- National Center for Biotechnology Information, National Library of Medicine, National Institutes of Health, Bethesda, MD, United States
Prokaryotic genomes are replete with mobile genetic elements (MGE) that span a continuum of replication autonomy. On numerous occasions during microbial evolution, diverse MGE lose their autonomy altogether but, rather than being quickly purged from the host genome, assume a new function that benefits the host, rendering the immobilized MGE subject to purifying selection, and resulting in its vertical inheritance. This mini-review highlights the diversity of the repurposed (exapted) MGE as well as the plethora of cellular functions that they perform. The principal contribution of the exaptation of MGE and their components is to the prokaryotic functional systems involved in biological conflicts, and in particular, defense against viruses and other MGE. This evolutionary entanglement between MGE and defense systems appears to stem both from mechanistic similarities and from similar evolutionary predicaments whereby both MGEs and defense systems tend to incur fitness costs to the hosts and thereby evolve mechanisms for survival including horizontal mobility, causing host addiction, and exaptation for functions beneficial to the host. The examples discussed demonstrate that the identity of an MGE, overall mobility and relationship with the host cell (mutualistic, symbiotic, commensal, or parasitic) are all factors that affect exaptation.
Introduction
Coevolution of mobile genetic elements (MGEs) and cellular organisms spans billions of years and is thought to have spurred innumerable evolutionary innovations (Werren, 2011; Koonin, 2016). Diverse MGEs that jointly comprise a vast mobilome are associated with essentially all known cellular organisms, with the possible exception of some intracellular parasitic and symbiotic bacteria (Frost et al., 2005; Iranzo et al., 2016; Carr et al., 2021). The relationship between MGEs and their cellular hosts spans a continuum, ranging from mutualistic to parasitic, changing between different MGE-host pairs as well as within the same pair over time (Jalasvuori and Koonin, 2015). One form of this dynamic relationship can be described as antagonistic coevolution, where an incessant arms-race takes place between the MGE and the host, driving the evolution of ornate defense and counter-defense systems encoded by both parties (Duggal and Emerman, 2012; Feschotte and Gilbert, 2012; Bernheim and Sorek, 2020). Intensive investigation of defense and counter-defense systems, such as CRISPR-Cas, uncovered back-and-forth shuttling of the system’s components between MGEs and their hosts over evolutionary time (Koonin et al., 2019). The evolutionary entanglement between MGEs and hosts is not restricted to defense systems alone, but to our knowledge, the full extent of such exchanges has not been systematically reviewed.
One key aspect of the evolutionary exchange between MGEs and cells is exaptation. Exaptation refers to the recruitment, driven by natural selection, of a biological entity for a new role unrelated to the original one with respect to the biological function, while exploiting the mechanistic features of the recruited entity (Gould and Vrba, 1982; Gould, 1997). We use this term instead of the more common “domestication” to emphasize the functional shifts that occur upon recruitment of MGEs or their components for cellular functions (Koonin and Krupovic, 2018; Koonin and Makarova, 2022). For example, a nuclease originally involved in transposition of a distinct variety of IS200/IS605-like transposons evolved into Cas9, the effector of type II CRISPR-Cas adaptive immunity (Kapitonov et al., 2016; Altae-Tran et al., 2021), whereas the transposase of another type of transposons gave rise to Cas1, the integrase involved in spacer acquisition by CRISPR-Cas systems (Krupovic et al., 2014). Exaptation can occur at different “depths”, ranging from the recruitment of an entire MGE for a new role to repurposing of a single component of an MGE (Koonin and Krupovic, 2018). Exaptation of MGE genes is linked to horizontal gene transfer (HGT), the major evolutionary force in prokaryotes (Doolittle, 1999; Koonin et al., 2001). Due to their high horizontal mobility, MGE double as vehicles for HGT. Indeed, some MGE carry large repertoires of diverse “cargo” genes, some of which increase the fitness of the recipient host cell and can even make the hosts “addicted” to the respective MGE (Nicolas et al., 2015; Hülter et al., 2017; Benler et al., 2021). An obvious example includes horizontally transferred antibiotic resistance genes that become essential in the presence of antibiotics (Liebert et al., 1999). Antibiotic resistance or other cargo genes are determinants of MGE-host symbioses that in many cases are mutualistic. However, in this review, we focus on exaptations of genes directly involved in MGE mobility, replication or persistence as well as exaptation of entire MGEs.
Main
Exaptation of Mobile Genetic Elements and Their Components for Host Defense Functions
The potential for MGE to replicate at the expense of their hosts necessitates the evolution of systems that can discern self from non-self and protect the host from the deleterious effects of the parasites by curtailing their reproduction (Rimer et al., 2014). Defense systems can be partitioned into two discrete classes, based on whether they respond to fixed non-self patterns (innate immunity) or memorize variable non-self patterns and mount a response against specific parasites (adaptive immunity). The evolution of both types of immune systems and the parasites which they defend against are intrinsically entangled through exaptations (Koonin et al., 2017; Koonin and Makarova, 2017; Koonin et al., 2019)
The adaptive arm of prokaryotic immunity is effectuated by CRISPR-Cas, which is the evolutionary product of a constellation of mobile genetic elements. The memory function of CRISPR-Cas is achieved via Cas1 and Cas2, which jointly excise segments of nucleic acid from foreign genetic elements and insert the segments into the CRISPR repeat arrays in the host chromosome (Nuñez et al., 2014; Amitai and Sorek, 2016). Phylogenomic study of Cas1 uncovered ancestral homologs encoded by a distinct family of MGE, self-synthesizing transposons dubbed casposons (Krupovic et al., 2014; Krupovic et al., 2017). The evolutionary relationship between Cas1 and the homologous transposases of the casposons (dubbed ‘casposases’) is matched by extensive biochemical similarities between the two enzymes (Hickman and Dyda, 2015; Béguin et al., 2016; Hickman et al., 2020). Similarly, phylogenetic analysis of Cas2 demonstrates an evolutionary connection to mRNA-degrading toxins of the VapD family (Koonin and Makarova, 2013). The VapD ribonucleases are toxin components of toxin-antitoxin (TA) modules, which themselves exhibit properties of selfish elements and can be considered a type of MGE that typically piggy-back on plasmids (Jalasvuori and Koonin, 2015; Jurėnas et al., 2022). In the CRISPR adaptation complex, Cas2 performs a structural, scaffolding role, whereas the function of the nuclease activity, which is retained by most but not all Cas2 proteins, remain unknown (Amitai and Sorek, 2016; Sternberg et al., 2016). Thus, a transposase and a toxin apparently were the evolutionary grist for the memory capability of CRISPR-Cas, the defining feature of adaptive immunity.
Numerous type III CRISPR-Cas systems include a reverse transcriptase (RT) that is typically fused to Cas1 and enables adaptation by capturing spacers directly from RNA, either transcripts of DNA genomes of MGE, or possibly, RNA viruses (Koonin and Makarova, 2017; Silas et al., 2017). This CRISPR-associated RT is most closely related to the RT of prokaryotic retrotransposons (group II self-splicing introns), from which it was apparently recruited for the role in CRISPR adaptation (Koonin and Makarova, 2017; Silas et al., 2017). Thus, in these systems, the RNA-memorizing capability of CRISPR-Cas was endowed by the exaptation of a third mobile genetic element.
The effector complexes of CRISPR-Cas systems are highly diverse, apparently, owing to their capture from distinct mobile genetic elements. The architecture of the effector module distinguishes the two classes of CRISPR-Cas. The effector is either a multisubunit complex composed of several Cas proteins (class 1) or a single, large, multidomain protein (class 2) (Makarova et al., 2015; Makarova et al., 2020). The origins of class 1 effector modules remain murky. Nevertheless, comparative genomic analysis points to a likely ancestral relationship with a distinct variety of Abortive Infection (ABI) modules (Burroughs et al., 2015; Koonin and Makarova, 2019; Burroughs and Aravind, 2020). The ABI modules are a type of toxin-antitoxin systems that, after being activated by virus infection, induce cell dormancy or death, via a variety of mechanisms, of which the most common one is indiscriminate RNA cleavage, thus preventing virus reproduction and spread (Harms et al., 2018; Fraikin et al., 2020). Type III CRISPR-Cas systems possess the same functionality whereby the non-specific “altruistic” response is triggered by the specific target recognition. The inferred origin of type III effector modules from ABI systems implies that Class 1 CRISPR effectors started out as innate immunity systems that became the executive component of adaptive immunity though the merger with the adaptation module derived from casposons and TA.
The class 2 effectors have a completely different evolutionary history, being derived from nucleases encoded by MGE on multiple, independent occasions (Shmakov et al., 2015; Faure et al., 2019). One particular superfamily of transposable elements, the IS200/IS605, donated the nucleases (IscB and TnpB, respectively) that gave rise to Cas9 and Cas12, the effectors of type II and type V CRISPR-Cas systems (Kapitonov et al., 2016; Altae-Tran et al., 2021). The link between IscB and Cas9 is apparent from the shared, unique domain architecture of these proteins, in which an HNH nuclease domain is inserted with the RuvC-like nuclease. In contrast, Cas12 proteins only contain the RuvC-like nuclease domain, similarly to TnpB. The ancestral status of the transposon-encoded nucleases with respect to the CRISPR effectors is supported by general considerations, namely, the small size and compactness of IscB and TnpB, and the simple organization of the transposons themselves compared to the CRISPR-Cas systems (Koonin and Makarova, 2022). More importantly in the phylogenetic trees of the two nuclease families, Cas9 forms a single clade embedded amongst transposon-encoded IscBs (Altae-Tran et al., 2021), whereas different Cas12 variants comprise several such clades in the TnpB (Faure et al., 2019). Thus, while Cas9 was derived from a transposon-encoded nuclease IscB in a single evolutionary event (Kapitonov et al., 2016; Altae-Tran et al., 2021), Cas12 apparently evolved from transposon nucleases of the TnpB family on multiple, independent occasions (Faure et al., 2019; Altae-Tran et al., 2021). Similarly to Cas9 and Cas12, the nucleases of IS200/IS605-like transposons form a complex with a distinct guide RNA encoded within the same transposon (Altae-Tran et al., 2021). However, in these elements, the guide RNAs are not responsible for cleaving foreign DNA, but rather might direct the transposons to specific integration sites in the host chromosomal DNA; the details of the functions of these nucleases in transposons remain to be studied (Altae-Tran et al., 2021; Karvelis et al., 2021). Collectively, these observations indicate that most if not all major components of CRISPR-Cas systems originated via exaptation of MGE genes.
Prokaryotes harbor a multifarious armament of innate immune systems that defend against parasites, and many of these defense machineries were captured from MGE. In particular, bacteriophage (phage) superinfection exclusion systems serve as a rich depot from which prokaryotes can arm themselves for defense. Superinfection exclusion refers to the ability of a primary infecting phage to prevent a subsequent infection by another phage (Gentile et al., 2019; Owen et al., 2021), a form of inter-MGE competition. For example, phage P2 carries three genes, fun, tin, and old, which endow its host with immunity against phage T5, T-even phages, and lambdoid phages, respectively (Haggård-Ljungquist et al., 1989; Mosig et al., 1997; Odegrip et al., 2006) (Figure 1A). In both phage and bacterial genomes, the fun gene is flanked by inverted repeats that enable site-specific recombination and exchange of fun between bacteria and P2-like phages (Nilsson et al., 2004). Thus, through site-specific recombination, bacteria directly capture a superinfection exclusion gene from one phage that provides immunity to infection by other phages. Similarly, OLD is the archetypical member of a family of ABC-ATPases fused to a TOPRIM nuclease domain that is found in diverse defense contexts (Aravind et al., 1998; Krishnan et al., 2020). In combination with a UvrD-family helicase and RNAseH-family exonuclease, OLD is part of a widespread system that provides cells with innate immunity against several distinct bacteriophages (Doron et al., 2018; Cheng et al., 2021). The old and fun superinfection exclusion genes are just two examples of innate immune systems found in prokaryotic genomes associated with mobile genetic elements (Makarova et al., 2013), reflecting a broad evolutionary pattern of continuous back and forth gene shuffling.
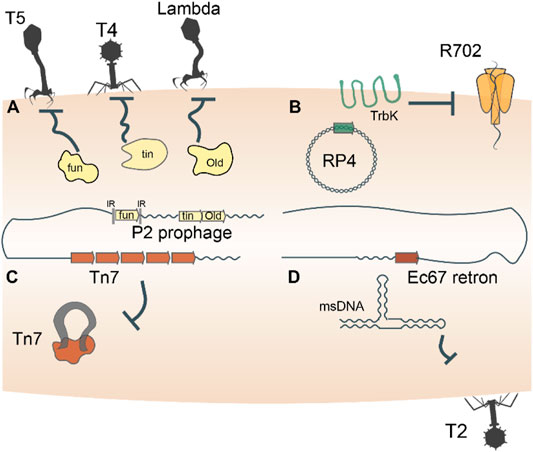
FIGURE 1. Entire mobile genetic elements double as defense systems. Autonomous MGEs, such as bacteriophages, plasmids, transposons and retrons, encode genes that defend the host cells against invasion by related or unrelated MGEs (A–D). For example, trbK encoded by the broad host range plasmid RP4 excludes plasmid R702. Such defense-related genes are frequently shuttled back and forth between MGEs and their host cells, such as, for example, the Old family nuclease encoded by phage P2.
Beyond the possession of genes dedicated to defense, mobile genetic elements themselves double as immune systems. As discussed above, bacteriophages furnish their hosts with immunity against secondary infections by related or unrelated phages through diverse mechanisms (Figure 1A). Plasmid incompatibility groups also can be viewed through the lens of inter-MGE competition, whereby the presence of one plasmid in a host cell precludes replication of another plasmid of the same incompatibility group (Cooper and Heinemann, 2000; Haase et al., 1996) (Figure 1B). Certain transposons including Tn7, Tn3 and Mu-like ones also exhibit defense phenotypes by rendering hundreds of kilobases of their genomic neighborhood refractory to integration of a second transposon (Arciszewska et al., 1989; Lambin et al., 2012; Stellwagen and Craig, 1997; Walker and Harshey, 2020) (Figure 1C). A distinct class of MGE, the reverse transcriptase-utilizing Retrons also abrogate bacteriophage infection of their hosts via mechanisms that remain to be characterized in detail (Gao et al., 2020; Millman et al., 2020) (Figure 1D). These “defensive” retrons might have been derived from an ancestral retrotransposon (group II intron). Generally, the dual role played by MGEs in both parasitizing and immunizing their host cells reflects the ‘shared interests’ between the two entities, which may be long-lasting or ephemeral.
Recruitment of Mobile Genetic Elements Genes for Replication, Recombination and Repair
Chromosome Replication
Most prokaryotic chromosomes and some MGE replicons are covalently closed circular DNA molecules. Circular DNA poses a topological challenge for the proper segregation of genetic material upon cell division (Midonet et al., 2014). To face this challenge, MGEs and prokaryotes utilize resolvases to cleave dimers of covalently closed DNA molecules into monomers (Aravind et al., 2000). There is substantial evidence that prokaryotes repurposed tyrosine superfamily resolvases encoded by MGE for the faithful chromosome segregation.
A well-characterized resolution system featuring a tyrosine superfamily resolvase is Xer/dif. Xer enzymes catalyze site-specific recombination at dif sites to resolve a circular DNA molecule into two individual molecules (Midonet et al., 2014) (Figure 2A). The bacterial XerC are closely related to the resolvases that mediate dimer resolution of plasmids and some Tn3-like transposons (Nicolas et al., 2015). Xer family recombinases are almost universal in bacteria and archaea (Smyshlyaev et al., 2021), but appear to have been displaced by homologs from MGEs on several occasions. In particular, chromosome segregation in Streptococcus and Lactobacillus is effectuated by XerS, a resolvase more closely related to those of bacteriophages than it is to XerC (Le Bourgeois et al., 2007; Cortez et al., 2010). In archaea, XerA resolves chromosomes, but can also recombine dif sites located on plasmids and exhibits signatures of recent acquisition from an integrated mobile element (Cortez et al., 2010; Midonet et al., 2014). Thus, prokaryotes routinely recruit MGE-encoded tyrosine resolvases to solve the topological problem of replicating a circular chromosome. Conversely, plasmids and phages can hijack bacterial Xer recombinases for the resolution of replication intermediates or, via the exaptation route, to integrate the phage genome into the bacterial chromosome (Midonet et al., 2014; Midonet et al., 2019).
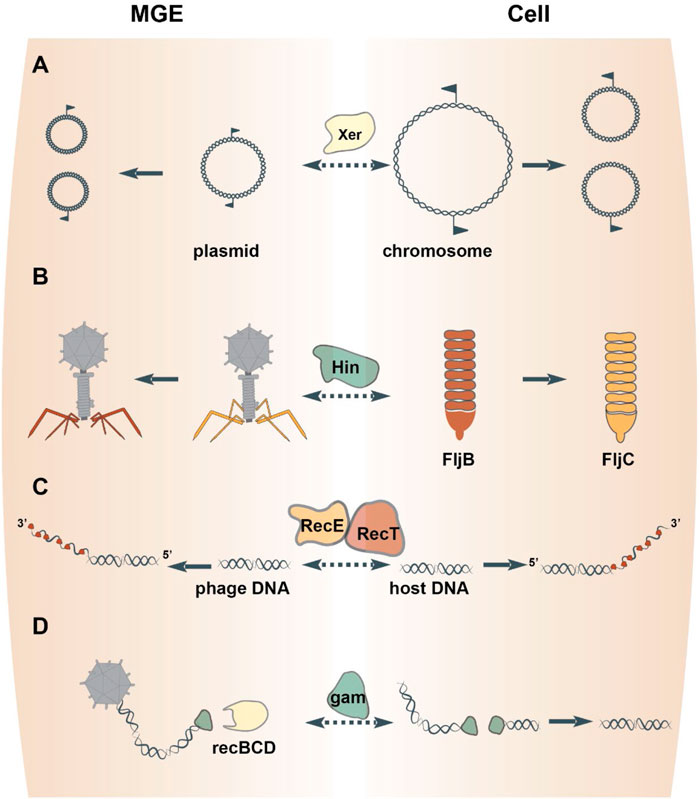
FIGURE 2. Proteins involved in DNA replication, recombination and repair freelance between MGEs and cells. Homologous resolvases mediate the resolution of both MGE and chromosome replication intermediates (A). DNA inverting serine recombinases direct the expression of alternative phage tail fiber genes or host flagellum genes (B). Exapted bacteriophage genes endow the host DNA repair pathways via homologous recombination (C) or nonhomologous end-joining (D).
MGEs also encode serine resolvases, which are unrelated to the tyrosine resolvases but play an analogous role (Aravind et al., 2000; Midonet et al., 2014; Smyshlyaev et al., 2021). To our knowledge, there is currently no evidence that prokaryotic chromosome segregation is ever mediated by serine resolvases, despite their analogous functionality.
Not all prokaryotes and MGE possess circular DNA replicons, some instead have linear molecules and covalently closed hairpin termini (Kobryn and Chaconas, 2002). Replication of such molecules also poses a topological challenge because bidirectional replication yields a circular dimer (Casjens, 1999; Kobryn et al., 2014). To face this challenge, MGE and prokaryotes encode telomerases that ensure the faithful segregation of linear DNA replicons. The paradigmatic telomerase is ResT, which is encoded by a linear plasmid in Borrelia burgdorferi. Indeed, loss of the plasmid-encoded ResT is lethal to B. burgdorferi due to defects in replicating the linear chromosomes (Byram et al., 2004). Thus, B. burgdoreferi outsources this critical step in DNA replication to an MGE.
Repurposed Mobile Genetic Element Enzymes Mediate Programmed Rearrangements of Host DNA and Proteins
Prokaryotes co-opted recombinases encoded by mobile genetic elements on multiple occasions to rearrange segments of chromosomal DNA. These recombinases belong to two unrelated superfamilies that are defined by the amino acid in their active site, either a serine or tyrosine. Chromosomal rearrangements mediated by enzymes of either superfamily serve to regulate transcription or create novel combinations of genes involved in various cellular processes (Weightman et al., 2002; Honarvar et al., 2003; Jiang et al., 2019).
One mechanism by which recombinases effectuate regulation is through DNA inversions, which either link alternative genes to the same promoter or switch the orientation of an individual gene’s promoter (Johnson, 2015). For example, DNA inversion mediated by the Salmonella enterica serine recombinase Hin results in the expression of one of two distinct flagellins (Johnson, 2015) (Figure 2B). Hin bears close sequence similarity to the serine recombinases encoded by the bacteriophages Mu and P1 (82% amino acid identity over the recombinase catalytic domain), which perform equivalent DNA inversion events in the phage genome to direct expression of different phage tail fibers (Harshey, 2015). Indeed, either phage enzyme can complement Hin-mutants to restore phase variation in Salmonella enterica (Simon et al., 1980). Such interchangeability of the DNA inverting serine recombinases between MGEs and their hosts has been documented for other pairs as well (Tominaga, 1997; Kutsukake et al., 2006). Thus, serine DNA invertases are frequently exchanged between MGEs and cells to regulate gene rearrangements.
A second mechanism by which recombinases effectuate regulation is through integration and excision of DNA. Enzymes from both serine and tyrosine recombinase superfamilies mediate the integration of all varieties of MGE, including plasmids, viruses and transposons, into prokaryotic chromosomes (Askora et al., 2011; Landy, 2015; Rice and Craig, 2015). In numerous cases where the MGE becomes incapable of horizontal transfer, such as via the loss of genes required for mobility, that MGE is repurposed as an excisable ‘switch’. For example, a defective prophage in L. monocytogenes is integrated into the comK gene, splitting the gene into two ORFs that are restored upon excision of the prophage (Pasechnek et al., 2020). Functionally analogous events mediate sporulation (Haraldsen and Sonenshein, 2003; Abe et al., 2017), nitrogen fixation (Golden et al., 1988; Carrasco et al., 1995) and DNA repair (Scott et al., 2008) in other prokaryotes. Thus, in many cases, integration and excision of MGEs catalyzed by their integrases serve to regulate transcription in prokaryotes.
Mobilization of prokaryotic self-splicing introns appears to provide a level of regulatory control to the benefit of the host cells, in which these elements reside. Mobile introns consist of a catalytic, self-splicing RNA (a ribozyme) and either a homing endonuclease (group I introns) or a RT-containing protein (group II introns) (Edgell et al., 2011). Although the mechanisms of mobility differ between group I and group II introns, ribozymes from both types of elements catalyze the intron excision from the parental RNA molecules (Hausner et al., 2014). Excision from parental RNA involves pairing of the extreme 5′ and 3′ ends of the intron at the intron-exon boundary and, consequently, restoration of the interrupted exon (Hausner et al., 2014). If the exon is a protein-coding gene, such restoration yields a fully functional protein upon translation. In Clostridium difficile, a group I intron is inserted upstream of a gene involved in bacterial virulence such that, upon excision, the ribosome-binding site is restored, stimulating translation (Lee et al., 2010). Critically, this group I intron lacks a homing endonuclease, and furthermore, the ribozyme activity is stimulated by a second messenger, cyclic diGMP, indicating that the ribozyme was exapted to tune translation (Lee et al., 2010). In other organisms, excision of group I or group II introns occurs in response to specific stimuli related to the gene product in which they reside (Belfort, 2017). For example, light stimulates excision of a group I intron from the photosynthesis gene psbA in Chlamydomonas chloroplasts (Deshpande et al., 1997; Lee and Herrin, 2003), suggesting that some introns function as molecular sensors of environmental cues.
Mobilization of a second, unrelated class of MGE, inteins, provides a degree of post-translational regulatory control. Like introns, inteins are mobilized to new DNA sites by homing endonucleases and are capable of self-splicing. Unlike introns, intein self-splicing and excision occurs after translation, from parental polypeptides (Novikova et al., 2016). Intein excision yields conditional post-translational regulatory control that is conceptually analogous to post-transcriptional control achieved by introns. For example, Pyrococcus horikoshii RadA hosts an intein that splices specifically in response to the presence of single-stranded DNA, the natural RadA substrate (Lennon et al., 2016). The RadA intein lacks a homing endonuclease and is therefore incapable of self transfer to new DNA sites, yet retains self-splicing capability (Lennon et al., 2016). Numerous other proteins host inteins that splice in response to environmental stimuli (Belfort, 2017), indicating that these inteins were repurposed as post-translational regulatory switches.
Capture of Mobile Genetic Elements Enzymes That Endow Prokaryotes With DNA Recombination and Repair Pathways
A cardinal mechanism of DNA repair is homologous recombination between paired strands of DNA. In E. coli, genetic analyses identified a homologous recombination pathway catalyzed by RecET (Kolodner et al., 1994). The nuclease activity of RecE generates single-stranded DNA overhangs that are subsequently bound by RecT to promote pairing and strand exchange between homologous segments of DNA (Kolodner et al., 1994) (Figure 2C). These enzymes are encoded by a defective prophage in E. coli, termed Rac (Kaiser and Murray, 1979). Rac is a prophage that lost ∼60% of its original DNA and is therefore incapable of replication or production of progeny virions (Casjens, 2003). Fully infectious Rac-like phages encode RecE homologs in similar genomic positions and mediate homologous recombination in their hosts (Figueroa-Bossi et al., 1997). This evidence indicates that the E. coli RecET homologous recombination pathway was captured from a temperate phage, perhaps, relatively recently because other, even closely related bacteria lack this prophage. Other bacteriophages encode ssDNA-binding proteins that promote homologous recombination, which belong to three unrelated superfamilies, RecT, Rad52 and ERF (Iyer et al., 2002). Like RecT, members from the other two superfamilies were captured from temperate phages because they are encoded sporadically throughout the bacterial domain and are flanked by phage-related genes that code for proteins involved in DNA recombination and repair (e.g., Holliday junction resolvases) (Iyer et al., 2002). Thus, genes of three unrelated superfamilies were recruited by bacteria from phages on multiple occasions for roles in homologous recombination.
A second mechanism of DNA repair is non-homologous end-joining (NHEJ). The joining reaction requires a DNA ligase and a DNA end-binding protein, known as Ku in eukaryotes. Homologs of Ku are present in prokaryotes (Aravind and Koonin, 2001; Doherty et al., 2001) and also mediate NHEJ by recruiting a DNA ligase and stimulating ligation of the two DNA ends (Weller et al., 2002). The prokaryotic Ku homologs are encoded within defective prophages related to the fully infectious E. coli phage Mu (di Fagagna et al., 2003). In Mu, the Ku homolog is known as gam, which is primarily involved in protecting Mu progeny from destruction by RecBCD during the lytic cycle, but can also mediate NHEJ of host DNA (Bhattacharyya et al., 2018) (Figure 2D). These observations collectively point to the shuttling of gam between phages and their bacterial hosts.
A special case of exaptation of MGEs to manipulate bacterial DNA is represented by Diversity-Generating Retroelements (DGR). DGRs are genetic cassettes composed of a reverse-transcriptase (RT) related to group II intron RTs, an accessory gene and cis-acting regulatory sequences (Doulatov et al., 2004; Handa et al., 2018). The DGRs broadly colonize both prokaryotic and phage genomes and introduce multiple mutations into specific target genes via highly error prone reverse transcription and retrohoming (Paul et al., 2017; Benler et al., 2018; Roux et al., 2021). The DGRs primarily introduce hypervariation into genes encoding cell-cell and virus-cell attachment but might contribute also to other cellular processes (Arambula et al., 2013; Vallota-Eastman et al., 2020). The frequent trafficking of DGRs between chromosomes, plasmids and phages (Wu et al., 2017) evinces the utility of accelerated protein sequence evolution mediated by this domesticated MGE.
Co-Option of Transcription Factors From Mobile Genetic Elements for Host Cell Gene Regulation
On multiple occasions, prokaryotes seem to have adopted MGE-encoded transcription factors to regulate transcriptional networks. The most common DNA-binding moiety in prokaryotic transcription factors is the ubiquitous helix-turn-helix (HTH) domain (Brown et al., 2003; Cuthbertson and Nodwell, 2013; Hoskisson and Rigali, 2009). Due to the small size of the HTH domain, robust phylogenetic reconstruction of the evolutionary history of HTH domain-containing genes proves difficult. Nevertheless, apparent monophyletic groups can be identified, several of which show clear signs of exchange between prokaryotes and MGEs (Aravind et al., 2005). The principal signature of such an event is the widespread presence of a given family of HTH domain-containing proteins in MGEs and a restricted distribution in prokaryotes. For example, Xre is the archetypical member of one of the families of HTH domains with tetrahelical quaternary structure (Aravind et al., 2005). Xre regulates the lysis-lysogeny of a degraded prophage in B. subtilis (McDonnell and McConnell, 1994), and homologs of Xre regulate the lysis-lysogeny decisions of autonomous bacteriophages, for example, the ner gene encoded by E. coli phage Mu (Tolias and Dubow, 1986) (Figure 3A). Ner exhibits 68% amino acid identity to SfsB (NP_417655.1), a transcription factor that is conserved in Enterobacteriaceae and regulates genes involved in maltose metabolism (Autexier and Dubow, 1992) (Figure 3B). The high sequence similarity between Ner and SfsB suggests a relatively recent exchange. Furthermore, overexpression of ner can complement sfsB by stimulating the expression of maltose metabolic genes in E. coli (Autexier and Dubow, 1992), underscoring the ease with which a transcription factor from a MGE can be recruited into the host regulatory cascades.
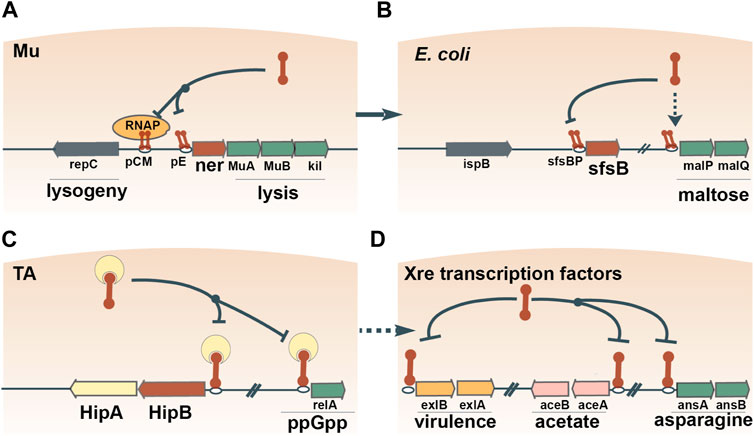
FIGURE 3. Recruitment of transcription factors from MGEs to regulate host gene expression. One example is Ner, which contains an Xre-family HTH domain and regulates the lysis-lysogeny switch of bacteriophage Mu (A). The amino acid sequence of Ner is 68% identical to the maltose operon-activating transcription factor SfsB, indicating the recent recruitment of a Ner homolog for the regulation of host carbon metabolism (B). Xre domains are widespread in antitoxin proteins, such as HipB, which autoregulate their own expression and the expression of other host genes (C). Antitoxins often disassociate from their TA operons and assume dedicated roles as transcription factors (D).
The antitoxins of toxin-antitoxin (TA) pairs have also been shown to double as cellular transcription factors, illustrating another plausible route of exaptation. Specifically, Xre-family HTH domains are widespread in antitoxins of type II TA systems (Freire et al., 2019; Makarova et al., 2009; Skjerning et al., 2019). Typically, the TA complex autoregulates its own transcription by binding to an operator(s) upstream of the TA operon via the HTH domain (Page and Peti, 2016). In E. coli, two different antitoxins bind to the operators of genes located outside of the cognate TA pair and simultaneously regulate multiple operons (Lin et al., 2013; Soo and Wood, 2013) (Figure 3C). These observations suggest that antitoxins first assume regulatory control of host genes, and then, disassociate from the TA pair and become dedicated transcription factors. Transcription factors containing Xre-family HTH domains regulate operons involved in various processes, such as virulence (Trouillon et al., 2020), acetate metabolism (Gerstmeir et al., 2004), asparagine metabolism (Sun and Setlow, 1993) and propionyl coenzyme A assimilation (Carter and Alber, 2015) (Figure 3D). Overall, Xre homologs function as transcription factors that often switch between regulatory roles in MGE and host gene expression.
The incorporation of MGE-encoded transcription factors into host regulatory networks likely extends beyond the Xre-family of HTH domains. For example, Ribbon-Helix-Helix (RHH) domains are common in MetJ/Arc-family transcription factors as well as in TAs, suggesting that the bacterial transcription factors of this family were originally derived from antitoxins (Aravind et al., 2005). Given that TAs and other MGEs are activated by various signals, such as DNA damage (Knowles et al., 2017) or the presence of other MGE (McKitterick and Seed, 2018; LeGault et al., 2021), recruitment of MGE-encoded transcription factors could be favorable for the host, enabling it to respond to the same stressors (Benler and Koonin, 2020).
Exaptation of Mobile Genetic Elements Genes for Functions in Cell Cycle Control, Cell Division, Chromosome Partitioning
Both MGEs and prokaryotic cells employ partitioning systems that ensure inheritance of DNA by the daughter cells upon binary fission of a parental cell. Three well-characterized partitioning systems all require an NTPase, a centromere-like site and a DNA-binding adaptor protein that connects the two (Gerdes et al., 2010). The paradigmatic ParABS system contains a P-loop superfamily ATPase (ParA) and was originally characterized for its role in plasmid segregation (Ogura and Hiraga, 1983). ParA can also orchestrate the segregation of the chromosome on which they reside (Gerdes et al., 2010). Phylogenetic analysis largely separates plasmid and chromosomal parA genes, but in some cases, ParA genes of plasmid origin are encoded on chromosomes (Gerdes et al., 2000) and are necessary for their segregation (Yamaichi et al., 2007). Furthermore, even within the plasmid-dominated branch of ParA homologs, a subgroup exists that is represented by the cell division proteins MinD and Mrp of E. coli (Gerdes et al., 2000). Therefore, the parsimonious evolutionary scenario appears to involve exaptation of ParA from an MGE for the function in bacterial chromosomal DNA segregation and cell division.
Beyond the capture of MGE-encoded partitioning enzymes, the chromosomes of some prokaryotes themselves might originate from MGEs. One prominent example is the second chromosome of Vibrio cholerae, which is hypothesized to derive from an ancestral plasmid (Heidelberg et al., 2000), given the presence of multiple TA systems that are common addiction modules carried by plasmids and other MGEs (Makarova et al., 2009). Furthermore, as discussed above, the Par genes encoded by chromosome II of V. cholerae are phylogenetically closely related to plasmid Par genes, in contrast to those encoded on chromosome I that appear to be genuine cellular genes (Gerdes et al., 2000). Deletion of the par genes on chromosome II results in its loss upon cell division and is followed by cell death, which phenotypically resembles programmed cell death caused by free toxins released from their cognate antitoxins (Yamaichi et al., 2007). Together, these observations suggest that V. cholerae chromosome II evolved from a TA-carrying plasmid the maintenance of which was further reinforced by the capture of essential, housekeeping genes (McGeoch and Bell, 2008; Hülter et al., 2017). In other bacteria with multiple chromosomes, the ParS sites differ substantially between the primary and secondary chromosomes, again suggesting distinct evolutionary histories, with the secondary chromosomes evolving from plasmids (Livny et al., 2007). Thus, conversion of plasmids into chromosomes could be a common route of evolution in prokaryotes and seems to represent a distinct form of exaptation that involves “domestication” of an MGE replicon itself.
Mobile Genetic Elements Repurposed for Intra- and Intercellular Trafficking, Secretion and Vesicular Transport
Bacteriophage tails have been domesticated on multiple independent occasions for the secretion and transfer of gene products from bacterial cells and/or transfer to other cells. In particular, some Uroviricota phages (e.g., T4) possess contractile tails that puncture their host cell envelopes and serve as a conduit for the delivery of encapsidated DNA and proteins into the cytoplasm (Miller et al., 2003). Phage tails were neofunctionalized to secrete proteins to the benefit of the cell (Leiman et al., 2009; Lossi et al., 2013; Pell et al., 2009). Such devices, known as type VI secretion systems, are widespread and were likely captured from different phages on independent occasions (Böck et al., 2017; Denise et al., 2020) (Figure 4). The proteins transferred by type VI secretion systems are commensurately diverse. but many function as toxins that exert anti-bacterial or anti-eukaryotic activity (Cherrak et al., 2019). One such toxin apparently evolved from the phage tail tip protein (M. Iyer et al., 2021), whereas numerous other T6SS toxins possess cognate antitoxins (Nolan et al., 2021), suggesting that TA systems were recruited for inter-species competition. Other proteins secreted via phage tails perform non-competitive roles (Russell et al., 2014). For example, a phage tail-like structure produced by Pseudomonas luteoviolacea delivers a cargo protein that stimulates the metamorphic transition of marine tubeworm larvae into juveniles (Ericson et al., 2019; Cavalcanti et al., 2020). Overall, domesticated bacteriophage tails represent a major route for the secretion of diverse proteins from prokaryotic cells and their delivery to various targets.
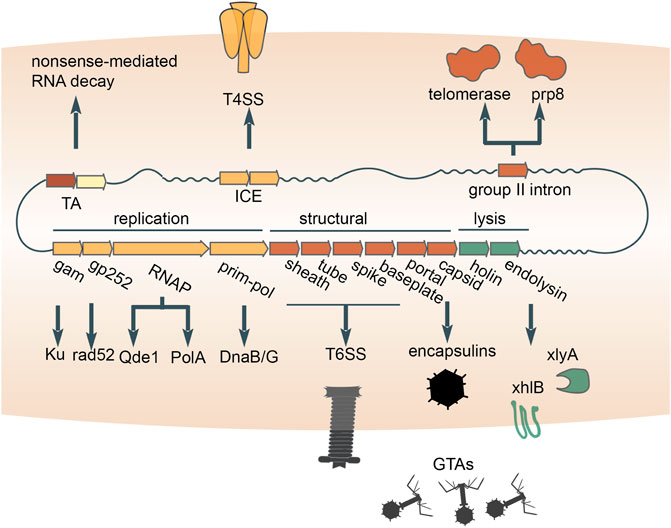
FIGURE 4. Multiple contributions of distinct mobile genetic elements to intercellular transfer pathways and eukaryogenesis. Exaptations of toxin-antitoxins (TAs), integrative and conjugative elements (ICE), bacterial retrotransposons and double-stranded DNA bacteriophages are diagrammed. An RNA-cleaving toxin apparently was incorporated into the eukaryotic nonsense-mediated RNA decay system. The conjugation apparatus of ICE was exapted for the transfer of proteins (type 4 secretion systems). Recruitment of the reverse transcriptase from bacterial retrotransposons yielded the key component of the eukaryotic spliceosome, Prp8 (accompanied by inactivation of the reverse transcriptase), as well as telomerases which retain the activity. The structural module of double-stranded DNA bacteriophages was repurposed for the delivery of proteins (type 6 secretion systems) or host DNA (GTAs) between cells. The replication and lysis modules donated multiple genes that play diverse roles in both prokaryotes and eukaryotes.
Prokaryotes transfer proteins and DNA across cell envelopes via an apparatus that was captured from conjugative mobile genetic elements. The MGEs that self-transfer via conjugation include plasmids and transposons. One of the key enzymes involved in conjugation is an HUH-superfamily endonuclease (Ilyina and Koonin, 1992), which nicks a DNA strand of the MGE prior to transfer (Alvarez-Martinez and Christie, 2009). A second, conserved enzyme is an FtsK/HerA-superfamily ATPase that pumps DNA bound to the endonuclease through mating bridges (Iyer et al., 2004). Phylogenomic analysis of the conserved ATPase shows that conjugation systems of MGEs repeatedly lose the endonuclease and thus can no longer mediate self-transfer (Guglielmini et al., 2012). These non-autonomous MGEs mediate non-conjugation-related secretion of protein and DNA (Guglielmini et al., 2012). By coopting the conjugative machinery from MGEs (Figure 4), prokaryotes opened up another route for the transfer of macromolecules out of the cell.
Another route by which prokaryotes transfer DNA is through repurposed bacteriophage virions. Highly degraded and fragmented phage genomes integrated in the genomes of many bacteria package host DNA, rather than the prophage DNA, into mini-phage virions (Bárdy et al., 2020; Esterman et al., 2021; Kogay et al., 2019; Lang et al., 2012; Shakya et al., 2017) (Figure 4). Because these mini-phage particles lyse the cell from within and transfer the packaged DNA to a recipient host, they are known as Gene Transfer Agents (GTA), where the transfer of DNA from the primary host cell is thought to confer a selective advantage for the recipient cells and ultimately for the population as a whole (Lang et al., 2012). Thus, the GTAs are domesticated, defective phages that have been exapted to serve as dedicated vehicles for DNA transfer within microbial populations. A notable, related system are the pirate phages, such as Staphylococcus aureus pathogenicity islands (SaPis). The SaPis and other pirate phages spread by hijacking the particles of a superinfecting phage (Novick and Ram, 2017; Novick, 2019). However, the SaPi particles are harmless to the host bacterium because they have no capacity to reproduce via the lytic cycle. In this process, the pirate phage both spreads its genome and protects the host population from killing by the pirated phage.
Besides mediating intercellular transfer of macromolecules, capsids of dsDNA phages might have been enlisted for the intracellular trafficking and compartmentalization of proteins. All tailed dsDNA bacteriophages and archaeal viruses (virus realm Duplodnaviria) encase their genomes within icosahedral capsid made of the HK97-fold major capsid protein (Koonin et al., 2020). Shells built from HK97-fold proteins with significant similarity to phage capsid proteins are encoded by standalone genes in numerous bacterial and archaeal genomes, where they form icosahedral particles known as encapsulins, which sequester diverse cargo proteins (Fontana et al., 2014; Giessen and Silver, 2017; Twarock and Luque, 2019; Nichols et al., 2021). Although the specific evolutionary relationships between encapsulins and phage capsids remain to be elucidated, a plausible evolutionary scenario is that encapsulins were domesticated from double-stranded DNA viruses on one or more occasions (Krupovic and Koonin, 2017) (Figure 4), providing prokaryotes with a means to sequester reactants into a nanocompartment.
Genes captured from bacteriophages contribute to the formation of bacterial biofilms, vesicles and spores. The proteins encoded in the phage lysis gene cassettes permeabilize cytoplasmic membranes and enzymatically degrade host cell peptidoglycan from within, releasing progeny viral particles for subsequent infections (Cahill and Young, 2019). Programmed lysis by phage lytic genes releases the macromolecular components of the cell, in particular DNA, a principal constituent of biofilm matrices. Biofilm matrix formation mediated by the lysis cassette of a domesticated bacteriophage has been observed in Pseudomonas aeruginosa (Heussler et al., 2015). In Bacillus subtilis, phage lysis cassettes instead mediate the formation of membrane vesicles (Toyofuku et al., 2017) or spores (Real et al., 2005). In Caulobacterales, a phage lytic enzyme was coopted as a key gene required for cellular morphological development (Randich et al., 2019). These examples highlight the utility of phage lysis cassettes for cellular wall remodeling or destruction of individual cells within larger populations, resulting in population level benefits.
Multiple Contributions of Prokaryotic Mobile Genetic Elements to Eukaryogenesis
Apart from their diverse input to the evolution of various functional systems in bacteria and archaea, prokaryotic MGE made major contributions to the origin of eukaryotes, partly, through the mitochondrial endosymbiont. Here we can give only a brief account of these recruitments of MGE genes, but leaving them out would fail to give justice to the evolutionary role of these MGE. Most bacteriophages encode polymerases that replicate and transcribe their genetic information, and on at least one occasion, such polymerases displaced the functionally analogous bacterial polymerases during eukaryogenesis. Strikingly, three enzymes that are encoded in eukaryotic nuclear genomes and involved in the replication and transcription of mitochondrial genomes have readily traceable phage ancestry. Specifically, the mitochondrial DNA-dependent DNA polymerase (DNAP) of the A family, DnaB-DnaG-like helicase-primase and single-subunit DNA-directed RNA polymerase (RNAP) are all more closely related to the corresponding polymerases of T7/T3-like phages than to any bacterial polymerases (Filée et al., 2002) (Figure 4). The case of the RNAP is particularly notable because the phage single subunit RNAP, originally apparently derived from a bacterial A family DNAP (Cheetham and Steitz, 2000), became the enzyme responsible for the expression of the mitochondrial genome, displacing the multisubunit RNAP that is universal in all cellular life forms. Most likely, all these enzymes were exapted from a prophage that was integrated in the genome of the ancestral α-proteobacterium that gave rise to mitochondria as a result of endosymbiosis (Filée and Forterre, 2005; Shutt and Gray, 2006). The non-orthologous displacement of the RNAP occurred early in the evolution of the mitochondria, but probably, many millions of years post-endosymbiosis because the mitochondrial genomes of at least some jacobids, such as Reclinomonas americana, encode a typical bacterial multisubunit RNAP (Burger et al., 2013; Gray et al., 2020).
Many components of eukaryotic innate immune and damage control systems as well as repair and splicing machineries seem to derive from prokaryotic MGEs. Such connections include the apparent origin of components of the eukaryotic nonsense-mediated mRNA decay system from bacterial TA modules (Anantharaman and Aravind, 2003) and the animal apoptosis proteins Bax/Bak from phage lysis cassettes (Saier et al., 2015). A component of the eukaryotic RNAi machinery, the QDE1 family RNA-dependent RNA polymerase, appears to have evolved from a distinct bacteriophage RNAP (Drobysheva et al., 2021; Iyer et al., 2003; Shabalina and Koonin, 2008) (Figure 4). Two proteins that play central roles in homologous recombination and NHEJ (double-strand break repair) in eukaryotes, Rad52 and Ku, respectively, appear to have originated in bacteriophages (Aravind and Koonin, 2001; di Fagagna et al., 2003). Both the telomerase that restores chromosomal termini and the key protein of the eukaryotic spliceosome were captured from a reverse-transcriptase encoded by group II introns, that is, bacterial retrotransposons (Dlakić and Mushegian, 2011; Gladyshev and Arkhipova, 2011; Lambowitz et al., 2015) (Figure 4). The relationships between eukaryotic proteins and their ancestors from prokaryotic MGE are often subtle and hard to detect, so the full extent of the contribution of these elements to eukaryogenesis awaits a systematic investigation with the most powerful available tools for protein sequence and structure comparison.
Concluding Remarks
The broad repertoire of exaptations surveyed here extends to numerous functional systems, abundantly illustrating the evolutionary entanglement between MGEs and their prokaryote hosts. Nevertheless, the overarching principle inferred from the study of defensive exaptations is readily applicable. That is, molecular components evolved by MGEs are expediently recruited for mechanistically similar but biologically distinct roles in the cell owing to their fundamental biochemical utility. This principle has been captured in the “guns for hire” metaphor (Koonin et al., 2019), which emphasizes the perennial shuttling of genes, gene modules and whole replicons between MGE and their hosts. The most prominent contribution of prokaryotic MGE is to the molecular componentry of functional systems that are involved in various biological conflicts, in particular, defense against viruses and other MGEs. To wit, the complex molecular machinery of CRISPR, the prokaryotic adaptive immune system, apparently was assembled primarily if not completely from components exapted from MGE. Moreover, restriction-modification and TA modules, the most common innate immunity systems in prokaryotes, themselves can be considered a distinct variety of MGE that, while lacking their own replicative machinery, attain extensive horizontal mobility by routinely piggy-backing on plasmids and viruses (Kobayashi, 2001; Fraikin et al., 2020). This evolutionary entanglement of MGE and defense systems appears to be far from accidental but rather reflects a deep unifying feature. Indeed, both types of genetic elements are generally deleterious, stronger in the case of MGE and weaker in the case of defense systems (that are beneficial only during the brief periods of exposure to the respective MGEs), to the organisms in which they reside (Iranzo et al., 2017). Therefore, these elements evolved and exploit various mechanisms of survival that include horizontal mobility, causing host addiction, and exaptation for roles beneficial to the host.
It has been noted that replicators form a continuous spectrum with regard to the degree of their replicative autonomy and cooperativity (Jalasvuori and Koonin, 2015; Koonin et al., 2019). The numerous cases of exaptation and shuttling of components between MGE and hosts as well as among different varieties of MGE show that this continuity also encompasses uninterrupted flow of genetic material across the spectrum. Crucially, the coevolution of MGEs and their cellular hosts cannot be reduced to arms race, but rather involves the entire gamut of cooperation, inter-MGE competition, and exaptation. Exaptation of MGE and their components pervades the history of most if not all cellular organisms, and hardly any MGE seem to evade exaptation of at least some of their components. Moreover, these exaptations substantially contributed to evolutionary transitions, such as the origin of eukaryotes. Comprehensive investigation of the flow of genetic information between MGE and cellular life forms should provide major insight into the evolution of life.
Author Contributions
SB and EK wrote the paper.
Funding
Funding for this project was provided by the Intramural Research Program of the National Institutes of Health (National Library of Medicine).
Conflict of Interest
The authors declare that the research was conducted in the absence of any commercial or financial relationships that could be construed as a potential conflict of interest.
Publisher’s Note
All claims expressed in this article are solely those of the authors and do not necessarily represent those of their affiliated organizations, or those of the publisher, the editors, and the reviewers. Any product that may be evaluated in this article, or claim that may be made by its manufacturer, is not guaranteed or endorsed by the publisher.
References
Abe, K., Takamatsu, T., and Sato, T. (2017). Mechanism of Bacterial Gene Rearrangement: SprA-Catalyzed Precise DNA Recombination and its Directionality Control by SprB Ensure the Gene Rearrangement and Stable Expression of spsM during Sporulation in Bacillus Subtilis. Nucleic Acids Res. 45, 6669–6683. doi:10.1093/nar/gkx466
Altae-Tran, H., Kannan, S., Demircioglu, F. E., Oshiro, R., Nety, S. P., McKay, L. J., et al. (2021). The Widespread IS200/IS605 Transposon Family Encodes Diverse Programmable RNA-Guided Endonucleases. Science 374, 57–65. doi:10.1126/science.abj6856
Alvarez-Martinez, C. E., and Christie, P. J. (2009). Biological Diversity of Prokaryotic Type IV Secretion Systems. Microbiol. Mol. Biol. Rev. 73, 775–808. doi:10.1128/mmbr.00023-09
Amitai, G., and Sorek, R. (2016). CRISPR-cas Adaptation: Insights into the Mechanism of Action. Nat. Rev. Microbiol. 14, 67–76. doi:10.1038/nrmicro.2015.14
Anantharaman, V., and Aravind, L. (2003). New Connections in the Prokaryotic Toxin-Antitoxin Network: Relationship with the Eukaryotic Nonsense-Mediated RNA Decay System. Genome Biol. 4, R81. doi:10.1186/gb-2003-4-12-r81
Arambula, D., Wong, W., Medhekar, B. A., Guo, H., Gingery, M., Czornyj, E., et al. (2013). Surface Display of a Massively Variable Lipoprotein by a Legionella Diversity-Generating Retroelement. Proc. Natl. Acad. Sci. 110, 8212–8217. doi:10.1073/pnas.1301366110
Aravind, L., Anantharaman, V., Balaji, S., Babu, M., and Iyer, L. (2005). The many Faces of the helix-turn-helix Domain: Transcription Regulation and beyond. FEMS Microbiol. Rev. 29, 231–262. doi:10.1016/j.femsre.2004.12.008
Aravind, L., and Koonin, E. V. (2001). Prokaryotic Homologs of the Eukaryotic DNA-End-Binding Protein Ku, Novel Domains in the Ku Protein and Prediction of a Prokaryotic Double-Strand Break Repair System. Genome Res. 11, 1365–1374. doi:10.1101/gr.181001
Aravind, L., Leipe, D. D., and Koonin, E. V. (1998). Toprim--a Conserved Catalytic Domain in Type IA and II Topoisomerases, DnaG-type Primases, OLD Family Nucleases and RecR Proteins. Nucleic Acids Res. 26, 4205–4213. doi:10.1093/nar/26.18.4205
Aravind, L., Makarova, K. S., and Koonin, E. V. (2000). SURVEY and SUMMARY: holliday junction Resolvases and Related Nucleases: Identification of New Families, Phyletic Distribution and Evolutionary Trajectories. Nucleic Acids Res. 28, 3417–3432. doi:10.1093/nar/28.18.3417
Arciszewska, L. K., Drake, D., and Craig, N. L. (1989). Transposon Tn7. J. Mol. Biol. 207, 35–52. doi:10.1016/0022-2836(89)90439-7
Askora, A., Kawasaki, T., Fujie, M., and Yamada, T. (2011). Resolvase-like Serine Recombinase Mediates Integration/excision in the Bacteriophage ϕRSM. J. Biosci. Bioeng. 111, 109–116. doi:10.1016/j.jbiosc.2010.10.001
Autexier, C., and Dubow, M. S. (1992). The Escherichia coli Mu/D108 Phage Ner Homologue Gene (Nlp) Is Transcribed and Evolutionary Conserved Among the Enterobacteriaceae. Gene 114, 13–18. doi:10.1016/0378-1119(92)90701-p
Bárdy, P., Füzik, T., Hrebík, D., Pantůček, R., Thomas Beatty, J., and Plevka, P. (2020). Structure and Mechanism of DNA Delivery of a Gene Transfer Agent. Nat. Commun. 11, 3034.
Béguin, P., Charpin, N., Koonin, E. V., Forterre, P., and Krupovic, M. (2016). Casposon Integration Shows strong Target Site Preference and Recapitulates Protospacer Integration by CRISPR-Cas Systems. Nucleic Acids Res. 44, 10367–10376. doi:10.1093/nar/gkw821
Belfort, M. (2017). Mobile Self-Splicing Introns and Inteins as Environmental Sensors. Curr. Opin. Microbiol. 38, 51–58. doi:10.1016/j.mib.2017.04.003
Benler, S., Cobián-Güemes, A. G., McNair, K., Hung, S.-H., Levi, K., Edwards, R., et al. (2018). A Diversity-Generating Retroelement Encoded by a Globally Ubiquitous Bacteroides Phage. Microbiome 6, 191. doi:10.1186/s40168-018-0573-6
Benler, S., Faure, G., Altae-Tran, H., Shmakov, S., Zheng, F., Koonin, E., et al. (2021). Cargo Genes of Tn7-like Transposons Comprise an Enormous Diversity of Defense Systems, Mobile Genetic Elements, and Antibiotic Resistance Genes. mBio 12, e02938–02921. doi:10.1128/mbio.02938-21
Benler, S., and Koonin, E. V. (2020). Phage Lysis‐lysogeny Switches and Programmed Cell Death: Danse Macabre. BioEssays 42, 2000114. doi:10.1002/bies.202000114
Bernheim, A., and Sorek, R. (2020). The Pan-Immune System of Bacteria: Antiviral Defence as a Community Resource. Nat. Rev. Microbiol. 18, 113–119. doi:10.1038/s41579-019-0278-2
Bhattacharyya, S., Soniat, M. M., Walker, D., Jang, S., Finkelstein, I. J., and Harshey, R. M. (2018). Phage Mu Gam Protein Promotes NHEJ in Concert withEscherichia Coliligase. Proc. Natl. Acad. Sci. USA 115, E11614–E11622. doi:10.1073/pnas.1816606115
Böck, D., Medeiros, J. M., Tsao, H. F., Penz, T., Weiss, G. L., Aistleitner, K., et al. (2017). In Situ architecture, Function, and Evolution of a Contractile Injection System. Science 357, 713–717. doi:10.1126/science.aan7904
Brown, N. L., Stoyanov, J. V., Kidd, S. P., and Hobman, J. L. (2003). The MerR Family of Transcriptional Regulators. FEMS Microbiol. Rev. 27, 145–163. doi:10.1016/s0168-6445(03)00051-2
Burger, G., Gray, M. W., Forget, L., and Lang, B. F. (2013). Strikingly Bacteria-like and Gene-Rich Mitochondrial Genomes throughout Jakobid Protists. Genome Biol. Evol. 5, 418–438. doi:10.1093/gbe/evt008
Burroughs, A. M., and Aravind, L. (2020). Identification of Uncharacterized Components of Prokaryotic Immune Systems and Their Diverse Eukaryotic Reformulations. J. Bacteriol. 202. doi:10.1128/JB.00365-20
Burroughs, A. M., Zhang, D., Schäffer, D. E., Iyer, L. M., and Aravind, L. (2015). Comparative Genomic Analyses Reveal a Vast, Novel Network of Nucleotide-Centric Systems in Biological Conflicts, Immunity and Signaling. Nucleic Acids Res. 43, 10633–10654. doi:10.1093/nar/gkv1267
Byram, R., Stewart, P. E., and Rosa, P. (2004). The Essential Nature of the Ubiquitous 26-Kilobase Circular Replicon of Borrelia Burgdorferi. J. Bacteriol. 186, 3561–3569. doi:10.1128/jb.186.11.3561-3569.2004
Cahill, J., and Young, R. (2019). “Phage Lysis: Multiple Genes for Multiple Barriers,” in Advances in Virus Research. Editors M. Kielian, T. C. Mettenleiter, and M. J. Roossinck (Academic Press), 33–70. doi:10.1016/bs.aivir.2018.09.003
Carr, V. R., Shkoporov, A., Hill, C., Mullany, P., and Moyes, D. L. (2021). Probing the Mobilome: Discoveries in the Dynamic Microbiome. Trends Microbiol. 29, 158–170. doi:10.1016/j.tim.2020.05.003
Carrasco, C. D., Buettner, J. A., and Golden, J. W. (1995). Programmed DNA Rearrangement of a Cyanobacterial hupL Gene in Heterocysts. Proc. Natl. Acad. Sci. 92, 791–795. doi:10.1073/pnas.92.3.791
Carter, M. S., and Alber, B. E. (2015). Transcriptional Regulation by the Short-Chain Fatty Acyl Coenzyme A Regulator (ScfR) PccR Controls Propionyl Coenzyme A Assimilation by Rhodobacter Sphaeroides. J. Bacteriol. 197, 3048–3056. doi:10.1128/jb.00402-15
Casjens, S. (1999). Evolution of the Linear DNA Replicons of the Borrelia Spirochetes. Curr. Opin. Microbiol. 2, 529–534. doi:10.1016/s1369-5274(99)00012-0
Casjens, S. (2003). Prophages and Bacterial Genomics: what Have We Learned So Far? Mol. Microbiol. 49, 277–300. doi:10.1046/j.1365-2958.2003.03580.x
Cavalcanti, G. S., Alker, A. T., Delherbe, N., Malter, K. E., and Shikuma, N. J. (2020). The Influence of Bacteria on Animal Metamorphosis. Annu. Rev. Microbiol. 74, 137–158. doi:10.1146/annurev-micro-011320-012753
Cheetham, G. M., and Steitz, T. A. (2000). Insights into Transcription: Structure and Function of Single-Subunit DNA-dependent RNA Polymerases. Curr. Opin. Struct. Biol. 10, 117–123. doi:10.1016/s0959-440x(99)00058-5
Cheng, R., Huang, F., Wu, H., Lu, X., Yan, Y., Yu, B., et al. (2021). A Nucleotide-Sensing Endonuclease from the Gabija Bacterial Defense System. Nucleic Acids Res. 49, 5216–5229. doi:10.1093/nar/gkab277
Cherrak, Y., Flaugnatti, N., Durand, E., Journet, L., Cascales, E., Sandkvist, M., et al. (2019). Structure and Activity of the Type VI Secretion System. Microbiol. Spectr. 7, 7–411. doi:10.1128/microbiolspec.PSIB-0031-2019
Cooper, T. F., and Heinemann, J. A. (2000). Postsegregational Killing Does Not Increase Plasmid Stability but Acts to Mediate the Exclusion of Competing Plasmids. Proc. Natl. Acad. Sci. 97, 12643–12648. doi:10.1073/pnas.220077897
Cortez, D., Quevillon-Cheruel, S., Gribaldo, S., Desnoues, N., Sezonov, G., Forterre, P., et al. (2010). Evidence for a Xer/dif System for Chromosome Resolution in Archaea. Plos Genet. 6, e1001166. doi:10.1371/journal.pgen.1001166
Cuthbertson, L., and Nodwell, J. R. (2013). The TetR Family of Regulators. Microbiol. Mol. Biol. Rev. 77, 440–475. doi:10.1128/mmbr.00018-13
Denise, R., Abby, S. S., and Rocha, E. P. C. (2020). The Evolution of Protein Secretion Systems by Co-option and Tinkering of Cellular Machineries. Trends Microbiol. 28, 372–386. doi:10.1016/j.tim.2020.01.005
Deshpande, N. N., Bao, Y., and Herrin, D. L. (1997). Evidence for Light/redox-Regulated Splicing of psbA Pre-RNAs in Chlamydomonas Chloroplasts. Rna 3, 37
di Fagagna, F. d. A., Weller, G. R., Doherty, A. J., and Jackson, S. P. (2003). The Gam Protein of Bacteriophage Mu Is an Orthologue of Eukaryotic Ku. EMBO Rep. 4, 47–52. doi:10.1038/sj.embor.embor709
Dlakić, M., and Mushegian, A. (2011). Prp8, the Pivotal Protein of the Spliceosomal Catalytic center, Evolved from a Retroelement-Encoded Reverse Transcriptase. RNA 17, 799–808. doi:10.1261/rna.2396011
Doherty, A. J., Jackson, S. P., and Weller, G. R. (2001). Identification of Bacterial Homologues of the Ku DNA Repair Proteins. FEBS Lett. 500, 186–188. doi:10.1016/s0014-5793(01)02589-3
Doolittle, W. F. (1999). Phylogenetic Classification and the Universal Tree. Science 284, 2124–2128. doi:10.1126/science.284.5423.2124
Doron, S., Melamed, S., Ofir, G., Leavitt, A., Lopatina, A., Keren, M., et al. (2018). Systematic Discovery of Antiphage Defense Systems in the Microbial Pangenome. Science 359, eaar4120. doi:10.1126/science.aar4120
Doulatov, S., Hodes, A., Dai, L., Mandhana, N., Liu, M., Deora, R., et al. (2004). Tropism Switching in Bordetella Bacteriophage Defines a Family of Diversity-Generating Retroelements. Nature 431, 476–481. doi:10.1038/nature02833
Drobysheva, A. V., Panafidina, S. A., Kolesnik, M. V., Klimuk, E. I., Minakhin, L., Yakunina, M. V., et al. (2021). Structure and Function of Virion RNA Polymerase of a crAss-like Phage. Nature 589, 306–309. doi:10.1038/s41586-020-2921-5
Duggal, N. K., and Emerman, M. (2012). Evolutionary Conflicts between Viruses and Restriction Factors Shape Immunity. Nat. Rev. Immunol. 12, 687–695. doi:10.1038/nri3295
Edgell, D. R., Chalamcharla, V. R., and Belfort, M. (2011). Learning to Live Together: Mutualism between Self-Splicing Introns and Their Hosts. BMC Biol. 9, 22. doi:10.1186/1741-7007-9-22
Ericson, C. F., Eisenstein, F., Medeiros, J. M., Malter, K. E., Cavalcanti, G. S., Zeller, R. W., et al. (2019). A Contractile Injection System Stimulates Tubeworm Metamorphosis by Translocating a Proteinaceous Effector. eLife 8, e46845. doi:10.7554/eLife.46845
Esterman, E. S., Wolf, Y. I., Kogay, R., Koonin, E. V., and Zhaxybayeva, O. (2021). Evolution of DNA Packaging in Gene Transfer Agents. Virus. Evol. 7, veab015. doi:10.1093/ve/veab015
Faure, G., Shmakov, S. A., Yan, W. X., Cheng, D. R., Scott, D. A., Peters, J. E., et al. (2019). CRISPR-cas in mobile Genetic Elements: Counter-defence and beyond. Nat. Rev. Microbiol. 17, 513–525. doi:10.1038/s41579-019-0204-7
Feschotte, C., and Gilbert, C. (2012). Endogenous Viruses: Insights into Viral Evolution and Impact on Host Biology. Nat. Rev. Genet. 13, 283–296. doi:10.1038/nrg3199
Figueroa-Bossi, N., Coissac, E., Netter, P., and Bossi, L. (1997). Unsuspected Prophage-like Elements in Salmonella typhimurium. Mol. Microbiol. 25, 161–173. doi:10.1046/j.1365-2958.1997.4451807.x
Filée, J., Forterre, P., Sen-Lin, T., and Laurent, J. (2002). Evolution of DNA Polymerase Families: Evidences for Multiple Gene Exchange between Cellular and Viral Proteins. J. Mol. Evol. 54, 763–773. doi:10.1007/s00239-001-0078-x
Filée, J., and Forterre, P. (2005). Viral Proteins Functioning in Organelles: a Cryptic Origin? Trends Microbiol. 13, 510–513.
Fontana, J., Nemecek, D., McHugh, C. A., Aksyuk, A. A., Cheng, N., Winkler, D. C., et al. (2014). Phage Capsid-like Structure of Myxococcus Xanthus Encapsulin, a Protein Shell that Stores Iron. Microsc. Microanal 20, 1244–1245. doi:10.1017/s1431927614007958
Fraikin, N., Goormaghtigh, F., and Van Melderen, L. (2020). Type II Toxin-Antitoxin Systems: Evolution and Revolutions. J. Bacteriol. 202. doi:10.1128/JB.00763-19
Freire, D. M., Gutierrez, C., Garza-Garcia, A., Grabowska, A. D., Sala, A. J., Ariyachaokun, K., et al. (2019). An NAD+ Phosphorylase Toxin Triggers Mycobacterium tuberculosis Cell Death. Mol. Cel 73, 1282–1291. doi:10.1016/j.molcel.2019.01.028
Frost, L. S., Leplae, R., Summers, A. O., and Toussaint, A. (2005). Mobile Genetic Elements: the Agents of Open Source Evolution. Nat. Rev. Microbiol. 3, 722–732. doi:10.1038/nrmicro1235
Gao, L., Altae-Tran, H., Bohning, F., Makarova, K. S., Segel, M., Schmidt-Burgk, J. L., et al. (2020). Diverse Enzymatic Activities Mediate Antiviral Immunity in Prokaryotes. Science. doi:10.1126/science.aba0372
Gentile, G. M., Wetzel, K. S., Dedrick, R. M., Montgomery, M. T., Garlena, R. A., Jacobs-Sera, D., et al. (2019). More Evidence of Collusion: a New Prophage-Mediated Viral Defense System Encoded by Mycobacteriophage Sbash. mBio 10. doi:10.1128/mBio.00196-19
Gerdes, K., Møller-Jensen, J., and Bugge Jensen, R. (2000). Plasmid and Chromosome Partitioning: Surprises from Phylogeny. Mol. Microbiol. 37, 455–466. doi:10.1046/j.1365-2958.2000.01975.x
Gerdes, K., Howard, M., and Szardenings, F. (2010). Pushing and Pulling in Prokaryotic DNA Segregation. Cell 141, 927–942. doi:10.1016/j.cell.2010.05.033
Gerstmeir, R., Cramer, A., Dangel, P., Schaffer, S., and Eikmanns, B. J. (2004). RamB, a Novel Transcriptional Regulator of Genes Involved in Acetate Metabolism of Corynebacterium Glutamicum. J. Bacteriol. 186, 2798–2809. doi:10.1128/jb.186.9.2798-2809.2004
Giessen, T. W., and Silver, P. A. (2017). Widespread Distribution of Encapsulin Nanocompartments Reveals Functional Diversity. Nat. Microbiol. 2, 17029. doi:10.1038/nmicrobiol.2017.29
Gladyshev, E. A., and Arkhipova, I. R. (2011). A Widespread Class of Reverse Transcriptase-Related Cellular Genes. Proc. Natl. Acad. Sci. 108, 20311–20316. doi:10.1073/pnas.1100266108
Golden, J. W., Carrasco, C. D., Mulligan, M. E., Schneider, G. J., and Haselkorn, R. (1988). Deletion of a 55-Kilobase-Pair DNA Element from the Chromosome during Heterocyst Differentiation of Anabaena Sp. Strain PCC 7120. J. Bacteriol. 170, 5034–5041. doi:10.1128/jb.170.11.5034-5041.1988
Gould, S. J. (1997). The Exaptive Excellence of Spandrels as a Term and Prototype. Proc. Natl. Acad. Sci. 94, 10750–10755. doi:10.1073/pnas.94.20.10750
Gould, S. J., and Vrba, E. S. (1982). Exaptation-a Missing Term in the Science of Form. Paleobiology 8, 4–15. doi:10.1017/s0094837300004310
Gray, M. W., Burger, G., Derelle, R., Klimeš, V., Leger, M. M., Sarrasin, M., et al. (2020). The Draft Nuclear Genome Sequence and Predicted Mitochondrial Proteome of Andalucia Godoyi, a Protist with the Most Gene-Rich and Bacteria-like Mitochondrial Genome. BMC Biol. 18, 22. doi:10.1186/s12915-020-0741-6
Guglielmini, J., de la Cruz, F., and Rocha, E. P. C. (2012). Evolution of Conjugation and Type IV Secretion Systems. Mol. Biol. Evol. 30, 315–331. doi:10.1093/molbev/mss221
Haase, J., Kalkum, M., and Lanka, E. (1996). TrbK, a Small Cytoplasmic Membrane Lipoprotein, Functions in Entry Exclusion of the IncP Alpha Plasmid RP4. J. Bacteriol. 178, 6720–6729. doi:10.1128/jb.178.23.6720-6729.1996
Haggård-Ljungquist, E., Barreiro, V., Calendar, R., Kurnit, D. M., and Cheng, H. (1989). The P2 Phage Old Gene: Sequence, Transcription and Translational Control. Gene 85, 25–33. doi:10.1016/0378-1119(89)90460-5
Handa, S., Jiang, Y., Tao, S., Foreman, R., Schinazi, R. F., Miller, J. F., et al. (2018). Template-assisted Synthesis of Adenine-Mutagenized cDNA by a Retroelement Protein Complex. Nucleic Acids Res. 46, 9711–9725. doi:10.1093/nar/gky620
Haraldsen, J. D., and Sonenshein, A. L. (2003). Efficient Sporulation in Clostridium difficile Requires Disruption of the σK Gene. Mol. Microbiol. 48, 811–821. doi:10.1046/j.1365-2958.2003.03471.x
Harms, A., Brodersen, D. E., Mitarai, N., and Gerdes, K. (2018). Toxins, Targets, and Triggers: An Overview of Toxin-Antitoxin Biology. Mol. Cel 70, 768–784. doi:10.1016/j.molcel.2018.01.003
Harshey, R. M. (2015). Transposable Phage Mu. In Mobile DNA III, 669–691. doi:10.1128/9781555819217.ch31
Hausner, G., Hafez, M., and Edgell, D. R. (2014). Bacterial Group I Introns: mobile RNA Catalysts. Mobile DNA 5, 8. doi:10.1186/1759-8753-5-8
Heidelberg, J. F., Eisen, J. A., Nelson, W. C., Clayton, R. A., Gwinn, M. L., Dodson, R. J., et al. (2000). DNA Sequence of Both Chromosomes of the Cholera Pathogen Vibrio cholerae. Nature 406, 477–483. doi:10.1038/35020000
Heussler, G. E., Cady, K. C., Koeppen, K., Bhuju, S., Stanton, B. A., and O'Toole, G. A. (2015). Clustered Regularly Interspaced Short Palindromic Repeat-dependent, Biofilm-specific Death of Pseudomonas aeruginosa Mediated by Increased Expression of Phage-Related Genes. mBio 6, e00129–15. doi:10.1128/mBio.00129-15
Hickman, A. B., Kailasan, S., Genzor, P., Haase, A. D., and Dyda, F. (2020). Casposase Structure and the Mechanistic Link between DNA Transposition and Spacer Acquisition by CRISPR-Cas. Elife 9. doi:10.7554/eLife.50004
Hickman, A. B., and Dyda, F. (2015). The Casposon-Encoded Cas1 Protein from Aciduliprofundum Boonei Is a DNA Integrase that Generates Target Site Duplications. Nucleic Acids Res. 43, 10576–10587. doi:10.1093/nar/gkv1180
Honarvar, S., Choi, B.-K., and Schifferli, D. M. (2003). Phase Variation of the 987P-like CS18 Fimbriae of Human Enterotoxigenic Escherichia coli Is Regulated by Site-specific Recombinases. Mol. Microbiol. 48, 157–171. doi:10.1046/j.1365-2958.2003.03419.x
Hoskisson, P. A., and Rigali, S. (2009). “Chapter 1 Variation in Form and Function,” in Advances in Applied Microbiology (Academic Press), 1–22. doi:10.1016/s0065-2164(09)69001-8
Hülter, N., Ilhan, J., Wein, T., Kadibalban, A. S., Hammerschmidt, K., and Dagan, T. (2017). An Evolutionary Perspective on Plasmid Lifestyle Modes. Curr. Opin. Microbiol. 38, 74–80. doi:10.1016/j.mib.2017.05.001
Ilyina, T. V., and Koonin, E. V. (1992). Conserved Sequence Motifs in the Initiator Proteins for Rolling circle DNA Replication Encoded by Diverse Replicons from Eubacteria, Eucaryotes and Archaebacteria. Nucl. Acids Res. 20, 3279–3285. doi:10.1093/nar/20.13.3279
Iranzo, J., Cuesta, J. A., Manrubia, S., Katsnelson, M. I., and Koonin, E. V. (2017). Disentangling the Effects of Selection and Loss Bias on Gene Dynamics. Proc. Natl. Acad. Sci. USA 114, E5616–E5624. doi:10.1073/pnas.1704925114
Iranzo, J., Puigbò, P., Lobkovsky, A. E., Wolf, Y. I., and Koonin, E. V. (2016). Inevitability of Genetic Parasites. Genome Biol. Evol. 8, 2856–2869. doi:10.1093/gbe/evw193
Iyer, L., Krishnan, A., Burroughs, A. M., Aravind, L., and Aravind, L. (2021). Jumbo Phages: A Comparative Genomic Overview of Core Functions and Adaptions for Biological Conflicts. Viruses 13, 63. doi:10.3390/v13010063
Iyer, L. M., Koonin, E. V., and Aravind, L. (2003). Evolutionary Connection between the Catalytic Subunits of DNA-dependent RNA Polymerases and Eukaryotic RNA-dependent RNA Polymerases and the Origin of RNA Polymerases. BMC Struct. Biol. 3, 1. doi:10.1186/1472-6807-3-1
Iyer, L. M., Koonin, E. V., and Aravind, L. (2002). Classification and Evolutionary History of the Single-Strand Annealing Proteins, RecT, Redβ, ERF and RAD52. BMC Genomics 3, 8. doi:10.1186/1471-2164-3-8
Iyer, L. M., Makarova, K. S., Koonin, E. V., and Aravind, L. (2004). Comparative Genomics of the FtsK-HerA Superfamily of Pumping ATPases: Implications for the Origins of Chromosome Segregation, Cell Division and Viral Capsid Packaging. Nucleic Acids Res. 32, 5260–5279. doi:10.1093/nar/gkh828
Jalasvuori, M., and Koonin, E. V. (2015). Classification of Prokaryotic Genetic Replicators: between Selfishness and Altruism. Ann. N.Y. Acad. Sci. 1341, 96–105. doi:10.1111/nyas.12696
Jiang, X., Hall, A. B., Arthur, T. D., Plichta, D. R., Covington, C. T., Poyet, M., et al. (2019). Invertible Promoters Mediate Bacterial Phase Variation, Antibiotic Resistance, and Host Adaptation in the Gut. Science 363, 181–187. doi:10.1126/science.aau5238
Johnson, R. C. (2015). “Site-specific DNA Inversion by Serine Recombinases,” in Mobile DNA III, 199–236. doi:10.1128/9781555819217.ch9
Jurėnas, D., Fraikin, N., Goormaghtigh, F., and Van Melderen, L. (2022). Biology and Evolution of Bacterial Toxin-Antitoxin Systems. Nat. Rev. Microbiol.
Kaiser, K., and Murray, N. E. (1979). Physical Characterisation of the “Rac Prophage” in E. coli K12. Mol. Gen. Genet. 175, 159–174. doi:10.1007/bf00425532
Kapitonov, V. V., Makarova, K. S., Koonin, E. V., and Zhulin, I. B. (2016). ISC, a Novel Group of Bacterial and Archaeal DNA Transposons that Encode Cas9 Homologs. J. Bacteriol. 198, 797–807. doi:10.1128/jb.00783-15
Karvelis, T., Druteika, G., Bigelyte, G., Budre, K., Zedaveinyte, R., Silanskas, A., et al. (2021). Transposon-associated TnpB Is a Programmable RNA-Guided DNA Endonuclease. Nature. doi:10.1038/s41586-021-04058-1
Knowles, B., Bailey, B., Boling, L., Breitbart, M., Cobián-Güemes, A., Del Campo, J., et al. (2017). Variability and Host Density independence in Inductions-Based Estimates of Environmental Lysogeny. Nat. Microbiol. 2, 17064. doi:10.1038/nmicrobiol.2017.64
Kobayashi, I. (2001). Behavior of Restriction-Modification Systems as Selfish mobile Elements and Their Impact on Genome Evolution. Nucleic Acids Res. 29, 3742–3756. doi:10.1093/nar/29.18.3742
Kobryn, K., Chaconas, G., Rice, P., and Craig, N. (2014). Hairpin Telomere Resolvases. Microbiol. Spectr. 2, 2–617. doi:10.1128/microbiolspec.MDNA3-0023-2014
Kobryn, K., and Chaconas, G. (2002). ResT, a Telomere Resolvase Encoded by the Lyme Disease Spirochete. Mol. Cel 9, 195–201. doi:10.1016/s1097-2765(01)00433-6
Kogay, R., Neely, T. B., Birnbaum, D. P., Hankel, C. R., Shakya, M., and Zhaxybayeva, O. (2019). Machine-Learning Classification Suggests that Many Alphaproteobacterial Prophages May Instead Be Gene Transfer Agents. Genome Biol. Evol. 11, 2941–2953. doi:10.1093/gbe/evz206
Kolodner, R., Hall, S. D., and Luisi-DeLuca, C. (1994). Homologous Pairing Proteins Encoded by the Escherichia Coii recE and recT Genes. Mol. Microbiol. 11, 23–30. doi:10.1111/j.1365-2958.1994.tb00286.x
Koonin, E. V., Dolja, V. V., Krupovic, M., Varsani, A., Wolf, Y. I., Yutin, N., et al. (2020). Global Organization and Proposed Megataxonomy of the Virus World. Microbiol. Mol. Biol. Rev. 84, e00061–00019. doi:10.1128/MMBR.00061-19
Koonin, E. V., and Krupovic, M. (2018). The Depths of Virus Exaptation. Curr. Opin. Virol. 31, 1–8. doi:10.1016/j.coviro.2018.07.011
Koonin, E. V., Makarova, K. S., and Aravind, L. (2001). Horizontal Gene Transfer in Prokaryotes: Quantification and Classification. Annu. Rev. Microbiol. 55, 709–742. doi:10.1146/annurev.micro.55.1.709
Koonin, E. V., and Makarova, K. S. (2022). Evolutionary Plasticity and Functional Versatility of CRISPR Systems. Plos Biol. 20, e3001481. doi:10.1371/journal.pbio.3001481
Koonin, E. V., and Makarova, K. S. (2017). Mobile Genetic Elements and Evolution of CRISPR-Cas Systems: All the Way There and Back. Genome Biol. Evol. 9, 2812–2825. doi:10.1093/gbe/evx192
Koonin, E. V., and Makarova, K. S. (2019). Origins and Evolution of CRISPR-Cas Systems. Phil. Trans. R. Soc. B 374, 20180087. doi:10.1098/rstb.2018.0087
Koonin, E. V., Makarova, K. S., and Wolf, Y. I. (2017). Evolutionary Genomics of Defense Systems in Archaea and Bacteria. Annu. Rev. Microbiol. 71, 233–261. doi:10.1146/annurev-micro-090816-093830
Koonin, E. V., Makarova, K. S., Wolf, Y. I., and Krupovic, M. (2019). Evolutionary Entanglement of mobile Genetic Elements and Host Defence Systems: Guns for Hire. Nat. Rev. Genet., 1–13. doi:10.1038/s41576-019-0172-9
Koonin, E. V. (2016). Viruses and mobile Elements as Drivers of Evolutionary Transitions. Phil. Trans. R. Soc. B 371, 20150442. doi:10.1098/rstb.2015.0442
Krishnan, A., Burroughs, A. M., Iyer, L. M., and Aravind, L. (2020). Comprehensive Classification of ABC ATPases and Their Functional Radiation in Nucleoprotein Dynamics and Biological Conflict Systems. Nucleic Acids Res. 48, 10045–10075. doi:10.1093/nar/gkaa726
Krupovic, M., Béguin, P., and Koonin, E. V. (2017). Casposons: mobile Genetic Elements that Gave Rise to the CRISPR-Cas Adaptation Machinery. Curr. Opin. Microbiol. 38, 36–43. doi:10.1016/j.mib.2017.04.004
Krupovic, M., and Koonin, E. V. (2017). Multiple Origins of Viral Capsid Proteins from Cellular Ancestors. Proc. Natl. Acad. Sci. USA 114, E2401–E2410. doi:10.1073/pnas.1621061114
Krupovic, M., Makarova, K. S., Forterre, P., Prangishvili, D., and Koonin, E. V. (2014). Casposons: a New Superfamily of Self-Synthesizing DNA Transposons at the Origin of Prokaryotic CRISPR-Cas Immunity. BMC Biol. 12, 36. doi:10.1186/1741-7007-12-36
Kutsukake, K., Nakashima, H., Tominaga, A., and Abo, T. (2006). Two DNA Invertases Contribute to Flagellar Phase Variation in Salmonella enterica Serovar Typhimurium Strain LT2. J. Bacteriol. 188, 950–957. doi:10.1128/jb.188.3.950-957.2006
Lambin, M., Nicolas, E., Oger, C. A., Nguyen, N., Prozzi, D., and Hallet, B. (2012). Separate Structural and Functional Domains of Tn4430 Transposase Contribute to Target Immunity. Mol. Microbiol. 83, 805–820. doi:10.1111/j.1365-2958.2012.07967.x
Lambowitz, A. M., Belfort, M., Lambowitz, A., and Craig, N. (2015). Mobile Bacterial Group II Introns at the Crux of Eukaryotic Evolution. Microbiol. Spectr. 3, MDNA3–2014. doi:10.1128/microbiolspec.MDNA3-0050-2014
Landy, A. (2015). “The λ Integrase Site-specific Recombination Pathway,” in Mobile DNA III, 91–118. doi:10.1128/9781555819217.ch4
Lang, A. S., Zhaxybayeva, O., and Beatty, J. T. (2012). Gene Transfer Agents: Phage-like Elements of Genetic Exchange. Nat. Rev. Microbiol. 10, 472–482. doi:10.1038/nrmicro2802
Le Bourgeois, P., Bugarel, M., Campo, N., Daveran-Mingot, M.-L., Labonté, J., Lanfranchi, D., et al. (2007). The Unconventional Xer Recombination Machinery of Streptococci/Lactococci. Plos Genet. 3, e117. doi:10.1371/journal.pgen.0030117
Lee, E. R., Baker, J. L., Weinberg, Z., Sudarsan, N., and Breaker, R. R. (2010). An Allosteric Self-Splicing Ribozyme Triggered by a Bacterial Second Messenger. Science 329, 845–848. doi:10.1126/science.1190713
Lee, J., and Herrin, D. L. (2003). Mutagenesis of a Light-Regulated psbA Intron Reveals the Importance of Efficient Splicing for Photosynthetic Growth. Nucleic Acids Res. 31, 4361–4372. doi:10.1093/nar/gkg643
LeGault, K. N., Hays, S. G., Angermeyer, A., McKitterick, A. C., Johura, F. T., Sultana, M., et al. (2021). Temporal Shifts in Antibiotic Resistance Elements Govern Phage-Pathogen Conflicts. Science 373, eabg2166. doi:10.1126/science.abg2166
Leiman, P. G., Basler, M., Ramagopal, U. A., Bonanno, J. B., Sauder, J. M., Pukatzki, S., et al. (2009). Type VI Secretion Apparatus and Phage Tail-Associated Protein Complexes Share a Common Evolutionary Origin. Proc. Natl. Acad. Sci. 106, 4154–4159. doi:10.1073/pnas.0813360106
Lennon, C. W., Stanger, M., and Belfort, M. (2016). Protein Splicing of a Recombinase Intein Induced by ssDNA and DNA Damage. Genes Dev. 30 (24), 2663–2668. doi:10.1101/gad.289280.116
Liebert, C. A., Hall, R. M., and Summers, A. O. (1999). Transposon Tn 21 , Flagship of the Floating Genome. Microbiol. Mol. Biol. Rev. 63, 507–522. doi:10.1128/mmbr.63.3.507-522.1999
Lin, C.-Y., Awano, N., Masuda, H., Park, J.-H., and Inouye, M. (2013). Transcriptional Repressor HipB Regulates the Multiple Promoters in Escherichia coli. J. Mol. Microbiol. Biotechnol. 23, 440–447. doi:10.1159/000354311
Livny, J., Yamaichi, Y., and Waldor, M. K. (2007). Distribution of Centromere-like parS Sites in Bacteria: Insights from Comparative Genomics. J. Bacteriol. 189, 8693–8703. doi:10.1128/jb.01239-07
Lossi, N. S., Manoli, E., Förster, A., Dajani, R., Pape, T., Freemont, P., et al. (2013). The HsiB1C1 (TssB-TssC) Complex of the Pseudomonas aeruginosa Type VI Secretion System Forms a Bacteriophage Tail Sheathlike Structure. J. Biol. Chem. 288, 7536–7548. doi:10.1074/jbc.m112.439273
Makarova, K. S., Wolf, Y. I., Alkhnbashi, O. S., Costa, F., Shah, S. A., Saunders, S. J., et al. (2015). An Updated Evolutionary Classification of CRISPR-Cas Systems. Nat. Rev. Microbiol. 13, 722–736. doi:10.1038/nrmicro3569
Makarova, K. S., Wolf, Y. I., Iranzo, J., Shmakov, S. A., Alkhnbashi, O. S., Brouns, S. J. J., et al. (2020). Evolutionary Classification of CRISPR-Cas Systems: a Burst of Class 2 and Derived Variants. Nat. Rev. Microbiol. 18, 67–83. doi:10.1038/s41579-019-0299-x
Makarova, K. S., Wolf, Y. I., and Koonin, E. V. (2013). Comparative Genomics of Defense Systems in Archaea and Bacteria. Nucleic Acids Res. 41, 4360–4377. doi:10.1093/nar/gkt157
Makarova, K. S., Wolf, Y. I., and Koonin, E. V. (2009). Comprehensive Comparative-Genomic Analysis of Type 2 Toxin-Antitoxin Systems and Related mobile Stress Response Systems in Prokaryotes. Biol. Direct 4, 19. doi:10.1186/1745-6150-4-19
McDonnell, G. E., and McConnell, D. J. (1994). Overproduction, Isolation, and DNA-Binding Characteristics of Xre, the Repressor Protein from the Bacillus Subtilis Defective Prophage PBSX. J. Bacteriol. 176, 5831–5834. doi:10.1128/jb.176.18.5831-5834.1994
McGeoch, A. T., and Bell, S. D. (2008). Extra-chromosomal Elements and the Evolution of Cellular DNA Replication Machineries. Nat. Rev. Mol. Cel Biol 9, 569–574. doi:10.1038/nrm2426
McKitterick, A. C., and Seed, K. D. (2018). Anti-phage Islands Force Their Target Phage to Directly Mediate Island Excision and Spread. Nat. Commun. 9, 2348. doi:10.1038/s41467-018-04786-5
Midonet, C., Barre, F. X., Rice, P., and Craig, N. (2014). Xer Site-specific Recombination: Promoting Vertical and Horizontal Transmission of Genetic Information. Microbiol. Spectr. 2, 2–629. doi:10.1128/microbiolspec.MDNA3-0056-2014
Midonet, C., Miele, S., Paly, E., Guerois, R., and Barre, F.-X. (2019). The TLCΦ Satellite Phage Harbors a Xer Recombination Activation Factor. Proc. Natl. Acad. Sci. USA 116, 18391–18396. doi:10.1073/pnas.1902905116
Miller, E. S., Kutter, E., Mosig, G., Arisaka, F., Kunisawa, T., and Rüger, W. (2003). Bacteriophage T4 Genome. Microbiol. Mol. Biol. Rev. 67, 86–156. doi:10.1128/mmbr.67.1.86-156.2003
Millman, A., Bernheim, A., Stokar-Avihail, A., Fedorenko, T., Voichek, M., Leavitt, A., et al. (2020). Bacterial Retrons Function in Anti-phage Defense. Cell 183, 1551–1561. doi:10.1016/j.cell.2020.09.065
Mosig, G., Yu, S., Myung, H., Haggård-Ljungquist, E., Davenport, L., Carlson, K., et al. (1997). A Novel Mechanism of Virus-Virus Interactions: Bacteriophage P2 Tin Protein Inhibits Phage T4 DNA Synthesis by Poisoning the T4 Single-Stranded DNA Binding Protein, Gp32. Virology 230, 72–81. doi:10.1006/viro.1997.8464
Nichols, R. J., LaFrance, B., Phillips, N. R., Radford, D. R., Oltrogge, L. M., Valentin-Alvarado, L. E., et al. (2021). Discovery and Characterization of a Novel Family of Prokaryotic Nanocompartments Involved in Sulfur Metabolism. eLife 10, e59288. doi:10.7554/elife.59288
Nicolas, E., Lambin, M., Dandoy, D., Galloy, C., Nguyen, N., Oger, C. A., et al. (2015). “The Tn3-Family of Replicative Transposons,” in Mobile DNA III, 693–726. doi:10.1128/9781555819217.ch32
Nilsson, A. S., Karlsson, J. L., and Haggård-Ljungquist, E. (2004). Site-Specific Recombination Links the Evolution of P2-like Coliphages and Pathogenic Enterobacteria. Mol. Biol. Evol. 21, 1–13. doi:10.1093/molbev/msg223
Nolan, L. M., Cain, A. K., Clamens, T., Furniss, R. C. D., Manoli, E., Sainz-Polo, M. A., et al. (2021). Identification of Tse8 as a Type VI Secretion System Toxin from Pseudomonas aeruginosa that Targets the Bacterial Transamidosome to Inhibit Protein Synthesis in Prey Cells. Nat. Microbiol. 6, 1199–1210. doi:10.1038/s41564-021-00950-8
Novick, R. P. (2019). Pathogenicity Islands and Their Role in Staphylococcal Biology. Microbiol. Spectr. 7, 2019. doi:10.1128/microbiolspec.GPP3-0062-2019
Novick, R. P., and Ram, G. (2017). Staphylococcal Pathogenicity Islands - Movers and Shakers in the Genomic Firmament. Curr. Opin. Microbiol. 38, 197–204. doi:10.1016/j.mib.2017.08.001
Novikova, O., Jayachandran, P., Kelley, D. S., Morton, Z., Merwin, S., Topilina, N. I., et al. (2016). Intein Clustering Suggests Functional Importance in Different Domains of Life. Mol. Biol. Evol. 33, 783–799. doi:10.1093/molbev/msv271
Nuñez, J. K., Kranzusch, P. J., Noeske, J., Wright, A. V., Davies, C. W., and Doudna, J. A. (2014). Cas1-Cas2 Complex Formation Mediates Spacer Acquisition during CRISPR-Cas Adaptive Immunity. Nat. Struct. Mol. Biol. 21, 528–534. doi:10.1038/nsmb.2820
Odegrip, R., Nilsson, A. S., and Haggård-Ljungquist, E. (2006). Identification of a Gene Encoding a Functional Reverse Transcriptase within a Highly Variable Locus in the P2-like Coliphages. J. Bacteriol. 188, 1643–1647. doi:10.1128/jb.188.4.1643-1647.2006
Ogura, T., and Hiraga, S. (1983). Partition Mechanism of F Plasmid: Two Plasmid Gene-Encoded Products and a Cis-Acting Region Are Involved in Partition. Cell 32, 351–360. doi:10.1016/0092-8674(83)90454-3
Owen, S. V., Wenner, N., Dulberger, C. L., Rodwell, E. V., Bowers-Barnard, A., Quinones-Olvera, N., et al. (2021). Prophages Encode Phage-Defense Systems with Cognate Self-Immunity. Cell Host & Microbe 29, 1620–1633. doi:10.1016/j.chom.2021.09.002
Page, R., and Peti, W. (2016). Toxin-antitoxin Systems in Bacterial Growth Arrest and Persistence. Nat. Chem. Biol. 12, 208–214. doi:10.1038/nchembio.2044
Pasechnek, A., Rabinovich, L., Stadnyuk, O., Azulay, G., Mioduser, J., Argov, T., et al. (2020). Active Lysogeny in Listeria Monocytogenes Is a Bacteria-Phage Adaptive Response in the Mammalian Environment. Cel Rep. 32, 107956. doi:10.1016/j.celrep.2020.107956
Paul, B. G., Burstein, D., Castelle, C. J., Handa, S., Arambula, D., Czornyj, E., et al. (2017). Retroelement-guided Protein Diversification Abounds in Vast Lineages of Bacteria and Archaea. Nat. Microbiol. 2, 17045. doi:10.1038/nmicrobiol.2017.45
Pell, L. G., Kanelis, V., Donaldson, L. W., Lynne Howell, P., and Davidson, A. R. (2009). The Phage Major Tail Protein Structure Reveals a Common Evolution for Long-Tailed Phages and the Type VI Bacterial Secretion System. Proc. Natl. Acad. Sci. 106, 4160–4165. doi:10.1073/pnas.0900044106
Randich, A. M., Kysela, D. T., Morlot, C., and Brun, Y. V. (2019). Origin of a Core Bacterial Gene via Co-option and Detoxification of a Phage Lysin. Curr. Biol. 29, 1634–1646. doi:10.1016/j.cub.2019.04.032
Real, G., Pinto, S. M., Schyns, G., Costa, T., Henriques, A. O., and Moran, C. P. (2005). A Gene Encoding a Holin-like Protein Involved in Spore Morphogenesis and Spore Germination in Bacillus Subtilis. J. Bacteriol. 187, 6443–6453. doi:10.1128/jb.187.18.6443-6453.2005
Rice, P. A., and Craig, N. (2015). Serine Resolvases. Microbiol. Spectr. 3, MDNA3–201422. doi:10.1128/microbiolspec.MDNA3-0045-2014
Rimer, J., Cohen, I. R., and Friedman, N. (2014). Do all Creatures Possess an Acquired Immune System of Some Sort? Bioessays 36, 273–281. doi:10.1002/bies.201300124
Roux, S., Paul, B. G., Bagby, S. C., Nayfach, S., Allen, M. A., Attwood, G., et al. (2021). Ecology and Molecular Targets of Hypermutation in the Global Microbiome. Nat. Commun. 12, 3076. doi:10.1038/s41467-021-23402-7
Russell, A. B., Peterson, S. B., and Mougous, J. D. (2014). Type VI Secretion System Effectors: Poisons with a Purpose. Nat. Rev. Microbiol. 12, 137–148. doi:10.1038/nrmicro3185
Saier, M. H., Reddy, B. L., and Margolin, W. (2015). Holins in Bacteria, Eukaryotes, and Archaea: Multifunctional Xenologues with Potential Biotechnological and Biomedical Applications. J. Bacteriol. 197, 7–17. doi:10.1128/jb.02046-14
Scott, J., Thompson-Mayberry, P., Lahmamsi, S., King, C. J., and McShan, W. M. (2008). Phage-associated Mutator Phenotype in Group A streptococcus. J. Bacteriol. 190, 6290–6301. doi:10.1128/jb.01569-07
Shabalina, S., and Koonin, E. (2008). Origins and Evolution of Eukaryotic RNA Interference. Trends Ecol. Evol. 23, 578–587. doi:10.1016/j.tree.2008.06.005
Shakya, M., Soucy, S. M., and Zhaxybayeva, O. (2017). Insights into Origin and Evolution of α-proteobacterial Gene Transfer Agents. Virus. Evol. 3, vex036. doi:10.1093/ve/vex036
Shmakov, S., Abudayyeh, O. O., Makarova, K. S., Wolf, Y. I., Gootenberg, J. S., Semenova, E., et al. (2015). Discovery and Functional Characterization of Diverse Class 2 CRISPR-Cas Systems. Mol. Cel 60, 385–397. doi:10.1016/j.molcel.2015.10.008
Shutt, T. E., and Gray, M. W. (2006). Twinkle, the Mitochondrial Replicative DNA Helicase, Is Widespread in the Eukaryotic Radiation and May Also Be the Mitochondrial DNA Primase in Most Eukaryotes. J. Mol. Evol. 62, 588–599. doi:10.1007/s00239-005-0162-8
Silas, S., Makarova, K. S., Shmakov, S., Páez-Espino, D., Mohr, G., Liu, Y., et al. (2017). On the Origin of Reverse Transcriptase-Using CRISPR-Cas Systems and Their Hyperdiverse, Enigmatic Spacer Repertoires. mBio 8 17. doi:10.1128/mBio.00897-17
Simon, M., Zieg, J., Silverman, M., Mandel, G., and Doolittle, R. (1980). Phase Variation: Evolution of a Controlling Element. Science 209, 1370–1374. doi:10.1126/science.6251543
Skjerning, R. B., Senissar, M., Winther, K. S., Gerdes, K., and Brodersen, D. E. (2019). The RES Domain Toxins of RES-Xre Toxin-Antitoxin Modules Induce Cell Stasis by Degrading NAD+. Mol. Microbiol. 111, 221–236. doi:10.1111/mmi.14150
Smyshlyaev, G., Bateman, A., and Barabas, O. (2021). Sequence Analysis of Tyrosine Recombinases Allows Annotation of mobile Genetic Elements in Prokaryotic Genomes. Mol. Syst. Biol. 17, e9880. doi:10.15252/msb.20209880
Soo, V. W. C., and Wood, T. K. (2013). Antitoxin MqsA Represses Curli Formation through the Master Biofilm Regulator CsgD. Sci. Rep. 3, 3186. doi:10.1038/srep03186
Stellwagen, A. E., and Craig, N. L. (1997). Gain-of-Function Mutations in TnsC, an ATP-dependent Transposition Protein that Activates the Bacterial Transposon Tn7. Genetics 145, 573–585. doi:10.1093/genetics/145.3.573
Sternberg, S. H., Richter, H., Charpentier, E., and Qimron, U. (2016). Adaptation in CRISPR-Cas Systems. Mol. Cel 61, 797–808. doi:10.1016/j.molcel.2016.01.030
Sun, D., and Setlow, P. (1993). Cloning and Nucleotide Sequence of the Bacillus Subtilis ansR Gene, Which Encodes a Repressor of the Ans Operon Coding for L-Asparaginase and L-Aspartase. J. Bacteriol. 175, 2501–2506. doi:10.1128/jb.175.9.2501-2506.1993
Tolias, P. P., and Dubow, M. S. (1986). The Overproduction and Characterization of the Bacteriophage Mu Regulatory DNA-Binding Protein Ner. Virology 148, 298–311. doi:10.1016/0042-6822(86)90327-2
Tominaga, A. (1997). The Site-specific Recombinase Encoded by pinD in Shigella dysenteriae Is Due to the Presence of a Defective Mu Prophage. Microbiology 143, 2057–2063. doi:10.1099/00221287-143-6-2057
Toyofuku, M., Cárcamo-Oyarce, G., Yamamoto, T., Eisenstein, F., Hsiao, C.-C., Kurosawa, M., et al. (2017). Prophage-triggered Membrane Vesicle Formation through Peptidoglycan Damage in Bacillus Subtilis. Nat. Commun. 8, 481. doi:10.1038/s41467-017-00492-w
Trouillon, J., Sentausa, E., Ragno, M., Robert-Genthon, M., Lory, S., Attrée, I., et al. (2020). Species-specific Recruitment of Transcription Factors Dictates Toxin Expression. Nucleic Acids Res. 48, 2388–2400. doi:10.1093/nar/gkz1232
Twarock, R., and Luque, A. (2019). Structural Puzzles in Virology Solved with an Overarching Icosahedral Design Principle. Nat. Commun. 10, 4414. doi:10.1038/s41467-019-12367-3
Vallota-Eastman, A., Arrington, E. C., Meeken, S., Roux, S., Dasari, K., Rosen, S., et al. (2020). Role of Diversity-Generating Retroelements for Regulatory Pathway Tuning in Cyanobacteria. BMC Genomics 21, 664. doi:10.1186/s12864-020-07052-5
Walker, D. M., and Harshey, R. M. (2020). Deep Sequencing Reveals New Roles for MuB in Transposition Immunity and Target-Capture, and Redefines the Insular Ter Region of E. coli. Mobile DNA 11, 26. doi:10.1186/s13100-020-00217-9
Weightman, A. J., Topping, A. W., Hill, K. E., Lee, L. L., Sakai, K., Slater, J. H., et al. (2002). Transposition of DEH , a Broad-Host-Range Transposon Flanked by IS Ppu12 , in Pseudomonas Putida Is Associated with Genomic Rearrangements and Dehalogenase Gene Silencing. J. Bacteriol. 184, 6581–6591. doi:10.1128/jb.184.23.6581-6591.2002
Weller, G. R., Kysela, B., Roy, R., Tonkin, L. M., Scanlan, E., Della, M., et al. (2002). Identification of a DNA Nonhomologous End-Joining Complex in Bacteria. Science 297, 1686–1689. doi:10.1126/science.1074584
Werren, J. H. (2011). Selfish Genetic Elements, Genetic Conflict, and Evolutionary Innovation. Proc. Natl. Acad. Sci. 108, 10863–10870. doi:10.1073/pnas.1102343108
Wu, L., Gingery, M., Abebe, M., Arambula, D., Czornyj, E., Handa, S., et al. (2017). Diversity-generating Retroelements: Natural Variation, Classification and Evolution Inferred from a Large-Scale Genomic Survey. Nucleic Acids Res. 46, 11–24. doi:10.1093/nar/gkx1150
Keywords: mobile genetic element, exaptation, antivirus defense mechanisms, biological conflict systems, horizontal gene transfer
Citation: Benler S and Koonin EV (2022) Recruitment of Mobile Genetic Elements for Diverse Cellular Functions in Prokaryotes. Front. Mol. Biosci. 9:821197. doi: 10.3389/fmolb.2022.821197
Received: 24 November 2021; Accepted: 08 February 2022;
Published: 24 March 2022.
Edited by:
Marlene Belfort, Albany State University, United StatesReviewed by:
Sean D Colloms, University of Glasgow, United KingdomBlair Gordon Paul, Marine Biological Laboratory (MBL), United States
Copyright © 2022 Benler and Koonin. This is an open-access article distributed under the terms of the Creative Commons Attribution License (CC BY). The use, distribution or reproduction in other forums is permitted, provided the original author(s) and the copyright owner(s) are credited and that the original publication in this journal is cited, in accordance with accepted academic practice. No use, distribution or reproduction is permitted which does not comply with these terms.
*Correspondence: Eugene V. Koonin, a29vbmluQG5jYmkubmxtLm5paC5nb3Y=