- 1Department of Informatics, Systems and Communication, University of Milano-Bicocca, Milan, Italy
- 2Department of Environmental Sciences, Informatics and Statistics, Ca’ Foscari University of Venice, Venice, Italy
- 3Bicocca Bioinformatics, Biostatistics and Bioimaging Centre—B4, Milan, Italy
- 4SYSBIO/ISBE.IT Centre of Systems Biology, Milan, Italy
- 5Department of Human and Social Sciences, University of Bergamo, Bergamo, Italy
- 6Department of Biotechnology and Biosciences, University of Milano-Bicocca, Milan, Italy
Calcium homeostasis and signaling processes in Saccharomyces cerevisiae, as well as in any eukaryotic organism, depend on various transporters and channels located on both the plasma and intracellular membranes. The activity of these proteins is regulated by a number of feedback mechanisms that act through the calmodulin-calcineurin pathway. When exposed to hypotonic shock (HTS), yeast cells respond with an increased cytosolic calcium transient, which seems to be conditioned by the opening of stretch-activated channels. To better understand the role of each channel and transporter involved in the generation and recovery of the calcium transient—and of their feedback regulations—we defined and analyzed a mathematical model of the calcium signaling response to HTS in yeast cells. The model was validated by comparing the simulation outcomes with calcium concentration variations before and during the HTS response, which were observed experimentally in both wild-type and mutant strains. Our results show that calcium normally enters the cell through the High Affinity Calcium influx System and mechanosensitive channels. The increase of the plasma membrane tension, caused by HTS, boosts the opening probability of mechanosensitive channels. This event causes a sudden calcium pulse that is rapidly dissipated by the activity of the vacuolar transporter Pmc1. According to model simulations, the role of another vacuolar transporter, Vcx1, is instead marginal, unless calcineurin is inhibited or removed. Our results also suggest that the mechanosensitive channels are subject to a calcium-dependent feedback inhibition, possibly involving calmodulin. Noteworthy, the model predictions are in accordance with literature results concerning some aspects of calcium homeostasis and signaling that were not specifically addressed within the model itself, suggesting that it actually depicts all the main cellular components and interactions that constitute the HTS calcium pathway, and thus can correctly reproduce the shaping of the calcium signature by calmodulin- and calcineurin-dependent complex regulations. The model predictions also allowed to provide an interpretation of different regulatory schemes involved in calcium handling in both wild-type and mutants yeast strains. The model could be easily extended to represent different calcium signals in other eukaryotic cells.
1 Introduction
Calcium ions (Ca2+) have many physiological functions and are ubiquitously used by prokaryotic and eukaryotic unicellular organisms, as well as by multicellular eukaryotes (Plattner and Verkhratsky, 2015b; Plattner and Verkhratsky, 2015a; Giorgi et al., 2018; Bagur and Hajnóczky, 2017). Ca2+ represents a universal intracellular messenger that modulates a plethora of processes, such as the control of cell proliferation, programmed cell death, neurotransmission, secretion, vesicular transport, cytoskeleton rearrangement, and transcription (Berridge et al., 2000). Ca2+ sequestration in different cellular compartments is the key to maintaining appropriate concentration gradients, which are required to generate the temporal and spatial patterns exploited by cells to encode signals about their own status or the extracellular environment (Berridge et al., 2003; Dupont et al., 2007; Purvis and Lahav, 2013). Specific Ca2+ signals are triggered only upon an intra- or extra-cellular stimulus; in the absence of such events, the cell must maintain properly low and non-signaling Ca2+ concentrations, in a narrow range of 50–200 nM in eukaryotic cells. This feature is implemented by an extensive and well conserved cellular toolkit comprised of Ca2+-sensing proteins, buffers, channels, pumps, and exchangers (Plattner and Verkhratsky, 2015b). Although Ca2+ signals could possibly be generated in all cell compartments, they are mainly studied in the cytosol, and are the result of the release from intracellular stores or the influx from the extracellular environment, or both. The disruption of Ca2+ homeostasis system can potentially lead to unwanted (in)activation of signaling cascades, and in turn cause cell defects or even cell death (Carafoli, 2004; Sammels et al., 2010).
To help unraveling the role of channels and transporters in maintaining Ca2+ homeostasis, in this work we provide a mathematical model of the hypotonic shock (HTS) response in budding yeast cells. HTS consists in a sudden variation of the osmotic pressure, due to a consistent dilution of the solution concentration to which the cell is exposed. Following a HTS, water flows into the cell, causing an increase in cell volume and turgor pressure that might induce the cell burst. To avoid cytolysis, yeasts have evolved mechanisms to sense and respond to HTS, by rapidly triggering at least three different mechanisms: 1) the cytosolic Ca2+ concentration is transiently increased; 2) the osmolyte glycerol is released to the medium in order to relieve osmotic pressure; 3) phospholypase C hydrolizes PI(4,5)P2 generating the major second messengers, diacylglycerol (DAG) and inositol-(3,4,5)-tris-phosphate (IP3). In mammalian cells, DAG is well-known as a protein kinase C activator, while IP3 is involved in triggering the release of Ca2+ from intracellular compartments such as endoplasmic reticulum or Golgi (Pinton et al., 1998; Berridge et al., 2000); it is not clear if this applies to yeast as well, but a role for inositol phosphate in calcium release was previously reported (Belde et al., 1993; Tisi et al., 2002, 2004).
The yeast S. cerevisiae has evolved a cell wall that, having less elasticity than the plasma membrane, prevents the cell from excessive expansion (Aguilar-Uscanga and Francois, 2003; Alsteens et al., 2008; Orlean, 2012). The adaptation of the cell wall to the environmental challenges is controlled by the cell wall integrity (CWI) signaling pathway, which shows a complex interrelationship with Ca2+ signaling (Hohmann, 2002; Levin, 2011).
The mathematical model presented in this work was defined by integrating well-established experimental evidences with plausible hypotheses on the functioning of HTS response. The main components of the model comprise: 1) the Ca2+ membrane transporters, namely, the High Affinity Calcium influx System (HACS) and mechanosensitive channels (MS); 2) the vacuolar transporters Pmc1 and Vcx1; 3) the Ca2+-binding messenger protein calmodulin (CaM), and the Ca2+-CaM-dependant phosphatase calcineurin (CaN). The model is formalised as a system of coupled Ordinary Differential Equations (ODEs), and it can be conceptually divided in two modules: a biophysical module, describing the physical properties of yeast cells (e.g., volume, turgor pressure, and membrane tension), and a biochemical module, describing the changes in the concentration of protein and molecules involved in Ca2+ signaling upon HTS, and including the feedback regulation via the calmodulin-calcineurin pathway.
The model simulations are in accordance with published experimental results, suggesting that the biophysical and the biochemical modules are able to explain the role and interplay among the essential components involved in the HTS response. Our analysis shows that calcium enters the resting cell through the HACS and the MS channels. However, upon HTS, the increase of the plasma membrane tension amplifies the opening probability of MS channels, thus causing a sudden Ca2+ pulse. The rapid recovery of the basal Ca2+ levels in the cytosol primarily involves Pmc1, differently than in other signalling processes where massive amounts of Ca2+ enter the cytoplasm requiring Vcx1 to engage in the recovery (Miseta et al., 1999b). According to our simulations, the involvement of Vcx1 is actually marginal, unless Vcx1 inhibition by calcineurin is removed. Our results also suggest that the MS channels are subject to a calcium-dependent feedback inhibition, possibly involving calmodulin, since it is not relieved by calcineurin removal. This suggests that the very sharp signature of HTS-induced calcium peak would be obtained by the rapid closure of the MS channels triggered by this feedback loop.
The paper is organized as follows: Section 2 provides a detailed description of the cellular components and processes that were taken into account in the HTS response model; in Section 3 we explain how the two modules of the model were formalised and simulated; Section 4 provides a detailed description of the modules and their parameters; Section 5 shows the results we obtained by comparing experimental data with our model simulations; finally, we discuss these results in Section 6 and draw final conclusions in Section 7.
2 Biological Background
The main cellular components appearing in the mathematical model are represented in Figure 1. Their biological function and mutual regulation in controlling Ca2+ homeostasis and signaling, especially upon HTS, are described in the following sections.
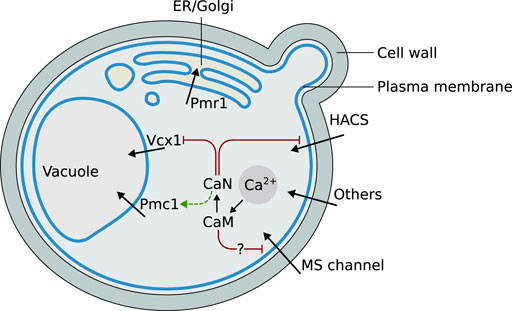
FIGURE 1. Graphical schematization of the role and localization of the Ca2+ signaling components included in the model, and their mutual regulations. Abbreviations: ER = endoplasmic reticulum, HACS = High Affinity Calcium influx System, CaN = calcineurin, CaM = calmodulin, MS = mechanosensitive.
2.1 Calcium Transport and Homeostasis
2.1.1 Membrane Transporters
The High Affinity Calcium influx System (HACS) is composed of Cch1 and Mid1. Cch1 is a homolog of the pore-forming α1 subunit of mammalian voltage-gated Ca2+ channels (Fischer et al., 1997), while Mid1 is a stretch-activated Ca2+-permeable nonselective cation channel whose secondary structure is similar to the non-pore-forming α2δ subunit that associates with the mammalian α1 (Iida et al., 1994). Cch1 and Mid1 cooperate in many yeast processes, such as mating pheromone-induced Ca2+ uptake (Paidhungat and Garrett, 1997), store-operated Ca2+ entry (Locke et al., 2000), ER stress-induced Ca2+ uptake (Bonilla et al., 2002), and hyperosmotic stress-induced increase of cytosolic Ca2+ (Matsumoto et al., 2002). Cch1 seems to respond to membrane depolarization, as the HACS is dependent on the presence of Kch1 and Kch2, two putative potassium transporters that mediate K+ influx and, likely, plasma membrane depolarization in pheromone-induced conditions (Stefan C. P. et al., 2013), as well as upon ER stress (Stefan and Cunningham, 2013) or glucose addition (Ma et al., 2021). Some lines of evidence suggest that Mid1 and Cch1 can also function independently from each other (Kanzaki et al., 1999), in different compartments (Yoshimura et al., 2004) or upon different stimuli (Courchesne and Ozturk, 2003).
Besides other yet poorly characterized Ca2+ influx systems in the plasma membrane (Eilam and Othman, 1990; Muller et al., 2001; Muller et al., 2003; Groppi et al., 2011), the presence of another plasma membrane Ca2+ influx system was implied based on the fact that the hypotonic stress-induced [Ca2+]cyt increase was not inhibited by removing all known transporters (Rigamonti et al., 2015). Its molecular identity is unknown but it is likely to include a Transient Receptor Potential (TRP)-like protein, Flc2, which provides the channel with a [Ca2+]ext-dependent inhibition. Flc2 is a member of the fungal spray family, which comprises TRP-like poly-cystic-kidney-disease (PKD)-related calcium channels (Tisi et al., 2016). TRP channels are well conserved and their regulation is polymodal. Almost all TRP channels appear to function as homo- or hetero-tetramers. TRP channels are regulated by a very large spectrum of chemical and physical stimuli such as phosphoinositides, Ca2+, cyclic nucleotides, temperature, voltage, osmotic stress, and membrane shearing. A single TRP channel can exhibit sensitivity to multiple types of stimuli and thus mediates integrated responses (Zheng, 2013).
Differently than in plant and animal cells, Ca2+ efflux proteins have not been detected on the plasma membrane of yeast cells. However, Ca2+ is presumably excluded from the cytoplasm by yet unknown Ca2+ transport mechanisms. Early experiments showed that the presence of potassium or sodium in the medium—and their consequent influx in the cells—induces efflux of Ca2+ (Eilam, 1982b). Analogous results were obtained with low external pH, suggesting the presence of a Ca2+/H+ antiport located on the plasma membrane (Eilam, 1982a; Hong et al., 2013).
2.1.2 Vacuolar Transporters.
Ca2+ is massively stored in the vacuole by two active transporters: Pmc1, a high affinity low capacity Ca2+-ATPase (Cunningham K. and Fink G., 1994), and Vcx1, a low affinity high capacity Ca2+/H+ exchanger. Vcx1 has a major role in shaping the calcium signal, since its high capacity can rapidly attenuate a large burst of cytosolic Ca2+ concentration (Miseta et al., 1999b). The free Ca2+ concentration in the budding yeast vacuole is estimated to be of about 30 μM, although larger amounts are stored as inorganic phosphates (Dunn et al., 1994). The vacuolar membrane of S. cerevisiae also contains Yvc1, a TRP-like calcium channel (Palmer et al., 2001; Denis and Cyert, 2002). Proper Ca2+ concentration in the endoplasmic reticulum (ER) organelle is critical to its functions; in S. cerevisiae it is maintained at 10 μM (Strayle et al., 1999), well below the concentration found in this compartment in higher eukaryotes, where it is the main internal storage for calcium ions (Stefan C. J. et al., 2013). Cls2/Csg2, an ER-localized protein, was originally proposed to play a role in Ca2+ efflux from the ER (Beeler et al., 1994; Tanida et al., 1996). However, Csg2 is likely implicated in the mannosylation of the inositol-phosphoceramide (IPC), corroborating the evidences about the sphingolipid roles in regulating ionic channels (Birchwood et al., 2001; Montefusco et al., 2014). Although initially underestimated, the central role of the Golgi apparatus in calcium homeostasis and signaling is now well appreciated in eukaryotic cells (Pizzo et al., 2011), also in yeast cells (Miseta et al., 1999a; Wuytack et al., 2003). Pmr1, the budding yeast Golgi P-type Ca2+/Mn2+-ATPase, was the first member of the secretory pathway Ca2+-ATPase (SPCA) subfamily identified (Rudolph et al., 1989; Antebi and Fink, 1992; Sorin et al., 1997); its role is pivotal for the maintenance of proper Ca2+ levels in both the Golgi apparatus (Halachmi and Eilam, 1996; Miseta et al., 1999a), and the ER (Durr et al., 1998; Strayle et al., 1999). Early reports suggested that yeast mitochondria have little, if any, role in accumulating Ca2+ (Carafoli et al., 1970; Balcavage et al., 1973), and their Ca2+ levels appear to be comparable to those of cytosol (Jung et al., 2004; Niedzwiecka et al., 2018).
2.1.3 The Calmodulin-Calcineurin Pathway
Calmodulin (CaM) is a highly conserved and ubiquitous Ca2+-binding protein that modulates the activity of many target enzymes, mainly in response to increasing intracellular Ca2+ concentrations (Cyert, 2001), which trigger distinct structural rearrangements, and modes of target activation (Nakashima et al., 2012; Ogura et al., 2012b; Ogura et al., 2012a; Ishida et al., 2002). The number of identified target proteins for mammalian CaM is, according to the Calmodulin Target Database, nearly 300 (Yap et al., 2000), whereas far fewer are known for the yeast CaM (Cyert, 2001). During stress responses, the yeast CaM functions primarily through the activation of a small fraction of its targets: the calmodulin-dependent protein kinases (encoded by CMK1, CMK2), and calcineurin. Calcineurin is a Ca2+/calmodulin-dependent serine/threonine-specific protein phosphatase, and represents the major Ca2+ signaling effector (Cyert and Thorner, 1992; Groppi et al., 2011; Li et al., 2011). Calcineurin regulates Ca2+ homeostasis and signaling both at the transcriptional level, for example by regulating the transcription of the Pmc1 encoding gene, and via the transcription factor Crz1 (Yoshimoto et al., 2002; Cyert, 2003), and at the post-translational level, for example by direct dephosphorylation of Vcx1. Some calcineurin targets involved in Ca2+ homeostasis are the HACS and the vacuolar Ca2+/H+ exchanger Vcx1 (Cunningham and Fink, 1996; Miseta et al., 1999b; Kingsbury and Cunningham, 2000).
2.2 Hypotonic Shock Response
Upon HTS, after the transient osmotic swelling, mammalian cells re-adjust their volume by a mechanism known as regulatory volume decrease (RVD) (Okada et al., 2001). In some cell types, RVD is accomplished by means of stretch-activated Ca2+ channels which mediate a rapid increase of the cytosolic Ca2+ concentration due to both Ca2+ influx and Ca2+ release from intracellular stores, which in turn is often the signal that triggers release of osmolytes, reducing osmotic gradients and helping volume regulation (Jakab et al., 2002). In addition, the HTS-induced activation of ion conducting pathways leads to profound changes in the plasma membrane potential, which determines the direction of the ion fluxes depending on the respective equilibrium potentials and the modulation of voltage-gated ionic channels.
Upon HTS, a large fraction of glycerol, an intracellular osmolyte, is released in yeast cells to the extracellular environment within 2–3 min through the activation of Fps1 channels (Tamas et al., 1999), which is inhibited neither by gadolinium, which instead completely blocks Ca2+ increase (Batiza et al., 1996), nor by membrane potential alterations (Kayingo et al., 2001). Fps1 was proposed as a mechanosensitive channel directly activated by the induced membrane stretch, whereas the known post-translational modifications probably fine-tune its activity under basal conditions (Ahmadpour et al., 2014).
Phosphoinositides are negatively charged membrane lipids that serve as versatile molecules involved in protein regulation, assembly of actin cytoskeleton, vesicle trafficking and Ca2+ signaling. Several phosphoinositides are substrates for phospholipases, thereby generating a number of products that serve as second messenger with biological functions on their own (Strahl and Thorner, 2007). For example, the plasma membrane lipid phosphatidylinositol 4,5-bisphosphate (PtdIns (4,5) P2) can be depleted by activation of phospholipase C (PLC), producing diacylglycerol (DAG) and the diffusible molecule Ins (1,4,5) P3 (IP3), which in mammals is a fundamental signaling molecule. In budding yeast, as in all eukaryotic cells, different phosphoinositide species are generated in a compartment-specific manner and, hence, can be regarded as distinct markers for each organelle (Odorizzi et al., 2000; Strahl and Thorner, 2007; Balla, 2013) and contribute to specific regulation of protein activity, such as ion channels (Hille et al., 2015). Upon HTS, S. cerevisiae cells hydrolyze plasma membrane PtdIns (4,5) P2 with a mechanism dependent on Plc1 but independent on the extracellular Ca2+ concentration. This process liberates IP3, which is then rapidly phosphorylated in IP6. In addition, another phosphoinositide, PtdIns4P, and is rapidly synthesized and then progressively consumed in the next minutes (Perera et al., 2004). Although a link between these dynamics and Ca2+ signaling has not been explored yet, it is tempting to suggest one. In fact, the rapid depletion of the plasma membrane signature lipid PtdIns-(4,5)-P2 could influence the activity of some channels. Flc2 is the best candidate, since it resides on plasma membrane and contains a putative lipid-binding domain. Channels localized on Golgi or ER could also be influenced by changes in phosphoinositides abundances: PtdIns4P transient increase could regulate Golgi channels, since PtdIns4P is the signature lipid of this organelle. Some Ca2+ regulation seems to be at stake in HTS because PLC1 gene deletion, which abolishes PtdIns-(4,5)-P2 depletion and IP6 production, causes a greater increase in calcium influx after HTS compared to a wild type strain (Tisi et al., 2002). It is worth noting, however, that PLC1 deletion does not affect PtdIns4P dynamics upon HTS (Perera et al., 2004).
The immediate and transient (∼2 min) cytosolic Ca2+ pulse (Batiza et al., 1996; Rigamonti et al., 2015) triggered by HTS in S. cerevisiae cells is generated both by influx from the extracellular medium and efflux from intracellular stores. An early study on yeast cells grown in synthetic medium reported that this increase was mediated by an instantaneous release of Ca2+ from intracellular stores and then sustained by influx of extracellular calcium. In fact, addition of an extracellular Ca2+ chelator, BAPTA, affected later stages of the response without affecting the initial, and rapid cytosolic Ca2+ rise (Batiza et al., 1996). Moreover, the same study showed that the HTS-induced Ca2+ response was dependent on both intensity of the shock and type of growth medium, the latter affecting also the pre-stimulus baseline [Ca2+]cyt. The increase of Ca2+ was inhibited in a dose-dependent manner by pre-treatment with gadolinium, a blocker of stretch-activated channels, and suggesting that the membrane stretching that occurs following HTS-induced cell swelling is directly sensed by Ca2+ channels (Batiza et al., 1996). In yeast cells grown in YPD—a complex, nutrient-rich medium—and challenged with HTS by diluting the medium with distilled water, an estimate of the initial rate of calcium increase at micromolar [Ca2+]ext could be fitted by a Hill function, suggesting that the calcium increase in response to HTS was caused by the activation of a single channel or transporter located on the plasma membrane (Rigamonti et al., 2015).
The HTS response was also measured for mutants lacking proteins known to be involved in calcium signaling and homeostasis. cch1Δ mutants, lacking a functional HACS channel on the plasma membrane, responded to HTS with a higher calcium peak at all [Ca2+]ext considered, suggesting a negative regulatory role for this protein during HTS. Mutants in other known influx pathways, on the other hand, and had a response similar to the wild-type. Calcium levels are affected in calcineurin mutants, suggesting that this Ca2+-dependent effector shapes the calcium signal during HTS through dephosphorylation of target transporters and/or by modulating their long-term expression. In addition to display altered resting calcium levels, mutants lacking calcineurin respond to HTS with a dramatically reduced peak. Flc2 was found to be involved in the HTS-induced calcium response, since deletion of FLC2 increases the initial rate of the Ca2+ increase compared with wild-type, suggesting an inhibitory role of this protein on the channel that is activated by the HTS. Based on the experimental evidences described above, a model is proposed that includes only the essential players in the HTS-induced calcium response. In non signaling conditions, Ca2+ enters the cell through HACS channel and other unidentified influx pathways. A still unidentified mechanosensitive calcium channel is located on the plasma membrane and activated by the increased membrane tension caused by HTS. In addition, this channel appears to be negatively regulated by Flc2. Since the elevation of [Ca2+]cyt is transient, some intracellular transporters must restore the steady-state levels of cytosolic Ca2+. This signal attenuation is probably performed by the Golgi-localized Pmr1, together with vacuolar-localized Pmc1 and Vcx1.
3 Methods
3.1 Model Definition and Simulation
The mathematical model of HTS response in S. cerevisiae was defined on the basis of available experimental evidences (Rigamonti et al., 2015), and can be conceptually divided in two modules:
1 the biophysical module describes the changes in volume and other cell parameters, such as turgor pressure and membrane tension. This module allows for quantitatively following all changes in the physical state of the cell depending on cytosolic and extracellular osmolarities. Such parameters, in turn, regulate the activity of some components of the biochemical module. In particular, stretch-activated channels open following a sudden increase of membrane tension, promoting Ca2+ diffusion through them;
2 the biochemical module describes all the relevant reactions that take place in the cell—or between the cell and the extracellular environment—during the HTS response. This module comprises two compartments: the cytosol and the extracellular environment.
The model was formalized as a system of coupled ODEs. Mass-action kinetics was used to model the physical interactions between Ca2+, calmodulin, and calcineurin. Most transport reactions were modeled by means of the Michaelis-Menten kinetics, as substantiated by previous studies (Ohsumi and Anraku, 1983; Wei et al., 1999; Takita et al., 2001; Teng et al., 2008).
Stretch-activated channels were modeled differently: they can be viewed as pores, whose opening probability depends on membrane tension. In particular, the opening probability of mechanosensitive channels was shown to follow a Boltzmann distribution (Gustin et al., 1988; Sukharev et al., 1999; Jiang and Sun, 2013). For the sake of simplicity, in this work the Boltzmann equation employs turgor pressure (note that membrane tensions can be calculated from turgor pressure using Laplace’s law for a thin-walled sphere (Gustin et al., 1988; Sackin, 1995)). The opening probability (Popen) of mechanosensitive channels was thus formally defined as:
where P is turgor pressure, PMS is the value of turgor pressure at which Popen is equal to 0.5, and gMS is a slope parameter.
Since ions pass through the channel pore down their electrochemical gradient, we assume that calcium ions flow according to their concentration gradient. The calcium flux j is then given by:
where k is a rate parameter and Δc is the Ca2+ concentration gradient across the membrane.
The model was simulated with COPASI (version: 4.19) (Hoops et al., 2006), using the LSODA algorithm (Petzold, 1983) with default settings. Simulation outputs consist in time traces of species concentrations over a period of 160 s, in line with the stress response duration of yeast cells. The model is available as an SBML Level 2 Version 5 file (ID MODEL2112030001) in the BioModels repository (Malik-Sheriff et al., 2020): https://www.ebi.ac.uk/biomodels/MODEL2112030001.
3.2 Parameter Estimation
Unknown parameters were estimated with COPASI (Hoops et al., 2006), using the available implementation of the Particle Swarm Optimization (PSO) algorithm (Kennedy and Eberhart, 1995). PSO is a global optimization algorithm based on the concept of “swarm intelligence”, which was shown to be effective in solving optimization problems characterized by multi-modal and noisy fitness landscapes, such as the ones related to the parameter estimation problem of biochemical systems (Nobile et al., 2018; Tangherloni et al., 2019; Besozzi et al., 2020).
To estimate the unknown parameters, simulations were fitted against available experimental time traces of [Cacyt], measured in different experimental conditions in Rigamonti et al. (2015), including deletion mutants in key proteins of the HTS response and a wide range of extracellular Ca2+ concentrations. It is worth mentioning that, in the parameter estimation process, we took into account the well known fact that the intracellular calcium levels are kept in a narrow range despite wide variations in external conditions, thanks to calcium buffering and sensing and feedback mechanisms (Cunningham K. W. and Fink G. R., 1994). Thus, while reaction constants must be the same across different experiments, the concentrations of proteins bound to Ca2+ can vary. According to this line of reasoning, these parameters were not forced to be the same for all experiments. Since the model describes a stimulus response, the steady-state pre-stress conditions were also included in the parameter estimation process. Search ranges for all unknown parameters were set to be within biologically plausible numeric intervals.
4 Model Definition
4.1 Definition of the Biophysical Module
The biophysical module describes the variation of the physical parameters of the cell, such as the cell volume and turgor pressure. The following mathematical description of volume regulation under osmotic stress is a simplified version of a previously published model, which was carefully parameterized using hyperosmotic shock data (Schaber and Klipp, 2008).
4.1.1 Volume
Assuming that volume changes are only due to water flow and not to solute flow, the total cell volume V (in L) can be defined as the sum of an osmotically active volume (water volume) Vos and an osmotically inactive volume (solid volume) Vb:
with Vb assumed to be constant. The extracellular volume, Vex, is much greater than the volume occupied by the cell. Therefore, for the sake of simplicity, we assume that Vex = V (0) ⋅ 1,000, where V (0) is the initial cell volume.
The water flow is driven by gradients of water potential and hydrodynamic potential (Griffin, 1981; Kleinhans, 1998), which can be formalized as:
where Lp is the hydraulic conductivity (in dm⋅MPa−1⋅s−1), A is the cell surface area (in dm2) and P is the intracellular hydrostatic pressure exerted on the cell wall—i.e., the turgor (in MPa)—which equilibrates ΔΠ under steady-state conditions
where
with
The van’t Hoff law can be used to express the osmotic pressure in terms of concentration of osmotically active molecules: ΔΠ = cPCRTΔc, where Δc is the concentration and cPC is a conversion factor relating concentrations in M to pressures in MPa. Thus, Eq. 6 can be written as:
where Δc is substituted with [Osme] − [Osmi], where [Osme] and [Osmi] are extracellular and intracellular concentration (in μmol⋅L−1) of osmotically active molecules, respectively. If Eq. 7 is initially at steady-state
Both intracellular and extracellular osmolarities are the sum of concentrations of permeable solutes (glycerol) and non-permeable solutes. The extracellular osmolarity is thus:
where
The intracellular osmolarity, accounting for cell volume variation, is defined as:
where [Glyi] is the intracellular glycerol concentration,
4.1.2 Turgor Pressure
The water potential gradient maintained by all cells across their membrane is balanced by a hydrostatic pressure called turgor. In walled cells, turgor pressure causes the cell membrane to exert a force on the cell wall, which expands due to its elasticity. The elastic-theory of turgor pressure states that the change in turgor pressure P is proportional to a relative change in cell volume:
where the proportionality factor ϵ is called volumetric elastic modulus, or Young’s modulus (in MPa).
The dependence of turgor pressure on volume can be deduced by integration:
By defining V0 as the volume when turgor becomes zero, the turgor pressure can be expressed as a function of volume:
It is known that glycerol efflux and synthesis is tightly regulated according to environmental conditions (Talemi et al., 2016). However, given the small time-scales considered here, internal glycerol concentration is assumed to be constant.
4.1.3 Hypotonic Shock
Hypotonic shock is applied to the cell by diluting the medium with distilled water, thus decreasing its osmolarity. This dilution is modeled as follows:
where
4.2 Definition of the Biochemical Module
The concentration of calcium ions in the cytosol changes due to fluxes across different channels and transporters. The cytosol is also provided with calmodulin—a protein involved in the binding and sensing of calcium ions (Cyert, 2001)—that can activate calcineurin, the main calmodulin effector. HTS is applied by diluting the medium in which cells grow with distilled water, which has also the effect of reducing the availability of calcium ions. Since the extracellular volume is way larger than the volume occupied by all cells, the reduction of extracellular calcium ions caused by the cell uptake can be neglected. Therefore, the extracellular Ca2+ concentration depends only on dilution factor and mixing time (see Eq. 14):
with all concentrations expressed in nM, and t, toff and tm expressed in seconds.
The rate of change of the Ca2+ concentration in the cytosol can be written as the sum of fluxes of the relevant channels and transporters (described below):
where the js are Ca2+ fluxes (in nM⋅s−1) across calcium channels and transporters.
Very often, channels and transporters show kinetics that can be described with the Michaelis-Menten equation (i.e. the transporter saturates at high substrate concentrations) (Christopher, 2002; Weijiu, 2012; Fridlyand et al., 2003). Indeed, this is the case for the intracellular transporters Pmr1, Vcx1, Pmc1 (see references in Table 2). Pmr1 indirectly replenishes the endoplasmic reticulum with Ca2+, while Vcx1 and Pmc1 are responsible for its sequestration into the vacuole. Ca2+ enters the cell through the plasma membrane-located HACS channel and other transporters whose molecular identities are yet unknown (Batiza et al., 1996; Locke et al., 2000; Tisi et al., 2002; Cui et al., 2009a). Here, the influx associated with this unknown transport is simply called jIN—to recall its function—and is assumed to have a Michaelis-Menten kinetics.
Experimental evidences strongly suggest that the increase of cytosolic Ca2+ in cells challenged with HTS is caused by the opening of a MS channel on the plasma membrane (Batiza et al., 1996; Rigamonti et al., 2015). This calcium influx pathway has not been molecularly identified yet, and it is here denoted by jMS. The equations describing the fluxes are:
where Popen is the opening probability of the MS channel (see below), kCch1 and kMS are rate parameters (in s−1), vs. are rate constants (in nM⋅s−1), and all other ks are Michaelis constants (in nM).
The opening probability of MS channels follows a Boltzmann distribution (Gustin et al., 1988; Sukharev et al., 1999; Jiang and Sun, 2013). MS channels are gated by membrane tension (Gustin et al., 1988; Sackin, 1995) but here, for the sake of simplicity, turgor pressure is used instead (see Section 3.1 and Eq. 1 for a justification). The opening probability of the MS channel is then:
where P is the cell turgor pressure in MPa (see Eq. 13), PMS is the turgor pressure (in MPa) at which Popen is equal to 0.5, and gMS is a slope parameter (in MPa).
4.2.1 Feedback Regulation
Inside the cytosol, yeast calmodulin binds three Ca2+ ions with high cooperativity (Davis et al., 1986; Nakashima et al., 1999):
By using the law of mass action, the rate equation for Ca2+-bound calmodulin can be formalized as:
where [CaMb] is the concentration of Ca2+-bound calmodulin (in nM), CaMt is the total calmodulin concentration (in nM),
Calcineurin, a protein phosphatase, is activated upon binding with the Ca2+-bound calmodulin. By the law of mass action we can state that:
where [CaNb] is the concentration of calmodulin-bound calcineurin (in nM), CaNt is the total calcineurin concentration (in nM),
Following HTS, calcium ions enter the yeast cell through MS channels located on the plasma membrane. After the initial rise in Ca2+, the signal dissipation observed in the successive seconds must be the result of either an increased activity of one of the intracellular transporters or a feedback inhibition on the MS channels. Since no positive regulation is known for any of the relevant transporters, in the present model the latter mechanism is assumed. This assumption is supported by circumstantial evidence suggesting that the yeast MS channel interacts with a homologous of TRP proteins (Rigamonti et al., 2015). TRP proteins form tetrameric ion channels which frequently interact with—and are inhibited by—calmodulin (Rhoads and Friedberg, 1997; Zhu, 2005). It is also known that activated calcineurin post-transcriptionally inhibits Vcx1 and HACS activity (Cunningham and Fink, 1996; Miseta et al., 1999b; Locke et al., 2000). The following equations are thus used to model the feedback inhibition:
where kICch1, kIMS and kIVcx1 are inhibition constants (in nM−1).
4.3 Estimation of the Unknown Parameters
Unknown parameters of the model were fitted against time traces of cytosolic Ca2+ measurements from HTS experiments conducted by Rigamonti et al. (2015) and by R. Tisi, unpublished results (Figure 2), as described in Materials and Methods. In these experiments, wild-type S. cerevisiae cells were grown in YPD medium, which has an estimated osmolarity of 0.26 Osm/L (Schaber et al., 2010). Specifically, the parameters of the biophysical module (Table 1) were set to reflect those experimental conditions.
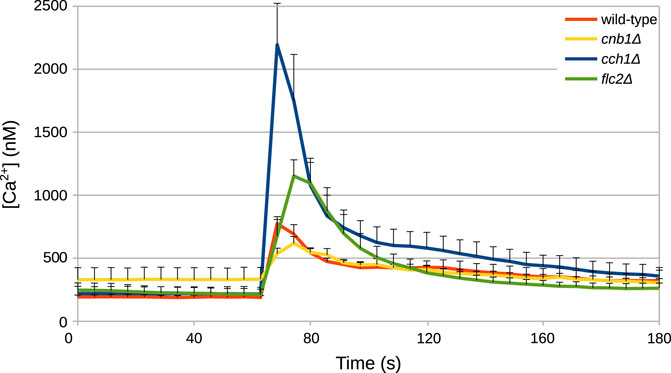
FIGURE 2. Example of time traces of cytosolic Ca2+ concentration in S. cerevisiae cells challenged with HTS. HTS was applied by diluting the growth medium with four volumes of distilled water at t =60. The final concentration of Ca2+ in the medium was 5.9 μM. Time traces were taken from Rigamonti et al. (2015) and from Tisi R., unpublished results.
In addition to the wild-type strain, we simulated three mutant strains: cnb1Δ, lacking functional calcineurin; flc2Δ, lacking a putative TRP-like channel subunit; and cch1Δ, lacking a functional HACS channel. Within the model, a mutant can be simulated by setting to zero the parameter associated with the function carried out by the removed gene. Hence, the cnb1Δ strain was obtained by setting to zero the total amount of calcineurin in the cell (CaNt), and the cch1Δ by setting to zero the rate parameter kCch1. However, these parameter settings were not sufficient to reproduce the behavior displayed by the three mutants. Thus, we performed additional parameter estimations exploiting available experimental time traces of the mutant strains (from Rigamonti et al. (2015) and R. Tisi, unpublished results, Figure 2).
In particular, PMC1 expression strongly depends on calcineurin (Cunningham and Fink, 1996), but the model can not automatically adjust PMC1 expression in the cnb1Δ mutant, since it lacks any description of transcriptional processes. To take into account the reduction of PMC1 expression in this mutant, we performed a separate parameter estimation to infer the value of the vPmc1 parameter (i.e., the Vmax parameter of a Michaelis-Menten equation (Eq. 20), which is proportional to the enzyme abundance) in the cnb1Δ model. By doing so, it was possible to assess the reduction of Pmc1 transporters in this mutant with respect to the wild-type. We argue that the estimated value of vPmc1 for the cnb1Δ mutant could be regarded as an estimate of the basal expression level of the Pmc1 protein, when Cnb1 is not stimulating the PMC1 gene transcription. Thus, we used the same value of vPmc1 to simulate the cch1Δ model. In fact, in order to maintain a physiological Ca2+ level when the Ca2+ income is lower, a novel steady state balance has to be achieved, where Pmc1 abundance has to be adjusted so that Ca2+ remains available to be provided to the secretory pathway as well. This is achieved by finely tuning the homeostasis regulatory circuit, through calcineurin transcriptional control on PMC1 gene, leading to a Pmc1 activity nearby the basal level estimated for the cnb1Δ mutant.
Adopting a similar approach to the one described above, we estimated the value of kMS for the flc2Δ strain, in order to account for the observed increased activity of the MS channel in this strain (Rigamonti et al., 2015). It is not known whether the increased activity of this channel is due to an increased channel abundance or simply to an increased channel activity. In any case, the rate parameter kMS is—like the Vmax of a Michaelis-Menten equation—proportional to the number of channels.
Analysis of the parameter estimation results led to the conclusion that the model could be simplified without affecting the simulations. In particular, removal of (a) the feedback inhibition on the HACS channel, mediated by calcineurin (Eq. 27), and of (b) the influx pathway that was called “IN” (Eq. 17), did not cause any significant difference on the simulation outcomes. Therefore, the feedback on HACS and the jIN influx were removed and all simulations and parameter values reported here are related to this simplified model. Table 2 lists all the parameters used for the wild-type model, while Table 3 contains only the values that changed depending on the strain.
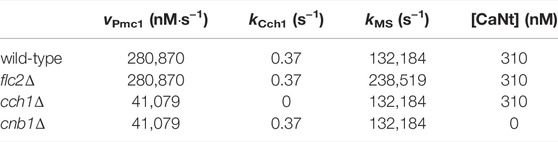
TABLE 3. List of parameters that change depending on the modeled mutant strain. All other parameters were kept as in the wild-type model (Table 2).
5 Results
5.1 Model Simulations
The simulations of the full model of HTS response correctly reproduce the dynamics of the Ca2+ transients (Figures 3A–D), as well as the steady-state levels and peak values of cytosolic Ca2+ (Figures 3E,F) of both wild-type and all mutant strains.
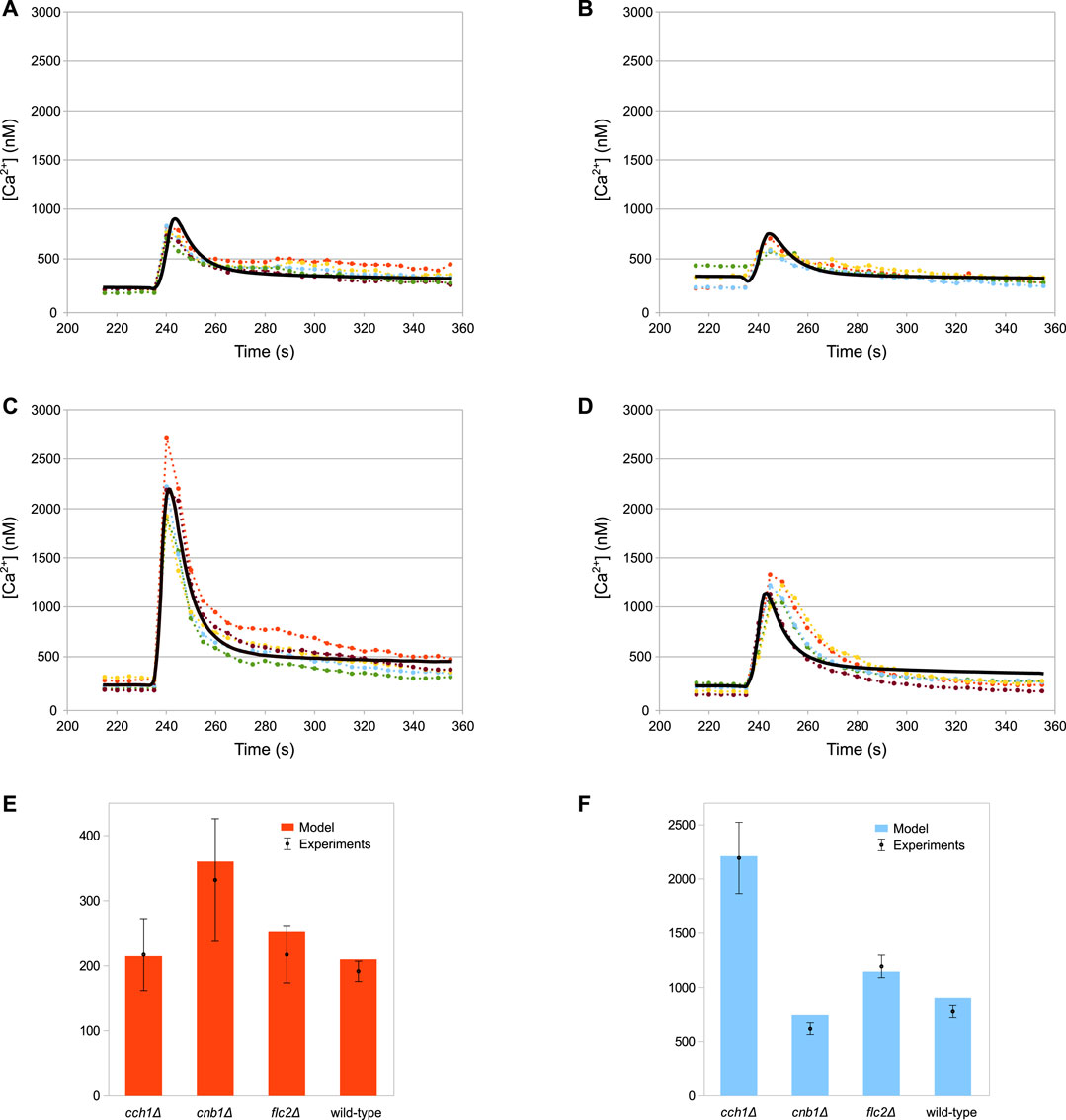
FIGURE 3. Comparison of simulation outcomes and experimental measurements. In Figures (A–D) the dotted lines represent experimental measurements and different colours indicate replicates, while the black lines represent model simulations. (A) Wild-type. (B) cnb1Δ. (C) cch1Δ. (D) flc2Δ. (E) Baseline cytosolic Ca2+ concentration (nM). (F) Max peak cytosolic Ca2+ concentration (nM). HTS was applied to the cells at 235 s by adding four volumes of distilled water to the medium (E,F) Plots constructed using the same data shown in (A–D); bars represent standard deviations.
The results of the simulations for the wild-type strain are shown in Figure 4. According to the model, after the HTS the HACS activity decreases due to the sudden shortage of the extracellular Ca2+ (Figure 4A), while the MS channels on the plasma membrane open rapidly (Figure 4B, inset). The cytosolic Ca2+-dependent inhibition on the MS channels provides a way to decrease the activity of this channel, thus helping to restore low intracellular calcium levels after the stimulus. In fact, the dynamics reported in Figure 4B shows that only in the presence of feedback inhibition the flux through this channel is still decreasing at time t > 280s. These outcomes also suggest that the main pump responsible for signal attenuation is Pmc1, the Ca2+-ATPase located on the vacuole, while Pmr1 and Vcx1 have a negligible role in this respect (Figures 4C,D). In particular, while the predicted rate of Ca2+ sequestration by Pmr1 is predicted to be consistently slow, the Vcx1 activity is kept down by the feedback inhibition mediated by calcineurin (Figure 4D).
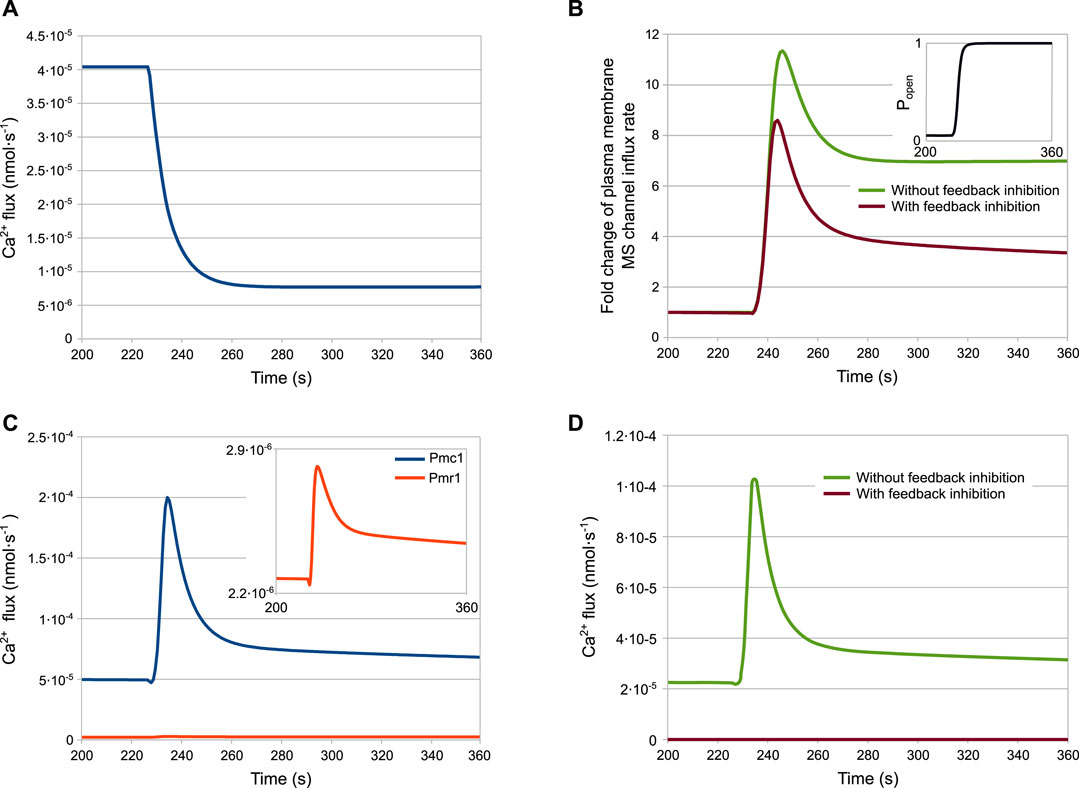
FIGURE 4. Fluxes involved in the HTS response of the wild-type strain. (A) HACS flux. (B) Effect of calmodulin inhibition on MS channels. (C) Pmc1 and Pmr1 fluxes. (D) Effect of calcineurin inhibition on Vcx1 (A–D) Ca2+ fluxes through channels and transporters as predicted by the model (parameters are given in Table 2) (B,D) Fluxes with or without inhibition are depicted by plotting jMS and
As described above, the peak response of cch1Δ mutants—lacking functional HACS—is considerably higher than in the wild-type strain (Figure 3F). According to the model, the peak difference is not ascribable to an increased activity of the MS channels in this strain with respect to the wild-type. In fact, in this mutant, Ca2+ fluxes through both MS channels and Pmc1 are considerably lower than both wild-type and flc2Δ strains (Figures 5A,C). This behaviour is the result of the reduced PMC1 expression (Table 3):
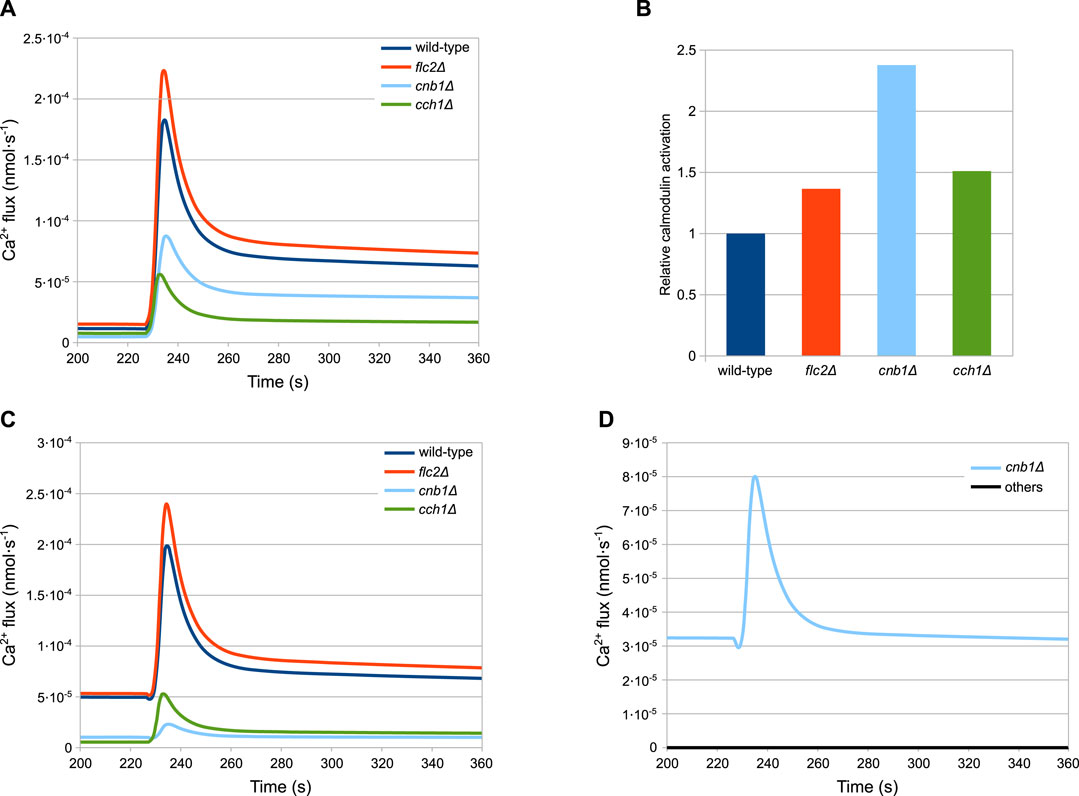
FIGURE 5. Simulations showing the main differences among the considered strains. (A) MS influx. (B) Calmodulin activation. (C) Pmc1 flux. (D) Vcx1 flux (A,C,D) Fluxes through the most relevant channels. (B) Calmodulin activation, shown relative to the wild-type level, before HTS is applied. Time traces of calcineurin are similar and are not shown for the sake of figure readability. (D) Vcx1 fluxes of all mutants but cnb1Δ are overlapping and therefore are coloured black.
Since Pmc1 is the main transporter responsible for Ca2+ sequestration from the cytosol, reducing its activity increases the level of Ca2+, which in turn inhibits the MS channels through the activated calmodulin (Figure 5B). This feedback process ensures that the steady-state calcium levels are comparable to those of the wild-type. However, the reduced activity of Pmc1 causes a higher peak when the MS channels open following HTS. Rigamonti et al. suggested that the increased Ca2+ peak in flc2Δ is caused by the removal of an inhibitory effect on the MS channels (Rigamonti et al., 2015). To test this hypothesis, during the parameter estimation process we let the kMS parameter of flc2Δ mutant assume different values compared to other strains (Table 3). The simulations show that, in line with this, a difference in the MS channels rate compared to the wild-type is sufficient to explain the increased peak observed during HTS (Figure 5A).
The cnb1Δ mutants show an increased steady-state level of cytosolic Ca2+ and a lower peak compared to all other strains (Figure 3). As described in Section 4.3, for this mutant cells we estimated the reduction of PMC1 expression. Our model predicts that Pmc1 abundance is about 16 times lower compared to the wild-type, which is counterbalanced by the increased activity of Vcx1 that is no longer inhibited by calcineurin. This compensation mechanism, however, fails to fully replace the decreased activity of Pmc1, and thus the mutant displays higher steady-state calcium levels. The higher calcium levels result in more activated calmodulin (Figure 5B), which in turn inhibits the MS channels leading to a lower peak (Figure 5A).
Figure 6 shows a prediction of HTS response in not yet tested experimental conditions, since the technical difficulty in achieving a tight control of the speed of dilution could not be overcome. Variability in the speed and shape of the signal was observed in different experiments, particularly when an automatic injector was not applied, thus we decided to investigate the effect of this parameter variation with in silico experiments. The tm parameter of the model defines the mixing time, i.e. the speed of dilution. The simulations of the wild-type strain with different mixing times produces an unexpected pattern (Figure 6A). In particular, while the speed of the response turns out to be linearly dependent on the mixing time (Figure 6B, red curve), the peak Ca2+ value is not. The peak values grow with the mixing time until a maximum at tm = 15s, before decreasing again (Figure 6B, blue curve).
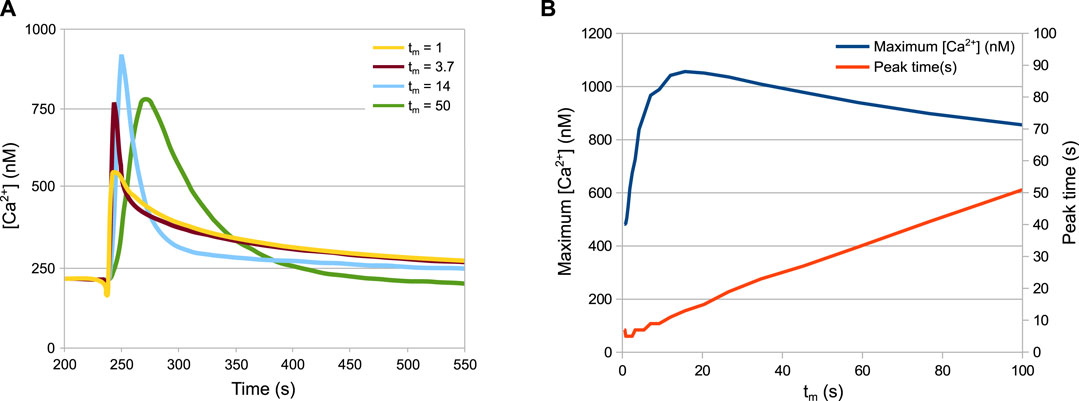
FIGURE 6. Behaviour of the system depending on the speed of the HTS. (A) Ca2+ peaks in response to different dilution speeds. (B) Maximum Ca2+ values and time of maximum Ca2+ values depending on the dilution speed. The tm parameter is inversely proportional to the speed of dilution. Increasing the speed of dilution increases the speed of response but not necessarily the peak height.
The model also predicts that the inactivation of the MS channels—simulated by setting to zero the parameter kMS—would reduce the steady-state cytosolic Ca2+ concentration from 215 to 160 nM.
6 Discussion
S. cerevisiae cells have to adapt to changes in growth conditions that arise both naturally, in the environment where they live, and artificially, during human exploitation. All cells sense extracellular osmolarity and fine-tune their biophysical parameters to ensure survival (Pedersen et al., 2011). Generation of a Ca2+ transient in response to HTS seems to be an ubiquitous phenomenon shared by yeast, plant and mammalian cells, and it is caused by the opening of MS channels (Sachs and Morris, 1998; Cox et al., 2013). In the yeast S. cerevisiae, many proteins involved in Ca2+ handling have been identified (Cui et al., 2009b; Tisi et al., 2016). However, only recently the specific roles of each channel or transporter in building, attenuating and shaping the observed Ca2+ dynamics are being investigated. The model presented here can help in bridging the gap between genetic analyses and phenotypic observations regarding Ca2+ signaling in yeast cells.
Simulations of the biophysical module alone showed that the volume of yeast cells increases only by a few percent relative to the pre-stress volume. This is in contrast to non-walled cells, whose volume can increase up to 50% (Weskamp et al., 2000). This difference is not surprising, as yeast cells must face harsher and unpredictable osmotic conditions than single cells inside a multicellular organism, and have therefore evolved a rigid cell wall.
In S. cerevisiae cells the vacuole is the main organelle for Ca2+ storage (Dunn et al., 1994), while in mammalian cells this role is played by the ER (Montero et al., 1995; Strayle et al., 1999). In fact, our simulations shows that most of the Ca2+ is transported into the vacuole. In all modeled strains, with the exception of cnb1Δ, Pmc1 appears to be the main vacuolar transporter. As expected, removal of calcineurin have opposite effects on vacuolar transporters: it reduces the expression of PMC1, but it increases the activity of Vcx1. However, our simulations suggest that the loss of Pmc1 activity is not entirely counterbalanced by Vcx1, and thus the cytosolic calcium level is higher than in the wild-type. These results are in accordance with calcium accumulation measurements showing that pmc1Δ mutants accumulate only 20% calcium compared to wild-type (Cunningham and Fink, 1996). In addition, the predictions made by our model—namely, that Vcx1 has a small role in calcium accumulation unless calcineurin is removed—are supported by the fact that vcx1Δ mutants accumulate the same amount of calcium as the wild-type, and that Vcx1 has a larger role in Ca2+ sequestration in cnb1Δ mutants (Cunningham and Fink, 1996). Pmr1 is important for the maintenance of proper calcium levels in the ER, where the steady-state free concentration is only 10 μM (Strayle et al., 1999). Our simulations suggest also that Pmr1 has a marginal role during the HTS response, as well as in maintaining low steady-state calcium level into the cytosol. However, no definitive comparison can be drawn between model predictions and experimental results because pmr1Δ mutants display pleiotropic defects that are probably direct consequences of ER calcium depletion (Rudolph et al., 1989; Antebi and Fink, 1992).
Yeast cells are equipped with a variety of calcium influx pathways, each with seemingly different functions (Eilam and Othman, 1990; Iida et al., 1994; Fischer et al., 1997; Muller et al., 2003). Our model initially included three calcium entry pathways: HACS (the high affinity calcium system composed of at least Cch1 and Mid1), MS channels, and another calcium influx of unknown molecular identity. The presence of MS channels on the yeast plasma membrane was demonstrated by patch-clamp experiments (Gustin et al., 1988), but their molecular identities are still unknown. Parameter estimation revealed that the additional influx (here called “IN”) was unnecessary in this model, and that HACS, and the MS channels alone are sufficient to explain the observed data. The removal of “IN”, which was the only active transporter on the plasma membrane introduced in the model, implies that in the simulations Ca2+ enters the cell only by passive transport, driven by the gradient in concentration and by the membrane potential. In fact, experiments demonstrated that in energy-depleted cells, where maintenance of membrane potential is defective, there was no Ca2+ influx (Eilam and Chernichovsky, 1987).
According to our simulations, even during steady-state conditions, and Ca2+ enters the cell through unstimulated MS channels. Indeed, most channels are known to be “leaky”, i.e., they stochastically open even in non signaling conditions. Since the flux through a single channel can be several orders of magnitude higher than that of a single transporter (Milo et al., 2010), even brief channel openings can theoretically supplement the cytosol with a significant amount of Ca2+. It has been shown that treatment with gadolinium, a blocker of stretch-activated channels, eliminates the Ca2+ rise in response to HTS without significantly affecting the steady-state Ca2+ concentration (Batiza et al., 1996). Accordingly, removing MS channels from our model decreases steady-state calcium levels from 215 to 160 nM, a value which is still well within the physiological range (Cunningham K. W. and Fink G. R., 1994). During model definition, a feedback inhibition was introduced on MS channels, mediated by calmodulin. This is the only assumption made in the model that is not yet supported by strong experimental evidence. We suggest that some kind of calcium-dependent feedback must exist, which either increases the activity of intracellular transporters or decreases the activity of MS channels. Many ion channels are inhibited by calmodulin (Saimi and Kung, 2002) and performing a parameter estimation with the model lacking this feedback inhibition produced poor results, in particular for the cnb1Δ mutant.
HACS channel has been shown to be activated following a number of external stimuli, such as alkaline stress and mating pheromone (Iida et al., 1994; Viladevall et al., 2004), but it is also involved in calcium uptake during normal growth, as evidenced by long-term calcium accumulation studies (Muller et al., 2001). In addition, HACS seems to physically interact with, and be inhibited by, calcineurin (Muller et al., 2001; Bonilla and Cunningham, 2003). After the parameter estimation process, it emerged that in our model the equation describing this negative regulation was unnecessary to reproduce the experimental data. This result suggests that, in the growth conditions considered here, feedback inhibition by calcineurin does not change significantly, since calcineurin activity is low in the chosen cultural conditions (Groppi et al., 2011).
Flc2 belongs to a TRP-like fungal family of putative yeast calcium transporters, together with Yor365c, Flc1, and Flc3 (Tisi et al., 2016). This raises the possibility that also the MS channels, with which Flc2 seems to interact, might belong to the aforementioned family. Our simulations support the hypothesis that Flc2 exerts an inhibitory role on the MS channels located on the plasma membrane (Rigamonti et al., 2015). In fact, the observed increase of calcium response and calcineurin hyperactivation in the flc2Δ mutant (Rigamonti et al., 2015) could be reproduced with the model just by increasing the activity of the MS channels. Flc2 may directly or indirectly influence the MS channel by affecting its stability, function or localization.
Most of the model predictions are in accordance with experimental results that were not used for the parameter estimation process, suggesting that the model provides an accurate description of the HTS response in yeast cells as well as the most relevant transcriptional and post-transcriptional regulations involved in calcium handling. In particular, the model suggests the following regulatory scheme (Figure 7). Normally, Ca2+ enters the cell via HACS and MS channel leak. Pmc1 is the main intracellular transporter that keeps Ca2+ cytosolic level within the physiological range by pumping it into the vacuole. In the wild-type, Vcx1 is almost completely inhibited by calcineurin (Figure 7A). Mutants defective in HACS channel are still able to maintain physiological levels of cytosolic Ca2+ because calcineurin-dependent expression of Pmc1 is decreased. For the same reason, when this strain is challenged with HTS, the sudden Ca2+ influx is more slowly attenuated by Pmc1 (Figure 7C). In flc2Δ mutants, the flux through the MS channels is increased by removal of the inhibitory effect of Flc2 (Figure 7B). Lastly, mutants lacking calcineurin express less Pmc1, but the inhibitory effect on Vcx1 is relieved. Vcx1 only partially compensates for the reduced Pmc1 activity and the resulting higher Ca2+ levels activate calmodulin, that in turn inhibits MS channels (Figure 7D).
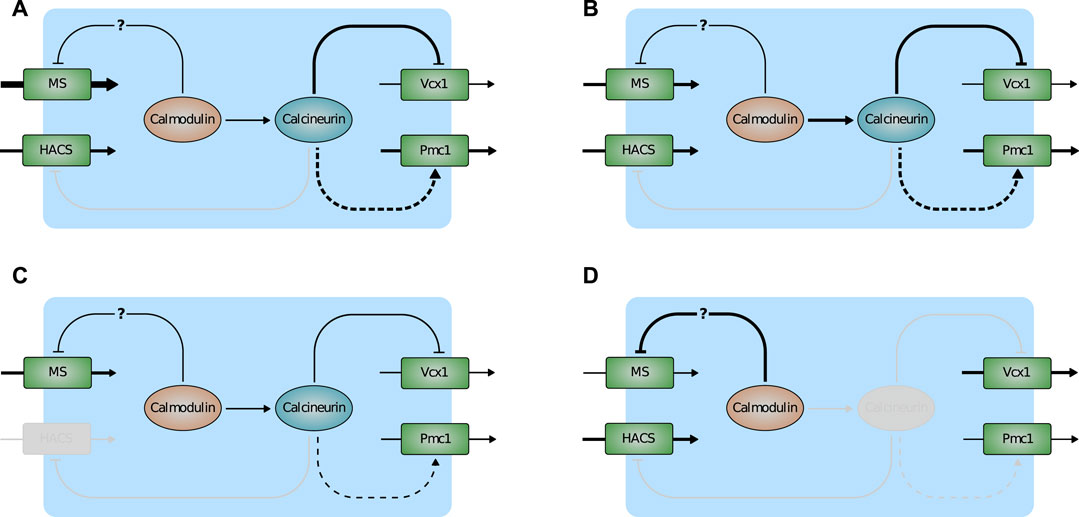
FIGURE 7. Scheme depicting the main predictions of the model. (A) Wild-type. (B) flc2Δ. (C) cch1Δ. (D) cnb1Δ. Activation is indicated by arrows, inactivation by T-bar arrows. Plain and dashed lines indicate post-transcriptional and transcriptional regulations. Line thickness represents the strenght of fluxes/(in)activations. Inactive components of the pathway are greyed-out.
The function of the Ca2+ transient is still unclear. Calcineurin regulates gene expression by promoting Crz1 movement into the nucleus (Stathopoulos and Cyert, 1997; Cai et al., 2008). During HTS, Crz1 stays in the nucleus for about 5 mins (Oh et al., 2012), but its activity is only slightly increased (Rigamonti et al., 2015). In mammalian cells, calcium is often required for regulatory volume decrease (Jakab et al., 2002), while in yeast cells it could be implicated in the regulation of the cell wall integrity pathway (Levin, 2011; Rigamonti et al., 2015). In addition to the transient increase of Ca2+, HTS rapidly stimulates the Plc1-dependent hydrolysis of phosphatidylinositol 4,5-bisphosphate (Perera et al., 2004), an event that in many cell types elicits complex calcium responses (Hille et al., 2015). However, yeast plc1Δ mutants are still able to generate the Ca2+ signal during HTS (Tisi et al., 2002) and, conversely, Plc1 activity is independent from calcium availability in the medium (Perera et al., 2004), suggesting that in yeast these events might be independent.
7 Conclusion
As extensively described, the simulations of our model are in agreement with the experimental data, suggesting that the cellular components included in the model and their interactions are sufficient to explain the biological data in our hands. The model was defined on the basis of experimental evidences from the literature, and the parameter estimation process was performed against data from both the wild-type and mutant cells, in order to maximise the prediction’s reliability. The model presented in this work could be extended to include the response to several other stimuli known to elicit a calcium signal in yeast (Iida et al., 1990; Courchesne and Ozturk, 2003; Groppi et al., 2011). Such extensions of the model can be achieved either by modifying the current system of ODEs, or by adopting hybrid modeling approaches (Spolaor et al., 2019b; Nobile et al., 2020), in order to include other functional modules in the model that can better describe the different cellular processes involved in Ca2+ signalling (including, e.g., gene regulation and expression). Extended versions of the model could be useful for understanding the role of known channels and transporters, suggesting novel putative regulatory mechanisms and gaining new insights on Ca2+ signaling that can be further investigated experimentally.
Being Ca2+ an essential signaling ion, proteins involved in its homeostasis are increasingly studied as potential targets of antifungal drugs (Kwun et al., 2021; Li et al., 2021; Wang et al., 2021). A model of Ca2+ signaling could be used to predict the outcomes of the inhibition of a particular protein on the calcium physiology of fungal cells, thus helping to identify the best drug targets. Moreover, such models could be used to study in silico the relationships between pathogenic fungi and human cells (Spolaor et al., 2019a), accelerating the discovery of new antifungal treatments. Since Ca2+ signaling is well conserved among fungi (Tisi et al., 2016), a model of S. cerevisiae would require only slight modifications to describe what happens in other, pathogenic fungi.
Finally, as a future development, we plan to perform a large-scale sensitivity analysis of the model, in order to determine the most relevant parameters governing the response to hypotonic shock. Global sensitivity analysis requires a massive amount of independent simulations, which can lead to a huge computational effort (Nobile and Mauri, 2017). In order to make this analysis feasible, we will re-implement the model using the Python library ginSODA (Nobile et al., 2019), which provides the possibility of offloading a massive number of simulations of an ODE-based model to the GPU, thus strongly reducing the overall running time.
Data Availability Statement
The model presented in this study can be found in the BioModels repository, with accession number ID MODEL2112030001, at the following link https://www.ebi.ac.uk/biomodels/MODEL2112030001.
Author Contributions
RT and DB conceived and designed the study. RT produced the experimental data. MR defined the model, performed the simulations and analysis. SS oversaw data analysis and curation, and model sharing. SS, MR, and RT wrote the first draft of the manuscript. MN, PC, and DB critically reviewed and edited the manuscript. RT and DB acquired funding. All authors reviewed and approved the manuscript.
Funding
This work was partially supported by the SYSBIO/ISBE. IT Research Centre of Systems Biology.
Conflict of Interest
The authors declare that the research was conducted in the absence of any commercial or financial relationships that could be construed as a potential conflict of interest.
Publisher’s Note
All claims expressed in this article are solely those of the authors and do not necessarily represent those of their affiliated organizations, or those of the publisher, the editors and the reviewers. Any product that may be evaluated in this article, or claim that may be made by its manufacturer, is not guaranteed or endorsed by the publisher.
Footnotes
1
References
Aguilar-Uscanga, B., and Francois, J. M. (2003). A Study of the Yeast Cell wall Composition and Structure in Response to Growth Conditions and Mode of Cultivation. Lett. Appl. Microbiol. 37, 268–274. doi:10.1046/j.1472-765x.2003.01394.x
Ahmadpour, D., Geijer, C., Tamás, M. J., Lindkvist-Petersson, K., and Hohmann, S. (2014). Yeast Reveals Unexpected Roles and Regulatory Features of Aquaporins and Aquaglyceroporins. Biochim. Biophys. Acta (BBA) - Gen. Subjects 1840, 1482–1491. doi:10.1016/j.bbagen.2013.09.027
Alsteens, D., Dupres, V., Mc Evoy, K., Wildling, L., Gruber, H. J., and Dufrêne, Y. F. (2008). Structure, Cell wall Elasticity and Polysaccharide Properties of Living Yeast Cells, as Probed by AFM. Nanotechnology 19, 384005. doi:10.1088/0957-4484/19/38/384005
Antebi, A., and Fink, G. R. (1992). The Yeast Ca(2+)-ATPase Homologue, PMR1, Is Required for normal Golgi Function and Localizes in a Novel Golgi-like Distribution. MBoC 3, 633–654. doi:10.1091/mbc.3.6.633
Bagur, R., and Hajnóczky, G. (2017). Intracellular Ca2+ Sensing: Its Role in Calcium Homeostasis and Signaling. Mol. Cel 66, 780–788. doi:10.1016/j.molcel.2017.05.028
Balcavage, W. X., Lloyd, J. L., Mattoon, J. R., Ohnishi, T., and Scarpa, A. (1973). Cation Movements and Respiratory Response in Yeast Mitochondria Treated with High Ca2+ Concentrations. Biochim. Biophys. Acta (BBA) - Bioenerg. 305, 41–51. doi:10.1016/0005-2728(73)90229-6
Balla, T. (2013). Phosphoinositides: Tiny Lipids with Giant Impact on Cell Regulation. Physiol. Rev. 93, 1019–1137. doi:10.1152/physrev.00028.2012
Batiza, A. F., Schulz, T., Masson, P. H., and Masson, P. H. (1996). Yeast Respond to Hypotonic Shock with a Calcium Pulse. J. Biol. Chem. 271, 23357–23362. doi:10.1074/jbc.271.38.23357
Beeler, T., Gable, K., Zhao, C., and Dunn, T. (1994). A Novel Protein, CSG2p, Is Required for Ca2+ Regulation in Saccharomyces cerevisiae. J. Biol. Chem. 269, 7279–7284. doi:10.1016/s0021-9258(17)37280-0
Belde, P. J. M., Vossen, J. H., Borst-Pauwels, G. W. F. H., and Theuvenet, A. P. R. (1993). Inositol 1,4,5-trisphosphate Releases Ca2+ from Vacuolar Membrane Vesicles of Saccharomyces cerevisiae. FEBS Lett. 323, 113–118. doi:10.1016/0014-5793(93)81460-h
Berridge, M. J., Bootman, M. D., and Roderick, H. L. (2003). Calcium Signalling: Dynamics, Homeostasis and Remodelling. Nat. Rev. Mol. Cel Biol. 4, 517–529. doi:10.1038/nrm1155
Berridge, M. J., Lipp, P., and Bootman, M. D. (2000). The Versatility and Universality of Calcium Signalling. Nat. Rev. Mol. Cel Biol. 1, 11–21. doi:10.1038/35036035
Besozzi, D., Manzoni, L., Nobile, M. S., Spolaor, S., Castelli, M., Vanneschi, L., et al. (2020). Computational Intelligence for Life Sciences. Fundamenta Informaticae 171, 57–80.
Birchwood, C. J., Saba, J. D., Dickson, R. C., and Cunningham, K. W. (2001). Calcium Influx and Signaling in Yeast Stimulated by Intracellular Sphingosine 1-phosphate Accumulation. J. Biol. Chem. 276, 11712–11718. doi:10.1074/jbc.m010221200
Bonilla, M., and Cunningham, K. W. (2003). Mitogen-activated Protein Kinase Stimulation of Ca2+ Signaling Is Required for Survival of Endoplasmic Reticulum Stress in Yeast. MBoC 14, 4296–4305. doi:10.1091/mbc.e03-02-0113
Bonilla, M., Nastase, K. K., and Cunningham, K. W. (2002). Essential Role of Calcineurin in Response to Endoplasmic Reticulum Stress. EMBO J. 21, 2343–2353. doi:10.1093/emboj/21.10.2343
Cai, L., Dalal, C. K., and Elowitz, M. B. (2008). Frequency-modulated Nuclear Localization Bursts Coordinate Gene Regulation. Nature 455, 485–490. doi:10.1038/nature07292
Carafoli, E., Balcavage, W. X., Lehninger, A. L., and Mattoon, J. R. (1970). Ca2+ Metabolism in Yeast Cells and Mitochondria. Biochim. Biophys. Acta (BBA) - Bioenerg. 205, 18–26. doi:10.1016/0005-2728(70)90057-5
Carafoli, E. (2004). Calcium-mediated Cellular Signals: a story of Failures. Trends Biochem. Sci. 29, 371–379. doi:10.1016/j.tibs.2004.05.006
Christopher, P. F. (2002). Computational Cell Biology. In Interdisciplinary Applied Mathematics; V. 20. Springer-Verlag: New York.
Courchesne, W. E., and Ozturk, S. (2003). Amiodarone Induces a caffeine‐inhibited, MID1‐dependent Rise in Free Cytoplasmic Calcium in Saccharomyces cerevisiae. Mol. Microbiol. 47, 223–234. doi:10.1046/j.1365-2958.2003.03291.x
Cox, C. D., Nomura, T., Ziegler, C. S., Campbell, A. K., Wann, K. T., and Martinac, B. (2013). Selectivity Mechanism of the Mechanosensitive Channel MscS Revealed by Probing Channel Subconducting States. Nat. Commun. 4, 2137. doi:10.1038/ncomms3137
Cui, J., Kaandorp, J. A., Ositelu, O. O., Beaudry, V., Knight, A., Nanfack, Y. F., et al. (2009a). Simulating Calcium Influx and Free Calcium Concentrations in Yeast. Cell Calcium 45, 123–132. doi:10.1016/j.ceca.2008.07.005
Cui, J., Kaandorp, J. A., Sloot, P. M. A., Lloyd, C. M., and Filatov, M. V. (2009b). Calcium Homeostasis and Signaling in Yeast Cells and Cardiac Myocytes. FEMS Yeast Res. 9, 1137–1147. doi:10.1111/j.1567-1364.2009.00552.x
Cunningham, K. W., and Fink, G. R. (1994b). Calcineurin-dependent Growth Control in Saccharomyces cerevisiae Mutants Lacking PMC1, a Homolog of Plasma Membrane Ca2+ ATPases. J. Cel Biol. 124, 351–363. doi:10.1083/jcb.124.3.351
Cunningham, K. W., and Fink, G. R. (1994a). Ca2+ Transport in Saccharomyces cerevisiae. J. Exp. Biol. 196, 157–166. doi:10.1242/jeb.196.1.157
Cunningham, K. W., and Fink, G. R. (1996). Calcineurin Inhibits VCX1-dependent H+/Ca2+ Exchange and Induces Ca2+ ATPases in Saccharomyces cerevisiae. Mol. Cel. Biol. 16, 2226–2237. doi:10.1128/mcb.16.5.2226
Cyert, M. S. (2003). Calcineurin Signaling in Saccharomyces cerevisiae: How Yeast Go Crazy in Response to Stress. Biochem. Biophysical Res. Commun. 311, 1143–1150. doi:10.1016/s0006-291x(03)01552-3
Cyert, M. S. (2001). Genetic Analysis of Calmodulin and its Targets in Saccharomyces cerevisiae. Annu. Rev. Genet. 35, 647–672. doi:10.1146/annurev.genet.35.102401.091302
Cyert, M. S., and Thorner, J. (1992). Regulatory Subunit (CNB1 Gene Product) of Yeast Ca2+/calmodulin-dependent Phosphoprotein Phosphatases Is Required for Adaptation to Pheromone. Mol. Cel. Biol. 12, 3460–3469. doi:10.1128/mcb.12.8.3460
Davis, T. N., Urdea, M. S., Masiarz, F. R., and Thorner, J. (1986). Isolation of the Yeast Calmodulin Gene: Calmodulin Is an Essential Protein. Cell 47, 423–431. doi:10.1016/0092-8674(86)90599-4
Denis, V., and Cyert, M. S. (2002). Internal Ca2+ Release in Yeast Is Triggered by Hypertonic Shock and Mediated by a TRP Channel Homologue. J. Cel Biol. 156, 29–34. doi:10.1083/jcb.200111004
Dunn, T., Gable, K., and Beeler, T. (1994). Regulation of Cellular Ca2+ by Yeast Vacuoles. J. Biol. Chem. 269, 7273–7278. doi:10.1016/s0021-9258(17)37279-4
Dupont, G., Combettes, L., and Leybaert, L. (2007). Calcium Dynamics: Spatio‐Temporal Organization from the Subcellular to the Organ Level. Int. Rev. Cytol. 261, 193–245. doi:10.1016/s0074-7696(07)61005-5
Dürr, G., Strayle, J., Plemper, R., Elbs, S., Klee, S. K., Catty, P., et al. (1998). Themedial-Golgi Ion Pump Pmr1 Supplies the Yeast Secretory Pathway with Ca2+ and Mn2+ Required for Glycosylation, Sorting, and Endoplasmic Reticulum-Associated Protein Degradation. MBoC 9, 1149–1162. doi:10.1091/mbc.9.5.1149
Eilam, Y. (1982a). Studies on Calcium Efflux in the Yeast Saccharomyces cerevisiae. Microbios 35, 99–110.
Eilam, Y., and Chernichovsky, D. (1987). Uptake of Ca2+ Driven by the Membrane Potential in Energy-Depleted Yeast Cells. Microbiology 133, 1641–1649. doi:10.1099/00221287-133-6-1641
Eilam, Y., and Othman, M. (1990). Activation of Ca2+ influx by Metabolic Substrates in Saccharomyces cerevisiae: Role of Membrane Potential and Cellular ATP Levels. J. Gen. Microbiol. 136, 861–866. doi:10.1099/00221287-136-5-861
Eilam, Y. (1982b). The Effect of Monovalent Cations on Calcium Efflux in Yeasts. Biochim. Biophys. Acta (BBA) - Biomembranes 687, 8–16. doi:10.1016/0005-2736(82)90164-x
Fischer, M., Schnell, N., Chattaway, J., Davies, P., Dixon, G., and Sanders, D. (1997). The Saccharomyces cerevisiae CCH1 Gene Is Involved in Calcium Influx and Mating. FEBS Lett. 419, 259–262. doi:10.1016/s0014-5793(97)01466-x
Fridlyand, L. E., Tamarina, N., and Philipson, L. H. (2003). Modeling of Ca2+ Flux in Pancreatic β-cells: Role of the Plasma Membrane and Intracellular Stores. Am. J. Physiology-Endocrinology Metab. 285, E138–E154. doi:10.1152/ajpendo.00194.2002
Giorgi, C., Marchi, S., and Pinton, P. (2018). The Machineries, Regulation and Cellular Functions of Mitochondrial Calcium. Nat. Rev. Mol. Cel Biol 19, 713–730. doi:10.1038/s41580-018-0052-8
Griffin, D. M. (1981). “Water and Microbial Stress,” in Advances in Microbial Ecology (Springer), 91–136. doi:10.1007/978-1-4615-8306-6_3
Groppi, S., Belotti, F., Brandão, R. L., Martegani, E., and Tisi, R. (2011). Glucose-induced Calcium Influx in Budding Yeast Involves a Novel Calcium Transport System and Can Activate Calcineurin. Cell Calcium 49, 376–386. doi:10.1016/j.ceca.2011.03.006
Gustin, M. C., Zhou, X.-L., Martinac, B., and Kung, C. (1988). A Mechanosensitive Ion Channel in the Yeast Plasma Membrane. Science 242, 762–765. doi:10.1126/science.2460920
Halachmi, D., and Eilam, Y. (1996). Elevated Cytosolic Free Ca2+ concentrations and Massive Ca2+ accumulation within Vacuoles, in Yeast Mutant lacking PMR1, a Homolog of Ca2+-ATPase. FEBS Lett. 392, 194–200. doi:10.1016/0014-5793(96)00799-5
Hille, B., Dickson, E. J., Kruse, M., Vivas, O., and Suh, B.-C. (2015). Phosphoinositides Regulate Ion Channels. Biochim. Biophys. Acta (BBA) - Mol. Cel Biol. Lipids 1851, 844–856. doi:10.1016/j.bbalip.2014.09.010
Ho, B., Baryshnikova, A., and Brown, G. W. (2018). Unification of Protein Abundance Datasets Yields a Quantitative Saccharomyces cerevisiae Proteome. Cel Syst. 6, 192–205. doi:10.1016/j.cels.2017.12.004
Hohmann, S. (2002). Osmotic Stress Signaling and Osmoadaptation in Yeasts. Microbiol. Mol. Biol. Rev. 66, 300–372. doi:10.1128/mmbr.66.2.300-372.2002
Hong, S., Cong, X., Jing, H., Xia, Z., Huang, X., Hu, X., et al. (2013). Characterization of Ca2+/H+ Exchange in the Plasma Membrane of Saccharomyces cerevisiae. Arch. Biochem. Biophys. 537, 125–132. doi:10.1016/j.abb.2013.07.005
Hoops, S., Sahle, S., Gauges, R., Lee, C., Pahle, J., Simus, N., et al. (2006). COPASI--a COmplex PAthway SImulator. Bioinformatics 22, 3067–3074. doi:10.1093/bioinformatics/btl485
Iida, H., Nakamura, H., Ono, T., Okumura, M. S., and Anraku, Y. (1994). MID1, a Novel Saccharomyces cerevisiae Gene Encoding a Plasma Membrane Protein, Is Required for Ca2+ Influx and Mating. Mol. Cel. Biol. 14, 8259–8271. doi:10.1128/mcb.14.12.8259
Iida, H., Yagawa, Y., and Anraku, Y. (1990). Essential Role for Induced Ca2+ Influx Followed by [Ca2+]i Rise in Maintaining Viability of Yeast Cells Late in the Mating Pheromone Response Pathway. A Study of [Ca2+]i in Single Saccharomyces cerevisiae Cells with Imaging of Fura-2. J. Biol. Chem. 265, 13391–13399. doi:10.1016/s0021-9258(19)38311-5
Ishida, H., Nakashima, K.-i., Kumaki, Y., Nakata, M., Hikichi, K., and Yazawa, M. (2002). The Solution Structure of Apocalmodulin from Saccharomyces cerevisiae Implies a Mechanism for its Unique Ca2+ Binding Property. Biochemistry 41, 15536–15542. doi:10.1021/bi020330r
Jakab, M., Fuerst, J., Gschwentner, M., Bottà, G., Garavaglia, M.-L., Bazzini, C., et al. (2002). Mechanisms Sensing and Modulating Signals Arising from Cell Swelling. Cell. Physiol. Biochem. 12, 235–258. doi:10.1159/000067895
Jiang, H., and Sun, S. X. (2013). Cellular Pressure and Volume Regulation and Implications for Cell Mechanics. Biophysical J. 105, 609–619. doi:10.1016/j.bpj.2013.06.021
Jung, D. W., Bradshaw, P. C., Litsky, M., and Pfeiffer, D. R. (2004). Ca2+ Transport in Mitochondria from Yeast Expressing Recombinant Aequorin. Anal. Biochem. 324, 258–268. doi:10.1016/j.ab.2003.10.029
Kanzaki, M., Nagasawa, M., Kojima, I., Sato, C., Naruse, K., Sokabe, M., et al. (1999). Molecular Identification of a Eukaryotic, Stretch-Activated Nonselective Cation Channel. Science 285, 882–886. doi:10.1126/science.285.5429.882
Kayingo, G., Kilian, S., and Prior, B. (2001). Conservation and Release of Osmolytes by Yeasts during Hypo-Osmotic Stress. Arch. Microbiol. 177, 29–35. doi:10.1007/s00203-001-0358-2
Kedem, O., and Katchalsky, A. (1958). Thermodynamic Analysis of the Permeability of Biological Membranes to Non-electrolytes. Biochim. Biophys. Acta 27, 229–246. doi:10.1016/0006-3002(58)90330-5
Kennedy, J., and Eberhart, R. (1995). “Particle Swarm Optimization,” In Proceedings of IEEE International Conference on Neural Networks. IEEE, 1942–1948.
Kingsbury, T. J., and Cunningham, K. W. (2000). A Conserved Family of Calcineurin Regulators. Genes Dev. 14, 1595–1604. doi:10.1101/gad.14.13.1595
Kleinhans, F. W. (1998). Membrane Permeability Modeling: Kedem-Katchalsky vs a Two-Parameter Formalism. Cryobiology 37, 271–289. doi:10.1006/cryo.1998.2135
Kwun, M. S., Lee, H. J., and Lee, D. G. (2021). β-amyrin-induced Apoptosis in Candida albicans Triggered by Calcium. Fungal Biol.. doi:10.1016/j.funbio.2021.03.006
Levin, D. E. (2011). Regulation of Cell wall Biogenesis in Saccharomyces cerevisiae: the Cell wall Integrity Signaling Pathway. Genetics 189, 1145–1175. doi:10.1534/genetics.111.128264
Li, H., Rao, A., and Hogan, P. G. (2011). Interaction of Calcineurin with Substrates and Targeting Proteins. Trends Cel Biol. 21, 91–103. doi:10.1016/j.tcb.2010.09.011
Li, W., Shrivastava, M., Lu, H., and Jiang, Y. (2021). Calcium-calcineurin Signaling Pathway in Candida albicans: A Potential Drug Target, 249. Microbiological research, 126786. doi:10.1016/j.micres.2021.126786
Locke, E. G., Bonilla, M., Liang, L., Takita, Y., and Cunningham, K. W. (2000). A Homolog of Voltage-Gated Ca2+ Channels Stimulated by Depletion of Secretory Ca2+ in Yeast. Mol. Cel. Biol. 20, 6686–6694. doi:10.1128/mcb.20.18.6686-6694.2000
Luyten, K., Albertyn, J., Skibbe, W. F., Prior, B. A., Ramos, J., Thevelein, J. M., et al. (1995). Fps1, a Yeast Member of the MIP Family of Channel Proteins, Is a Facilitator for Glycerol Uptake and Efflux and Is Inactive under Osmotic Stress. EMBO J. 14, 1360–1371. doi:10.1002/j.1460-2075.1995.tb07122.x
Ma, T.-Y., Deprez, M.-A., Callewaert, G., and Winderickx, J. (2021). Coordinated Glucose-Induced Ca2+ and Ph Responses in Yeast Saccharomyces cerevisiae. Cell Calcium 100, 102479. doi:10.1016/j.ceca.2021.102479
Malik-Sheriff, R. S., Glont, M., Nguyen, T. V. N., Tiwari, K., Roberts, M. G., Xavier, A., et al. (2020). BioModels-15 Years of Sharing Computational Models in Life Science. Nucleic Acids Res. 48, D407–D415. doi:10.1093/nar/gkz1055
Matsumoto, T. K., Ellsmore, A. J., Cessna, S. G., Low, P. S., Pardo, J. M., Bressan, R. A., et al. (2002). An Osmotically Induced Cytosolic Ca2+ Transient Activates Calcineurin Signaling to Mediate Ion Homeostasis and Salt Tolerance of Saccharomyces cerevisiae. J. Biol. Chem. 277, 33075–33080. doi:10.1074/jbc.m205037200
Milo, R., Jorgensen, P., Moran, U., Weber, G., and Springer, M. (2010). BioNumbers-the Database of Key Numbers in Molecular and Cell Biology. Nucleic Acids Res. 38, D750–D753. doi:10.1093/nar/gkp889
Miseta, A., Fu, L., Kellermayer, R., Buckley, J., and Bedwell, D. M. (1999a). The Golgi Apparatus Plays a Significant Role in the Maintenance of Ca2+ Homeostasis in the vps33Δ Vacuolar Biogenesis Mutant of Saccharomyces cerevisiae. J. Biol. Chem. 274, 5939–5947. doi:10.1074/jbc.274.9.5939
Miseta, A., Kellermayer, R., Aiello, D. P., Fu, L., and Bedwell, D. M. (1999b). The Vacuolar Ca2+/H+ exchanger Vcx1p/Hum1p Tightly Controls Cytosolic Ca2+ levels in S. cerevisiae. FEBS Lett. 451, 132–136. doi:10.1016/s0014-5793(99)00519-0
Montefusco, D. J., Matmati, N., and Hannun, Y. A. (2014). The Yeast Sphingolipid Signaling Landscape. Chem. Phys. Lipids 177, 26–40. doi:10.1016/j.chemphyslip.2013.10.006
Montero, M., Brini, M., Marsault, R., Alvarez, J., Sitia, R., Pozzan, T., et al. (1995). Monitoring Dynamic Changes in Free Ca2+ Concentration in the Endoplasmic Reticulum of Intact Cells. EMBO J. 14, 5467–5475. doi:10.1002/j.1460-2075.1995.tb00233.x
Muller, E. M., Locke, E. G., and Cunningham, K. W. (2001). Differential Regulation of Two Ca2+ Influx Systems by Pheromone Signaling in Saccharomyces cerevisiae. Genetics 159, 1527–1538. doi:10.1093/genetics/159.4.1527
Muller, E. M., Mackin, N. A., Erdman, S. E., and Cunningham, K. W. (2003). Fig1p Facilitates Ca2+ Influx and Cell Fusion during Mating of Saccharomyces cerevisiae. J. Biol. Chem. 278, 38461–38469. doi:10.1074/jbc.m304089200
Nakashima, K.-i., Ishida, H., Ohki, S.-y., Hikichi, K., and Yazawa, M. (1999). Calcium Binding Induces Interaction between the N- and C-Terminal Domains of Yeast Calmodulin and Modulates its Overall Conformation. Biochemistry 38, 98–104. doi:10.1021/bi982067t
Nakashima, K., Ishida, H., Nakatomi, A., and Yazawa, M. (2012). Specific Conformation and Ca2+-Binding Mode of Yeast Calmodulin: Insight into Evolutionary Development. J. Biochem. 152, 27–35. doi:10.1093/jb/mvs048
Niedzwiecka, K., Tisi, R., Penna, S., Lichocka, M., Plochocka, D., and Kucharczyk, R. (2018). Two Mutations in Mitochondrial ATP6 Gene of ATP Synthase, Related to Human Cancer, Affect ROS, Calcium Homeostasis and Mitochondrial Permeability Transition in Yeast. Biochim. Biophys. Acta (BBA) - Mol. Cel Res. 1865, 117–131. doi:10.1016/j.bbamcr.2017.10.003
Nobile, M. S., and Mauri, G. (2017). “Accelerated Analysis of Biological Parameters Space Using GPUs,” in International Conference on Parallel Computing Technologies (Springer), 70–81. doi:10.1007/978-3-319-62932-2_6
Nobile, M. S., Tangherloni, A., Rundo, L., Spolaor, S., Besozzi, D., Mauri, G., et al. (2018). “Computational Intelligence for Parameter Estimation of Biochemical Systems,” in 2018 IEEE Congress on Evolutionary Computation (CEC) (IEEE)–18. doi:10.1109/cec.2018.8477873
Nobile, M. S., Votta, G., Palorini, R., Spolaor, S., De Vitto, H., Cazzaniga, P., et al. (2020). Fuzzy Modeling and Global Optimization to Predict Novel Therapeutic Targets in Cancer Cells. Bioinformatics 36, 2181–2188. doi:10.1093/bioinformatics/btz868
Odorizzi, G., Babst, M., and Emr, S. D. (2000). Phosphoinositide Signaling and the Regulation of Membrane Trafficking in Yeast. Trends Biochem. Sci. 25, 229–235. doi:10.1016/s0968-0004(00)01543-7
Ogura, K., Kumeta, H., Takahasi, K., Kobashigawa, Y., Yoshida, R., Itoh, H., et al. (2012a). Solution Structures of Yeast Saccharomyces cerevisiae Calmodulin in Calcium- and Target Peptide-Bound States Reveal Similarities and Differences to Vertebrate Calmodulin. Genes Cells 17, 159–172. doi:10.1111/j.1365-2443.2012.01580.x
Ogura, K., Okamura, H., Katahira, M., Katoh, E., and Inagaki, F. (2012b). Conformational Dynamics of Yeast Calmodulin in the Ca2+-Bound State Probed Using NMR Relaxation Dispersion. FEBS Lett. 586, 2548–2554. doi:10.1016/j.febslet.2012.06.031
Oh, S., Lee, S. S., Ryu, H. R., Park, J. W., Peter, M., and Jeon, N. L. (2012). “Unraveling Mechano-Stress Responsive Signaling Networks in Budding Yeast via Microfluidic Devices,” in 16th International Conference on Miniaturized Systems for Chemistry and Life Sciences (MicroTAS 2012), 1 449–451.
Ohsumi, Y., and Anraku, Y. (1983). Calcium Transport Driven by a Proton Motive Force in Vacuolar Membrane Vesicles of Saccharomyces cerevisiae. J. Biol. Chem. 258, 5614–5617. doi:10.1016/s0021-9258(20)81935-8
Okada, Y., Maeno, E., Shimizu, T., Dezaki, K., Wang, J., and Morishima, S. (2001). Receptor‐mediated Control of Regulatory Volume Decrease (RVD) and Apoptotic Volume Decrease (AVD). J. Physiol. 532, 3–16. doi:10.1111/j.1469-7793.2001.0003g.x
Orlean, P. (2012). Architecture and Biosynthesis of the Saccharomyces cerevisiae Cell wall. Genetics 192, 775–818. doi:10.1534/genetics.112.144485
Paidhungat, M., and Garrett, S. (1997). A Homolog of Mammalian, Voltage-Gated Calcium Channels Mediates Yeast Pheromone-Stimulated Ca2+ Uptake and Exacerbates the cdc1(Ts) Growth Defect. Mol. Cel. Biol. 17, 6339–6347. doi:10.1128/mcb.17.11.6339
Palmer, C. P., Zhou, X.-L., Lin, J., Loukin, S. H., Kung, C., and Saimi, Y. (2001). A TRP Homolog in Saccharomyces cerevisiae Forms an Intracellular Ca2+ permeable Channel in the Yeast Vacuolar Membrane. Proc. Natl. Acad. Sci. U.S.A. 98, 7801–7805. doi:10.1073/pnas.141036198
Pedersen, S. F., Kapus, A., and Hoffmann, E. K. (2011). Osmosensory Mechanisms in Cellular and Systemic Volume Regulation. Jasn 22, 1587–1597. doi:10.1681/asn.2010121284
Perera, N. M., Michell, R. H., and Dove, S. K. (2004). Hypo-osmotic Stress Activates Plc1p-dependent Phosphatidylinositol 4,5-bisphosphate Hydrolysis and Inositol Hexakisphosphate Accumulation in Yeast. J. Biol. Chem. 279, 5216–5226. doi:10.1074/jbc.m305068200
Petzold, L. (1983). Automatic Selection of Methods for Solving Stiff and Nonstiff Systems of Ordinary Differential Equations. SIAM J. Sci. Stat. Comput. 4, 136–148. doi:10.1137/0904010
Pinton, P., Pozzan, T., and Rizzuto, R. (1998). The Golgi Apparatus Is an Inositol 1,4,5-Trisphosphate-Sensitive Ca2+ Store, with Functional Properties Distinct from Those of the Endoplasmic Reticulum. EMBO J. 17, 5298–5308. doi:10.1093/emboj/17.18.5298
Pizzo, P., Lissandron, V., Capitanio, P., and Pozzan, T. (2011). Ca2+ Signalling in the Golgi Apparatus. Cell Calcium 50, 184–192. doi:10.1016/j.ceca.2011.01.006
Plattner, H., and Verkhratsky, A. (2015a). Evolution of Calcium Signalling. Cell Calcium 57, 121–122. doi:10.1016/j.ceca.2015.02.007
Plattner, H., and Verkhratsky, A. (2015b). The Ancient Roots of Calcium Signalling Evolutionary Tree. Cell Calcium 57, 123–132. doi:10.1016/j.ceca.2014.12.004
Purvis, J. E., and Lahav, G. (2013). Encoding and Decoding Cellular Information through Signaling Dynamics. Cell 152, 945–956. doi:10.1016/j.cell.2013.02.005
Reed, R. H., Chudek, J. A., Foster, R., and Gadd, G. M. (1987). Osmotic Significance of Glycerol Accumulation in Exponentially Growing Yeasts. Appl. Environ. Microbiol. 53, 2119–2123. doi:10.1128/aem.53.9.2119-2123.1987
Rhoads, A. R., and Friedberg, F. (1997). Sequence Motifs for Calmodulin Recognition. FASEB j. 11, 331–340. doi:10.1096/fasebj.11.5.9141499
Rigamonti, M., Groppi, S., Belotti, F., Ambrosini, R., Filippi, G., Martegani, E., et al. (2015). Hypotonic Stress-Induced Calcium Signaling in Saccharomyces cerevisiae Involves TRP-like Transporters on the Endoplasmic Reticulum Membrane. Cell Calcium 57, 57–68. doi:10.1016/j.ceca.2014.12.003
Rudolph, H. K., Antebi, A., Fink, G. R., Buckley, C. M., Dorman, T. E., LeVitre, J., et al. (1989). The Yeast Secretory Pathway Is Perturbed by Mutations in PMR1, a Member of a Ca2+ ATPase Family. Cell 58, 133–145. doi:10.1016/0092-8674(89)90410-8
Sachs, F., and Morris, C. E. (1998). “Mechanosensitive Ion Channels in Nonspecialized Cells,” in Reviews of Physiology Biochemistry and Pharmacology (Springer), 132, 1–77.
Sackin, H. (1995). Stretch-activated Ion Channels. Kidney Int. 48, 1134–1147. doi:10.1038/ki.1995.397
Saimi, Y., and Kung, C. (2002). Calmodulin as an Ion Channel Subunit. Annu. Rev. Physiol. 64, 289–311. doi:10.1146/annurev.physiol.64.100301.111649
Sammels, E., Parys, J. B., Missiaen, L., De Smedt, H., and Bultynck, G. (2010). Intracellular Ca2+ Storage in Health and Disease: a Dynamic Equilibrium. Cell Calcium 47, 297–314. doi:10.1016/j.ceca.2010.02.001
Schaber, J., Adrover, M. À., Eriksson, E., Pelet, S., Petelenz-Kurdziel, E., Klein, D., et al. (2010). Biophysical Properties of Saccharomyces cerevisiae and Their Relationship with HOG Pathway Activation. Eur. Biophys. J. 39, 1547–1556. doi:10.1007/s00249-010-0612-0
Schaber, J., and Klipp, E. (2008). Short-term Volume and Turgor Regulation in Yeast. Essays Biochem. 45, 147–160. doi:10.1042/bse0450147
Nobile, M. S., Cazzaniga, P., Besozzi, D., and Mauri, G. (2019). ginSODA: Massive Parallel Integration of Stiff ODE Systems on GPUs. J. Supercomput 75, 7844–7856. doi:10.1007/s11227-018-2549-5
Sorin, A., Rosas, G., and Rao, R. (1997). PMR1, a Ca2+-ATPase in Yeast Golgi, Has Properties Distinct from Sarco/endoplasmic Reticulum and Plasma Membrane Calcium Pumps. J. Biol. Chem. 272, 9895–9901. doi:10.1074/jbc.272.15.9895
Spolaor, S., Gribaudo, M., Iacono, M., Kadavy, T., Komínková Oplatková, Z., Mauri, G., et al. (2019a). “Towards Human Cell Simulation,” in High-Performance Modelling and Simulation for Big Data Applications (Cham: Springer), 221–249. doi:10.1007/978-3-030-16272-6_8
Spolaor, S., Nobile, M. S., Mauri, G., Cazzaniga, P., and Besozzi, D. (2019b). Coupling Mechanistic Approaches and Fuzzy Logic to Model and Simulate Complex Systems. IEEE Trans. Fuzzy Syst. 28, 1748–1759.
Starovasnik, M. A., Davis, T. N., and Klevit, R. E. (1993). Similarities and Differences between Yeast and Vertebrate Calmodulin: an Examination of the Calcium-Binding and Structural Properties of Calmodulin from the Yeast Saccharomyces cerevisiae. Biochemistry 32, 3261–3270. doi:10.1021/bi00064a008
Stathopoulos, A. M., and Cyert, M. S. (1997). Calcineurin Acts through the CRZ1/TCN1-Encoded Transcription Factor to Regulate Gene Expression in Yeast. Genes Dev. 11, 3432–3444. doi:10.1101/gad.11.24.3432
Stefan, C. J., Manford, A. G., and Emr, S. D. (2013a). ER-PM Connections: Sites of Information Transfer and Inter-organelle Communication. Curr. Opin. Cel Biol. 25, 434–442. doi:10.1016/j.ceb.2013.02.020
Stefan, C. P., and Cunningham, K. W. (2013). Kch1 Family Proteins Mediate Essential Responses to Endoplasmic Reticulum Stresses in the Yeasts Saccharomyces cerevisiae and Candida Albicans. J. Biol. Chem. 288, 34861–34870. doi:10.1074/jbc.m113.508705
Stefan, C. P., Zhang, N., Sokabe, T., Rivetta, A., Slayman, C. L., Montell, C., et al. (2013b). Activation of an Essential Calcium Signaling Pathway in Saccharomyces cerevisiae by Kch1 and Kch2, Putative Low-Affinity Potassium Transporters. Eukaryot. Cel 12, 204–214. doi:10.1128/ec.00299-12
Strahl, T., and Thorner, J. (2007). Synthesis and Function of Membrane Phosphoinositides in Budding Yeast, Saccharomyces cerevisiae. Biochim. Biophys. Acta (BBA) - Mol. Cel Biol. Lipids 1771, 353–404. doi:10.1016/j.bbalip.2007.01.015
Strayle, J., Pozzan, T., and Rudolph, H. K. (1999). Steady-state Free Ca2+ in the Yeast Endoplasmic Reticulum Reaches Only 10 microM and Is Mainly Controlled by the Secretory Pathway Pump Pmr1. EMBO J. 18, 4733–4743. doi:10.1093/emboj/18.17.4733
Sukharev, S. I., Sigurdson, W. J., Kung, C., Sachs, F., and Sukharev, S. I. (1999). Energetic and Spatial Parameters for Gating of the Bacterial Large Conductance Mechanosensitive Channel, MscL. J. Gen. Physiol. 113, 525–540. doi:10.1085/jgp.113.4.525
Takita, Y., Engstrom, L., Ungermann, C., and Cunningham, K. W. (2001). Inhibition of the Ca2+-ATPase Pmc1p by the V-SNARE Protein Nyv1p. J. Biol. Chem. 276, 6200–6206. doi:10.1074/jbc.m009191200
Talemi, S. R., Tiger, C.-F., Andersson, M., Babazadeh, R., Welkenhuysen, N., Klipp, E., et al. (2016). Systems Level Analysis of the Yeast Osmo-Stat. Sci. Rep. 6, 30950. doi:10.1038/srep30950
Tamas, M. J., Luyten, K., Sutherland, F. C. W., Hernandez, A., Albertyn, J., Valadi, H., et al. (1999). Fps1p Controls the Accumulation and Release of the Compatible Solute Glycerol in Yeast Osmoregulation. Mol. Microbiol. 31, 1087–1104. doi:10.1046/j.1365-2958.1999.01248.x
Tangherloni, A., Spolaor, S., Cazzaniga, P., Besozzi, D., Rundo, L., Mauri, G., et al. (2019). Biochemical Parameter Estimation vs. Benchmark Functions: A Comparative Study of Optimization Performance and Representation Design. Appl. Soft Comput. 81, 105494. doi:10.1016/j.asoc.2019.105494
Tanida, I., Takita, Y., Hasegawa, A., Ohya, Y., and Anraku, Y. (1996). Yeast Cls2p/Csg2p Localized on the Endoplasmic Reticulum Membrane Regulates a Non-exchangeable Intracellular Ca2+ Pool Cooperatively with Calcineurin. FEBS Lett. 379, 38–42. doi:10.1016/0014-5793(95)01478-0
Teng, J., Goto, R., Iida, K., Kojima, I., and Iida, H. (2008). Ion-channel Blocker Sensitivity of Voltage-Gated Calcium-Channel Homologue Cch1 in Saccharomyces cerevisiae. Microbiology (Reading, Engl.) 154, 3775–3781. doi:10.1099/mic.0.2008/021089-0
Tisi, R., Baldassa, S., Belotti, F., and Martegani, E. (2002). Phospholipase C Is Required for Glucose-Induced Calcium Influx in Budding Yeast. FEBS Lett. 520, 133–138. doi:10.1016/s0014-5793(02)02806-5
Tisi, R., Belotti, F., Wera, S., Winderickx, J., Thevelein, J. M., and Martegani, E. (2004). Evidence for Inositol Triphosphate as a Second Messenger for Glucose-Induced Calcium Signalling in Budding Yeast. Curr. Genet. 45, 83–89. doi:10.1007/s00294-003-0465-5
Tisi, R., Rigamonti, M., Groppi, S., and Belotti, F. (2016). Calcium Homeostasis and Signaling in Fungi and Their Relevance For Pathogenicity of Yeasts and Filamentous Fungi. AIMS Mol. Sci. 3, 505–549. doi:10.3934/molsci.2016.4.505
Viladevall, L., Serrano, R., Ruiz, A., Domenech, G., Giraldo, J., Barceló, A., et al. (2004). Characterization of the Calcium-Mediated Response to Alkaline Stress in Saccharomyces cerevisiae. J. Biol. Chem. 279, 43614–43624. doi:10.1074/jbc.m403606200
Wang, L., Zhang, Y., Yang, D., Zhang, S., Han, D., and Luo, Y. (2021). Aureoverticillactam, a Potent Antifungal Macrocyclic Lactam from Streptomyces Aureoverticillatus Nh6, Generates Calcium Dyshomeostasis Induced Cell Apoptosis via the Phospholipase C Pathway in fusarium Oxysporum F. Sp. Cubense Race 4. Phytopathology.
Wei, Y., Marchi, V., Wang, R., and Rao, R. (1999). An N-Terminal EF Hand-like Motif Modulates Ion Transport by Pmr1, the Yeast Golgi Ca2+/Mn2+-ATPase. Biochemistry 38, 14534–14541. doi:10.1021/bi9911233
Weijiu, L. (2012). Introduction to Modeling Biological Cellular Control Systems, 6. Springer Science & Business Media.
Weskamp, M., Seidl, W., and Grissmer, S. (2000). Characterization of the Increase in [Ca2+] I during Hypotonic Shock and the Involvement of Ca2+-Activated K+ Channels in the Regulatory Volume Decrease in Human Osteoblast-like Cells. J. Membr. Biol. 178, 11–20. doi:10.1007/s002320010010
Wuytack, F., Raeymaekers, L., and Missiaen, L. (2003). PMR1/SPCA Ca2+ Pumps and the Role of the Golgi Apparatus as a Ca2+ Store. Pflugers Arch. - Eur. J. Physiol. 446, 148–153. doi:10.1007/s00424-003-1011-5
Yap, K. L., Kim, J., Truong, K., Sherman, M., Yuan, T., and Ikura, M. (2000). Calmodulin Target Database. J. Struct. Funct. Genomics 1, 8–14. doi:10.1023/a:1011320027914
Yoshimoto, H., Saltsman, K., Gasch, A. P., Li, H. X., Ogawa, N., Botstein, D., et al. (2002). Genome-wide Analysis of Gene Expression Regulated by the calcineurin/Crz1p Signaling Pathway in Saccharomyces cerevisiae. J. Biol. Chem. 277, 31079–31088. doi:10.1074/jbc.m202718200
Yoshimura, H., Tada, T., and Iida, H. (2004). Subcellular Localization and Oligomeric Structure of the Yeast Putative Stretch-Activated Ca2+ Channel Component Mid1. Exp. Cel Res. 293, 185–195. doi:10.1016/j.yexcr.2003.09.020
Zheng, J. (2013). Molecular Mechanism of TRP Channels. Compr. Physiol. 3, 221–242. doi:10.1002/cphy.c120001
Keywords: calcium signaling, hypotonic shock response, mathematical model, Saccharomyces cerevisiae, calmodulin-calcineurin pathway
Citation: Spolaor S, Rovetta M, Nobile MS, Cazzaniga P, Tisi R and Besozzi D (2022) Modeling Calcium Signaling in S. cerevisiae Highlights the Role and Regulation of the Calmodulin-Calcineurin Pathway in Response to Hypotonic Shock. Front. Mol. Biosci. 9:856030. doi: 10.3389/fmolb.2022.856030
Received: 16 January 2022; Accepted: 04 April 2022;
Published: 18 May 2022.
Edited by:
Alberto Jesus Martin, Universidad Mayor, ChileReviewed by:
Laszlo Csernoch, University of Debrecen, HungaryShivendra Gajraj Tewari, Biotechnology HPC Software Applications Institute (BHSAI), United States
Copyright © 2022 Spolaor, Rovetta, Nobile, Cazzaniga, Tisi and Besozzi. This is an open-access article distributed under the terms of the Creative Commons Attribution License (CC BY). The use, distribution or reproduction in other forums is permitted, provided the original author(s) and the copyright owner(s) are credited and that the original publication in this journal is cited, in accordance with accepted academic practice. No use, distribution or reproduction is permitted which does not comply with these terms.
*Correspondence: Renata Tisi, cmVuYXRhLnRpc2lAdW5pbWliLml0; Daniela Besozzi, ZGFuaWVsYS5iZXNvenppQHVuaW1pYi5pdA==