- 1Department of Urology, The First Affiliated Hospital of Nanjing Medical University, Nanjing, China
- 2Department of Oncology, Xiangyang Central Hospital, Affiliated Hospital of Hubei University of Arts and Science, Xiangyang, China
- 3Department of Urology, The Second Affiliated Hospital of Nanjing Medical University, Nanjing, China
Renal fibrosis is a common feature of chronic kidney disease (CKD), and can lead to the destruction of normal renal structure and loss of kidney function. Little progress has been made in reversing fibrosis in recent years. Ferroptosis is more immunogenic than apoptosis due to the release and activation of damage-related molecular patterns (DAMPs) signals. In this paper, the relationship between renal fibrosis and ferroptosis was reviewed from the perspective of iron metabolism and lipid peroxidation, and some pharmaceuticals or chemicals associated with both ferroptosis and renal fibrosis were summarized. Other programmed cell death and ferroptosis in renal fibrosis were also firstly reviewed for comparison and further investigation.
1 Introduction
Chronic kidney disease (CKD) is an important public health concern causing a high economic cost to the health systems, with an overall prevalence of more than 10% (Hill et al., 2016). Progressive CKD often results in end-stage renal failure and the only options are dialysis or kidney transplantation. Renal fibrosis is a common characteristic of CKD independent of the underlying etiology, leading to the destruction of normal kidney structure and loss of renal function. Renal fibrosis, one of the common characteristics of CKD, includes tubulointerstitial fibrosis and glomerulosclerosis, regardless of the underlying etiology, is synthesized from an imbalance between excessive synthesis and reduced breakdown of the extracellular matrix, and it may be due to normal wound healing response become uncontrolled, with an uncontrolled inflammatory response and myofibroblast proliferation, eventually leading to destruction of normal kidney structure and loss of renal function (el Nahas et al., 1997). Currently, therapies against renal fibrosis exhibit limited effectiveness, and little progress has been made in reversing fibrosis in recent years.
In 2003, Sonam Dolma et al. discovered a new form of cell death induced by erastin without nuclear morphological changes, DNA fragmentation, and caspase-3 activation, which could not be reversed by caspase inhibitors (Dolma et al., 2003) but could be inhibited by iron chelator (Yang and Stockwell, 2008). Subsequently, Dixon named this cell death “ferroptosis” in 2012 (Dixon et al., 2012), which is characterized by lipid peroxidation-mediated membrane damage, leading to iron-dependent regulated necrosis (Stockwell et al., 2017). Different from autophagy, necrosis, pyroptosis, and apoptosis, ferroptosis morphologically exhibits normal nuclei and shrinking mitochondria with condensed mitochondrial membrane, diminished or abolished mitochondria cristae, and ruptured outer mitochondrial membrane (Yagoda et al., 2007; Cao and Dixon, 2016; Latunde-Dada, 2017). The connection between necrotic cell death and long-term renal fibrosis may be dominated by the inflammatory infiltration, which could be defined by the type of necrotic death (Tang et al., 2020). Although our knowledge about the role of ferroptosis in renal fibrosis is still in its infancy, the cytotoxicity of iron and lipid peroxidation has been known since the 1900s and 1950s, respectively (Moore and Hawkes, 1908; Bieri, 1959). Before the proposing of ferroptosis, many studies confirmed that the ferroptosis-related indicators, including GPXs, iron, and lipid peroxides are participated in renal fibrosis (Sponsel et al., 1996; Ueda et al., 1996; Zachara et al., 2004; Himmelfarb, 2005; Dominguez et al., 2006; Seiler et al., 2008). This review is to summarize the relationship between renal fibrosis and ferroptosis from the perspective of iron metabolism and lipid peroxidation.
2 Mechanism of Ferroptosis
2.1 Oxidative Stress and Lipid Peroxidation in Ferroptosis
Ferroptosis is a regulated form of cell death characterized by iron-dependent oxidative stress induced lipid peroxidation (Stockwell et al., 2017). In general, ferroptosis is mainly caused by the inactivation of cellular antioxidant system; it is caspase- and necrosome-independent, which is precisely regulated at epigenetic, transcriptional, post-transcriptional and post-translational levels (Galluzzi et al., 2018).
2.1.1 Oxidative Stress
Oxidative stress is s a disruption of redox signaling and control (Jones, 2006), resulting from either excessive free radicals or insufficient degradation of these free radicals. Oxidative stress is a causative factor in the pathogenesis and pathophysiology of numerous degenerative disorders.
Firstly, ROS and reactive nitrogen species (RNS) are the most common oxidative compounds, which can mediate oxidative stress under various pathophysiological conditions, and are removed by antioxidant defense mechanisms. Secondly, reduced glutathione, is an essential intracellular antioxidant synthesized by the ATP-dependent cytosolic enzymes glutamate-cysteine ligase (GCL) and glutathione synthetase (GSS) in a two-step synthesis of cysteine, glutamate and glycine (Stockwell et al., 2017). Thirdly, extracellular cystine and intracellular cysteine are essential for the maintenance of glutathione biosynthesis and for the inhibition of a type of cell death, which can also be prevented by iron chelators or lipophilic antioxidants (α-tocopherol) (Bannai et al., 1977; Murphy et al., 1989). Cysteine is imported into cells through the oxidized form-cystine of system Xc− where cystine is immediately reduced to cysteine within the cells. Finally, GSH is a major soluble non-enzymatic antioxidant, which relieves the oxidative damage and protects cellular macromolecules, including membrane lipids, against ROS by donating electrons to free radicals. This donation leads to its own oxidation, which converts GSH into glutathione disulfide (GSSG). In this process, glutathione peroxidases (GPXs) play an antioxidant role and converts potentially toxic lipid hydroperoxides (L-OOH) to non-toxic lipid alcohols (L-OH) by buffering H2O2 with GSH (Battin and Brumaghim, 2009).
2.1.2 Lipid Peroxidation
Oxidative stress might impact various macromolecules including lipids, proteins and nucleic acid, leading to the formation of oxidative damage products. Lipid peroxidation, broadly defined as the insertion of hydroperoxyl group into a lipid, has recently been identified as a major driver of ferroptosis (Yang and Stockwell, 2016). This process can be induced by a variety of oxidants (superoxides, H2O2, and highly reactive hydroxyl radicals) or by exposure to xenobiotics and environmental pollutants. As the main component of cellular membranes, lipids have an indispensable role in maintaining cell structural integrity. Polyunsaturated fatty acids (PUFAs), especially arachidonic acid (AA) or adrenic acid (AdA), are most susceptible to peroxidation, which is quite important for the maintenance of cellular membrane fluidity (Figure 1; de Carvalho and Caramujo, 2018).
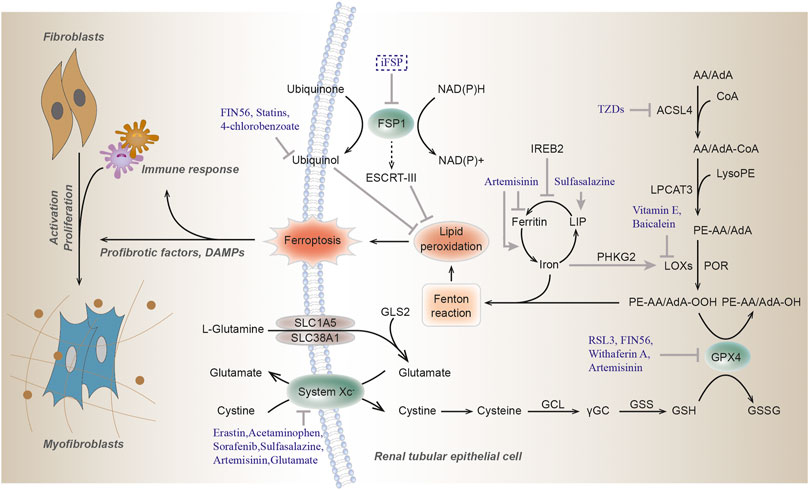
FIGURE 1. Molecular mechanisms of ferroptosis. Ferroptosis is triggered by lethal lipid peroxidation caused by accumulation of intracellular free iron and/or dysfunction of antioxidant system. The commonly used ferroptosis regulators are shown here. Arrows indicate activation, while blunt lines indicate inhibition. GSH, glutathione; GPX4, glutathione peroxidase 4; GSSG, glutathione disulfide; GCL, glutamate-cysteine ligase; γGC, γ-glutamyl cysteine; GSS, glutathione synthetase; AA, arachidonic acid; AdA, adrenic acid; ACSL4, acyl-CoA synthetase 4; TZDs, thiazolidinediones; LPCAT3, lysophosphatidylcholine acyltransferase 3; LOXs, lipoxygenase; POR, cytochrome P450 oxidoreductase; FSP1, Ferroptosis suppressor protein 1; IREB2, iron responsive element binding protein 2; PHKG2, phosphorylase kinase G2; LIP, labile iron pool; GLS2, glutaminase 2; SLC1A5, solute carrier family 1 member 5; SLC38A1, solute carrier family 38 member A; NAD(P)H, nicotinamide adenine dinucleotide phosphate; ESCRT-III, endosomal sorting complex required for transport-III; RSL3, (1S,3R)-RSL3.
In the non-enzymatic pathway, lipid peroxidation can be mediated by carbon- and oxygen-centered radicals initiated by Fenton reaction (see below) or it can also be carried out in an orderly manner by enzyme pathway. Acyl-CoA Synthetase Long Chain Family Member 4 (ACSL4) catalyzes the addition of CoA to the long-chain polyunsaturated bonds of AA or AdA to produce AA or AdA acyl Co-A derivatives, thereby promoting the esterification of PUFA into phospholipids (Kagan et al., 2017). It incorporates cellular membranes with PUFAs and drives ferroptosis through the accumulation of oxidized cellular membrane phospholipids (Doll et al., 2017). Then these derivatives are esterified into phosphatidylethanolamines (PEs) containing AA or AdA (AA-PE or AdA-PE) by Lysophosphatidylcholine Acyltransferase 3 (LPCAT3). Lipoxygenases (LOXs) form a family of iron-containing lipid peroxide enzymes with highly conserved Fe-coordination sphere positioned in a helical core and shows the substrate specificity (Newcomer and Brash, 2015). According to the location specificity of arachidonic acid oxidation, lipoxygenase was named and classified as ALOXE3 (arachidonate lipoxygenase 3), ALOX5 (arachidonate 5-lipoxygenase), ALOX12 (arachidonate 12-lipoxygenase, 12S type), ALOX12B (arachidonate 12-lipoxygenase, 12R type), ALOX15 (arachidonate 15-lipoxygenase), and ALOX15B (arachidonate 15-lipoxygenase type B) in humans. Finally, AA-PE and AdA-PE, were oxidized by lipoxygenases (LOXs) to generate lipid hydroperoxides, thus proximately execute ferroptosis. The antioxidant α-tocopherol and α-tocotrienol could regulate ferroptosis by suppressing the LOX, then generating doubly and triply-oxygenated (15-hydroperoxy)-diacylated phosphatidylethanolamine (PE) species (Kagan et al., 2017). Furthermore, cytochrome P450 oxidoreductase (POR) were recently reported to generate lipid hydroperoxides by an ALOX-independent manner (Figure 1; Zou et al., 2020).
The GPX4, an antioxidant enzyme and a central blocker of ferroptosis, is the only glutathione peroxidase that uses GSH as a co-substrate to reduce phospholipid hydroperoxides to hydroxy phospholipid in the membranes (Yang et al., 2014). Decreased detoxification of GPX4, depletion of GSH and increased LOXs activity are the main driving factors of ferroptosis (Seiler et al., 2008; Yang and Stockwell, 2016). Selenium is a component of selenocysteine at the catalytic site of GPX4. Similar to ferrostatin-1, selenium has a strong inhibitory effect on cerebral hemorrhage and can reduce the concentration of ferroptosis, which is a specific ferroptosis inhibitor (Ingold et al., 2018). In addition, lipophilic antioxidant ubiquinone (CoQ10) exerts a fundamental role in the mitochondrial electron transport chain and can hamper ferroptosis in a GSH-independent manner (Doll et al., 2019). Glutamate is converted from glutamine by glutaminase and is required for the induction of ferroptosis during serum-induced injury after amino acid starvation (Gao et al., 2015; Altman et al., 2016). Then glutamate can also be exchanged for cystine via xCT. Ferroptosis suppressor protein 1 (FSP1), also named as apoptosis-inducing factor mitochondria associated 2 (AIFM2), acts as an NADH-dependent oxidoreductase to catalyze oxidase ubiquinone into reduced ubiquinol and prevents lipid peroxides and incorporation into membranes and lipoproteins, in parallel to the canonical glutathione-based GPX4 pathway (Bersuker et al., 2019; Doll et al., 2019). The newly found Dihydroorotate dehydrogenase (DHODH), inhibits ferroptosis in the mitochondrial intima by reducing the ubiquitin ketone to ubiquitin alcohol, operating in parallel to mitochondrial GPX4, but not to cytosolic GPX4 or FSP1 (Mao et al., 2021; Figure 1).
2.2 Iron Homeostasis and Ferroptosis
Iron is an important trace element that plays an important role in many key biological processes. Iron is a key cofactor in electron transfer processes and redox reactions because of its ability to switch between specific oxidative forms; however, iron’s redox potential might lead to cytotoxicity when iron is overloaded (Gozzelino and Arosio, 2016).
2.2.1 Iron Overload Induces Ferroptosis
The excessive intracellular labile irons can directly participate in the redox cycle, providing electrons to expedite ROS production and lipid peroxidation via the Fenton reaction, a series of reactions in which this labile iron reacts with endogenous hydrogen peroxide or superoxide to form oxygen centered radicals (Fe2+ + H2O2
Compelling evidence indicated that ferroptosis can be motivated by elevated iron levels and inhibited by iron chelation (Dixon et al., 2012). Several proteins impacting iron homeostasis or transportation can influence the sensitivity of ferroptosis (Figure 2; Dixon et al., 2012; Sun et al., 2015; Yuan et al., 2016; Kim et al., 2018). Ferritin plays a major role in protecting against ferroptosis through storing intracellular iron (Fang et al., 2020). It further emphasizes the essentiality of iron in ferroptosis, which requires transferrin and TFR1 as specific markers of ferroptosis (Gao et al., 2015; Feng et al., 2020). DMT1 inhibition could block the translocation of lysosomal iron, leading to the accumulation of lysosomal iron and cell death with ferroptotic features (Song et al., 2021). NCOA4-mediated ferritinophagy promotes ferroptosis by degradation of ferritin (Hou et al., 2016). In kidney, the liver-derived hormone hepcidin serves as a promising therapeutic target in conditions such as post-cardiopulmonary bypass AKI and delayed graft function after renal transplantation (Scindia et al., 2019). Reportedly, it could promote erastin-induced ferroptosis by degrading ferroportin, the sole iron export protein (Geng et al., 2018). Recently, prominin 2 has been found to facilitate the formation of ferritin-containing multivesicular bodies (MVB) and exosomes in mammary epithelial, thus driving ferroptosis resistance (Belavgeni et al., 2019; Brown et al., 2019). Heat shock protein beta-1 (HSPB1) can inhibit TfR1 recycling (Chen et al., 2006), its up-regulation can confine erastin-induced iron uptake through suppressing the ferroptosis (Sun et al., 2015). In addition to participating in Fenton reaction, iron is also transported to several iron-containing enzymes involved in lipid peroxidation, thus in turn actuating ferroptosis. For instance, phosphorylase kinase G2 (PHKG2) plays a significant role in promoting iron accumulation and regulating iron availability to LOXs (Yang et al., 2016). CDGSH iron-sulfur domain (CISD) has also been shown to bind a redox-active [2Fe-2S] cluster and participates in regulating iron storage and redox reactions (Fidai et al., 2016). Down-regulating CISD1 level can promote iron-mediated intramitochondrial lipid peroxidation contributing to erastin-induced ferroptosis (Yuan et al., 2016). Silencing CISD2 gene can raise lipid ROS production and mitochondrial iron content, making drug-resistant head and neck cancer cells sensitive to sulfasalazine-induced ferroptosis (Kim et al., 2018). Accumulation of iron responsive element binding protein 2 (IREB2) can induce iron aggregation, thus contributing to the ferroptosis (Li Y. et al., 2020), while the silencing of IREB2 gene leads to mutual changes in the expression of known iron absorption, metabolism and storage genes TFR1, iron-sulfur cluster assembly enzyme (ISCU), FTH1, FTL and in erastin sensitivity (Salahudeen et al., 2009; Vashisht et al., 2009).
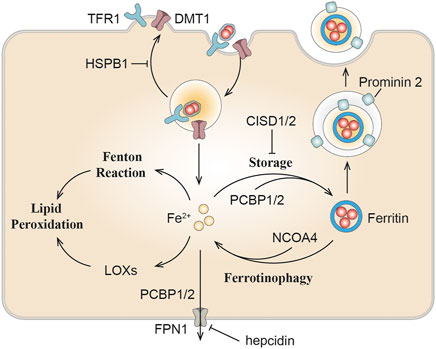
FIGURE 2. The role of iron metabolism in ferroptosis. Iron homeostasis or transportation including can influence ferroptosis sensitivity. DMT1, divalent metal transporter 1; TFR1, transferrin receptor 1; NCOA4, nuclear receptor coactivator 4; PCBP, Poly(rC) binding protein; LOXs, lipoxygenase; HSPB1, heat shock protein beta-1; CISD, CDGSH iron-sulfur domain.
3 Ferroptosis in Chronic Renal Injury and Fibrosis
3.1 Lipid Peroxidation in Chronic Kidney Disease
The final common pathological denominator in the case of chronic kidney injury is renal fibrosis, and the extent of tubulointerstitial fibrosis is the best predictor of renal survival for CKD patients (Zeisberg and Neilson, 2010). Oxidative stress is present from the early stages of CKD and continues to increase as kidney function deteriorate due to antioxidant consumption and increased ROS production (Sureshbabu et al., 2015; Daenen et al., 2019; Duni et al., 2019; Gyuraszova et al., 2019).
Diverse renal abnormalities can trigger oxidative stress and accelerate the kidney disorders. Fibrotic and damaged tubular and glomerular cells in CKD may enhance ROS production, which is a major agent of oxidative stress (Daenen et al., 2019). Compared with healthy control group, the levels of GPX and GSH in renal cortex of CKD model rats and CKD patients were significantly decreased, while the levels of ROS and MDA were significantly increased (Zachara et al., 2004; Tbahriti et al., 2013). Lipid aldehydes, the end product of many oxidation reactions, including 4-hydroxy-2-hexenal (4-HHE) and 4-hydroxy-2-nonenal (4-HNE) (Himmelfarb, 2005), and their plasma concentrations are significantly higher in CKD patients (Soulage et al., 2020). Malondialdehyde (MDA), is the final product of peroxidation of polyfluorinated fatty acids and an indicator of ferroptosis (Kim et al., 2018), which is commonly raised in the serum of CKD patients (Taccone-Gallucci et al., 1989; Pei et al., 2016). These markers, including 4-HHE, 4-HNE, and MDA have all been widely used in the detection of ferroptosis recently. In HEK-293T cells, GPX4 was shown to block TNFα-mediated activation of NF-кB (Li et al., 2018). Moreover, recent researches have reflected that ferroptosis could facilitate renal damage in diabetic nephropathy (DN), and lipid peroxidation is increased in DN mice, and the expressions of ACSL4, SLC7A11, and SLC3A2 are up-regulated, while GPX4 is down-regulated (Wang Y. et al., 2020). ACSL4 inhibitor rosiglitazone alleviated renal impairment in DN by suppressing ferroptosis (Wang Y. et al., 2020). It is established that pathological changes of DN include renal hypertrophy, enlargement of glomerular capillaries, mesangial expansion, and glomerular basement membrane thickening caused by ECM deposition, which ultimately lead to inflammation, endothelial dysfunction, and renal fibrosis (Jha et al., 2016).
3.2 Iron Metabolism in Kidney
3.2.1 Iron Homeostasis in Kidney
Normally, nutritional iron, which consists of both ferric iron (Fe3+) and ferrous iron (Fe2+), is absorbed by duodenal enterocytes. Fe3+ could bind to transferrin (TF) on the cell membrane to form transferrin-bound iron (TBI), which is captured by transferrin receptor 1 (TFR1) into the endosomes. Intracellular Fe3+ is reduced to Fe2+ by iron reductase (represented by Steap3). Fe2+ would then be released into the labile iron pool (LIP) in the cytoplasm by the divalent metal transporter 1 (DMT1) (Dhungana et al., 2004; Knutson, 2007). Endosomal Fe3+ is quickly reduced to Fe2+ by an oxidoreductase activity. Excess iron ions are either released through the basal membrane protein ferroportin 1 (FPN1) to the circulation or stored in ferritin, the major iron storage protein (Arosio et al., 2009). Ferritin is a spherical heteropolymer capable of storing 4,500 iron atoms, consisting of a ferritin heavy chain (FTH1) and a ferritin light chain (FTL), both of which can sequestrate and store iron in a non-toxic and bioavailable form. Iron is incorporated into ferritin via ferritin iron pores as Fe2+, which could be formed by intestinal absorption or erythrocyte degradation. FTH1 catalyzes the oxidation of Fe2+ to Fe3+, while FTL promotes the nucleation of ferrihydrite, enabling inert deposits of Fe3+, which can hardly be used in cells or generate ROS (Philpott, 2018). Poly(rC) binding proteins (PCBPs) (especially PCBP1 and PCBP2), are important iron chaperones to regulate the metalation of iron-containing proteins and the storage and export of Fe2+ (Shi et al., 2008). They directly bind ferritin and deliver labile irons to ferritin for storage (Philpott et al., 2017). For intracellular utilization, iron needs to be released from ferritin and reduced to Fe2+ again. Ferritin degradation mediated by nuclear receptor coactivator 4 (NCOA4) is required for the maintenance of mitochondrial respiratory activity, respiratory chain complex assembly, mitochondrial iron storage, and membrane potential (Figure 3; Fujimaki et al., 2019). However, it has been reported that iron excess in red blood cells in turn triggers the ubiquitin-mediated NCOA4 degradation by the E3-9 ligase HERC2 (Ryu et al., 2018).
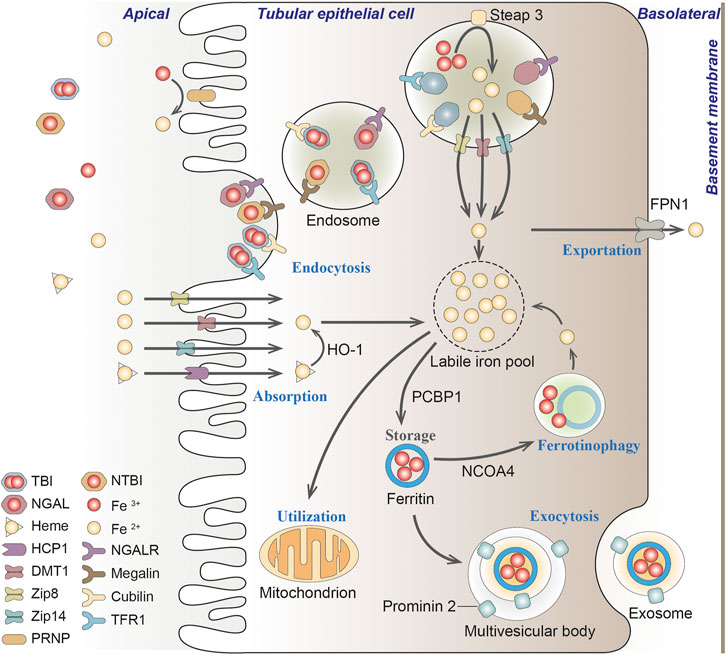
FIGURE 3. Iron transportation in renal tubules. Filtered iron is almost completely reabsorbed in renal tubular, where it would be then stored in ferritin, utilized in the mitochondria or exported from the cell. TBI, transferrin-bound iron; NTBI, non-transferrin-bound iron; NGAL, neutrophil gelatinase-associated lipocalin; HO-1, heme oxygenase-1; PCBP1, Poly(rC) binding protein 1; NCOA4, nuclear receptor coactivator 4; FPN1, protein ferroportin 1; HCP1, heme carrier protein 1; DMT1, divalent metal transporter 1; Zip8, zinc transporter ZIP8; Zip14, zinc transporter ZIP14; PRNP, prion protein; NGALR, neutrophil gelatinase-associated lipocalin receptor; TFR1, transferrin receptor 1.
In the kidney, circulating iron can be filtered through the glomeruli into the tubules under physiological conditions (Zhang et al., 2007; Smith and Thevenod, 2009). The filtered iron is almost completely reabsorbed in the renal tubules, and then stored in ferritin, utilized in the mitochondria or exported from the cell (Smith and Thevenod, 2009). If systemic iron is overloaded and transferrin is mostly saturated, iron would loosely bind to other filterable non-transferrin proteins, such as neutrophil gelatinase-associated lipocalin (NGAL), albumin, hemoglobin, myoglobin and hepcidin, or small molecules such as citrate, acetate, and phosphate (Swinkels et al., 2006; Smith and Thevenod, 2009; Brittenham, 2011; Kroot et al., 2011; Paragas et al., 2012; de Swart et al., 2016; Rosa et al., 2017; Figure 3).
The reabsorption of filtered transferrin-bound iron (TBI), hemoglobin and myoglobin in the renal tubules is mainly through megalin-dependent cubilin-mediated endocytosis via binding to TFR1 expressed in the apical membrane (Kozyraki et al., 2001). Renal TFR1 expression is decreased during parenterally induced iron loading (Weiss et al., 2018), but increased under low iron conditions (Smith et al., 2019). NGAL is a promising biomarker for acute or chronic kidney disease, where iron binds to NGAL and is enucleated by neutrophil gelatinase-associated lipid calin receptor (NGALR) in distal tubules (Langelueddecke et al., 2012). Heme is reabsorbed through the heme importer, namely, heme carrier protein 1 (HCP1), in proximal and distal tubules (Le Blanc et al., 2012). Transporters in different nephron segments that promote the uptake of other non-transferrin proteins binding iron on the plasma membrane has not been fully elucidated (Thévenod and Wolff, 2016). Reportedly, zinc transporter ZIP8 (Zip8), zinc transporter ZIP14 (Zip14) and DMT1 were present in the apical membrane of human proximal and distal tubules (Canonne-Hergaux and Gros, 2002; Veuthey et al., 2014; van Raaij et al., 2018b). The endocytic iron is released from the transport protein, and is then reduced and exported to the cytosol mainly through DMT1, where Fe3+ is excluded under the excitation of an H+ electrochemical potential gradient, and potentially pass through the Zip14 or Zip8 (He et al., 2006; Pinilla-Tenas et al., 2011). Since DMT1 optimally transports ferrous iron at pH 5.0–6.0, whereas Zip8 and Zip14 at pH 6.5–7.5 (He et al., 2006; Pinilla-Tenas et al., 2011), it is speculated that DMT1 may mainly facilitate the uptake of free iron distal tubules, while Zip8 and Zip14 may play a better role in the proximal tubules (Figure 3; van Swelm et al., 2020).
There is no evidence for basolateral uptake of TBI, heme, hemoglobin, or non-transferrin-bound iron (NTBI) in the basolateral renal tubules so far, whereas the iron exporter-ferroportin exists in the basolateral membrane of proximal tubules (van Raaij et al., 2018a). As a master regulator of iron homeostasis, hepcidin regulates this ferroportin-mediated iron export and intracellular H-ferritin levels (Vyoral and Jiri, 2017). In podocytes, hemoglobin can be taken up by megalin-cubilin endocytosis catabolized by heme oxygenase-1 (HO-1), thus resulting in increased oxidative stress (Rubio-Navarro et al., 2018; Duann et al., 2019). In addition, iron transport and regulation also exist in mesangial cells, since ferritin, TFR1, HO-1, and IRP1 are all expressed in these cells (Cheng et al., 2015; Liu and Templeton, 2015).
3.2.2 Iron Overload and Toxicity in Kidney
Iron plays a key role in mitochondria, promoting oxidative stress and ROS production, leading to cell apoptosis, mitochondrial damage and vascular calcification (Nakanishi et al., 2020). In 1988, the deleterious role of iron in renal disease was first described as being involved in free radical mediated renal damage after ischemia and lipid peroxidation, while iron chelators can reduce these injury (Paller and Hedlund, 1988), suggesting the exsistance of ferroptosis. Mitochondria are extremely dependent on iron metabolism and are the target of iron transport in these cells. The tubular epithelium possesses abundance of mitochondria to support active transport, but quite susceptible to hypoxia, toxic compounds, proteinuria, metabolic disorders. Kidneys can be exposed to toxic levels of bound iron (e.g., transferrin), or free iron, due to redundant filtration of iron into renal tubules, alterations in cellular iron localization or compartmentalization in the kidney (Martines et al., 2013; van Raaij et al., 2018b; Figure 4).
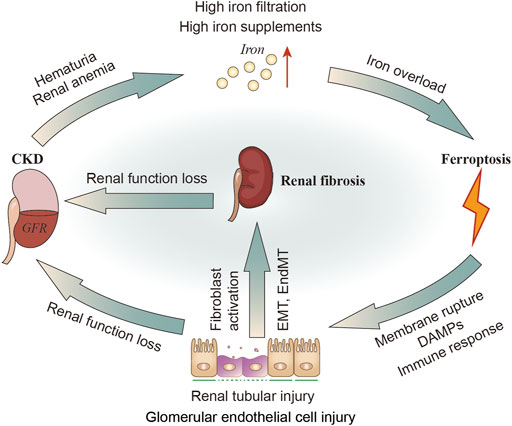
FIGURE 4. The relationship of CKD, iron metabolism, ferroptosis in renal tubular cells and renal fibrosis. In patients with CKD, hematuria and renal anemia could cause increased iron filtration in renal tubules and excessive exogenous intake of iron. Iron overload may induce ferroptosis, leading to renal injury and DAMPs signals release as well as inflammatory and immune responses. The injured tubular can then activate fibroblasts or directly transform into myofibroblasts through EMT. Kidney injury or renal fibrosis can further reduce the GFR. DAMPs, damage-related molecular patterns; GFR; glomerular filtration rate.
It was found that kidney and urinary iron content are elevated in patients with CKD (Sears et al., 1966; Wang et al., 2001; Shah et al., 2007; van Raaij et al., 2018b) and animal models of CKD (Nankivell et al., 1994; Ueda et al., 1996; Harris and Tay, 1997; Naito et al., 2015). Altered molecular iron handling may lead to iron deposition in kidney (van Raaij et al., 2018b). When these iron or iron-containing molecules exceed the storage capacity of ferritin or the cell’s ability to export iron, they damage renal tubular cells (Sponsel et al., 1996; Zager and Burkhart, 1997; Martines et al., 2013; Naito et al., 2015). Exposure to either the Fe2+ or Fe3+ (100 μM, 30–80 h) may impair healing of denuded areas made within confluent monolayers of renal tubular epithelial cell lines (Sponsel et al., 1996). Fe2+ (FeSO4 100 μM) can stimulate H2O2 production in renal tubular cells in vitro (Zager and Burkhart, 1997). Fe2+ can also cause cytopathic changes including numerous iron-particles-containing lysosomes and distortional mitochondria (Sponsel et al., 1996; Figure 4). Apart from the tubules, cultured human glomerular endothelial cells (HGECs) have been shown to express TFR1, FPN1 and DMT1. The endothelial injury factor-Ang II could induce iron incorporation followed by an increase in labile iron in HGECs (Tajima et al., 2010). Iron chelators have been confirmed to improve renal function in patients with glomerular disease, and minimize the reactivity of iron and remove iron from the kidneys or relieving iron-related oxidative stress (Rajapurkar et al., 2013). Iron restriction can also delay the progress of DN in diabetic rats (Matsumoto et al., 2013). In addition, administration of iron chelation can alleviate renal interstitial fibrosis and suppress the increments of collagen type III and TGF-β (Naito et al., 2015).
Iron-mediated cell injury or ferroptosis is considered as a core of the pathogenesis of acute kidney injury (Scindia et al., 2019). Iron homeostasis is becoming the focus of new therapeutic interventions for kidney diseases. Although iron overload or toxicity are not equivalent to ferroptosis, there is reason to believe that these “iron induced oxidative stress or lipid peroxidation” are at least related to ferroptotic events.
3.3 Ferroptosis in Renal Fibrosis
ROS play a wide role in the oxidation of lipids, proteins, and DNA, resulting in damage to the tubules and vascular endothelium, thus secreting profibrotic factors (Pisani et al., 2013; Daenen et al., 2019). Inhibition of oxidative stress has always been an anti-fibrotic target (Wang et al., 2019). Oxidative stress can cause fibroblast/pericyte activation (Bondi et al., 2010), tubular epithelial-mesenchymal transition (EMT) (He et al., 2015; Song et al., 2018), fibrocyte/macrophage recruitment (Wagner et al., 2012; Lee et al., 2016), and endothelial-mesenchymal transition (EndMT) (Echeverria et al., 2013; Montorfano et al., 2014). Reportedly, HK2 (human kidney tubular cell) has been reported to secrete profibrotic factors and facilitate fibroblast proliferation and activation during ferroptosis (Zhang et al., 2021). Oxidative stress induced glomerular endothelial cell dysfunction (Sol et al., 2020), mesangial sclerosis (Lu et al., 2018), podocyte abnormality (Okamura and Pennathur, 2015; Zschiedrich et al., 2017), and parietal epithelial cell injury (Odaci et al., 2015; Su et al., 2015) can lead to glomerulosclerosis (Tang et al., 2011; Su et al., 2019). Increased production of ROS (including OH−, H2O2, lipid peroxides, and superoxide anion free radicals) in podocytes can directly damage podocytes themselves, which consequently meditate glomerular injury (Gwinner et al., 1997; Marshall et al., 2006). Numerous pathologic processes mediated by oxidative stress associated factors can stimulate glomerular endothelial cells to undergo EndMT, which is consistent with increased mesenchymal marker expression including α-smooth muscle actin (αSMA) and fibroblast specific protein 1, and the production of ECM proteins (Liang et al., 2016; Dejana et al., 2017; Figure 4).
Although oxidative stress is known as an inducer for ferroptosis, we have to notice it is the “lipid ROS” and subsequently membrane rupture that represent the occurrence of ferroptosis, instead of the “ROS” (Dixon et al., 2012). Ferroptosis triggers inflammatory and immune responses through the release and activation of damage-related molecular patterns (DAMPs) signals, which makes it to be an attractive therapeutic target for renal fibrosis especially renal graft fibrosis (Linkermann et al., 2013; Martin-Sanchez et al., 2017). Synchronous tubule necrosis can be induced in renal ischemia/reperfusion (I/R) injury model, oxalate crystal-induced AKI model and diabetic nephropathy (DN) tubule cell death model (Linkermann et al., 2014; Wang Y. et al., 2020; Li S. et al., 2021). Erastin or TGF-β can induce ferroptosis, promote fibroblast-to-myofibroblast differentiation resulting in fibrosis (Gong et al., 2019). Recent studies have shown that ureteral obstruction can induce ferroptosis in renal tubular epithelial cells in vivo, and HK-2 cells (human proximal tubular cell line) to secrete various profibrotic factors which further promoted the proliferation and activation of fibroblast during ferroptosis (Zhang et al., 2021). Here, we first briefly summarize the role of several key regulators of ferroptosis in renal fibrosis, though the specific mechanism of these prooxidative factor in triggering ferroptosis and mediating renal fibrosis has far been elucidated.
3.3.1 HO-1
HO-1, an inducible enzyme, catabolizes the heme group into carbon monoxide (CO) and biliverdin, which can further convert to bilirubin, and ferrous iron. HO-1 induction could protect the kidney from oxidative stress showing anti-inflammatory and anti-apoptotic activity in DN (Abo El Gheit and Emam, 2016), which was also proved to reduce glomerular sclerosis and intimal hyperplasia, being a therapeutic target to improve the long-term outcomes after kidney transplantation (Bedard et al., 2005; Corona et al., 2020). Additionally, compared with wild-type mice, HO-1−/− mice had higher levels of renal fibrosis (Kovtunovych et al., 2010), and more susceptible to myocardial I/R (Abo El Gheit and Emam, 2016). Absence of HO-1 was associated with immune response deficiency, such as Th1-weighted shift in cytokine responses (Kapturczak et al., 2004), and defects in erythrophagocytosing macrophages (Kovtunovych et al., 2010), thus supporting it a protective role in kidney diseases. However, activation of HO-1 triggers ferroptosis through iron overloading and excessive lipid peroxides, thus promoting renal fibrosis area and collagen I in DN mice (Chang et al., 2018; Feng et al., 2021). Increased HIF-1α/HO-1 level, iron content, ferritin level and lipid peroxides were detected in this DN kidneys, indicating that ferroptosis might enhance DN and damage renal tubules through HIF-1α/HO-1 pathway (Feng et al., 2021). Researches on neurodegenerative diseases and neoplastic disorders also demonstrated HO-1 has pro-ferroptotic effects on cells through iron accumulation and pro-oxidant effects (Chang et al., 2018; Li R. et al., 2020; Fernández-Mendívil et al., 2021).
Resultingly, though induction of HO-1 has been a potential therapeutic target in kidney diseases, attention must be paid to the safe range of HO-1 induction, owing to the pro-ferroptotic role of HO-1 in raising intracellular ferrous load and redox homeostasis (Chang et al., 2018; Li R. et al., 2020; Fernández-Mendívil et al., 2021).
3.3.2 NOX4
NADPH oxidase (NOXs) plays the most important role in many enzyme systems involved in ROS production in the kidney (Jha et al., 2016). Nox4 is a major source of ROS in the renal cells, linking with myofibroblast differentiation and tubulointerstitial fibrosis through various pathways. Although Nox4 is expressed in a variety of kidney cells, including epithelial, endothelial, podocytes, mesangial cells, and fibroblasts, its role in fibrosis is controversial (Yang et al., 2018). Inhibition of NOX4 could protect from ferroptosis in various cells via suppressing H2O2 and lipid peroxides production (Dixon et al., 2012; Wang et al., 2018; Chen et al., 2019). Manipulating Nox4 expression in mice proximal tubules cells could upregulate renal fibronectin and TGF-β level (Sedeek et al., 2010). It is confirmed that rapamycin can decrease MDA and NOX4 levels, inhibit the NADPH oxidase activity, and elevate the GSH level and SOD activity, thus inhibiting the ROS production of mesangial cells in DN (Lu et al., 2018). Nrf2 is known to regulate the antioxidant response and the activity of several ferroptosis-related proteins, involving iron homeostasis, intermediate metabolism, and GSH metabolism; Nrf2-mediated up-regulation of HO-1, as described above, could facilitate heme degradation, free iron release, and lipid peroxidation, thus inducing ferroptosis (Dodson et al., 2019; Fang et al., 2019). The up-regulation of Nrf2 expression by Fenofibrate can inhibit iron droop, thus alleviating renal pathological changes in DN mice (Li S. et al., 2021). HIF-1α could negatively regulate ferroptosis induction in human cancer cells (Yang M. et al., 2019), which is also mechanically antithetical to oxidative stress mentioned above (Nlandu Khodo et al., 2012). This is contrary to the traditional view that NOXs promotes ferroptosis by oxidative stress-induced lipid peroxidation (Xie et al., 2016a; Wang J. et al., 2020; Park et al., 2021). Different subcellular localization, cell types, molecular concentrations, disease types or/and stages may be responsible for this functional variability, and more research is warranted on cell-specific and inducible overexpression or knockout systems in vivo.
3.3.3 TP53
TP53 (p53) is considered as a tumor suppressor due to its ability to promote cell death or permanently inhibit cell proliferation. P53 can negative regulate SLC7A11, leading to reduced glutathione production and increased ROS (Jiang et al., 2015), target SAT1 which increase the level of ALOX15 (Ou et al., 2016), suggesting that p53 represents a novel inducer of ferroptosis. However, p53 can also regulate GSL2 (glutaminase 2) to influence glutamine metabolism and block dipeptidyl-peptidase-4 (DPP4) activity, which finally defense lipid peroxidation and ferroptosis (Hu et al., 2010; Xie et al., 2017). Reportedly, CRISPR/Cas9 mediated p53 deletion cells were sensitive to ferroptosis, implying that p53 hold a pro-survival function in ferroptosis, which requires p53 targeting CDKN1A (P21) expression (Tarangelo et al., 2018). These context-dependent roles of p53 in ferroptosis may result from fine-tuning its phosphorylation, ubiquitination, methylation, acetylation, and other modifications.
P53 also plays a critical role in non-neoplastic diseases including renal fibrosis and CKD, which acts as a critical co-factor for several key fibrotic and cell cycle effectors including TGF-β1, CTGF, PAI-1, P21 (Kortlever et al., 2006; Yang et al., 2010; Samarakoon et al., 2012). P53 inhibitor pifithrin-α was proved to alleviate renal experimental fibrogenesis consistent with decreased CTGF and TGF-β1 levels (Yang et al., 2010). Interestingly, the p53-dependent P21, shows both effect in ferroptosis and renal fibrosis (Tarangelo et al., 2018; Das et al., 2020), but whether a direct link exists between ferroptosis, renal fibrosis, and P21 has not been reported.
4 Ferroptosis Regulators and Renal Fibrosis
4.1 Ferroptosis Inhibitors
Many drugs or compounds have been reported to inhibit renal fibrosis in vitro (Table 1) or in vivo (Table 2) and have also been proved to inhibit ferroptosis (Table 3).
4.1.1 Antioxidants
Several antioxidants can suppress ferroptosis through limiting lipid peroxidation and have been verified to alleviate renal fibrosis from different causes in vivo and/or in vitro through different targets and mechanisms, including Vitamin E, CoQ10, N-acetylcysteine (NAC), Trolox, Diphenylene iodonium, and Zileuton (Jenkins et al., 2001; Ho et al., 2007; Piao et al., 2013; Shen et al., 2016; Atmaca et al., 2017; Giam et al., 2017; Gonzalez et al., 2017; Helmy et al., 2018; Zhao et al., 2018; Li C. et al., 2019; Montford et al., 2019; Farias et al., 2020).
4.1.2 Iron Chelators
Reportedly, deferoxamine prevents renal interstitial fibrosis in the UUO mice (Ikeda et al., 2014). Long-term oral administration of deferiprone have been shown to have renal protective effects in both diabetic and non-diabetic glomerular disease (Rajapurkar et al., 2013). These two iron chelating agents have anti-iron toxicity at 100 μM (Do Van et al., 2016).
4.1.3 CYP450 Substrate
Certain cytochrome P450 substrate drugs have the anti-ferroptotic effect by scavenging activity against lipid peroxyl radicals in various cell lines including NRK49F (rat kidney fibroblast), NRK52E (rat kidney tubular cell), and HK2 (Mishima et al., 2020). These drugs inhibit the ferroptotic pathological changes of different renal cell types and ameliorates renal damage, indicating the therapeutic promise of this repurposed drugs (e.g., Triiodothyronine, and Carvedilol) (Wong et al., 2001; Jovanovic et al., 2005; Lu et al., 2013; Adedoyin et al., 2018; El Agaty, 2018).
4.1.4 Thiazolidinediones
The PPAR-γ agonists thiazolidinediones (TZDs), including rosiglitazone, troglitazone, and pioglitazone, could prevent ferroptosis probably by inhibiting ACSL4, even in PPARγ KO cells (Doll et al., 2017; Kagan et al., 2017; Wang Y. et al., 2020). However, non-thiazolidinediones PPARγ agonists failed to rescue cells from RSL3-induced ferroptosis, suggesting that thiazolidinediones might exert anti-ferroptosis effect regardless of PPARγ (Doll et al., 2017). Reportedly, these drugs can alleviate the glomerulosclerosis and tubulointerstitial fibrosis of various renal disease models (McCarthy et al., 2000; Chung et al., 2005; Panchapakesan et al., 2005; Omasu et al., 2007; Kawai et al., 2009; Han et al., 2010; Bilan et al., 2011; Koch et al., 2012; Shui et al., 2017; Sun et al., 2017; Németh et al., 2019; Sun et al., 2019; Wei et al., 2020), and ameliorate chronic renal allograft dysfunction (Kiss et al., 2010; Deng et al., 2019).
4.1.5 DPP4 Inhibitors
In the absence of TP53, ferroptosis can be inhibited by downregulation of DPP4 or administration of DPP4 inhibitors (e.g., alogliptin, linagliptin, and vildagliptin) (Xie et al., 2017). Additionally, DPP4 represents a precise druggable target in CKD. These DPP4 inhibitors were proved to exhibit renoprotective effects and ameliorate the glomerulosclerosis and tubulointerstitial fibrosis in different diseases (Kanasaki et al., 2014; Vavrinec et al., 2014; Tanaka et al., 2016; Tsuprykov et al., 2016; Uchida et al., 2017; Oraby et al., 2019; Pengrattanachot et al., 2020; Tang et al., 2021).
4.1.6 Others
Recent studies have demonstrated that liproxstatin-1 attenuates renal fibrosis induced by UUO through inhibiting ferroptosis in renal tubular epithelial cells (Zhang et al., 2021). Ferrostatin-1 is a widely used ferroptosis blocker, which exhibits renoprotective effects in obesity-induced renal fibrosis, inflammatory cell infiltration by inhibiting ferroptosis in high-fat diet mice (Li et al., 2022). It might reverse pathological parameters and improve renal function of folic acid-induced AKI mice, which is a model for studying nephrotoxic tubule damage and gradual progression of renal fibrosis (Li X. et al., 2020). Puerarin has been demonstrated to protect against heart failure via mitigating ferroptosis (Liu et al., 2018), which also ameliorates renal fibrosis by reducing oxidative stress induced-epithelial cell apoptosis via MAPK signal pathways (Zhou et al., 2017). Baicalein restrains ferroptosis by abrogating ALOX-mediated lipid peroxidation (Xie et al., 2016b; Yang et al., 2016), and can inhibit renal fibrosis in a variety of ways (Wang W. et al., 2015; Wang et al., 2016; Hu et al., 2017). U0126, the ERK inhibitor, was proved to protect against cell death induced by erastin in MEFs (Gao et al., 2015). It has also been reported to ameliorate renal fibrosis in different renal proximal tubular epithelial cells (Chen et al., 2014; Weng et al., 2020). FG-4592 pretreatment plays a protective role at the early stage of folic acid-induced renal fibrosis and other kidney injury through alleviating ferroptosis (Li X. et al., 2020) However, in the UUO mice model, even high dose treatment of FG-4592 has little effect on renal fibrosis (Kabei et al., 2020).
4.2 Ferroptosis Inducers
Interventions preventing cell death could initially prevent parenchymal cell loss thus protecting the kidney early in the course of kidney damage, but they may also facilitate fibrosis by blocking the downregulation of fibroblast numbers later in the course of the renal disease. EMT, charactered by loss of E-cadherin, can facilitate ferroptosis, and upregulate transcription factors, including SNAI1, ZEB1, and, TWIST1, inducing EMT-mediated tumor metastasis, elevates ferroptosis sensitivity in turn (Wu et al., 2019), while E-cadherin-mediated cell-cell contacts has been demonstrated to protect against ferroptosis (Yang W.-H. et al., 2019; Wenz et al., 2019; Wu et al., 2019). Furthermore, human cancer cell lines and organoids with a highly mesenchymal-like cell state are selectively vulnerable to ferroptosis (Viswanathan et al., 2017). It is speculated that EMT might confer susceptibility to ferroptosis-based therapies in cancers (Chen et al., 2021). Analogizing to cancer cells, it is possible that induction of ferroptosis could also attenuate renal fibrosis by selectively killing mesenchymal-like cells transited from epithelium and endothelium.
Interestingly, some ferroptosis inducers (e.g., Acetaminophen, artemisinin and its derivatives, sorafenib, sulfasalazine, and withaferin A) have also been demonstrated to alleviate renal fibrosis in various models, suggesting a complex relationship between ferroptosis and renal fibrosis (Table 4). Reportedly, sorafenib, an xCT inhibitor, can inhibit the TGF-β/Smad3-induced EMT signaling (Jia et al., 2015) and suppress the CXCR3/CXCL11-mediated macrophage infiltration (Ma et al., 2016), ameliorating renal fibrosis. Besides, an xCT inhibitor-sulfasalazine also play a protective role against renal fibrosis in the UUO rats, with decreased level of TGF-β1, ROS, and lipid peroxides (Demirbilek et al., 2007). Acetaminophen, a resistance indicator of fibrosis, and a reduction of tubular EMT in glomerular sclerosis may prevent renal oxidative stress in an obese Zucker mouse model (Wang et al., 2013; Lőrincz et al., 2015), and exerts renoprotective effects (Wang C. et al., 2015). In different models, artemisinin and its derivatives have been reported to induce ferroptosis by triggering ROS, promoting ferritin degradation, and regulating xCT/GPX4 axis (Louandre et al., 2013; Louandre et al., 2015; Sun et al., 2016), thus alleviating renal fibrosis (Cao et al., 2016; Zhang et al., 2017; Wen et al., 2019; Zhang et al., 2019; Liu et al., 2020). Withaferin A can down-regulate pro-fibrotic factors, inflammatory signaling molecules, and ER stress-related molecules and improve kidney function by decreasing uric acid (Chen et al., 2020; Zhao et al., 2021), it could also induce ferroptosis via the NRF2 pathway by targeting KEAP1 or inactivating GPX4 (Hassannia et al., 2018).
Besides, erastin and sorafenib could induce ferroptosis in hepatic stellate cells and are potential drugs for the treatment of hepatic fibrosis (Zhang et al., 2018). Neratinib, a demonstrated ferroptosis inducer in breast cancer (Nagpal et al., 2019), has also been shown to inhibit liver fibrosis (Park et al., 2020). Fluvastatin suppressed interstitial renal fibrosis and oxidative stress in UUO mice model (Moriyama et al., 2001) and NRK-49F cells (Ikeuchi et al., 2004). Overall, these highlight the possibility of inducing ferroptosis to achieve remission of renal fibrosis.
5 Other Programmed Cell Death and Ferroptosis in Renal Fibrosis
It is considered that ferroptosis interacts extensively with other modes of cell death, and even distinct from other types of programmed cell death (PCD). Multiple types of PCD have been discovered and well-studied, including apoptosis, necroptosis, pyroptosis and ferroptosis, which we would like to briefly overview their relationship with renal fibrosis.
Apoptosis is regarded as a mechanism of self-protection that eliminates dead or damaged cells that form during the physiological process of aging, and is the best-known form of PCD which was first described in 1972 (Argüelles et al., 2019). Inhibition of apoptosis has been shown to reduce kidney fibrosis (Tanaka et al., 2003; Portilla, 2014), and a number of molecules or drugs have been reported to protect the kidneys by inhibiting apoptosis (Tanaka et al., 2005).
Necroptosis, a mode of cell death dependent on the activity of RIPK1 and RIPK3 kinases, which is characterized by permeable and rupture of cell membranes can also trigger necroinflammation and release of DAMPs (Belavgeni et al., 2020). Inhibiting necroptosis was demonstrated to attenuate different type of renal fibrosis (Zhu et al., 2015; Zhu et al., 2016; Xiao et al., 2017; Dai et al., 2020; Huang et al., 2020).
Pyroptosis is caused by the cleavage of Gasdermins by various caspases, and is subsequentially executed by the insertion of the N-terminal fragment of cleaved Gasdermins into the plasma membrane, facilitate plasma membrane rupture and the release of pro-inflammatory products, which has been considered as a key fibrotic mechanism in the kidney pathology development (Kesavardhana et al., 2020; Cuevas and Pelegrín, 2021). It is a form of lytic programmed cell death initiated by inflammasomes, and HMGB1 released from pyroptotic renal tubular cells amplified inflammatory responses, which recruits immune cells to promote tissue healing and contributes to renal fibrogenesis (Raupach et al., 2006; Li Y. et al., 2021). GSDME-mediated pyroptosis is responsible for renal tubule injury and subsequentially fibrosis induced by ureteral obstruction, 5/6 nephrectomy, and DN (Li W. et al., 2021; Li Y. et al., 2021; Wu et al., 2021). For more details, interested readers may refer to these excellent recently published reviews.
In general, apoptosis is a non-lytic form of cell death and is described as an active programmed cell division process to avoid triggering inflammation to remain immunologically silent, which may confine the contribution of subsequently renal fibrosis (Fink and Cookson, 2005). However, ferroptosis, pyroptosis, and necroptosis are all considered as lytic cell death, which can contribute to the release of several immune-stimulatory DAMPs, that trigger immune responses (Tang et al., 2020). Different lytic cell deaths serve distinct purposes, determine different profiles of the inflammatory infiltrate and thus determine the association between cell death and long-term renal fibrosis (Belavgeni et al., 2020).
The critical role of necroptotic pathway lies in the host response to pathogen infection, which is triggered when apoptosis is hindered (Upton et al., 2010; Brault and Oberst, 2017). Pyroptosis is a primary cellular response following the sensing of pathogens as well as danger signals, which mainly includes DAMPs, pathogen-associated molecular patterns (PAMPs). The most significant initiator of pyroptosis is NLRP3 inflammasome which can be activated by various stimuli (Jo et al., 2016). However, as mentioned above, a key feature of ferroptosis is intracellular overload iron and catalysis of lipid peroxidation, which are both common and worsen in CKD with disease progression (Naito et al., 2015). In addition, proactive high-dose iron supplementation due to renal anemia also led to renal iron toxicity (Tanaka and Tanaka, 2015; van Raaij et al., 2018b). Thus, diverse chronic kidney disease creates an appropriate condition for the occurrence of ferroptosis. Although these different types of PCD have multiple functions in various physiological states and in a host’s response to external damage, there is clear evidence of crosstalk or interactions (Wang and Kanneganti, 2021).
6 Conclusion and Perspective
We have witnessed an outbreak of ferroptosis research in biomedicine over the past decades. Generally, it is a form of iron-dependent, lipid peroxidation-mediated regulated necrosis, which is strictly under controlled. This type of cell death may play a fairly important role in a wider range of physiological and pathophysiological processes (Qiu et al., 2020). On the other hand, a variety of studies on ferroptosis have detected only a few non-specific indicators, such as glutathione (GSH) levels and GPX4 levels, to demonstrate the presence of ferroptosis, which may possibly exaggerate the role of ferroptosis to some extent due to the variety of oxidative stress.
Despite a large number of recent studies have implicated hat ferroptosis is related to renal fibrosis, its complex and finely concrete mechanism is still far from being resolved. More research is warrant to elucidate whether and how pharmaceuticals or chemicals mentioned in this article affect renal fibrosis by regulating ferroptosis.
Author Contributions
RYT, MG, YHM and YZ developed the theoretical formalism. ZJW, JJZ, HZ and CJS contributed to the final version of the manuscript.
Funding
This study was supported by the National Natural Science Foundation of China (Grant no. 82070769).
Conflict of Interest
The authors declare that the research was conducted in the absence of any commercial or financial relationships that could be construed as a potential conflict of interest.
Publisher’s Note
All claims expressed in this article are solely those of the authors and do not necessarily represent those of their affiliated organizations, or those of the publisher, the editors and the reviewers. Any product that may be evaluated in this article, or claim that may be made by its manufacturer, is not guaranteed or endorsed by the publisher.
References
Abo El Gheit, R., and Emam, M. (2016). Targeting Heme Oxygenase-1 in Early Diabetic Nephropathy in Streptozotocin-Induced Diabetic Rats. Acta Physiol. Hung 103 (4), 413–427. doi:10.1556/2060.103.2016.4.001
Adedoyin, O., Boddu, R., Traylor, A., Lever, J. M., Bolisetty, S., George, J. F., et al. (2018). Heme Oxygenase-1 Mitigates Ferroptosis in Renal Proximal Tubule Cells. Am. J. Physiology-Renal Physiol. 314 (5), F702–F714. doi:10.1152/ajprenal.00044.2017
Altman, B. J., Stine, Z. E., and Dang, C. V. (2016). From Krebs to Clinic: Glutamine Metabolism to Cancer Therapy. Nat. Rev. Cancer. 16 (10), 619–634. doi:10.1038/nrc.2016.71
Argüelles, S., Guerrero‐Castilla, A., Cano, M., Muñoz, M. F., and Ayala, A. (2019). Advantages and Disadvantages of Apoptosis in the Aging Process. Ann. N.Y. Acad. Sci. 1443 (1), 20–33. doi:10.1111/nyas.14020
Arosio, P., Ingrassia, R., and Cavadini, P. (2009). Ferritins: a Family of Molecules for Iron Storage, Antioxidation and More. Biochim. Biophys. Acta (Bba) - Gen. Subjects 1790 (7), 589–599. doi:10.1016/j.bbagen.2008.09.004
Atmaca, M., Gulhan, B., Korkmaz, E., Inozu, M., Soylemezoglu, O., Candan, C., et al. (2017). Follow-up Results of Patients with ADCK4 Mutations and the Efficacy of CoQ10 Treatment. Pediatr. Nephrol. 32 (8), 1369–1375. doi:10.1007/s00467-017-3634-3
Bannai, S., Tsukeda, H., and Okumura, H. (1977). Effect of Antioxidants on Cultured Human Diploid Fibroblasts Exposed to Cystine-Free Medium. Biochem. Biophysical Res. Commun. 74 (4), 1582–1588. doi:10.1016/0006-291x(77)90623-4
Battin, E. E., and Brumaghim, J. L. (2009). Antioxidant Activity of Sulfur and Selenium: a Review of Reactive Oxygen Species Scavenging, Glutathione Peroxidase, and Metal-Binding Antioxidant Mechanisms. Cell Biochem Biophys. 55 (1), 1–23. doi:10.1007/s12013-009-9054-7
Bedard, E. L. R., Jiang, J., Parry, N., Wang, H., Liu, W., Garcia, B., et al. (2005). Peritransplant Treatment with Cobalt Protoporphyrin Attenuates Chronic Renal Allograft Rejection. Transpl. Int 18 (3), 341–349. doi:10.1111/j.1432-2277.2004.00062.x
Belavgeni, A., Bornstein, S. R., and Linkermann, A. (2019). Prominin-2 Suppresses Ferroptosis Sensitivity. Developmental Cell 51 (5), 548–549. doi:10.1016/j.devcel.2019.11.004
Belavgeni, A., Meyer, C., Stumpf, J., Hugo, C., and Linkermann, A. (2020). Ferroptosis and Necroptosis in the Kidney. Cell Chem. Biol. 27 (4), 448–462. doi:10.1016/j.chembiol.2020.03.016
Bersuker, K., Hendricks, J. M., Li, Z., Magtanong, L., Ford, B., Tang, P. H., et al. (2019). The CoQ Oxidoreductase FSP1 Acts Parallel to GPX4 to Inhibit Ferroptosis. Nature 575 (7784), 688–692. doi:10.1038/s41586-019-1705-2
Bieri, J. G. (1959). An Effect of Selenium and Cystine on Lipide Peroxidation in Tissues Deficient in Vitamin E. Nature 184 (Suppl. 15), 1148–1149. doi:10.1038/1841148a0
Bilan, V. P., Salah, E. M., Bastacky, S., Jones, H. B., Mayers, R. M., Zinker, B., et al. (2011). Diabetic Nephropathy and Long-Term Treatment Effects of Rosiglitazone and Enalapril in Obese ZSF1 Rats. J. Endocrinol. 210 (3), 293–308. doi:10.1530/JOE-11-0122
Bondi, C. D., Manickam, N., Lee, D. Y., Block, K., Gorin, Y., Abboud, H. E., et al. (2010). NAD(P)H Oxidase Mediates TGF-Beta1-Induced Activation of Kidney Myofibroblasts. J. Am. Soc. Nephrol. 21 (1), 93–102. doi:10.1681/ASN.2009020146
Brault, M., and Oberst, A. (2017). Controlled Detonation: Evolution of Necroptosis in Pathogen Defense. Immunol. Cell Biol. 95 (2), 131–136. doi:10.1038/icb.2016.117
Brittenham, G. M. (2011). Iron-Chelating Therapy for Transfusional Iron Overload. N. Engl. J. Med. 364 (2), 146–156. doi:10.1056/NEJMct1004810
Brown, C. W., Amante, J. J., Chhoy, P., Elaimy, A. L., Liu, H., Zhu, L. J., et al. (2019). Prominin2 Drives Ferroptosis Resistance by Stimulating Iron Export. Developmental Cell 51 (5), 575–586. doi:10.1016/j.devcel.2019.10.007
Canonne-Hergaux, F., and Gros, P. (2002). Expression of the Iron Transporter DMT1 in Kidney from Normal and Anemic Mk Mice. Kidney Int. 62 (1), 147–156. doi:10.1046/j.1523-1755.2002.00405.x
Cao, J., Wang, W., Li, Y., Xia, J., Peng, Y., Zhang, Y., et al. (2016). Artesunate Attenuates Unilateral Ureteral Obstruction-Induced Renal Fibrosis by Regulating the Expressions of Bone Morphogenetic Protein-7 and Uterine Sensitization-Associated Gene-1 in Rats. Int. Urol. Nephrol. 48 (4), 619–629. doi:10.1007/s11255-016-1232-0
Cao, J. Y., and Dixon, S. J. (2016). Mechanisms of Ferroptosis. Cell. Mol. Life Sci. 73 (11-12), 2195–2209. doi:10.1007/s00018-016-2194-1
Chang, L., Chiang, S.-K., Chen, S.-E., Yu, Y.-L., Chou, R.-H., and Chang, W.-C. (2018). Heme Oxygenase-1 Mediates BAY 11-7085 Induced Ferroptosis. Cancer Lett. 416, 124–137. doi:10.1016/j.canlet.2017.12.025
Chen, C.-M., Chung, Y.-P., Liu, C.-H., Huang, K.-T., Guan, S.-S., Chiang, C.-K., et al. (2020). Withaferin A Protects against Endoplasmic Reticulum Stress-Associated Apoptosis, Inflammation, and Fibrosis in the Kidney of a Mouse Model of Unilateral Ureteral Obstruction. Phytomedicine 79, 153352. doi:10.1016/j.phymed.2020.153352
Chen, H., Zheng, C., Zhang, Y., Chang, Y.-Z., Qian, Z.-M., and Shen, X. (2006). Heat Shock Protein 27 Downregulates the Transferrin Receptor 1-mediated Iron Uptake. Int. J. Biochem. Cell Biol. 38 (8), 1402–1416. doi:10.1016/j.biocel.2006.02.006
Chen, W.-C., Lin, H.-H., and Tang, M.-J. (2014). Regulation of Proximal Tubular Cell Differentiation and Proliferation in Primary Culture by Matrix Stiffness and ECM Components. Am. J. Physiology-Renal Physiol. 307 (6), F695–F707. doi:10.1152/ajprenal.00684.2013
Chen, X., Kang, R., Kroemer, G., and Tang, D. (2021). Broadening Horizons: the Role of Ferroptosis in Cancer. Nat. Rev. Clin. Oncol. 18, 280–296. doi:10.1038/s41571-020-00462-0
Chen, X., Xu, S., Zhao, C., and Liu, B. (2019). Role of TLR4/NADPH Oxidase 4 Pathway in Promoting Cell Death Through Autophagy and Ferroptosis During Heart Failure. Biochem. Biophysical Res. Commun. 516 (1), 37–43. doi:10.1016/j.bbrc.2019.06.015
Cheng, H.-T., Yen, C.-J., Chang, C.-C., Huang, K.-T., Chen, K.-H., Zhang, R.-Y., et al. (2015). Ferritin Heavy Chain Mediates the Protective Effect of Heme Oxygenase-1 Against Oxidative Stress. Biochim. Biophys. Acta (Bba) - Gen. Subjects. 1850 (12), 2506–2517. doi:10.1016/j.bbagen.2015.09.018
Chung, B. H., Li, C., Sun, B. K., Lim, S. W., Ahn, K. O., Yang, J. H., et al. (2005). Rosiglitazone Protects against Cyclosporine-Induced Pancreatic and Renal Injury in Rats. Am. J. Transpl. 5 (8), 1856–1867. doi:10.1111/j.1600-6143.2005.00979.x
Corona, D., Ekser, B., Gioco, R., Caruso, M., Schipa, C., Veroux, P., et al. (2020). Heme-Oxygenase and Kidney Transplantation: A Potential for Target Therapy? Biomolecules 10 (6), 840. doi:10.3390/biom10060840
Cuevas, S., and Pelegrín, P. (2021). Pyroptosis and Redox Balance in Kidney Diseases. Antioxid. Redox Signaling 35 (1), 40–60. doi:10.1089/ars.2020.8243
Daenen, K., Andries, A., Mekahli, D., Van Schepdael, A., Jouret, F., and Bammens, B. (2019). Oxidative Stress in Chronic Kidney Disease. Pediatr. Nephrol. 34 (6), 975–991. doi:10.1007/s00467-018-4005-4
Dai, Q., Zhang, Y., Liao, X., Jiang, Y., Lv, X., Yuan, X., et al. (2020). Fluorofenidone Alleviates Renal Fibrosis by Inhibiting Necroptosis through RIPK3/MLKL Pathway. Front. Pharmacol. 11, 534775. doi:10.3389/fphar.2020.534775
Das, S., Neelamegam, K., Peters, W. N., Periyasamy, R., and Pandey, K. N. (2020). Depletion of cyclic‐GMP Levels and Inhibition of cGMP‐dependent Protein Kinase Activate P21 Cip1/p27 Kip1 Pathways and lead to Renal Fibrosis and Dysfunction. FASEB j. 34 (9), 11925–11943. doi:10.1096/fj.202000754R
de Carvalho, C., and Caramujo, M. (2018). The Various Roles of Fatty Acids. Molecules 23 (10), 2583. doi:10.3390/molecules23102583
de Swart, L., Hendriks, J. C. M., van der Vorm, L. N., Cabantchik, Z. I., Evans, P. J., Hod, E. A., et al. (2016). Second International Round Robin for the Quantification of Serum Non-transferrin-bound Iron and Labile Plasma Iron in Patients with Iron-Overload Disorders. Haematologica 101 (1), 38–45. doi:10.3324/haematol.2015.133983
Dejana, E., Hirschi, K. K., and Simons, M. (2017). The Molecular Basis of Endothelial Cell Plasticity. Nat. Commun. 8, 14361. doi:10.1038/ncomms14361
Demirbilek, S., Emre, M. H., Aydın, E. N., Edali, M. N., Aksoy, R. T., Akın, M., et al. (2007). Sulfasalazine Reduces Inflammatory Renal Injury in Unilateral Ureteral Obstruction. Pediatr. Nephrol. 22 (6), 804–812. doi:10.1007/s00467-006-0416-8
Deng, J., Xia, Y., Zhou, Q., Wang, X., Xiong, C., Shao, X., et al. (2019). Protective Effect of Rosiglitazone on Chronic Renal Allograft Dysfunction in Rats. Transpl. Immunol. 54, 20–28. doi:10.1016/j.trim.2019.01.002
Dhungana, S., Taboy, C. H., Zak, O., Larvie, M., Crumbliss, A. L., and Aisen, P. (2004). Redox Properties of Human Transferrin Bound to its Receptor. Biochemistry 43 (1), 205–209. doi:10.1021/bi0353631
Dixon, S. J., Lemberg, K. M., Lamprecht, M. R., Skouta, R., Zaitsev, E. M., Gleason, C. E., et al. (2012). Ferroptosis: an Iron-dependent Form of Nonapoptotic Cell Death. Cell 149 (5), 1060–1072. doi:10.1016/j.cell.2012.03.042
Do Van, B., Gouel, F., Jonneaux, A., Timmerman, K., Gelé, P., Pétrault, M., et al. (2016). Ferroptosis, a Newly Characterized Form of Cell Death in Parkinson's Disease that Is Regulated by PKC. Neurobiol. Dis. 94, 169–178. doi:10.1016/j.nbd.2016.05.011
Dodson, M., Castro-Portuguez, R., and Zhang, D. D. (2019). NRF2 Plays a Critical Role in Mitigating Lipid Peroxidation and Ferroptosis. Redox Biol. 23, 101107. doi:10.1016/j.redox.2019.101107
Doll, S., Freitas, F. P., Shah, R., Aldrovandi, M., da Silva, M. C., Ingold, I., et al. (2019). FSP1 Is a Glutathione-independent Ferroptosis Suppressor. Nature 575 (7784), 693–698. doi:10.1038/s41586-019-1707-0
Doll, S., Proneth, B., Tyurina, Y. Y., Panzilius, E., Kobayashi, S., Ingold, I., et al. (2017). ACSL4 Dictates Ferroptosis Sensitivity by Shaping Cellular Lipid Composition. Nat. Chem. Biol. 13 (1), 91–98. doi:10.1038/nchembio.2239
Dolma, S., Lessnick, S. L., Hahn, W. C., and Stockwell, B. R. (2003). Identification of Genotype-Selective Antitumor Agents Using Synthetic Lethal Chemical Screening in Engineered Human Tumor Cells. Cancer cell 3 (3), 285–296. doi:10.1016/s1535-6108(03)00050-3
Dominguez, J. H., Wu, P., Hawes, J. W., Deeg, M., Walsh, J., Packer, S. C., et al. (2006). Renal Injury: Similarities and Differences in Male and Female Rats with the Metabolic Syndrome. Kidney Int. 69 (11), 1969–1976. doi:10.1038/sj.ki.5000406
Duann, P., Lin, P.-H., and Lianos, E. A. (2019). Data on Characterization of Metalloporphyrin-Mediated HO-1 and DAF Induction in Rat Glomeruli and Podocytes. Data in brief 22, 279–285. doi:10.1016/j.dib.2018.11.108
Duni, A., Liakopoulos, V., Roumeliotis, S., Peschos, D., and Dounousi, E. (2019). Oxidative Stress in the Pathogenesis and Evolution of Chronic Kidney Disease: Untangling Ariadne's Thread. Int.J. Mol Sci. 20 (15), 3711. doi:10.3390/ijms20153711
Echeverría, C., Montorfano, I., Sarmiento, D., Becerra, A., Nuñez‐Villena, F., Figueroa, X. F., et al. (2013). Lipopolysaccharide Induces a Fibrotic‐like Phenotype in Endothelial Cells. J. Cell. Mol. Med. 17 (6), 800–814. doi:10.1111/jcmm.12066
El Agaty, S. M. (2018). Triiodothyronine Attenuates the Progression of Renal Injury in a Rat Model of Chronic Kidney Disease. Can. J. Physiol. Pharmacol. 96 (6), 603–610. doi:10.1139/cjpp-2017-0252
el Nahas, A. M., Muchaneta-Kubara, E. C., Essaway, M., and Soylemezoglu, O. (1997). Renal Fibrosis: Insights into Pathogenesis and Treatment. Int. J. Biochem. Cell Biol. 29 (1), 55–62. doi:10.1016/s1357-2725(96)00119-7
Fang, X., Cai, Z., Wang, H., Han, D., Cheng, Q., Zhang, P., et al. (2020). Loss of Cardiac Ferritin H Facilitates Cardiomyopathy via Slc7a11-Mediated Ferroptosis. Circ. Res. 127 (4), 486–501. doi:10.1161/circresaha.120.316509
Fang, X., Wang, H., Han, D., Xie, E., Yang, X., Wei, J., et al. (2019). Ferroptosis as a Target for protection against Cardiomyopathy. Proc. Natl. Acad. Sci. U.S.A. 116 (7), 2672–2680. doi:10.1073/pnas.1821022116
Farias, J. S., Santos, K. M., Lima, N. K. S., Cabral, E. V., Aires, R. S., Veras, A. C., et al. (2020). Maternal Endotoxemia Induces Renal Collagen Deposition in Adult Offspring: Role of NADPH oxidase/TGF-β1/mmp-2 Signaling Pathway. Arch. Biochem. Biophys. 684, 108306. doi:10.1016/j.abb.2020.108306
Feng, H., Schorpp, K., Jin, J., Yozwiak, C. E., Hoffstrom, B. G., Decker, A. M., et al. (2020). Transferrin Receptor Is a Specific Ferroptosis Marker. Cell Rep. 30 (10), 3411–3423. e3417. doi:10.1016/j.celrep.2020.02.049
Feng, X., Wang, S., Sun, Z., Dong, H., Yu, H., Huang, M., et al. (2021). Ferroptosis Enhanced Diabetic Renal Tubular Injury via HIF-1α/HO-1 Pathway in Db/db Mice. Front. Endocrinol. 12, 626390. doi:10.3389/fendo.2021.626390
Fernández-Mendívil, C., Luengo, E., Trigo-Alonso, P., García-Magro, N., Negredo, P., and López, M. G. (2021). Protective Role of Microglial HO-1 Blockade in Aging: Implication of Iron Metabolism. Redox Biol. 38, 101789. doi:10.1016/j.redox.2020.101789
Fidai, I., Wachnowsky, C., and Cowan, J. A. (2016). Mapping Cellular Fe-S Cluster Uptake and Exchange Reactions - Divergent Pathways for Iron-Sulfur Cluster Delivery to Human Ferredoxins. Metallomics 8 (12), 1283–1293. doi:10.1039/c6mt00193a
Fink, S. L., and Cookson, B. T. (2005). Apoptosis, Pyroptosis, and Necrosis: Mechanistic Description of Dead and Dying Eukaryotic Cells. Infect. Immun. 73 (4), 1907–1916. doi:10.1128/iai.73.4.1907-1916.2005
Fox, C., Cocchiaro, P., Oakley, F., Howarth, R., Callaghan, K., Leslie, J., et al. (2016). Inhibition of Lysosomal Protease Cathepsin D Reduces Renal Fibrosis in Murine Chronic Kidney Disease. Sci. Rep. 6, 20101. doi:10.1038/srep20101
Fujimaki, M., Furuya, N., Saiki, S., Amo, T., Imamichi, Y., and Hattori, N. (2019). Iron Supply via NCOA4-Mediated Ferritin Degradation Maintains Mitochondrial Functions. Mol. Cell Biol 39 (14), e00010. doi:10.1128/mcb.00010-19
Galluzzi, L., Vitale, I., Aaronson, S. A., Abrams, J. M., Adam, D., Agostinis, P., et al. (2018). Molecular Mechanisms of Cell Death: Recommendations of the Nomenclature Committee on Cell Death 2018. Cell Death Differ 25 (3), 486–541. doi:10.1038/s41418-017-0012-4
Gao, M., Monian, P., Pan, Q., Zhang, W., Xiang, J., and Jiang, X. (2016). Ferroptosis Is an Autophagic Cell Death Process. Cell Res 26 (9), 1021–1032. doi:10.1038/cr.2016.95
Gao, M., Monian, P., Quadri, N., Ramasamy, R., and Jiang, X. (2015). Glutaminolysis and Transferrin Regulate Ferroptosis. Mol. Cell 59 (2), 298–308. doi:10.1016/j.molcel.2015.06.011
Geng, N., Shi, B. J., Li, S. L., Zhong, Z. Y., Li, Y. C., Xua, W. L., et al. (2018). Knockdown of Ferroportin Accelerates Erastin-Induced Ferroptosis in Neuroblastoma Cells. Eur. Rev. Med. Pharmacol. Sci. 22 (12), 3826–3836. doi:10.26355/eurrev_201806_15267
Giam, B., Kuruppu, S., Chu, P.-Y., Smith, A. I., Marques, F. Z., Fiedler, A., et al. (2017). N-Acetylcysteine Attenuates the Development of Renal Fibrosis in Transgenic Mice with Dilated Cardiomyopathy. Sci. Rep. 7 (1), 17718. doi:10.1038/s41598-017-17927-5
Gong, Y., Wang, N., Liu, N., and Dong, H. (2019). Lipid Peroxidation and GPX4 Inhibition Are Common Causes for Myofibroblast Differentiation and Ferroptosis. DNA Cell Biol. 38 (7), 725–733. doi:10.1089/dna.2018.4541
Gonzalez, A. A., Zamora, L., Reyes-Martinez, C., Salinas-Parra, N., Roldan, N., Cuevas, C. A., et al. (2017). (Pro)renin Receptor Activation Increases Profibrotic Markers and Fibroblast-Like Phenotype through MAPK-dependent ROS Formation in Mouse Renal Collecting Duct Cells. Clin. Exp. Pharmacol. Physiol. 44 (11), 1134–1144. doi:10.1111/1440-1681.12813
Gozzelino, R., and Arosio, P. (2016). Iron Homeostasis in Health and Disease. Int. J. Mol Sci. 17 (1), 130. doi:10.3390/ijms17010130
Gwinner, W., Landmesser, U., Brandes, R. P., Kubat, B., Plasger, J., Eberhard, O., et al. (1997). Reactive Oxygen Species and Antioxidant Defense in Puromycin Aminonucleoside Glomerulopathy. J. Am Soc Nephrol. 8 (11), 1722–1731. doi:10.1681/asn.v8111722
Gyurászová, M., Kovalčíková, A. G., Renczés, E., Kmeťová, K., Celec, P., Bábíčková, J., et al. (2019). Oxidative Stress in Animal Models of Acute and Chronic Renal Failure. Dis. Markers 2019, 1–10. doi:10.1155/2019/8690805
Han, J.-Y., Kim, Y.-J., Kim, L., Choi, S.-J., Park, I.-S., Kim, J.-M., et al. (2010). PPARγ Agonist and Angiotensin II Receptor Antagonist Ameliorate Renal Tubulointerstitial Fibrosis. J. Korean Med. Sci. 25 (1), 35–41. doi:10.3346/jkms.2010.25.1.35
Harris, D. C., and Tay, Y.-C. (1997). Mitochondrial Function in Rat Renal Cortex in Response to Proteinuria and Iron. Clin. Exp. Pharmacol. Physiol. 24 (12), 916–922. doi:10.1111/j.1440-1681.1997.tb02719.x
Hassannia, B., Wiernicki, B., Ingold, I., Qu, F., Van Herck, S., Tyurina, Y. Y., et al. (2018). Nano-Targeted Induction of Dual Ferroptotic Mechanisms Eradicates High-Risk Neuroblastoma. J. Clin. Invest. 128 (8), 3341–3355. doi:10.1172/jci99032
He, L., Girijashanker, K., Dalton, T. P., Reed, J., Li, H., Soleimani, M., et al. (2006). ZIP8, Member of the Solute-Carrier-39 (SLC39) Metal-Transporter Family: Characterization of Transporter Properties. Mol. Pharmacol. 70 (1), 171–180. doi:10.1124/mol.106.024521
He, T., Guan, X., Wang, S., Xiao, T., Yang, K., Xu, X., et al. (2015). Resveratrol Prevents High Glucose-Induced Epithelial-Mesenchymal Transition in Renal Tubular Epithelial Cells by Inhibiting NADPH oxidase/ROS/ERK Pathway. Mol. Cell Endocrinol. 402, 13–20. doi:10.1016/j.mce.2014.12.010
Helmy, M. M., Hashim, A. A., and Mouneir, S. M. (2018). Zileuton Alleviates Acute Cisplatin Nephrotoxicity: Inhibition of Lipoxygenase Pathway Favorably Modulates the Renal Oxidative/Inflammatory/Caspase-3 axis. Prostaglandins & other lipid mediators 135, 1–10. doi:10.1016/j.prostaglandins.2018.01.001
Hider, R. C., and Kong, X. (2013). Iron Speciation in the Cytosol: an Overview. Dalton Trans. 42 (9), 3220–3229. doi:10.1039/c2dt32149a
Hill, N. R., Fatoba, S. T., Oke, J. L., Hirst, J. A., O’Callaghan, C. A., Lasserson, D. S., et al. (2016). Global Prevalence of Chronic Kidney Disease - A Systematic Review and Meta-Analysis. Plos One 11 (7), e0158765. doi:10.1371/journal.pone.0158765
Himmelfarb, J. (2005). Relevance of Oxidative Pathways in the Pathophysiology of Chronic Kidney Disease. Cardiol. Clin. 23 (3), 319–330. doi:10.1016/j.ccl.2005.03.005
Ho, C., Lee, P.-H., Huang, W.-J., Hsu, Y.-C., Lin, C.-L., and Wang, J.-Y. (2007). Methylglyoxal-induced Fibronectin Gene Expression through Ras-Mediated NADPH Oxidase Activation in Renal Mesangial Cells. Nephrology 12 (4), 348–356. doi:10.1111/j.1440-1797.2007.00809.x
Hou, L., Huang, R., Sun, F., Zhang, L., and Wang, Q. (2019). NADPH Oxidase Regulates Paraquat and Maneb-Induced Dopaminergic Neurodegeneration through Ferroptosis. Toxicology 417, 64–73. doi:10.1016/j.tox.2019.02.011
Hou, W., Xie, Y., Song, X., Sun, X., Lotze, M. T., Zeh, H. J., et al. (2016). Autophagy Promotes Ferroptosis by Degradation of Ferritin. Autophagy 12 (8), 1425–1428. doi:10.1080/15548627.2016.1187366
Hu, Q., Gao, L., Peng, B., and Liu, X. (2017). Baicalin and Baicalein Attenuate Renal Fibrosis In Vitro via Inhibition of the TGF-Β1 Signaling Pathway. Exp. Ther. Med. 14 (4), 3074–3080. doi:10.3892/etm.2017.4888
Hu, W., Zhang, C., Wu, R., Sun, Y., Levine, A., and Feng, Z. (2010). Glutaminase 2, a Novel P53 Target Gene Regulating Energy Metabolism and Antioxidant Function. Proc. Natl. Acad. Sci. U.S.A. 107 (16), 7455–7460. doi:10.1073/pnas.1001006107
Huang, H., Jin, W. W., Huang, M., Ji, H., Capen, D. E., Xia, Y., et al. (2020). Gentamicin-Induced Acute Kidney Injury in an Animal Model Involves Programmed Necrosis of the Collecting Duct. J. Am. Soc. Nephrol. 31 (9), 2097–2115. doi:10.1681/ASN.2019020204
Ikeda, Y., Ozono, I., Tajima, S., Imao, M., Horinouchi, Y., Izawa-Ishizawa, Y., et al. (2014). Iron Chelation by Deferoxamine Prevents Renal Interstitial Fibrosis in Mice with Unilateral Ureteral Obstruction. PloS one 9 (2), e89355. doi:10.1371/journal.pone.0089355
Ikeuchi, H., Kuroiwa, T., Yamashita, S., Hiramatsu, N., Maeshima, A., Kaneko, Y., et al. (2004). Fluvastatin Reduces Renal Fibroblast Proliferation and Production of Type III Collagen: Therapeutic Implications for Tubulointerstitial Fibrosis. Nephron Exp. Nephrol. 97 (4), e115–e122. doi:10.1159/000079176
Ingold, I., Berndt, C., Schmitt, S., Doll, S., Poschmann, G., Buday, K., et al. (2018). Selenium Utilization by GPX4 Is Required to Prevent Hydroperoxide-Induced Ferroptosis. Cell 172 (3), 409–422. doi:10.1016/j.cell.2017.11.048
Jenkins, J. K., Huang, H., Ndebele, K., and Salahudeen, A. K. (2001). Vitamin e Inhibits Renal Mrna Expression of cox ii, ho i, tgf??, and Osteopontin in the Rat model of Cyclosporine Nephrotoxicity. Transplantation 71 (2), 331–334. doi:10.1097/00007890-200101270-00028
Jha, J. C., Banal, C., Chow, B. S. M., Cooper, M. E., and Jandeleit-Dahm, K. (2016). Diabetes and Kidney Disease: Role of Oxidative Stress. Antioxid. Redox Signaling 25 (12), 657–684. doi:10.1089/ars.2016.6664
Jia, L., Ma, X., Gui, B., Ge, H., Wang, L., Ou, Y., et al. (2015). Sorafenib Ameliorates Renal Fibrosis through Inhibition of TGF-β-Induced Epithelial-Mesenchymal Transition. Plos One 10 (2), e0117757. doi:10.1371/journal.pone.0117757
Jiang, L., Kon, N., Li, T., Wang, S.-J., Su, T., Hibshoosh, H., et al. (2015). Ferroptosis as a P53-Mediated Activity during Tumour Suppression. Nature 520 (7545), 57–62. doi:10.1038/nature14344
Jo, E.-K., Kim, J. K., Shin, D.-M., and Sasakawa, C. (2016). Molecular Mechanisms Regulating NLRP3 Inflammasome Activation. Cell Mol Immunol 13 (2), 148–159. doi:10.1038/cmi.2015.95
Jones, D. P. (2006). Redefining Oxidative Stress. Antioxid. Redox Signal. 8 (9-10), 1865–1879. doi:10.1089/ars.2006.8.1865
Jovanovic, D., Jovovic, D., Mihailovic-Stanojevic, N., Miloradovic, Z., Dimitrijevic, J., Maksic, N., et al. (2005). Influence of Carvedilol on Chronic Renal Failure Progression in Spontaneously Hypertensive Rats with Adriamycin Nephropathy. Clin. Nephrol 63 (6), 446–453. doi:10.5414/cnp63446
Kabei, K., Tateishi, Y., Shiota, M., Osada-Oka, M., Nishide, S., Uchida, J., et al. (2020). Effects of Orally Active Hypoxia Inducible Factor Alpha Prolyl Hydroxylase Inhibitor, FG4592 on Renal Fibrogenic Potential in Mouse Unilateral Ureteral Obstruction Model. J. Pharmacol. Sci. 142 (3), 93–100. doi:10.1016/j.jphs.2019.12.002
Kagan, V. E., Mao, G., Qu, F., Angeli, J. P. F., Doll, S., Croix, C. S., et al. (2017). Oxidized Arachidonic and Adrenic PEs Navigate Cells to Ferroptosis. Nat. Chem. Biol. 13 (1), 81–90. doi:10.1038/nchembio.2238
Kanasaki, K., Shi, S., Kanasaki, M., He, J., Nagai, T., Nakamura, Y., et al. (2014). Linagliptin-mediated DPP-4 Inhibition Ameliorates Kidney Fibrosis in Streptozotocin-Induced Diabetic Mice by Inhibiting Endothelial-To-Mesenchymal Transition in a Therapeutic Regimen. Diabetes 63 (6), 2120–2131. doi:10.2337/db13-1029
Kapturczak, M. H., Wasserfall, C., Brusko, T., Campbell-Thompson, M., Ellis, T. M., Atkinson, M. A., et al. (2004). Heme Oxygenase-1 Modulates Early Inflammatory Responses: Evidence From the Heme Oxygenase-1-Deficient Mouse. Am. J. Pathol. 165 (3), 1045–1053. doi:10.1016/s0002-9440(10)63365-2
Karuppagounder, S. S., Alin, L., Chen, Y., Brand, D., Bourassa, M. W., Dietrich, K., et al. (2018). N-Acetylcysteine Targets 5 Lipoxygenase-Derived, Toxic Lipids and can Synergize With Prostaglandin E2 to Inhibit Ferroptosis And Improve Outcomes Following Hemorrhagic Stroke in Mice. Ann. Neurol. 84 (6), 854–872. doi:10.1002/ana.25356
Kawai, T., Masaki, T., Doi, S., Arakawa, T., Yokoyama, Y., Doi, T., et al. (2009). PPAR-γ Agonist Attenuates Renal Interstitial Fibrosis and Inflammation Through Reduction of TGF-β. Lab. Invest. 89 (1), 47–58. doi:10.1038/labinvest.2008.104
Kesavardhana, S., Malireddi, R. K. S., and Kanneganti, T.-D. (2020). Caspases in Cell Death, Inflammation, and Pyroptosis. Annu. Rev. Immunol. 38, 567–595. doi:10.1146/annurev-immunol-073119-095439
Kim, E. H., Shin, D., Lee, J., Jung, A. R., and Roh, J.-L. (2018). CISD2 Inhibition Overcomes Resistance to Sulfasalazine-Induced Ferroptotic Cell Death in Head and Neck Cancer. Cancer Lett. 432, 180–190. doi:10.1016/j.canlet.2018.06.018
Kiss, E., Popovic, Z. V., Bedke, J., Adams, J., Bonrouhi, M., Babelova, A., et al. (2010). Peroxisome Proliferator-Activated Receptor (Ppar)Gamma Can Inhibit Chronic Renal Allograft Damage. Am. J. Pathol. 176 (5), 2150–2162. doi:10.2353/ajpath.2010.090370
Knutson, M. D. (2007). Steap Proteins: Implications for Iron and Copper Metabolism. Nut. Rev. 65 (7), 335–340. doi:10.1111/j.1753-4887.2007.tb00311.x10.1301/nr.2007.jul.335340
Koch, A., Völzke, A., Wünsche, C., Meyer zu Heringdorf, D., Huwiler, A., and Pfeilschifter, J. (2012). Thiazolidinedione-dependent Activation of Sphingosine Kinase 1 Causes an Anti-fibrotic Effect in Renal Mesangial Cells. Br. J. Pharmacol. 166 (3), 1018–1032. doi:10.1111/j.1476-5381.2012.01824.x
Koppenol, W. H. (1993). The Centennial of the Fenton Reaction. Free Radic. Biol. Med. 15 (6), 645–651. doi:10.1016/0891-5849(93)90168-t
Kortlever, R. M., Higgins, P. J., and Bernards, R. (2006). Plasminogen Activator Inhibitor-1 Is a Critical Downstream Target of P53 in the Induction of Replicative Senescence. Nat. Cell Biol 8 (8), 877–884. doi:10.1038/ncb1448
Kovtunovych, G., Eckhaus, M. A., Ghosh, M. C., Ollivierre-Wilson, H., and Rouault, T. A. (2010). Dysfunction of the Heme Recycling System in Heme Oxygenase 1-deficient Mice: Effects on Macrophage Viability and Tissue Iron Distribution. Blood 116 (26), 6054–6062. doi:10.1182/blood-2010-03-272138
Kozyraki, R., Fyfe, J., Verroust, P. J., Jacobsen, C., Dautry-Varsat, A., Gburek, J., et al. (2001). Megalin-Dependent Cubilin-Mediated Endocytosis Is a Major Pathway for the Apical Uptake of Transferrin in Polarized Epithelia. Proc. Natl. Acad. Sci. U.S.A. 98 (22), 12491–12496. doi:10.1073/pnas.211291398
Kroot, J. J., Tjalsma, H., Fleming, R. E., and Swinkels, D. W. (2011). Hepcidin in Human Iron Disorders: Diagnostic Implications. Clin. Chem. 57 (12), 1650–1669. doi:10.1373/clinchem.2009.140053
Kumar, C., Igbaria, A., D'Autreaux, B., Planson, A.-G., Junot, C., Godat, E., et al. (2011). Glutathione Revisited: a Vital Function in Iron Metabolism and Ancillary Role in Thiol-Redox Control. EMBO J. 30 (10), 2044–2056. doi:10.1038/emboj.2011.105
Langelueddecke, C., Roussa, E., Fenton, R. A., Wolff, N. A., Lee, W.-K., and Thévenod, F. (2012). Lipocalin-2 (24p3/Neutrophil Gelatinase-Associated Lipocalin (NGAL)) Receptor Is Expressed in Distal Nephron and Mediates Protein Endocytosis. J. Biol. Chem. 287 (1), 159–169. doi:10.1074/jbc.M111.308296
Latunde-Dada, G. O. (2017). Ferroptosis: Role of Lipid Peroxidation, Iron and Ferritinophagy. Biochim. Biophys. Acta (Bba) - Gen. Subjects 1861 (8), 1893–1900. doi:10.1016/j.bbagen.2017.05.019
Le Blanc, S., Garrick, M. D., and Arredondo, M. (2012). Heme Carrier Protein 1 Transports Heme and Is Involved in Heme-Fe Metabolism. Am. J. Physiology-Cell Physiol. 302 (12), C1780–C1785. doi:10.1152/ajpcell.00080.2012
Lee, W.-J., Liu, S.-H., Chiang, C.-K., Lin, S.-Y., Liang, K.-W., Chen, C.-H., et al. (2016). Aryl Hydrocarbon Receptor Deficiency Attenuates Oxidative Stress-Related Mesangial Cell Activation and Macrophage Infiltration and Extracellular Matrix Accumulation in Diabetic Nephropathy. Antioxid. Redox Signaling 24 (4), 217–231. doi:10.1089/ars.2015.6310
Li, C., Deng, X., Xie, X., Liu, Y., Friedmann Angeli, J. P., and Lai, L. (2018). Activation of Glutathione Peroxidase 4 as a Novel Anti-inflammatory Strategy. Front. Pharmacol. 9, 1120. doi:10.3389/fphar.2018.01120
Li, C., Xie, N., Li, Y., Liu, C., Hou, F. F., and Wang, J. (2019). N-Acetylcysteine Ameliorates Cisplatin-Induced Renal Senescence and Renal Interstitial Fibrosis through Sirtuin1 Activation and P53 Deacetylation. Free Radic. Biol. Med. 130, 512–527. doi:10.1016/j.freeradbiomed.2018.11.006
Li, Y., Feng, D., Wang, Z., Zhao, Y., Sun, R., Tian, D., et al. (2019). Ischemia-induced ACSL4 Activation Contributes to Ferroptosis-Mediated Tissue Injury in Intestinal Ischemia/reperfusion. Cell Death Differ 26 (11), 2284–2299. doi:10.1038/s41418-019-0299-4
Li, J., Yang, J., Zhu, B., Fan, J., Hu, Q., and Wang, L. (2022). Tectorigenin Protects against Unilateral Ureteral Obstruction by Inhibiting Smad3‐mediated Ferroptosis and Fibrosis. Phytotherapy Res. 36 (1), 475–487. doi:10.1002/ptr.7353
Li, R., Zhang, J., Zhou, Y., Gao, Q., Wang, R., Fu, Y., et al. (2020). Transcriptome Investigation and In Vitro Verification of Curcumin-Induced HO-1 as a Feature of Ferroptosis in Breast Cancer Cells. Oxidative Med. Cell Longevity 2020, 1–18. doi:10.1155/2020/3469840
Li, X., Zou, Y., Xing, J., Fu, Y.-Y., Wang, K.-Y., Wan, P.-Z., et al. (2020). Pretreatment with Roxadustat (Fg-4592) Attenuates Folic Acid-Induced Kidney Injury Through Antiferroptosis Via Akt/Gsk-3 Β/Nrf2 Pathway. Oxidative Med. Cell. longevity 2020, 1–17. doi:10.1155/2020/6286984
Li, Y., Jin, C., Shen, M., Wang, Z., Tan, S., Chen, A., et al. (2020). Iron Regulatory Protein 2 Is Required for Artemether -mediated Anti-hepatic Fibrosis through Ferroptosis Pathway. Free Radic. Biol. Med. 160, 845–859. doi:10.1016/j.freeradbiomed.2020.09.008
Li, S., Zheng, L., Zhang, J., Liu, X., and Wu, Z. (2021). Inhibition of Ferroptosis by Up-Regulating Nrf2 Delayed the Progression of Diabetic Nephropathy. Free Radic. Biol. Med. 162, 435–449. doi:10.1016/j.freeradbiomed.2020.10.323
Li, W., Sun, J., Zhou, X., Lu, Y., Cui, W., and Miao, L. (2021). Mini-Review: GSDME-Mediated Pyroptosis in Diabetic Nephropathy. Front. Pharmacol. 12, 780790. doi:10.3389/fphar.2021.780790
Li, Y., Yuan, Y., Huang, Z.-X., Chen, H., Lan, R., Wang, Z., et al. (2021). GSDME-mediated Pyroptosis Promotes Inflammation and Fibrosis in Obstructive Nephropathy. Cell Death Differ 28 (8), 2333–2350. doi:10.1038/s41418-021-00755-6
Liang, X., Duan, N., Wang, Y., Shu, S., Xiang, X., Guo, T., et al. (2016). Advanced Oxidation Protein Products Induce Endothelial-To-Mesenchymal Transition in Human Renal Glomerular Endothelial Cells through Induction of Endoplasmic Reticulum Stress. J. Diabetes its Complications 30 (4), 573–579. doi:10.1016/j.jdiacomp.2016.01.009
Linkermann, A., Bräsen, J. H., Darding, M., Jin, M. K., Sanz, A. B., Heller, J.-O., et al. (2013). Two Independent Pathways of Regulated Necrosis Mediate Ischemia-Reperfusion Injury. Proc. Natl. Acad. Sci. U.S.A. 110 (29), 12024–12029. doi:10.1073/pnas.1305538110
Linkermann, A., Skouta, R., Himmerkus, N., Mulay, S. R., Dewitz, C., De Zen, F., et al. (2014). Synchronized Renal Tubular Cell Death Involves Ferroptosis. Proc. Natl. Acad. Sci. U.S.A. 111 (47), 16836–16841. doi:10.1073/pnas.1415518111
Liu, B., Zhao, C., Li, H., Chen, X., Ding, Y., and Xu, S. (2018). Puerarin Protects against Heart Failure Induced by Pressure Overload through Mitigation of Ferroptosis. Biochem. biophysical Res. Commun. 497 (1), 233–240. doi:10.1016/j.bbrc.2018.02.061
Liu, P., Zhang, B., Chen, Z., He, Y., Du, Y., Liu, Y., et al. (2020). m6A-Induced lncRNA MALAT1 Aggravates Renal Fibrogenesis in Obstructive Nephropathy through the miR-145/FAK Pathway. Aging 12 (6), 5280–5299. doi:10.18632/aging.102950
Liu, Y., and Templeton, D. M. (2015). Iron-Dependent Turnover of IRP-1/c-Aconitase in Kidney Cells. Metallomics 7 (5), 766–775. doi:10.1039/c4mt00315b
Liu, Y., Wang, W., Li, Y., Xiao, Y., Cheng, J., and Jia, J. (2015). The 5-Lipoxygenase Inhibitor Zileuton Confers Neuroprotection against Glutamate Oxidative Damage by Inhibiting Ferroptosis. Biol. Pharm. Bull. 38 (8), 1234–1239. doi:10.1248/bpb.b15-00048
Lőrincz, T., Jemnitz, K., Kardon, T., Mandl, J., and Szarka, A. (2015). Ferroptosis Is Involved in Acetaminophen Induced Cell Death. Pathol. Oncol. Res. 21 (4), 1115–1121. doi:10.1007/s12253-015-9946-3
Louandre, C., Ezzoukhry, Z., Godin, C., Barbare, J.-C., Mazière, J.-C., Chauffert, B., et al. (2013). Iron-Dependent Cell Death of Hepatocellular Carcinoma Cells Exposed to Sorafenib. Int. J. Cancer 133 (7), 1732–1742. doi:10.1002/ijc.28159
Louandre, C., Marcq, I., Bouhlal, H., Lachaier, E., Godin, C., Saidak, Z., et al. (2015). The Retinoblastoma (Rb) Protein Regulates Ferroptosis Induced by Sorafenib in Human Hepatocellular Carcinoma Cells. Cancer Lett. 356 (2 Pt B), 971–977. doi:10.1016/j.canlet.2014.11.014
Lu, Q., Zhou, Y., Hao, M., Li, C., Wang, J., Shu, F., et al. (2018). The mTOR Promotes Oxidative Stress-Induced Apoptosis of Mesangial Cells in Diabetic Nephropathy. Mol. Cell Endocrinol. 473, 31–43. doi:10.1016/j.mce.2017.12.012
Lu, X., Chen, Z., Liang, H., Li, Z., Zou, X., Luo, H., et al. (2013). Thyroid Hormone Inhibits TGFβ1 Induced Renal Tubular Epithelial to Mesenchymal Transition by Increasing miR34a Expression. Cell Signal. 25 (10), 1949–1954. doi:10.1016/j.cellsig.2013.06.005
Ma, W., Tao, L., Wang, X., Liu, Q., Zhang, W., Li, Q., et al. (2016). Sorafenib Inhibits Renal Fibrosis Induced by Unilateral Ureteral Obstruction via Inhibition of Macrophage Infiltration. Cell Physiol Biochem 39 (5), 1837–1849. doi:10.1159/000447883
Mao, C., Liu, X., Zhang, Y., Lei, G., Yan, Y., Lee, H., et al. (2021). DHODH-mediated Ferroptosis Defence Is a Targetable Vulnerability in Cancer. Nature 593, 586–590. doi:10.1038/s41586-021-03539-7
Marshall, C. B., Pippin, J. W., Krofft, R. D., and Shankland, S. J. (2006). Puromycin Aminonucleoside Induces Oxidant-dependent DNA Damage in Podocytes In Vitro and In Vivo. Kidney Int. 70 (11), 1962–1973. doi:10.1038/sj.ki.5001965
Martin-Sanchez, D., Ruiz-Andres, O., Poveda, J., Carrasco, S., Cannata-Ortiz, P., Sanchez-Niño, M. D., et al. (2017). Ferroptosis, but Not Necroptosis, Is Important in Nephrotoxic Folic Acid-Induced AKI. J. Am. Soc. Nephrol. 28 (1), 218–229. doi:10.1681/asn.2015121376
Martines, A. M. F., Masereeuw, R., Tjalsma, H., Hoenderop, J. G., Wetzels, J. F. M., and Swinkels, D. W. (2013). Iron Metabolism in the Pathogenesis of Iron-Induced Kidney Injury. Nat. Rev. Nephrol. 9 (7), 385–398. doi:10.1038/nrneph.2013.98
Matsumoto, M., Sasaki, N., Tsujino, T., Akahori, H., Naito, Y., and Masuyama, T. (2013). Iron Restriction Prevents Diabetic Nephropathy in Otsuka Long-Evans Tokushima Fatty Rat. Ren. Fail. 35 (8), 1156–1162. doi:10.3109/0886022X.2013.819729
McCarthy, K. J., Routh, R. E., Shaw, W., Walsh, K., Welbourne, T. C., and Johnson, J. H. (2000). Troglitazone Halts Diabetic Glomerulosclerosis by Blockade of Mesangial Expansion. Kidney Int. 58 (6), 2341–2350. doi:10.1046/j.1523-1755.2000.00418.x
Miotto, G., Rossetto, M., Di Paolo, M. L., Orian, L., Venerando, R., Roveri, A., et al. (2020). Insight into the Mechanism of Ferroptosis Inhibition by Ferrostatin-1. Redox Biol. 28, 101328. doi:10.1016/j.redox.2019.101328
Mishima, E., Sato, E., Ito, J., Yamada, K.-i., Suzuki, C., Oikawa, Y., et al. (2020). Drugs Repurposed as Antiferroptosis Agents Suppress Organ Damage, Including AKI, by Functioning as Lipid Peroxyl Radical Scavengers. J. Am. Soc. Nephrol. 31 (2), 280–296. doi:10.1681/ASN.2019060570
Montford, J. R., Bauer, C., Dobrinskikh, E., Hopp, K., Levi, M., Weiser-Evans, M., et al. (2019). Inhibition of 5-Lipoxygenase Decreases Renal Fibrosis and Progression of Chronic Kidney Disease. Am. J. Physiology-Renal PhysiologyRenal Physiol. 316 (4), F732–F742. doi:10.1152/ajprenal.00262.2018
Montorfano, I., Becerra, A., Cerro, R., Echeverría, C., Sáez, E., Morales, M. G., et al. (2014). Oxidative Stress Mediates the Conversion of Endothelial Cells into Myofibroblasts via a TGF-Β1 and TGF-β2-Dependent Pathway. Lab. Invest. 94 (10), 1068–1082. doi:10.1038/labinvest.2014.100
Moore, B., and Hawkes, J. L. (1908). An Investigation of the Toxic Actions of Dilute Solutions of the Salts of Certain Heavy Metals (viz.: Copper, Iron, Nickel, Cobalt, Manganese, Zinc, Silver, and Lead) Upon the Bacillus Typhosus, with a View to Practical Application in the Purification of Shell-Fish. Biochem. J. 3 (6-8), 313–345. doi:10.1042/bj0030313
Moriyama, T., Kawada, N., Nagatoya, K., Takeji, M., Horio, M., Ando, A., et al. (2001). Fluvastatin Suppresses Oxidative Stress and Fibrosis in the Interstitium of Mouse Kidneys with Unilateral Ureteral Obstruction. Kidney Int. 59 (6), 2095–2103. doi:10.1046/j.1523-1755.2001.0590062095.x
Murphy, T. H., Miyamoto, M., Sastre, A., Schnaar, R. L., and Coyle, J. T. (1989). Glutamate Toxicity in a Neuronal Cell Line Involves Inhibition of Cystine Transport Leading to Oxidative Stress. Neuron 2 (6), 1547–1558. doi:10.1016/0896-6273(89)90043-3
Nagpal, A., Redvers, R. P., Ling, X., Ayton, S., Fuentes, M., Tavancheh, E., et al. (2019). Neoadjuvant Neratinib Promotes Ferroptosis and Inhibits Brain Metastasis in a Novel Syngeneic Model of Spontaneous Her2 +Ve Breast Cancer Metastasis. Breast Cancer Res. 21 (1), 94. doi:10.1186/s13058-019-1177-1
Naito, Y., Fujii, A., Sawada, H., Oboshi, M., Iwasaku, T., Okuhara, Y., et al. (2015). Association Between Renal Iron Accumulation and Renal Interstitial Fibrosis in a Rat Model of Chronic Kidney Disease. Hypertens. Res. 38 (7), 463–470. doi:10.1038/hr.2015.14
Nakanishi, T., Nanami, M., and Kuragano, T. (2020). The Pathogenesis of CKD Complications; Attack of Dysregulated Iron and Phosphate Metabolism. Free Radic. Biol. Med. 157, 55–62. doi:10.1016/j.freeradbiomed.2020.01.024
Nankivell, B. J., Chen, J., Boadle, R. A., and Harris, D. C. (1994). The Role of Tubular Iron Accumulation in the Remnant Kidney. J. Am. Soc. Nephrol. 4 (8), 1598–1607. doi:10.1681/asn.v481598
Németh, Á., Mózes, M. M., Calvier, L., Hansmann, G., and Kökény, G. (2019). The PPARγ Agonist Pioglitazone Prevents TGF-β Induced Renal Fibrosis by Repressing EGR-1 and STAT3. BMC Nephrol. 20 (1), 245. doi:10.1186/s12882-019-1431-x
Newcomer, M. E., and Brash, A. R. (2015). The Structural Basis for Specificity in Lipoxygenase Catalysis. Protein Sci. 24 (3), 298–309. doi:10.1002/pro.2626
Neyens, E., and Baeyens, J. (2003). A Review of Classic Fenton's Peroxidation as an Advanced Oxidation Technique. J. Hazard. Mater. 98 (1-3), 33–50. doi:10.1016/s0304-3894(02)00282-0
Nlandu Khodo, S., Dizin, E., Sossauer, G., Szanto, I., Martin, P.-Y., Feraille, E., et al. (2012). NADPH-oxidase 4 Protects against Kidney Fibrosis during Chronic Renal Injury. J. Am. Soc. Nephrol. 23 (12), 1967–1976. doi:10.1681/ASN.2012040373
Odacı, E., Ünal, D., Mercantepe, T., Topal, Z., Hancı, H., Türedi, S., et al. (2015). Pathological Effects of Prenatal Exposure to a 900 MHz Electromagnetic Field on the 21-Day-Old Male Rat Kidney. Biotech. Histochem. 90 (2), 93–101. doi:10.3109/10520295.2014.947322
Okamura, D. M., and Pennathur, S. (2015). The Balance of powers: Redox Regulation of Fibrogenic Pathways in Kidney Injury. Redox Biol. 6, 495–504. doi:10.1016/j.redox.2015.09.039
Omasu, F., Oda, T., Yamada, M., Yoshizawa, N., Yamakami, K., Sakurai, Y., et al. (2007). Effects of Pioglitazone and Candesartan on Renal Fibrosis and the Intrarenal Plasmin cascade in Spontaneously Hypercholesterolemic Rats. Am. J. Physiology-Renal Physiol. 293 (4), F1292–F1298. doi:10.1152/ajprenal.00232.2007
Oraby, M. A., El-Yamany, M. F., Safar, M. M., Assaf, N., and Ghoneim, H. A. (2019). Amelioration of Early Markers of Diabetic Nephropathy by Linagliptin in Fructose-Streptozotocin-Induced Type 2 Diabetic Rats. Nephron 141 (4), 273–286. doi:10.1159/000495517
Ou, Y., Wang, S. J., Li, D., Chu, B., and Gu, W. (2016). Activation of SAT1 Engages Polyamine Metabolism with P53-Mediated Ferroptotic Responses. Proc. Natl. Acad. Sci. U S A. 113 (44), E6806–E6812. doi:10.1073/pnas.1607152113
Paller, M. S., Hedlund, B. E., Sikora, J. J., Faassen, A., and Waterfield, R. (1988). Role of Iron in Postischemic Renal Injury in the Rat. Kidney Int. 34 (4), 474–480. doi:10.1038/ki.1988.205
Panchapakesan, U., Sumual, S., Pollock, C. A., and Chen, X. (2005). PPARγ Agonists Exert Antifibrotic Effects in Renal Tubular Cells Exposed to High Glucose. Am. J. Physiology-Renal Physiol. 289 (5), F1153–F1158. doi:10.1152/ajprenal.00097.2005
Paragas, N., Qiu, A., Hollmen, M., Nickolas, T. L., Devarajan, P., and Barasch, J. (2012). NGAL-siderocalin in Kidney Disease. Biochim. Biophys. Acta (Bba) - Mol. Cell Res. 1823 (9), 1451–1458. doi:10.1016/j.bbamcr.2012.06.014
Park, M. W., Cha, H. W., Kim, J., Kim, J. H., Yang, H., Yoon, S., et al. (2021). NOX4 Promotes Ferroptosis of Astrocytes by Oxidative Stress-Induced Lipid Peroxidation via the Impairment of Mitochondrial Metabolism in Alzheimer's Diseases. Redox Biol. 41, 101947. doi:10.1016/j.redox.2021.101947
Park, Y. J., An, H.-T., Park, J.-S., Park, O., Duh, A. J., Kim, K., et al. (2020). Tyrosine Kinase Inhibitor Neratinib Attenuates Liver Fibrosis by Targeting Activated Hepatic Stellate Cells. Sci. Rep. 10 (1), 14756. doi:10.1038/s41598-020-71688-2
Pei, Y., Xu, Y., Ruan, J., Rong, L., Jiang, M., Mo, Y., et al. (2016). Plasma Oxidative Stress Level of IgA Nephropathy in Children and the Effect of Early Intervention with Angiotensin-Converting Enzyme Inhibitors. J. Renin Angiotensin Aldosterone Syst. 17 (2), 147032031664724. doi:10.1177/1470320316647240
Pengrattanachot, N., Cherngwelling, R., Jaikumkao, K., Pongchaidecha, A., Thongnak, L., Swe, M. T., et al. (2020). Atorvastatin Attenuates Obese-Induced Kidney Injury and Impaired Renal Organic Anion Transporter 3 Function through Inhibition of Oxidative Stress and Inflammation. Biochim. Biophys. Acta (Bba) - Mol. Basis Dis. 1866 (6), 165741. doi:10.1016/j.bbadis.2020.165741
Philpott, C. C., Ryu, M.-S., Frey, A., and Patel, S. (2017). Cytosolic Iron Chaperones: Proteins Delivering Iron Cofactors in the Cytosol of Mammalian Cells. J. Biol. Chem. 292 (31), 12764–12771. doi:10.1074/jbc.R117.791962
Philpott, C. C. (2018). The Flux of Iron Through Ferritin in Erythrocyte Development. Curr. Opin. Hematol. 25 (3), 183–188. doi:10.1097/MOH.0000000000000417
Piao, S. G., Kang, S. H., Lim, S. W., Chung, B. H., Doh, K. C., Heo, S. B., et al. (2013). Influence of N-Acetylcysteine on Klotho Expression and its Signaling Pathway in Experimental Model of Chronic Cyclosporine Nephropathy in Mice. Transplantation 96 (2), 146–153. doi:10.1097/TP.0b013e318296c9a9
Pinilla-Tenas, J. J., Sparkman, B. K., Shawki, A., Illing, A. C., Mitchell, C. J., Zhao, N., et al. (2011). Zip14 Is a Complex Broad-Scope Metal-Ion Transporter Whose Functional Properties Support Roles in the Cellular Uptake of Zinc and Nontransferrin-Bound Iron. Am. J. Physiology-Cell Physiol. 301 (4), C862–C871. doi:10.1152/ajpcell.00479.2010
Pisani, A., Riccio, E., Andreucci, M., Faga, T., Ashour, M., Di Nuzzi, A., et al. (2013). Role of Reactive Oxygen Species in Pathogenesis of Radiocontrast-Induced Nephropathy. Biomed. Res. Int. 2013, 1–6. doi:10.1155/2013/868321
Portilla, D. (2014). Apoptosis, Fibrosis and Senescence. Nephron Clin. Pract. 127 (1-4), 65–69. doi:10.1159/000363717
Qiu, Y., Cao, Y., Cao, W., Jia, Y., and Lu, N. (2020). The Application of Ferroptosis in Diseases. Pharmacol. Res. 159, 104919. doi:10.1016/j.phrs.2020.104919
Rajapurkar, M. M., Hegde, U., Bhattacharya, A., Alam, M. G., and Shah, S. V. (2013). Effect of Deferiprone, an Oral Iron Chelator, in Diabetic and Non-diabetic Glomerular Disease. Toxicol. Mech. Methods 23 (1), 5–10. doi:10.3109/15376516.2012.730558
Raupach, B., Peuschel, S.-K., Monack, D. M., and Zychlinsky, A. (2006). Caspase-1-Mediated Activation of Interleukin-1β (IL-1β) and IL-18 Contributes to Innate Immune Defenses against Salmonella Enterica Serovar Typhimurium Infection. Infect. Immun. 74 (8), 4922–4926. doi:10.1128/iai.00417-06
Rosa, L., Cutone, A., Lepanto, M., Paesano, R., and Valenti, P. (2017). Lactoferrin: A Natural Glycoprotein Involved in Iron and Inflammatory Homeostasis. Int. J. Mol. Sci. 18 (9), 1985. doi:10.3390/ijms18091985
Rubio‐Navarro, A., Sanchez‐Niño, M. D., Guerrero‐Hue, M., García‐Caballero, C., Gutiérrez, E., Yuste, C., et al. (2018). Podocytes Are New Cellular Targets of Haemoglobin‐Mediated Renal Damage. J. Pathol. 244 (3), 296–310. doi:10.1002/path.5011
Ryu, M.-S., Duck, K. A., and Philpott, C. C. (2018). Ferritin Iron Regulators, PCBP1 and NCOA4, Respond to Cellular Iron Status in Developing Red Cells. Blood Cell Mol. Dis. 69, 75–81. doi:10.1016/j.bcmd.2017.09.009
Salahudeen, A. A., Thompson, J. W., Ruiz, J. C., Ma, H.-W., Kinch, L. N., Li, Q., et al. (2009). An E3 Ligase Possessing an Iron-Responsive Hemerythrin Domain Is a Regulator of Iron Homeostasis. Science 326 (5953), 722–726. doi:10.1126/science.1176326
Samarakoon, R., Overstreet, J. M., Higgins, S. P., and Higgins, P. J. (2012). TGF-β1 → SMAD/p53/USF2 → PAI-1 Transcriptional axis in Ureteral Obstruction-Induced Renal Fibrosis. Cell Tissue Res 347 (1), 117–128. doi:10.1007/s00441-011-1181-y
Scindia, Y., Leeds, J., and Swaminathan, S. (2019). Iron Homeostasis in Healthy Kidney and its Role in Acute Kidney Injury. Semin. Nephrol. 39 (1), 76–84. doi:10.1016/j.semnephrol.2018.10.006
Sears, D. A., Anderson, P. R., Foy, A. L., Williams, H. L., and Crosby, W. H. (1966). Urinary Iron Excretion and Renal Metabolism of Hemoglobin in Hemolytic Diseases. Blood 28 (5), 708–725. doi:10.1182/blood.v28.5.708.708
Sedeek, M., Callera, G., Montezano, A., Gutsol, A., Heitz, F., Szyndralewiez, C., et al. (2010). Critical Role of Nox4-Based NADPH Oxidase in Glucose-Induced Oxidative Stress in the Kidney: Implications in Type 2 Diabetic Nephropathy. Am. J. Physiology-Renal Physiol. 299 (6), F1348–F1358. doi:10.1152/ajprenal.00028.2010
Seiler, A., Schneider, M., Förster, H., Roth, S., Wirth, E. K., Culmsee, C., et al. (2008). Glutathione Peroxidase 4 Senses and Translates Oxidative Stress into 12/15-lipoxygenase Dependent- and AIF-Mediated Cell Death. Cell Metab. 8 (3), 237–248. doi:10.1016/j.cmet.2008.07.005
Shah, S. V., Baliga, R., Rajapurkar, M., and Fonseca, V. A. (2007). Oxidants in Chronic Kidney Disease. J. Am. Soc. Nephrol. 18 (1), 16–28. doi:10.1681/ASN.2006050500
Shen, Y., Miao, N.-J., Xu, J.-L., Gan, X.-X., Xu, D., Zhou, L., et al. (2016). N-acetylcysteine Alleviates Angiotensin II-Mediated Renal Fibrosis in Mouse Obstructed Kidneys. Acta Pharmacol. Sin 37 (5), 637–644. doi:10.1038/aps.2016.12
Shi, H., Bencze, K. Z., Stemmler, T. L., and Philpott, C. C. (2008). A Cytosolic Iron Chaperone that Delivers Iron to Ferritin. Science 320 (5880), 1207–1210. doi:10.1126/science.1157643
Shimada, K., Skouta, R., Kaplan, A., Yang, W. S., Hayano, M., Dixon, S. J., et al. (2016). Global Survey of Cell Death Mechanisms Reveals Metabolic Regulation of Ferroptosis. Nat. Chem. Biol. 12 (7), 497–503. doi:10.1038/nchembio.2079
Shui, G.-X., Sang, D., Yin, X., Cai, Y., and Sun, W. (2017). Dahuang Fuzi Decoction Attenuates Renal Fibrosis and Ameliorates Mitochondrial Dysfunction in Chronic Aristolochic Acid Nephropathy. Evid Based. Complement. Altern. medicine. eCAM 2017, 1–8. doi:10.1155/2017/9536458
Smith, C. P., Lee, W.-K., Haley, M., Poulsen, S. B., Thévenod, F., and Fenton, R. A. (2019). Proximal Tubule Transferrin Uptake Is Modulated by Cellular Iron and Mediated by Apical Membrane Megalin-Cubilin Complex and Transferrin Receptor 1. J. Biol. Chem. 294 (17), 7025–7036. doi:10.1074/jbc.RA118.006390
Smith, C. P., and Thévenod, F. (2009). Iron Transport and the Kidney. Biochim. Biophys. Acta (Bba) - Gen. Subjects 1790 (7), 724–730. doi:10.1016/j.bbagen.2008.10.010
Sol, M., Kamps, J. A. A. M., van den Born, J., van den Heuvel, M. C., van der Vlag, J., Krenning, G., et al. (2020). Glomerular Endothelial Cells as Instigators of Glomerular Sclerotic Diseases. Front. Pharmacol. 11, 573557. doi:10.3389/fphar.2020.573557
Song, Y., Tao, Q., Yu, L., Li, L., Bai, T., Song, X., et al. (2018). Activation of Autophagy Contributes to the Renoprotective Effect of Postconditioning on Acute Kidney Injury and Renal Fibrosis. Biochem. Biophysical Res. Commun. 504 (4), 641–646. doi:10.1016/j.bbrc.2018.09.003
Song, Y., Wang, B., Zhu, X., Hu, J., Sun, J., Xuan, J., et al. (2021). Human Umbilical Cord Blood-Derived MSCs Exosome Attenuate Myocardial Injury by Inhibiting Ferroptosis in Acute Myocardial Infarction Mice. Cell Biol Toxicol 37 (1), 51–64. doi:10.1007/s10565-020-09530-8
Soulage, C. O., Pelletier, C. C., Florens, N., Lemoine, S., Dubourg, L., Juillard, L., et al. (2020). Two Toxic Lipid Aldehydes, 4-Hydroxy-2-Hexenal (4-HHE) and 4-Hydroxy-2-Nonenal (4-HNE), Accumulate in Patients with Chronic Kidney Disease. Toxins 12 (9), 567. doi:10.3390/toxins12090567
Sponsel, H. T., Alfrey, A. C., Hammond, W. S., Durr, J. A., Ray, C., and Anderson, R. J. (1996). Effect of Iron on Renal Tubular Epithelial Cells. Kidney Int. 50 (2), 436–444. doi:10.1038/ki.1996.334
Stockwell, B. R., Friedmann Angeli, J. P., Bayir, H., Bush, A. I., Conrad, M., Dixon, S. J., et al. (2017). Ferroptosis: A Regulated Cell Death Nexus Linking Metabolism, Redox Biology, and Disease. Cell 171 (2), 273–285. doi:10.1016/j.cell.2017.09.021
Su, H., Chen, S., He, F.-F., Wang, Y.-M., Bondzie, P., and Zhang, C. (2015). New Insights into Glomerular Parietal Epithelial Cell Activation and its Signaling Pathways in Glomerular Diseases. Biomed. Res. Int. 2015, 1–8. doi:10.1155/2015/318935
Su, H., Wan, C., Song, A., Qiu, Y., Xiong, W., and Zhang, C. (2019). Oxidative Stress and Renal Fibrosis: Mechanisms and Therapies. Adv. Exp. Med. Biol. 1165, 585–604. doi:10.1007/978-981-13-8871-2_29
Sun, L., Xu, T., Chen, Y., Qu, W., Sun, D., Song, X., et al. (2019). Pioglitazone Attenuates Kidney Fibrosis via miR-21-5p Modulation. Life Sci. 232, 116609. doi:10.1016/j.lfs.2019.116609
Sun, L., Yuan, Q., Xu, T., Yao, L., Feng, J., Ma, J., et al. (2017). Pioglitazone Improves Mitochondrial Function in the Remnant Kidney and Protects against Renal Fibrosis in 5/6 Nephrectomized Rats. Front. Pharmacol. 8, 545. doi:10.3389/fphar.2017.00545
Sun, X., Ou, Z., Chen, R., Niu, X., Chen, D., Kang, R., et al. (2016). Activation of the P62-Keap1-NRF2 Pathway Protects against Ferroptosis in Hepatocellular Carcinoma Cells. Hepatology 63 (1), 173–184. doi:10.1002/hep.28251
Sun, X., Ou, Z., Xie, M., Kang, R., Fan, Y., Niu, X., et al. (2015). HSPB1 as a Novel Regulator of Ferroptotic Cancer Cell Death. Oncogene 34 (45), 5617–5625. doi:10.1038/onc.2015.32
Sureshbabu, A., Ryter, S. W., and Choi, M. E. (2015). Oxidative Stress and Autophagy: Crucial Modulators of Kidney Injury. Redox Biol. 4, 208–214. doi:10.1016/j.redox.2015.01.001
Swinkels, D. W., Janssen, M. C., Bergmans, J., and Marx, J. J. (2006). Hereditary Hemochromatosis: Genetic Complexity and New Diagnostic Approaches. Clin. Chem. 52 (6), 950–968. doi:10.1373/clinchem.2006.068684
Taccone-Gallucci, M., Lubrano, R., Belli, A., Meloni, C., Morosetti, M., Meschini, L., et al. (1989). Disappearance of Oxidative Damage to Red Blood Cell Membranes in Uremic Patients Following Renal Transplant. ASAIO Trans. 35 (3), 533–534. doi:10.1097/00002216-198907000-00116
Tajima, S., Tsuchiya, K., Horinouchi, Y., Ishizawa, K., Ikeda, Y., Kihira, Y., et al. (2010). Effect of Angiotensin II on Iron-Transporting Protein Expression and Subsequent Intracellular Labile Iron Concentration in Human Glomerular Endothelial Cells. Hypertens. Res. 33 (7), 713–721. doi:10.1038/hr.2010.63
Tanaka, S., and Tanaka, T. (2015). How to Supplement Iron in Patients with Renal Anemia. Nephron 131 (2), 138–144. doi:10.1159/000440773
Tanaka, T., Matsumoto, M., Inagi, R., Miyata, T., Kojima, I., Ohse, T., et al. (2005). Induction of Protective Genes by Cobalt Ameliorates Tubulointerstitial Injury in the Progressive Thy1 Nephritis. Kidney Int. 68 (6), 2714–2725. doi:10.1111/j.1523-1755.2005.00742.x
Tanaka, T., Miyata, T., Inagi, R., Kurokawa, K., Adler, S., Fujita, T., et al. (2003). Hypoxia-induced Apoptosis in Cultured Glomerular Endothelial Cells: Involvement of Mitochondrial Pathways. Kidney Int. 64 (6), 2020–2032. doi:10.1046/j.1523-1755.2003.00301.x
Tanaka, Y., Kume, S., Chin-Kanasaki, M., Araki, H., Araki, S.-i., Ugi, S., et al. (2016). Renoprotective Effect of DPP-4 Inhibitors against Free Fatty Acid-Bound Albumin-Induced Renal Proximal Tubular Cell Injury. Biochem. Biophysical Res. Commun. 470 (3), 539–545. doi:10.1016/j.bbrc.2016.01.109
Tang, D.-q., Wei, Y.-q., Yin, X.-x., Lu, Q., Hao, H.-h., Zhai, Y.-p., et al. (2011). In Vitro suppression of Quercetin on Hypertrophy and Extracellular Matrix Accumulation in Rat Glomerular Mesangial Cells Cultured by High Glucose. Fitoterapia 82 (6), 920–926. doi:10.1016/j.fitote.2011.05.001
Tang, P. M.-K., Zhang, Y.-Y., Hung, J. S.-C., Chung, J. Y.-F., Huang, X.-R., To, K.-F., et al. (2021). DPP4/CD32b/NF-κB Circuit: A Novel Druggable Target for Inhibiting CRP-Driven Diabetic Nephropathy. Mol. Ther. 29 (1), 365–375. doi:10.1016/j.ymthe.2020.08.017
Tang, R., Xu, J., Zhang, B., Liu, J., Liang, C., Hua, J., et al. (2020). Ferroptosis, Necroptosis, and Pyroptosis in Anticancer Immunity. J. Hematol. Oncol. 13 (1), 110. doi:10.1186/s13045-020-00946-7
Tarangelo, A., Magtanong, L., Bieging-Rolett, K. T., Li, Y., Ye, J., Attardi, L. D., et al. (2018). p53 Suppresses Metabolic Stress-Induced Ferroptosis in Cancer Cells. Cell Rep. 22 (3), 569–575. doi:10.1016/j.celrep.2017.12.077
Tbahriti, H. F., Kaddous, A., Bouchenak, M., and Mekki, K. (2013). Effect of Different Stages of Chronic Kidney Disease and Renal Replacement Therapies on Oxidant-Antioxidant Balance in Uremic Patients. Biochem. Res. Int. 2013, 1–6. doi:10.1155/2013/358985
Thévenod, F., and Wolff, N. A. (2016). Iron Transport in the Kidney: Implications for Physiology and Cadmium Nephrotoxicity. Metallomics 8 (1), 17–42. doi:10.1039/c5mt00215j
Tsuprykov, O., Ando, R., Reichetzeder, C., von Websky, K., Antonenko, V., Sharkovska, Y., et al. (2016). The Dipeptidyl Peptidase Inhibitor Linagliptin and the Angiotensin II Receptor Blocker Telmisartan Show Renal Benefit by Different Pathways in Rats with 5/6 Nephrectomy. Kidney Int. 89 (5), 1049–1061. doi:10.1016/j.kint.2016.01.016
Uchida, T., Oda, T., Matsubara, H., Watanabe, A., Takechi, H., Oshima, N., et al. (2017). Renoprotective Effects of a Dipeptidyl Peptidase 4 Inhibitor in a Mouse Model of Progressive Renal Fibrosis. Ren. Fail. 39 (1), 340–349. doi:10.1080/0886022X.2017.1279553
Ueda, N., Baliga, R., and Shah, S. V. (1996). Role of 'catalytic' Iron in an Animal Model of Minimal Change Nephrotic Syndrome. Kidney Int. 49 (2), 370–373. doi:10.1038/ki.1996.54
Upton, J. W., Kaiser, W. J., and Mocarski, E. S. (2010). Virus Inhibition of RIP3-dependent Necrosis. Cell Host & Microbe 7 (4), 302–313. doi:10.1016/j.chom.2010.03.006
van Raaij, S., van Swelm, R., Bouman, K., Cliteur, M., van den Heuvel, M. C., Pertijs, J., et al. (2018a). Publisher Correction: Tubular Iron Deposition and Iron Handling Proteins in Human Healthy Kidney and Chronic Kidney Disease. Sci. Rep. 8 (1), 13390. doi:10.1038/s41598-018-31457-8
van Raaij, S., van Swelm, R., Bouman, K., Cliteur, M., van den Heuvel, M. C., Pertijs, J., et al. (2018b). Tubular Iron Deposition and Iron Handling Proteins in Human Healthy Kidney and Chronic Kidney Disease. Sci. Rep. 8 (1), 9353. doi:10.1038/s41598-018-27107-8
van Swelm, R. P. L., Wetzels, J. F. M., and Swinkels, D. W. (2020). The Multifaceted Role of Iron in Renal Health and Disease. Nat. Rev. Nephrol. 16 (2), 77–98. doi:10.1038/s41581-019-0197-5
Vashisht, A. A., Zumbrennen, K. B., Huang, X., Powers, D. N., Durazo, A., Sun, D., et al. (2009). Control of Iron Homeostasis by an Iron-Regulated Ubiquitin Ligase. Science 326 (5953), 718–721. doi:10.1126/science.1176333
Vavrinec, P., Henning, R., Landheer, S., Wang, Y., Deelman, L., Dokkum, R., et al. (2014). Vildagliptin Restores Renal Myogenic Function and Attenuates Renal Sclerosis Independently of Effects on Blood Glucose or Proteinuria in Zucker Diabetic Fatty Rat. Curr. Vasc. Pharmacol. 12 (6), 836–844. doi:10.2174/15701611113116660151
Veuthey, T., Hoffmann, D., Vaidya, V. S., and Wessling-Resnick, M. (2014). Impaired Renal Function and Development in Belgrade Rats. Am. J. Physiology-Renal Physiol. 306 (3), F333–F343. doi:10.1152/ajprenal.00285.2013
Viswanathan, V. S., Ryan, M. J., Dhruv, H. D., Gill, S., Eichhoff, O. M., Seashore-Ludlow, B., et al. (2017). Dependency of a Therapy-Resistant State of Cancer Cells on a Lipid Peroxidase Pathway. Nature 547 (7664), 453–457. doi:10.1038/nature23007
Vyoral, D., and Jiri Petrak, P. (2017). Therapeutic Potential of Hepcidin − the Master Regulator of Iron Metabolism. Pharmacol. Res. 115, 242–254. doi:10.1016/j.phrs.2016.11.010
Wagner, B., Tan, C., Barnes, J. L., Ahuja, S., Davis, T. L., Gorin, Y., et al. (2012). Nephrogenic Systemic Fibrosis. Am. J. Pathol. 181 (6), 1941–1952. doi:10.1016/j.ajpath.2012.08.026
Wang, C., Blough, E., Arvapalli, R., Dai, X., Triest, W. E., Leidy, J. W., et al. (2015). Acetaminophen Attenuates Glomerulosclerosis in Obese Zucker Rats via Reactive Oxygen species/p38MAPK Signaling Pathways. Free Radic. Biol. Med. 81, 47–57. doi:10.1016/j.freeradbiomed.2015.01.008
Wang, W., Zhou, P.-h., Xu, C.-g., Zhou, X.-j., Hu, W., and Zhang, J. (2015). Baicalein Attenuates Renal Fibrosis by Inhibiting Inflammation via Down-Regulating NF-Κb and MAPK Signal Pathways. J. Mol. Hist. 46 (3), 283–290. doi:10.1007/s10735-015-9621-8
Wang, C., Blough, E. R., Arvapalli, R., Dai, X., Paturi, S., Manne, N., et al. (2013). Metabolic Syndrome-Induced Tubulointerstitial Injury: Role of Oxidative Stress and Preventive Effects of Acetaminophen. Free Radic. Biol. Med. 65, 1417–1426. doi:10.1016/j.freeradbiomed.2013.10.005
Wang, H., Nishiya, K., Ito, H., Hosokawa, T., Hashimoto, K., and Moriki, T. (2001). Iron Deposition in Renal Biopsy Specimens from Patients with Kidney Diseases. Am. J. Kidney Dis. 38 (5), 1038–1044. doi:10.1053/ajkd.2001.28593
Wang, J., Deng, B., Liu, Q., Huang, Y., Chen, W., Li, J., et al. (2020). Pyroptosis and Ferroptosis Induced by Mixed Lineage Kinase 3 (MLK3) Signaling in Cardiomyocytes Are Essential for Myocardial Fibrosis in Response to Pressure Overload. Cell Death Dis 11 (7), 574. doi:10.1038/s41419-020-02777-3
Wang, Y., Bi, R., Quan, F., Cao, Q., Lin, Y., Yue, C., et al. (2020). Ferroptosis Involves in Renal Tubular Cell Death in Diabetic Nephropathy. Eur. J. Pharmacol. 888, 173574. doi:10.1016/j.ejphar.2020.173574
Wang, Q.-L., Yuan, J.-L., Tao, Y.-Y., Zhang, Y., Liu, P., and Liu, C.-H. (2010). Fuzheng Huayu Recipe and Vitamin E Reverse Renal Interstitial Fibrosis through Counteracting TGF-Β1-Induced Epithelial-To-Mesenchymal Transition. J. ethnopharmacology 127 (3), 631–640. doi:10.1016/j.jep.2009.12.011
Wang, W., Zhou, P.-h., Xu, C.-g., Zhou, X.-j., Hu, W., and Zhang, J. (2016). Baicalein Ameliorates Renal Interstitial Fibrosis by Inducing Myofibroblast Apoptosisin Vivoandin Vitro. BJU Int. 118 (1), 145–152. doi:10.1111/bju.13219
Wang, Y., and Kanneganti, T.-D. (2021). From Pyroptosis, Apoptosis and Necroptosis to PANoptosis: A Mechanistic Compendium of Programmed Cell Death Pathways. Comput. Struct. Biotechnol. J. 19, 4641–4657. doi:10.1016/j.csbj.2021.07.038
Wang, Y., Pang, L., Zhang, Y., Lin, J., and Zhou, H. (2019). Fenofibrate Improved Interstitial Fibrosis of Renal Allograft through Inhibited Epithelial-Mesenchymal Transition Induced by Oxidative Stress. Oxidative Med. Cell Longevity 2019, 1–12. doi:10.1155/2019/8936856
Wang, Z., Ding, Y., Wang, X., Lu, S., Wang, C., He, C., et al. (2018). Pseudolaric Acid B Triggers Ferroptosis in Glioma Cells via Activation of Nox4 and Inhibition of xCT. Cancer Lett. 428, 21–33. doi:10.1016/j.canlet.2018.04.021
Wei, S., Xu, C., Zhang, Y., Shi, Z., Wu, M., and Yang, B. (2020). Ultrasound Assisted a Peroxisome Proliferator-Activated Receptor (PPAR)γ Agonist-Loaded Nanoparticle-Microbubble Complex to Attenuate Renal Interstitial Fibrosis. Int. J. nanomedicine 15, 7315–7327. doi:10.2147/IJN.S262052
Weiss, A., Spektor, L., A. Cohen, L., Magid Gold, I., Zhang, D.-L., Truman-Rosentsvit, M., et al. (2018). Orchestrated Regulation of Iron Trafficking Proteins in the Kidney during Iron Overload Facilitates Systemic Iron Retention. Plos One 13 (10), e0204471. doi:10.1371/journal.pone.0204471
Wen, Y., Pan, M. M., Lv, L. L., Tang, T. T., Zhou, L. T., Wang, B., et al. (2019). Artemisinin Attenuates Tubulointerstitial Inflammation and Fibrosis via the NF‐κB/NLRP3 Pathway in Rats with 5/6 Subtotal Nephrectomy. J. Cell Biochem 120 (3), 4291–4300. doi:10.1002/jcb.27714
Weng, C.-H., Li, Y.-J., Wu, H.-H., Liu, S.-H., Hsu, H.-H., Chen, Y.-C., et al. (2020). Interleukin-17A Induces Renal Fibrosis through the ERK and Smad Signaling Pathways. Biomed. Pharmacother. 123, 109741. doi:10.1016/j.biopha.2019.109741
Wenz, C., Faust, D., Linz, B., Turmann, C., Nikolova, T., and Dietrich, C. (2019). Cell-cell Contacts Protect against T-BuOOH-Induced Cellular Damage and Ferroptosis In Vitro. Arch. Toxicol. 93 (5), 1265–1279. doi:10.1007/s00204-019-02413-w
Wong, V. Y., Laping, N. J., Nelson, A. H., Contino, L. C., Olson, B. A., Gygielko, E., et al. (2001). Renoprotective Effects of Carvedilol in Hypertensive-Stroke Prone Rats May Involve Inhibition of TGFβ Expression. Br. J. Pharmacol. 134 (5), 977–984. doi:10.1038/sj.bjp.0704329
Wu, J., Minikes, A. M., Gao, M., Bian, H., Li, Y., Stockwell, B. R., et al. (2019). Intercellular Interaction Dictates Cancer Cell Ferroptosis via NF2-YAP Signalling. Nature 572 (7769), 402–406. doi:10.1038/s41586-019-1426-6
Wu, M., Xia, W., Jin, Q., Zhou, A., Wang, Q., Li, S., et al. (2021). Gasdermin E Deletion Attenuates Ureteral Obstruction- and 5/6 Nephrectomy-Induced Renal Fibrosis and Kidney Dysfunction. Front. Cell Dev. Biol. 9, 754134. doi:10.3389/fcell.2021.754134
Xiao, X., Du, C., Yan, Z., Shi, Y., Duan, H., and Ren, Y. (2017). Inhibition of Necroptosis Attenuates Kidney Inflammation and Interstitial Fibrosis Induced by Unilateral Ureteral Obstruction. Am. J. Nephrol. 46 (2), 131–138. doi:10.1159/000478746
Xie, Y., Hou, W., Song, X., Yu, Y., Huang, J., Sun, X., et al. (2016a). Ferroptosis: Process and Function. Cell Death Differ 23 (3), 369–379. doi:10.1038/cdd.2015.158
Xie, Y., Song, X., Sun, X., Huang, J., Zhong, M., Lotze, M. T., et al. (2016b). Identification of Baicalein as a Ferroptosis Inhibitor by Natural Product Library Screening. Biochem. Biophysical Res. Commun. 473 (4), 775–780. doi:10.1016/j.bbrc.2016.03.052
Xie, Y., Zhu, S., Song, X., Sun, X., Fan, Y., Liu, J., et al. (2017). The Tumor Suppressor P53 Limits Ferroptosis by Blocking DPP4 Activity. Cell Rep. 20 (7), 1692–1704. doi:10.1016/j.celrep.2017.07.055
Xu, Y., Li, X., Cheng, Y., Yang, M., and Wang, R. (2020). Inhibition of ACSL4 Attenuates Ferroptotic Damage after Pulmonary Ischemia‐reperfusion. FASEB j. 34 (12), 16262–16275. doi:10.1096/fj.202001758R
Yao, X., Zhang, Y., Hao, J., Duan, H.-Q., Zhao, C.-X., et al. (2019). Deferoxamine Promotes Recovery of Traumatic Spinal Cord Injury by Inhibiting Ferroptosis. Neural Regen. Res. 14 (3), 532–541. doi:10.4103/1673-5374.245480
Yagoda, N., von Rechenberg, M., Zaganjor, E., Bauer, A. J., Yang, W. S., Fridman, D. J., et al. (2007). RAS-RAF-MEK-dependent Oxidative Cell Death Involving Voltage-dependent Anion Channels. Nature 447 (7146), 865–869. doi:10.1038/nature05859
Yang, L., Besschetnova, T. Y., Brooks, C. R., Shah, J. V., and Bonventre, J. V. (2010). Epithelial Cell Cycle Arrest in G2/M Mediates Kidney Fibrosis after Injury. Nat. Med. 16 (5), 535–543. doi:10.1038/nm.2144
Yang M, M., Chen, P., Liu, J., Zhu, S., Kroemer, G., Klionsky, D. J., et al. (2019). Clockophagy Is a Novel Selective Autophagy Process Favoring Ferroptosis. Sci. Adv. 5 (7), eaaw2238. doi:10.1126/sciadv.aaw2238
Yang, W.-H., Ding, C.-K. C., Sun, T., Rupprecht, G., Lin, C.-C., Hsu, D., et al. (2019). The Hippo Pathway Effector TAZ Regulates Ferroptosis in Renal Cell Carcinoma. Cell Rep. 28 (10), 2501–2508. e2504. doi:10.1016/j.celrep.2019.07.107
Yang, Q., Wu, F.-r., Wang, J.-n., Gao, L., Jiang, L., Li, H.-D., et al. (2018). Nox4 in Renal Diseases: An Update. Free Radic. Biol. Med. 124, 466–472. doi:10.1016/j.freeradbiomed.2018.06.042
Yang, W. S., Kim, K. J., Gaschler, M. M., Patel, M., Shchepinov, M. S., and Stockwell, B. R. (2016). Peroxidation of Polyunsaturated Fatty Acids by Lipoxygenases Drives Ferroptosis. Proc. Natl. Acad. Sci. U.S.A. 113 (34), E4966–E4975. doi:10.1073/pnas.1603244113
Yang, W. S., SriRamaratnam, R., Welsch, M. E., Shimada, K., Skouta, R., Viswanathan, V. S., et al. (2014). Regulation of Ferroptotic Cancer Cell Death by GPX4. Cell 156 (1-2), 317–331. doi:10.1016/j.cell.2013.12.010
Yang, W. S., and Stockwell, B. R. (2016). Ferroptosis: Death by Lipid Peroxidation. Trends Cell Biol. 26 (3), 165–176. doi:10.1016/j.tcb.2015.10.014
Yang, W. S., and Stockwell, B. R. (2008). Synthetic Lethal Screening Identifies Compounds Activating Iron-dependent, Nonapoptotic Cell Death in Oncogenic-RAS-Harboring Cancer Cells. Chem. Biol. 15 (3), 234–245. doi:10.1016/j.chembiol.2008.02.010
Yuan, H., Li, X., Zhang, X., Kang, R., and Tang, D. (2016). CISD1 Inhibits Ferroptosis by protection against Mitochondrial Lipid Peroxidation. Biochem. Biophysical Res. Commun. 478 (2), 838–844. doi:10.1016/j.bbrc.2016.08.034
Zachara, B. A., Wlodarczyk, Z., Masztalerz, M., Adamowicz, A., Gromadzinska, J., and Wasowicz, W. (2004). Selenium Concentrations and Glutathione Peroxidase Activities in Blood of Patients before and after Allogenic Kidney Transplantation. Biol. Trace Elem. Res. 97 (1), 1–14. doi:10.1385/BTER:97:1:1
Zager, R. A., and Burkhart, K. (1997). Myoglobin Toxicity in Proximal Human Kidney Cells: Roles of Fe, Ca2+, H2O2, and Terminal Mitochondrial Electron Transport. Kidney Int. 51 (3), 728–738. doi:10.1038/ki.1997.104
Zeisberg, M., and Neilson, E. G. (2010). Mechanisms of Tubulointerstitial Fibrosis. J. Am. Soc. Nephrol. 21 (11), 1819–1834. doi:10.1681/ASN.2010080793
Zhang, B., Chen, X., Ru, F., Gan, Y., Li, B., Xia, W., et al. (2021). Liproxstatin-1 Attenuates Unilateral Ureteral Obstruction-Induced Renal Fibrosis by Inhibiting Renal Tubular Epithelial Cells Ferroptosis. Cell Death Dis 12 (9), 843. doi:10.1038/s41419-021-04137-1
Zhang, B., Liu, P., Zhou, Y., Chen, Z., He, Y., Mo, M., et al. (2019). Dihydroartemisinin Attenuates Renal Fibrosis through Regulation of Fibroblast Proliferation and Differentiation. Life Sci. 223, 29–37. doi:10.1016/j.lfs.2019.03.020
Zhang, D., Meyron-Holtz, E., and Rouault, T. A. (2007). Renal Iron Metabolism: Transferrin Iron Delivery and the Role of Iron Regulatory Proteins. J. Am. Soc. Nephrol. 18 (2), 401–406. doi:10.1681/ASN.2006080908
Zhang, Y., Li, H., Zhu, J., Wei, T., Peng, Y., Li, R., et al. (2017). Role of Artesunate in TGF-Β1-Induced Renal Tubular Epithelial-Mesenchymal Transdifferentiation in NRK-52E Cells. Mol. Med. Rep. 16 (6), 8891–8899. doi:10.3892/mmr.2017.7728
Zhang, Z., Yao, Z., Wang, L., Ding, H., Shao, J., Chen, A., et al. (2018). Activation of Ferritinophagy Is Required for the RNA-Binding Protein ELAVL1/HuR to Regulate Ferroptosis in Hepatic Stellate Cells. Autophagy 14 (12), 2083–2103. doi:10.1080/15548627.2018.1503146
Zhao, X., Wang, J., Tang, L., Li, P., Ru, J., and Bai, Y. (2021). Withaferin A Protects against Hyperuricemia Induced Kidney Injury and its Possible Mechanisms. Bioengineered 12 (1), 589–600. doi:10.1080/21655979.2021.1882761
Zhao, Y., Zhang, W., Jia, Q., Feng, Z., Guo, J., Han, X., et al. (2018). High Dose Vitamin E Attenuates Diabetic Nephropathy via Alleviation of Autophagic Stress. Front. Physiol. 9, 1939. doi:10.3389/fphys.2018.01939
Zhou, X., Bai, C., Sun, X., Gong, X., Yang, Y., Chen, C., et al. (2017). Puerarin Attenuates Renal Fibrosis by Reducing Oxidative Stress Induced-Epithelial Cell Apoptosis via MAPK Signal Pathways In Vivo and In Vitro. Ren. Fail. 39 (1), 423–431. doi:10.1080/0886022X.2017.1305409
Zhu, Y., Cui, H., Gan, H., Xia, Y., Wang, L., Wang, Y., et al. (2015). Necroptosis Mediated by Receptor Interaction Protein Kinase 1 and 3 Aggravates Chronic Kidney Injury of Subtotal Nephrectomised Rats. Biochem. biophysical Res. Commun. 461 (4), 575–581. doi:10.1016/j.bbrc.2015.03.164
Zhu, Y., Cui, H., Xia, Y., and Gan, H. (2016). RIPK3-Mediated Necroptosis and Apoptosis Contributes to Renal Tubular Cell Progressive Loss and Chronic Kidney Disease Progression in Rats. PloS one 11 (6), e0156729. doi:10.1371/journal.pone.0156729
Zou, Y., Li, H., Graham, E. T., Deik, A. A., Eaton, J. K., Wang, W., et al. (2020). Cytochrome P450 Oxidoreductase Contributes to Phospholipid Peroxidation in Ferroptosis. Nat. Chem. Biol. 16 (3), 302–309. doi:10.1038/s41589-020-0472-6
Keywords: renal fibrosis, ferroptosis, iron homeostasis, lipid peroxidation, programmed cell death
Citation: Zhang Y, Mou Y, Zhang J, Suo C, Zhou H, Gu M, Wang Z and Tan R (2022) Therapeutic Implications of Ferroptosis in Renal Fibrosis. Front. Mol. Biosci. 9:890766. doi: 10.3389/fmolb.2022.890766
Received: 06 March 2022; Accepted: 12 April 2022;
Published: 17 May 2022.
Edited by:
Yanqing Liu, Columbia University, United StatesReviewed by:
Zhenyi Su, Columbia University, United StatesQiaosi Tang, Calico Life Sciences LLC, United States
Yanchun Zhang, Icahn School of Medicine at Mount Sinai, United States
Copyright © 2022 Zhang, Mou, Zhang, Suo, Zhou, Gu, Wang and Tan. This is an open-access article distributed under the terms of the Creative Commons Attribution License (CC BY). The use, distribution or reproduction in other forums is permitted, provided the original author(s) and the copyright owner(s) are credited and that the original publication in this journal is cited, in accordance with accepted academic practice. No use, distribution or reproduction is permitted which does not comply with these terms.
*Correspondence: Ruoyun Tan, dGFucnVveXVuMTEyQHZpcC5zaW5hLmNvbQ==