- 1Research Institute for Medicines (iMed.ULisboa), Faculty of Pharmacy, Universidade de Lisboa, Lisboa, Portugal
- 2Department of Pharmaceutical Sciences and Medicines, Faculty of Pharmacy, Universidade de Lisboa, Lisboa, Portugal
- 3Department of Chemistry, Technical University of Munich, Munich, Germany
Aquaglyceroporins, a sub-class of aquaporins that facilitate the diffusion of water, glycerol and other small uncharged solutes across cell membranes, have been recognized for their important role in human physiology and their involvement in multiple disorders, mostly related to disturbed energy homeostasis. Aquaglyceroporins dysfunction in a variety of pathological conditions highlighted their targeting as novel therapeutic strategies, boosting the search for potent and selective modulators with pharmacological properties. The identification of selective inhibitors with potential clinical applications has been challenging, relying on accurate assays to measure membrane glycerol permeability and validate effective functional blockers. Additionally, biologicals such as hormones and natural compounds have been revealed as alternative strategies to modulate aquaglyceroporins via their gene and protein expression. This review summarizes the current knowledge of aquaglyceroporins’ involvement in several pathologies and the experimental approaches used to evaluate glycerol permeability and aquaglyceroporin modulation. In addition, we provide an update on aquaglyceroporins modulators reported to impact disease, unveiling aquaglyceroporin pharmacological targeting as a promising approach for innovative therapeutics.
Introduction
Aquaporins (AQPs) are transmembrane protein channels found in all life forms, including archaea, eubacteria, fungi, and plants (Zardoya, 2005). AQPs facilitate the bidirectional transport of water and small polar solutes (such as glycerol) across cell membranes, triggered by osmotic or solute gradients (Verkman, 2012). In humans, the 13 AQPs (AQP0-12) identified so far are widely distributed in the body and found in many tissues and cell types. According to their pore selectivity, AQPs are grouped in 1) orthodox aquaporins (AQP0, AQP1, AQP2, AQP4, AQP5, AQP6 and AQP8) that are considered primary selective to water, 2) aquaglyceroporins (AQP3, AQP7, AQP9 and AQP10) that in addition to water, also transport glycerol and other small neutral solutes; and 3) subcellular or unorthodox aquaporins (AQP11 and AQP12) with distinct evolutionary pathway, localizing in intracellular membranes (Ishibashi et al., 2014) and with uncertain permeability (Ishibashi et al., 2021), although AQP11 was shown as a water and glycerol channel (Madeira et al., 2014a). A few members can also transport ammonia (ammoniaporins) (Jahn et al., 2004), hydrogen peroxide (peroxiporins) (Rodrigues et al., 2016; Prata et al., 2019; Rodrigues et al., 2019; Bestetti et al., 2020), and gases such as CO2, O2 (Geyer et al., 2013) or NO (Herrera et al., 2006), among other solutes (Wu and Beitz, 2007). Aquaporin research has gained much interest in recent years due to their physiological importance and implication in a broad range of disorders (Soveral et al., 2016), prompting the discover of AQP-modulators for potential therapeutic applications (Soveral and Casini, 2017). In particular, the aquaglyceroporin sub-family has attracted the attention of the research community due to their important roles in health and disease, being revealed as crucial players in a number of metabolic and inflammatory disorders (Madeira et al., 2015; da Silva and Soveral, 2017; da Silva et al., 2018a; Calamita et al., 2018; da Silva et al., 2018b; Mendez-Gimenez et al., 2018; da Silva et al., 2020a), including infectious responses (Tesse et al., 2021), inflammasome activation (da Silva et al., 2020b) and migration and survival of immune cells (da Silva and Soveral, 2021).
This review summarizes the current knowledge of aquaglyceroporins involvement in pathophysiological conditions and describe the experimental approaches to evaluate glycerol permeability and aquaglyceroporin modulation. In addition, we provide an update on aquaglyceroporins modulators reported to impact in disease, revealing aquaglyceroporins’ potential as drug targets for new therapies against pathologies with dysregulated aquaglyceroporin expression.
Pathophysiological Roles of Mammalian Aquaglyceroporins
The aquaglyceroporins (AQP3, AQP7, AQP9, and AQP10) are involved in a series of malfunctions and diseases, most of them related to their ability to facilitate glycerol diffusion across cell membranes, a feature that is determined by a larger constriction region of their size selectivity filter compared to orthodox aquaporins (pore diameter of about 2.8 Å and 3.5 Å in human orthodox and aquaglyceroporins, respectively) (Hub and de Groot, 2008).
Glycerol is an important molecule in energy metabolism for its crucial involvement in lipogenesis in feeding conditions and lipolysis and gluconeogenesis during fasting, contributing for energy homeostasis (Rodriguez et al., 2015a). Due to their ability to transport glycerol bridging tissues from different organs, aquaglyceroporins are key players in many physiological conditions and their dysfunction is related to disease (Soveral et al., 2016), as depicted in Figure 1. Increasing evidence show their involvement in a broad spectrum of pathophysiological conditions, such as skin dehydration, obesity, fatty liver, diabetes, intestinal disorders, cardiovascular disease and cancer, among others, highlighting the great pharmacological potential of these transmembrane channels (Soveral et al., 2016).
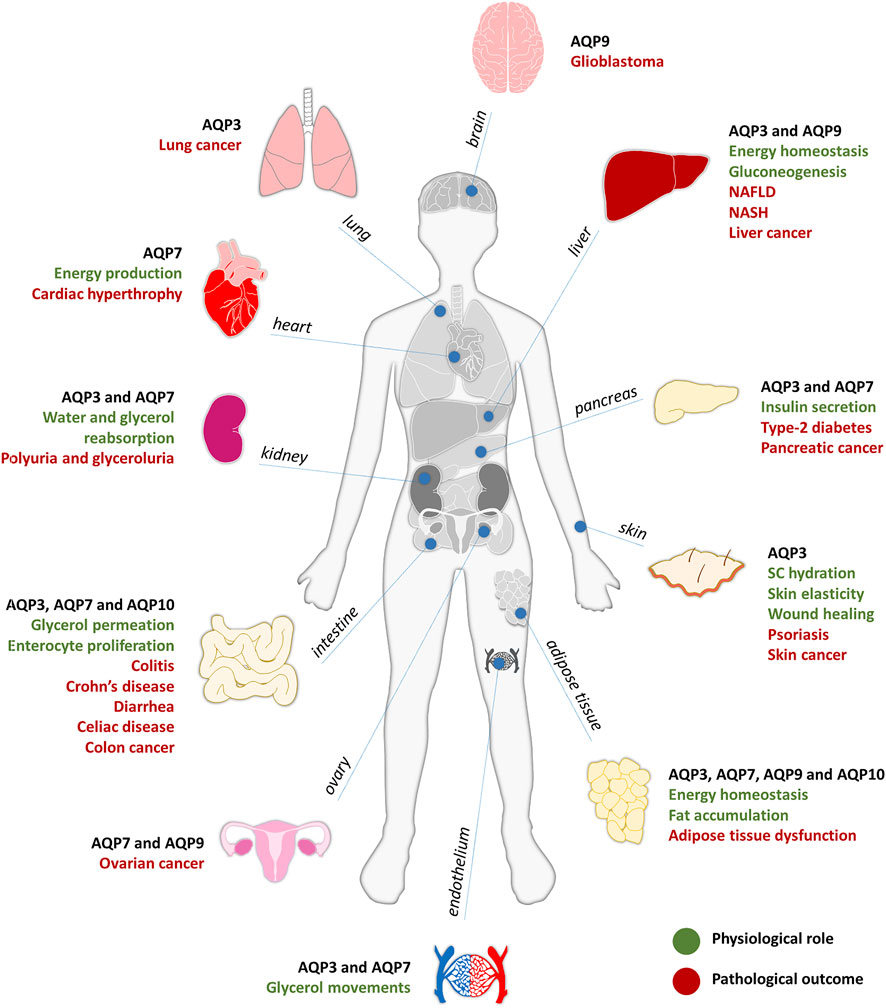
FIGURE 1. Aquaglyceroporins involvement in physiology (in green) and pathology (in red), described for each organ of the human body.
Skin represents the first line of defense of the human body covering the entire external body surface, where aquaglyceroporins are essential for skin physiology (da Silva et al., 2021). AQP3 is found in the basal layer of epidermal keratinocytes where it plays an important role in epidermal and stratum corneum (SC) hydration, since glycerol acts as a humectant that preserves the skin moisture (Verkman, 2009). AQP3-deficient mice show reduced SC hydration, accompanied with impaired skin elasticity and delayed wound healing (Hara-Chikuma and Verkman, 2006; Hara-Chikuma and Verkman, 2008a). This outcome results from the reduction of glycerol permeation, followed by decreased SC and epidermis glycerol content (Hara et al., 2002; Verkman, 2009). Glycerol administration can correct these skin defects, overcoming the absence of this aquaglyceroporin in epidermal keratinocytes (Hara and Verkman, 2003; Hara-Chikuma and Verkman, 2008b; Verkman, 2009). Moreover, when AQP3 is overexpressed, epidermal proliferation is enhanced due to the higher AQP3-mediated glycerol permeability and can result in hyperproliferative skin disorders such as psoriasis and skin tumors (da Silva et al., 2021; Hara-Chikuma and Verkman, 2008a; Verkman et al., 2014).
Adipose tissue and liver are the main organs involved in body energy homeostasis. AQP7 is expressed in both adipocyte and capillary plasma membranes of human adipose tissue (Skowronski et al., 2007; Laforenza et al., 2013) while AQP9 is localized in the plasma membrane of adipocytes and hepatocytes, being both key regulators of fat accumulation and glucose homeostasis (Maeda et al., 2009; Madeira et al., 2015). For this reason, they have been associated with metabolic disorders such as obesity and metabolic syndrome (da Silva and Soveral, 2017; da Silva et al., 2018b). Although other aquaglyceroporins have also been detected in human adipocytes (Rodriguez et al., 2011; Laforenza et al., 2013; Madeira et al., 2014a), AQP7 is the main glycerol channel responsible for the efflux and uptake of glycerol from adipocytes (Marrades et al., 2006; Maeda, 2012). During fasting or exercise, AQP7 gene expression is increased, whereas in the feeding state, AQP7 is downregulated, with expression inversely related with plasma insulin levels (Kishida et al., 2000). AQP7 knockout mice reveal adipocyte hypertrophy and increased adipocyte mass due to the reduced glycerol permeability of the plasma membrane and increased glycerol kinase activity, resulting in intracellular triglycerides accumulation (Hara-Chikuma et al., 2005; Hibuse et al., 2005; Rodriguez et al., 2006). Adipocyte lipid storage above normal levels and associated lipotoxicity can lead to inflammation, insulin resistance and obesity (da Silva and Soveral, 2017; Marrades et al., 2006), a cluster of disorders representing the metabolic syndrome (da Silva et al., 2018b). Furthermore, in a model of obese rats with type 2 diabetes (Kishida et al., 2000; Lee et al., 2005), AQP7 mRNA expression in adipose tissue was upregulated, possibly contributing to an augmented glycerol input for hepatic gluconeogenesis and subsequent hyperglycemia (Rojek et al., 2008). Interestingly, AQP7 is regulated in response to physical training in a gender-dependent manner, with women adipose tissue showing higher abundance when compared to men (Lebeck et al., 2012). AQP10 was also found expressed in human adipocytes and was proposed to represent an alternative pathway to AQP7 for glycerol efflux from adipose tissue (Laforenza et al., 2013), stimulated by the decreased pH observed during lipolysis (Gotfryd et al., 2018), assuring the maintenance of normal or low glycerol contents inside the adipocyte and protecting humans from obesity.
In the endocrine pancreas, AQP7 is the main aquaglyceroporin and is responsible for glycerol uptake into β-cells (Delporte et al., 2009). AQP7 knockout mice showed increased β-cell glycerol and triglycerides content and augmented glycerol kinase activity, associated with increased pancreatic insulin mRNA levels and insulin secretion at an elevated rate (Matsumura et al., 2007). These findings suggest that AQP7 is a key player in the regulation of insulin biosynthesis and secretion, indirectly controlling intracellular glycerol content and triglycerides synthesis (Rodriguez et al., 2011; Mendez-Gimenez et al., 2018). More recently, AQP7 was found to modulate inflammatory phenotype of endocrine pancreatic beta-cells, and to play a role in β-cells proliferation, adhesion, and migration (da Silva et al., 2020a).
In the liver, AQP9 was identified as the major route for glycerol uptake where it can be used in gluconeogenesis (Rojek et al., 2007; Jelen et al., 2011). AQP9-null obese mice with type 2 diabetes showed a significant increase in plasma glycerol levels accompanied by low fasting glucose levels. These findings suggest an impairment in glucose production due to AQP9 deficiency, limiting hepatic glycerol uptake for gluconeogenesis and highlighting AQP9 as a crucial player in both glycerol and glucose metabolism (Rojek et al., 2007; Maeda et al., 2009; Jelen et al., 2011; Rodriguez et al., 2011). Additionally, in a model of non-alcoholic fatty liver disease (NAFLD), AQP9 overexpression resulted in increased intracellular triglycerides, free fatty acids and glycerol levels, leading to the aggravation of steatosis, and this could be reversed with AQP9 suppression (Wang et al., 2013). Recently, hepatic AQP9 was found downregulated in obese patients with NAFLD and steatohepatitis (NASH), with even lower levels in patients with insulin-resistance, suggesting a compensatory mechanism to prevent glycerol availability for triglycerides accumulation and hepatic gluconeogenesis (Rodriguez et al., 2014). Interestingly, although hepatic AQP9 protein level was similar in obese male and female patients, the lower glycerol permeability detected in obese women hepatocytes might suggest a decreased risk of developing insulin resistance and NAFLD (Rodriguez et al., 2014; Rodriguez et al., 2015b).
In the heart, AQP7 has an important role in energy production (Hibuse et al., 2005). AQP7 knockout mice exhibited lower cardiac glycerol and ATP contents, with development of cardiac hypertrophy and higher mortality when cardiac muscle is in conditions of pressure overload (Hibuse et al., 2009). It has been recently proposed that AQP7-mediated glycerol metabolism plays a key role preventing myocardial ischemia-related damage (Ishihama et al., 2021).
In endothelium, AQP3 was identified as the predominant aquaglyceroporin, being responsible for endothelial glycerol permeation (da Silva et al., 2018a). AQP7 was shown to be localized in mice endothelium of both white and brown adipose tissue, heart, and skeletal muscle. In addition, AQP7 expression is upregulated in streptozocin-induced diabetes mellitus, indicating that insulin can regulate adipose tissue function through control of glycerol influx and outflow through the vascular endothelium (Skowronski et al., 2007). AQP7 expression in the endothelium of adipose tissue capillaries and in adipocytes plasma membrane was also confirmed in humans while AQP10 was reported to be localized in the adipocytes and not in the microvessels (Laforenza et al., 2013). Moreover, in the human small intestine, an AQP10 variant was identified in the capillary endothelial cells in small intestine villi (Li et al., 2005).
In intestinal inflammatory disorders, AQP3 is expressed in the basolateral membrane of colonic epithelial cells and was associated with enterocyte proliferation in a AQP3 null mice model of colitis, that showed epithelial cell damage and loss, colonic hemorrhage, impaired enterocyte proliferation and reduced survival compared to wild-type mice (Laforenza, 2012). With oral glycerol administration, these symptoms were reversed by significantly improving survival and reducing the severity of colitis, revealing the important role of AQP3 in enterocyte proliferation due to its glycerol-transporting function. Thus, AQP3 modulation was suggested with great potential for disorders associated with abnormal enterocyte proliferation, such as Crohn’s disease (Thiagarajah et al., 2007). Additionally, in 2,4,6-trinitrobenzene sulfonic acid (TNBS)-induced rat colitis model, that resembles human Crohn’s Disease, AQP3 and AQP8 mRNA and protein expression were found downregulated, with aggravated intestine inflammation and injury (Zhao et al., 2014). In addition, AQP3, AQP7, AQP10 and AQP11 expression was found strongly decreased in patients with celiac disease, that was recovered by dietary gluten withdrawal (Laforenza et al., 2010). Furthermore, AQP3 and AQP2 were found upregulated in a magnesium sulphate-induced diarrhea mice model which could be reverted with tannin extract treatment that has antidiarrheal properties. Tannin extract downregulates AQP2 and AQP3 expression through the suppression of PKA/pCREB signaling pathway, leading to decreased fecal water content in the colon (Liu et al., 2014).
The kidney is critical for the maintenance of body water homeostasis and the involvement of AQPs in acute and chronic kidney diseases is well recognized (Su et al., 2020). Although AQP2 and AQP1 have a prominent role in urine concentration, AQP3 expression at the basolateral membrane of collecting duct principal cells is increased by thirst and by vasopressin or aldosterone secretion, representing a potential water exit pathway from these cells (Noda et al., 2010). Mice lacking AQP3 show decreased urine concentration ability and polyuria (Ma et al., 2000; Verkman, 2008a; Verkman et al., 2014). AQP7 is localized in the proximal tubule apical membrane, where it facilitates glycerol and water transport (Rojek et al., 2008; Noda et al., 2010). Studies with AQP7 knockout mice showed glyceroluria, suggesting a role of AQP7 in glycerol reabsorption in the proximal straight tubule that cannot be compensated by other aquaglyceroporins (Sohara et al., 2005; Noda et al., 2010).
Increasing evidence show the implication of aquaglyceroporins in cancer and metastasis, where their expression level is in general positively correlated with tumor stage and aggressiveness (Papadopoulos and Saadoun, 2015). AQP3 is the aquaglyceroporin most implicated in tumorigenesis, being aberrantly overexpressed in cancers of different origin, such as skin cancer (Hara-Chikuma and Verkman, 2008c), colon (Zhang et al., 2020), lung (Liu Y. L. et al., 2007), liver (Chen et al., 2018) and pancreatic cancer (Direito et al., 2017). AQP3-facilitated glycerol transport generates ATP and mediates the growth and survival of tumor cells (Verkman et al., 2014) and, additionally, AQP3 ability to permeate H2O2 may promote tissue inflammation by increasing reactive oxygen species in macrophages (Hara-Chikuma et al., 2020) and contribute to the setting of inflammatory response (da Silva et al., 2020b; da Silva and Soveral, 2021). Targeting AQP3 expression reduces several intracellular signaling pathways, leading to reduced cell proliferation, migration, and invasion (De Ieso and Yool, 2018), suggesting that AQP3 modulators could help in the prevention and therapy of several tumors with abnormal expression of this aquaglyceroporin (Aikman et al., 2018). AQP7 and AQP9 are also highly expressed in malignant ovarian tumor cells comparing to benign tumor or normal tissue, suggesting their role in ovarian carcinogenesis (Yang et al., 2011). Furthermore, AQP9 was also found upregulated in human glioblastoma probably due to its contribution for energy metabolism and its ability of lactate and glycerol clearance from the extracellular space to counteract the lactate acidosis that is associated with this specific type of cancer (Warth et al., 2007; Tan et al., 2008). In hepatocellular carcinoma (HCC), AQP3 was upregulated, and AQP7 and AQP9 were downregulated (Chen et al., 2016), being significantly associated with the aggressive features of HCC. In addition, AQP9 downregulation was also correlated with apoptosis resistance (Jablonski et al., 2007), invasion and epithelial-to-mesenchymal transition (EMT) (Zhang et al., 2016).
Overall, due to their involvement in a broad range of pathophysiological conditions, aquaglyceroporins revealed as promising targets for drug discovery, boosting the search for potent and selective modulators with pharmacological properties.
Aquaglyceroporin Functional Assays
The identification and validation of aquaglyceroporin modulators (inhibitors) relies on in vitro and in silico assays to measure glycerol permeability in membranes, providing structure-activity relationships able to validate drug hits (Madeira et al., 2016). The methods currently used to evaluate glycerol permeability and screen potential aquaglyceroporin modulators are summarized in Figure 2.
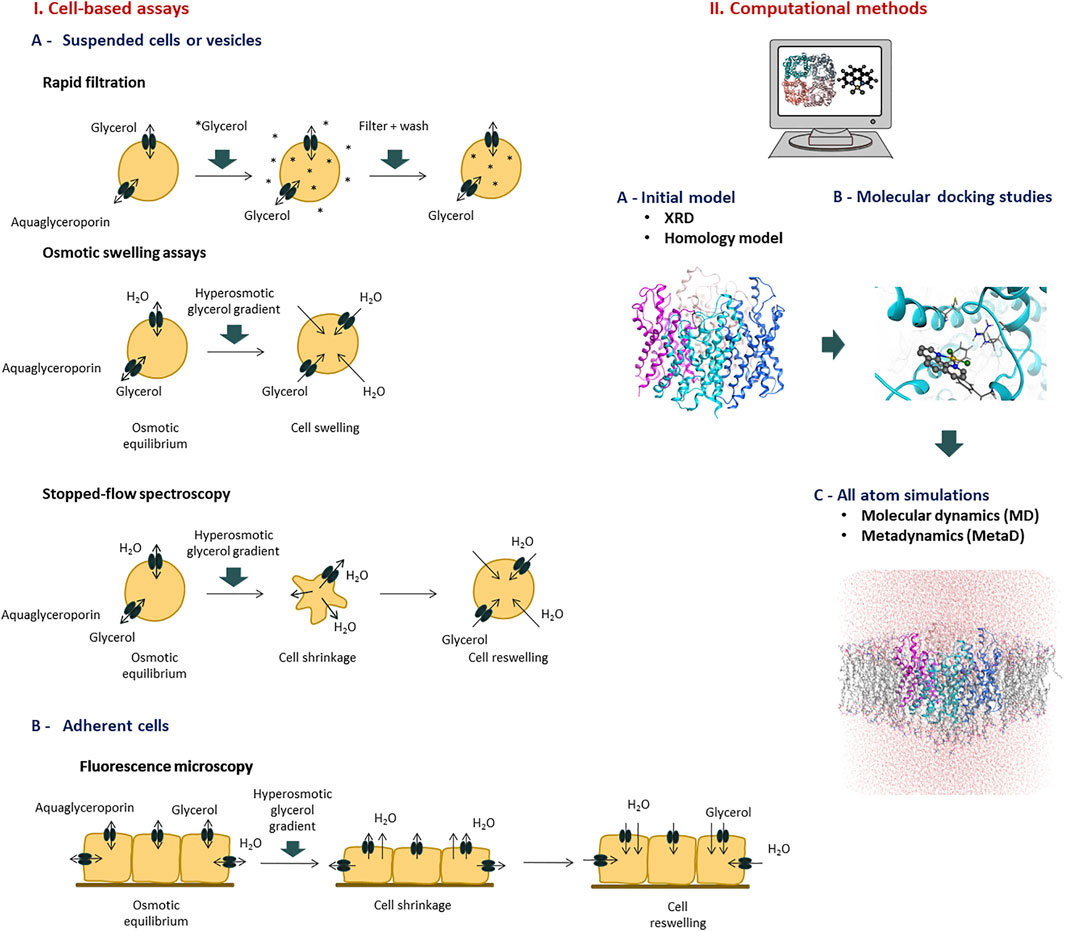
FIGURE 2. Methods to evaluate aquaglyceroporins function and screen potential modulators. Representative assays of I) Cell based assays, and II) Computational methods.
Cell-Based Assays
Aquaglyceroporin activity has been detected in procaryotic and eucaryotic cells and isolated membranes from a wide range of organisms, such as bacteria (Froger et al., 2001), yeast (Soveral et al., 2007; Soveral et al., 2008; Mosca et al., 2018), and mammalian cells (da Silva et al., 2018a; da Silva et al., 2020a; Madeira et al., 2013), using whole cells or isolated intracellular vesicles (Noronha et al., 2014). In addition, the functional characterization of procaryotic and eukaryotic aquaporins has been achieved by heterologous expression in oocytes of the frog Xenopus laevis (Chauvigne et al., 2021) or in the yeast strain Saccharomyces cerevisiae devoid of endogenous aquaporins, where their glycerol transporting ability as well as their mechanisms of gating were uncovered (Bjorkskov et al., 2017; Gotfryd et al., 2018; Mosca et al., 2018; Sabir et al., 2020). The appropriate matching of cell model and functional assay is crucial to obtain accurate information on aquaglyceroporin physiological relevance, identify potential modulators and evaluate their impact in the selected pathophysiological conditions.
Rapid Filtration Technique
The rapid filtration technique has generally been used to measure the uptake of solute into cells and isolated membrane vesicles. Uptake of glycerol across the cell membrane is facilitated by aquaglyceroporins, thus measuring the accumulation rate of radiolabeled (usually 14C or 3H) glycerol provides an experimental approach to determine aquaglyceroporin activity in a variety of cell types and vesicles. Cells are rapidly mixed with an incubation medium containing radioactively labeled permeant solute. After appropriate time intervals, the uptake is blocked by adding stop solution and cells are dropped on a filter that is subsequently washed and counted for radioactivity. Efflux from vesicles pre-loaded with labelled substrates can also be measured using similar procedures. Although these assays are simple and rapid and can provide mechanistic information on transport kinetics (Liu Y. et al., 2007; Ishii et al., 2011; Katano et al., 2014), some limitation include the high costs of radiolabeled glycerol and its disposal and the current safety policies that restrict radioactivity handling and may limit their usage.
Osmotic Swelling Assays
The osmotic lysis assay has been largely used for evaluation of aquaglyceroporins modulation in erythrocytes (Campos et al., 2011; Martins et al., 2012). Human erythrocytes express AQP3, and when challenged with hyperosmotic glycerol solutions, cells swell and eventually hemolyze due to glycerol and water influx. The rate of swelling and hemolysis can be monitored spectrophotometrically and used to calculate the glycerol permeability (Liu Y. et al., 2007; Campos et al., 2011; Madeira et al., 2016).
Xenopus laevis oocytes have a low intrinsic water permeability and were the first cells used to show AQP1 inhibition by mercury chloride (Preston et al., 1992). Due to their lack of endogenous AQP expression, Xenopus oocytes have been considered a good model to study aquaglyceroporin activity and regulation (Beitz et al., 2009; Madeira et al., 2016). Oocytes are microinjected with mRNA encoding aquaglyceroporins and then subjected to an inwardly directed solute gradient, leading to solute and water influx and swelling. The oocyte swelling kinetics is followed by video microscopy where the increase in cell volume is quantified (Beitz et al., 2009). Xenopus oocytes swelling assays have revealed limitations due to cell geometry and extracellular unstirred layers artifacts, that may explain the significant variability reported in inhibition studies (Liu et al., 2002; Verkman et al., 2014; Tradtrantip et al., 2017).
Fluorescence Microscopy Assays
Fluorescence microscopy can be used to evaluate the permeability of living cells. AQP-expressing cells grown as a monolayer culture are loaded with a membrane-permeant non-fluorescent precursor (e.g., calcein acetoxymethyl ester) which is cleaved inside the cell by esterases, resulting in an impermeable fluorescent form that is trapped intracellularly (Madeira et al., 2014b). Volume changes induced by solute gradients are detected by the change in fluorescence intensity under a fluorescence microscope (Madeira et al., 2013; Madeira et al., 2014b) or alternatively, using a plate-reader that detects the fluorescence of the whole cell layer (Mola et al., 2009; Fenton et al., 2010; Sonntag et al., 2019). While the fluorescence microscope setup allows single cell imaging and analyzing permeability in a single cell mode, the plate reader has the advantage of a high throughput system. However, possible artifacts due to dye leakage or binding to cell membrane represent a significant limitation of this technique (Zelenina and Brismar, 2000; Tradtrantip et al., 2017).
Another fluorescence microscopy method, that does not require dye loading, uses a genetically encoded yellow fluorescent protein (YFP-H148Q-V163S), whose fluorescence is quenched by chloride. The cytoplasmic chloride concentration changes as the cell volume increases or decreases, resulting in altered YFP fluorescence (Galietta et al., 2001; Baumgart et al., 2012; Esteva-Font et al., 2013). The chloride sensor YFP is not affected by leakage artifacts but can be influenced by intracellular pH and anion concentrations, besides is limited time resolution (Galietta et al., 2001; Tradtrantip et al., 2017).
Stopped-Flow Spectroscopy
Stopped-flow spectroscopy allows to follow the cell volume changes after a rapidly imposed osmotic or solute gradient. It has been used to quantify water and solute permeabilities in suspended cells, vesicles or liposomes (Madeira et al., 2016; Tradtrantip et al., 2017). To assess aquaglyceroporin function, a cell suspension in isosmotic buffer is rapidly mixed with an equal volume of a hyperosmotic glycerol solution creating an inwardly directed glycerol gradient. The first cell shrinkage due to water efflux caused by the osmotic gradient is followed by water and glycerol influx and subsequent cell reswelling. These cell volume changes can be followed by optical properties of the solution, either light scattering (which is dependent of the cell volume), or fluorescence emission (if cells are loaded with a volume sensitive fluorophore) (Soveral et al., 2007; Madeira et al., 2016). Considering the linear correlation between cell volume and the optical properties of the system, the time course of cell volume changes until osmotic equilibrium is reached allows the quantification of glycerol permeability (Martins et al., 2012; Madeira et al., 2016). Stopped-flow light scattering is susceptible to various artifacts because the light intensity from cells depends not only on cell volume but also on cell shape and refractive index of intracellular and extracellular media. Although fluorescence measurements are relatively insensitive to cell shape, they show similar limitations to the above described for microscopy assays (Tradtrantip et al., 2017; Abir-Awan et al., 2019). Finally, the membrane optical properties can also be influenced by compounds’ treatment, such as AQP modulators.
Analysis of Glycerol Permeability and Activation Energy
The evaluation of glycerol permeability (Pgly) is based on the rate of glycerol movement through membranes caused by a glycerol chemical gradient. When using cell volume measurements (swelling assays, stopped-flow or microscopy), Pgly can be calculated by fitting a single exponential equation to the signals obtained after imposing inwardly glycerol gradients, using the relation Pgly = k (V0/A) (cm s−1) where k is the single exponential rate constant and V0/A is the initial cell volume to area ratio (Campos et al., 2011; Martins et al., 2012). Alternatively, mathematical models using non-linear equations and numerical integrations can be applied for an accurate determination of Pgly (Madeira et al., 2013).
The activation energy (Ea) of glycerol transport is a measure of the energy barrier needed for glycerol diffusion across the plasma membrane. The Ea value is much lower when glycerol permeation occurs via aquaglyceroporins than via simple diffusion through lipid bilayer, making this parameter extremely valuable to ascertain aquaglyceroporin activity and the proof of concept for the identification of new inhibitors. Ea is assessed by performing the permeability assay along a range of temperatures. A weak dependence on the assay temperature indicates that glycerol diffusion via the hydrophilic protein pore dominates over the lipid pathway. Ea (kcal mol−1) can be calculated by the slope of an Arrhenius plot (ln Pgly as a function of 1/T) (Madeira et al., 2016).
Computational Methods
The determination of high-resolution atomic structures of various AQP isoforms was the starting point for the use of computational techniques to investigate AQPs as novel targets in drug discovery. In silico approaches have contributed to the understanding of AQPs structure and function, their selectivity for water or other substrates, as well as elucidating structure-activity relationships (Roux and Schulten, 2004; Verkman et al., 2014; Madeira et al., 2016). Until now, the mammalian aquaporins with available high-resolution structures are AQP0 (Harries et al., 2004), AQP1 (Murata et al., 2000), AQP2 (Frick et al., 2014), AQP4 (Ho et al., 2009), AQP5 (Horsefield et al., 2008), AQP7 (de Mare et al., 2020) and AQP10 (Gotfryd et al., 2018). Molecular dynamics (MD) simulations have contributed to explain AQPs function since this technique can follow the rapid water and solute transport through AQPs at an atomic resolution and predict the binding sites of ions and molecules (Roux and Schulten, 2004; Hub et al., 2009). Regarding aquaglyceroporins, the structures of AQP3 and AQP9 have not been solved yet. Therefore, in these cases, three-dimensional homology models were assembled to construct their structures and overcome this limitation allowing to proceed with both classical MD simulations and protein-ligand docking studies (Martins et al., 2012; Wacker et al., 2013). The same homology modeling approach was applied to obtain AQP7 structure before its X-ray structure was solved (Madeira et al., 2014b).
Protein-ligand docking studies have been used as a virtual screening method of potential AQP modulators, being able to predict their binding modes to the protein (Martins et al., 2012; Madeira et al., 2014b; Yadav et al., 2020). Additionally, molecular docking can be combined with molecular dynamics to confirm the proposed inhibitor binding sites, that can also be characterized through density functional theory calculations (Muller et al., 2008; Martins et al., 2012; Verkman et al., 2014; de Almeida et al., 2017).
AQPs are assembled as tetramers in the cell membrane (Figure 3). An AQP monomer features an hourglass shaped pore formed by six transmembrane helices, connected by five loops (A through E) and two semi-helices meeting at the center of the channel (Figure 3). Close to the extracellular side, the aromatic/arginine selectivity filter (ar/R SF), the narrowest point of the pore, is responsible for size-exclusion of molecules. Underneath, the pore center is defined by two highly conserved asparagine-proline-alanine (NPA) motifs, contained in the B and E loops and semi-helices. The NPA region has been postulated to be responsible for exclusion of charged solutes through the formation of an electrostatic barrier (Chakrabarti et al., 2004). Specifically, the electrostatic field in this region governs not only the structure and fluctuations of the unprotonated water chain, but also the free energy barrier for an excess proton (Chakrabarti et al., 2004).
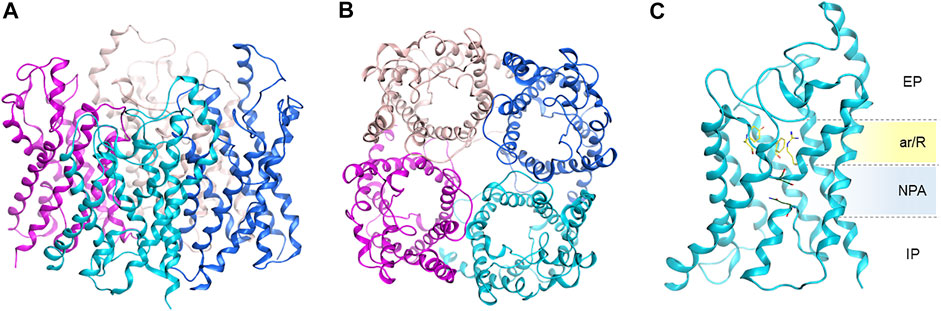
FIGURE 3. Homology model of human AQP3 tetramer. (A) Side, and (B) top view; (C) AQP3 monomer side view showing position of the ar/R selectivity filter and NPA motifs. EP, extracellular pocket, IP, intracellular pocket. Figure generated using the MOE software (Chemical Computing Group ULC, 2021).
MD studies have helped elucidating the role of the selectivity filters and their effects on the permeation of water and other small uncharged solutes (de Groot and Grubmuller, 2001; Janosi and Ceccarelli, 2013). However, while MD calculations can provide free-energies of substrate permeation via AQPs by collecting the relevant equilibrium configuration probability distributions, they cannot provide kinetic information of solute conductance. The latter is required for an unbiased mechanistic analysis over a physiologically significant timeframe. In this context, Metadynamics (MetaD) atomistic simulations were used to reconstruct the free-energy profile for substrate permeation from multiple independent runs (Figure 2). MetaD accelerates rare event occurrence along collective variables (CV) and it has recently been successfully applied to elucidate the mechanisms of glycerol and hydrogen peroxide permeation via human AQP3 (Wragg et al., 2019; Wragg et al., 2020) as well as of AQP10’s covalent inhibition by small molecules (Pimpao et al., 2021).
Aquaglyceroporin Modulators With Pharmacological Action
The discovery of AQPs inhibitors with potential clinical applications has been challenging due to the wide AQP distribution in tissues, the similarity between AQP-isoforms and the structural limitations displayed by the narrow channel pore that is difficult to target, if mere blockage of the substrate permeation by steric effects is envisaged (Verkman et al., 2014; Tradtrantip et al., 2017). However, the pharmacological modulation of aquaglyceroporins has been reported and correlated with potential therapeutic effects in diseases where these proteins are abnormally expressed. Here, we will describe the chemical and biological molecules reported to act as direct inhibitors of aquaglyceroporin function or as modulators of their expression (down or up-regulation), with examples represented in Figure 4. These two types of modulators are included and categorized accordingly in Table 1. In this review, only aquaglyceroporin modulators with reported beneficial effects or claimed to ameliorate the outcome of diseases were considered.
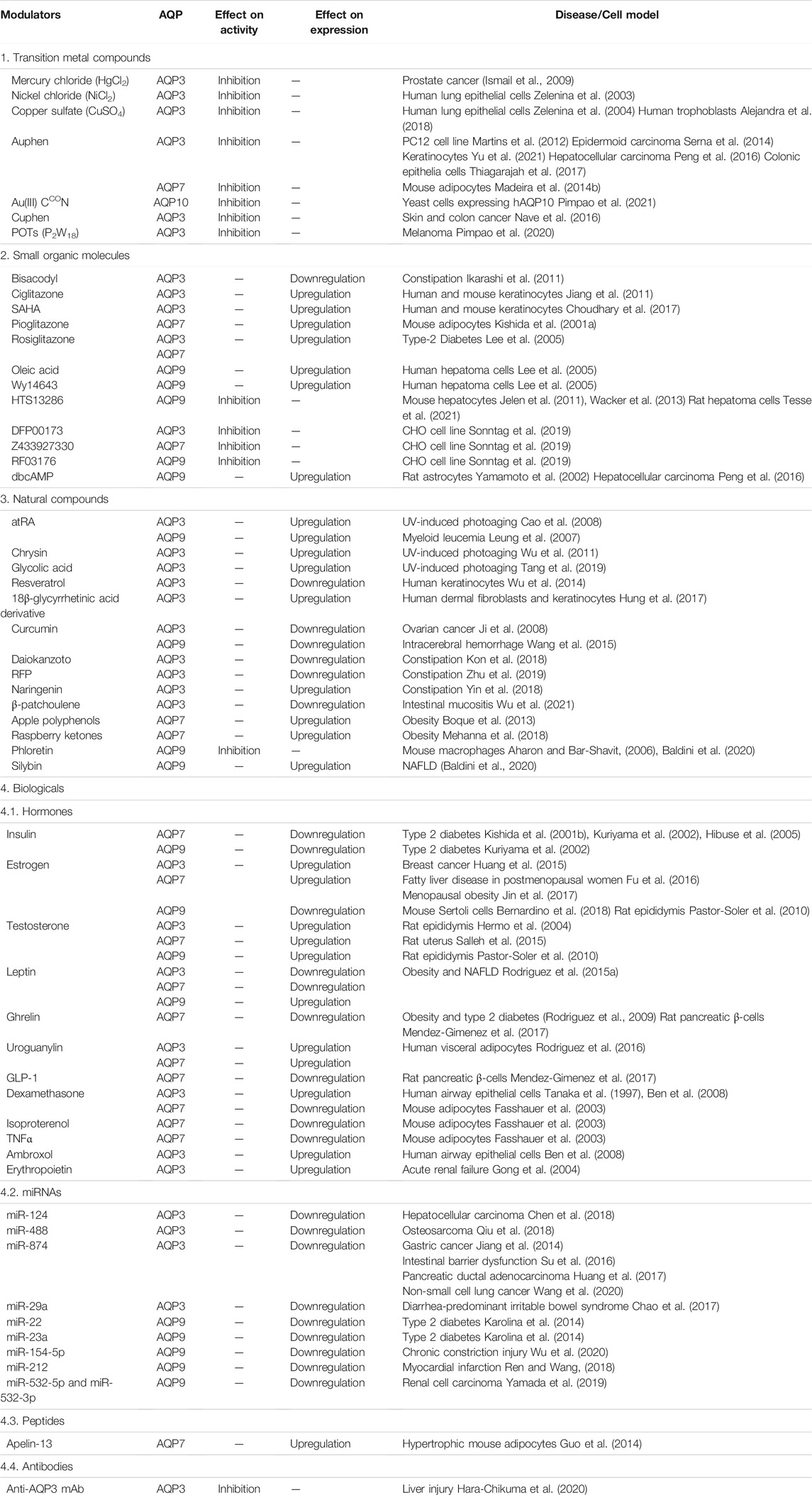
TABLE 1. Classes of compounds (transition metal compounds, small molecules, natural compounds and biologicals) modulators of aquaglyceroporin function or expression and related diseases where they may exert a beneficial pharmacological action.
Transition Metal Compounds
Mercury compounds such as mercury chloride (HgCl2) and p-chloromercuribenzene sulphonate (pCMBS) were the first metal compounds identified as AQP inhibitors and are still used in functional assays despite their associated toxicity and lack of selectivity (Macey and Farmer, 1970; Castle, 2005; Rubenwolf et al., 2012). The mechanism of inhibition has been shown to rely on direct binding of Hg2+ to thiol moieties in the AQP channel (Savage and Stroud, 2007; Spinello et al., 2016). Interestingly, mercury chloride was reported to improve the sensitivity of prostate cancer cells to cryotherapy, promoting a higher efficacy of this treatment by inhibiting AQP3 (Ismail et al., 2009). Nickel chloride and copper sulphate were found to inhibit AQP3 in human lung epithelial cells (Zelenina et al., 2003; Zelenina et al., 2004). Later, copper sulphate was used to assess AQP3 role on the extravillous throphoblast (EVT) cell migration and its importance for placentation (Alejandra et al., 2018). Although both nickel chloride and copper sulphate were identified as AQP3 inhibitors, no therapeutic effects have so far been reported for these transition metal compounds.
AQP3 has been associated with cell proliferation in cancer cells, namely in skin tumors where it is highly expressed (Verkman, 2008b). In recent years, our group has identified gold-based compounds with bidentate N-donor ligands as selective AQP3 inhibitors, with no effects towards orthodox aquaporins (de Almeida et al., 2014). In this series, a gold(III) complex [Au(phen)Cl2]Cl (Auphen, phen = 1,10-phenanthroline, Figure 5) (Martins et al., 2012) was found to decrease cell proliferation in AQP3-expressing cells, revealing its potential for the treatment of carcinomas with large AQP3 expression (Serna et al., 2014). The mechanism of inhibition is suggested to occur via gold binding to a specific cysteine residue in the AQP3 channel, via formation of direct Au-sulfur bonds (Martins et al., 2012; Serna et al., 2014). Further MD studies were conducted for another potent and selective Au(III) inhibitor—the compound [Au(BzMeI)Cl2]PF6 (AuBzMeI, BzMeI = 1-methyl-2-(pyridin-2-yl)-benzimidazole, Figure 5). The obtained results showed that protein conformational changes upon metal binding to Cys40 in AQP3 are responsible for the observed inhibition of glycerol permeation, and not direct steric blockage of the channel by the metal compound. Overall, in a number of complementary studies (Martins et al., 2013; de Almeida et al., 2017; Graziani et al., 2017), multi-level theoretical approaches have identified three key aspects for AQP3 inhibition by gold compounds: 1) speciation of the gold(III) complex prior protein binding (formation of aquo-complexes), 2) stabilization of non-covalent adducts between the compound organic ligands and the extracellular pore side, and 3) conformational changes induced within the pore by the coordinative binding of Au(III) ions leading to pore closure.
Auphen was also shown to inhibit AQP7 in adipocytes (Madeira et al., 2013; Madeira et al., 2014b) and was used to unveil a role of AQP3 in keratinocytes autophagy (Yu et al., 2021), to suppress growth of hepatocellular carcinoma by downregulating AQP3 (Peng et al., 2016), and to impair cell migration via AQP3 blockage in colonic epithelia (Thiagarajah et al., 2017). Additionally, AQP3 inhibition by Auphen in monocytic THP-1 cells was shown to reduce IL-1β release and pyroptosis by preventing NLRP3 inflammasome activation induced by reswelling, nigericin and ATP (da Silva et al., 2020b).
Recently, new organogold derivatives of general formula [Au(C^N)Cl2] (C^N = cyclometalated ligand), being endowed with increased stability in aqueous environment relative to the benchmark inhibitor Auphen (Casini et al., 2008; Bertrand and Casini, 2014), were shown to inhibit human AQP10 in a yeast model (Pimpao et al., 2021). Some of the tested gold complexes have been reported to inhibit cancer cells proliferation in vitro (Bertrand et al., 2015) and in vivo (Babak et al., 2021). Thus, the Au(III) C^N complex featuring a metformin ancillary ligand (AuMet, Figure 5) was 6000-fold more cytotoxic compared to uncoordinated metformin and significantly reduced tumor burden in mice with aggressive breast cancers. Noteworthy, the organogold compounds feature a peculiar reactivity with cysteine residues, whereby, following gold adduct formation, the reaction proceeds towards irreversible cysteine arylation, whereby coupling the C^N ligand (2-benzoylpyridine) to the side-chain thiol occurs via reductive elimination at the Au(III) centre. MetaD provided initial mechanistic insights on the effects of the inhibitors’ binding to AQP10, showing that cysteine arylation reduces glycerol conductance (Pimpao et al., 2021). In details, upon covalent binding of the C^N ligand to Cys209 in AQP10, the pathway of glycerol permeation was significantly altered due to an overall shrinkage of the pore, while water flux was only minimally affected (Figure 6).
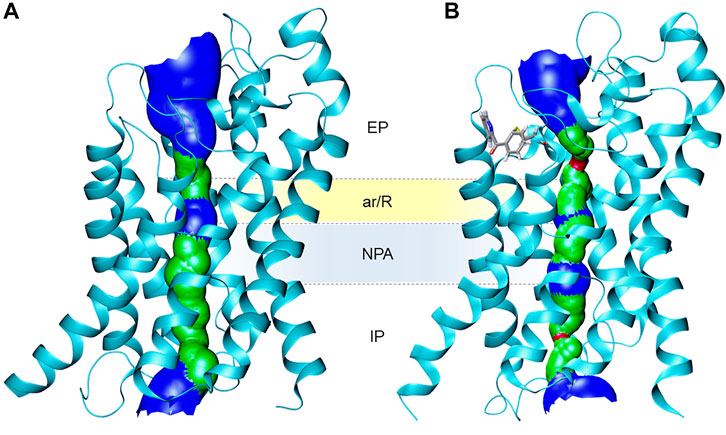
FIGURE 6. Ribbon representation of (A) the human AQP10 monomer and (B) the AQP10-C^N adduct with modified Cys209, showing the effects of pore size as a 3D representation (based on VDW radius: red = smaller than single H2O, green = single H2O, blue = larger than single H2O). Cys209-C^N fragment is shown in stick representation with atoms coloured by atom type (red = oxygen, blue = nitrogen, grey = carbon, yellow = sulfur). Figure generated using HOLE (Smart et al., 1996) and VMD software (Humphrey et al., 1996).
The copper(II) analogue of Auphen, [Cu(phen)Cl2] (Cuphen, Figure 5) was also observed to inhibit AQP3, although less potently than Auphen, and to reduce glycerol permeation in AQP3-expressing melanoma (Nave et al., 2016; Pinho et al., 2019) and colon cancer cells (Pinho et al., 2021), leading to anti-proliferative effects and cell migration impairment. Of note, when formulated in nanoliposomes targeting solid tumors, Cuphen-loaded nanoliposomes were able to reduce tumor growth in mice bearing melanoma (Pinho et al., 2019) and colon cancer (Pinho et al., 2021). Finally, the ability of polyoxotungstates (POTs), in particular P2W18, to inhibit AQP3 and impair melanoma cell migration was reported, suggesting that the well-known anticancer properties of these compounds may in part be due to the blockage of AQP3-mediated permeability (Pimpao et al., 2020). Altogether, these studies unveil the potential of metallodrugs as anticancer agents against tumors where AQP3 is highly expressed.
Small Organic Molecules
Small molecules with low molecular weight (<900 Da) have been reported to modulate aquaglyceroporins expression and function (Figure 4), exerting a beneficial influence on disease outcomes. For instance, bisacodyl, a laxative widely used to treat constipation, was reported to decrease AQP3 protein expression in rat colon through activation of macrophages and increased PGE2 secretion and was closely associated with an increase in fecal water content, suggesting that bisacodyl may exert a laxative effect due to AQP3 modulation (Ikarashi et al., 2011). Ciglitazone, a peroxisome proliferator-activated receptor gamma (PPARγ) activator, and Suberanilohydroxamic acid (SAHA), a histone deacetylase (HDAC) inhibitor, were reported to increase AQP3 mRNA and protein levels in human and mouse keratinocytes, leading to an increase in glycerol uptake. These studies indicate the potential of ciglitazone and SAHA as therapeutic agents in skin disorders (Jiang et al., 2011; Choudhary et al., 2017).
The promoters of the genes encoding AQP3, AQP7 and AQP9 exhibit putative peroxisome proliferator response elements (PPRE), suggesting that PPAR can influence AQP expression (Kishida et al., 2001a; Patsouris et al., 2004; Jiang et al., 2011). In fact, pioglitazone, a PPARγ agonist, activates PPARγ and leads to augmented AQP7 mRNA levels and promoter activity in mice adipocytes (Kishida et al., 2001a). Rosiglitazone was also shown to increase AQP7 mRNA levels in the mesenteric fat and induce glycerol kinase mRNA expression in both fat depots on OLETF rats, a type 2 diabetes model. These findings indicate that rosiglitazone can regulate AQP7 and glycerol kinase expression in adipose tissue, playing an important role in glycerol metabolic pathway. AQP9 and glycerol kinase mRNA levels were not affected with rosiglitazone administration to OLETF rats (Lee et al., 2005). However, AQP9 protein expression was upregulated after treatment with oleic acid and Wy14643 in cultured human hepatoma cells. These results suggest that fatty acids can promote glycerol availability in the liver through AQP9 upregulation. AQP3 mRNA levels were also found to be increased in the outer medulla of OLETF rat kidney after rosiglitazone treatment, which could be related to the fluid retention caused by this compound (Lee et al., 2005).
A series of small molecules were tested as aquaglyceroporin inhibitors highlighting HTS13286 as AQP9 inhibitor and with potential to reduce intracellular glycerol accumulation and impair gluconeogenesis in murine liver (Jelen et al., 2011; Wacker et al., 2013). A recent study reported the ability of HTS13286 to prevent LPS-induced systemic inflammation via AQP9 blockage in rat hepatoma cells (Tesse et al., 2021). However, due to its low water solubility, HTS13286 may not be appropriate for in vivo experiments, with structural analogues with higher potency and increased water solubility being suggested as promising molecules to control gluconeogenesis in type-2 diabetes (Jelen et al., 2011; Wacker et al., 2013). Recently, the compounds DFP00173 and Z433927330 were shown as potent AQP3 and AQP7 inhibitors, and RF03176 was able to significantly impair H2O2 permeability in AQP9-expressing CHO cells. These three compounds exert their inhibitory effect by binding to AQP cytoplasmic entrance (Sonntag et al., 2019).
Dibutyryl cyclic adenosine monophosphate (dbcAMP), a cell-permeable synthetic analogue of cAMP, was found to upregulate AQP9 mRNA and protein expression in rat astrocytes. The effects of dbcAMP on AQP9 mRNA levels were reverted by pre-treating astrocytes with protein kinase A (PKA) inhibitors, suggesting that PKA is involved in the regulation of AQP9 expression (Yamamoto et al., 2002). The increase on AQP9 mRNA and protein levels through dbcAMP modulation was also confirmed in a mice model of HCC, resulting in tumor growth suppression with decrease in tumor size (Peng et al., 2016).
Natural Compounds
Natural compounds are bioactive products derived from natural sources, usually plants, that are known for their beneficial effects in disease prevention and in reducing the risk of chronic diseases including cardiovascular diseases and cancer (Maraldi et al., 2014; Tesse et al., 2018). These bioactive compounds can exert therapeutic effects on AQPs by influencing their expression and/or function (Tesse et al., 2018; Portincasa and Calamita, 2019).
UV radiation causes human skin photoaging, characterized by dehydration and delayed wound healing, and was found to downregulate AQP3 protein expression in human keratinocytes. All-trans retinoic acid (atRA), chrysin and glycolic acid were found to revert the UV-induced AQP3 downregulation in human keratinocytes, revealing their protective role against UV-induced photoaging (Cao et al., 2008; Wu et al., 2011; Tang et al., 2019). Interestingly, both atRA and chrysin exert their effect through activation of ERK signaling pathway and involvement on redox signaling and oxidative stress (Cao et al., 2008; Wu et al., 2011). atRA can also induce AQP9 mRNA and protein expression resulting in increased As2O3 uptake in myeloid leukemia cells and, consequently, augmented arsenic sensitivity (Leung et al., 2007). In addition, resveratrol was shown to downregulate AQP3 mRNA and protein expression in a SIRT1/ARNT/ERK dependent manner in normal human epidermal keratinocytes, resulting in the impairment of cell proliferation, which was also observed in AQP3-knocked down cells (Wu et al., 2014). Glycyrrhizin is a bioactive compound of licorice that can be hydrolyzed to 18β-glycyrrhetinic acid which has immunomodulatory, anti-inflammatory, anti-carcinogenic and antioxidant properties. Interestingly, a 18β-glycyrrhetinic acid derivative was found to increase AQP3 mRNA and protein expression and enhance both proliferation and migration in human dermal fibroblasts and keratinocytes, revealing its potential usage in skin diseases with impaired wound healing and skin lesions (Hung et al., 2017).
Curcumin, a non-flavonoid polyphenol known for its anticancer properties, suppresses EGF-induced AQP3 protein expression upregulation and decreases cell migration in cultured human ovarian cancer cells, with inhibitory effect on EGF-induced EGFR, PI3K/AKT, and MEK/ERK activation. The same effect was observed with AQP3 knockdown or by treating cells with AQP3 inhibitors (NiCl2 and CuSO4) (Ji et al., 2008). This same compound was reported to attenuate brain edema and neurological deficits in mice with intracerebral hemorrhage (ICH), through the reduction of AQP9 mRNA and protein expression via reduced activation of NF-kB pathway, showing therapeutic potential as a treatment for this disease (Wang et al., 2015). Daiokanzoto administration in rats, a combination of sennoside A and glycyrrhizin, and treatment with a purified resin glycoside fraction from Pharbitis Semen (RFP) on human colonic epithelial cells suppresses AQP3 protein expression accompanied with an increase in fecal water content, indicating that both compounds can exert a laxative effect through AQP3 modulation and could be used for chronic constipation treatment (Kon et al., 2018; Zhu et al., 2019). Surprisingly, in mice colon with loperamide-induced constipation, the flavanone naringenin was found to upregulate AQP3 mRNA and protein expression, as opposed to the effect of daiokanzoto and RFP, but also causing an increase in fecal water content (Yin et al., 2018). Recently, β-patchoulene, the main active ingredient extracted from patchouli oil, was reported to decrease AQP3 abnormal protein expression in intestinal mucositis-induced rats through inactivation of cAMP/PKA/CREB signaling pathway, restoring water transport and attenuating diarrhea symptoms (Wu et al., 2021).
Raspberry ketones and apple polyphenols were reported to upregulate AQP7 mRNA expression in diet-induced obese rats (Boque et al., 2013; Fiorentini et al., 2015; Mehanna et al., 2018). Moreover, apple polyphenols could revert the AQP7 promoter hypomethylation caused by high-fat sucrose diet. The observed increase in glycerol release in ex vivo lipolysis experiments and the reduction in adipocyte size, could be explained by AQP7 upregulation for its crucial role in adipocyte glycerol permeability and control of triglyceride accumulation (Boque et al., 2013; Fiorentini et al., 2015). Phloretin, a chalcone abundant in apples, was identified as a non-specific AQP9 inhibitor that can reduce the size of bone-resorbing cells, the osteoclasts, with inhibition of osteoclast differentiation, a biological process mediated by AQP9 (Tsukaguchi et al., 1999; Aharon and Bar-Shavit, 2006). Recently, silybin, the major bioactive component of milk thistle, was found to upregulate AQP9 mRNA and protein expression in hepatic steatosis with increase in glycerol uptake, preventing triglycerides accumulation and liver dysfunction with decreased fat-induced autophagy. These novel findings suggest a therapeutic effect towards hepatic steatosis in NAFLD (Baldini et al., 2020).
Biologicals
Hormones, microRNAs (miRNAs) and peptides, called “biologicals,” are naturally occurring molecules with therapeutic properties which have also been described as modulators of aquaglyceroporins’ expression, as described below.
Hormones
Modulation of aquaglyceroporins’ expression by hormones has been well documented in the literature. The tight regulation of AQP7 expression in insulin-sensitive tissues and the modulation of AQPs by gonadal steroids in female and male reproductive systems, are examples of the important roles played by these protein channels in metabolism and reproductive health (Wawrzkiewicz-Jalowiecka et al., 2021). Insulin is known to repress AQP7 transcription in mice adipocytes (Kishida et al., 2001b), with AQP7 KO mice showing insulin resistance and triglycerides accumulation in adipose tissue (Hibuse et al., 2005). In fact, both AQP7 and AQP9 expression are inversely modulated by insulin, leading to increased AQP7 and AQP9 expression during fasting and decreased expression during feeding (Kuriyama et al., 2002; Galli et al., 2021).
The estrogen 17β-estradiol was reported to decrease lipogenesis by upregulating the mRNA and protein expression of AQP7 in mice, being related to the amelioration of ovariectomy-induced hepatic steatosis that is characterized by a decrease in estrogen secretion. These findings suggest that estrogen may play a key role on hepatocyte protection from steatosis through increase of AQP7 expression (Fu et al., 2016). The therapeutic effect of this hormone in menopause-related diseases was once again associated with modulation of AQP7 expression. 17β-estradiol supplement reversed the AQP7 mRNA and protein expression downregulation and glycerol kinase upregulation in visceral adipose tissue in ovariectomized rats, with no effect on the subcutaneous adipose tissue, suggesting its therapeutic potential to prevent the development of obesity in postmenopausal women (Jin et al., 2017). Moreover, high levels of estrogen resulted in AQP9 downregulation in Sertoli cells (Bernardino et al., 2018; Carrageta et al., 2020) which was also observed in male rat epididymis efferent ducts, confirming AQP9 estrogen sensitivity. However, this effect could be reverted with testosterone administration (Pastor-Soler et al., 2010). Additionally, in orchiectomized rat epididymis, AQP3 expression was suppressed and was partially recovered by testosterone treatment (Hermo et al., 2004). Testosterone also induced AQP7 upregulation in the uterus of ovariectomized rats, leading to decreased uterine fluid volume (Salleh et al., 2015). In addition, AQP3 upregulation by estrogen in estrogen receptor-positive breast cancer and by both estrogen and progesterone in endometrial cells, affected cancer cell migration and invasion through reorganization of actin cytoskeleton and EMT (Huang et al., 2015; Cui et al., 2018).
Leptin is an endocrine hormone with a well-known role in body energy homeostasis. Leptin deficiency was associated with obesity and NAFLD showing increased AQP3 and AQP7 expression levels in white adipose tissue with no changes in AQP9 expression. Leptin chronic administration to leptin deficient ob/ob mice decreased AQP3 and AQP7 mRNA and protein levels in adipocytes while hepatic AQP9 was increased, unveiling a potential strategy to control lipid accumulation in adipose tissue and liver while maintaining normal glycemia in obese individuals (Rodriguez et al., 2009). Leptin treatment reduced the transcription of the lipogenic factor PPARγ, and AQP7 induced expression with rosiglitazone administration, a well-know PPARγ agonist, in murine adipocytes. However, leptin treatment in AML12 hepatocytes was reported to increase basal and rosiglitazone-induced AQP9 transcription with slight downregulation of PPARγ mRNA expression (Rodriguez et al., 2015a).
The gut hormone ghrelin downregulates AQP7 protein expression, responsible for lipolysis-derived glycerol efflux in human visceral adipose tissue and plays a role in the excessive fat accumulation in obese individuals (Rodriguez et al., 2009). Both forms of acylated and deacyl ghrelin were reported to downregulate AQP7 mRNA and protein levels in rat pancreatic β-cells together with increased triglycerides content. Another gut hormone, uroguanylin, stimulates lipolysis in human adipocytes and upregulates in parallel genes involved in glycerol (AQP3 and AQP7) and free fatty acids (FATP1 and CD36) efflux (Rodriguez et al., 2016). Moreover, glucagon-like-peptide 1 (GLP-1) also downregulates AQP7 in the same pancreatic cells, with an increase in insulin release. AQP7 reduced levels by ghrelin and GLP-1 can promote intracellular glycerol accumulation, a substrate for triglycerides biosynthesis and insulin synthesis and secretion in β-cells (Mendez-Gimenez et al., 2017).
Dexamethasone and ambroxol were identified as AQP3 modulators, capable of upregulating its mRNA and protein expression with increase in water permeability in human airway epithelial cells. Considering the role of AQP3-mediated water transport in the airways, increasing AQP3 expression through modulation with corticosteroids as dexamethasone and ambroxol could exert a therapeutic effect on pulmonary diseases with airway hypersecretion through clearance of pulmonary fluid (Tanaka et al., 1997; Ben et al., 2008). Moreover, AQP7 mRNA levels were downregulated by treating 3T3-L1 adipocytes with isoproterenol, tumor necrosis factor alpha (TNFα) and dexamethasone (Fasshauer et al., 2003).
Erythropoietin is a glycoprotein hormone, naturally produced by the peritubular cells of the kidney. Erythropoietin was found to prevent the ischemia-induced downregulation of AQP3 protein expression in rats with acute renal failure, improving their urinary concentrating capability. Moreover, the combination of erythropoietin with α-melanocyte-stimulating hormone boosted this effect, being proposed to prevent ischemia/reperfusion injuries through upregulation of renal AQPs (Gong et al., 2004).
MicroRNAs
MicroRNAs (miRNAs) are small, single-stranded non-coding RNAs that have emerged as important post-transcriptional regulators of gene expression (Kan et al., 2012; Christopher et al., 2016; Gomes et al., 2018). miRNAs have been identified as endogenous modulators of AQPs, indicating their potential as a therapeutic approach in AQP-related disorders (Gomes et al., 2018).
Overexpression of AQP3 has been observed in different types of cancer such as lung, pancreas, skin, cornea and colon cancer, where it is involved in cell migration, invasion, proliferation, and EMT (Verkman et al., 2008; Marlar et al., 2017). Accordingly, most of the miRNAs described until now as AQP3 modulators exert their beneficial effects in cancer cells by attenuating their growth and metastasis throughout the body. For instance, the overexpression of miR-124 was reported to downregulate AQP3 mRNA and protein expression, leading to the inhibition of proliferation and migration of HCC cells. Moreover, circular RNA HIPK3 (circHIPK3) was identified as a miR-124 sponge, thereby regulating the proliferation and migration of the HCC cells via miR-124-AQP3 axis through miR-124 downregulation and AQP3 upregulation. When circHIPK3 was silenced, both AQP3 mRNA and protein levels were reduced and miR-124 was upregulated, resulting in reduced HCC cells proliferation in vitro and suppressed tumor growth in vivo (Chen et al., 2018).
In osteosarcoma (OS) tissues and cells, miR-488 was found to be downregulated while AQP3 was markedly increased. Accordingly, the introduction of miR-488 leads to a suppression of the proliferation, invasion and EMT of OS cells through the downregulation of AQP3 mRNA and protein expression by binding to AQP3 3′UTR, supporting the idea that both miR-488 and AQP3 could have prognostic value in OS (Qiu et al., 2018). miR-874 has been reported to target AQP3 in gastric cancer (GC) (Jiang et al., 2014), pancreatic ductal adenocarcinoma (PDAC) (Huang et al., 2017) and non-small cell lung cancer (NSCLC) (Wang et al., 2020). In GC tissues and cells, mir-874 can inhibit cell proliferation, migration and invasion through reduction in AQP3 protein expression (Jiang et al., 2014). Moreover, a mouse model of intestinal ischaemia reperfusion injury showed increased miR-874 levels and decreased AQP3 protein levels, an effect that was similarly observed in Caco-2 cells under hypoxia, where miR-874 induced paracellular permeability by inhibiting AQP3 expression contributing to the impairment of intestinal barrier integrity (Su et al., 2016). This same effect was reported for miR-29a in diarrhea-predominant irritable bowel syndrome rat models (Chao et al., 2017). Overexpression of long non-coding RNA (lncRNA) H19, a miR-874 sponge, could revert the miR-874 induced effects and reduce paracellular permeability, suggesting its potential role in reestablishing the intestinal barrier function (Su et al., 2016). In PDAC cells, miR-874 downregulates AQP3 mRNA and protein expression that in turn can lead to inhibition of cell growth and promotion of cell apoptosis through mTOR signaling pathway modulation (Huang et al., 2017). Regarding NSCLC, the same miRNA was reported to inhibit proliferation, invasion and EMT in vitro through downregulation of AQP3 mRNA and protein levels with inhibition of PI3K/AKT signaling pathway and was also found to suppress cell growth in vivo (Wang et al., 2020).
Further studies identified miR-22 and miR-23a as AQP9 modulators by downregulating its mRNA and protein expression in cultured human hepatocytes and in diabetic rats livers, suggesting that their role in the regulation of glycerol entrance in these cells could be beneficial for the control of glycerol-based gluconeogenesis that is often augmented in diabetes (Karolina et al., 2014). AQP9 was also reported to be modulated by miR-154-5p, which in turn is regulated by lncRNA MALAT-1 in chronic constriction injury (CCI) rats. The downregulation of this lncRNA was found to attenuate neuropathic pain in rats by binding to miR-154-5p that in turn can inhibit AQP9 mRNA and protein expression, unraveling the potential of MALAT-1/miR-154-5p/AQP9 axis as a therapeutic target for neuropathic pain (Wu et al., 2020). Additionally, in rats with myocardial infarction (MI), miR-212 overexpression was suggested to downregulate AQP9 mRNA and protein expression through PI3K/AKT signaling pathway activation, thereby exerting its therapeutic effect by reducing cardiomyocytes apoptosis, promoting vascular regeneration and attenuating ventricular remodeling (Ren and Wang, 2018). Moreover, recent findings showed AQP9 as a target for both miR-532-5p and miR-532-3p in renal cell carcinoma (RCC) cells, being its aberrant expression intimately associated with poor prognosis of RCC patients. However, when both miR-532-5p and miR-532-5p were overexpressed in RCC cells resulting in reduction of AQP9 mRNA and protein levels, cell proliferation, migration and invasion were attenuated. Similar effect was attained with AQP9 knockdown with decrease in cancer aggressiveness, suggesting AQP9 as a promising therapeutic target for RCC (Yamada et al., 2019).
Peptides
Peptides have been receiving great attention for their ability to establish specific and high-affinity interactions with endogenous receptors (Martinez-Villaluenga and Hernandez-Ledesma, 2020). In fact, bioactive peptides can act as hormones, neurotransmitters, and antimicrobial agents in vivo, with reported beneficial effects on blood pressure and lipid metabolism as well as anticancer, immunomodulatory, analgesic, antioxidant and anti-inflammatory activity (Tinoco and Saghatelian, 2011; Cicero et al., 2017). Considering their health-promoting effects, peptides have been investigated for their potential role as aquaporin modulators.
Apelin-13 is a small peptide found in the adipose tissue that is thought to be implicated on energy metabolism and insulin sensitivity, being also associated with obesity. This peptide was shown to decrease triglycerides accumulation in hypertrophic adipocytes in vitro through AQP7 mRNA and protein upregulation via PI3K signaling pathway, revealing that apelin-13 is a promising starting point to develop novel treatments against obesity and related health problems (Guo et al., 2014).
Antibodies
Recently, Hara-Chikuma et al. (2020) developed an anti-AQP3 monoclonal antibody that targets an extracellular epitope of AQP3 in macrophages. The administration of this anti-AQP3 monoclonal antibody in a liver injury mice model resulted in the suppression of inflammation and liver injury through the inhibition of AQP3-mediated H2O2 transport, NF-κB signaling and macrophage activation. This study emerges as a starting point for antibodies’ production to specifically target AQPs and develop directed therapies against AQP-associated disorders.
Conclusion
Aquaglyceroporin modulators have gained much attention in recent years and their development is currently considered a promising strategy for treatment of AQP-associated pathologies. The vast array of compounds reported to affect aquaglyceroporins expression or function has been growing, as an attempt to validate their pharmacological activity for human diseases. However, the application of aquaglyceroporin modulators in clinical trials is still far to be implemented, possibly due the lack of selective and potent modulators able to be administered in vivo.
Different classes of molecules, from metal compounds and small molecules to natural compounds and biologicals, have been reported to effectively target aquaglyceroporins, either modifying pore permeability or protein expression level. Yet, their mechanisms of action are obscure and the proof of concept for their pharmacological activity in most animal models of disease is still unmet. While advanced atomistic simulations can provide valuable information on the mechanisms of selectivity and substrate transport via AQPs, as well as insights into the mechanisms of their inhibition by different substances, further studies are needed to improve the efficiency and selectivity of modulators, avoiding cross-reactivity with other targets and potential side effects. Given the broad range of diseases where aquaglyceroporins play a role, the design and development of new potent and selective modulators may pave the way to the discovery of innovative therapeutics.
Author Contributions
CP, IVdS, and GS: conceptualization. CP and GS: initial draft preparation. CP, DW, and IVdS: figure preparation. AC and GS: supervision, critical revision. All authors discussed and approved the final manuscript.
Funding
This work was funded by Fundação para a Ciência e Tecnologia (FCT), Portugal, through grant PTDC/BTM-SAL/28977/2017, fellowship 2020.04974.BD to C. Pimpão and projects UIDB/04138/2020 and UIDP/04138/2020 to iMed.ULisboa.
Conflict of Interest
The authors declare that the research was conducted in the absence of any commercial or financial relationships that could be construed as a potential conflict of interest.
Publisher’s Note
All claims expressed in this article are solely those of the authors and do not necessarily represent those of their affiliated organizations, or those of the publisher, the editors and the reviewers. Any product that may be evaluated in this article, or claim that may be made by its manufacturer, is not guaranteed or endorsed by the publisher.
References
Abir-Awan, M., Kitchen, P., Salman, M. M., Conner, M. T., Conner, A. C., and Bill, R. M. (2019). Inhibitors of Mammalian Aquaporin Water Channels. Int. J. Mol. Sci. 20 (7), 1589. doi:10.3390/ijms20071589
Aharon, R., and Bar-Shavit, Z. (2006). Involvement of Aquaporin 9 in Osteoclast Differentiation. J. Biol. Chem. 281 (28), 19305–19309. doi:10.1074/jbc.M601728200
Aikman, B., de Almeida, A., Meier-Menches, S. M., and Casini, A. (2018). Aquaporins in Cancer Development: Opportunities for Bioinorganic Chemistry to Contribute Novel Chemical Probes and Therapeutic Agents. Metallomics 10 (5), 696–712. doi:10.1039/c8mt00072g
Alejandra, R., Natalia, S., and Alicia, E. D. (2018). The Blocking of Aquaporin-3 (AQP3) Impairs Extravillous Trophoblast Cell Migration. Biochem. Biophys. Res. Commun. 499 (2), 227–232. doi:10.1016/j.bbrc.2018.03.133
Babak, M. V., Chong, K. R., Rapta, P., Zannikou, M., Tang, H. M., Reichert, L., et al. (2021). Interfering with Metabolic Profile of Triple-Negative Breast Cancers Using Rationally Designed Metformin Prodrugs. Angew. Chem. Int. Ed. Engl. 60 (24), 13405–13413. doi:10.1002/anie.202102266
Baldini, F., Portincasa, P., Grasselli, E., Damonte, G., Salis, A., Bonomo, M., et al. (2020). Aquaporin-9 Is Involved in the Lipid-Lowering Activity of the Nutraceutical Silybin on Hepatocytes through Modulation of Autophagy and Lipid Droplets Composition. Biochim. Biophys. Acta Mol. Cel Biol Lipids 1865 (3), 158586. doi:10.1016/j.bbalip.2019.158586
Baumgart, F., Rossi, A., and Verkman, A. S. (2012). Light Inactivation of Water Transport and Protein-Protein Interactions of Aquaporin-Killer Red Chimeras. J. Gen. Physiol. 139 (1), 83–91. doi:10.1085/jgp.201110712
Beitz, E., Becker, D., von Bulow, J., Conrad, C., Fricke, N., Geadkaew, A., et al. (2009). In Vitro analysis and Modification of Aquaporin Pore Selectivity. Handb Exp. Pharmacol. 190, 77–92. doi:10.1007/978-3-540-79885-9_4
Ben, Y., Chen, J., Zhu, R., Gao, L., and Bai, C. (2008). Upregulation of AQP3 and AQP5 Induced by Dexamethasone and Ambroxol in A549 Cells. Respir. Physiol. Neurobiol. 161 (2), 111–118. doi:10.1016/j.resp.2007.12.007
Bernardino, R. L., Carrageta, D. F., Silva, A. M., Calamita, G., Alves, M. G., Soveral, G., et al. (2018). Estrogen Modulates Glycerol Permeability in Sertoli Cells through Downregulation of Aquaporin-9. Cells 7 (10), 153. doi:10.3390/cells7100153
Bertrand, B., and Casini, A. (2014). A golden Future in Medicinal Inorganic Chemistry: the Promise of Anticancer Gold Organometallic Compounds. Dalton Trans. 43 (11), 4209–4219. doi:10.1039/c3dt52524d
Bertrand, B., Spreckelmeyer, S., Bodio, E., Cocco, F., Picquet, M., Richard, P., et al. (2015). Exploring the Potential of Gold(III) Cyclometallated Compounds as Cytotoxic Agents: Variations on the C^N Theme. Dalton Trans. 44 (26), 11911–11918. doi:10.1039/c5dt01023c
Bestetti, S., Galli, M., Sorrentino, I., Pinton, P., Rimessi, A., Sitia, R., et al. (2020). Human Aquaporin-11 Guarantees Efficient Transport of H2O2 across the Endoplasmic Reticulum Membrane. Redox Biol. 28, 101326. doi:10.1016/j.redox.2019.101326
Bjorkskov, F. B., Krabbe, S. L., Nurup, C. N., Missel, J. W., Spulber, M., Bomholt, J., et al. (2017). Purification and Functional Comparison of Nine Human Aquaporins Produced in Saccharomyces cerevisiae for the Purpose of Biophysical Characterization. Sci. Rep. 7 (1), 16899. doi:10.1038/s41598-017-17095-6
Boque, N., de la Iglesia, R., de la Garza, A. L., Milagro, F. I., Olivares, M., Banuelos, O., et al. (2013). Prevention of Diet-Induced Obesity by Apple Polyphenols in Wistar Rats through Regulation of Adipocyte Gene Expression and DNA Methylation Patterns. Mol. Nutr. Food Res. 57 (8), 1473–1478. doi:10.1002/mnfr.201200686
Calamita, G., Perret, J., and Delporte, C. (2018). Aquaglyceroporins: Drug Targets for Metabolic Diseases?. Front. Physiol. 9, 851. doi:10.3389/fphys.2018.00851
Campos, E., Moura, T. F., Oliva, A., Leandro, P., and Soveral, G. (2011). Lack of Aquaporin 3 in Bovine Erythrocyte Membranes Correlates with Low Glycerol Permeation. Biochem. Biophys. Res. Commun. 408 (3), 477–481. doi:10.1016/j.bbrc.2011.04.057
Cao, C., Wan, S., Jiang, Q., Amaral, A., Lu, S., Hu, G., et al. (2008). All-trans Retinoic Acid Attenuates Ultraviolet Radiation-Induced Down-Regulation of Aquaporin-3 and Water Permeability in Human Keratinocytes. J. Cel Physiol 215 (2), 506–516. doi:10.1002/jcp.21336
Carrageta, D. F., Bernardino, R. L., Soveral, G., Calamita, G., Alves, M. G., and Oliveira, P. F. (2020). Aquaporins and Male (In)fertility: Expression and Role throughout the Male Reproductive Tract. Arch. Biochem. Biophys. 679, 108222. doi:10.1016/j.abb.2019.108222
Casini, A., Hartinger, C., Gabbiani, C., Mini, E., Dyson, P. J., Keppler, B. K., et al. (2008). Gold(III) Compounds as Anticancer Agents: Relevance of Gold-Protein Interactions for Their Mechanism of Action. J. Inorg. Biochem. 102 (3), 564–575. doi:10.1016/j.jinorgbio.2007.11.003
Castle, N. A. (2005). Aquaporins as Targets for Drug Discovery. Drug Discov. Today 10 (7), 485–493. doi:10.1016/S1359-6446(05)03390-8
Chakrabarti, N., Tajkhorshid, E., Roux, B., and Pomes, R. (2004). Molecular Basis of Proton Blockage in Aquaporins. Structure 12 (1), 65–74. doi:10.1016/j.str.2003.11.017
Chao, G., Wang, Y., Zhang, S., Yang, W., Ni, Z., and Zheng, X. (2017). MicroRNA-29a Increased the Intestinal Membrane Permeability of Colonic Epithelial Cells in Irritable Bowel Syndrome Rats. Oncotarget 8 (49), 85828–85837. doi:10.18632/oncotarget.20687
Chauvigne, F., Ferre, A., and Cerda, J. (2021). The Xenopus Oocyte as an Expression System for Functional Analyses of Fish Aquaporins. Methods Mol. Biol. 2218, 11–28. doi:10.1007/978-1-0716-0970-5_2
Chemical Computing Group ULC (2021). Molecular Operating Environment (MOE): Chemical Computing Group ULC. 1010 Sherbooke St. West, Suite #910, Montreal, QC, Canada: Chemical Computing Group ULC, H3A 2R7.
Chen, G., Shi, Y., Liu, M., and Sun, J. (2018). circHIPK3 Regulates Cell Proliferation and Migration by Sponging miR-124 and Regulating AQP3 Expression in Hepatocellular Carcinoma. Cell Death Dis 9 (2), 175. doi:10.1038/s41419-017-0204-3
Chen, X. F., Li, C. F., Lu, L., and Mei, Z. C. (2016). Expression and Clinical Significance of Aquaglyceroporins in Human Hepatocellular Carcinoma. Mol. Med. Rep. 13 (6), 5283–5289. doi:10.3892/mmr.2016.5184
Choudhary, V., Olala, L. O., Kagha, K., Pan, Z. Q., Chen, X., Yang, R., et al. (2017). Regulation of the Glycerol Transporter, Aquaporin-3, by Histone Deacetylase-3 and P53 in Keratinocytes. J. Invest. Dermatol. 137 (9), 1935–1944. doi:10.1016/j.jid.2017.04.031
Christopher, A. F., Kaur, R. P., Kaur, G., Kaur, A., Gupta, V., and Bansal, P. (2016). MicroRNA Therapeutics: Discovering Novel Targets and Developing Specific Therapy. Perspect. Clin. Res. 7 (2), 68–74. doi:10.4103/2229-3485.179431
Cicero, A. F. G., Fogacci, F., and Colletti, A. (2017). Potential Role of Bioactive Peptides in Prevention and Treatment of Chronic Diseases: a Narrative Review. Br. J. Pharmacol. 174 (11), 1378–1394. doi:10.1111/bph.13608
Cui, D., Sui, L., Han, X., Zhang, M., Guo, Z., Chen, W., et al. (2018). Aquaporin-3 Mediates Ovarian Steroid Hormone-Induced Motility of Endometrial Epithelial Cells. Hum. Reprod. 33 (11), 2060–2073. doi:10.1093/humrep/dey290
da Silva, I. V., Cardoso, C., Mendez-Gimenez, L., Camoes, S. P., Fruhbeck, G., Rodriguez, A., et al. (2020a). Aquaporin-7 and Aquaporin-12 Modulate the Inflammatory Phenotype of Endocrine Pancreatic Beta-Cells. Arch. Biochem. Biophys. 691, 108481. doi:10.1016/j.abb.2020.108481
da Silva, I. V., Cardoso, C., Martinez-Banaclocha, H., Casini, A., Pelegrin, P., and Soveral, G. (2020b). Aquaporin-3 Is Involved in NLRP3-Inflammasome Activation Contributing to the Setting of Inflammatory Response. Cell Mol Life Sci 78 (6), 3073–3085. doi:10.1007/s00018-020-03708-3
da Silva, I. V., Barroso, M., Moura, T., Castro, R., and Soveral, G. (2018a). Endothelial Aquaporins and Hypomethylation: Potential Implications for Atherosclerosis and Cardiovascular Disease. Int. J. Mol. Sci. 19 (1), 130. doi:10.3390/ijms19010130
da Silva, I. V., Rodrigues, J. S., Rebelo, I., Miranda, J. P. G., and Soveral, G. (2018b). Revisiting the Metabolic Syndrome: the Emerging Role of Aquaglyceroporins. Cel Mol Life Sci 75 (11), 1973–1988. doi:10.1007/s00018-018-2781-4
da Silva, I. V., Silva, A. G., Pimpao, C., and Soveral, G. (2021). Skin Aquaporins as Druggable Targets: Promoting Health by Addressing the Disease. Biochimie 188, 35–44. doi:10.1016/j.biochi.2021.05.019
da Silva, I. V., and Soveral, G. (2021). Aquaporins in Immune Cells and Inflammation: New Targets for Drug Development. Int. J. Mol. Sci. 22 (4), 1845. doi:10.3390/ijms22041845
da Silva, I. V., and Soveral, G. (2017). Aquaporins in Obesity. Adv. Exp. Med. Biol. 969, 227–238. doi:10.1007/978-94-024-1057-0_15
de Almeida, A., Mosca, A. F., Wragg, D., Wenzel, M., Kavanagh, P., Barone, G., et al. (2017). The Mechanism of Aquaporin Inhibition by Gold Compounds Elucidated by Biophysical and Computational Methods. Chem. Commun. (Camb) 53 (27), 3830–3833. doi:10.1039/c7cc00318h
de Almeida, A., Soveral, G., and Casini, A. (2014). Gold Compounds as Aquaporin Inhibitors: New Opportunities for Therapy and Imaging. Med. Chem. Commun. 5 (10), 1444–1453. doi:10.1039/c4md00265b
de Groot, B. L., and Grubmuller, H. (2001). Water Permeation across Biological Membranes: Mechanism and Dynamics of Aquaporin-1 and GlpF. Science 294 (5550), 2353–2357. doi:10.1126/science.1066115
De Ieso, M. L., and Yool, A. J. (2018). Mechanisms of Aquaporin-Facilitated Cancer Invasion and Metastasis. Front. Chem. 6, 135. doi:10.3389/fchem.2018.00135
de Mare, S. W., Venskutonyte, R., Eltschkner, S., de Groot, B. L., and Lindkvist-Petersson, K. (2020). Structural Basis for Glycerol Efflux and Selectivity of Human Aquaporin 7. Structure 28 (2), 215–222. e3. doi:10.1016/j.str.2019.11.011
Delporte, C., Virreira, M., Crutzen, R., Louchami, K., Sener, A., Malaisse, W. J., et al. (2009). Functional Role of Aquaglyceroporin 7 Expression in the Pancreatic Beta-Cell Line BRIN-BD11. J. Cel Physiol 221 (2), 424–429. doi:10.1002/jcp.21872
Direito, I., Paulino, J., Vigia, E., Brito, M. A., and Soveral, G. (2017). Differential Expression of Aquaporin-3 and Aquaporin-5 in Pancreatic Ductal Adenocarcinoma. J. Surg. Oncol. 115 (8), 980–996. doi:10.1002/jso.24605
Esteva-Font, C., Phuan, P. W., Anderson, M. O., and Verkman, A. S. (2013). A Small Molecule Screen Identifies Selective Inhibitors of Urea Transporter UT-A. Chem. Biol. 20 (10), 1235–1244. doi:10.1016/j.chembiol.2013.08.005
Fasshauer, M., Klein, J., Lossner, U., Klier, M., Kralisch, S., and Paschke, R. (2003). Suppression of Aquaporin Adipose Gene Expression by Isoproterenol, TNFalpha, and Dexamethasone. Horm. Metab. Res. 35 (4), 222–227. doi:10.1055/s-2003-39478
Fenton, R. A., Moeller, H. B., Nielsen, S., de Groot, B. L., and Rutzler, M. (2010). A Plate Reader-Based Method for Cell Water Permeability Measurement. Am. J. Physiol. Ren. Physiol 298 (1), F224–F230. doi:10.1152/ajprenal.00463.2009
Fiorentini, D., Zambonin, L., Dalla Sega, F. V., and Hrelia, S. (2015). Polyphenols as Modulators of Aquaporin Family in Health and Disease. Oxid Med. Cel Longev 2015, 196914. doi:10.1155/2015/196914
Frick, A., Eriksson, U. K., de Mattia, F., Oberg, F., Hedfalk, K., Neutze, R., et al. (2014). X-ray Structure of Human Aquaporin 2 and its Implications for Nephrogenic Diabetes Insipidus and Trafficking. Proc. Natl. Acad. Sci. U S A. 111 (17), 6305–6310. doi:10.1073/pnas.1321406111
Froger, A., Rolland, J. P., Bron, P., Lagree, V., Caherec, F. L., Deschamps, S., et al. (2001). Functional Characterization of a Microbial Aquaglyceroporin. Microbiology (Reading) 147 (Pt 5), 1129–1135. doi:10.1099/00221287-147-5-1129
Fu, X., Xing, L., Xu, W., and Shu, J. (2016). Treatment with Estrogen Protects against Ovariectomy-Induced Hepatic Steatosis by Increasing AQP7 Expression. Mol. Med. Rep. 14 (1), 425–431. doi:10.3892/mmr.2016.5236
Galietta, L. J., Haggie, P. M., and Verkman, A. S. (2001). Green Fluorescent Protein-Based Halide Indicators with Improved Chloride and Iodide Affinities. FEBS Lett. 499 (3), 220–224. doi:10.1016/s0014-5793(01)02561-3
Galli, M., Hameed, A., Zbikowski, A., and Zabielski, P. (2021). Aquaporins in Insulin Resistance and Diabetes: More Than Channels. Redox Biol. 44, 102027. doi:10.1016/j.redox.2021.102027
Geyer, R. R., Musa-Aziz, R., Qin, X., and W, F. (2013). Boron: Relative CO(2)/NH(3) Selectivities of Mammalian Aquaporins 0-9. Am. J. Physiol. Cel Physiol 304 (10), C985–C994. doi:10.1152/ajpcell.00033.2013
Gomes, A., da Silva, I. V., Rodrigues, C. M. P., Castro, R. E., and Soveral, G. (2018). The Emerging Role of microRNAs in Aquaporin Regulation. Front. Chem. 6, 238. doi:10.3389/fchem.2018.00238
Gong, H., Wang, W., Kwon, T. H., Jonassen, T., Li, C., Ring, T., et al. (2004). EPO and Alpha-MSH Prevent Ischemia/reperfusion-Induced Down-Regulation of AQPs and Sodium Transporters in Rat Kidney. Kidney Int. 66 (2), 683–695. doi:10.1111/j.1523-1755.2004.00791.x
Gotfryd, K., Mosca, A. F., Missel, J. W., Truelsen, S. F., Wang, K., Spulber, M., et al. (2018). Human Adipose Glycerol Flux Is Regulated by a pH Gate in AQP10. Nat. Commun. 9 (1), 4749. doi:10.1038/s41467-018-07176-z
Graziani, V., Marrone, A., Re, N., Coletti, C., Platts, J. A., and Casini, A. (2017). A Multi-Level Theoretical Study to Disclose the Binding Mechanisms of Gold(III)-Bipyridyl Compounds as Selective Aquaglyceroporin Inhibitors. Chemistry 23 (55), 13802–13813. doi:10.1002/chem.201703092
Guo, M., Chen, F., Lin, T., Peng, Y., Li, W., Zhu, X., et al. (2014). Apelin-13 Decreases Lipid Storage in Hypertrophic Adipocytes In Vitro through the Upregulation of AQP7 Expression by the PI3K Signaling Pathway. Med. Sci. Monit. 20, 1345–1352. doi:10.12659/MSM.890124
Hara, M., Ma, T., and Verkman, A. S. (2002). Selectively Reduced Glycerol in Skin of Aquaporin-3-Deficient Mice May Account for Impaired Skin Hydration, Elasticity, and Barrier Recovery. J. Biol. Chem. 277 (48), 46616–46621. doi:10.1074/jbc.M209003200
Hara, M., and Verkman, A. S. (2003). Glycerol Replacement Corrects Defective Skin Hydration, Elasticity, and Barrier Function in Aquaporin-3-Deficient Mice. Proc. Natl. Acad. Sci. U S A. 100 (12), 7360–7365. doi:10.1073/pnas.1230416100
Hara-Chikuma, M., Sohara, E., Rai, T., Ikawa, M., Okabe, M., Sasaki, S., et al. (2005). Progressive Adipocyte Hypertrophy in Aquaporin-7-Deficient Mice: Adipocyte Glycerol Permeability as a Novel Regulator of Fat Accumulation. J. Biol. Chem. 280 (16), 15493–15496. doi:10.1074/jbc.C500028200
Hara-Chikuma, M., Tanaka, M., Verkman, A. S., and Yasui, M. (2020). Inhibition of Aquaporin-3 in Macrophages by a Monoclonal Antibody as Potential Therapy for Liver Injury. Nat. Commun. 11 (1), 5666. doi:10.1038/s41467-020-19491-5
Hara-Chikuma, M., and Verkman, A. S. (2006). Physiological Roles of Glycerol-Transporting Aquaporins: the Aquaglyceroporins. Cel Mol Life Sci 63 (12), 1386–1392. doi:10.1007/s00018-006-6028-4
Hara-Chikuma, M., and Verkman, A. S. (2008a). Roles of Aquaporin-3 in the Epidermis. J. Invest. Dermatol. 128 (9), 2145–2151. doi:10.1038/jid.2008.70
Hara-Chikuma, M., and Verkman, A. S. (2008b). Aquaporin-3 Facilitates Epidermal Cell Migration and Proliferation during Wound Healing. J. Mol. Med. (Berl) 86 (2), 221–231. doi:10.1007/s00109-007-0272-4
Hara-Chikuma, M., and Verkman, A. S. (2008c). Prevention of Skin Tumorigenesis and Impairment of Epidermal Cell Proliferation by Targeted Aquaporin-3 Gene Disruption. Mol. Cel Biol 28 (1), 326–332. doi:10.1128/MCB.01482-07
Harries, W. E., Akhavan, D., Miercke, L. J., Khademi, S., and Stroud, R. M. (2004). The Channel Architecture of Aquaporin 0 at a 2.2-A Resolution. Proc. Natl. Acad. Sci. U S A. 101 (39), 14045–14050. doi:10.1073/pnas.0405274101
Hermo, L., Krzeczunowicz, D., and Ruz, R. (2004). Cell Specificity of Aquaporins 0, 3, and 10 Expressed in the Testis, Efferent Ducts, and Epididymis of Adult Rats. J. Androl. 25 (4), 494–505. doi:10.1002/j.1939-4640.2004.tb02820.x
Herrera, M., Hong, N. J., and Garvin, J. L. (2006). Aquaporin-1 Transports NO across Cell Membranes. Hypertension 48 (1), 157–164. doi:10.1161/01.HYP.0000223652.29338.77
Hibuse, T., Maeda, N., Funahashi, T., Yamamoto, K., Nagasawa, A., Mizunoya, W., et al. (2005). Aquaporin 7 Deficiency Is Associated with Development of Obesity through Activation of Adipose Glycerol Kinase. Proc. Natl. Acad. Sci. U S A. 102 (31), 10993–10998. doi:10.1073/pnas.0503291102
Hibuse, T., Maeda, N., Nakatsuji, H., Tochino, Y., Fujita, K., Kihara, S., et al. (2009). The Heart Requires Glycerol as an Energy Substrate through Aquaporin 7, a Glycerol Facilitator. Cardiovasc. Res. 83 (1), 34–41. doi:10.1093/cvr/cvp095
Ho, J. D., Yeh, R., Sandstrom, A., Chorny, I., Harries, W. E., Robbins, R. A., et al. (2009). Crystal Structure of Human Aquaporin 4 at 1.8 A and its Mechanism of Conductance. Proc. Natl. Acad. Sci. U S A. 106 (18), 7437–7442. doi:10.1073/pnas.0902725106
Horsefield, R., Norden, K., Fellert, M., Backmark, A., Tornroth-Horsefield, S., Terwisscha van Scheltinga, A. C., et al. (2008). High-resolution X-ray Structure of Human Aquaporin 5. Proc. Natl. Acad. Sci. U S A. 105 (36), 13327–13332. doi:10.1073/pnas.0801466105
Huang, X., Huang, L., and Shao, M. (2017). Aquaporin 3 Facilitates Tumor Growth in Pancreatic Cancer by Modulating mTOR Signaling. Biochem. Biophys. Res. Commun. 486 (4), 1097–1102. doi:10.1016/j.bbrc.2017.03.168
Huang, Y. T., Zhou, J., Shi, S., Xu, H. Y., Qu, F., Zhang, D., et al. (2015). Identification of Estrogen Response Element in Aquaporin-3 Gene that Mediates Estrogen-Induced Cell Migration and Invasion in Estrogen Receptor-Positive Breast Cancer. Sci. Rep. 5, 12484. doi:10.1038/srep12484
Hub, J. S., and de Groot, B. L. (2008). Mechanism of Selectivity in Aquaporins and Aquaglyceroporins. Proc. Natl. Acad. Sci. U S A. 105 (4), 1198–1203. doi:10.1073/pnas.0707662104
Hub, J. S., Grubmuller, H., and de Groot, B. L. (2009). Dynamics and Energetics of Permeation through Aquaporins. What Do We Learn from Molecular Dynamics Simulations?. Handb Exp. Pharmacol. 190, 57–76. doi:10.1007/978-3-540-79885-9_3
Humphrey, W., Dalke, A., and Schulten, K. (1996). VMD: Visual Molecular Dynamics. J. Mol. Graphics 14 (1), 33–38. doi:10.1016/0263-7855(96)00018-5
Hung, C. F., Hsiao, C. Y., Hsieh, W. H., Li, H. J., Tsai, Y. J., Lin, C. N., et al. (2017). 18ss-glycyrrhetinic Acid Derivative Promotes Proliferation, Migration and Aquaporin-3 Expression in Human Dermal Fibroblasts. PLoS One 12 (8), e0182981. doi:10.1371/journal.pone.0182981
Ikarashi, N., Baba, K., Ushiki, T., Kon, R., Mimura, A., Toda, T., et al. (2011). The Laxative Effect of Bisacodyl Is Attributable to Decreased Aquaporin-3 Expression in the colon Induced by Increased PGE2 Secretion from Macrophages. Am. J. Physiol. Gastrointest. Liver Physiol. 301 (5), G887–G895. doi:10.1152/ajpgi.00286.2011
Ishibashi, K., Tanaka, Y., and Morishita, Y. (2014). The Role of Mammalian Superaquaporins inside the Cell. Biochim. Biophys. Acta 1840 (5), 1507–1512. doi:10.1016/j.bbagen.2013.10.039
Ishibashi, K., Tanaka, Y., and Morishita, Y. (2021). The Role of Mammalian Superaquaporins inside the Cell: An Update. Biochim. Biophys. Acta Biomembr 1863 (7), 183617. doi:10.1016/j.bbamem.2021.183617
Ishihama, S., Yoshida, S., Yoshida, T., Mori, Y., Ouchi, N., Eguchi, S., et al. (2021). LPL/AQP7/GPD2 Promotes Glycerol Metabolism under Hypoxia and Prevents Cardiac Dysfunction during Ischemia. FASEB J. 35 (12), e22048. doi:10.1096/fj.202100882R
Ishii, M., Ohta, K., Katano, T., Urano, K., Watanabe, J., Miyamoto, A., et al. (2011). Dual Functional Characteristic of Human Aquaporin 10 for Solute Transport. Cell Physiol Biochem 27 (6), 749–756. doi:10.1159/000330083
Ismail, M., Bokaee, S., Morgan, R., Davies, J., Harrington, K. J., and Pandha, H. (2009). Inhibition of the Aquaporin 3 Water Channel Increases the Sensitivity of Prostate Cancer Cells to Cryotherapy. Br. J. Cancer 100 (12), 1889–1895. doi:10.1038/sj.bjc.6605093
Jablonski, E. M., Mattocks, M. A., Sokolov, E., Koniaris, L. G., Hughes, F. M., Fausto, N., et al. (2007). Decreased Aquaporin Expression Leads to Increased Resistance to Apoptosis in Hepatocellular Carcinoma. Cancer Lett. 250 (1), 36–46. doi:10.1016/j.canlet.2006.09.013
Jahn, T. P., Moller, A. L., Zeuthen, T., Holm, L. M., Klaerke, D. A., Mohsin, B., et al. (2004). Aquaporin Homologues in Plants and Mammals Transport Ammonia. FEBS Lett. 574 (1-3), 31–36. doi:10.1016/j.febslet.2004.08.004
Janosi, L., and Ceccarelli, M. (2013). The Gating Mechanism of the Human Aquaporin 5 Revealed by Molecular Dynamics Simulations. PLoS One 8 (4), e59897. doi:10.1371/journal.pone.0059897
Jelen, S., Wacker, S., Aponte-Santamaria, C., Skott, M., Rojek, A., Johanson, U., et al. (2011). Aquaporin-9 Protein Is the Primary Route of Hepatocyte Glycerol Uptake for Glycerol Gluconeogenesis in Mice. J. Biol. Chem. 286 (52), 44319–44325. doi:10.1074/jbc.M111.297002
Ji, C., Cao, C., Lu, S., Kivlin, R., Amaral, A., Kouttab, N., et al. (2008). Curcumin Attenuates EGF-Induced AQP3 Up-Regulation and Cell Migration in Human Ovarian Cancer Cells. Cancer Chemother. Pharmacol. 62 (5), 857–865. doi:10.1007/s00280-007-0674-6
Jiang, B., Li, Z., Zhang, W., Wang, H., Zhi, X., Feng, J., et al. (2014). miR-874 Inhibits Cell Proliferation, Migration and Invasion through Targeting Aquaporin-3 in Gastric Cancer. J. Gastroenterol. 49 (6), 1011–1025. doi:10.1007/s00535-013-0851-9
Jiang, Y. J., Kim, P., Lu, Y. F., and Feingold, K. R. (2011). PPARgamma Activators Stimulate Aquaporin 3 Expression in Keratinocytes/epidermis. Exp. Dermatol. 20 (7), 595–599. doi:10.1111/j.1600-0625.2011.01269.x
Jin, B., Chen, X., Xing, L., Xu, W., Fu, X., Zhu, J., et al. (2017). Tissue-specific Effects of Estrogen on Glycerol Channel Aquaporin 7 Expression in an Ovariectomized Mouse Model of Menopause. Climacteric 20 (4), 385–390. doi:10.1080/13697137.2017.1319920
Kan, A. A., van Erp, S., Derijck, A. A., de Wit, M., Hessel, E. V., O'Duibhir, E., et al. (2012). Genome-wide microRNA Profiling of Human Temporal Lobe Epilepsy Identifies Modulators of the Immune Response. Cel Mol Life Sci 69 (18), 3127–3145. doi:10.1007/s00018-012-0992-7
Karolina, D. S., Armugam, A., Sepramaniam, S., Pek, S., Wong, M. T., Lim, S., et al. (2014). miR-22 and miR-23a Control Glycerol-dependent Gluconeogenesis by Regulating Aquaporin 9 Expression. Metabolomics 4 (S2), 002. doi:10.4172/2153-0769.S2-002
Katano, T., Ito, Y., Ohta, K., Yasujima, T., Inoue, K., and Yuasa, H. (2014). Functional Characteristics of Aquaporin 7 as a Facilitative Glycerol Carrier. Drug Metab. Pharmacokinet. 29 (3), 244–248. doi:10.2133/dmpk.dmpk-13-rg-121
Kishida, K., Kuriyama, H., Funahashi, T., Shimomura, I., Kihara, S., Ouchi, N., et al. (2000). Aquaporin Adipose, a Putative Glycerol Channel in Adipocytes. J. Biol. Chem. 275 (27), 20896–20902. doi:10.1074/jbc.M001119200
Kishida, K., Shimomura, I., Nishizawa, H., Maeda, N., Kuriyama, H., Kondo, H., et al. (2001a). Enhancement of the Aquaporin Adipose Gene Expression by a Peroxisome Proliferator-Activated Receptor Gamma. J. Biol. Chem. 276 (51), 48572–48579. doi:10.1074/jbc.M108213200
Kishida, K., Shimomura, I., Kondo, H., Kuriyama, H., Makino, Y., Nishizawa, H., et al. (2001b). Genomic Structure and Insulin-Mediated Repression of the Aquaporin Adipose (AQPap), Adipose-specific Glycerol Channel. J. Biol. Chem. 276 (39), 36251–36260. doi:10.1074/jbc.M106040200
Kon, R., Yamamura, M., Matsunaga, Y., Kimura, H., Minami, M., Kato, S., et al. (2018). Laxative Effect of Repeated Daiokanzoto Is Attributable to Decrease in Aquaporin-3 Expression in the colon. J. Nat. Med. 72 (2), 493–502. doi:10.1007/s11418-018-1174-1
Kuriyama, H., Shimomura, I., Kishida, K., Kondo, H., Furuyama, N., Nishizawa, H., et al. (2002). Coordinated Regulation of Fat-specific and Liver-specific Glycerol Channels, Aquaporin Adipose and Aquaporin 9. Diabetes 51 (10), 2915–2921. doi:10.2337/diabetes.51.10.2915
Laforenza, U., Miceli, E., Gastaldi, G., Scaffino, M. F., Ventura, U., Fontana, J. M., et al. (2010). Solute Transporters and Aquaporins Are Impaired in Celiac Disease. Biol. Cel 102 (8), 457–467. doi:10.1042/BC20100023
Laforenza, U., Scaffino, M. F., and Gastaldi, G. (2013). Aquaporin-10 Represents an Alternative Pathway for Glycerol Efflux from Human Adipocytes. PLoS One 8 (1), e54474. doi:10.1371/journal.pone.0054474
Laforenza, U. (2012). Water Channel Proteins in the Gastrointestinal Tract. Mol. Aspects Med. 33 (5-6), 642–650. doi:10.1016/j.mam.2012.03.001
Lebeck, J., Ostergard, T., Rojek, A., Fuchtbauer, E. M., Lund, S., Nielsen, S., et al. (2012). Gender-specific Effect of Physical Training on AQP7 Protein Expression in Human Adipose Tissue. Acta Diabetol. 49 (Suppl. 1), S215–S226. doi:10.1007/s00592-012-0430-1
Lee, D. H., Park, D. B., Lee, Y. K., An, C. S., Oh, Y. S., Kang, J. S., et al. (2005). The Effects of Thiazolidinedione Treatment on the Regulations of Aquaglyceroporins and Glycerol Kinase in OLETF Rats. Metabolism 54 (10), 1282–1289. doi:10.1016/j.metabol.2005.04.015
Leung, J., Pang, A., Yuen, W. H., Kwong, Y. L., and Tse, E. W. (2007). Relationship of Expression of Aquaglyceroporin 9 with Arsenic Uptake and Sensitivity in Leukemia Cells. Blood 109 (2), 740–746. doi:10.1182/blood-2006-04-019588
Li, H., Kamiie, J., Morishita, Y., Yoshida, Y., Yaoita, E., Ishibashi, K., et al. (2005). Expression and Localization of Two Isoforms of AQP10 in Human Small Intestine. Biol. Cel 97 (11), 823–829. doi:10.1042/BC20040091
Liu, C., Zheng, Y., Xu, W., Wang, H., and Lin, N. (2014). Rhubarb Tannins Extract Inhibits the Expression of Aquaporins 2 and 3 in Magnesium Sulphate-Induced Diarrhoea Model. Biomed. Res. Int. 2014, 619465. doi:10.1155/2014/619465
Liu, Y. L., Matsuzaki, T., Nakazawa, T., Murata, S., Nakamura, N., Kondo, T., et al. (2007). Expression of Aquaporin 3 (AQP3) in normal and Neoplastic Lung Tissues. Hum. Pathol. 38 (1), 171–178. doi:10.1016/j.humpath.2006.07.015
Liu, Y., Promeneur, D., Rojek, A., Kumar, N., Frokiaer, J., Nielsen, S., et al. (2007). Aquaporin 9 Is the Major Pathway for Glycerol Uptake by Mouse Erythrocytes, with Implications for Malarial Virulence. Proc. Natl. Acad. Sci. U S A. 104 (30), 12560–12564. doi:10.1073/pnas.0705313104
Liu, Z., Shen, J., Carbrey, J. M., Mukhopadhyay, R., Agre, P., and Rosen, B. P. (2002). Arsenite Transport by Mammalian Aquaglyceroporins AQP7 and AQP9. Proc. Natl. Acad. Sci. U S A. 99 (9), 6053–6058. doi:10.1073/pnas.092131899
Ma, T., Song, Y., Yang, B., Gillespie, A., Carlson, E. J., Epstein, C. J., et al. (2000). Nephrogenic Diabetes Insipidus in Mice Lacking Aquaporin-3 Water Channels. Proc. Natl. Acad. Sci. U S A. 97 (8), 4386–4391. doi:10.1073/pnas.080499597
Macey, R. I., and Farmer, R. E. (1970). Inhibition of Water and Solute Permeability in Human Red Cells. Biochim. Biophys. Acta 211 (1), 104–106. doi:10.1016/0005-2736(70)90130-6
Madeira, A., Camps, M., Zorzano, A., Moura, T. F., and Soveral, G. (2013). Biophysical Assessment of Human Aquaporin-7 as a Water and Glycerol Channel in 3T3-L1 Adipocytes. PLoS One 8 (12), e83442. doi:10.1371/journal.pone.0083442
Madeira, A., Fernandez-Veledo, S., Camps, M., Zorzano, A., Moura, T. F., Ceperuelo-Mallafre, V., et al. (2014a). Human Aquaporin-11 Is a Water and Glycerol Channel and Localizes in the Vicinity of Lipid Droplets in Human Adipocytes. Obesity (Silver Spring) 22 (9), 2010–2017. doi:10.1002/oby.20792
Madeira, A., de Almeida, A., de Graaf, C., Camps, M., Zorzano, A., Moura, T. F., et al. (2014b). A Gold Coordination Compound as a Chemical Probe to Unravel Aquaporin-7 Function. Chembiochem 15 (10), 1487–1494. doi:10.1002/cbic.201402103
Madeira, A., Moura, T. F., and Soveral, G. (2015). Aquaglyceroporins: Implications in Adipose Biology and Obesity. Cel Mol Life Sci 72 (4), 759–771. doi:10.1007/s00018-014-1773-2
Madeira, A., Moura, T. F., and Soveral, G. (2016). Detecting Aquaporin Function and Regulation. Front. Chem. 4, 3. doi:10.3389/fchem.2016.00003
Maeda, N., Hibuse, T., and Funahashi, T. (2009). Role of Aquaporin-7 and Aquaporin-9 in Glycerol Metabolism; Involvement in Obesity. Handb Exp. Pharmacol. 190, 233–249. doi:10.1007/978-3-540-79885-9_12
Maeda, N. (2012). Implications of Aquaglyceroporins 7 and 9 in Glycerol Metabolism and Metabolic Syndrome. Mol. Aspects Med. 33 (5-6), 665–675. doi:10.1016/j.mam.2012.02.004
Maraldi, T., Vauzour, D., and Angeloni, C. (2014). Dietary Polyphenols and Their Effects on Cell Biochemistry and Pathophysiology 2013. Oxid Med. Cel Longev 2014, 576363. doi:10.1155/2014/576363
Marlar, S., Jensen, H. H., Login, F. H., and Nejsum, L. N. (2017). Aquaporin-3 in Cancer. Int. J. Mol. Sci. 18 (10), 2106. doi:10.3390/ijms18102106
Marrades, M. P., Milagro, F. I., Martinez, J. A., and Moreno-Aliaga, M. J. (2006). Differential Expression of Aquaporin 7 in Adipose Tissue of Lean and Obese High Fat Consumers. Biochem. Biophys. Res. Commun. 339 (3), 785–789. doi:10.1016/j.bbrc.2005.11.080
Martinez-Villaluenga, C., and Hernandez-Ledesma, B. (2020). Peptides for Health Benefits 2019. Int. J. Mol. Sci. 21 (7), 2543. doi:10.3390/ijms21072543
Martins, A. P., Ciancetta, A., de Almeida, A., Marrone, A., Re, N., Soveral, G., et al. (2013). Aquaporin Inhibition by Gold(III) Compounds: New Insights. ChemMedChem 8 (7), 1086–1092. doi:10.1002/cmdc.201300107
Martins, A. P., Marrone, A., Ciancetta, A., Galan Cobo, A., Echevarria, M., Moura, T. F., et al. (2012). Targeting Aquaporin Function: Potent Inhibition of Aquaglyceroporin-3 by a Gold-Based Compound. PLoS One 7 (5), e37435. doi:10.1371/journal.pone.0037435
Matsumura, K., Chang, B. H., Fujimiya, M., Chen, W., Kulkarni, R. N., Eguchi, Y., et al. (2007). Aquaporin 7 Is a Beta-Cell Protein and Regulator of Intraislet Glycerol Content and Glycerol Kinase Activity, Beta-Cell Mass, and Insulin Production and Secretion. Mol. Cel Biol 27 (17), 6026–6037. doi:10.1128/MCB.00384-07
Mehanna, E. T., Barakat, B. M., ElSayed, M. H., and Tawfik, M. K. (2018). An Optimized Dose of Raspberry Ketones Controls Hyperlipidemia and Insulin Resistance in Male Obese Rats: Effect on Adipose Tissue Expression of Adipocytokines and Aquaporin 7. Eur. J. Pharmacol. 832, 81–89. doi:10.1016/j.ejphar.2018.05.028
Mendez-Gimenez, L., Becerril, S., Camoes, S. P., da Silva, I. V., Rodrigues, C., Moncada, R., et al. (2017). Role of Aquaporin-7 in Ghrelin- and GLP-1-Induced Improvement of Pancreatic Beta-Cell Function after Sleeve Gastrectomy in Obese Rats. Int. J. Obes. (Lond) 41 (9), 1394–1402. doi:10.1038/ijo.2017.135
Mendez-Gimenez, L., Ezquerro, S., da Silva, I. V., Soveral, G., Fruhbeck, G., and Rodriguez, A. (2018). Pancreatic Aquaporin-7: A Novel Target for Anti-diabetic Drugs?. Front. Chem. 6, 99. doi:10.3389/fchem.2018.00099
Mola, M. G., Nicchia, G. P., Svelto, M., Spray, D. C., and Frigeri, A. (2009). Automated Cell-Based Assay for Screening of Aquaporin Inhibitors. Anal. Chem. 81 (19), 8219–8229. doi:10.1021/ac901526k
Mosca, A. F., de Almeida, A., Wragg, D., Martins, A. P., Sabir, F., Leoni, S., et al. (2018). Molecular Basis of Aquaporin-7 Permeability Regulation by pH. Cells 7 (11), 207. doi:10.3390/cells7110207
Muller, E. M., Hub, J. S., Grubmuller, H., and de Groot, B. L. (2008). Is TEA an Inhibitor for Human Aquaporin-1?. Pflugers Arch. 456 (4), 663–669. doi:10.1007/s00424-007-0422-0
Murata, K., Mitsuoka, K., Hirai, T., Walz, T., Agre, P., Heymann, J. B., et al. (2000). Structural Determinants of Water Permeation through Aquaporin-1. Nature 407 (6804), 599–605. doi:10.1038/35036519
Nave, M., Castro, R. E., Rodrigues, C. M., Casini, A., Soveral, G., and Gaspar, M. M. (2016). Nanoformulations of a Potent Copper-Based Aquaporin Inhibitor with Cytotoxic Effect against Cancer Cells. Nanomedicine (Lond) 11 (14), 1817–1830. doi:10.2217/nnm-2016-0086
Noda, Y., Sohara, E., Ohta, E., and Sasaki, S. (2010). Aquaporins in Kidney Pathophysiology. Nat. Rev. Nephrol. 6 (3), 168–178. doi:10.1038/nrneph.2009.231
Noronha, H., Agasse, A., Martins, A. P., Berny, M. C., Gomes, D., Zarrouk, O., et al. (2014). The Grape Aquaporin VvSIP1 Transports Water across the ER Membrane. J. Exp. Bot. 65 (4), 981–993. doi:10.1093/jxb/ert448
Papadopoulos, M. C., and Saadoun, S. (2015). Key Roles of Aquaporins in Tumor Biology. Biochim. Biophys. Acta 1848 (10 Pt B), 2576–2583. doi:10.1016/j.bbamem.2014.09.001
Pastor-Soler, N. M., Fisher, J. S., Sharpe, R., Hill, E., Van Hoek, A., Brown, D., et al. (2010). Aquaporin 9 Expression in the Developing Rat Epididymis Is Modulated by Steroid Hormones. Reproduction 139 (3), 613–621. doi:10.1530/REP-09-0284
Patsouris, D., Mandard, S., Voshol, P. J., Escher, P., Tan, N. S., Havekes, L. M., et al. (2004). PPARα Governs Glycerol Metabolism. J. Clin. Invest. 114 (1), 94–103. doi:10.1172/jci20468
Peng, R., Zhao, G. X., Li, J., Zhang, Y., Shen, X. Z., Wang, J. Y., et al. (2016). Auphen and Dibutyryl cAMP Suppress Growth of Hepatocellular Carcinoma by Regulating Expression of Aquaporins 3 and 9 In Vivo. World J. Gastroenterol. 22 (12), 3341–3354. doi:10.3748/wjg.v22.i12.3341
Pimpao, C., da Silva, I. V., Mosca, A. F., Pinho, J. O., Gaspar, M. M., Gumerova, N. I., et al. (2020). The Aquaporin-3-Inhibiting Potential of Polyoxotungstates. Int. J. Mol. Sci. 21 (7), 2467. doi:10.3390/ijms21072467
Pimpao, C., Wragg, D., Bonsignore, R., Aikman, B., Pedersen, P. A., Leoni, S., et al. (2021). Mechanisms of Irreversible Aquaporin-10 Inhibition by Organogold Compounds Studied by Combined Biophysical Methods and Atomistic Simulations. Metallomics 13 (9), mfab053. doi:10.1093/mtomcs/mfab053
Pinho, J. O., Amaral, J. D., Castro, R. E., Rodrigues, C. M., Casini, A., Soveral, G., et al. (2019). Copper Complex Nanoformulations Featuring Highly Promising Therapeutic Potential in Murine Melanoma Models. Nanomedicine (Lond) 14 (7), 835–850. doi:10.2217/nnm-2018-0388
Pinho, J. O., da Silva, I. V., Amaral, J. D., Rodrigues, C. M. P., Casini, A., Soveral, G., et al. (2021). Therapeutic Potential of a Copper Complex Loaded in pH-Sensitive Long Circulating Liposomes for colon Cancer Management. Int. J. Pharm. 599, 120463. doi:10.1016/j.ijpharm.2021.120463
Portincasa, P., and Calamita, G. (2019). Phytocompounds Modulating Aquaporins: Clinical Benefits Are Anticipated. Food Chem. 274, 642–650. doi:10.1016/j.foodchem.2018.09.029
Prata, C., Hrelia, S., and Fiorentini, D. (2019). Peroxiporins in Cancer. Int. J. Mol. Sci. 20 (6), 1371. doi:10.3390/ijms20061371
Preston, G. M., Carroll, T. P., Guggino, W. B., and Agre, P. (1992). Appearance of Water Channels in Xenopus Oocytes Expressing Red Cell CHIP28 Protein. Science 256 (5055), 385–387. doi:10.1126/science.256.5055.385
Qiu, J., Zhang, Y., Chen, H., and Guo, Z. (2018). MicroRNA-488 Inhibits Proliferation, Invasion and EMT in Osteosarcoma Cell Lines by Targeting Aquaporin 3. Int. J. Oncol. 53 (4), 1493–1504. doi:10.3892/ijo.2018.4483
Ren, N., and Wang, M. (2018). microRNA-212-induced protection of the Heart against Myocardial Infarction Occurs via the Interplay between AQP9 and PI3K/Akt Signaling Pathway. Exp. Cel Res 370 (2), 531–541. doi:10.1016/j.yexcr.2018.07.018
Rodrigues, C., Mosca, A. F., Martins, A. P., Nobre, T., Prista, C., Antunes, F., et al. (2016). Rat Aquaporin-5 Is pH-Gated Induced by Phosphorylation and Is Implicated in Oxidative Stress. Int. J. Mol. Sci. 17 (12), 2090. doi:10.3390/ijms17122090
Rodrigues, C., Pimpao, C., Mosca, A. F., Coxixo, A. S., Lopes, D., da Silva, I. V., et al. (2019). Human Aquaporin-5 Facilitates Hydrogen Peroxide Permeation Affecting Adaption to Oxidative Stress and Cancer Cell Migration. Cancers (Basel) 11 (7), 932. doi:10.3390/cancers11070932
Rodriguez, A., Catalan, V., Gomez-Ambrosi, J., and Fruhbeck, G. (2011). Aquaglyceroporins Serve as Metabolic Gateways in Adiposity and Insulin Resistance Control. Cell Cycle 10 (10), 1548–1556. doi:10.4161/cc.10.10.15672
Rodriguez, A., Catalan, V., Gomez-Ambrosi, J., and Fruhbeck, G. (2006). Role of Aquaporin-7 in the Pathophysiological Control of Fat Accumulation in Mice. FEBS Lett. 580 (20), 4771–4776. doi:10.1016/j.febslet.2006.07.080
Rodriguez, A., Gena, P., Mendez-Gimenez, L., Rosito, A., Valenti, V., Rotellar, F., et al. (2014). Reduced Hepatic Aquaporin-9 and Glycerol Permeability Are Related to Insulin Resistance in Non-alcoholic Fatty Liver Disease. Int. J. Obes. (Lond) 38 (9), 1213–1220. doi:10.1038/ijo.2013.234
Rodriguez, A., Gomez-Ambrosi, J., Catalan, V., Ezquerro, S., Mendez-Gimenez, L., Becerril, S., et al. (2016). Guanylin and Uroguanylin Stimulate Lipolysis in Human Visceral Adipocytes. Int. J. Obes. (Lond) 40 (9), 1405–1415. doi:10.1038/ijo.2016.66
Rodriguez, A., Gomez-Ambrosi, J., Catalan, V., Gil, M. J., Becerril, S., Sainz, N., et al. (2009). Acylated and Desacyl Ghrelin Stimulate Lipid Accumulation in Human Visceral Adipocytes. Int. J. Obes. (Lond) 33 (5), 541–552. doi:10.1038/ijo.2009.40
Rodriguez, A., Moreno, N. R., Balaguer, I., Mendez-Gimenez, L., Becerril, S., Catalan, V., et al. (2015a). Leptin Administration Restores the Altered Adipose and Hepatic Expression of Aquaglyceroporins Improving the Non-alcoholic Fatty Liver of Ob/ob Mice. Sci. Rep. 5, 12067. doi:10.1038/srep12067
Rodriguez, A., Marinelli, R. A., Tesse, A., Fruhbeck, G., and Calamita, G. (2015b). Sexual Dimorphism of Adipose and Hepatic Aquaglyceroporins in Health and Metabolic Disorders. Front. Endocrinol. (Lausanne) 6, 171. doi:10.3389/fendo.2015.00171
Rojek, A. M., Skowronski, M. T., Fuchtbauer, E. M., Fuchtbauer, A. C., Fenton, R. A., Agre, P., et al. (2007). Defective Glycerol Metabolism in Aquaporin 9 (AQP9) Knockout Mice. Proc. Natl. Acad. Sci. U S A. 104 (9), 3609–3614. doi:10.1073/pnas.0610894104
Rojek, A., Praetorius, J., Frokiaer, J., Nielsen, S., and Fenton, R. A. (2008). A Current View of the Mammalian Aquaglyceroporins. Annu. Rev. Physiol. 70, 301–327. doi:10.1146/annurev.physiol.70.113006.100452
Roux, B., and Schulten, K. (2004). Computational Studies of Membrane Channels. Structure 12 (8), 1343–1351. doi:10.1016/j.str.2004.06.013
Rubenwolf, P. C., Georgopoulos, N. T., Kirkwood, L. A., Baker, S. C., and Southgate, J. (2012). Aquaporin Expression Contributes to Human Transurothelial Permeability In Vitro and Is Modulated by NaCl. PLoS One 7 (9), e45339. doi:10.1371/journal.pone.0045339
Sabir, F., Gomes, S., Loureiro-Dias, M. C., Soveral, G., and Prista, C. (2020). Molecular and Functional Characterization of Grapevine NIPs through Heterologous Expression in Aqy-Null Saccharomyces cerevisiae. Int. J. Mol. Sci. 21 (2), 663. doi:10.3390/ijms21020663
Salleh, N., Mokhtar, H. M., Kassim, N. M., and Giribabu, N. (2015). Testosterone Induces Increase in Aquaporin (AQP)-1, 5, and 7 Expressions in the Uteri of Ovariectomized Rats. J. Membr. Biol. 248 (6), 1097–1105. doi:10.1007/s00232-015-9823-8
Savage, D. F., and Stroud, R. M. (2007). Structural Basis of Aquaporin Inhibition by Mercury. J. Mol. Biol. 368 (3), 607–617. doi:10.1016/j.jmb.2007.02.070
Serna, A., Galan-Cobo, A., Rodrigues, C., Sanchez-Gomar, I., Toledo-Aral, J. J., Moura, T. F., et al. (2014). Functional Inhibition of Aquaporin-3 with a Gold-Based Compound Induces Blockage of Cell Proliferation. J. Cel Physiol 229 (11), 1787–1801. doi:10.1002/jcp.24632
Skowronski, M. T., Lebeck, J., Rojek, A., Praetorius, J., Fuchtbauer, E. M., Frokiaer, J., et al. (2007). AQP7 Is Localized in Capillaries of Adipose Tissue, Cardiac and Striated Muscle: Implications in Glycerol Metabolism. Am. J. Physiol. Ren. Physiol 292 (3), F956–F965. doi:10.1152/ajprenal.00314.2006
Smart, O. S., Neduvelil, J. G., Wang, X., Wallace, B. A., and Sansom, M. S. P. (1996). HOLE: A Program for the Analysis of the Pore Dimensions of Ion Channel Structural Models. J. Mol. Graphics 14 (6), 354–360. doi:10.1016/s0263-7855(97)00009-x
Sohara, E., Rai, T., Miyazaki, J., Verkman, A. S., Sasaki, S., and Uchida, S. (2005). Defective Water and Glycerol Transport in the Proximal Tubules of AQP7 Knockout Mice. Am. J. Physiol. Ren. Physiol 289 (6), F1195–F1200. doi:10.1152/ajprenal.00133.2005
Sonntag, Y., Gena, P., Maggio, A., Singh, T., Artner, I., Oklinski, M. K., et al. (2019). Identification and Characterization of Potent and Selective Aquaporin-3 and Aquaporin-7 Inhibitors. J. Biol. Chem. 294 (18), 7377–7387. doi:10.1074/jbc.RA118.006083
Soveral, G., and Casini, A. (2017). Aquaporin Modulators: a Patent Review (2010-2015). Expert Opin. Ther. Pat 27 (1), 49–62. doi:10.1080/13543776.2017.1236085
Soveral, G., Madeira, A., Loureiro-Dias, M. C., and Moura, T. F. (2008). Membrane Tension Regulates Water Transport in Yeast. Biochim. Biophys. Acta 1778 (11), 2573–2579. doi:10.1016/j.bbamem.2008.07.018
Soveral, G., Madeira, A., Loureiro-Dias, M. C., and Moura, T. F. (2007). Water Transport in Intact Yeast Cells as Assessed by Fluorescence Self-Quenching. Appl. Environ. Microbiol. 73 (7), 2341–2343. doi:10.1128/AEM.02519-06
Soveral, G., Nielsen, S., and Casini, A. (2016). Aquaporins in Health and Disease: New Molecular Targets for Drug Discovery. Boca Raton, FL: CRC Press, Taylor & Francis Group.
Spinello, A., de Almeida, A., Casini, A., and Barone, G. (2016). The Inhibition of Glycerol Permeation through Aquaglyceroporin-3 Induced by Mercury(II): A Molecular Dynamics Study. J. Inorg. Biochem. 160, 78–84. doi:10.1016/j.jinorgbio.2015.11.027
Su, W., Cao, R., Zhang, X. Y., and Guan, Y. (2020). Aquaporins in the Kidney: Physiology and Pathophysiology. Am. J. Physiol. Ren. Physiol 318 (1), F193–F203. doi:10.1152/ajprenal.00304.2019
Su, Z., Zhi, X., Zhang, Q., Yang, L., Xu, H., and Xu, Z. (2016). LncRNA H19 Functions as a Competing Endogenous RNA to Regulate AQP3 Expression by Sponging miR-874 in the Intestinal Barrier. FEBS Lett. 590 (9), 1354–1364. doi:10.1002/1873-3468.12171
Tan, G., Sun, S. Q., and Yuan, D. L. (2008). Expression of the Water Channel Protein Aquaporin-9 in Human Astrocytic Tumours: Correlation with Pathological Grade. J. Int. Med. Res. 36 (4), 777–782. doi:10.1177/147323000803600420
Tanaka, M., Inase, N., Fushimi, K., Ishibashi, K., Ichioka, M., Sasaki, S., et al. (1997). Induction of Aquaporin 3 by Corticosteroid in a Human Airway Epithelial Cell Line. Am. J. Physiol. 273 (5), L1090–L1095. doi:10.1152/ajplung.1997.273.5.L1090
Tang, S. C., Tang, L. C., Liu, C. H., Liao, P. Y., Lai, J. C., and Yang, J. H. (2019). Glycolic Acid Attenuates UVB-Induced Aquaporin-3, Matrix Metalloproteinase-9 Expression, and Collagen Degradation in Keratinocytes and Mouse Skin. Biochem. J. 476 (10), 1387–1400. doi:10.1042/BCJ20180974
Tesse, A., Gena, P., Rutzler, M., and Calamita, G. (2021). Ablation of Aquaporin-9 Ameliorates the Systemic Inflammatory Response of LPS-Induced Endotoxic Shock in Mouse. Cells 10 (2), 435. doi:10.3390/cells10020435
Tesse, A., Grossini, E., Tamma, G., Brenner, C., Portincasa, P., Marinelli, R. A., et al. (2018). Aquaporins as Targets of Dietary Bioactive Phytocompounds. Front. Mol. Biosci. 5, 30. doi:10.3389/fmolb.2018.00030
Thiagarajah, J. R., Chang, J., Goettel, J. A., Verkman, A. S., and Lencer, W. I. (2017). Aquaporin-3 Mediates Hydrogen Peroxide-dependent Responses to Environmental Stress in Colonic Epithelia. Proc. Natl. Acad. Sci. U S A. 114 (3), 568–573. doi:10.1073/pnas.1612921114
Thiagarajah, J. R., Zhao, D., and Verkman, A. S. (2007). Impaired Enterocyte Proliferation in Aquaporin-3 Deficiency in Mouse Models of Colitis. Gut 56 (11), 1529–1535. doi:10.1136/gut.2006.104620
Tinoco, A. D., and Saghatelian, A. (2011). Investigating Endogenous Peptides and Peptidases Using Peptidomics. Biochemistry 50 (35), 7447–7461. doi:10.1021/bi200417k
Tradtrantip, L., Jin, B. J., Yao, X., Anderson, M. O., and Verkman, A. S. (2017). Aquaporin-Targeted Therapeutics: State-Of-The-Field. Adv. Exp. Med. Biol. 969, 239–250. doi:10.1007/978-94-024-1057-0_16
Tsukaguchi, H., Weremowicz, S., Morton, C. C., and Hediger, M. A. (1999). Functional and Molecular Characterization of the Human Neutral Solute Channel Aquaporin-9. Am. J. Physiol. 277 (5), F685–F696. doi:10.1152/ajprenal.1999.277.5.F685
Verkman, A. S., Anderson, M. O., and Papadopoulos, M. C. (2014). Aquaporins: Important but Elusive Drug Targets. Nat. Rev. Drug Discov. 13 (4), 259–277. doi:10.1038/nrd4226
Verkman, A. S. (2012). Aquaporins in Clinical Medicine. Annu. Rev. Med. 63, 303–316. doi:10.1146/annurev-med-043010-193843
Verkman, A. S. (2009). Aquaporins: Translating Bench Research to Human Disease. J. Exp. Biol. 212 (Pt 11), 1707–1715. doi:10.1242/jeb.024125
Verkman, A. S. (2008a). Dissecting the Roles of Aquaporins in Renal Pathophysiology Using Transgenic Mice. Semin. Nephrol. 28 (3), 217–226. doi:10.1016/j.semnephrol.2008.03.004
Verkman, A. S. (2008b). Mammalian Aquaporins: Diverse Physiological Roles and Potential Clinical Significance. Expert Rev. Mol. Med. 10, e13. doi:10.1017/S1462399408000690
Verkman, A. S., Hara-Chikuma, M., and Papadopoulos, M. C. (2008). Aquaporins--new Players in Cancer Biology. J. Mol. Med. (Berl) 86 (5), 523–529. doi:10.1007/s00109-008-0303-9
Wacker, S. J., Aponte-Santamaria, C., Kjellbom, P., Nielsen, S., de Groot, B. L., and Rutzler, M. (2013). The Identification of Novel, High Affinity AQP9 Inhibitors in an Intracellular Binding Site. Mol. Membr. Biol. 30 (3), 246–260. doi:10.3109/09687688.2013.773095
Wang, B. F., Cui, Z. W., Zhong, Z. H., Sun, Y. H., Sun, Q. F., Yang, G. Y., et al. (2015). Curcumin Attenuates Brain Edema in Mice with Intracerebral Hemorrhage through Inhibition of AQP4 and AQP9 Expression. Acta Pharmacol. Sin 36 (8), 939–948. doi:10.1038/aps.2015.47
Wang, C., Lv, Z. L., Kang, Y. J., Xiang, T. X., Wang, P. L., and Jiang, Z. (2013). Aquaporin-9 Downregulation Prevents Steatosis in Oleic Acid-Induced Non-alcoholic Fatty Liver Disease Cell Models. Int. J. Mol. Med. 32 (5), 1159–1165. doi:10.3892/ijmm.2013.1502
Wang, S., Wu, Y., Yang, S., Liu, X., Lu, Y., Liu, F., et al. (2020). miR-874 Directly Targets AQP3 to Inhibit Cell Proliferation, Mobility and EMT in Non-small Cell Lung Cancer. Thorac. Cancer 11 (6), 1550–1558. doi:10.1111/1759-7714.13428
Warth, A., Mittelbronn, M., Hulper, P., Erdlenbruch, B., and Wolburg, H. (2007). Expression of the Water Channel Protein Aquaporin-9 in Malignant Brain Tumors. Appl. Immunohistochem. Mol. Morphol. 15 (2), 193–198. doi:10.1097/01.pai.0000213110.05108.e9
Wawrzkiewicz-Jalowiecka, A., Lalik, A., and Soveral, G. (2021). Recent Update on the Molecular Mechanisms of Gonadal Steroids Action in Adipose Tissue. Int. J. Mol. Sci. 22 (10), 5226. doi:10.3390/ijms22105226
Wragg, D., de Almeida, A., Casini, A., and Leoni, S. (2019). Unveiling the Mechanisms of Aquaglyceroporin-3 Water and Glycerol Permeation by Metadynamics. Chemistry 25 (37), 8713–8718. doi:10.1002/chem.201902121
Wragg, D., Leoni, S., and Casini, A. (2020). Aquaporin-driven Hydrogen Peroxide Transport: a Case of Molecular Mimicry?. RSC Chem. Biol. 1 (5), 390–394. doi:10.1039/d0cb00160k
Wu, B., and Beitz, E. (2007). Aquaporins with Selectivity for Unconventional Permeants. Cel Mol Life Sci 64 (18), 2413–2421. doi:10.1007/s00018-007-7163-2
Wu, J., Gan, Y., Luo, H., Xu, N., Chen, L., Li, M., et al. (2021). Beta-Patchoulene Ameliorates Water Transport and the Mucus Barrier in 5-Fluorouracil-Induced Intestinal Mucositis Rats via the cAMP/PKA/CREB Signaling Pathway. Front. Pharmacol. 12, 689491. doi:10.3389/fphar.2021.689491
Wu, J., Wang, C., and Ding, H. (2020). LncRNA MALAT1 Promotes Neuropathic Pain Progression through the miR1545p/AQP9 axis in CCI Rat Models. Mol. Med. Rep. 21 (1), 291–303. doi:10.3892/mmr.2019.10829
Wu, N. L., Fang, J. Y., Chen, M., Wu, C. J., Huang, C. C., and Hung, C. F. (2011). Chrysin Protects Epidermal Keratinocytes from UVA- and UVB-Induced Damage. J. Agric. Food Chem. 59 (15), 8391–8400. doi:10.1021/jf200931t
Wu, Z., Uchi, H., Morino-Koga, S., Shi, W., and Furue, M. (2014). Resveratrol Inhibition of Human Keratinocyte Proliferation via SIRT1/ARNT/ERK Dependent Downregulation of Aquaporin 3. J. Dermatol. Sci. 75 (1), 16–23. doi:10.1016/j.jdermsci.2014.03.004
Yadav, D. K., Kumar, S., Choi, E. H., Chaudhary, S., and Kim, M. H. (2020). Computational Modeling on Aquaporin-3 as Skin Cancer Target: A Virtual Screening Study. Front. Chem. 8, 250. doi:10.3389/fchem.2020.00250
Yamada, Y., Arai, T., Kato, M., Kojima, S., Sakamoto, S., Komiya, A., et al. (2019). Role of Pre-miR-532 (miR-532-5p and miR-532-3p) in Regulation of Gene Expression and Molecular Pathogenesis in Renal Cell Carcinoma. Am. J. Clin. Exp. Urol. 7 (1), 11–30.
Yamamoto, N., Sobue, K., Fujita, M., Katsuya, H., and Asai, K. (2002). Differential Regulation of Aquaporin-5 and -9 Expression in Astrocytes by Protein Kinase A. Mol. Brain Res. 104 (1), 96–102. doi:10.1016/s0169-328x(02)00322-4
Yang, J. H., Yan, C. X., Chen, X. J., and Zhu, Y. S. (2011). Expression of Aquaglyceroporins in Epithelial Ovarian Tumours and Their Clinical Significance. J. Int. Med. Res. 39 (3), 702–711. doi:10.1177/147323001103900302
Yin, J., Liang, Y., Wang, D., Yan, Z., Yin, H., Wu, D., et al. (2018). Naringenin Induces Laxative Effects by Upregulating the Expression Levels of C-Kit and SCF, as Well as Those of Aquaporin 3 in Mice with Loperamide-Induced Constipation. Int. J. Mol. Med. 41 (2), 649–658. doi:10.3892/ijmm.2017.3301
Yu, S., Li, L. H., Lee, C. H., Jeyakannu, P., Wang, J. J., and Hong, C. H. (2021). Arsenic Leads to Autophagy of Keratinocytes by Increasing Aquaporin 3 Expression. Sci. Rep. 11 (1), 17523. doi:10.1038/s41598-021-96822-6
Zardoya, R. (2005). Phylogeny and Evolution of the Major Intrinsic Protein Family. Biol. Cel 97 (6), 397–414. doi:10.1042/BC20040134
Zelenina, M., Bondar, A. A., Zelenin, S., and Aperia, A. (2003). Nickel and Extracellular Acidification Inhibit the Water Permeability of Human Aquaporin-3 in Lung Epithelial Cells. J. Biol. Chem. 278 (32), 30037–30043. doi:10.1074/jbc.M302206200
Zelenina, M., and Brismar, H. (2000). Osmotic Water Permeability Measurements Using Confocal Laser Scanning Microscopy. Eur. Biophys. J. 29 (3), 165–171. doi:10.1007/pl00006645
Zelenina, M., Tritto, S., Bondar, A. A., Zelenin, S., and Aperia, A. (2004). Copper Inhibits the Water and Glycerol Permeability of Aquaporin-3. J. Biol. Chem. 279 (50), 51939–51943. doi:10.1074/jbc.M407645200
Zhang, M., Li, T., Zhu, J., Tuo, B., and Liu, X. (2020). Physiological and Pathophysiological Role of Ion Channels and Transporters in the Colorectum and Colorectal Cancer. J. Cel Mol Med 24 (17), 9486–9494. doi:10.1111/jcmm.15600
Zhang, W. G., Li, C. F., Liu, M., Chen, X. F., Shuai, K., Kong, X., et al. (2016). Aquaporin 9 Is Down-Regulated in Hepatocellular Carcinoma and its Over-expression Suppresses Hepatoma Cell Invasion through Inhibiting Epithelial-To-Mesenchymal Transition. Cancer Lett. 378 (2), 111–119. doi:10.1016/j.canlet.2016.05.021
Zhao, G., Li, J., Wang, J., Shen, X., and Sun, J. (2014). Aquaporin 3 and 8 Are Down-Regulated in TNBS-Induced Rat Colitis. Biochem. Biophys. Res. Commun. 443 (1), 161–166. doi:10.1016/j.bbrc.2013.11.067
Keywords: aquaporin, glycerol permeability, functional assays, computational methods, regulation, expression modulators, inhibitors
Citation: Pimpão C, Wragg D, da Silva IV, Casini A and Soveral G (2022) Aquaglyceroporin Modulators as Emergent Pharmacological Molecules for Human Diseases. Front. Mol. Biosci. 9:845237. doi: 10.3389/fmolb.2022.845237
Received: 29 December 2021; Accepted: 13 January 2022;
Published: 03 February 2022.
Edited by:
Cesare Indiveri, University of Calabria, ItalyReviewed by:
Tiziano Verri, University of Salento, ItalyUmberto Laforenza, University of Pavia, Italy
Amaia Rodríguez, University of Navarra, Spain
Copyright © 2022 Pimpão, Wragg, da Silva, Casini and Soveral. This is an open-access article distributed under the terms of the Creative Commons Attribution License (CC BY). The use, distribution or reproduction in other forums is permitted, provided the original author(s) and the copyright owner(s) are credited and that the original publication in this journal is cited, in accordance with accepted academic practice. No use, distribution or reproduction is permitted which does not comply with these terms.
*Correspondence: Angela Casini, YW5nZWxhLmNhc2luaUB0dW0uZGU=; Graça Soveral, Z3NvdmVyYWxAZmYudWxpc2JvYS5wdA==