion
Most of the glycoengineering approaches developed for yeasts proceed via the formation of non-native, truncated glycan structures in the ER. To date it is unclear if and to which extent these deviating structures might impact the quality of the produced glycoproteins or affect their turnover by ERAD. Most of the studies on ER protein quality control systems were done with artificial folding-deficient glycoproteins. However, how about folding-proficient glycoproteins that would be ultimately produced in glycoengineered yeasts? Among these relevant proteins are antibodies, and the comparably smaller proteins erythropoietin, granulocyte-macrophage colony-stimulating factor, and interleukin 10 that were expressed in glycoengineered P. pastoris or S. cerevisiae (Hamilton et al., 2006; Li et al., 2006; Jacobs et al., 2009; Parsaie Nasab et al., 2013; Piirainen et al., 2021). Importantly, these glycoproteins do not depend on glycans for proper folding and can be manufactured either as aglycones, in glycosylation incompetent host cells such as Escherichia coli or in vitro systems (Higgins, 2010; Yin et al., 2012). Thus, it is plausible that the altered glycan structures do not directly lead to misfolding of these proteins. However, they might affect the folding kinetics and lead to undesired interaction with the ERAD system.
IgG molecules have been shown to interact with molecular chaperones, protein disulfide isomerases and peptidylprolyl isomerase activities. Moreover, UGGT, and small amounts of calnexin were detected in samples of immunoprecipitated IgG indicating the involvement of the calnexin cycle in the folding of IgG (Meunier et al., 2002). In vitro studies with yeast Cne1p and chemically unfolded egg yolk IgY carrying Glc1Man7-9GlcNAc2 structures indicated that Cne1p reduces aggregation (Xu et al., 2004a). Whether and to which extent the antibody folding process is dependent on Cne1p in vivo is unknown. Glycan structures formed in both glycoengineering approaches that are based on altering the LLO biosynthesis partially or completely bypass the calnexin-dependent protein folding (Figure 2, route C and D).
The exposed α1-6 linked mannose on the Man7GlcNAc2 glycan is the canonical glycan motif recognized by Yos9p (Szathmary et al., 2005; Clerc et al., 2009). The identical exposed α1-6 linked mannose is also generated by expression of α1,2 mannosidase activity in ER that trims glycans to a Man5GlcNAc2 structure but lacks the complete A-branch (Figure 2, route B). Whether this glycan is recognized by Yos9p is unknown. Alternatively, exposed α1-6 linked mannose residues, such as the one generated by ALG3 deletion are recognized by Yos9p (Szathmary et al., 2005; Clerc et al., 2009). The identical α1-6 linked mannose is present in ALG3 deletion expressing α1,2 mannosidase activity and ALG11 ALG3 deletion strains, though the interaction of this glycan with Yos9p remains unknown (Figure 2, route C,D). Overall, the glycoengineering approaches have the potential to generate glycan structures that are recognized by Yos9p without the need for prior processing by Htm1p-Pdi1p complex.
What is the impact of ERAD system on the clearance of an IgG? In wild-type S. cerevisiae, deletion of ERAD components HRD1, HRD3, YOS9, HMT1 and UBC7 delayed IgG cellular clearance. Moreover, antibody clearance in an ALG3 deletion strain with functional ERAD was comparable to the clearance in wild-type strain indicating that an exposed α1-6 linked mannose is sufficient for ER clearance (de Ruijter and Frey, 2015). Furthermore, deletion of HRD1, but not of HTM1 or YOS9 reduced clearance rates in an ALG3 ALG11 deletion strain (Piirainen and Frey, 2020). This indicates that glycan-independent mechanisms additionally can contribute to IgG clearance in yeast.
In summary, the modifications introduced by glycoengineering into the N-glycan processing pathway have the potential to impair glycan-dependent protein folding, i.e., by by-passing calnexin and to generate glycan structure that are recognized by ERAD. However, our current understanding is incomplete and further studies will be required.
Author Contributions
MP collected the majority of the background information. AF and MP contributed equally to the writing of the manuscript.
Conflict of Interest
The authors declare that the research was conducted in the absence of any commercial or financial relationships that could be construed as a potential conflict of interest.
Publisher’s Note
All claims expressed in this article are solely those of the authors and do not necessarily represent those of their affiliated organizations, or those of the publisher, the editors and the reviewers. Any product that may be evaluated in this article, or claim that may be made by its manufacturer, is not guaranteed or endorsed by the publisher.
References
Aebi, M., Gassenhuber, J., Domdey, H., and Heesen, S. t. (1996a). Cloning and Characterization of the ALG3 Gene of Saccharomyces cerevisiae. Glycobiology 6, 439–444. doi:10.1093/glycob/6.4.439
Aebi, M., Gassenhuber, J., Domdey, H., and Heesen, S. t. (1996b). Cloning and Characterization of the ALG3 Gene of Saccharomyces cerevisiae. Glycobiology 6, 439–444. doi:10.1093/glycob/6.4.439
Anyaogu, D. C., Hansen, A. H., Hoof, J. B., Majewska, N. I., Contesini, F. J., Paul, J. T., et al. (2021). Glycoengineering of Aspergillus nidulans to Produce Precursors for Humanized N-Glycan Structures. Metab. Eng. 67, 153–163. doi:10.1016/j.ymben.2021.06.001
Barnes, G., Hansen, W. J., Holcomb, C. L., and Rine, J. (1984). Asparagine-linked Glycosylation in Saccharomyces cerevisiae: Genetic Analysis of an Early Step. Mol. Cell. Biol. 4, 2381–2388. doi:10.1128/mcb.4.11.2381
Bause, E., Bieberich, E., Rolfs, A., Volker, C., and Schmidt, B. (1993). Molecular Cloning and Primary Structure of Man9-Mannosidase from Human Kidney. Eur. J. Biochem. 217, 535–540. doi:10.1111/j.1432-1033.1993.tb18274.x
Bays, N. W., Gardner, R. G., Seelig, L. P., Joazeiro, C. A., and Hampton, R. Y. (2001). Hrd1p/Der3p Is a Membrane-Anchored Ubiquitin Ligase Required for ER-Associated Degradation. Nat. Cell. Biol. 3, 24–29. doi:10.1038/35050524
Bendiak, B., and Schachter, H. (1987). Control of Glycoprotein Synthesis. Kinetic Mechanism, Substrate Specificity, and Inhibition Characteristics of UDP-N-Acetylglucosamine:alpha-D-Mannoside Beta 1-2 N-Acetylglucosaminyltransferase II from Rat Liver. J. Biol. Chem. 262, 5784–5790. doi:10.1016/s0021-9258(18)45643-8
Bickel, T., Lehle, L., Schwarz, M., Aebi, M., and Jakob, C. A. (2005). Biosynthesis of Lipid-Linked Oligosaccharides in Saccharomyces cerevisiae. J. Biol. Chem. 280, 34500–34506. doi:10.1074/jbc.M506358200
Bobrowicz, P., Davidson, R. C., Li, H., Potgieter, T. I., Nett, J. H., Hamilton, S. R., et al. (2004). Engineering of an Artificial Glycosylation Pathway Blocked in Core Oligosaccharide Assembly in the Yeast Pichia pastoris: Production of Complex Humanized Glycoproteins with Terminal Galactose. Glycobiology 14, 757–766. doi:10.1093/glycob/cwh104
Burda, P., and Aebi, M. (1998). The ALG10 Locus of Saccharomyces cerevisiae Encodes the Alpha-1,2 Glucosyltransferase of the Endoplasmic Reticulum: the Terminal Glucose of the Lipid-Linked Oligosaccharide Is Required for Efficient N-Linked Glycosylation. Glycobiology 8, 455–462. doi:10.1093/glycob/8.5.455
Burda, P., Jakob, C. A., Beinhauer, J., Hegemann, J. H., and Aebi, M. (1999). Ordered Assembly of the Asymmetrically Branched Lipid-Linked Oligosaccharide in the Endoplasmic Reticulum Is Ensured by the Substrate Specificity of the Individual Glycosyltransferases. Glycobiology 9, 617–625. doi:10.1093/glycob/9.6.617
Burda, P., te Heesen, S., Brachat, A., Wach, A., Düsterhöft, A., Aebi, M., et al. (1996). Stepwise Assembly of the Lipid-Linked Oligosaccharide in the Endoplasmic Reticulum of Saccharomyces cerevisiae: Identification of the ALG9 Gene Encoding a Putative Mannosyl Transferase. Proc. Natl. Acad. Sci. U.S.A. 93, 7160–7165. doi:10.1073/pnas.93.14.7160
Buschhorn, B. A., Kostova, Z., Medicherla, B., and Wolf, D. H. (2004). A Genome-wide Screen Identifies Yos9p as Essential for ER-Associated Degradation of Glycoproteins. FEBS Lett. 577, 422–426. doi:10.1016/j.febslet.2004.10.039
Callewaert, N., Laroy, W., Cadirgi, H., Geysens, S., Saelens, X., Min Jou, W., et al. (2001). Use of HDEL-taggedTrichoderma Reeseimannosyl Oligosaccharide 1,2-α-D-Mannosidase forN-Glycan Engineering inPichia Pastoris. FEBS Lett. 503, 173–178. doi:10.1016/s0014-5793(01)02676-x
Camirand, A., Heysen, A., Grondin, B., and Herscovics, A. (1991). Glycoprotein Biosynthesis in Saccharomyces cerevisiae. Isolation and Characterization of the Gene Encoding a Specific Processing Alpha-Mannosidase. J. Biol. Chem. 266, 15120–15127. doi:10.1016/s0021-9258(18)98594-7
Carvalho, P., Goder, V., and Rapoport, T. A. (2006). Distinct Ubiquitin-Ligase Complexes Define Convergent Pathways for the Degradation of ER Proteins. Cell. 126, 361–373. doi:10.1016/j.cell.2006.05.043
Carvalho, P., Stanley, A. M., and Rapoport, T. A. (2010). Retrotranslocation of a Misfolded Luminal ER Protein by the Ubiquitin-Ligase Hrd1p. Cell. 143, 579–591. doi:10.1016/j.cell.2010.10.028
Chantret, I., Dancourt, J., Barbat, A., and Moore, S. E. H. (2005). Two Proteins Homologous to the N- and C-Terminal Domains of the Bacterial Glycosyltransferase Murg Are Required for the Second Step of Dolichyl-Linked Oligosaccharide Synthesis in Saccharomyces cerevisiae. J. Biol. Chem. 280, 9236–9242. doi:10.1074/jbc.M413941200
Chiba, Y., Suzuki, M., Yoshida, S., Yoshida, A., Ikenaga, H., Takeuchi, M., et al. (1998). Production of Human Compatible High Mannose-type (Man5GlcNAc2) Sugar Chains in Saccharomyces cerevisiae. J. Biol. Chem. 273, 26298–26304. doi:10.1074/jbc.273.41.26298
Choi, B.-K., Warburton, S., Lin, H., Patel, R., Boldogh, I., Meehl, M., et al. (2012). Improvement of N-Glycan Site Occupancy of Therapeutic Glycoproteins Produced in Pichia pastoris. Appl. Microbiol. Biotechnol. 95, 671–682. doi:10.1007/s00253-012-4067-3
Cipollo, J. F., Trimble, R. B., Chi, J. H., Yan, Q., and Dean, N. (2001). The Yeast ALG11 Gene Specifies Addition of the Terminal α1,2-Man to the Man5GlcNAc2-PP-dolicholN-Glycosylation Intermediate Formed on the Cytosolic Side of the Endoplasmic Reticulum. J. Biol. Chem. 276, 21828–21840. doi:10.1074/jbc.M010896200
Clerc, S., Hirsch, C., Oggier, D. M., Deprez, P., Jakob, C., Sommer, T., et al. (2009). Htm1 Protein Generates the N-Glycan Signal for Glycoprotein Degradation in the Endoplasmic Reticulum. J. Cell. Biol. 184, 159–172. doi:10.1083/jcb.200809198
Couto, J. R., Huffaker, T. C., and Robbins, P. W. (1984). Cloning and Expression in Escherichia coli of a Yeast Mannosyltransferase from the Asparagine-Linked Glycosylation Pathway. J. Biol. Chem. 259, 378–382. doi:10.1016/s0021-9258(17)43670-2
De Pourcq, K., Tiels, P., Van Hecke, A., Geysens, S., Vervecken, W., and Callewaert, N. (2012a). Engineering Yarrowia Lipolytica to Produce Glycoproteins Homogeneously Modified with the Universal Man3GlcNAc2 N-Glycan Core. PLoS One 7, e39976. doi:10.1371/journal.pone.0039976
De Pourcq, K., Vervecken, W., Dewerte, I., Valevska, A., Van Hecke, A., and Callewaert, N. (2012b). Engineering the Yeast Yarrowia Lipolytica for the Production of Therapeutic Proteins Homogeneously Glycosylated with Man8GlcNAc2 and Man5GlcNAc2. Microb. Cell. Fact. 11, 53. doi:10.1186/1475-2859-11-53
de Ruijter, J. C., and Frey, A. D. (2015). Analysis of Antibody Production in Saccharomyces cerevisiae: Effects of ER Protein Quality Control Disruption. Appl. Microbiol. Biotechnol. 99, 9061–9071. doi:10.1007/s00253-015-6807-7
Fernández, F. S., Trombetta, S. E., Hellman, U., and Parodi, A. J. (1994). Purification to Homogeneity of UDP-Glucose:glycoprotein Glucosyltransferase from Schizosaccharomyces pombe and Apparent Absence of the Enzyme Fro Saccharomyces cerevisiae. J. Biol. Chem. 269, 30701
Frank, C. G., and Aebi, M. (2005). ALG9 Mannosyltransferase Is Involved in Two Different Steps of Lipid-Linked Oligosaccharide Biosynthesis. Glycobiology 15, 1156–1163. doi:10.1093/glycob/cwj002
Frank, C. G., Sanyal, S., Rush, J. S., Waechter, C. J., and Menon, A. K. (2008). Does Rft1 Flip an N-Glycan Lipid Precursor? Nature 454, E3–E4. doi:10.1038/nature07165
Friedlander, R., Jarosch, E., Urban, J., Volkwein, C., and Sommer, T. (2000). A Regulatory Link between ER-Associated Protein Degradation and the Unfolded-Protein Response. Nat. Cell. Biol. 2, 379–384. doi:10.1038/35017001
Friedmann, E., Salzberg, Y., Weinberger, A., Shaltiel, S., and Gerst, J. E. (2002). YOS9, the Putative Yeast Homolog of a Gene Amplified in Osteosarcomas, Is Involved in the Endoplasmic Reticulum (ER)-Golgi Transport of GPI-Anchored Proteins. J. Biol. Chem. 277, 35274–35281. doi:10.1074/jbc.M201044200
Gauss, R., Jarosch, E., Sommer, T., and Hirsch, C. (2006). A Complex of Yos9p and the HRD Ligase Integrates Endoplasmic Reticulum Quality Control into the Degradation Machinery. Nat. Cell. Biol. 8, 849–854. doi:10.1038/ncb1445
Gauss, R., Kanehara, K., Carvalho, P., Ng, D. T. W., and Aebi, M. (2011). A Complex of Pdi1p and the Mannosidase Htm1p Initiates Clearance of Unfolded Glycoproteins from the Endoplasmic Reticulum. Mol. Cell. 42, 782–793. doi:10.1016/j.molcel.2011.04.027
Hamilton, S. R., Bobrowicz, P., Bobrowicz, B., Davidson, R. C., Li, H., Mitchell, T., et al. (2003). Production of Complex Human Glycoproteins in Yeast. Science 301, 1244–1246. doi:10.1126/science.1088166
Hamilton, S. R., Davidson, R. C., Sethuraman, N., Nett, J. H., Jiang, Y., Rios, S., et al. (2006). Humanization of Yeast to Produce Complex Terminally Sialylated Glycoproteins. Science 313, 1441–1443. doi:10.1126/science.1130256
Helenius, A., and Aebi, M. (2004). Roles of N-Linked Glycans in the Endoplasmic Reticulum. Annu. Rev. Biochem. 73, 1019–1049. doi:10.1146/annurev.biochem.73.011303.073752
Helenius, J., Ng, D. T. W., Marolda, C. L., Walter, P., Valvano, M. A., and Aebi, M. (2002). Translocation of Lipid-Linked Oligosaccharides across the ER Membrane Requires Rft1 Protein. Nature 415, 447–450. doi:10.1038/415447a
Higgins, E. (2010). Carbohydrate Analysis throughout the Development of a Protein Therapeutic. Glycoconj J. 27, 211–225. doi:10.1007/s10719-009-9261-x
Hiller, M. M., Finger, A., Schweiger, M., and Wolf, D. H. (1996). ER Degradation of a Misfolded Luminal Protein by the Cytosolic Ubiquitin-Proteasome Pathway. Science 273, 1725–1728. doi:10.1126/science.273.5282.1725
Hirsch, C., Blom, D., and Ploegh, H. L. (2003). A Role for N-Glycanase in the Cytosolic Turnover of Glycoproteins. EMBO J. 22, 1036–1046. doi:10.1093/emboj/cdg107
Huffaker, T. C., and Robbins, P. W. (1982). Temperature-sensitive Yeast Mutants Deficient in Asparagine-Linked Glycosylation. J. Biol. Chem. 257, 3203–3210. doi:10.1016/s0021-9258(19)81096-7
Huffaker, T. C., and Robbins, P. W. (1983). Yeast Mutants Deficient in Protein Glycosylation. Proc. Natl. Acad. Sci. U.S.A. 80, 7466–7470. doi:10.1073/pnas.80.24.7466
Jacobs, P. P., Geysens, S., Vervecken, W., Contreras, R., and Callewaert, N. (2009). Engineering Complex-type N-Glycosylation in Pichia pastoris Using GlycoSwitch Technology. Nat. Protoc. 4, 58–70. doi:10.1038/nprot.2008.213
Jakob, C. A., Burda, P., Roth, J., and Aebi, M. (1998). Degradation of Misfolded Endoplasmic Reticulum Glycoproteins in saccharomyces Cerevisiae Is Determined by a Specific Oligosaccharide Structure. J. Cell. Biol. 142, 1223–1233. doi:10.1083/jcb.142.5.1223
Kainz, E., Gallmetzer, A., Hatzl, C., Nett, J. H., Li, H., Schinko, T., et al. (2008). N-glycan Modification in Aspergillus Species. Appl. Environ. Microbiol. 74, 1076–1086. doi:10.1128/AEM.01058-07
Kamiya, Y., Kamiya, D., Yamamoto, K., Nyfeler, B., Hauri, H.-P., and Kato, K. (2008). Molecular Basis of Sugar Recognition by the Human L-type Lectins ERGIC-53, VIPL, and VIP36. J. Biol. Chem. 283, 1857–1861. doi:10.1074/jbc.M709384200
Kniss, A., Kazemi, S., Löhr, F., Berger, M., Rogov, V. V., Güntert, P., et al. (2018). Structural Investigation of Glycan Recognition by the ERAD Quality Control Lectin Yos9. J. Biomol. NMR 72, 1–10. doi:10.1007/s10858-018-0201-6
Kukuruzinska, M. A., and Robbins, P. W. (1987). Protein Glycosylation in Yeast: Transcript Heterogeneity of the ALG7 Gene. Proc. Natl. Acad. Sci. U.S.A. 84, 2145–2149. doi:10.1073/pnas.84.8.2145
Lee, M.-H., Hsu, T.-L., Lin, J.-J., Lin, Y.-J., Kao, Y.-Y., Chang, J.-J., et al. (2020). Constructing a Human Complex Type N-Linked Glycosylation Pathway in Kluyveromyces Marxianus. PLoS One 15, e0233492. doi:10.1371/journal.pone.0233492
Li, H., Sethuraman, N., Stadheim, T. A., Zha, D., Prinz, B., Ballew, N., et al. (2006). Optimization of Humanized IgGs in Glycoengineered Pichia pastoris. Nat. Biotechnol. 24, 210–215. doi:10.1038/nbt1178
Liu, Y.-C., Fujimori, D. G., and Weissman, J. S. (2016). Htm1p-Pdi1p Is a Folding-Sensitive Mannosidase that Marks N-Glycoproteins for ER-Associated Protein Degradation. Proc. Natl. Acad. Sci. U.S.A. 113, E4015–E4024. doi:10.1073/pnas.1608795113
Martinez Benitez, E., Stolz, A., and Wolf, D. H. (2011). Yos9, a Control Protein for Misfolded Glycosylated and Non-glycosylated Proteins in ERAD. FEBS Lett. 585, 3015–3019. doi:10.1016/j.febslet.2011.08.021
Mehnert, M., Sommer, T., and Jarosch, E. (2013). Der1 Promotes Movement of Misfolded Proteins through the Endoplasmic Reticulum Membrane. Nat. Cell. Biol. 15, 1186–1196. doi:10.1038/ncb282210.1038/ncb2882
Mehnert, M., Sommermeyer, F., Berger, M., Kumar Lakshmipathy, S., Gauss, R., Aebi, M., et al. (2015). The Interplay of Hrd3 and the Molecular Chaperone System Ensures Efficient Degradation of Malfolded Secretory Proteins. MBoC 26, 185–194. doi:10.1091/mbc.E14-07-1202
Metzger, M. B., Liang, Y.-H., Das, R., Mariano, J., Li, S., Li, J., et al. (2013). A Structurally Unique E2-Binding Domain Activates Ubiquitination by the ERAD E2, Ubc7p, through Multiple Mechanisms. Mol. Cell. 50, 516–527. doi:10.1016/j.molcel.2013.04.004
Meunier, L., Usherwood, Y.-K., Chung, K. T., and Hendershot, L. M. (2002). A Subset of Chaperones and Folding Enzymes Form Multiprotein Complexes in Endoplasmic Reticulum to Bind Nascent Proteins. MBoC 13, 4456–4469. doi:10.1091/mbc.E0210.1091/mbc.e02-05-0311
Misago, M., Liao, Y. F., Kudo, S., Eto, S., Mattei, M. G., Moremen, K. W., et al. (1995). Molecular Cloning and Expression of cDNAs Encoding Human Alpha-Mannosidase II and a Previously Unrecognized Alpha-Mannosidase IIx Isozyme. Proc. Natl. Acad. Sci. U.S.A. 92, 11766–11770. doi:10.1073/pnas.92.25.11766
O'Reilly, M. K., Zhang, G., and Imperiali, B. (2006). In Vitro evidence for the Dual Function of Alg2 and Alg11: Essential Mannosyltransferases in N-Linked Glycoprotein Biosynthesis. Biochemistry 45, 9593–9603. doi:10.1021/bi060878o
Oh, D.-B., Park, J.-S., Kim, M. W., Cheon, S. A., Kim, E. J., Moon, H. Y., et al. (2008). Glycoengineering of the Methylotrophic yeastHansenula Polymorpha for the Production of Glycoproteins with Trimannosyl coreN-Glycan by Blocking Core Oligosaccharide Assembly. Biotechnol. J. 3, 659–668. doi:10.1002/biot.200700252
Oppenheimer, C. L., and Hill, R. L. (1981). Purification and Characterization of a Rabbit Liver Alpha 1 Goes to 3 Mannoside Beta 1 Goes to 2 N-Acetylglucosaminyltransferase. J. Biol. Chem. 256, 799–804. doi:10.1016/s0021-9258(19)70047-7
Parlati, F., Dominguez, M., Bergeron, J. J. M., and Thomas, D. Y. (1995). Saccharomyces cerevisiae CNE1 Encodes an Endoplasmic Reticulum (ER) Membrane Protein with Sequence Similarity to Calnexin and Calreticulin and Functions as a Constituent of the ER Quality Control Apparatus. J. Biol. Chem. 270, 244–253. doi:10.1074/jbc.270.1.244
Parsaie Nasab, F., Aebi, M., Bernhard, G., and Frey, A. D. (2013). A Combined System for Engineering Glycosylation Efficiency and Glycan Structure in Saccharomyces cerevisiae. Appl. Environ. Microbiol. 79, 997–1007. doi:10.1128/AEM.02817-12
Pfeiffer, A., Stephanowitz, H., Krause, E., Volkwein, C., Hirsch, C., Jarosch, E., et al. (2016). A Complex of Htm1 and the Oxidoreductase Pdi1 Accelerates Degradation of Misfolded Glycoproteins. J. Biol. Chem. 291, 12195–12207. doi:10.1074/jbc.M115.703256
Piirainen, M. A., Boer, H., De Ruijter, J. C., and Frey, A. D. (2016). A Dual Approach for Improving Homogeneity of a Human-type N-Glycan Structure in Saccharomyces cerevisiae. Glycoconj. J. 33, 189–199. doi:10.1007/s10719-016-9656-4
Piirainen, M. A., and Frey, A. D. (2020). Investigating the Role of ERAD on Antibody Processing in Glycoengineered Saccharomyces cerevisiae. FEMS Yeast Res. 20, 1–11. doi:10.1093/femsyr/foaa002
Piirainen, M. A., Salminen, H., and Frey, A. D. (2021). Production of Galactosylated Complex-type N-Glycans in Glycoengineered Saccharomyces cerevisiae. Appl. Microbiol. Biotechnol. 106, 301–315. doi:10.1007/s00253-021-11727-8
Quan, E. M., Kamiya, Y., Kamiya, D., Denic, V., Weibezahn, J., Kato, K., et al. (2008). Defining the Glycan Destruction Signal for Endoplasmic Reticulum-Associated Degradation. Mol. Cell. 32, 870–877. doi:10.1016/j.molcel.2008.11.017
Reiss, G., Heesen, S. t., Zimmerman, J., Robbins, P., and Aebi, M. (1996). Isolation of the ALG6 Locus of Saccharomyces cerevisiae Required for Glucosylation in the N-Linked Glycosylation Pathway. Glycobiology 6, 493–498. doi:10.1093/glycob/6.5.493
Runge, K. W., and Robbins, P. W. (1986). A New Yeast Mutation in the Glucosylation Steps of the Asparagine-Linked Glycosylation Pathway. Formation of a Novel Asparagine-Linked Oligosaccharide Containing Two Glucose Residues. J. Biol. Chem. 261, 15582–15590. doi:10.1016/s0021-9258(18)66754-7
Rush, J. S., Gao, N., Lehrman, M. A., Matveev, S., and Waechter, C. J. (2009). Suppression of Rft1 Expression Does Not Impair the Transbilayer Movement of Man5GlcNAc2-P-P-Dolichol in Sealed Microsomes from Yeast. J. Biol. Chem. 284, 19835–19842. doi:10.1074/jbc.M109.000893
Sanyal, S., Frank, C. G., and Menon, A. K. (2008). Distinct Flippases Translocate Glycerophospholipids and Oligosaccharide Diphosphate Dolichols across the Endoplasmic Reticulum. Biochemistry 47, 7937–7946. doi:10.1021/bi800723n
Sato, K., and Nakano, A. (2002). Emp47p and its Close Homolog Emp46p Have a Tyrosine-Containing Endoplasmic Reticulum Exit Signal and Function in Glycoprotein Secretion inSaccharomyces Cerevisiae. MBoC 13, 2518–2532. doi:10.1091/mbc.e02-01-0027
Satoh, T., Sato, K., Kanoh, A., Yamashita, K., Yamada, Y., Igarashi, N., et al. (2006). Structures of the Carbohydrate Recognition Domain of Ca2+-independent Cargo Receptors Emp46p and Emp47p. J. Biol. Chem. 281, 10410–10419. doi:10.1074/jbc.M512258200
Shah, N., Kuntz, D. A., and Rose, D. R. (2008). Golgi α-mannosidase II Cleaves Two Sugars Sequentially in the Same Catalytic Site. Proc. Natl. Acad. Sci. U.S.A. 105, 9570–9575. doi:10.1073/pnas.0802206105
Sharma, C. B., Knauer, R., and Lehle, L. (2001). Biosynthesis of Lipid-Linked Oligosaccharides in Yeast: the ALG3 Gene Encodes the Dol-P-Man:Man5GlcNAc2-PP-Dol Mannosyltransferase. Biol. Chem. 382, 321–328. doi:10.1515/BC.2001.039
Smith, M. H., Rodriguez, E. H., and Weissman, J. S. (2014). Misfolded Proteins Induce Aggregation of the Lectin Yos9. J. Biol. Chem. 289, 25670–25677. doi:10.1074/jbc.M114.583344
Song, H., Qian, W., Wang, H., and Qiu, B. (2010). Identification and Functional Characterization of the HpALG11 and the HpRFT1 Genes Involved in N-Linked Glycosylation in the Methylotrophic Yeast Hansenula Polymorpha. Glycobiology 20, 1665–1674. doi:10.1093/glycob/cwq121
Stagljar, I., Te Heesen, S., and Aebi, M. (1994). New Phenotype of Mutations Deficient in Glucosylation of the Lipid-Linked Oligosaccharide: Cloning of the ALG8 Locus. Proc. Natl. Acad. Sci. U.S.A. 91, 5977–5981. doi:10.1073/pnas.91.13.5977
Suzuki, T., Park, H., Hollingsworth, N. M., Sternglanz, R., and Lennarz, W. J. (2000). PNG1, a Yeast Gene Encoding a Highly Conserved peptide:N-Glycanase. J. Cell. Biol. 149, 1039–1052. doi:10.1083/jcb.149.5.1039
Szathmary, R., Bielmann, R., Nita-Lazar, M., Burda, P., and Jakob, C. A. (2005). Yos9 Protein Is Essential for Degradation of Misfolded Glycoproteins and May Function as Lectin in ERAD. Mol. Cell. 19, 765–775. doi:10.1016/j.molcel.2005.08.015
Tabas, I., and Kornfeld, S. (1978). The Synthesis of Complex-type Oligosaccharides. III. Identification of an Alpha-D-Mannosidase Activity Involved in a Late Stage of Processing of Complex-type Oligosaccharides. J. Biol. Chem. 253, 7779–7786. doi:10.1016/s0021-9258(17)34437-x
Tremblay, L. O., Dyke, N. C., and Herscovics, A. (1998). Molecular Cloning, Chromosomal Mapping and Tissue-specific Expression of a Novel Human 1,2-mannosidase Gene Involved in N-Glycan Maturation. Glycobiology 8, 585–595. doi:10.1093/glycob/8.6.585
Tremblay, L. O., and Herscovics, A. (2000). Characterization of a cDNA Encoding a Novel Human Golgi α1,2-Mannosidase (IC) Involved in N-Glycan Biosynthesis. J. Biol. Chem. 275, 31655–31660. doi:10.1074/jbc.M004935200
Trombetta, E. S., Simons, J. F., and Helenius, A. (1996). Endoplasmic Reticulum Glucosidase II Is Composed of a Catalytic Subunit, Conserved from Yeast to Mammals, and a Tightly Bound Noncatalytic HDEL-Containing Subunit. J. Biol. Chem. 271, 27509–27516. doi:10.1074/jbc.271.44.27509
Vasic, V., Denkert, N., Schmidt, C. C., Riedel, D., Stein, A., and Meinecke, M. (2020). Hrd1 Forms the Retrotranslocation Pore Regulated by Auto-Ubiquitination and Binding of Misfolded Proteins. Nat. Cell. Biol. 22, 274–281. doi:10.1038/s41556-020-0473-4
Vella, G. J., Paulsen, H., and Schachter, H. (1984). Control of Glycoprotein Synthesis. IX. A Terminal Manα1-3Manβ1- Sequence in the Substrate Is the Minimum Requirement for UDP-N-Acetyl-D-Glucosamine:α-D- Mannoside (GlcNAc to Manα1-3) β2-N-acetylglucosaminyltransferase I. Can. J. Biochem. Cell. Biol. 62, 409–417. doi:10.1139/o84-056
Verchère, A., Cowton, A., Jenni, A., Rauch, M., Häner, R., Graumann, J., et al. (2021). Complexity of the Eukaryotic Dolichol-Linked Oligosaccharide Scramblase Suggested by Activity Correlation Profiling Mass Spectrometry. Sci. Rep. 11, 1–14. doi:10.1038/s41598-020-80956-0
Verostek, M. F., Atkinson, P. H., and Trimble, R. B. (1993). Glycoprotein Biosynthesis in the Alg3 Saccharomyces cerevisiae Mutant. II. Structure of Novel Man6-10GlcNAc2 Processing Intermediates on Secreted Invertase. J. Biol. Chem. 268, 12104–12115. doi:10.1016/s0021-9258(19)50314-3
Vervecken, W., Kaigorodov, V., Callewaert, N., Geysens, S., De Vusser, K., and Contreras, R. (2004). In Vivo synthesis of Mammalian-like, Hybrid-type N-Glycans in Pichia pastoris. Appl. Environ. Microbiol. 70, 2639–2646. doi:10.1128/AEM.70.5.2639
Walsh, G. (2018). Biopharmaceutical Benchmarks 2018. Nat. Biotechnol. 36, 1136–1145. doi:10.1038/nbt.4305
Wilkinson, B. M., Purswani, J., and Stirling, C. J. (2006). Yeast GTB1 Encodes a Subunit of Glucosidase II Required for Glycoprotein Processing in the Endoplasmic Reticulum. J. Biol. Chem. 281, 6325–6333. doi:10.1074/jbc.M510455200
Wolf, D. H., and Stolz, A. (2012). The Cdc48 Machine in Endoplasmic Reticulum Associated Protein Degradation. Biochimica Biophysica Acta (BBA) - Mol. Cell. Res. 1823, 117–124. doi:10.1016/j.bbamcr.2011.09.002
Wu, X., Siggel, M., Ovchinnikov, S., Mi, W., Svetlov, V., Nudler, E., et al. (2020). Structural Basis of ER-Associated Protein Degradation Mediated by the Hrd1 Ubiquitin Ligase Complex. Science 368. doi:10.1126/science.aaz2449
Xie, W., Kanehara, K., Sayeed, A., and Ng, D. T. W. (2009). Intrinsic Conformational Determinants Signal Protein Misfolding to the Hrd1/Htm1 Endoplasmic Reticulum-Associated Degradation System. MBoC 20, 3317–3329. doi:10.1091/mbc.e09-03-0231
Xu, X., Azakami, H., and Kato, A. (2004a). P-domain and Lectin Site Are Involved in the Chaperone Function ofSaccharomyces Cerevisiaecalnexin Homologue. FEBS Lett. 570, 155–160. doi:10.1016/j.febslet.2004.06.039
Xu, X., Kanbara, K., Azakami, H., and Kato, A. (2004b). Expression and Characterization of Saccharomyces cerevisiae Cne1p, a Calnexin Homologue. J. Biochem. 135, 615–618. doi:10.1093/jb/mvh074
Keywords: protein N-glycosylation, endoplasmic reticulum associated protein degradation (ERAD), endoplasmic reticulum quality control (ERQC), yeast, glycoengineering
Citation: Piirainen MA and Frey AD (2022) The Impact of Glycoengineering on the Endoplasmic Reticulum Quality Control System in Yeasts. Front. Mol. Biosci. 9:910709. doi: 10.3389/fmolb.2022.910709
Received: 01 April 2022; Accepted: 02 May 2022;
Published: 02 June 2022.
Edited by:
Matthew P. DeLisa, Cornell University, United StatesReviewed by:
Adam Barb, University of Georgia, United StatesJorg De Ruijter, VTT Technical Research Centre of Finland Ltd., Finland
Tadashi Suzuki, Riken, Japan
Copyright © 2022 Piirainen and Frey. This is an open-access article distributed under the terms of the Creative Commons Attribution License (CC BY). The use, distribution or reproduction in other forums is permitted, provided the original author(s) and the copyright owner(s) are credited and that the original publication in this journal is cited, in accordance with accepted academic practice. No use, distribution or reproduction is permitted which does not comply with these terms.
*Correspondence: Alexander D. Frey, YWxleGFuZGVyLmZyZXlAYWFsdG8uZmk=