- 1The Affiliated Hospital of Kunming University of Science and Technology, Department of Neurosurgery, The First People's Hospital of Yunnan Province, Kunming, Yunnan, China
- 2Department of Neurosurgery, The First People’s Hospital of Yunnan Province, The Affiliated Hospital of Kunming University of Science and Technology, Kunming, Yunnan, China
Ferroptosis is an emerging form of cell death characterized by the over-accumulation of iron-dependent lipid peroxidation. Ferroptosis directly or indirectly disturbs glutathione peroxidases cycle through diverse pathways, impacting the cellular antioxidant capacities, aggravating accumulation of reactive oxygen species in lipid, and it finally causes oxidative overload and cell death. Ferroptosis plays a significant role in the pathophysiological processes of many diseases. Glioblastoma is one of the most common primary malignant brain tumors in the central nervous system in adults. Although there are many treatment plans for it, such as surgical resection, radiotherapy, and chemotherapy, they are currently ineffective and the recurrent rate is almost up to 100%. The therapies abovementioned have a strong relationship with ferroptosis at the cellular and molecular level according to the results reported by numerous researchers. The regulation of ferroptosis can significantly determine the outcome of the cells of glioblastoma. Thus ferroptosis, as a regulated form of programed cell death, has the possibility for treating glioblastoma.
Introduction
Ferroptosis is an emerging form of regulatable programed cell death compared with apoptosis, necroptosis, and pyroptosis [(Zhang et al., 2021a); (Shi et al., 2022a); (Zhou et al., 2019); (Bertheloot et al., 2021); (Li and Huang, 2022)]. In 2012, Dixon et al. named this regulatable cell death mode which could be inhibited by iron chelating agent as iron death on the basis of summarizing previous studies on cell deaths (Dixon et al., 2012). It is a metabolic process (Xu et al., 2021), not an active one that requires transcription induction or post-translational modification by specific death effectors in response to lethal stimuli. On the contrary, it is considered to be part of cell destruction or cell clearance.
Different from the previously described cell death modes, ferroptosis showed necrosis-like morphology: shrunk mitochondria, increased membrane density and narrowed crista, and ruptured cell membrane, whereas morphological changes were not evident in the nucleus; this kind of programmed cell death is initiated by abnormal metabolism of iron ions in cells, which causes the breakdown of the steady state of oxidoreduction reaction. The inhibition of the synthesis of reduced glutathione (GSH) and the intensification of oxidation reaction are the main causes of ferroptosis. A series of reactions that cause ferroptosis are iron dependent, triggered by intracellular changes in iron metabolism, leading to lipid peroxidation and finally cell death. (Lu et al., 2017; Mou et al., 2020; Aguilera et al., 2022). This mechanism is involved in pathophysiological conditions (Tang et al., 2018), including inflammation, tissue injuries, and cancerous transformation (Zhang et al., 2021a), among which glioblastoma (GBM) is one.
GBM, known for its devastating progression and dismal prognosis (Martínez-Garcia et al., 2018), is the most common primary malignant brain tumor in adults and accounts for over 50% of all high-grade gliomas (Martínez-Garcia et al., 2018). Its main characteristics are high malignancy, aggressive invasion, frequent brain or spinal cord metastasis and inevitable recurrence (Gu et al., 2016). According to the WHO grading system, GBM is classified as a Grade IV astrocytoma consisting of mostly low differentiated neoplastic astrocytes mixed with cells having different extent of differentiation (Ou-Yang et al., 2018) This is mainly due to the coexistence of multiple tumor cell populations with different degrees of differentiation, especially tumor cells that showing stem cell-like characteristics (Wang et al., 2019). Glioma stem cells have pretty strong renewal ability (Adamo et al., 2017), low differentiation, and high resistance to radiation and chemotherapy [(Broadley et al., 2011); (Sesé et al., 2022); (Liu et al., 2017)]. At present, there is no effective drug to treat it efficaciously, and surgery can only resect part of the tumor if it locates at functional or cranial base areas. Even if it can be removed completely with a negative intraoperative pathological edge, it virtually recurs through the mechanism we have not known yet. Therefore, the treatment scheme for recurrence can only remove the tumor to the maximum extent, and then carry out subsequent adjuvant chemotherapy and radiotherapy to destroy the tumor cells and inhibit the growth of tumor cells as much as possible (Berardinelli et al., 2019). However, the residual tumor cells are very easy to generate tolerance to radiotherapy and chemotherapy, and thus the recurrence rate of the tumor is as high as 100% [(Chanez et al., 2022); (Dissaux et al., 2021)]. After a series of changes in the internal structure of recurrent tumor cells, clonal evolution trees showed higher heterogeneity (Yang et al., 2020), and their malignancy is higher than the first time; that may be the reason why now no clinically available methods can effectively treat it. The average survival time of GBM patients after diagnosis is 12–15 months (Shi et al., 2015). It is urgent to develop molecular targeted drugs that are capable of effectively controlling the growth of GBM tumor cells based on their metabolic pathways.
Although few studies on the relationship between ferroptosis and GBM have been reported, especially using ferroptosis as a treatment or/and diagnostic method and the exploration of the relevant mechanism on cell death, recurrence and drug resistance between the two. We believe it is valuable to discuss this issue and it is what we attempted to deliver through this review.
Iron metabolism affects the ferroptosis
Iron is a vital element for the survival of organisms. It is indispensable for the metabolism of many substances in the body [(Dutt et al., 2022); (Stallhofer et al., 2022)]. Iron in the digestive tract is mainly absorbed in the duodenum and upper jejunum in the form of Fe2+ (Karaskova et al., 2021). Then, in the epithelial cells of small intestinal mucosa, Fe2+ is oxidized to Fe3+, and part of Fe3+ in the blood is further bound to the transported by transferrin (TF) and receptor (TFR) on the cell membrane and transported into the cell [(Piskin et al., 2022); (Zhao et al., 2022)], which reacts with the metal reductase Six-transmembrane epithelial antigen of prostate 3 (STEAP3) in the endoplasmic reticulum to form Fe2+; Fe2+ is transported to cells through transferrin receptor protein 1 (TFR1). The body regulates the storage of iron ions through the expression of ferritin and its related genes according to its own needs, such as ferritin heavy chain 1 (FTH1), ferritin light chain (FTL) and heat shock protein B1 (HSPB1) (Yang et al., 2022a); HSPB1 inhibits the expression of TFR1, thereby reducing the intracellular iron concentration. Therefore, overexpression of HSPB1 will inhibit ferroptosis [(Yuan et al., 2022); (Liu et al., 2021)]. In addition, the expression of FTL and FTH1 is regulated by iron responsive element binding protein 2 (IREBP2), and the overexpression of IREBP2 will inhibit iron death induced by erastin as well. The biological toxicity of iron ions is mediated by Fenton reaction, which transforms Fe2+ into Fe3+ and generates hydroxyl radicals, oxidative proteins et cetera (Zhang et al., 2022a; Gong et al., 2022). Knockout of Tf gene or down-regulation of TF can inhibit iron overload and cell apoptosis. Autophagy can regulate the amount of transferrin and lipid in cells to regulate iron metabolism, and ultimately affect iron death sensitivity, while ferritin selective autophagy can also regulate fine ferroptosis sensitivity. Other proteins may as well affect iron sensitivity in iron metabolism (Zhu and Fan, 2021) (Figure 1).
Lipid metabolism and reactive oxygen species accumulation regulate ferroptosis
Under normal circumstances, the substance in the organism is in a dynamic change process. When the body is disturbed by external or internal irresistible stimulus, the balance of homeostasis will be impaired (Badr et al., 2020). For example, oxidoreduction reaction disbalance and production of reactive oxygen species (ROS) in GBM (Reichert et al., 2020), the normal physiological conditions are disturbed, and the antioxidant capacity of cells will be relatively reduced (Burić et al., 2019; McNamee et al., 2021). A series of chain reactions of peroxidation will lead to the accumulation of lipid peroxidation in cells. Lipid peroxidation is a key step in ferroptosis (Lee and Roh, 2022), which can be divided into the synthesis of phospholipids with polyunsaturated fatty acids (PUFAs) as the substrate and the two peroxidation reactions. PUFAs are the key substances in lipid peroxidation (Yang et al., 2016). Firstly, PUFA.coenzyme A (PUFA.CoA) was synthesized with PUFAs under the action of acetyl CoA synthetase long-chain family member 4 (ACSL4) and then PUFA-phospholipids (PUFA-PL) was generated under the action of lysophospholipidacyltransferase 3 (LPCAT3) and incorporated into the cell components. PUFA-PL will participate in the next series of chain reactions as the substrate of peroxidation. Next, it can be divided into two ways to generate free radicals with strong oxidation capacity. Fenton reaction and lipoxygenase pathway will generate a large number of hydroxyl radicals and oxygen radicals (Xing et al., 2021). These free radicals will attack PUFA-PL, forming a cycle of peroxidation. Because cells are in a state of imbalance, this reaction will be terminated only when the substrate is exhausted. As one of the components of cells, its large consumption will inevitably lead to the damage of cell structure. In the lipoxygenase pathway (Tomita et al., 2019), 15-lipoxygenase (15-LOX) first combines with phosphatidylethanolamine binding protein 1 (PEBP1), and then oxidizes PUFA on the cell membrane to PUFA.phosphatidylethanolamines-OOH (PUFA.PE-OOH), thus causing ferroptosis (Kagan et al., 2020; Anthonymuthu et al., 2021; Mikulska-Ruminska et al., 2021). In addition to the end-products that will destroy the normal structure of cells and generate lipid peroxidation (Chng et al., 2021), the above peroxidation reactions will also produce some cytotoxic active aldehydes, which will attack phospholipids, proteins and even nucleic acids in cells (Gao et al., 2018; Liu et al., 2020; Zhao et al., 2020). NF-E2-relatedfactor2 (NRF2) plays an important role in regulating the homeostasis of cells and a key factor in oxidoreduction reaction (Zhang et al., 2016; Fan et al., 2017). Relevant studies have shown that when NRF2 is up-regulated in tumors, it is closely related to the poor prognosis of primary malignant brain tumors (Chew et al., 2021); the NRF2-Keap1 system regulates the expression of human antioxidant proteins, which is a typical antioxidant reaction pathway. Fe2+ is regulated by NRF2. Normally, it is inactive. When the intracellular peroxide increases, it will stimulate NRF2 to activate the downstream antioxidant enzymes to inhibit the oxidation reaction. Glutathione peroxidase 4 (GPX4) is also a key factor in the antioxidant system, which converts the lipid peroxide into non-toxic aliphatic alcohol and reduces H2O2 (Shin et al., 2018; Wu et al., 2022).
Lipid peroxidation in ferroptosis is regulated by several regulatory axes. Among them, GPX4 is recognized as the key regulatory target (Hayashima and Katoh, 2022). GSH and GPX4 in the signal axis of cystine/glutathione/glutathione peroxidase 4 can associate with each other to reduce PUFA-PL-OOH to PUFA-PL-OH, resultantly preventing the continuation of ferroptosis (Li et al., 2022a). Cystine is one of the components of glutathione, which requires the reverse transporter system Xc- to transport from outside the cell. Therefore, this transporter is also an important site for regulating ferroptosis. The signal axis of ferroptosis suppressor protein 1 (FSP1) and coenzyme Q10 (CoQ10) is also one of the regulatory sites of ferroptosis. When the GPX4 system is lacking (Doll et al., 2019), the increased expression of FSP1 can up-regulate the NADH dependent CoQ10 reduction reaction, so that the oxygen free radicals in the peroxidation cycle reaction caused by it are reduced to prevent ferroptosis (Santoro, 2020; Yang et al., 2022b). GTP cyclohydrolase 1 (GCH1)/tetrahydrobiopterin (BH4)/dihydrofolate reductase (DHFR) signal axis, in which the overexpression of GCH1 can selectively protect membrane phospholipids with two PUFA tails from peroxidation, preventing ferroptosis from occurring as well (Hu et al., 2022; Jiang et al., 2022) (Figure 1).
Immunotherapy for glioblastoma
Iron is pivotal for cell survival. Cancer cells are more prone to undergo ferroptosis than normal cells, and oxidized membrane lipids on ferroptotic cells can mediate the phagocytosis of macrophages to keep immune response. In addition, immune detection point inhibitor treatment may make cancer cells sensitive to ferroptosis (Shi et al., 2022b), it is expected to overcome chemical resistance and strengthen the death of immunogenicity cells. Enhanced ferroptosis was shown to induce activation and infiltration of immune cells, but to weaken the cytotoxicity of anti-tumor cells. It was found that tumor-associated macrophages were involved in ferroptosis-mediated immunosuppression (Liu et al., 2022a), which provides a new vision for immunotherapy for GBM (Yu et al., 2022).
Inhibition of asparagine-linked glycosylation 3 (ALG3) stimulates cancer cell immunogenic ferroptosis to potentiate immunotherapy. ALG3 is an a-1, 3-mannosyltransferase involved in protein glycosylation in the endoplasmic reticulum. Liu et al. reported that inhibition of ALG3 would induce defects in post-translational N-linked glycosylation modification and lead to excessive lipid accumulation through sterol regulatory element binding proteins (SREBPs)-dependent adipogenesis in cancer cells. Lipid peroxidation mediated by N-linked glycosylation deficiency induces the immunogenic ferroptosis of cancer cells and promotes the pro-inflammatory microenvironment, thus enhancing the anti-tumor immune response (Liu et al., 2022b).
Ir(III) complex containing a ferrocene-modified diphosphine ligand that localizes in lysosomes. Under the acidic environments of lysosomes, Ir(III) can effectively catalyze a Fenton-like reaction, produce hydroxyl radicals, induce lipid peroxidation, down-regulate GPX4, resulting in ferroptosis, and thus inhibit tumor cell growth (Wang et al., 2022).
In recent years, immunotherapy has raised fervent attention. One strategy is to activate lymphocytes that can recognize the tumor cells specifically and induce the cancer cells’ death by releasing perforin and granzyme. Accordingly, relevant studies pointed out that active CD8+ T cells release IFNγ that down-regulate the expression of Solute carrier (SLC) family 7 member 11 (SLC7A11) and SLC family 3 member 2 (SLC3A2), thereby inhibiting the absorption of cystine and enhancing lipid peroxidation and ferroptosis of tumor cells (Figure 2).
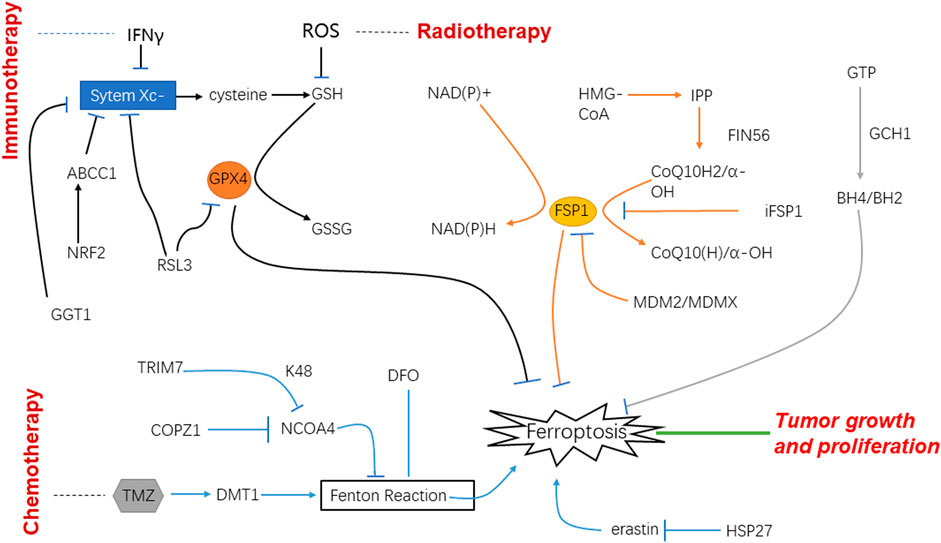
FIGURE 2. Schematic of mechanisms of ferroptosis and glioblastoma under the treatment of immunotherapy, radiotherapy and chemotherapy.
Radiotherapy for glioblastoma
Ionizing radiation can produce a lot of free radicals in irradiated cells. These free radicals are the factors that cause side effects when ionizing radiation is used to treat diseases. Among them, lipid peroxidation is an important influence of ionizing radiation on cell membranes, which is one of the key targets of radiation. On the other hand, lipid peroxidation is an important metabolic pathway for ferroptosis. Therefore, the effect of ionizing radiation on tumor cells should be related to the ferroptotic process to a large extent (Agrawal and Kale, 2001). It was found that after radiotherapy, the tumor cells showed typical morphological changes of ferroptosis such as changed density of mitochondria, shrunk cell membranes, and decreased mitochondrial cristae. It indicates that radiotherapy can increase the expression of key enzymes by generating a large number of ROS, thus promoting lipid peroxidation, and ultimately leading to ferroptosis (Zhang et al., 2022b). CuS@mSiO2@MnO2 nanocomposite, as a radiosensitizer that can effectively exhaust GSH was used in vitro; it uses up GSH and induces ferroptosis and apoptosis. The experiment performed in vivo also showed that tumor cells were damaged. Therefore, it is speculated that radiotherapy may induce ferroptosis after the depletion of GSH to play its role in the treatment of tumors (Li et al., 2021) (Figure 2).
Chemotherapy for glioblastoma
Temozolomide (TMZ) is the first-line drug for clinical chemotherapy for GBM in recent decades. Although it is the most effective drug for GBM for now, resistance to it especially in recurrent GBM can be noted very common. Drug resistance is one of the most significant factors which bring about a poor prognosis. NRF2 is an important transcription factor involved in chemotherapy resistance, and it can play a key role in inducing ferroptosis through GSH regulation. According to the experimental data, high-level of NRF2 leads to the resistance to TMZ therapy for GBM through the up-regulation of ATP-binding cassette subfamily C member 1 (ABCC1) which is the target of NRF2 and able to antagonize ferroptosis. For this rationale, ferroptosis induction may be an important treatment strategy to reverse the drug resistance of GBM with high NRF2 and ABCC1 expression (de Souza et al., 2022). Most chemotherapy medicines exert efficacy on tumor cells through signaling transduction which are bound up with oxygen-free radicals and ROS; under the circumstance, ferroptosis will play important role in the therapy of malignant tumors. Nowadays, TMZ is a new medicine for GBM and considered the most effective one, and thus widely used for patients in clinical practice (Zhou and Ma, 2022). There have been in vitro studies using TG905 cells showing that the divalent metal transporter 1 (DMT1) and ROS in GBM increases when treated with TMZ, whereas GPX4 is decreased (Su et al., 2022), in the meantime it observes an iron-independent cells death. Thus, we have good reason to believe that TMZ can induce the ferroptosis by targeting the expression of DMT1 in GBM cells and inhibiting tumor cells growth (Song et al., 2021).
It is reported that heat shock protein 27 (HSP27) is the new regulator of ferroptosis in tumor cells (Liu et al., 2021); GBM cells are protected by overexpression of HSP27 to escape from ferroptosis that is induced by erastin (Yuan et al., 2022). Therefore, HSP27 can be a regulatory spot of ferroptosis and used as a potential therapy target for GBM.
Clinical studies have shown that programmed cell death one (PD-1)/programmed cell death ligand one (PD-L1) have low efficacy on GBM due to low immunogenicity. In the experiment, Fe3O4-siPD-L1@M-BV2 increases the siPD-L1 and Fe2+ of the drug-resistant GBM in mice tissue. Fe3O4-siPD-L1@M-BV2 is associated with ferroptosis and immune activation, inhibiting the growth of drug-resistant GBM (Liu et al., 2022c).
Chen et al. reported that IONP@PTX can inhibit cell migration and invasion after incubating with cells. They made the conclusion that the level of iron ions, ROS and lipid peroxidation in cells is increased, suggesting that IONP@PTX might affect GBM through ferroptosis. More importantly, the influence of IONP@PTX on GBM can be regulated by other external factors such as 3-methyladenine (3-MA) and rapamycin. Another advantage is that IONP@PTX has no obvious toxic effect on GBM xenotransplantation mice, which may make IONP@PTX a potential treatment for GBM with high safety based on ferroptosis (Chen and Wen, 2022).
Some scholars reported that NF-κB activation protein (NKAP) knockout will increase the level of lipid peroxidation in naive T cells and induce cell death in colon cancer cells. Another experiment showed the consistent results that knockout of NKAP induces the death of GBM; silencing NKAP increases the sensitivity of cells to iron death inducers, and exogenous overexpression of NAKP can positively regulate an iron death defense protein, SLC7A11 to reduce the sensitivity of cells to iron death inducers. RNA and protein immunoprecipitation can prove that NKAP and N6-methyladenosine (m6A) interact on SLC7A11 transcription. Thus, inhibiting NKAP expression or knocking out its gene will increase the level of ferroptosis in cells, and may become a potential therapeutic direction to the treatment of GBM (Sun et al., 2022).
Ferroptosis is mainly caused by an imbalance of ROS and lipid peroxidation. One of the processes of lipid peroxidation can be inhibited by GPX4. In consequence, blocking the expression of GPX4 or reducing its production will greatly increase the degree of ferroptosis. γ-glutamine transferase 1 (GGT1) can inhibit the formation of the substrate in the process of synthesizing GPX4. Given this, GGT inhibitor is a potential treatment scheme for GBM, and it will be more effective if it is supplemented with iron death inducers (Hayashima and Katoh, 2022).
Based on the analysis of existing data, the overexpression of coatomer protein complex subunit zeta 1 (COPZ1) is related to the increase in tumor grade and poor prognosis of GBM patients. Via immunohistochemistry and western blot analysis, it is noted that the expression of COPZ1 protein in GBM was significantly higher than that in normal tissues. Knockout of Copz1 gene using iRNA could inhibit the growth of GBM in vitro, but also lead to a series of intracellular metabolic disorders, including the imbalance of iron metabolism. Therefore, COPZ1 can be a key regulatory point of ferroptosis, and become a potential therapeutic target for GBM (Zhang et al., 2021b).
Dihydroartemisinin (DHA) has the advantages of selective cytotoxicity and low drug resistance. These characteristics make DHA one of the new research directions of anti-tumor therapy. Through the effect of DHA on normal cells and GBM, it is found that GPX4 in tumor cells is significantly reduced, ROS and peroxidative lipids are increased, and these effects can be reversed by using ferroptosis inhibitors, which demonstrates DHA can inhibit tumor growth by changing the intracellular GPX4 to cause ferroptosis. DHA is one of the potential drugs for GBM (Yi et al., 2020) (Figure 2).
Ferroptosis in glioblastoma
The main component of brain tissue is lipid. At present, there is no effective treatment for GBM, and the recurrence rate and mortality rate are nearly 100% (Mitre et al., 2022). Currently, ferroptosis as the research field remains many unrevealed areas, which may become a potentially effective treatment scheme. The brain is more vulnerable to oxidative stress than other tissues, because the activity of antioxidant enzymes is low and the content of PUFAs is high, which makes it prone to lipid peroxidation (Rao et al., 2000). The disorder of lipid metabolism is the key link of ferroptosis. It was found that expression of tripartite motif protein 7 (TRIM7) is more in GBM cells than in normal cells. When the TRIM7 is silent, the growth and development of the body is inhibited, but the level of ferroptosis is increased; while the TRIM7 is overexpressed, it can promote the growth and development of the body and inhibit the death of GBM cells. It can be concluded that when the ferroptosis level is inhibited, the death of GBM cells is also reduced, and there is a positive correlation between them. This experiment also indicates that when TRIM7 is missing, GBM is sensitive to the treatment of TMZ. As a potential treatment scheme for GBM, it is uncertain whether the sensitivity of TRIM7 to ferroptosis will make the treatment of TMZ more effective (Li et al., 2022b). Some experiments also found that the use of iron inducers can increase the sensitivity of TMZ. TMZ is a first-line drug for GBM (Song et al., 2021), and it is clear that its mechanism is to cause base pair mismatch, leading to cell apoptosis. However, several reports suggest that, it can activate nuclear factor NRF2 and transcription factor 4 (ATF4) at the same time to inhibit iron death. To sum up, ferroptosis participated not only in the sensitivity of TMZ in the treatment of GBM, but also in the formation of drug resistance (Chen et al., 2017; Hu et al., 2020; Li et al., 2022b; de Souza et al., 2022). Iron overload is another key link of ferroptosis. The high degree of malignancy of tumors is characterized by rapid growth and proliferation and strong invasiveness. Genes and proteins related to growth and development are overexpressed, such as poly(C)-binding protein 2 (PCBP2), DMT1, STEAP3, FTH and FTL, which can change iron storage capacity (Cheng et al., 2018); the expression of TF and TFR also increased significantly. A large amount of free iron would be transported and absorbed into the cells through selective endocytosis, and excessive iron would increase lipid peroxidation accordingly. In GBM cells with overloaded iron and rich PUFAs, plenty of peroxides can rapidly accumulate and lead to ferroptosis (Park et al., 2019). STEAP3 can promote TFR1 expression and increase cell iron content by activating STAT3-forkhead box protein M1 (FOXM1) axis (Sendamarai et al., 2008; Isobe et al., 2011), thus inducing epithelial mesenchymal transformation (EMT) in GBM, which is a route for GBM invasion and metastasis (Chang et al., 2017; Terry et al., 2017).
Expectation
Ferroptosis is a newly discovered iron dependent programmed cell death in recent years, which is different from apoptosis, necrosis and necroptosis. It has a unique mechanism of occurrence and effectiveness. It will be a potential therapeutic scheme in tumor treatment, and more and more targeted ferroptosis therapies are under study. Relevant pharmaceutical industries are also actively exploring the specific mechanism of iron death, trying to link it with cancer treatment to find a breakthrough. So far, although ferroptosis has made some theoretical achievements and curative effect in animal experiments, it has not made virtual progress. As aforementioned, some scholars proposed even contradictory conclusions. Some pointed out that TMZ treatment could strengthen ferroptosis and might be one of the mechanisms of tumor cell killing, whereas other researchers opposed this kind of conclusion and observed the opposite that TMZ might inhibit ferroptosis to result in drug resistance. This is an interesting phenomenon, and we speculate that TMZ treatment indeed up-regulates ferroptosis and causes the death of tumor cells. However, at the late stage of the course, the GBM cells may induce numerous ferroptotic inhibitors and the ferroptosis is significantly suppressed. This is worthy of being studied in the future. GBM, as one of the most common primary malignant tumors in adults, has not only a high recurrence rate but also an approximately mortality rate of 100%. Increasing evidence indicates that ferroptosis plays a certain role in immunotherapy, radiotherapy and chemotherapy for GBM. However, the research on its regulation and the mechanism of ferroptosis treatment has not made significant progress. It is still necessary to take persistent efforts to clarify the mechanism of the relationship between ferroptosis and GBM, to provide new ideas for the treatment, and in the meantime to bring up prevention or early diagnosis methods.
Author contributions
QL, XL, and YZ initiated the idea and wrote the manuscript; WH made valuable suggestions; HZ and TL revised the paper and coordinated the study and are shared correspondence.
Funding
This work was funded by the National Natural Science Foundation of China (Grant No. 82001278), The Fund for Young Doctors with the First People’s Hospital of Yunnan Province (Grant No. KHBS-2020-014), Yunnan Fundamental Research Projects (Grant No. 202101AU070106), and Joint Projects of Yunnan Provincial Science and Technology Department and Kunming Medical University for Applied Basic Research (Grant No. 202101AY070001-252), The Fund for Young Doctors with the First People’s Hospital of Yunnan Province (Grant No. KHBS-2022-018).
Conflict of interest
The authors declare that the research was conducted in the absence of any commercial or financial relationships that could be construed as a potential conflict of interest.
Publisher’s note
All claims expressed in this article are solely those of the authors and do not necessarily represent those of their affiliated organizations, or those of the publisher, the editors and the reviewers. Any product that may be evaluated in this article, or claim that may be made by its manufacturer, is not guaranteed or endorsed by the publisher.
References
Adamo, A., Fiore, D., De Martino, F., Roscigno, G., Affinito, A., Donnarumma, E., et al. (2017). RYK promotes the stemness of glioblastoma cells via the WNT/β-catenin pathway. Oncotarget 8, 13476–13487. doi:10.18632/oncotarget.14564
Agrawal, A., and Kale, R. K. (2001). Radiation induced peroxidative damage: Mechanism and significance. Indian J. Exp. Biol. 39, 291–309.
Aguilera, A., Berdun, F., Bartoli, C., Steelheart, C., Alegre, M., Bayir, H., et al. (2022). C-ferroptosis is an iron-dependent form of regulated cell death in cyanobacteria. J. Cell Biol. 221, e201911005. null. doi:10.1083/jcb.201911005
Anthonymuthu, T. S., Tyurina, Y. Y., Sun, W. Y., Mikulska-Ruminska, K., Shrivastava, I. H., Tyurin, V. A., et al. (2021). Resolving the paradox of ferroptotic cell death: Ferrostatin-1 binds to 15LOX/PEBP1 complex, suppresses generation of peroxidized ETE-PE, and protects against ferroptosis. Redox Biol. 38, 101744. doi:10.1016/j.redox.2020.101744
Badr, C. E., Silver, D. J., Siebzehnrubl, F. A., and Deleyrolle, L. P. (2020). Metabolic heterogeneity and adaptability in brain tumors. Cell. Mol. Life Sci. 77, 5101–5119. doi:10.1007/s00018-020-03569-w
Berardinelli, F., Tanori, M., Muoio, D., Buccarelli, M., di Masi, A., Leone, S., et al. (2019). G-quadruplex ligand RHPS4 radiosensitizes glioblastoma xenograft in vivo through a differential targeting of bulky differentiated- and stem-cancer cells. J. Exp. Clin. Cancer Res. 38, 311. doi:10.1186/s13046-019-1293-x
Bertheloot, D., Latz, E., and Franklin, B. S. (2021). Necroptosis, pyroptosis and apoptosis: An intricate game of cell death. Cell. Mol. Immunol. 18, 1106–1121. doi:10.1038/s41423-020-00630-3
Broadley, K. W., Hunn, M. K., Farrand, K. J., Price, K. M., Grasso, C., Miller, R. J., et al. (2011). Side population is not necessary or sufficient for a cancer stem cell phenotype in glioblastoma multiforme. Stem cells 29, 452–461. doi:10.1002/stem.582
Burić, S. S., Podolski-Renić, A., Dinić, J., Stanković, T., Jovanović, M., Hadžić, S., et al. (2019). Modulation of antioxidant potential with coenzyme Q10 suppressed invasion of temozolomide-resistant rat glioma in vitro and in vivo. Oxid. Med. Cell. Longev. 2019, 3061607. doi:10.1155/2019/3061607
Chanez, B., Appay, R., Guille, A., Lagarde, A., Colin, C., Adelaide, J., et al. (2022). Genomic analysis of paired IDHwt glioblastomas reveals recurrent alterations of MPDZ at relapse after radiotherapy and chemotherapy. J. Neurol. Sci. 436, 120207. doi:10.1016/j.jns.2022.120207
Chang, L., Li, K., and Guo, T. (2017). miR-26a-5p suppresses tumor metastasis by regulating EMT and is associated with prognosis in HCC. Clin. Transl. Oncol. 19, 695–703. doi:10.1007/s12094-016-1582-1
Chen, D., Rauh, M., Buchfelder, M., Eyupoglu, I. Y., and Savaskan, N. (2017). The oxido-metabolic driver ATF4 enhances temozolamide chemo-resistance in human gliomas. Oncotarget 8, 51164–51176. doi:10.18632/oncotarget.17737
Chen, H., and Wen, J. (2022). Iron oxide nanoparticles loaded with paclitaxel inhibits glioblastoma by enhancing autophagy-dependent ferroptosis pathway. Eur. J. Pharmacol. 921, 174860. doi:10.1016/j.ejphar.2022.174860
Cheng, C., Geng, F., Cheng, X., and Guo, D. (2018). Lipid metabolism reprogramming and its potential targets in cancer. Cancer Commun. 38, 27. doi:10.1186/s40880-018-0301-4
Chew, L. Y., Zhang, H., He, J., and Yu, F. (2021). The Nrf2-Keap1 pathway is activated by steroid hormone signaling to govern neuronal remodeling. Cell Rep. 36, 109466. doi:10.1016/j.celrep.2021.109466
Chng, C. P., Sadovsky, Y., Hsia, K. J., and Huang, C. (2021). Site-specific peroxidation modulates lipid bilayer mechanics. Extreme Mech. Lett. 42, 101148. null. doi:10.1016/j.eml.2020.101148
de Souza, I., Monteiro, L. K. S., Guedes, C. B., Silva, M. M., Andrade-Tomaz, M., Contieri, B., et al. (2022). High levels of NRF2 sensitize temozolomide-resistant glioblastoma cells to ferroptosis via ABCC1/MRP1 upregulation. Cell Death Dis. 13, 591. doi:10.1038/s41419-022-05044-9
Dissaux, G., Dissaux, B., Bourhis, D., Schick, U., and Querellou, S. (2021). 18F-FET PET/CT in early subventricular zone recurrence of adult glioblastoma. Clin. Nucl. Med. 46, 499–500. doi:10.1097/RLU.0000000000003639
Dixon, S. J., Lemberg, K. M., Lamprecht, M. R., Skouta, R., Zaitsev, E. M., Gleason, C. E., et al. (2012). Ferroptosis: An iron-dependent form of nonapoptotic cell death. Cell 149, 1060–1072. doi:10.1016/j.cell.2012.03.042
Doll, S., Freitas, F. P., Shah, R., Aldrovandi, M., da Silva, M. C., Ingold, I., et al. (2019). FSP1 is a glutathione-independent ferroptosis suppressor. Nature 575, 693–698. doi:10.1038/s41586-019-1707-0
Dutt, S., Hamza, I., and Bartnikas, T. B. (2022). Molecular mechanisms of iron and heme metabolism. Annu. Rev. Nutr. 42, 311–335. doi:10.1146/annurev-nutr-062320-112625
Fan, Z., Wirth, A. K., Chen, D., Wruck, C. J., Rauh, M., Buchfelder, M., et al. (2017). Nrf2-Keap1 pathway promotes cell proliferation and diminishes ferroptosis. Oncogenesis 6, e371. doi:10.1038/oncsis.2017.65
Gao, M., Meng, X., Guo, X., Zhu, J., Fan, A., Wang, Z., et al. (2018). All-active antitumor micelles via triggered lipid peroxidation. J. Control. Release 286, 381–393. doi:10.1016/j.jconrel.2018.08.003
Gong, C., Zhai, J., Wang, X., Zhu, W., Yang, D., Luo, Y., et al. (2022). Synergistic improving photo-Fenton and photo-catalytic degradation of carbamazepine over FeS2/Fe2O3/organic acid with H2O2in-situ generation. Chemosphere 307, 136199. doi:10.1016/j.chemosphere.2022.136199
Gu, H., Feng, J., Wang, H., Qian, Y., Yang, L., Chen, J., et al. (2016). Celastrus orbiculatus extract inhibits the migration and invasion of human glioblastoma cells in vitro. BMC Complement. Altern. Med. 16, 387. doi:10.1186/s12906-016-1232-8
Hayashima, K., and Katoh, H. (2022). Expression of gamma-glutamyltransferase 1 in glioblastoma cells confers resistance to cystine deprivation-induced ferroptosis. J. Biol. Chem. 298, 101703. doi:10.1016/j.jbc.2022.101703
Hu, Q., Wei, W., Wu, D., Huang, F., Li, M., Li, W., et al. (2022). Blockade of GCH1/BH4 Axis activates ferritinophagy to mitigate the resistance of colorectal cancer to erastin-induced ferroptosis. Front. Cell Dev. Biol. 10, 810327. doi:10.3389/fcell.2022.810327
Hu, Z., Mi, Y., Qian, H., Guo, N., Yan, A., Zhang, Y., et al. (2020). A potential mechanism of temozolomide resistance in glioma-ferroptosis. Front. Oncol. 10, 897. doi:10.3389/fonc.2020.00897
Isobe, T., Baba, E., Arita, S., Komoda, M., Tamura, S., Shirakawa, T., et al. (2011). Human STEAP3 maintains tumor growth under hypoferric condition. Exp. Cell Res. 317, 2582–2591. doi:10.1016/j.yexcr.2011.07.022
Jiang, Y., Zhao, J., Li, R., Liu, Y., Zhou, L., Wang, C., et al. (2022). CircLRFN5 inhibits the progression of glioblastoma via PRRX2/GCH1 mediated ferroptosis. J. Exp. Clin. Cancer Res. 41, 307. doi:10.1186/s13046-022-02518-8
Kagan, V. E., Tyurina, Y. Y., Vlasova, , Kapralov, A. A., Amoscato, A. A., Anthonymuthu, T. S., et al. (2020). Redox epiphospholipidome in programmed cell death signaling: Catalytic mechanisms and regulation. Front. Endocrinol. 11, 628079. doi:10.3389/fendo.2020.628079
Karaskova, E., Pospisilova, D., Velganova-Veghova, M., Geryk, M., Volejnikova, J., Holub, D., et al. (2021). Importance of hepcidin in the etiopathogenesis of anemia in inflammatory bowel disease. Dig. Dis. Sci. 66, 3263–3269. doi:10.1007/s10620-020-06652-1
Lee, J., and Roh, J. L. (2022). Induction of ferroptosis in head and neck cancer: A novel bridgehead for fighting cancer resilience. Cancer Lett. 546, 215854. doi:10.1016/j.canlet.2022.215854
Li, F. J., Long, H. Z., Zhou, Z. W., Luo, H. Y., Xu, S. G., and Gao, L. C. (2022). System Xc -/GSH/GPX4 axis: An important antioxidant system for the ferroptosis in drug-resistant solid tumor therapy. Front. Pharmacol. 13, 910292. doi:10.3389/fphar.2022.910292
Li, K., Chen, B., Xu, A., Shen, J., Li, K., Hao, K., et al. (2022). TRIM7 modulates NCOA4-mediated ferritinophagy and ferroptosis in glioblastoma cells. Redox Biol. 56, 102451. doi:10.1016/j.redox.2022.102451
Li, S., and Huang, Y. (2022). Ferroptosis: An iron-dependent cell death form linking metabolism, diseases, immune cell and targeted therapy. Clin. Transl. Oncol. 24, 1–12. doi:10.1007/s12094-021-02669-8
Li, X., Wang, Q., Yu, S., Zhang, M., Liu, X., Deng, G., et al. (2021). Multifunctional MnO2-based nanoplatform-induced ferroptosis and apoptosis for synergetic chemoradiotherapy. Nanomedicine 16, 2343–2361. doi:10.2217/nnm-2021-0286
Liu, B., Ji, Q., Cheng, Y., Liu, M., Zhang, B., Mei, Q., et al. (2022). Biomimetic GBM-targeted drug delivery system boosting ferroptosis for immunotherapy of orthotopic drug-resistant GBM. J. Nanobiotechnology 20, 161. doi:10.1186/s12951-022-01360-6
Liu, C. C., Li, H. H., Lin, J. H., Chiang, M. C., Hsu, T. W., Li, A. F., et al. (2021). Esophageal cancer stem-like cells resist ferroptosis-induced cell death by active hsp27-GPX4 pathway. Biomolecules 12, 48. null. doi:10.3390/biom12010048
Liu, P., Feng, Y., Li, H., Chen, X., Wang, G., Xu, S., et al. (2020). Ferrostatin-1 alleviates lipopolysaccharide-induced acute lung injury via inhibiting ferroptosis. Cell. Mol. Biol. Lett. 25, 10. doi:10.1186/s11658-020-00205-0
Liu, P., Lin, C., Liu, Z., Zhu, C., Lin, Z., Xu, D., et al. (2022). Inhibition of ALG3 stimulates cancer cell immunogenic ferroptosis to potentiate immunotherapy. Cell. Mol. Life Sci. 79, 352. doi:10.1007/s00018-022-04365-4
Liu, T., Zhu, C., Chen, X., Guan, G., Zou, C., Shen, S., et al. (2022). Ferroptosis, as the most enriched programmed cell death process in glioma, induces immunosuppression and immunotherapy resistance. Neuro. Oncol. 24, 1113–1125. doi:10.1093/neuonc/noac033
Liu, Y., Shen, Y., Sun, T., and Yang, W. (2017). Mechanisms regulating radiosensitivity of glioma stem cells. Neoplasma 64, 655–665. doi:10.4149/neo_2017_502
Lu, B., Chen, X. B., Ying, M. D., He, Q. J., Cao, J., and Yang, B. (2017). The role of ferroptosis in cancer development and treatment response. Front. Pharmacol. 8, 992. doi:10.3389/fphar.2017.00992
Martínez-Garcia, M., Álvarez-Linera, J., Carrato, C., Ley, L., Luque, R., Maldonado, X., et al. (2018). SEOM clinical guidelines for diagnosis and treatment of glioblastoma (2017). Clin. Transl. Oncol. 20, 22–28. doi:10.1007/s12094-017-1763-6
McNamee, J. P., Grybas, V. S., Qutob, S. S., and Bellier, P. V. (2021). Effects of 1800 MHz radiofrequency fields on signal transduction and antioxidant proteins in human A172 glioblastoma cells. Int. J. Radiat. Biol. 97, 1316–1323. doi:10.1080/09553002.2021.1934751
Mikulska-Ruminska, K., Anthonymuthu, T. S., Levkina, A., Shrivastava, I. H., Kapralov, A. A., Bayır, H., et al. (2021). NO• represses the oxygenation of arachidonoyl PE by 15lox/PEBP1: Mechanism and role in ferroptosis. Int. J. Mol. Sci. 22, 5253. null. doi:10.3390/ijms22105253
Mitre, A. O., Florian, A. I., Buruiana, A., Boer, A., Moldovan, I., Soritau, O., et al. (2022). Ferroptosis involvement in glioblastoma treatment. Medicina 58, 319. null. doi:10.3390/medicina58020319
Mou, Y. W., Li, Z. Y., Yang, X., Chen, S. Y., Hou, S. S., Zhang, E. G., et al. (2020). Research progress of ferroptosis-related mechanism and diseases. Zhonghua Lao Dong Wei Sheng Zhi Ye Bing Za Zhi 38, 797–800. doi:10.3760/cma.j.cn121094-20190925-00392
Ou-Yang, Q., He, X., Yang, A., Li, B., and Xu, M. (2018). Interference with NTSR1 expression exerts an anti-invasion effect via the jun/miR-494/SOCS6 Axis of glioblastoma cells. Cell. Physiol. biochem. 49, 2382–2395. doi:10.1159/000493838
Park, K. J., Kim, J., Testoff, T., Adams, J., Poklar, M., Zborowski, M., et al. (2019). Quantitative characterization of the regulation of iron metabolism in glioblastoma stem-like cells using magnetophoresis. Biotechnol. Bioeng. 116, 1644–1655. doi:10.1002/bit.26973
Piskin, E., Cianciosi, D., Gulec, S., Tomas, M., and Capanoglu, E. (2022). Iron absorption: Factors, limitations, and improvement methods. ACS omega 7, 20441–20456. doi:10.1021/acsomega.2c01833
Rao, G. M., Rao, A. V., Raja, A., Rao, S., and Rao, A. (2000). Role of antioxidant enzymes in brain tumours. Clin. Chim. Acta. 296, 203–212. doi:10.1016/s0009-8981(00)00219-9
Reichert, C. O., de Freitas, F. A., Sampaio-Silva, J., Rokita-Rosa, L., Barros, P. L., Levy, D., et al. (2020). Ferroptosis mechanisms involved in neurodegenerative diseases. Int. J. Mol. Sci. 21, 8765. null. doi:10.3390/ijms21228765
Santoro, M. M. (2020). The antioxidant role of non-mitochondrial CoQ10: Mystery solved. Cell Metab. 31, 13–15. doi:10.1016/j.cmet.2019.12.007
Sendamarai, A. K., Ohgami, R. S., Fleming, M. D., and Lawrence, C. M. (2008). Structure of the membrane proximal oxidoreductase domain of human Steap3, the dominant ferrireductase of the erythroid transferrin cycle. Proc. Natl. Acad. Sci. U. S. A. 105, 7410–7415. doi:10.1073/pnas.0801318105
Sesé, B., Íñiguez-Muñoz, S., Ensenyat-Mendez, M., Llinàs-Arias, P., Ramis, G., Orozco, J. I. J., et al. (2022). Glioblastoma embryonic-like stem cells exhibit immune-evasive phenotype. Cancers 14, 2070. null. doi:10.3390/cancers14092070
Shi, J., Yang, N., Han, M., and Qiu, C. (2022). Emerging roles of ferroptosis in glioma. Front. Oncol. 12, 993316. doi:10.3389/fonc.2022.993316
Shi, L., Liu, Y., Li, M., and Luo, Z. (2022). Emerging roles of ferroptosis in the tumor immune landscape: From danger signals to anti-tumor immunity. FEBS J. 289 (2022), 3655–3665. doi:10.1111/febs.16034
Shi, Z. X., Rao, W., Wang, H., Wang, N. D., Si, J. W., Zhao, J., et al. (2015). Modeled microgravity suppressed invasion and migration of human glioblastoma U87 cells through downregulating store-operated calcium entry. Biochem. Biophys. Res. Commun. 457, 378–384. doi:10.1016/j.bbrc.2014.12.120
Shin, D., Kim, E. H., Lee, J., and Roh, J. L. (2018). Nrf2 inhibition reverses resistance to GPX4 inhibitor-induced ferroptosis in head and neck cancer. Free Radic. Biol. Med. 129, 454–462. doi:10.1016/j.freeradbiomed.2018.10.426
Song, Q., Peng, S., Sun, Z., Heng, X., and Zhu, X. (2021). Temozolomide drives ferroptosis via a DMT1-dependent pathway in glioblastoma cells. Yonsei Med. J. 62, 843–849. doi:10.3349/ymj.2021.62.9.843
Stallhofer, J., Veith, L., Diegelmann, J., Probst, P., Brand, S., Schnitzler, F., et al. (2022). Iron deficiency in inflammatory bowel disease is associated with low levels of vitamin D modulating serum hepcidin and intestinal ceruloplasmin expression. Clin. Transl. Gastroenterol. 13, e00450. doi:10.14309/ctg.0000000000000450
Su, J., Li, Y., Liu, Q., Peng, G., Qin, C., and Li, Y. (2022). Identification of SSBP1 as a ferroptosis-related biomarker of glioblastoma based on a novel mitochondria-related gene risk model and in vitro experiments. J. Transl. Med. 20, 440. doi:10.1186/s12967-022-03657-4
Sun, S., Gao, T., Pang, B., Su, X., Guo, C., Zhang, R., et al. (2022). RNA binding protein NKAP protects glioblastoma cells from ferroptosis by promoting SLC7A11 mRNA splicing in an m6A-dependent manner. Cell Death Dis. 13, 73. doi:10.1038/s41419-022-04524-2
Tang, Q., Bai, L., Zou, Z., Meng, P., Xia, Y., Cheng, S., et al. (2018). Ferroptosis is newly characterized form of neuronal cell death in response to arsenite exposure. Neurotoxicology 67, 27–36. doi:10.1016/j.neuro.2018.04.012
Terry, S., Savagner, P., Ortiz-Cuaran, S., Mahjoubi, L., Saintigny, P., Thiery, J. P., et al. (2017). New insights into the role of EMT in tumor immune escape. Mol. Oncol. 11, 824–846. doi:10.1002/1878-0261.12093
Tomita, K., Takashi, Y., Ouchi, Y., Kuwahara, Y., Igarashi, K., Nagasawa, T., et al. (2019). Lipid peroxidation increases hydrogen peroxide permeability leading to cell death in cancer cell lines that lack mtDNA. Cancer Sci. 110, 2856–2866. doi:10.1111/cas.14132
Wang, K. Y., Huang, R. Y., Tong, X. Z., Zhang, K. N., Liu, Y. W., Zeng, F., et al. (2019). Molecular and clinical characterization of TMEM71 expression at the transcriptional level in glioma. CNS Neurosci. Ther. 25, 965–975. doi:10.1111/cns.13137
Wang, W. J., Ling, Y. Y., Zhong, Y. M., Li, Z. Y., Tan, C. P., and Mao, Z. W. (2022). Ferroptosis-enhanced cancer immunity by a ferrocene-appended iridium(III) diphosphine complex. Angew. Chem. Int. Ed. Engl. 61, e202115247. doi:10.1002/anie.202115247
Wu, H., Luan, Y., Wang, H., Zhang, P., Liu, S., Wang, P., et al. (2022). Selenium inhibits ferroptosis and ameliorates autistic-like behaviors of BTBR mice by regulating the Nrf2/GPx4 pathway. Brain Res. Bull. 183, 38–48. doi:10.1016/j.brainresbull.2022.02.018
Xing, L., Liu, X. Y., Zhou, T. J., Wan, X., Wang, Y., and Jiang, H. L. (2021). Photothermal nanozyme-ignited Fenton reaction-independent ferroptosis for breast cancer therapy. J. Control. Release 339, 14–26. doi:10.1016/j.jconrel.2021.09.019
Xu, H., Ye, D., Ren, M., Zhang, H., and Bi, F. (2021). Ferroptosis in the tumor microenvironment: Perspectives for immunotherapy. Trends Mol. Med. 27, 856–867. doi:10.1016/j.molmed.2021.06.014
Yang, F. C., Wang, C., Zhu, J., Gai, Q. J., Mao, M., He, J., et al. (2022). Inhibitory effects of temozolomide on glioma cells is sensitized by RSL3-induced ferroptosis but negatively correlated with expression of ferritin heavy chain 1 and ferritin light chain. Lab. Invest. 102, 741–752. doi:10.1038/s41374-022-00779-7
Yang, F., Zou, Y., Gong, Q., Chen, J., Li, W. D., and Huang, Q. (2020). From astrocytoma to glioblastoma: A clonal evolution study. FEBS open bio 10, 744–751. doi:10.1002/2211-5463.12815
Yang, M., Tsui, M. G., Tsang, J. K. W., Goit, R. K., Yao, K. M., So, K. F., et al. (2022). Involvement of FSP1-CoQ10-NADH and GSH-GPx-4 pathways in retinal pigment epithelium ferroptosis. Cell Death Dis. 13, 468. doi:10.1038/s41419-022-04924-4
Yang, W. S., Kim, K. J., Gaschler, M. M., Patel, M., Shchepinov, M. S., and Stockwell, B. R. (2016). Peroxidation of polyunsaturated fatty acids by lipoxygenases drives ferroptosis. Proc. Natl. Acad. Sci. U. S. A. 113, E4966–E4975. doi:10.1073/pnas.1603244113
Yi, R., Wang, H., Deng, C., Wang, X., Yao, L., Niu, W., et al. (2020). Dihydroartemisinin initiates ferroptosis in glioblastoma through GPX4 inhibition. Biosci. Rep. 40, BSR20193314. null. doi:10.1042/BSR20193314
Yu, Y., Huang, Z., Chen, Q., Zhang, Z., Jiang, H., Gu, R., et al. (2022). Iron-based nanoscale coordination polymers synergistically induce immunogenic ferroptosis by blocking dihydrofolate reductase for cancer immunotherapy. Biomaterials 288, 121724. doi:10.1016/j.biomaterials.2022.121724
Yuan, F., Sun, Q., Zhang, S., Ye, L., Xu, Y., Xu, Z., et al. (2022). HSP27 protects against ferroptosis of glioblastoma cells. Hum. Cell 35, 238–249. doi:10.1007/s13577-021-00645-6
Zhang, J. J., Du, J., Kong, N., Zhang, G. Y., Liu, M. Z., and Liu, C. (2021). Mechanisms and pharmacological applications of ferroptosis: A narrative review. Ann. Transl. Med. 9, 1503. doi:10.21037/atm-21-1595
Zhang, X., Li, X., Zheng, C., Yang, C., Zhang, R., Wang, A., et al. (2022). Ferroptosis, a new form of cell death defined after radiation exposure. Int. J. Radiat. Biol. 98, 1201–1209. doi:10.1080/09553002.2022.2020358
Zhang, Y., Hou, Y., Liu, C., Li, Y., Guo, W., Wu, J. L., et al. (2016). Identification of an adaptor protein that facilitates Nrf2-Keap1 complex formation and modulates antioxidant response. Free Radic. Biol. Med. 97, 38–49. doi:10.1016/j.freeradbiomed.2016.05.017
Zhang, Y., Kong, Y., Ma, Y., Ni, S., Wikerholmen, T., Xi, K., et al. (2021). Loss of COPZ1 induces NCOA4 mediated autophagy and ferroptosis in glioblastoma cell lines. Oncogene 40, 1425–1439. doi:10.1038/s41388-020-01622-3
Zhang, Z., Pan, Y., Cun, J. E., Li, J., Guo, Z., Pan, Q., et al. (2022). A reactive oxygen species-replenishing coordination polymer nanomedicine disrupts redox homeostasis and induces concurrent apoptosis-ferroptosis for combinational cancer therapy. Acta Biomater. 151, 480–490. doi:10.1016/j.actbio.2022.07.055
Zhao, J., Wang, Y., Tao, L., and Chen, L. (2022). Iron transporters and ferroptosis in malignant brain tumors. Front. Oncol. 12, 861834. doi:10.3389/fonc.2022.861834
Zhao, X., Liu, Z., Gao, J., Li, H., Wang, X., Li, Y., et al. (2020). Inhibition of ferroptosis attenuates busulfan-induced oligospermia in mice. Toxicology 440, 152489. doi:10.1016/j.tox.2020.152489
Zhou, L., and Ma, J. (2022). MIR99AHG/miR-204-5p/TXNIP/Nrf2/ARE signaling pathway decreases glioblastoma temozolomide sensitivity. Neurotox. Res. 40, 1152–1162. doi:10.1007/s12640-022-00536-0
Zhou, Y., Shen, Y., Chen, C., Sui, X., Yang, J., Wang, L., et al. (2019). The crosstalk between autophagy and ferroptosis: What can we learn to target drug resistance in cancer? Cancer Biol. Med. 16, 630–646. doi:10.20892/j.issn.2095-3941.2019.0158
Keywords: ferroptosis, glioblastoma, iron metabolism, chemotherapy, radiotherapy, immunotherapy
Citation: Lu Q, Lu X, Zhang Y, Huang W, Zhou H and Li T (2023) Recent advances in ferroptosis and therapeutic strategies for glioblastoma. Front. Mol. Biosci. 9:1068437. doi: 10.3389/fmolb.2022.1068437
Received: 12 October 2022; Accepted: 02 December 2022;
Published: 13 January 2023.
Edited by:
Xin Wang, National Institutes of Health (NIH), United StatesReviewed by:
Wenqiang Li, First Affiliated Hospital of Zhengzhou University, ChinaJianhua Peng, The Affiliated Hospital of Southwest Medical University, China
Copyright © 2023 Lu, Lu, Zhang, Huang, Zhou and Li. This is an open-access article distributed under the terms of the Creative Commons Attribution License (CC BY). The use, distribution or reproduction in other forums is permitted, provided the original author(s) and the copyright owner(s) are credited and that the original publication in this journal is cited, in accordance with accepted academic practice. No use, distribution or reproduction is permitted which does not comply with these terms.
*Correspondence: Hu Zhou, 602856636@qq.com; Tao Li, taoli_kh@163.com
†These authors have contributed equally to this work