- 1S-Inova Biotech, Pós-Graduação em Biotecnologia, Universidade Católica Dom Bosco, Campo Grande, Brazil
- 2Centro de Análises Proteômicas e Bioquímicas, Programa de Pós-Graduação em Ciências Genômicas e Biotecnologia, Universidade Católica de Brasília, Brasília, Brazil
- 3Integrative Plant Research Laboratory, Departamento de Botânica e Ecologia, Instituto de Biociências, Universidade Federal de MT, Cuiabá, Brazil
- 4Embrapa Arroz e Feijão, Laboratório de Biotecnologia, Goiânia, GO, Brazil
- 5Pós-graduação em Biologia Animal, Instituto de Biologia, Universidade de Brasília, Brasília, DF, Brazil
Antimicrobial peptides are small molecules, up to 10 kDa, present in all kingdoms of life, including in plants. Several studies report that these molecules have a broad spectrum of activity, including antibacterial, antifungal, antiviral, and insecticidal activity. Thus, they can be employed in agriculture as alternative tools for phytopathogen and pest control. However, the application of peptides in agriculture can present challenges, such as loss of activity due to degradation of these molecules, off-target effects, and others. In this context, nanotechnology can offer versatile structures, including metallic nanoparticles, liposomes, polymeric nanoparticles, nanofibers, and others, which might act both in protection and in release of AMPs. Several polymers and biomaterials can be employed for the development of nanostructures, such as inorganic metals, natural or synthetic lipids, synthetic and hybrid polymers, and others. This review addresses the versatility of NanoAMPs (Nanoparticles in association with antimicrobial peptides), and their potential applications in agribusiness, as an alternative for the control of phytopathogens in crops.
1 Introduction
Antimicrobial peptides (AMP) are small (up to 10 kDa) cationic molecules, with amphipathic structures composed of hydrophobic and positively charged domains (Bin Hafeez et al., 2021; Sarkar et al., 2021). These molecules have been found in all kingdoms of life. Contrary to what their name suggests, AMPs can present miscellaneous activities besides antimicrobial, including antiviral and insecticide (Huan et al., 2020; Erdem Buyukkiraz and Kesmen, 2021; Gera et al., 2021). AMPs can be employed to improve agriculture production, including diseases control. The biotic stress caused by pests and pathogens represents one of the main challenges to food security (Savary et al., 2019). Biotic stress can lead to up to 40% yield losses in our main crops, especially in food-deficit regions with fast-growing populations (Oerke, 2005; Savary et al., 2019). Additionally, this scenario can be aggravated by current climate changes, which increase microbial infection risks and foliar herbivory (Chaloner et al., 2021; Hamann et al., 2021).
The development of crop varieties that are more resistant to diseases and pest attacks represents a fundamental step toward achieving food security worldwide (Dhankher and Foyer, 2018). In this context, AMPs (either exogenously applied or transgene expressed) represent two approaches for improving plant resistance to phytopathogens (Li J. et al., 2021). Although AMPs present potent activity and easy metabolization without adversely affecting food quality, some restrictions limit their application in agriculture, including high production cost, safety concerns related to toxicity, low stability during transport, and easy hydrolysis by proteases (Wang et al., 2016; Huan et al., 2020).
In this context, nanobiotechnology arises as an interface between nanotechnology and biotechnology (Amin et al., 2011). In this interdisciplinary research field, tools on the nanometer scale, such as nanodevices, nanoparticles, and other nanostructured systems can be employed in the development of biotech products and applied to solving problems involving biological sciences and their concerns, e.g., biocatalysis, biomedicine, and agriculture (Barabadi, 2017; Thiruvengadam et al., 2018; Worrall et al., 2018). Nanomaterials used as these tools can be made of inorganic metals, liposomes, polymers, nanofibers, and others (Duhan et al., 2017). Although nanoparticles (nanospheres or nanocapsules) are the most popular nanostructured systems, other types are also very useful in biotechnology, such as dendrimers, nanogels, and liposomes (Jiang et al., 2007).
All of these nanostructures, developed by several materials, can be employed as drug delivery systems (DDS) (Vega-Vasquez et al., 2020) due to their properties of harboring and/or attaching molecules of interest that will act in specific cells, tissues, or organs in a controlled release mechanism (Allen and Cullis, 2004; Jiang et al., 2007). This can makes nanomaterials very suitable vehicles for the gradual release of a wide range of molecules, including secondary metabolites, nucleic acids, proteins, and peptides. This release can improve the delivery at the desired target site, by addressing cells in a spatiotemporal manner (Martínez-Ballesta et al., 2018).
Taking this into account, nanotechnology has been used in the last few years to associate nanoscale delivery systems with AMPs (NanoAMPs) to stabilize these molecules. When compared to isolated AMPs, which have lower bioavailability and are usually unstable in the environment, the NanoAMPs can bypass this disadvantages and increase the biological effect on the target (Biswaro et al., 2018). NanoAMPs can also promote a controlled release of entrapped AMPs, therefore keeping a longer time of action, improving half-life time, decreasing potential toxicity, and promoting the biological activity in constant doses (Tan et al., 2021). Moreover, this association may be useful for enhancing the effectiveness of either AMPs or nanostructured systems themselves, or even boosting their activities synergistically through combinatorial formulations (León-Buitimea et al., 2020).
Among the main advantages of using NanoAMPs over free AMPs, it is possible to point out the AMP side-effects decrease, as lower bioavailability and the environment instability in the, lower administration frequency, a lower dose needed, constant levels of AMPs released, bioavailability enhanced by defense against degradation, maximization of biological activity, in addition to applying to a wide range of molecules (Patra et al., 2018). In this context, NanoAMPs can be an interesting alternative to bypass plant biotic stress and improve agricultural production.
2 A tool to achieve food security: The potential of antimicrobial peptides in agriculture
AMPs present several beneficial characteristics, including activity against several phytopathogens (fungi, bacteria, virus) and insects (Mulinari et al., 2007; Pinto et al., 2012); the capacity to generate direct and durable plant resistance; and small gene nature that facilitates stacking the coding sequence of multiple AMPs on single expression vectors (Islam et al., 2021). Besides, AMPs natural or synthetic also present ease of manipulation and optimization by computational approaches (in silico design). These approaches can includes, search by homology modeling, molecular dynamics and protein docking. The advantages of computational in silico methods include their low cost, faster procedure speed, simple process (Porto et al., 2018; Costa et al., 2020; Hashemi et al., 2021; Delaunay and Ha-Duong, 2022). These approaches can be employed i.e. to generation of derivatives with improved features, and a low metabolic cost of production (da Cunha et al., 2017; Porto et al., 2018), which reduces potential detrimental impacts on plant growth and productivity associated with the activation of the plant defense responses (Keymanesh et al., 2009; Campos et al., 2018; Sarkar et al., 2021).
Moreover, AMPs can be described as an eco-friendly and healthier alternative for controlling pest and pathogens (Li P. et al., 2021). By this way, several studies developed transgenic plants expressing AMPs, including rice, wheat, potato, tomato, banana and soybean to improve the resistance against biotic and abiotic stress (Supplementary Table S1). In summary these studies highlight that AMPs expression presents high potential to increase resilience to pests, pathogens and abiotic stress. Additionally, plants expressing these peptides can present a decrease in demand of chemical pesticides, that can cause risks to the environment and consumers’ health (Keymanesh et al., 2009).
In this context, are important highlights the limitations to AMP gene expression in plants and the challenges faced in development and commercialization of transgenic plant lines (Turnbull et al., 2021; Bakare et al., 2022; Sharma et al., 2022). Although plants are able to express antimicrobial peptides, some pitfalls can be faced, as the production of AMP in all plant structures, difference among expression and difficult to produce active plant AMPs in large quantities due the differences in plant cultivation, and the endogenous AMPs degradation by plant proteases (Bakare et al., 2022).
Furthermore, AMPs also can be applied in order to control plant diseases through non-transgenic methods, such as exogenous applications (i.e. spraying with or immersion in peptide solutions) and food coating (Wang et al., 2018a). Exogenous application of peptides PAF56 (GHRKKWFW) and cecropin A-melittin hybrid peptide BP21 (Ac-FKLFKKILKVL-NH2) in citrus can control post-harvest green mold, one of the main postharvest diseases, and blue mold and sour rot, caused respectively by Penicillium digitatum, Penicillium italicum, and Geotrichum candidum (Wang et al., 2018a; Wang et al., 2018b). Additionally, peptide O3TR and its derived lipopeptide C12O3TR were also employed to protect freshly harvested orange fruit against P. digitatum (Li et al., 2019).
Despite several studies indicating that AMPs stand out as a barrier to ward of phytopathogen and pest attacks, only a few studies have demonstrated positive applications in field conditions. This can be explained by challenges associated with upscaling production, or with stability of the peptides. Regarding AMP-derived plant resistance to biotic stress, few studies have moved from the laboratory to the most applicable field conditions, thus hampering our ability to use these peptides directly to protect agroecosystems (Huan et al., 2020). This situation may be explained by challenges usually associated with the production or activity of AMPs, including a reduction in defensive activity due to degradation of these molecules when in contact with microbial proteases or enzymes present in the digestive system of herbivores or due the environmental conditions such as sunlight, temperature and others, off-target effects leading to cytotoxicity to the consumer (in case of the transgenic plant) and high production costs for exogenous applications (Keymanesh et al., 2009; Biswaro et al., 2018; Huan et al., 2020). In this context, nanotechnology is now arising as a revolutionary and versatile alternative by which to optimize the biological and chemical properties of AMPs, and this may finally bring the benefits of these peptides to consumers.
3 Advantages of nanotechnology for antimicrobial peptide activity
Nanotechnology can be a promising alternative for the storage and administration of antimicrobial peptides, once nanostructures can protect AMPs from proteolysis and unwanted interactions and can promote a controlled, long-lasting, and targeted release of the peptide (Sandreschi et al., 2016). Additionally, these nanostructures have the potential to protect the AMP against environmental conditions such as sunlight, and variation in temperature, and others (Badea et al., 2015; López-Vargas et al., 2018; Felippim et al., 2020). Thus, NanoAMPs have been developed in recent years based on the association of nanoscale delivery systems with AMPs (Table 1).
These studies focus on human or animal health to stabilize these molecules compared to isolated AMPs, which have lower bioavailability and are usually unstable in the environment when used alone, thus reducing their biological effect on the target (Biswaro et al., 2018). Besides, NanoAMPs can promote a controlled release of entrapped AMPs, therefore maintaining a longer time of action, improving half-life time, decreasing potential toxicity and promoting biological activity in constant doses (Tan et al., 2021). Moreover, this association may be useful for enhancing the effectiveness of either AMPs or nanostructured systems themselves, or even boosting both their activities synergistically through combinatorial formulations (León-Buitimea et al., 2020).
In general, nanomaterials can be functionalized with AMPs, promoting the generation of NanoAMPs to bypass some challenges faced in AMP applications in agriculture including an increase in AMP stability, target activity, release of entrapped AMPs, biological activity and decreasing the potential toxicity of AMPs’ effects on the environment. Thus, NanoAMPs present great potential in agribusiness, considering their advantages and wide range of applications (Patra et al., 2018).
4 Nanoparticles in association with antimicrobial peptides: Promising applications of associating antimicrobial peptides with nanostructured systems
The development of nanometric structures complexed with bioactive molecules has shown a high impact in several areas, including agriculture (Duhan et al., 2017). This approach enables the controlled, efficient, and safe release of fertilizers, pesticides and herbicides in several plant crops (Santana et al., 2020; Zhang et al., 2020). Besides, nanotechnology employment in agriculture, has shown a role in increasing the abiotic stresses tolerance plants, including drought, heat, salinity and ion toxicity, oxidative stress and others. Studies that employed cerium oxide (CeO2) nanoparticles, called nanoceria showed the potent antioxidant properties that can decrease the drought-induced oxidative stress, by catalytic scavenging reactive oxygen species (ROS), in model plants well as in plants of interest economic (Wu et al., 2017; Djanaguiraman et al., 2018). Besides, the combination of nanoparticles with peptides can also be used for generating nanosensors capable of early stress detection (Zhang et al., 2011; Giraldo et al., 2019).
The wide-ranging potential of nanobiotechnology applications in agriculture can be related to the wide nanomaterials range employed in the nanoparticles development (Li J. et al., 2021). The nanomaterials differ in size, shape, composition, and physicochemical properties, and may vary in surface area and the reactivity of the molecule. These characteristics should promote an improvement in the solubility and half-life of the molecule, including AMPs, and a decrease in toxicity due to their ability to target the specific site of action (Reis et al., 2006; Bawa, 2009; Zhang et al., 2013). Additionally, different materials have been used for NanoAMP preparation, such as inorganic metals (Min et al., 2009; Goswami et al., 2010), liposomes (Luo et al., 2015), polymers (Rafiee et al., 2014; Kleine-Brueggeney et al., 2015) and nanofibers (Lahiani et al., 2015).
Metallic nanoparticles can be used as antimicrobial agents or nanocarriers for active substances. Among metallic nanoparticles, silver is known for its antimicrobial activity and is considered the most promising nanomaterial, mainly due to its bactericidal properties and adaptability to different substrates (Cho et al., 2005; Sharma et al., 2009). Moreover, silver has gained popularity due “green synthesis” production. These approaches involves metallic nanoparticles synthesis using bioactive agents including plants, bacteria and fungi to the bio reduction of metal ions in their elemental form, that presents size range 1–100 nm (Rafique et al., 2017; Rodrigues et al., 2021; Patil et al., 2022). The green synthesis depends on the employment of water solvent for nanoparticles yield. Bioreduction and biosorption are essential routs for that synthesis. Bioreduction can be described as the process in which metal ions are chemically reduced into their stable forms; and the biosorption process involves the binding of metal ions (generated by bioreduction) on the surface of bioactive agent (Gobalakrishnan et al., 2021; Kaur and Sidhu, 2021). The use of natural precursors for the biosynthesis of nanoparticles has some advantages when compared to conventional methods of synthesis, such as biocompatibility and low production costs, since these synthesis routes do not use toxic solvents or chemical precursors (El-Sherbiny and Salih, 2018; Gour and Jain, 2019). Other metal nanoparticles include copper, titanium dioxide, and gold, which are mostly used for the incorporation of fertilizers, with little research into disease management (Sadeghi et al., 2017).
NanoAMPs, developed using silver nanoparticles and AMPs, in general aim to deliver NanoAMPs to intracellular target sites and show lower cytotoxicity; additionally, enhanced AMP activity was observed in some studies (Ruden et al., 2009; Gakiya-Teruya et al., 2020; Gao et al., 2020; Zharkova et al., 2021). Concerning functionalization, the association of five different amphiphilic α-helical AMPs (PGLa, MSI-103, MAP, BP100, and TP10) with gold nanoparticles by attachment of the peptides to the gold core, exclusively via the N-terminal Cys, aimed to increase the stability of peptides against enzymes such as trypsin. This resulted in an improvement in the AMPs’ lifetime, antimicrobial activity against Gram-negative and positive bacteria, and stability towards trypsin action while AMPs maintained their conformational flexibility (Wadhwani et al., 2017). Additionally in biomedical studies, a PEG hydrogel was recently co-loaded with gold nanorods encapsulating the AMP named IK8. These nanoparticles ensuring IK8 proteolysis protection and release control. Consequently bactericidal activity was enhanced through photothermal activation based on laser irradiation (Moorcroft et al., 2020).
Liposomes are another nanostructure commonly applied in the protection of molecules. These nanostructures are spherical vesicles with an amphiphilic lipid bilayer membrane structure with mean diameters from nanometer to micrometer. Their properties, functionalities and stability depend on factors such as temperature, pH, ionic strength, concentration, and composition of phospholipids and the properties of the encapsulated molecule (Jesorka and Orwar, 2008). Liposomes are the most used drug delivery system and can be obtained from natural or synthetic lipids; an example is a phosphatidylcholine, which is one of the lipids most used in liposome formulation (Pinilla et al., 2021).
The application of liposomes is widely reported in several biomedical studies (Makowski et al., 2019; Wang et al., 2021). Additionally, in biomedical studies, a PEG hydrogel was recently co-loaded with gold nanorods encapsulating the AMP named IK8. These nanoparticles ensuring IK8 proteolysis protection and release control. Consequently bactericidal activity was enhanced through photothermal activation based on laser irradiation (Moorcroft et al., 2020). The usefulness of cubosomes (also called lipidic inverse bicontinuous cubic phase nanoparticles) as encapsulation systems for the delivery of AMPs has been validated (Meikle et al., 2017; Meikle et al., 2021). On the other hand, the role of liposomes in agriculture has been related to cell membrane model systems (Taylor et al., 2005; Isozumi et al., 2021), food preservation in the post-harvest process or industrial processing, and the protection of substances such as enzymes, vitamins, and antimicrobials, to improve food quality (Mozafari, 2005; da Silva Malheiros et al., 2010; Pinilla et al., 2021).
In this context, the plant application of NanoAMPs faces some challenges, including physical structures present in leaf, such as hair and cuticular wax which can be barriers to this approach. Nevertheless, nanoparticles obstruction depends on the physical characteristics such as particle size, epidermal structure, leaf area, and plant growth stage. Once the lipophilicity of leaf wax can promote the adsorption of hydrophobic or lipophilic nanoparticles, the nanoparticle material choice can be decisive to bypass such challenge (López-Vargas et al., 2018; Su et al., 2019; Hong et al., 2021).
Polymers are the main nanoparticles constituents used in drug delivery systems. Polymeric nanoparticles are formed by a polymeric matrix and can retain the molecule internally or adsorb to the polymeric structure (Vauthier and Bouchemal, 2009; Brandelli, 2012). They are more robust and stable particles than liposomes because they are held together by covalent bonds. Thus, several other polymeric nanoparticles have been used as vehicles for diverse AMPs with different applications, in several areas, including the health area. In this context, the influence of porosity and surface charge of mesoporous silica nanoparticles (MSN) on loading and release of AMP LL-37 was investigated and results showed that anionic mesoporous silica particles incorporated considerable amounts of LL-37 (cationic AMP). In addition, these particles protect LL-37 from degradation by proteases (Braun et al., 2016).
Nanofibers are one-dimensional nanomaterials produced with a wide range of natural, synthetic and hybrid polymers. As the name suggests, these are fiber-shaped nanomaterials with several unique properties such as nanoporosity, high surface area/volume and high mass transport properties (Meraz-Dávila et al., 2021). Factors such as temperature, viscosity, solution surface tension and electric field strength are important to nanostructures, but to nanofibers has larger importance, once define the quality and characteristics of these nanostructures (Deitzel et al., 2001).
Due to their property of high surface area to volume ratio, nanofibers have a great potential for carrier and release antimicrobials peptides. In addition, different modes of carrying molecules can be obtained; antimicrobials peptides can be loaded onto the surface of the nanofiber by adsorption, there may be adsorption of charged nanoparticles with the molecule on the surface of the fibers, or a layer-by-layer assembly on the cover allows some nanometers to deposit polyanions such as heparin (Yoo et al., 2009). Encapsulated synthetic AMP HHC-8 and MM-10 in poly (ε-caprolactone) nanoparticles (PCL-NPs), which triggered minimal degradation and sustained release of AMPs and improved their antimicrobial activity against mycobacteria, ensured the synergistic effect of NanoAMPs (Sharma et al., 2021). Thus, nanofibers present a strong potential for distributing antimicrobials in food systems.
5 Potential applications of nanoparticles in association with antimicrobial peptides in agriculture
The challenges faced by food production are distinct in pre-harvest and post-harvest phases. In pre-harvest phase, food production can be negatively affected by several phytopathogens. These organisms can include fungi, bacteria, viruses, parasites, and insects, which can cause the development of several diseases and promote several losses in food production. In the post-harvest phase, one of the most challenges faced is the losses caused by mold infections. In both scenarios, NanoAMPs can be a promising approach and offer different strategies/combinations to mitigate these problems. During pre-harvest, different NanoAMPs can be employed for disease control, and in post-harvest, the NanoAMPs can promote an increase in the shelf life of food, well as protection against mold infections (Brandelli, 2012; Duhan et al., 2017; Sadeghi et al., 2017; Biswaro et al., 2018; Ristaino et al., 2021; Rizzo et al., 2021; Singh et al., 2021).
In this context, some NanoAMPs examples applied in agriculture can be highlighted (Figure 1), including a NanoAMP called P-13@AgNPs, achieved through the rational development of an antimicrobial peptide (P-13) and its association with silver nanoparticle (AgNP), which results in better activity against Gram-negative and Gram-positive bacteria (Gao et al., 2020), including activity toward Bacillus pumilus, which can cause ginger rhizome rot disease (Peng et al., 2013), demonstrating the potential application of this NanoAMP in plant disease control.
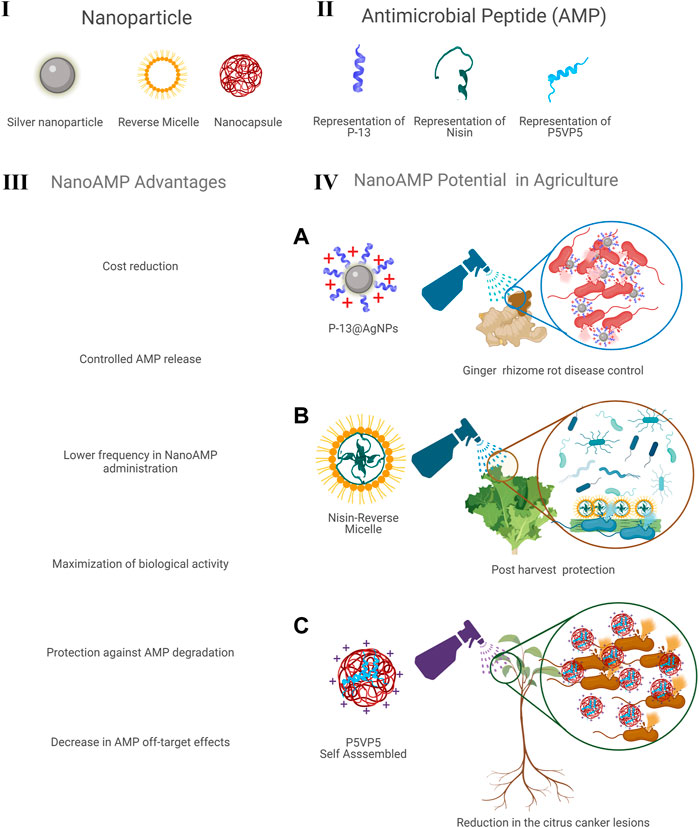
FIGURE 1. NanoAMPs description in which, nanoparticles (I) were associated with antimicrobial peptides (II) showing advantages (III) and potential applications in agriculture (IV). (A) Representation of P13@AgNPs, which presents potential to control ginger rhizome rot disease caused by Bacillus pumilus. (B) Representation of nisin peptide combined with reverse micelles that shows potential for post-harvest food protection. (C) Representation of self-assembled P5VP5 peptide that can be used to decrease citrus canker lesions caused by Xanthomonas axonopodis pv. citri. Figure developed with support of the Biorender (Biorender.com).
Additionally, NanoAMPs were developed using nisin (an important peptide in the food industry) in association with several lipid-based nanostructures, including liposomes, nanoemulsions, solid lipid nanoparticles (SLNs), and nanostructured lipid carriers (NLCs), have been employed in food conservation, and present potential for use in agriculture, specifically in post-harvest (Bahrami et al., 2019). These NanoAMPs can be employed in food preservation, since nisin presents activity against bacteria such as Listeria monocytogenes and Lactobacillus plantarum (Prombutara et al., 2012). However, a NanoAMP based on nisin associated with a nanoemulsion (reverse micelles through W/O microemulsions) presented antimicrobial activity, during an in vitro assay in lettuce fresh leaves (Chatzidaki et al., 2018), suggesting that these NanoAMPs can be employed in post-harvest.
Another interesting example of NanoAMPs employed in agriculture is the NanoAMP development called P5VP5. This peptide was engineered as a unique symmetrical cationic peptide
6 Challenges and perspectives
The employment of NanoAMPs is still strongly focused on the biomedical field (Mohid and Bhunia, 2020), especially for therapeutic applications (Teixeira et al., 2020; Gera et al., 2021), and studies concerning applications in agribusiness are still scarce. Although several tools are already available for the development of this area, many applications remain unexplored, making more studies and research necessary to develop solid solutions for problems faced in agriculture as well as in livestock. Some researchers (Perez-de-Luque and Rubiales, 2009) have already proposed the use of nanotechnology strategies for parasitic plant control, suggesting the nanoencapsulation of herbicides to be used against parasitic weeds, and noticing the potential of nanoparticles as magic bullets for the delivery of herbicides, chemicals, nucleic acids, enzymes and even AMPs targeting specific plant tissues for the treatment of viruses and microbial parasites. Additionally, some studies evaluate the environmental impacts of nanomaterials and conclude that most of nanoparticles are unlikely to have adverse effects on human health or on environment (McClements and Xiao, 2017; Lead et al., 2018; Sohal et al., 2018). Furthermore, the development and deployment of nanoAMPs can be employed in protected agriculture, i.e in glasshouses (Sarika et al., 2012).
Thus, NanoAMPs certainly have a great potential in agribusiness, considering the wide variety of applications described here and the various benefits, mainly in the improvement of productivity and safety against microbial contaminants. However, NanoAMPs remain underdeveloped for agribusiness applications which development currently underway and no commercial NanoAMPs products available in the sector.
Finally, we must consider that substantial work has already been done toward using free AMPs in agriculture as discussed above, and the use of either AMPs for antimicrobial activity or food preservation (Keymanesh et al., 2009) also provides a complete review of many. This paves the way for intensive research dedicated to improving the supply of nutrients, pesticides, herbicides and food preservatives through nanotechnology approaches, using what has already been tested through free AMPs.
Author contributions
MM drafted the manuscript, wrote the manuscript and designed Figure 1 and Table 1. TR, MC and GP wrote the manuscript. SD supervised the written manuscript. OF drafted the manuscript and supervised the written manuscript. All authors provided critical feedback and helped shape the research, analysis, and manuscript. All authors have read and approved the manuscript.
Funding
This work was supported by Fundação de Apoio ao Desenvolvimento do Ensino, Ciência e Tecnologia do Estado do Mato Grosso do Sul—FUNDECT, Fundação de Apoio à Pesquisa do Distrsito Federal—FAPDF, Conselho Nacional de Desenvolvimento Cientifico e Tecnológico—CNPq, and Coordenação de Aperfeiçoamento de Pessoal de Nível Superior—CAPES.
Conflict of interest
The authors declare that the research was conducted in the absence of any commercial or financial relationships that could be construed as a potential conflict of interest.
Publisher’s note
All claims expressed in this article are solely those of the authors and do not necessarily represent those of their affiliated organizations, or those of the publisher, the editors and the reviewers. Any product that may be evaluated in this article, or claim that may be made by its manufacturer, is not guaranteed or endorsed by the publisher.
Supplementary material
The Supplementary Material for this article can be found online at: https://www.frontiersin.org/articles/10.3389/fmolb.2022.890654/full#supplementary-material
References
Allen, T. M., and Cullis, P. R. (2004). Drug delivery systems: entering the mainstream. Science 303 (5665), 1818–1822. doi:10.1126/science.1095833
Almasia, N. I., Bazzini, A. A., Hopp, H. E., and Vazquez-Rovere, C. (2008). Overexpression of snakin-1 gene enhances resistance to Rhizoctonia solani and Erwinia carotovora in transgenic potato plants. Mol. Plant Pathol. 9 (3), 329–338. doi:10.1111/j.1364-3703.2008.00469.x
Amin, R., Hwang, S., and Park, S. H. (2011). Nanobiotechnology: An interface between nanotechnology and biotechnology. Nano 6 (02), 101–111. doi:10.1142/s1793292011002548
Badea, G., Lăcătuşu, I., Badea, N., Ott, C., and Meghea, A. (2015). Use of various vegetable oils in designing photoprotective nanostructured formulations for UV protection and antioxidant activity. Industrial Crops Prod. 67, 18–24. doi:10.1016/j.indcrop.2014.12.049
Bahrami, A., Delshadi, R., Jafari, S. M., and Williams, L. (2019). Nanoencapsulated nisin: An engineered natural antimicrobial system for the food industry. Trends Food Sci. Technol. 94, 20–31. doi:10.1016/j.tifs.2019.10.002
Bakare, O. O., Gokul, A., Fadaka, A. O., Wu, R., Niekerk, L.-A., Barker, A. M., et al. (2022). Plant antimicrobial peptides (PAMPs): Features, applications, production, expression, and challenges. Molecules 27 (12), 3703. doi:10.3390/molecules27123703
Barabadi, H. (2017). Nanobiotechnology: A promising scope of gold biotechnology. Cell. Mol. Biol. 63 (12), 3–4. doi:10.14715/cmb/2017.63.12.2
Bártová, V., Bárta, J., and Jarošová, M. (2019). Antifungal and antimicrobial proteins and peptides of potato (Solanum tuberosum L.) tubers and their applications. Appl. Microbiol. Biotechnol. 103 (14), 5533–5547. doi:10.1007/s00253-019-09887-9
Bawa, R. (2009). NanoBiotech 2008: Exploring global advances in nanomedicine. Nanomedicine 5 (1), 5–7. doi:10.1016/j.nano.2009.01.004
Bin Hafeez, A., Jiang, X., Bergen, P. J., and Zhu, Y. (2021). Antimicrobial peptides: An update on classifications and databases. Int. J. Mol. Sci. 22 (21), 11691. doi:10.3390/ijms222111691
Biswaro, L. S., da Costa Sousa, M. G., Rezende, T., Dias, S. C., and Franco, O. L. (2018). Antimicrobial peptides and nanotechnology, recent advances and challenges. Front. Microbiol. 9, 855. doi:10.3389/fmicb.2018.00855
Brandelli, A. (2012). Nanostructures as promising tools for delivery of antimicrobial peptides. Mini Rev. Med. Chem. 12 (8), 731–741. doi:10.2174/138955712801264774
Braun, K., Pochert, A., Linden, M., Davoudi, M., Schmidtchen, A., Nordstrom, R., et al. (2016). Membrane interactions of mesoporous silica nanoparticles as carriers of antimicrobial peptides. J. Colloid Interface Sci. 475, 161–170. doi:10.1016/j.jcis.2016.05.002
Campos, M. L., de Souza, C. M., de Oliveira, K. B. S., Dias, S. C., and Luiz Franco, O. L. (2018). The role of antimicrobial peptides in plant immunity. J. Exp. Bot. 69, 4997–5011. doi:10.1093/jxb/ery294
Chaloner, T. M., Gurr, S. J., and Bebber, D. P. (2021). Plant pathogen infection risk tracks global crop yields under climate change. Nat. Clim. Chang. 11 (8), 710–715. doi:10.1038/s41558-021-01104-8
Chatzidaki, M. D., Papadimitriou, K., Alexandraki, V., Balkiza, F., Georgalaki, M., Papadimitriou, V., et al. (2018). Reverse micelles as nanocarriers of nisin against foodborne pathogens. Food Chem. 255, 97–103. doi:10.1016/j.foodchem.2018.02.053
Cho, K.-H., Park, J.-E., Osaka, T., and Park, S.-G. (2005). The study of antimicrobial activity and preservative effects of nanosilver ingredient. Electrochimica Acta 51 (5), 956–960. doi:10.1016/j.electacta.2005.04.071
Costa, L. S., Pires, Á. S., Damaceno, N. B., Rigueiras, P. O., Maximiano, M. R., Franco, O. L., et al. (2020). In silico characterization of class II plant defensins from Arabidopsis thaliana. Phytochemistry 179, 112511. doi:10.1016/j.phytochem.2020.112511
da Cunha, N. B., Cobacho, N. B., Viana, J. F., Lima, L. A., Sampaio, K. B., Dohms, S. S., et al. (2017). The next generation of antimicrobial peptides (AMPs) as molecular therapeutic tools for the treatment of diseases with social and economic impacts. Drug Discov. Today 22 (2), 234–248. doi:10.1016/j.drudis.2016.10.017
da Silva Malheiros, P., Daroit, D. J., and Brandelli, A. (2010). Food applications of liposome-encapsulated antimicrobial peptides. Trends Food Sci. Technol. 21 (6), 284–292. doi:10.1016/j.tifs.2010.03.003
Deitzel, J. M., Kleinmeyer, J., Harris, D., and Tan, N. B. (2001). The effect of processing variables on the morphology of electrospun nanofibers and textiles. Polymer 42 (1), 261–272. doi:10.1016/s0032-3861(00)00250-0
Delaunay, M., and Ha-Duong, T. (2022). “Computational tools and strategies to develop peptide-based inhibitors of protein-protein interactions,” in Computational peptide science (Springer), 205–230.
Dhankher, O. P., and Foyer, C. H. (2018). Climate resilient crops for improving global food security and safety. Wiley Online Library.
Djanaguiraman, M., Nair, R., Giraldo, J. P., and Prasad, P. V. V. (2018). Cerium oxide nanoparticles decrease drought-induced oxidative damage in sorghum leading to higher photosynthesis and grain yield. ACS omega 3 (10), 14406–14416. doi:10.1021/acsomega.8b01894
Duhan, J. S., Kumar, R., Kumar, N., Kaur, P., Nehra, K., Duhan, S., et al. (2017). Nanotechnology: The new perspective in precision agriculture. Biotechnol. Rep. 15, 11–23. doi:10.1016/j.btre.2017.03.002
El-Sherbiny, I. M., and Salih, E. (2018). “Green synthesis of metallic nanoparticles using biopolymers and plant extracts,” in Green metal nanoparticles: Synthesis, characterization and their applications, 293–319.
Erdem Buyukkiraz, M., and Kesmen, Z. (2021). Antimicrobial peptides (AMPs): A promising class of antimicrobial compounds. J. Appl. Microbiol. 132, 1573–1596. doi:10.1111/jam.15314
Felippim, E. C., Marcato, P. D., and Maia Campos, P. M. B. G. (2020). Development of photoprotective formulations containing nanostructured lipid carriers: Sun protection factor, physical-mechanical and sensorial properties. AAPS PharmSciTech 21 (8), 311. doi:10.1208/s12249-020-01858-y
Gakiya-Teruya, M., Palomino-Marcelo, L., Pierce, S., Angeles-Boza, A. M., Krishna, V., Rodriguez-Reyes, J. C. F., et al. (2020). Enhanced antimicrobial activity of silver nanoparticles conjugated with synthetic peptide by click chemistry. J. Nanopart. Res. 22 (4), 90. doi:10.1007/s11051-020-04799-6
Gao, J., Na, H., Zhong, R., Yuan, M., Guo, J., Zhao, L., et al. (2020). One step synthesis of antimicrobial peptide protected silver nanoparticles: The core-shell mutual enhancement of antibacterial activity. Colloids Surf. B Biointerfaces 186, 110704. doi:10.1016/j.colsurfb.2019.110704
Gera, S., Kankuri, E., and Kogermann, K. (2021). Antimicrobial peptides - unleashing their therapeutic potential using nanotechnology. Pharmacol. Ther. 232. 107990. doi:10.1016/j.pharmthera.2021.107990
Ghag, S. B., Shekhawat, U. K. S., and Ganapathi, T. R. (2014). Transgenic banana plants expressing a Stellaria media defensin gene (Sm-AMP-D1) demonstrate improved resistance to Fusarium oxysporum. Plant Cell Tissue Organ Cult. 119 (2), 247–255. doi:10.1007/s11240-014-0529-x
Giraldo, J. P., Wu, H., Newkirk, G. M., and Kruss, S. (2019). Nanobiotechnology approaches for engineering smart plant sensors. Nat. Nanotechnol. 14 (6), 541–553. doi:10.1038/s41565-019-0470-6
Gobalakrishnan, S., Chidhambaram, N., and Chavali, M. (2021). “Role of greener syntheses at the nanoscale,” in Handbook of greener synthesis of nanomaterials and compounds (Elsevier), 107–134.
Goswami, A., Roy, I., Sengupta, S., and Debnath, N. (2010). Novel applications of solid and liquid formulations of nanoparticles against insect pests and pathogens. Thin solid films 519 (3), 1252–1257. doi:10.1016/j.tsf.2010.08.079
Gour, A., and Jain, N. K. (2019). Advances in green synthesis of nanoparticles. Artif. Cells Nanomed. Biotechnol. 47 (1), 844–851. doi:10.1080/21691401.2019.1577878
Hamann, E., Blevins, C., Franks, S. J., Jameel, M. I., and Anderson, J. T. (2021). Climate change alters plant–herbivore interactions. New Phytol. 229 (4), 1894–1910. doi:10.1111/nph.17036
Hashemi, Z. S., Zarei, M., Fath, M. K., Ganji, M., Farahani, M. S., Afsharnouri, F., et al. (2021). In silico approaches for the design and optimization of interfering peptides against protein–protein interactions. Front. Mol. Biosci. 8, 669431. doi:10.3389/fmolb.2021.669431
Hong, J., Wang, C., Wagner, D. C., Gardea-Torresdey, J. L., He, F., Rico, C. M., et al. (2021). Foliar application of nanoparticles: Mechanisms of absorption, transfer, and multiple impacts. Environ. Sci. Nano 8 (5), 1196–1210. doi:10.1039/d0en01129k
Huan, Y., Kong, Q., Mou, H., and Yi, H. (2020). Antimicrobial peptides: classification, design, application and research progress in multiple fields. Front. Microbiol. 11, 582779. doi:10.3389/fmicb.2020.582779
Imamura, T., Yasuda, M., Kusano, H., Nakashita, H., Ohno, Y., Kamakura, T., et al. (2010). Acquired resistance to the rice blast in transgenic rice accumulating the antimicrobial peptide thanatin. Transgenic Res. 19 (3), 415–424. doi:10.1007/s11248-009-9320-x
Islam, M., Mohamed, G., Polash, S. A., Hasan, M., Sultana, R., Saiara, N., et al. (2021). Antimicrobial peptides from plants: A cDNA-library based isolation, purification, characterization approach and elucidating their modes of action. Int. J. Mol. Sci. 22 (16), 8712. doi:10.3390/ijms22168712
Isozumi, N., Masubuchi, Y., Imamura, T., Mori, M., Koga, H., Ohki, S., et al. (2021). Structure and antimicrobial activity of NCR169, a nodule-specific cysteine-rich peptide of Medicago truncatula. Sci. Rep. 11 (1), 9923. doi:10.1038/s41598-021-89485-w
Jesorka, A., and Orwar, O. (2008). Liposomes: technologies and analytical applications. Annu. Rev. Anal. Chem. 1, 801–832. doi:10.1146/annurev.anchem.1.031207.112747
Jiang, W., Kim, B. Y., Rutka, J. T., and Chan, W. C. (2007). Advances and challenges of nanotechnology-based drug delivery systems. Expert Opin. Drug Deliv. 4 (6), 621–633. doi:10.1517/17425247.4.6.621
Kaur, K., and Sidhu, A. K. (2021). Green synthesis: An eco-friendly route for the synthesis of iron oxide nanoparticles. Front. Nanotechnol. 3, 47. doi:10.3389/fnano.2021.655062
Keymanesh, K., Soltani, S., and Sardari, S. (2009). Application of antimicrobial peptides in agriculture and food industry. World J. Microbiol. Biotechnol. 25 (6), 933–944. doi:10.1007/s11274-009-9984-7
Kleine-Brueggeney, H., Zorzi, G., Fecker, T., El Gueddari, N., Moerschbacher, B., Goycoolea, F., et al. (2015). A rational approach towards the design of chitosan-based nanoparticles obtained by ionotropic gelation. Colloids Surf. B Biointerfaces 135, 99–108. doi:10.1016/j.colsurfb.2015.07.016
Lahiani, M. H., Chen, J., Irin, F., Puretzky, A. A., Green, M. J., Khodakovskaya, M. V., et al. (2015). Interaction of carbon nanohorns with plants: uptake and biological effects. Carbon 81, 607–619. doi:10.1016/j.carbon.2014.09.095
León-Buitimea, A., Garza-Cárdenas, C. R., Garza-Cervantes, J. A., Lerma-Escalera, J. A., and Morones-Ramírez, J. R. (2020). The demand for new antibiotics: Antimicrobial peptides, nanoparticles, and combinatorial therapies as future strategies in antibacterial agent design. Front. Microbiol. 11 (1669). doi:10.3389/fmicb.2020.01669
Lead, J. R., Batley, G. E., Alvarez, P. J., Croteau, M. N., Handy, R. D., and McLaughlin, M. J. (2018). Nanomaterials in the environment: behavior, fate, bioavailability, and effects an updated review. Environ. Toxicol. Chem. 37 (8), 2029-2063.
Li, J., Hu, S., Jian, W., Xie, C., and Yang, X. (2021a). Plant antimicrobial peptides: structures, functions, and applications. Bot. Stud. 62 (1), 5. doi:10.1186/s40529-021-00312-x
Li, P., Huang, Y., Fu, C., Jiang, S. X., Peng, W., Jia, Y., et al. (2021b). Eco-friendly biomolecule-nanomaterial hybrids as next-generation agrochemicals for topical delivery. EcoMat 3 (5), e12132. doi:10.1002/eom2.12132
Li, X., Wang, W., Liu, S., Ruan, C., Yi, L., Deng, L., et al. (2019). Effects of the peptide H-OOWW-NH2 and its derived lipopeptide C12-OOWW-NH2 on controlling of citrus postharvest green mold. Postharvest Biol. Technol. 158, 110979. doi:10.1016/j.postharvbio.2019.110979
López-Vargas, E. R., Ortega-Ortíz, H., Cadenas-Pliego, G., de Alba Romenus, K., Cabrera de la Fuente, M., Benavides-Mendoza, A., et al. (2018). Foliar application of copper nanoparticles increases the fruit quality and the content of bioactive compounds in tomatoes. Appl. Sci. 8 (7), 1020. doi:10.3390/app8071020
Luo, Y., Teng, Z., Li, Y., and Wang, Q. (2015). Solid lipid nanoparticles for oral drug delivery: Chitosan coating improves stability, controlled delivery, mucoadhesion and cellular uptake. Carbohydr. Polym. 122, 221–229. doi:10.1016/j.carbpol.2014.12.084
Makowski, M., Silva, Í. C., Pais do Amaral, C., Gonçalves, S., and Santos, N. C. (2019). Advances in lipid and metal nanoparticles for antimicrobial peptide delivery. Pharmaceutics 11 (11), 588. doi:10.3390/pharmaceutics11110588
Martínez-Ballesta, M., Gil-Izquierdo, Á., García-Viguera, C., and Domínguez-Perles, R. (2018). Nanoparticles and controlled delivery for bioactive compounds: Outlining challenges for new “smart-foods” for health. Foods 7 (5), 72. doi:10.3390/foods7050072
McClements, D. J., and Xiao, H. (2017). Is nano safe in foods? Establishing the factors impacting the gastrointestinal fate and toxicity of organic and inorganic food-grade nanoparticles. npj Sci. Food 1 (1), 1-13.
Meikle, T. G., Dharmadana, D., Hoffmann, S. V., Jones, N. C., Drummond, C. J., Conn, C. E., et al. (2021). Analysis of the structure, loading and activity of six antimicrobial peptides encapsulated in cubic phase lipid nanoparticles. J. Colloid Interface Sci. 587, 90–100. doi:10.1016/j.jcis.2020.11.124
Meikle, T. G., Zabara, A., Waddington, L. J., Separovic, F., Drummond, C. J., Conn, C. E., et al. (2017). Incorporation of antimicrobial peptides in nanostructured lipid membrane mimetic bilayer cubosomes. Colloids Surf. B Biointerfaces 152, 143–151. doi:10.1016/j.colsurfb.2017.01.004
Meraz-Dávila, S., Garcia, C. E. P., and Feregrino-Perez, A. A. (2021). Challenges and advantages of electrospun nanofibers in agriculture: a review. Materials Research Express.
Min, J.-S., Kim, K.-S., Kim, S.-W., Jung, J.-H., Lamsal, K., Kim, S.-B., et al. (2009). Effects of colloidal silver nanoparticles on sclerotium-forming phytopathogenic fungi. Plant Pathology J. 25 (4), 376–380. doi:10.5423/ppj.2009.25.4.376
Mohid, S. A., and Bhunia, A. (2020). Combining antimicrobial peptides with nanotechnology: An emerging field in theranostics. Curr. Protein Pept. Sci. 21 (4), 413–428. doi:10.2174/1389203721666191231111634
Moorcroft, S. C. T., Roach, L., Jayne, D. G., Ong, Z. Y., and Evans, S. D. (2020). Nanoparticle-loaded Hydrogel for the light-activated release and photothermal enhancement of antimicrobial peptides. ACS Appl. Mat. Interfaces 12 (22), 24544–24554. doi:10.1021/acsami.9b22587
Mozafari, M. R. (2005). Liposomes: an overview of manufacturing techniques. Cell. Mol. Biol. Lett. 10 (4), 711–719.
Mulinari, F., Staniscuaski, F., Bertholdo-Vargas, L., Postal, M., Oliveira-Neto, O., Rigden, D., et al. (2007). Jaburetox-2Ec: an insecticidal peptide derived from an isoform of urease from the plant Canavalia ensiformis. Peptides 28 (10), 2042–2050. doi:10.1016/j.peptides.2007.08.009
Nadal, A., Montero, M., Badosa, E., Messeguer, J., Montesinos, L., Montesinos, E., et al. (2012). Constitutive expression of transgenes encoding derivatives of the synthetic antimicrobial peptide BP100: impact on rice host plant fitness. BMC Plant Biol. 12 (1), 159. doi:10.1186/1471-2229-12-159
Niu, L., Zhong, X., Zhang, Y., Yang, J., Xing, G., Li, H., et al. (2020). Enhanced tolerance to Phytophthora root and stem rot by over-expression of the plant antimicrobial peptide CaAMP1 gene in soybean. BMC Genet. 21 (1), 68. doi:10.1186/s12863-020-00872-0
Patil, M. D., Dhas, S. D., and Moholkar, A. V. (2022). “Green synthesis of nanocomposites: A greener approach for a cleaner future,” in Handbook of research on green synthesis and applications of nanomaterials. IGI global, 77–100.
Patra, J. K., Das, G., Fraceto, L. F., Campos, E. V. R., del Pilar Rodriguez-Torres, M., Acosta-Torres, L. S., et al. (2018). Nano based drug delivery systems: recent developments and future prospects. J. Nanobiotechnology 16 (1), 71. doi:10.1186/s12951-018-0392-8
Peng, Q., Yuan, Y., and Gao, M. (2013). Bacillus pumilus, a novel ginger rhizome rot pathogen in China. Plant Dis. 97 (10), 1308–1315. doi:10.1094/PDIS-12-12-1178-RE
Perez-de-Luque, A., and Rubiales, D. (2009). Nanotechnology for parasitic plant control. Pest Manag. Sci. 65 (5), 540–545. doi:10.1002/ps.1732
Pinilla, C. M. B., Lopes, N. A., and Brandelli, A. (2021). Lipid-based nanostructures for the delivery of natural antimicrobials. Molecules 26 (12), 3587. doi:10.3390/molecules26123587
Pinto, M. F., Fensterseifer, I. C., Migliolo, L., Sousa, D. A., de Capdville, G., Arboleda-Valencia, J. W., et al. (2012). Identification and structural characterization of novel cyclotide with activity against an insect pest of sugar cane. J. Biol. Chem. 287 (1), 134–147. doi:10.1074/jbc.M111.294009
Porto, W. F., Irazazabal, L., Alves, E. S., Ribeiro, S. M., Matos, C. O., Pires, Á. S., et al. (2018). In silico optimization of a guava antimicrobial peptide enables combinatorial exploration for peptide design. Nat. Commun. 9 (1), 1490. doi:10.1038/s41467-018-03746-3
Prombutara, P., Kulwatthanasal, Y., Supaka, N., Sramala, I., and Chareonpornwattana, S. (2012). Production of nisin-loaded solid lipid nanoparticles for sustained antimicrobial activity. Food control. 24 (1-2), 184–190. doi:10.1016/j.foodcont.2011.09.025
Rafiee, A., Alimohammadian, M. H., Gazori, T., Riazi-rad, F., Fatemi, S. M. R., Parizadeh, A., et al. (2014). Comparison of chitosan, alginate and chitosan/alginate nanoparticles with respect to their size, stability, toxicity and transfection. Asian Pac. J. Trop. Dis. 4 (5), 372–377. doi:10.1016/s2222-1808(14)60590-9
Rafique, M., Sadaf, I., Rafique, M. S., and Tahir, M. B. (2017). A review on green synthesis of silver nanoparticles and their applications. Artif. Cells Nanomed. Biotechnol. 45 (7), 1272–1291. doi:10.1080/21691401.2016.1241792
Reis, C. P., Neufeld, R. J., Ribeiro, A. J., and Veiga, F. (2006). Nanoencapsulation I. Methods for preparation of drug-loaded polymeric nanoparticles. Nanomedicine 2 (1), 8–21. doi:10.1016/j.nano.2005.12.003
Ristaino, J. B., Anderson, P. K., Bebber, D. P., Brauman, K. A., Cunniffe, N. J., Fedoroff, N. V., et al. (2021). The persistent threat of emerging plant disease pandemics to global food security. Proc. Natl. Acad. Sci. U. S. A. 118 (23), e2022239118. doi:10.1073/pnas.2022239118
Rizzo, D. M., Lichtveld, M., Mazet, J. A., Togami, E., and Miller, S. A. (2021). Plant health and its effects on food safety and security in a One Health framework: Four case studies. One health outlook 3 (1), 6. doi:10.1186/s42522-021-00038-7
Rodrigues, G., da Costa Sousa, M. G., Rezende, T. M. B., and Franco, O. L. (2021). Can metallic nanomaterials be green and sustainable? Curr. Opin. Environ. Sci. Health 24, 100292. doi:10.1016/j.coesh.2021.100292
Ruden, S., Hilpert, K., Berditsch, M., Wadhwani, P., and Ulrich, A. S. (2009). Synergistic interaction between silver nanoparticles and membrane-permeabilizing antimicrobial peptides. Antimicrob. Agents Chemother. 53 (8), 3538–3540. doi:10.1128/AAC.01106-08
Sadeghi, R., Rodriguez, R. J., Yao, Y., and Kokini, J. L. (2017). Advances in nanotechnology as they pertain to food and agriculture: benefits and risks. Annu. Rev. Food Sci. Technol. 8, 467–492. doi:10.1146/annurev-food-041715-033338
Sandreschi, S., Piras, A. M., Batoni, G., and Chiellini, F. (2016). Perspectives on polymeric nanostructures for the therapeutic application of antimicrobial peptides. Nanomedicine 11 (13), 1729–1744. doi:10.2217/nnm-2016-0057
Santana, I., Wu, H., Hu, P., and Giraldo, J. P. (2020). Targeted delivery of nanomaterials with chemical cargoes in plants enabled by a biorecognition motif. Nat. Commun. 11 (1), 2045. doi:10.1038/s41467-020-15731-w
Sarika, , Iquebal, M. A., and Rai, A. (2012). Biotic stress resistance in agriculture through antimicrobial peptides. Peptides 36 (2), 322–330. doi:10.1016/j.peptides.2012.05.012
Sarkar, T., Chetia, M., and Chatterjee, S. (2021). Antimicrobial peptides and proteins: From nature's reservoir to the laboratory and beyond. Front. Chem. 9, 691532. doi:10.3389/fchem.2021.691532
Savary, S., Willocquet, L., Pethybridge, S. J., Esker, P., McRoberts, N., Nelson, A., et al. (2019). The global burden of pathogens and pests on major food crops. Nat. Ecol. Evol. 3 (3), 430–439. doi:10.1038/s41559-018-0793-y
Sharma, A., Gaur, A., Kumar, V., Sharma, N., Patil, S. A., Verma, R. K., et al. (2021). Antimicrobial activity of synthetic antimicrobial peptides loaded in poly-Ɛ-caprolactone nanoparticles against mycobacteria and their functional synergy with rifampicin. Int. J. Pharm. 608, 121097. doi:10.1016/j.ijpharm.2021.121097
Sharma, P., Singh, S. P., Iqbal, H. M., Parra-Saldivar, R., Varjani, S., Tong, Y. W., et al. (2022). Genetic modifications associated with sustainability aspects for sustainable developments. Bioengineered 13 (4), 9508–9520. doi:10.1080/21655979.2022.2061146
Sharma, V. K., Yngard, R. A., and Lin, Y. (2009). Silver nanoparticles: green synthesis and their antimicrobial activities. Adv. Colloid Interface Sci. 145 (1-2), 83–96. doi:10.1016/j.cis.2008.09.002
Shuai, J., Guan, F., He, B., Hu, J., Li, Y., He, D., et al. (2019). Self-assembled nanoparticles of symmetrical cationic peptide against citrus pathogenic bacteria. J. Agric. Food Chem. 67 (20), 5720–5727. doi:10.1021/acs.jafc.9b00820
Singh, V. K., Singh, R., Kumar, A., and Bhadouria, R. (2021). “Current status of plant diseases and food security,” in Food security and plant disease management (Elsevier), 19–35.
Sohal, I. S., OFallon, K. S., Gaines, P., Demokritou, P., and Bello, D. (2018). Ingested engineered nanomaterials: state of science in nanotoxicity testing and future research needs Particle and Fibre Toxicol. 15 (1), 1–31.
Su, Y., Ashworth, V., Kim, C., Adeleye, A. S., Rolshausen, P., Roper, C., et al. (2019). Delivery, uptake, fate, and transport of engineered nanoparticles in plants: a critical review and data analysis. Environ. Sci. Nano 6 (8), 2311–2331. doi:10.1039/c9en00461k
Tan, P., Fu, H., and Ma, X. (2021). Design, optimization, and nanotechnology of antimicrobial peptides: From exploration to applications. Nano Today 39, 101229. doi:10.1016/j.nantod.2021.101229
Taylor, T. M., Weiss, J., Davidson, P. M., and Bruce, B. D. (2005). Liposomal nanocapsules in food science and agriculture. Crit. Rev. Food Sci. Nutr. 45 (7-8), 587–605. doi:10.1080/10408390591001135
Teixeira, M. C., Carbone, C., Sousa, M. C., Espina, M., Garcia, M. L., Sanchez-Lopez, E., et al. (2020). Nanomedicines for the delivery of antimicrobial peptides (AMPs). Nanomater. (Basel) 10 (3), E560. doi:10.3390/nano10030560
Thiruvengadam, M., Rajakumar, G., and Chung, I.-M. (2018). Nanotechnology: current uses and future applications in the food industry. 3 Biotech. 8 (1), 74. doi:10.1007/s13205-018-1104-7
Turnbull, C., Lillemo, M., and Hvoslef-Eide, T. A. (2021). Global regulation of genetically modified crops amid the gene edited crop boom–a review. Front. Plant Sci. 12, 630396. doi:10.3389/fpls.2021.630396
Vauthier, C., and Bouchemal, K. (2009). Methods for the preparation and manufacture of polymeric nanoparticles. Pharm. Res. 26 (5), 1025–1058. doi:10.1007/s11095-008-9800-3
Vega-Vasquez, P., Mosier, N. S., and Irudayaraj, J. (2020). Nanoscale drug delivery systems: From medicine to agriculture. Front. Bioeng. Biotechnol. 8, 79. doi:10.3389/fbioe.2020.00079
Wadhwani, P., Heidenreich, N., Podeyn, B., Burck, J., and Ulrich, A. S. (2017). Antibiotic gold: tethering of antimicrobial peptides to gold nanoparticles maintains conformational flexibility of peptides and improves trypsin susceptibility. Biomater. Sci. 5 (4), 817–827. doi:10.1039/c7bm00069c
Wang, C., Hong, T., Cui, P., Wang, J., and Xia, J. (2021). Antimicrobial peptides towards clinical application: Delivery and formulation. Adv. Drug Deliv. Rev. 175, 113818. doi:10.1016/j.addr.2021.05.028
Wang, J., Li, Z., Huang, S., Ye, X., Feng, D., and Zhang, Z. (2012). Development and characterization of SN1 transgenic wheat plants with enhanced resistance to Rhizoctonia cerealis and Bipolaris sorokiniana. A. A. S. 38 (5), 773–779. doi:10.3724/sp.j.1006.2012.00773
Wang, S., Zeng, X., Yang, Q., and Qiao, S. (2016). Antimicrobial peptides as potential alternatives to antibiotics in food animal industry. Int. J. Mol. Sci. 17 (5), 603. doi:10.3390/ijms17050603
Wang, W., Deng, L., Yao, S., and Zeng, K. (2018a). Control of green and blue mold and sour rot in citrus fruits by the cationic antimicrobial peptide PAF56. Postharvest Biol. Technol. 136, 132–138. doi:10.1016/j.postharvbio.2017.10.015
Wang, W., Liu, S., Deng, L., Ming, J., Yao, S., Zeng, K., et al. (2018b). Control of citrus post-harvest green molds, blue molds, and sour rot by the Cecropin A-Melittin hybrid peptide BP21. Front. Microbiol. 9, 2455. doi:10.3389/fmicb.2018.02455
Worrall, E. A., Hamid, A., Mody, K. T., Mitter, N., and Pappu, H. R. (2018). Nanotechnology for plant disease management. Agronomy 8 (12), 285. doi:10.3390/agronomy8120285
Wu, H., Tito, N., and Giraldo, J. P. (2017). Anionic cerium oxide nanoparticles protect plant photosynthesis from abiotic stress by scavenging reactive oxygen species. ACS Nano 11 (11), 11283–11297. doi:10.1021/acsnano.7b05723
Yoo, H. S., Kim, T. G., and Park, T. G. (2009). Surface-functionalized electrospun nanofibers for tissue engineering and drug delivery. Adv. Drug Deliv. Rev. 61 (12), 1033–1042. doi:10.1016/j.addr.2009.07.007
Zainal, Z., Marouf, E., Ismail, I., and Fei, C. (2009). Expression of the Capsicuum annum (chili) defensin gene in transgenic tomatoes confers enhanced resistance to fungal pathogens. Am. J. Plant Physiology 4 (2), 70–79. doi:10.3923/ajpp.2009.70.79
Zhang, J., Boghossian, A. A., Barone, P. W., Rwei, A., Kim, J.-H., Lin, D., et al. (2011). Single molecule detection of nitric oxide enabled by d (AT) 15 DNA adsorbed to near infrared fluorescent single-walled carbon nanotubes. J. Am. Chem. Soc. 133 (3), 567–581. doi:10.1021/ja1084942
Zhang, Y., Yan, J., Avellan, A., Gao, X., Matyjaszewski, K., Tilton, R. D., et al. (2020). Temperature-and pH-responsive star polymers as nanocarriers with potential for in vivo agrochemical delivery. ACS Nano 14 (9), 10954–10965. doi:10.1021/acsnano.0c03140
Zhang, Z.-H., Wang, X.-P., Ayman, W. Y., Munyendo, W. L., Lv, H.-X., Zhou, J.-P., et al. (2013). Studies on lactoferrin nanoparticles of gambogic acid for oral delivery. Drug Deliv. 20 (2), 86–93. doi:10.3109/10717544.2013.766781
Zharkova, M. S., Golubeva, O. Y., Orlov, D. S., Vladimirova, E. V., Dmitriev, A. V., Tossi, A., et al. (2021). Silver nanoparticles functionalized with antimicrobial polypeptides: Benefits and possible pitfalls of a novel anti-infective tool. Front. Microbiol. 12, 750556. doi:10.3389/fmicb.2021.750556
Keywords: antimicrobial peptides, nanostructure, crop production, biotic stress, food production, biotechnology, agribusiness
Citation: Maximiano MR, Rios TB, Campos ML, Prado GS, Dias SC and Franco OL (2022) Nanoparticles in association with antimicrobial peptides (NanoAMPs) as a promising combination for agriculture development. Front. Mol. Biosci. 9:890654. doi: 10.3389/fmolb.2022.890654
Received: 06 March 2022; Accepted: 15 July 2022;
Published: 23 August 2022.
Edited by:
Elizabete de Souza Cândido, Dom Bosco Catholic University, BrazilReviewed by:
Håvard Jenssen, Roskilde University, DenmarkMark Anthony Jackson, Queensland University of Technology, Australia
Edward Kalani Gilding, The University of Queensland, Australia
Copyright © 2022 Maximiano, Rios, Campos, Prado, Dias and Franco. This is an open-access article distributed under the terms of the Creative Commons Attribution License (CC BY). The use, distribution or reproduction in other forums is permitted, provided the original author(s) and the copyright owner(s) are credited and that the original publication in this journal is cited, in accordance with accepted academic practice. No use, distribution or reproduction is permitted which does not comply with these terms.
*Correspondence: Octávio Luiz Franco, b2NmcmFuY29AZ21haWwuY29t