- 1Department of Oral Medicine, School of Basic Medical Sciences, Capital Medical University, Beijing, China
- 2Eight Program of Clinical Medicine, Peking University Health Science Center, Beijing, China
- 3Department of Physiology and Pathophysiology, School of Basic Medical Sciences, Capital Medical University, Beijing, China
- 4Clinical Medicine of “5+3” Program, School of Basic Medical Sciences, Capital Medical University, Beijing, China
- 5Department of Biomedical Informatics, Faculty of Biomedical Engineering, Capital Medical University, Beijing, China
- 6Department of Dermatology, Tongren Hospital, Capital Medical University, Beijing, China
Peroxisome proliferator–activated receptor (PPAR)-α is a ligand-activated transcription factor distributed in various tissues and cells. It regulates lipid metabolism and plays vital roles in the pathology of the cardiovascular system. However, its roles in the gastrointestinal tract (GIT) are relatively less known. In this review, after summarizing the expression profile of PPAR-α in the GIT, we analyzed its functions in the GIT, including physiological control of the lipid metabolism and pathologic mediation in the progress of inflammation. The mechanism of this regulation could be achieved via interactions with gut microbes and further impact the maintenance of body circadian rhythms and the secretion of nitric oxide. These are also targets of PPAR-α and are well-described in this review. In addition, we also highlighted the potential use of PPAR-α in treating GIT diseases and the inadequacy of clinical trials in this field.
Highlights
In this review, after briefly introducing the characteristics of the PPAR family in the liver and cardiovascular system, we highlighted the specialties of PPAR-α and summarized its role in the gastrointestinal tract. It is responsible for the regulation of nutrient uptake and mediation of the inflammatory process. Moreover, studies also reported its participation in the maintenance of gastrointestinal circadian rhythms or circadian clock and satiety. These may provide novel and therapeutic targets for the treatment of gastrointestinal and systemic diseases.
Introduction
Since the discovery and cloning by Issemann et al. in 1990, peroxisome proliferator–activated receptors (PPARs) have received increasing attention for their multiple functions (Issemann and Green, 1990). Three subtype proteins found within the family are known as PPAR-α, PPAR-γ, and PPAR-β/δ, regulating the lipid metabolism and inflammation state (Dreyer et al., 1992; Bordet et al., 2006). They share common functions in metabolism and inflammatory regulation but are distinct from one another in both the distribution patterns and target molecules (Braissant et al., 1995). The common structure of the ligand-binding domain (LBD) in the shape of the letter Y laid the basis for the similarity and differences among this protein family (Mandard et al., 2004). The first arm containing hydrophilic amino acid residues is responsible for ligand binding and exists in all three subtypes, while the remaining two parts consisting of far fewer amino acid hydrophilic residues account for the specialties among them. PPAR-α is a transcription factor belonging to the nuclear receptor superfamily and could be activated by fibrates, eicosanoids, and fatty acids (Forman et al., 1997). However, contrary to steroid hormone receptors acting as homodimers, transcriptional regulation by PPARs requires heterodimerization with the retinoid X receptor (RXR; NR2B) in the same receptor superfamily (Mandard et al., 2004).
PPARs are ligand-activated transcription factors originally known to be activated by hepatocarcinogens and lead to peroxisome proliferation (Desvergne and Wahli, 1999). They are detected in a wide range of tissues, including endothelial and muscular cells and macrophages (Mφs) and monocytes. This endows them with a wide range of roles, including immune functions all over the body and regulations of a specific organ (Mandard et al., 2004). The well-recognized roles in alleviating heart dysfunction and hypertension in the cardiovascular system have been well-characterized (Goikoetxea et al., 2004; Usuda and Kanda, 2014), and their abilities to regulate fatty acid transportation and oxidation in the liver have been well-illustrated, further unveiling its association with various kinds of liver injuries (Botta et al., 2018; Kong et al., 2021). These could lead to some systematic diseases including diabetes and result in pathological dysfunctions in multiple organs (R. Moschen et al., 2012). In the meantime, studies have verified their roles in peripheral and neural inflammation (Piomelli, 2013). However, although much effort has been put into investigating its roles in the cardiovascular system, investigations on its roles in the gastrointestinal tract were relatively less. Recent analysis has certificated the distribution and activation of PPAR-α in the GIT with a higher level in the more differentiated cells near the lumen compared to those residing in the crypts (Mansén et al., 1996). Furthermore, studies also confirm the expression of PPAR-α in enterocytes along the small intestine with the highest levels in the duodenum and the jejunum. A higher level of PPAR-α is also found in villus tips than in crypts (Bünger et al., 2007). This expression pattern is similar to that of several other genes involved in dietary fat absorption, including microsomal triglyceride transfer protein (Mttp), diacylglycerol acyltransferase 1 (Dgat1), fatty acid translocase (Cd36), and fatty acid transport protein 4 (Fatp4), and lay the foundation for their wide interactions (Suzuki et al., 2009).
In this review, the roles of PPAR-α in the development of inflammation and regulation of metabolism are depicted and show its broad regulatory effects in the GIT and the whole body. Meanwhile, as agonists and antagonists are commonly used as drugs for the cardiovascular system (CVS), we evaluated the possibilities of their use in treating GIT diseases.
A Pivotal Regulator of Metabolism
As mentioned earlier, PPAR-α is involved in regulating the expression of various genes in lipid metabolism. However, despite the well-depicted regulation of genes associated with lipid metabolism in the liver, the regulation of genes by PPAR-α in the intestine is relatively less described (Steineger et al., 1994). In fact, in addition to the similarity in expression modes, Affymetrix arrays and quantitative RT-PCR analysis have demonstrated a PPAR-α–dependent upregulation of eight genes concerning transporters and phase I/II metabolism during fasting (the details of these genes are shown in Table 1) (van den Bosch et al., 2007). Several other studies also corroborated the increase of PPAR-α in mice and the downregulation of genes related to lipid metabolism (Escher et al., 2001; Shimakura et al., 2006).
Intestinal fatty acid–binding proteins (IFABPs) are important for regulating the uptake and transportation of the long-chain fatty acids (LCFAs) and significant biomarkers of gastrointestinal diseases (Holehouse et al., 1998; Kokesova et al., 2019). Detected more in proximal than in distal small intestine (Poirier et al., 1996), the IFABP expression in the rat jejunum showed significant enhancement during the postnatal development, concomitant with the increased mRNA level of PPAR in situ. Electrophoretic mobility shift assays revealed the existence of the PPAR-α-9-cis-retinoic acid receptor (RXRα), a heterodimer whose binding activities could be enhanced by an additional PPAR-α agonist WY-14643 (Mochizuki et al., 2001). Although this is inconsistent with some previous findings that the levels of PPAR-α and IFABPs show contrary variations under some treatment (Poirier et al., 1997), the regulation of metabolism by PPAR-α via gene transcription might be undeniable as more investigations utilizing different types of the PPAR-α agonist witnessed a concomitant increase of the IFABP level with PPAR-α (Mallordy et al., 1995).
Meanwhile, studies comparing the expression mode of genes between obesity-resistant A/J and obesity-prone C57BL/6J mice show a prominent upregulation of genes regulating lipid metabolism. However, this increase is restricted in the small intestine with no significant change in other organs such as the liver and white adipose tissue. Experiments in mouse Caco-2/TC7 cells and in human jejunal biopsies show that PPAR-α activation using WY-14643 increases the expression of ATP-binding cassette transporter A1 (ABCA1) (Knight et al., 2003). However, when WY-1463 was replaced by fibrates, the levels of both ABCA1 and protein-1c gene (SREBP-1c) increased. This is concomitant with the increase in the expression of genes modifying cholesterol trafficking and the decreased capacity of cholesterol esterification. Meanwhile, other findings show that the usage of fenofibrate, a selective PPAR-α agonist, and elafibranor (GFT505), a selective PPAR-α/δ agonist, did not remain the same in different experiments (Colin et al., 2013). Similar experiments further verified that this modulation could also be applied to the expression of genes regulating lipid metabolism (Kondo et al., 2006). For example, intraperitoneal (IP) administration of pirinixic acid (Wy-14643), a selective and highly potent PPAR-α agonist, stimulates fatty acid oxidation (FAO) and ketogenesis in the intestine This is concomitant with a significant increase in the expression of cytochrome P450 1A1(CPT 1A1) in the jejunum and duodenum and of HMG-CoAS2 in the jejunum (Stavinoha et al., 2004; Sérée et al., 2004). However, in this experiment neither CPT 1A nor HMG-CoAS2 expression was increased in the liver, suggesting a pivotal role the intestine plays in this regulation (Karimian Azari et al., 2013). Other genes in this type of regulation include fatty acid translocase (FAT)/cluster of differentiation 36 (CD36), fatty acid transport protein (FATP), NPC1L1, Acox1, Fabp1 (Hutch et al., 2020), and mitochondrial aspartate aminotransferase (mAspAT) (refer to Table 2 for more detailed information) (Uchida et al., 2011; Roberts, 1989; Valasek et al., 2007). Contrarily, Pan et al. found a PPAR-α independent way of OEA to increase the secretion of triacylglycerols, ApoB, and MTP in differentiated Caco-2 cells and primary enterocytes (Pan et al., 2018). Consistently, Mariana et al. also found no pertinence between the component of nutrient transporters and the level of PPAR-α (Losacco et al., 2018). Also, in many experiments, the level alteration in the intestine differs from that in the liver and the range of targeted genes varied with different kinds of agonists, indicating a complex mechanism waiting for investigation (Motojima et al., 1998). The use of PPAR-α agonists, both natural and synthetic, is an effective and widely accepted method to examine its functions (as shown in Table 2) (Lefebvre et al., 2006). Further analysis of these molecules, including oleoylethanolamide (OEA), palmitoylethanolamine (PEA), and WY-14643, provides the foundation for the understanding of the broad variety of PPAR-α functions.
All these findings show novel roles of PPAR-α in the intestine compared to those in the liver and are worth more investigations for full elucidation (Refer to Figure 1 for visual understanding).
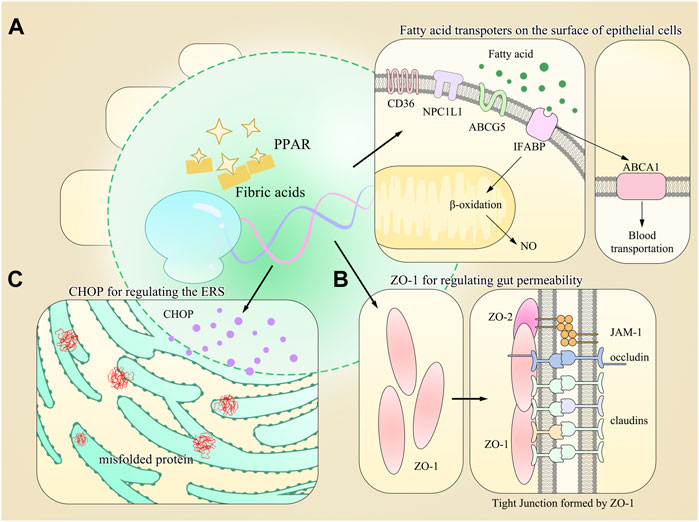
FIGURE 1. Model diagram of PPAR-α-regulating gene expression and multiple physiological processes in the gut. (A) Multiple types of fatty acid transporters are found on the surface of gastrointestinal epithelial cells, and most of their synthesis requires the activation of PPAR-α. (B) Other proteins maintaining the homeostasis of GIT are also regulated by PPAR-α, such as ZO-1 for gut permeability. (C) PPAR-α also regulates the expression of CHOP, which is responsible for regulating the endoplasmic reticulum stress (ERS).
Oleoylethanolamide, a Widely Accepted Endogenous Peroxisome Proliferator–Activated Receptor-α Agonist Used in Investigations
Oleoylethanolamide (OEA) is a kind of endogenous PPAR-α agonist with high affinity and plays an important role in the treatment of obesity and atherosclerosis. It is a structural analog of the endocannabinoid anandamide, an endogenous free fatty acid known for its role in regulating lipid metabolism (Rodríguez de Fonseca et al., 2001). It could be derived from digestion products by intestinal microbes and could be secreted endogenously by enterocytes (Obici et al., 2002). Meanwhile, it is also synthesized by astrocytes and neurons and could serve as a significant neurotransmitter regulating satiety (Cani et al., 2004). Most of these functions are mediated by PPAR-α, making it a potential target toward diabetes and giving it increasing significance considering the relationship with cardiovascular and neuron dysfunctions mentioned earlier (Koethe et al., 2009). It is also reported to bear a higher affinity compared with the other two agonists (Lo Verme et al., 2005; Brown et al., 2017). Moreover, the use of OEA supplements has been approved by the FDA for the treatment of obesity and shows prospective effects (Brown et al., 2018a). This is contrary to some previous studies revealing the side effects of OEA, indicating the requirement for more detailed studies (Nielsen et al., 2004; Brown et al., 2018b). These regulations in general help with the maintenance of a proper level of PPAR-α in the intestine and the homeostasis under its regulation.
Apart from the roles in regulating metabolite-associated gene expressions, OEA is also found to lower body weight and relieve hyperlipidemia in obese rats via the regulation of NO synthesis (Fu et al., 2003). Further analysis showed that it could also regulate satiety through a paracrine PPAR-α–mediated mechanism involving the recruitment of afferent sensory fibers (DiPatrizio and Piomelli, 2015). OEA produced by small-intestinal enterocytes during dietary fat digestion activates PPAR-α to trigger an afferent signal that causes satiety (Igarashi et al., 2017). Using a rat model of Roux-en-Y gastric bypass (RYGB), Hankir et al. found that marked reductions in fat appetite are due to enhanced gut lipid sensing through PPAR-α, which is in turn transmitted to the central nervous system (CNS) by sensory vagal afferents, culminating in increased dorsal striatal D1R signaling (Hankir et al., 2017). However, using multiple dopamine D2/D3 receptor agonists and celiac superior mesenteric ganglionectomy (CGX) or subdiaphragmatic vagal deafferentation (SDA), Shahana et al. showed that IP OEA’s anorectic effect may be secondary to impaired locomotion rather than physiological satiety and that vagal afferents do not mediate exogenous OEA’s anorectic effects. They also suggested a role for spinal afferents in addition to an alternative, non-neuronal signaling route (Fedele et al., 2018). Taken together, these findings raised the possibilities for the treatment of eating disorders by OEA and other PPAR-α–related products (Bünger et al., 2007).
Important Regulators of Inflammation
Intestinal bowel diseases (IBD), including mainly Crohn’s disease and ulcerative colitis, are relapsing and chronic GIT disorders becoming increasingly prevalent all over the world (Windsor and Kaplan, 2019). Despite the poor understanding of its pathogenesis, tissue examinations of various patients show different levels of mucosa injuries concomitant with the courses of diseases (Ahmad et al., 2017). These findings suggest the dysregulation of epithelial cell functions resulting from stimulations both directly from the lumen contents and cytokines secreted by lymphocytes and Mφ. As is known that metabolites could serve as mediators of IBD, it is reasonable to understand the underlying indirect role PPAR family proteins plays in the process of IBD (Roediger and Nance, 1986). Meanwhile, transcriptomic and proteomic profiling of human colon biopsy specimens showed the downregulation of PPAR signaling pathways in IBD (Jin et al., 2019). Studies also found disruption of the protective roles of PPAR-α agonists in PPAR-α-KO mice, indicating the pivotal functions it may have in the course of GIT diseases (Capasso et al., 2014). Also, the level of PPAR-α is decreased in a resection model of short bowel syndrome and is consistent with its level alteration in high malignant human tissue mucosa (Wang et al., 2007). Using human HCA7 cells, Jackson et al. further convinced the activation of PPRE-tk-luc, a PPRE-driven reporter gene, by PPAR-α using the transfection method (Jackson et al., 2003). In the dextran sodium sulfate (DSS)–induced mouse ulcerative colitis model, Manoharan et al. found that PPAR-α regulates the expression of IL-22 and antimicrobial peptides RegIIIb, RegIIIg, and calprotectin (Manoharan et al., 2016). IL-22 is an important member of the IL-10 cytokine family and has bidirectional functions for both anti-inflammation and pro-inflammation (Wei et al., 2020). However, the detailed mechanism by which PPAR-α activated NKp46+ ILC3 cells, the major producers of IL-22 under homeostatic conditions in the gut, still remains to be elucidated. Studies also corroborated that PPAR-α played defensive roles in the progression of IBD and CAC mainly via the stimulation of antimicrobial peptides RegIIIb and RegIIIg (Zheng et al., 2008; Killig et al., 2014) In interleukin 10 knockout (IL-10−/−) colitis mice, treatment with fenofibrate repressed interferon-gamma and IL-17 expression in isolated T cells. Considering the activation of PPAR-α by fenofibrate, this protection could be attributed to PPAR-α and put into clinical uses (Lee et al., 2007). Increased levels of Th17 and Th1 cells in this model may also account for injuries in the GIT as the secretion of IL-17 by Th17 is a core step in the progression of GIT disorders (Yang et al., 2017). An increasing number of other pro-inflammatory factors including IL-1b, IL-6, and TNF-α could be possible reasons for this enhancement in Th17 and Th1 cells. Concomitant with this, DNBS-treated PPAR-α–knockout (PPAR-αKO) mice experienced severer colon injuries accompanied with upregulation of ICAM-1 (Cuzzocrea et al., 2004). The levels of TNF-α and interleukin-1β (IL-1β) were also increased, resulting in antibody-mediated membrane dysfunctions (Stack et al., 1997). The decreased level of ICAM-1 and other adhesion molecules including VCAM-1 and P-selectin reduces the infiltration of neutrophils and ROS formation and thus aggravates the intestinal inflammation (Cuzzocrea et al., 2001). Apart from the anti-inflammatory roles of PPAR-α on DNBS-induced colitis, the functions of PPAR-α could also be enhanced by glucocorticoids (GCs). Other studies also show a less degree of colitis in WT mice compared to that in PPAR-αKO mice with an inhibition of p65 phosphorylation, which is an important regulator of the NF-κB pathway (Riccardi et al., 2009). Similarly, in human enterocytes (Caco-2), Shinsuke et al. also found the involvement of NF-κB after OEA injection (Otagiri et al., 2020). Also, in the splanchnic artery occlusion (SAO) shock model, administration of PEA 5 min before reperfusion significantly reduced the inflammatory parameters, including IL-1β and TNF-α. These effects were at least partly dependent on PPAR-α as the decrease of inflammatory markers was less significant in PPAR-α−/− mice than that in WT ones (Di Paola et al., 2012). In conclusion, all these studies provide novel insights into the roles of PPAR-α in mediating GIT inflammation and provide a potential target for pharmaceutical synthesis.
As an analogy of OEA, palmitoylethanolamide, a well-recognized PPAR-α agonist, could also exert an antiproliferative effect and downregulate VEGF signaling in Caco-2 through selective and PPAR-α-dependent inhibition of the Akt/mTOR pathway (Sarnelli et al., 2016). Several studies have corroborated the effect of palmitoylethanolamide in attenuating the GIT injuries using different models of both humans and mice (Borrelli et al., 2015). Mustafa et al. demonstrated its roles in modulating intestinal permeability in a PPAR-α–dependent method using the antagonist GW6471 (Karwad et al., 2017). In the intestine, PEA treatment also improves all macroscopic signs of UC and decreases the expression of the pro-inflammatory biomarkers, including PGE2, IL-1β, and TNFα. Further analysis shows that this effect is mediated mainly by selective targeting of the S100B/TLR4 axis on ECG and downstream inhibition of NF-кB-dependent inflammation (Esposito et al., 2014). Using mice with chronic intestinal inflammation induced by croton oil, Raffaele et al. found significantly decreased levels of PEA in inflammatory mice which could probably contribute to the exaggerated transition (Capasso et al., 2001). However, Cluny et al. showed no difference in gut mobility between PPAR-αKO and WT mice, indicating a PPAR-α–independent pathway in remaining elucidation (Cluny et al., 2009).
Apart from the roles as a significant mediator in IBD, PPAR-α can also regulate the progress of GIT cancer. Studies have found gastric gavage of the PPAR-α ligand bezafibrate inhibited the DSS-induced colitis by and lowered trefoil factor-2 content in colonic mucosa (Tanaka et al., 2001). It also inhibits the formation of aberrant crypt foci (ACF), which is recognized as a precursor lesion in colorectal cancer (Shivapurkar et al., 1997). Further investigations show increased expressions of cyclooxygenase-2 (COX-2), an important mediator in the development of colonic carcinoma in the human colorectal epithelial cell line HT-29 (Prescott and White, 1996; Ma et al., 2018). This could be explained by previous findings that show COX-2–mediated regulation is one of the important downstream pathways induced by TFF2 and enhanced the COX-2 expression via PPAR ligands in some human colorectal epithelial cells (Ikawa et al., 2001; Meade et al., 1999).
Mediation Between Metabolism and Inflammation
Acknowledged as the “second brain” in the human body, gut microbiota play essential roles in the gastrointestinal tract that is regarded as the largest digestive as well as immune organ (Ridaura and Belkaid, 2015). Based on our aforementioned analysis, PPAR-α exerts a significant influence on the components of gastrointestinal microbes and the host physical health condition via the regulation of gene transcription (Rooks and Garrett, 2016; Ashrafian et al., 2019; Hasan et al., 2019). Lactobacillus species are significant protectors of the GIT, and the reduction of their number is an important characterization in many GIT diseases (Tan et al., 2020). This regulation is partly mediated via the PPAR-α as sub-chronic OEA administration to mice fed with a normal chow pellet diet changes the fecal microbiota profile and shifts the Firmicutes: Bacteroidetes ratio in favor of Bacteroidetes (in particular Bacteroides genus). It also decreases the number of Firmicutes (Lactobacillus) and reduces the intestinal cytokine expression by immune cells isolated from Peyer’s patches. (Di Paola et al., 2018; Cai et al., 2020; Kersten et al., 1999), Meanwhile, the introduction of probiotic Lactobacillus plantarum into simian immunodeficiency virus (SIV)–inflamed intestinal lumen resulted in a higher level of PPAR-α concomitant with a recovered expression of PPAR-α–targeted genes (Crakes et al., 2019). Studies also found that mice fed with high-fat chow and supplemented with the probiotic bacteria Lactobacillus paracasei ssp. paracasei F19 (F19) exhibit significantly less body fat. This is also accompanied by a higher level of angiopoietin-like 4 (ANGPTL4), a circulating lipoprotein lipase (LPL) inhibitor regulated by PPAR-α and shows the protective roles of it (Aronsson et al., 2010). These findings corroborated the roles of PPAR-α with Lactobacillus and provided novel prospects for future studies. Apart from the roles in modulating GIT functions, the effect of this interaction could also alter the physiological and pathological conditions of other organs via metabolites transporting in blood as experiments found that exposure to high-fat diets and food deprivation enhances PPAR-α-dependent signaling in the liver and intestine. Lactobacillus plantarum FRT10 could also alleviate the high-fat diet-induced obesity in mice via regulating the PPAR-α signal pathway (Duparc et al., 2017).
Apart from the roles in regulating metabolism and inflammation, respectively, interactions between PPAR-α and gut microbiota also help with the maintenance of the circadian rhythms. This could be confirmed by its disruption under microbiota depletion and result in activation of the c-Jun expression, leading to the dysregulation of a serious set of genes related to inflammation (Mukherji et al., 2013).
Nitric oxide (NO) is one of the major biomarkers of GIT inflammation mainly synthesized by the inducible nitric oxide synthase (iNOS) enzyme in serum and affected tissues. It can exacerbate GIT inflammation and is elevated in times of colitis (Kamalian et al., 2020). Meanwhile, it also has close interplays with microbial components and liver metabolism (Yaguchi and Yaguchi, 2019). Studies using leukotriene B4, a PPAR-α agonist, have found naturally occurring PPAR agonists can inhibit the iNOS enzyme pathway. They further proposed the possibility of this modulation by the stress protein heme oxygenase 1, although the exact mechanisms wait for more investigations (Colville-Nash et al., 1998). Concomitantly, Fu et al. provided evidence for this correlation in OEA which was also recognized as a potential regulation of satiety (Fu et al., 2003; Sihag and Jones, 2018) (Refer to Figure 2 for vivid understanding).
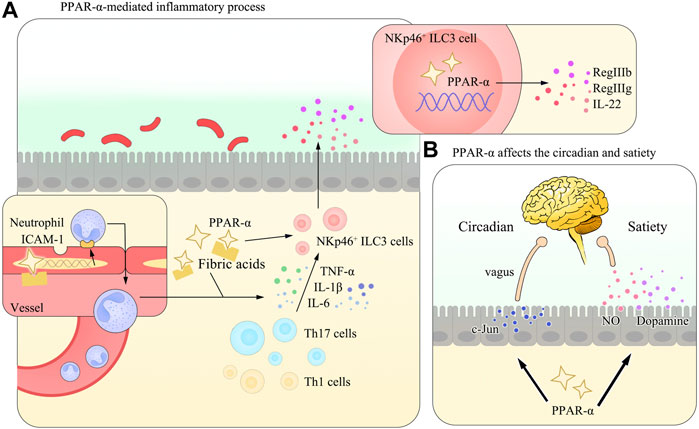
FIGURE 2. Diagram of the PPAR-α–mediated inflammatory process and the regulation of circadian rhythms or circadian clock and satiety in the gut. (A) In many gastrointestinal diseases, PPAR-α is activated and initiates the expression of multiple anti-inflammation mediators, including ICAM-1 in vascular epithelial cells and IL-22 in NKp46+ ILC3 cells. These effects help reverse the imbalance of the T-cell number and maintain the homeostasis of the GIT. (B) Apart from its role in regulating the inflammatory process, PPAR-α could also affect the circadian rhythms or circadian clock (via the regulation of the c-Jun expression) and satiety (by controlling the secretion of NO, as mentioned in the main body of the review, and Figure 3 and Table 3 are referred for detailed information) in the body. Studies have demonstrated its close relation with NO and dopamine, while the detailed mechanism remains to be elucidated.
Circadian Rhythm Regulation in the Gastrointestinal Tract and its Interplays With Peroxisome Proliferator–Activated Receptor-α
Studies have found that circadian rhythms or circadian clock regulation is achieved via the expression of key genes and downstream pathways, as shown in Table 4. These genes take control of a broad range of physiological activities and share close interactions. One typical role of PPAR-α in this process lies in its activation of the clock gene via RORα and subsequent influence on the expression of E4BP4. However, as far as we are concerned, the current analysis focused on the roles of PPAR-α in the CVS, and more investigations focusing on its roles in GIT are recommended. Refer to Figure 3 for photographic illustration.
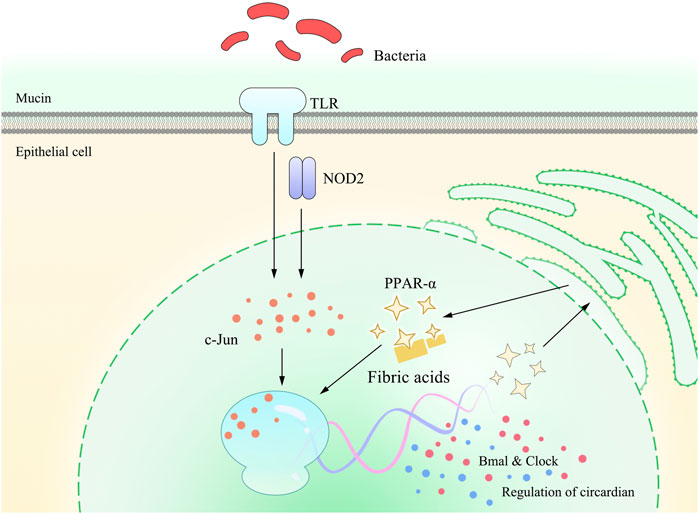
FIGURE 3. Pattern diagram of PPAR-α involved in TLRs and NOD2 on gastrointestinal circadian rhythms or circadian clock. Studies have found that gastrointestinal circadian rhythms or circadian clock is affected by the gut flora, which is mainly sensed via TLRs and NOD2. This stimulation is further detected by the c-Jun N-terminal kinase (JNK) and binds to the enhancer heptamer motif, resulting in the activation of PPAR-α and, in turn, activating the transcription of Bmal and Clock, which exert a direct impact on the regulation of gastrointestinal circadian rhythms or circadian clock.
Pharmacological Perspective of Peroxisome Proliferator–Activated Receptor-α
Numerous investigations have been put into the analysis of PPAR-α due to its wide distribution and multiple functions in a variety of tissues and cells (Berger and Moller, 2002). Long-chain fatty acids and their derivatives proved to be the main sources of natural PPAR-α agonists, while synthetic ones also play important roles in these studies (Kliewer et al., 1997). Although some agonists of PPAR-α have been used for treating different diseases, many of them are still in the experimental stage (Feng et al., 2016). Most clinical trials focused on their usage in metabolic diseases, especially those symptoms in the liver, while others concerning the gastrointestinal tract are relatively less (Petrosino et al., 2010). Also, considering the difference of distribution in humans and mice, more clinical trials are required in order to fully elucidate the mechanisms. Moreover, many exogenous nutrients and endogenous metabolites serve as ligands for PPAR-α while their functions and related dosage vary a lot. This increases the difficulty in developing clinical uses and requires further elucidation (O'Sullivan, 2016). However, we considered it worth the time and effort due to its potential usage in treating GIT diseases and decreasing the number of IBD patients all over the world.
Conclusion
PPAR-α has been recognized as an important regulator in the cardiovascular system and lipid metabolism. In addition, it also exerts substantial impacts on the GIT functions both physiologically and pathologically. Other than the well-known abilities to regulate lipid metabolism, PPAR-α mediates the process of inflammation via the regulation of cytokine secretion and activation of inflammatory pathways. Furthermore, the target genes of PPAR-α include those controlling gut circadian rhythms and the synthesis of NO, which could form an integrated regulatory network of GI functions. Meanwhile, many endogenous and exogenous food metabolites serve as agonists of PPAR-α, and their use in the treatment of GIT diseases is expected to shed light for a bright future.
Author Contributions
Y-XG and J-DX wrote the manuscript; B-YW, Y-XG, and HG designed the illustrations; C-WH, YW, and LG helped analyze the literature; J-DX revised the manuscript. All authors read and approved the final manuscript.
Funding
The research in the authors’ laboratory was supported by the National Natural Science Foundation of China (Grant Nos. 82174056 and 81673671).
Conflict of Interest
The authors declare that the research was conducted in the absence of any commercial or financial relationships that could be construed as a potential conflict of interest.
Publisher’s Note
All claims expressed in this article are solely those of the authors and do not necessarily represent those of their affiliated organizations, or those of the publisher, the editors and the reviewers. Any product that may be evaluated in this article, or claim that may be made by its manufacturer, is not guaranteed or endorsed by the publisher.
Acknowledgments
We appreciate Dr. Tao Xin from the Department of Applied Linguistics, School of Medical Humanities, Capital Medical University, for excellent language expression assistance.
References
Agarwal, N., Mishra, I., Rani, S., and Kumar, V. (2018). Temporal Expression of Clock Genes in central and Peripheral Tissues of Spotted Munia under Varying Light Conditions: Evidence for Circadian Regulation of Daily Physiology in a Non-photoperiodic Circannual Songbird Species. Chronobiology Int. 35, 617–632. doi:10.1080/07420528.2017.1422742
Ahmad, R., Sorrell, M. F., Batra, S. K., Dhawan, P., and Singh, A. B. (2017). Gut Permeability and Mucosal Inflammation: Bad, Good or Context Dependent. Mucosal Immunol. 10, 307–317. doi:10.1038/mi.2016.128
Aronsson, L., Huang, Y., Parini, P., Korach-André, M., Håkansson, J., Gustafsson, J. Å., et al. (2010). Decreased Fat Storage by Lactobacillus Paracasei Is Associated with Increased Levels of Angiopoietin-like 4 Protein (ANGPTL4). PLoS One 5. doi:10.1371/journal.pone.0013087
Ashrafian, F., Shahriary, A., Behrouzi, A., Moradi, H. R., Keshavarz Azizi Raftar, S., Lari, A., et al. (2019). Akkermansia Muciniphila-Derived Extracellular Vesicles as a Mucosal Delivery Vector for Amelioration of Obesity in Mice. Front. Microbiol. 10, 2155. doi:10.3389/fmicb.2019.02155
Berger, J., and Moller, D. E. (2002). The Mechanisms of Action of PPARs. Annu. Rev. Med. 53, 409–444. doi:10.1146/annurev.med.53.082901.104018
Bordet, R., Ouk, T., Petrault, O., Gelé, P., Gautier, S., Laprais, M., et al. (2006). PPAR: a New Pharmacological Target for Neuroprotection in Stroke and Neurodegenerative Diseases. Biochem. Soc. Trans. 34, 1341–1346. doi:10.1042/bst0341341
Borrelli, F., Romano, B., Petrosino, S., Pagano, E., Capasso, R., Coppola, D., et al. (2015). Palmitoylethanolamide, a Naturally Occurring Lipid, Is an Orally Effective Intestinal Anti-inflammatory Agent. Br. J. Pharmacol. 172, 142–158. doi:10.1111/bph.12907
Botta, M., Audano, M., Sahebkar, A., Sirtori, C. R., Mitro, N., and Ruscica, M. (2018). PPAR Agonists and Metabolic Syndrome: An Established Role? Int. J. Mol. Sci. 19. doi:10.3390/ijms19041197
Braissant, O., Foufelle, F., Scotto, C., Dauça, M., and Wahli, W. (1995). Differential Expression of Peroxisome ProliferatorActivated Receptors (PPARs): Tissue Distribution of PPAR-A, B, and -y in the Adult Rat. Endocrinology 137, 354–366. doi:10.1210/endo.137.1.8536636
Brown, J. D., Karimian Azari, E., and Ayala, J. E. (2017). Oleoylethanolamide: A Fat Ally in the Fight against Obesity. Physiol. Behav. 176, 50–58. doi:10.1016/j.physbeh.2017.02.034
Brown, J. D., McAnally, D., Ayala, J. E., Burmeister, M. A., Morfa, C., Smith, L., et al. (2018). Oleoylethanolamide Modulates Glucagon-like Peptide-1 Receptor Agonist Signaling and Enhances Exendin-4-Mediated Weight Loss in Obese Mice. Am. J. Physiology-Regulatory, Integr. Comp. Physiol. 315, R595–R608. doi:10.1152/ajpregu.00459.2017
Brown, M. A., Magee, L. A., Kenny, L. C., Karumanchi, S. A., McCarthy, F. P., Saito, S., et al. (2018). Hypertensive Disorders of Pregnancy. Hypertension 72, 24–43. doi:10.1161/hypertensionaha.117.10803
Bünger, M., van den Bosch, H. M., van der Meijde, J., Kersten, S., Hooiveld, G. J. E. J., and Müller, M. (2007). Genome-wide Analysis of PPARα Activation in Murine Small Intestine. Physiol. Genomics 30, 192–204. doi:10.1152/physiolgenomics.00198.2006
Cai, H., Wen, Z., Li, X., Meng, K., and Yang, P. (2020). Lactobacillus Plantarum FRT10 Alleviated High-Fat Diet-Induced Obesity in Mice through Regulating the PPARα Signal Pathway and Gut Microbiota. Appl. Microbiol. Biotechnol. 104, 5959–5972. doi:10.1007/s00253-020-10620-0
Cani, P. D., Montoya, M. L., Neyrinck, A. M., Delzenne, N. M., and Lambert, D. M. (2004). Potential Modulation of Plasma Ghrelin and Glucagon-like Peptide-1 by Anorexigenic Cannabinoid Compounds, SR141716A (Rimonabant) and Oleoylethanolamide. Br. J. Nutr. 92, 757–761. doi:10.1079/bjn20041256
Capasso, R., Izzo, A. A., Fezza, F., Pinto, A., Capasso, F., Mascolo, N., et al. (2001). Inhibitory Effect of Palmitoylethanolamide on Gastrointestinal Motility in Mice. Br. J. Pharmacol. 134, 945–950. doi:10.1038/sj.bjp.0704339
Capasso, R., Orlando, P., Pagano, E., Aveta, T., Buono, L., Borrelli, F., et al. (2014). Palmitoylethanolamide Normalizes Intestinal Motility in a Model of post-inflammatory Accelerated Transit: Involvement of CB1receptors and TRPV1 Channels. Br. J. Pharmacol. 171, 4026–4037. doi:10.1111/bph.12759
Cluny, N. L., Keenan, C. M., Lutz, B., Piomelli, D., and Sharkey, k. a. (2009). The Identification of Peroxisome Proliferator-Activated Receptor Alpha-independent Effects of Oleoylethanolamide on Intestinal Transit in Mice. Neurogastroenterology Motil. : official J. Eur. Gastrointest. Motil. Soc. 21, 420–429. doi:10.1111/j.1365-2982.2008.01248.x
Colin, S., Briand, O., Touche, V., Wouters, K., Baron, M., Pattou, F., et al. (2013). Activation of Intestinal Peroxisome Proliferator-Activated Receptor- Increases High-Density Lipoprotein Production. Eur. Heart J. 34, 2566–2574. doi:10.1093/eurheartj/ehs227
Colville-Nash, P. R., Qureshi, S. S., Willis, D., and Willoughby, D. A. (1998). Inhibition of Inducible Nitric Oxide Synthase by Peroxisome Proliferator-Activated Receptor Agonists: Correlation with Induction of Heme Oxygenase 1. J. Immunol. 161, 978–984.
Crakes, K. R., Santos Rocha, C., Grishina, I., Hirao, L. A., Napoli, E., Gaulke, C. A., et al. (2019). Pparα-Targeted Mitochondrial Bioenergetics Mediate Repair of Intestinal Barriers at the Host-Microbe Intersection during SIV Infection. Proc. Natl. Acad. Sci. U.S.A. 116, 24819–24829. doi:10.1073/pnas.1908977116
Cui, J. Y., Choudhuri, S., Knight, T. R., and Klaassen, C. D. (2010). Genetic and Epigenetic Regulation and Expression Signatures of Glutathione S-Transferases in Developing Mouse Liver. Toxicol. Sci. : official J. Soc. Toxicol. 116, 32–43. doi:10.1093/toxsci/kfq115
Cuzzocrea, S., Di Paola, R., Mazzon, E., Genovese, T., Muià, C., Centorrino, T., et al. (2004). Role of Endogenous and Exogenous Ligands for the Peroxisome Proliferators Activated Receptors Alpha (PPAR-α) in the Development of Inflammatory Bowel Disease in Mice. Lab. Invest. 84, 1643–1654. doi:10.1038/labinvest.3700185
Cuzzocrea, S., Mazzon, E., Dugo, L., Caputi, A. P., Riley, D. P., and Salvemini, D. (2001). Protective Effects of M40403, a Superoxide Dismutase Mimetic, in a Rodent Model of Colitis. Eur. J. Pharmacol. 432, 79–89. doi:10.1016/s0014-2999(01)01427-3
Desvergne, B., and Wahli, W. (1999). Peroxisome Proliferator-Activated Receptors: Nuclear Control of Metabolism. Endocr. Rev. 20 (5), 649–688. doi:10.1210/edrv.20.5.0380
Di Paola, M., Bonechi, E., Provensi, G., Costa, A., Clarke, G., Ballerini, C., et al. (2018). Oleoylethanolamide Treatment Affects Gut Microbiota Composition and the Expression of Intestinal Cytokines in Peyer's Patches of Mice. Sci. Rep. 8, 14881. doi:10.1038/s41598-018-32925-x
Di Paola, R., Impellizzeri, D., Torre, A., Mazzon, E., Cappellani, A., Faggio, C., et al. (2012). Effects of Palmitoylethanolamide on Intestinal Injury and Inflammation Caused by Ischemia-Reperfusion in Mice. J. Leukoc. Biol. 91, 911–920. doi:10.1189/jlb.0911485
DiPatrizio, N. V., and Piomelli, D. (2015). Intestinal Lipid-Derived Signals that Sense Dietary Fat. J. Clin. Invest. 125, 891–898. doi:10.1172/jci76302
Dreyer, C., Krey, G., Keller, H., Givel, F., Helftenbein, G., and Wahli, W. (1992). Control of the Peroxisomal β-oxidation Pathway by a Novel Family of Nuclear Hormone Receptors. Cell 68, 879–887. doi:10.1016/0092-8674(92)90031-7
Duparc, T., Plovier, H., Marrachelli, V. G., Van Hul, M., Essaghir, A., Ståhlman, M., et al. (2017). Hepatocyte MyD88 Affects Bile Acids, Gut Microbiota and Metabolome Contributing to Regulate Glucose and Lipid Metabolism. Gut 66, 620–632. doi:10.1136/gutjnl-2015-310904
Escher, P., Braissant, O., Basu-Modak, S., Michalik, L., Wahli, W., and Desvergne, B. (2001). Rat PPARs: Quantitative Analysis in Adult Rat Tissues and Regulation in Fasting and Refeeding. Endocrinology 142, 4195–4202. doi:10.1210/endo.142.10.8458
Esposito, G., Capoccia, E., Turco, F., Palumbo, I., Lu, J., Steardo, A., et al. (2014). Palmitoylethanolamide Improves colon Inflammation through an Enteric Glia/toll like Receptor 4-dependent PPAR-α Activation. Gut 63, 1300–1312. doi:10.1136/gutjnl-2013-305005
Fedele, S., Arnold, M., Krieger, J. P., Wolfstädter, B., Meyer, U., Langhans, W., et al. (2018). Oleoylethanolamide-induced Anorexia in Rats Is Associated with Locomotor Impairment. Physiol. Rep. 6. doi:10.14814/phy2.13517
Feng, X., Gao, X., Jia, Y., Zhang, H., Xu, Y., and Wang, G. (2016). PPAR-α Agonist Fenofibrate Decreased RANTES Levels in Type 2 Diabetes Patients with Hypertriglyceridemia. Med. Sci. Monit. 22, 743–751. doi:10.12659/msm.897307
Forman, B. M., Chen, J., and Evans, R. M. (1997). Hypolipidemic Drugs, Polyunsaturated Fatty Acids, and Eicosanoids Are Ligands for Peroxisome Proliferator-Activated Receptors Alpha and delta. Proc. Natl. Acad. Sci. U S A. 94, 4312–4317. doi:10.1073/pnas.94.9.4312
Fu, J., Gaetani, S., Oveisi, F., Lo Verme, J., Serrano, A., Rodríguez de Fonseca, F., et al. (2003). Oleylethanolamide Regulates Feeding and Body Weight through Activation of the Nuclear Receptor PPAR-α. Nature 425, 90–93. doi:10.1038/nature01921
Goikoetxea, M. J., Beaumont, J., and Díez, J. (2004). Peroxisome Proliferator-Activated Receptor Alpha and Hypertensive Heart Disease. Drugs 64 (Suppl. 2), 9–18. doi:10.2165/00003495-200464002-00003
Haidari, S., Tröltzsch, M., Knösel, T., Liokatis, P., Kasintsova, A., Eberl, M., et al. (2021). Fatty Acid Receptor CD36 Functions as a Surrogate Parameter for Lymph Node Metastasis in Oral Squamous Cell Carcinoma. Cancers (Basel) 13. doi:10.3390/cancers13164125
Haila, S., Hästbacka, J., Böhling, T., Karjalainen–Lindsberg, M.-L., Kere, J., and Saarialho–Kere, U. (2001). SLC26A2 (Diastrophic Dysplasia Sulfate Transporter) Is Expressed in Developing and Mature Cartilage but Also in Other Tissues and Cell Types. J. Histochem. Cytochem. 49, 973–982. doi:10.1177/002215540104900805
Hankir, M. K., Seyfried, F., Hintschich, C. A., Diep, T.-A., Kleberg, K., Kranz, M., et al. (2017). Gastric Bypass Surgery Recruits a Gut PPAR-α-Striatal D1R Pathway to Reduce Fat Appetite in Obese Rats. Cel Metab. 25, 335–344. doi:10.1016/j.cmet.2016.12.006
Hasan, A. U., Rahman, A., and Kobori, H. (2019). Interactions between Host PPARs and Gut Microbiota in Health and Disease. Int. J. Mol. Sci. 20. doi:10.3390/ijms20020387
Holehouse, E. L., Liu, M.-L., and Aponte, G. W. (1998). Oleic Acid Distribution in Small Intestinal Epithelial Cells Expressing Intestinal-Fatty Acid Binding Protein. Biochim. Biophys. Acta (Bba) - Lipids Lipid Metab. 1390, 52–64. doi:10.1016/s0005-2760(97)00176-8
Hutch, C. R., Trakimas, D. R., Roelofs, K., Pressler, J., Sorrell, J., Cota, D., et al. (2020). Oea Signaling Pathways and the Metabolic Benefits of Vertical Sleeve Gastrectomy. Ann. Surg. 271, 509–518. doi:10.1097/sla.0000000000003093
Igarashi, M., Narayanaswami, V., Kimonis, V., Galassetti, P. M., Oveisi, F., Jung, K.-M., et al. (2017). Dysfunctional Oleoylethanolamide Signaling in a Mouse Model of Prader-Willi Syndrome. Pharmacol. Res. 117, 75–81. doi:10.1016/j.phrs.2016.12.024
Ikawa, H., Kameda, H., Kamitani, H., Baek, S. J., Nixon, J. B., Hsi, L. C., et al. (2001). Effect of PPAR Activators on Cytokine-Stimulated Cyclooxygenase-2 Expression in Human Colorectal Carcinoma Cells. Exp. Cel Res. 267, 73–80. doi:10.1006/excr.2001.5233
Issemann, I., and Green, S. (1990). Activation of a Member of the Steroid Hormone Receptor Superfamily by Peroxisome Proliferators. Nature 347, 645. doi:10.1038/347645a0
Jackson, L., Wahli, W., Michalik, L., Watson, S. A., Morris, T., Anderton, K., et al. (2003). Potential Role for Peroxisome Proliferator Activated Receptor (PPAR) in Preventing colon Cancer. Gut 52, 1317–1322. doi:10.1136/gut.52.9.1317
Jin, L., Li, L., Hu, C., Paez-Cortez, J., Bi, Y., Macoritto, M., et al. (2019). Integrative Analysis of Transcriptomic and Proteomic Profiling in Inflammatory Bowel Disease Colon Biopsies. Inflamm. Bowel Dis. 25, 1906–1918. doi:10.1093/ibd/izz111
Kamalian, A., Asl, M. S., Dolatshahi, M., Afshari, K., Shamshiri, S., Roudsari, N. M., et al. (2020). Interventions of Natural and Synthetic Agents in Inflammatory Bowel Disease, Modulation of Nitric Oxide Pathways. World J. Gastroenterol. 26, 3365–3400. doi:10.3748/wjg.v26.i24.3365
Karimian Azari, E., Leitner, C., Jaggi, T., Langhans, W., and Mansouri, A. (2013). Possible Role of Intestinal Fatty Acid Oxidation in the Eating-Inhibitory Effect of the PPAR-α Agonist Wy-14643 in High-Fat Diet Fed Rats. PLoS One 8, e74869. doi:10.1371/journal.pone.0074869
Karwad, M. A., Macpherson, T., Wang, B., Theophilidou, E., Sarmad, S., Barrett, D. A., et al. (2017). Oleoylethanolamine and Palmitoylethanolamine Modulate Intestinal Permeability In Vitro via TRPV1 and PPARα. FASEB j. 31, 469–481. doi:10.1096/fj.201500132
Kersten, S., Seydoux, J., Peters, J. M., Gonzalez, F. J., Desvergne, B., and Wahli, W. (1999). Peroxisome Proliferator-Activated Receptor α Mediates the Adaptive Response to Fasting. J. Clin. Invest. 103, 1489–1498. doi:10.1172/jci6223
Killig, M., Glatzer, T., and Romagnani, C. (2014). Recognition Strategies of Group 3 Innate Lymphoid Cells. Front. Immunol. 5, 142. doi:10.3389/fimmu.2014.00142
Kliewer, S. A., Sundseth, S. S., Jones, S. A., Brown, P. J., Wisely, G. B., Koble, C. S., et al. (1997). Fatty Acids and Eicosanoids Regulate Gene Expression through Direct Interactions with Peroxisome Proliferator-Activated Receptors α and γ. Proc. Natl. Acad. Sci. U.S.A. 94, 4318–4323. doi:10.1073/pnas.94.9.4318
Knight, B. L., Patel, D. D., Humphreys, S. M., Wiggins, D., and Gibbons, G. F. (2003). Inhibition of Cholesterol Absorption Associated with a PPARα-dependent Increase in ABC Binding Cassette Transporter A1 in Mice. J. lipid Res. 44, 2049–2058. doi:10.1194/jlr.m300042-jlr200
Koethe, D., Schreiber, D., Giuffrida, A., Mauss, C., Faulhaber, J., Heydenreich, B., et al. (2009). Sleep Deprivation Increases Oleoylethanolamide in Human Cerebrospinal Fluid. J. Neural Transm. (Vienna) 116, 301–305. doi:10.1007/s00702-008-0169-6
Kokesova, A., Coufal, S., Frybova, B., Kverka, M., and Rygl, M. (2019). The Intestinal Fatty Acid-Binding Protein as a Marker for Intestinal Damage in Gastroschisis. PLoS One 14, e0210797. doi:10.1371/journal.pone.0210797
Kondo, H., Minegishi, Y., Komine, Y., Mori, T., Matsumoto, I., Abe, K., et al. (2006). Differential Regulation of Intestinal Lipid Metabolism-Related Genes in Obesity-Resistant A/J vs. Obesity-Prone C57BL/6J Mice. Am. J. Physiology-Endocrinology Metab. 291, E1092–E1099. doi:10.1152/ajpendo.00583.2005
Kong, L., Chen, J., Ji, X., Qin, Q., Yang, H., Liu, D., et al. (2021). Alcoholic Fatty Liver Disease Inhibited the Co-expression of Fmo5 and PPARα to Activate the NF-Κb Signaling Pathway, Thereby Reducing Liver Injury via Inducing Gut Microbiota Disturbance. J. Exp. Clin. Cancer Res. 40, 18. doi:10.1186/s13046-020-01782-w
Laleh, P., Yaser, K., and Alireza, O. (2019). Oleoylethanolamide: A Novel Pharmaceutical Agent in the Management of Obesity‐an Updated Review. J. Cell Physiol. 234, 7893–7902. doi:10.1002/jcp.27913
Lee, H. J., Jung, H., Cho, H., Lee, K., and Hwang, K. T. (2018). Black Raspberry Seed Oil Improves Lipid Metabolism by Inhibiting Lipogenesis and Promoting Fatty-Acid Oxidation in High-Fat Diet-Induced Obese Mice and Db/db Mice. Lipids 53, 491–504. doi:10.1002/lipd.12050
Lee, J. W., Bajwa, P. J., Carson, M. J., Jeske, D. R., Cong, Y., Elson, C. O., et al. (2007). Fenofibrate Represses Interleukin-17 and Interferon-γ Expression and Improves Colitis in Interleukin-10-Deficient Mice. Gastroenterology 133, 108–123. doi:10.1053/j.gastro.2007.03.113
Lefebvre, P., Chinetti, G., Fruchart, J. C., and Staels, B. (2006). Sorting Out the Roles of PPAR in Energy Metabolism and Vascular Homeostasis. J. Clin. Invest. 116, 571–580. doi:10.1172/jci27989
Lim, K.-H., and Staudt, L. M. (2013). Toll-like Receptor Signaling. Cold Spring Harbor Perspect. Biol. 5, a011247. doi:10.1101/cshperspect.a011247
Liu, C., Li, S., Liu, T., Borjigin, J., and Lin, J. D. (2007). Transcriptional Coactivator PGC-1α Integrates the Mammalian Clock and Energy Metabolism. Nature 447, 477–481. doi:10.1038/nature05767
Lo Verme, J., Fu, J., Astarita, G., La Rana, G., Russo, R., Calignano, A., et al. (2005). The Nuclear Receptor Peroxisome Proliferator-Activated Receptor-α Mediates the Anti-inflammatory Actions of Palmitoylethanolamide. Mol. Pharmacol. 67, 15–19. doi:10.1124/mol.104.006353
Long, T., Liu, Y., Qin, Y., DeBose-Boyd, R. A., and Li, X. (2021). Structures of Dimeric Human NPC1L1 Provide Insight into Mechanisms for Cholesterol Absorption. Sci. Adv. 7, 3997. doi:10.1126/sciadv.abh3997
Losacco, M. C., de Almeida, C. F. T., Hijo, A. H. T., Bargi-Souza, P., Gama, P., Nunes, M. T., et al. (2018). High-fat Diet Affects Gut Nutrients Transporters in Hypo and Hyperthyroid Mice by PPAR-A Independent Mechanism. Life Sci. 202, 35–43. doi:10.1016/j.lfs.2018.03.053
Ma, S., Lei, Y., Zhang, L., and Wang, J. (2018). Research on the Inhibiting Effect of Tanshinone IIA on colon Cancer Cell Growth via COX-2-Wnt/β-Catenin Signaling Pathway. J. BUON 23, 1337–1342.
Mallordy, A., Poirier, H., Besnard, P., Niot, I., and Carlier, H. (1995). Evidence for Transcriptional Induction of the Liver Fatty-Acid-Binding-Protein Gene by Bezafibrate in the Small Intestine. Eur. J. Biochem. 227, 801–807. doi:10.1111/j.1432-1033.1995.tb20204.x
Maltarollo, V. G., Kronenberger, T., Windshugel, B., Wrenger, C., Trossini, G. H. G., and Honorio, K. M. (2018). Advances and Challenges in Drug Design of PPARδ Ligands. Curr. Drug. Targets. 19, 144–154. doi:10.2174/1389450118666170414113159
Mandard, S., Müller, M., and Kersten, S. (2004). Peroxisome Proliferator-Activated Receptor a Target Genes. Cell Mol. Life Sci. (Cmls) 61, 393–416. doi:10.1007/s00018-003-3216-3
Manoharan, I., Suryawanshi, A., Hong, Y., Ranganathan, P., Shanmugam, A., Ahmad, S., et al. (2016). Homeostatic PPARα Signaling Limits Inflammatory Responses to Commensal Microbiota in the Intestine. J.I. 196, 4739–4749. doi:10.4049/jimmunol.1501489
Mansén, A., Guardiola-Diaz, H., Rafter, J., Branting, C., and Gustafsson, J.-Å. (1996). Expression of the Peroxisome Proliferator-Activated Receptor (PPAR) in the Mouse Colonic Mucosa. Biochem. biophysical Res. Commun. 222, 844–851. doi:10.1006/bbrc.1996.0832
Mastinu, A., Pira, M., Pani, L., Pinna, G. A., and Lazzari, P. (2012). NESS038C6, a Novel Selective CB1 Antagonist Agent with Anti-obesity Activity and Improved Molecular Profile. Behav. Brain Res. 234, 192–204. doi:10.1016/j.bbr.2012.06.033
Meade, E. A., McIntyre, T. M., Zimmerman, G. A., and Prescott, S. M. (1999). Peroxisome Proliferators Enhance Cyclooxygenase-2 Expression in Epithelial Cells. J. Biol. Chem. 274, 8328–8334. doi:10.1074/jbc.274.12.8328
Mochizuki, K., Suruga, K., Yagi, E., Takase, S., and Goda, T. (2001). The Expression of PPAR-Associated Genes Is Modulated through Postnatal Development of PPAR Subtypes in the Small Intestine. Biochim. Biophys. Acta (Bba) - Mol. Cel Biol. Lipids 1531, 68–76. doi:10.1016/s0167-4889(01)00071-4
Motojima, K., Passilly, P., Peters, J. M., Gonzalez, F. J., and Latruffe, N. (1998). Expression of Putative Fatty Acid Transporter Genes Are Regulated by Peroxisome Proliferator-Activated Receptor α and γ Activators in a Tissue- and Inducer-specific Manner. J. Biol. Chem. 273, 16710–16714. doi:10.1074/jbc.273.27.16710
Mukherji, A., Kobiita, A., Ye, T., and Chambon, P. (2013). Homeostasis in Intestinal Epithelium Is Orchestrated by the Circadian Clock and Microbiota Cues Transduced by TLRs. Cell 153, 812–827. doi:10.1016/j.cell.2013.04.020
Nielsen, M. J., Petersen, G., Astrup, A., and Hansen, H. S. (2004). Food Intake Is Inhibited by Oral Oleoylethanolamide. J. lipid Res. 45, 1027–1029. doi:10.1194/jlr.c300008-jlr200
O'Sullivan, S. E. (2016). An Update on PPAR Activation by Cannabinoids. Br. J. Pharmacol. 173, 1899–1910. doi:10.1111/bph.13497
Obici, S., Feng, Z., Morgan, K., Stein, D., Karkanias, G., and Rossetti, L. (2002). Central Administration of Oleic Acid Inhibits Glucose Production and Food Intake. Diabetes 51, 271–275. doi:10.2337/diabetes.51.2.271
Ochiai, Y., Uchida, Y., Tachikawa, M., Couraud, P. O., and Terasaki, T. (2019). Amyloid Beta25‐35impairs Docosahexaenoic Acid Efflux by Down‐regulating Fatty Acid Transport Protein 1 (FATP1/SLC27A1) Protein Expression in Human Brain Capillary Endothelial Cells. J. Neurochem. 150, 385–401. doi:10.1111/jnc.14722
Okamura-Oho, Y. (2004). Perturbation Due to Environmental Stress. Tanpakushitsu Kakusan Koso 49, 2850–2854.
Otagiri, S., Ohnishi, S., Ohara, M., Fu, Q., Yamamoto, K., Yamamoto, K., et al. (2020). Oleoylethanolamide Ameliorates Dextran Sulfate Sodium-Induced Colitis in Rats. Front. Pharmacol. 11, 1277. doi:10.3389/fphar.2020.01277
Pan, X., Schwartz, G. J., and Hussain, M. M. (2018). Oleoylethanolamide Differentially Regulates Glycerolipid Synthesis and Lipoprotein Secretion in Intestine and Liver. J. lipid Res. 59, 2349–2359. doi:10.1194/jlr.m089250
Petrosino, S., Iuvone, T., and Di Marzo, V. (2010). N-palmitoyl-ethanolamine: Biochemistry and New Therapeutic Opportunities. Biochimie 92, 724–727. doi:10.1016/j.biochi.2010.01.006
Piomelli, D. (2013). A Fatty Gut Feeling. Trends Endocrinol. Metab. 24, 332–341. doi:10.1016/j.tem.2013.03.001
Poirier, H., Niot, I., Degrace, P., Monnot, M. C., Bernard, A., and Besnard, P. (1997). Fatty Acid Regulation of Fatty Acid-Binding Protein Expression in the Small Intestine. Am. J. Physiol. 273, G289. doi:10.1152/ajpgi.1997.273.2.G289
Poirier, H., Degrace, P., Niot, I., Bernard, A., and Besnard, P. (1996). Localization and Regulation of the Putative Membrane Fatty-Acid Transporter (FAT) in the Small Intestine. Comparison with Fatty Acid-Binding Proteins (FABP). Eur. J. Biochem. 238, 368–373. doi:10.1111/j.1432-1033.1996.0368z.x
Pollinger, J., and Merk, D. (2017). Therapeutic Applications of the Versatile Fatty Acid Mimetic WY14643. Expert Opin. Ther. patents 27, 517–525. doi:10.1080/13543776.2017.1272578
Prabhat, A., Malik, I., Jha, N. A., Bhardwaj, S. K., and Kumar, V. (2020). Developmental Effects of Constant Light on Circadian Behaviour and Gene Expressions in Zebra Finches: Insights into Mechanisms of Metabolic Adaptation to Aperiodic Environment in Diurnal Animals. J. Photochem. Photobiol. B: Biol. 211, 111995. doi:10.1016/j.jphotobiol.2020.111995
Prescott, S. M., and White, R. L. (1996). Self-Promotion? Intimate Connections between APC and Prostaglandin H Synthase-2. Cell 87, 783–786. doi:10.1016/s0092-8674(00)81983-2
Riccardi, L., Mazzon, E., Bruscoli, S., Esposito, E., Crisafulli, C., Di Paola, R., et al. (2009). Peroxisome Proliferator-Activated Receptor-Alpha Modulates the Anti-inflammatory Effect of Glucocorticoids in a Model of Inflammatory Bowel Disease in Mice. Shock (Augusta, Ga.) 31, 308–316. doi:10.1097/shk.0b013e31818339e7
Ridaura, V., and Belkaid, Y. (2015). Gut Microbiota: the Link to Your Second Brain. Cell 161, 193–194. doi:10.1016/j.cell.2015.03.033
R. Moschen, A., Wieser, V., and Tilg, H. (2012). Adiponectin: Key Player in the Adipose Tissue-Liver Crosstalk. Curr. Med. Chem. 19, 5467–5473. doi:10.2174/092986712803833254
Roberts, W. C. (1989). Safety of Fenofibrate – US and Worldwide Experience. Cardiology 76, 169–179. doi:10.1159/000174488
Rodríguez de Fonseca, F., Navarro, M., Gómez, R., Escuredo, L., Nava, F., Fu, J., et al. (2001). An Anorexic Lipid Mediator Regulated by Feeding. Nature 414, 209–212. doi:10.1038/35102582
Roediger, W. E., and Nance, S. (1986). Metabolic Induction of Experimental Ulcerative Colitis by Inhibition of Fatty Acid Oxidation. Br. J. Exp. Pathol. 67, 773–782.
Rooks, M. G., and Garrett, W. S. (2016). Gut Microbiota, Metabolites and Host Immunity. Nat. Rev. Immunol. 16, 341–352. doi:10.1038/nri.2016.42
Sarnelli, G., Gigli, S., Capoccia, E., Iuvone, T., Cirillo, C., Seguella, L., et al. (2016). Palmitoylethanolamide Exerts Antiproliferative Effect and Downregulates VEGF Signaling in Caco-2 Human Colon Carcinoma Cell Line through a Selective PPAR-α-dependent Inhibition of Akt/mTOR Pathway. Phytother. Res. 30, 963–970. doi:10.1002/ptr.5601
Sasaki, Y., Raza-Iqbal, S., Tanaka, T., Murakami, K., Anai, M., Osawa, T., et al. (2019). Gene Expression Profiles Induced by a Novel Selective Peroxisome Proliferator-Activated Receptor α Modulator (SPPARMα) Pemafibrate. Int. J. Mol. Sci. 20. doi:10.3390/ijms20225682
Sérée, E., Villard, P.-H., Pascussi, J.-M., Pineau, T., Maurel, P., Nguyen, Q. B., et al. (2004). Evidence for a New Human CYP1A1 Regulation Pathway Involving PPAR-α and 2 PPRE Sites. Gastroenterology 127, 1436–1445. doi:10.1053/j.gastro.2004.08.023
Shih, M.-C., Hsu, N.-C., Huang, C.-C., Wu, T.-S., Lai, P.-Y., and Chung, B.-c. (2008). Mutation of MouseCyp11a1Promoter Caused Tissue-specific Reduction of Gene Expression and Blunted Stress Response without Affecting Reproduction. Mol. Endocrinol. 22, 915–923. doi:10.1210/me.2007-0222
Shimakura, J., Terada, T., Saito, H., Katsura, T., and Inui, K.-i. (2006). Induction of Intestinal Peptide Transporter 1 Expression during Fasting Is Mediated via Peroxisome Proliferator-Activated Receptor α. Am. J. Physiol. Gastrointest. Liver Physiol. 291, G851–G856. doi:10.1152/ajpgi.00171.2006
Shivapurkar, N., Huang, L., Ruggeri, B., Swalsky, P. A., Bakker, A., Finkelstein, S., et al. (1997). K-ras and P53 Mutations in Aberrant Crypt Foci and Colonic Tumors from colon Cancer Patients. Cancer Lett. 115, 39–46. doi:10.1016/s0304-3835(97)04709-5
Sihag, J., and Jones, P. J. H. (2018). Oleoylethanolamide: The Role of a Bioactive Lipid Amide in Modulating Eating Behaviour. Obes. Rev. 19, 178–197. doi:10.1111/obr.12630
Sivaprakasam, S., Bhutia, Y. D., Yang, S., and Ganapathy, V. (2017). Short‐Chain Fatty Acid Transporters: Role in Colonic Homeostasis. Compr. Physiol. 8, 299–314. doi:10.1002/cphy.c170014
Skaper, S. D., Facci, L., Barbierato, M., Zusso, M., Bruschetta, G., Impellizzeri, D., et al. (2015). N-palmitoylethanolamine and Neuroinflammation: a Novel Therapeutic Strategy of Resolution. Mol. Neurobiol. 52, 1034–1042. doi:10.1007/s12035-015-9253-8
Stack, W., Mann, S., Roy, A., Heath, P., Sopwith, M., Freeman, J., et al. (1997). Randomised Controlled Trial of CDP571 Antibody to Tumour Necrosis Factor-α in Crohn's Disease. The Lancet 349, 521–524. doi:10.1016/s0140-6736(97)80083-9
Stavinoha, M. A., RaySpellicy, J. W., Essop, M. F., Graveleau, C., Abel, E. D., Hart-Sailors, M. L., et al. (2004). Evidence for Mitochondrial Thioesterase 1 as a Peroxisome Proliferator-Activated Receptor-α-Regulated Gene in Cardiac and Skeletal Muscle. Am. J. Physiology-Endocrinology Metab. 287, E888–E895. doi:10.1152/ajpendo.00190.2004
Steineger, H. H., Sorensen, H. N., Tugwood, J. D., Skrede, S., Spydevold, O., and Gautvik, K. M. (1994). Dexamethasone and Insulin Demonstrate Marked and Opposite Regulation of the Steady-State mRNA Level of the Peroxisomal Proliferator-Activated Receptor (PPAR) in Hepatic Cells. Hormonal Modulation of Fatty-Acid-Induced Transcription. Eur. J. Biochem. 225, 967–974. doi:10.1111/j.1432-1033.1994.0967b.x
Suzuki, T., Mochizuki, K., and Goda, T. (2009). Localized Expression of Genes Related to Carbohydrate and Lipid Absorption along the Crypt-Villus axis of Rat Jejunum. Biochim. Biophys. Acta (Bba) - Gen. Subjects 1790, 1624–1635. doi:10.1016/j.bbagen.2009.08.004
Tan, B., Tang, H., Xu, Y., Chen, M. J., Wang, M. Z., and Qian, J. M. (2020). Protective Effect and Mechanism of Lactobacillus Rhamnosus on Immune Checkpoint Inhibitors Related Colitis in Mice. Zhonghua yi xue za zhi 100, 3332–3337. doi:10.3760/cma.j.cn112137-20200520-01598
Tanaka, T., Kohno, H., Yoshitani, S., Takashima, S., Okumura, A., Murakami, A., et al. (2001). Ligands for Peroxisome Proliferator-Activated Receptors and Chemically Induced Colitis and Formation of Aberrant Crypt Foci in Rats. Cancer Res. 61, 2424–2428.
Tognini, P., Murakami, M., Liu, Y., Eckel-Mahan, K. L., Newman, J. C., Verdin, E., et al. (2017). Distinct Circadian Signatures in Liver and Gut Clocks Revealed by Ketogenic Diet. Cel Metab. 26, 523–538. e5. doi:10.1016/j.cmet.2017.08.015
Uchida, A., Slipchenko, M. N., Cheng, J.-X., and Buhman, K. K. (2011). Fenofibrate, a Peroxisome Proliferator-Activated Receptor α Agonist, Alters Triglyceride Metabolism in Enterocytes of Mice. Biochim. Biophys. Acta (Bba) - Mol. Cel Biol. Lipids 1811, 170–176. doi:10.1016/j.bbalip.2010.12.011
Usuda, D., and Kanda, T. (2014). Peroxisome Proliferator-Activated Receptors for Hypertension. World J. Cardiol. 6, 744–754. doi:10.4330/wjc.v6.i8.744
Valasek, M. A., Clarke, S. L., and Repa, J. J. (2007). Fenofibrate Reduces Intestinal Cholesterol Absorption via PPARα-dependent Modulation of NPC1L1 Expression in Mouse. J. lipid Res. 48, 2725–2735. doi:10.1194/jlr.m700345-jlr200
Valizadeh, M., Aghasizadeh, M., Nemati, M., Hashemi, M., Aghaee-Bakhtiari, S. H., Zare-Feyzabadi, R., et al. (2021). The Association between a Fatty Acid Binding Protein 1 (FABP1) Gene Polymorphism and Serum Lipid Abnormalities in the MASHAD Cohort Study. Prostaglandins, Leukot. Essent. Fatty Acids 172, 102324. doi:10.1016/j.plefa.2021.102324
van den Bosch, H. M., Bünger, M., de Groot, P. J., van der Meijde, J., Hooiveld, G. J., and Müller, M. (2007). Gene Expression of Transporters and Phase I/II Metabolic Enzymes in Murine Small Intestine during Fasting. BMC genomics 8, 267. doi:10.1186/1471-2164-8-267
Vluggens, A., Andreoletti, P., Viswakarma, N., Jia, Y., Matsumoto, K., Kulik, W., et al. (2010). Functional Significance of the Two ACOX1 Isoforms and Their Crosstalks with PPARα and RXRα. Lab. Invest. 90, 696–708. doi:10.1038/labinvest.2010.46
Voigt, R. M., Forsyth, C. B., Green, S. J., Engen, P. A., and Keshavarzian, A. (2016). Circadian Rhythm and the Gut Microbiome. Int. Rev. Neurobiol. 131, 193–205. doi:10.1016/bs.irn.2016.07.002
Wang, L., Tang, Y., Rubin, D. C., and Levin, M. S. (2007). Chronically Administered Retinoic Acid Has Trophic Effects in the Rat Small Intestine and Promotes Adaptation in a Resection Model of Short Bowel Syndrome. Am. J. Physiology-Gastrointestinal Liver Physiol. 292, G1559–G1569. doi:10.1152/ajpgi.00567.2006
Wei, H.-X., Wang, B., and Li, B. (2020). IL-10 and IL-22 in Mucosal Immunity: Driving Protection and Pathology. Front. Immunol. 11, 1315. doi:10.3389/fimmu.2020.01315
Windsor, J. W., and Kaplan, G. G. (2019). Evolving Epidemiology of IBD. Curr. Gastroenterol. Rep. 21, 40. doi:10.1007/s11894-019-0705-6
Wu, J., Li, Y., Yu, J., Gan, Z., Wei, W., Wang, C., et al. (2020). Resveratrol Attenuates High-Fat Diet Induced Hepatic Lipid Homeostasis Disorder and Decreases m6A RNA Methylation. Front. Pharmacol. 11, 568006. doi:10.3389/fphar.2020.568006
Yaguchi, J., and Yaguchi, S. (2019). Evolution of Nitric Oxide Regulation of Gut Function. Proc. Natl. Acad. Sci. U.S.A. 116, 5607–5612. doi:10.1073/pnas.1816973116
Yang, F., Wang, D., Li, Y., Sang, L., Zhu, J., Wang, J., et al. (2017). Th1/Th2 Balance and Th17/Treg-Mediated Immunity in Relation to Murine Resistance to Dextran Sulfate-Induced Colitis. J. Immunol. Res. 2017, 7047201. doi:10.1155/2017/7047201
Yoshizaki, Y., Kawasaki, C., Cheng, K.-C., Ushikai, M., Amitani, H., Asakawa, A., et al. (2014). Ricekojireduced Body Weight Gain, Fat Accumulation, and Blood Glucose Level in High-Fat Diet-Induced Obese Mice. PeerJ 2, e540. doi:10.7717/peerj.540
Yu, F., Zhang, T., Zhou, C., Xu, H., Guo, L., Chen, M., et al. (2019). The Circadian Clock Gene Bmal1 Controls Intestinal Exporter MRP2 and Drug Disposition. Theranostics 9, 2754–2767. doi:10.7150/thno.33395
Yu, S.-Y., Hsiao, C.-T., Izawa, M., Yusa, A., Ishida, H., Nakamura, S., et al. (2018). Distinct Substrate Specificities of Human GlcNAc-6-Sulfotransferases Revealed by Mass Spectrometry-Based Sulfoglycomic Analysis. J. Biol. Chem. 293, 15163–15177. doi:10.1074/jbc.ra118.001937
Zhang, Z., Yi, P., Tu, C., Zhan, J., Jiang, L., and Zhang, F. (2019). Curcumin Inhibits ERK/c-Jun Expressions and Phosphorylation against Endometrial Carcinoma. Biomed. Res. Int. 2019, 8912961. doi:10.1155/2019/8912961
Zheng, Y., Valdez, P. A., Danilenko, D. M., Hu, Y., Sa, S. M., Gong, Q., et al. (2008). Interleukin-22 Mediates Early Host Defense against Attaching and Effacing Bacterial Pathogens. Nat. Med. 14, 282–289. doi:10.1038/nm1720
Glossary
ABCA1 ATP-binding cassette transporter A1 ATP-binding cassette, sub-family A, member 1
ABCA1 ATP-binding cassette transporter A1 ATP-binding cassette, sub-family A, member 1
ACF aberrant crypt foci
ANGPTL4, a circulating lipoprotein lipase angiopoietin-like 4
Cd36 fatty acid translocase
CD36 cluster of differentiation 36
CGX celiac superior mesenteric ganglionectomy
Chst4 carbohydrate (chondroitin 6/keratan) sulfotransferase 4
CNS central nervous system
COX-2 cyclooxygenase-2
CPT 1A1 cytochrome P450 1A1
CVS cardiovascular system
Cypt4a10 cytochrome P450, family 4, subfamily a, polypeptide 10
Dgat1 diacylglycerol acyltransferase 1
DSS dextran sodium sulfate
Dtd Slc26a2 solute carrier family 26 (sulfate transporter), member 2
ERS endoplasmic reticulum stress
F19 paracasei ssp paracasei F19
FAT fatty acid translocase
FATP fatty acid transport protein
Fatp4 fatty acid transport protein 4
GCs glucocorticoids
GFT505 elafibranor
GIT gastrointestinal tract
IBD intestinal bowel diseases
IFABPs intestinal fatty acid–binding proteins
IL-1b interleukin-1b
iNOS inducible nitric oxide synthase
IP intraperitoneal
LBD ligand-binding domain
LCFAs long-chain fatty acids
mAspAT mitochondrial aspartate aminotransferase
Mgst1 microsomal glutathione S-transferase 1
Mttp microsomal triglyceride transfer protein
Mφ macrophages
NO nitric oxide
OEA oleoylethanolamide
PEA palmitoylethanolamine
PPAR peroxisome proliferator–activated receptor
PPAR-αKO PPAR-α-knockout mice
RXR; NR2B retinoid X receptor
RYGB Roux-en-Y gastric bypass
SAO splanchnic artery occlusion
SDA subdiaphragmatic vagal deafferentation
Sert Slc6a4 solute carrier family 6 (neurotransmitter transporter, serotonin), member 4
SIV simian immunodeficiency virus
Slc25a36 solute carrier family 25, member 36
Smct1 Slc5a8 solute carrier family 5 (iodide transporter), member 8
TNF-a tumor necrosis factor-alpha
Wy-14643 pirinixic acid
Keywords: peroxisome proliferator–activated receptor (PPAR)-α, gastrointestinal diseases, metabolism, transcription, disorder
Citation: Guo Y-X, Wang B-Y, Gao H, Hua R-X, Gao L, He C-W, Wang Y and Xu J-D (2022) Peroxisome Proliferator–Activated Receptor-α: A Pivotal Regulator of the Gastrointestinal Tract. Front. Mol. Biosci. 9:864039. doi: 10.3389/fmolb.2022.864039
Received: 28 January 2022; Accepted: 14 March 2022;
Published: 26 April 2022.
Edited by:
Emil Alexov, Clemson University, United StatesReviewed by:
Kristina Brooke Martinez-Guryn, Midwestern University, United StatesRoberta Imperatore, University of Sannio, Italy
Copyright © 2022 Guo, Wang, Gao, Hua, Gao, He, Wang and Xu. This is an open-access article distributed under the terms of the Creative Commons Attribution License (CC BY). The use, distribution or reproduction in other forums is permitted, provided the original author(s) and the copyright owner(s) are credited and that the original publication in this journal is cited, in accordance with accepted academic practice. No use, distribution or reproduction is permitted which does not comply with these terms.
*Correspondence: Jing-Dong Xu, eHVqaW5nZG9uZ0AxNjMuY29t
†These authors have contributed equally to this work