- 1Centre for Blood Research, University of British Columbia, Vancouver, BC, Canada
- 2Department of Biochemistry and Molecular Biology, University of British Columbia, Vancouver, BC, Canada
- 3Versiti Blood Research Institute, Milwaukee, WI, United States
- 4Department of Cell Biology, Neurobiology, and Anatomy, Medical College of Wisconsin, Milwaukee, WI, United States
- 5Department of Oral Biological and Medical Sciences, University of British Columbia, Vancouver, BC, Canada
Platelets are anucleate cells that are essential for hemostasis and wound healing. Upon activation of the cell surface receptors by their corresponding extracellular ligands, platelets undergo rapid shape change driven by the actin cytoskeleton; this shape change reaction is modulated by a diverse array of actin-binding proteins. One actin-binding protein, filamin A (FLNA), cross-links and stabilizes subcortical actin filaments thus providing stability to the cell membrane. In addition, FLNA binds the intracellular portion of multiple cell surface receptors and acts as a critical intracellular signaling scaffold that integrates signals between the platelet’s plasma membrane and the actin cytoskeleton. This mini-review summarizes how FLNA transduces critical cell signals to the platelet cytoskeleton.
Introduction
Platelets play a central role in hemostasis and wound healing (Hou et al., 2015; Etulain, 2018), and circulate in their quiescent form as discs that become activated following exposure to damaged blood vessel walls and/or soluble agonists (Jurk and Kehrel, 2005). The ligation of platelet receptors by their corresponding agonists triggers intracellular signaling pathways that result in platelet aggregation and granule secretion. The hemostatic process culminates in the conversion of the platelet “plug” into a fibrin clot (recently reviewed in (Sang et al., 2021)). Following activation, platelets change shape from a discoid to a flattened morphology characterized by multiple spike- and sheet-like cell surface extensions (Bearer et al., 2002; Sorrentino et al., 2015; Bender and Palankar, 2021).
Platelet shape change is directly mediated by dynamic nature of the actin cytoskeleton. There are two actin pools: monomeric or globular G-actin, and polymeric or filamentous F-actin (Pollard, 2016; Romero et al., 2020). The actin cytoskeleton is dynamically assembled and disassembled in response to environmental cues; actin assembly near the plasma membrane creates cell surface protrusions that mark the shape change reaction, which is critical for platelet adhesion and aggregation at sites of vascular injury (Falet et al., 2017).
A well-documented activation pathway in platelets involves the stimulation, by thrombin, of protease-activated receptor (PARs), which activates the associated Gq protein and its downstream effector, phospholipase C beta (PLCβ) (Michelson, 2013). Activated PLCβ then degrades phosphatidylinositol 4,5-bisphosphate (PI4,5P2) to diacylglycerol (DAG) and inositol 1,4,5-trisphosphate (I1,4,5P3), which releases calcium (Ca2+) from the dense tubular system thus increasing intracellular calcium levels ([Ca2+]i). DAG and Ca2+ activate protein kinase C (PKC) (Job and Lagnado, 1998; DeMali et al., 2003). Assembly of G-actin into F-actin near the plasma membrane creates cell surface protrusions termed filopodia and lamellipodia; this process is largely driven by the small GTPases Rac1 and Cdc42 (Azim et al., 2000; Bodie et al., 2001; Aslan and McCarty, 2013). In an alternative signaling pathway, ligation of the glycoprotein VI (GPVI) receptor by collagen also culminates in actin reorganization via increases in [Ca2+]i and activation of PKC. Irrespective of the agonist, the shape change that occurs in activated platelets requires the transmission of elaborate signals from the plasma membrane to the actin cytoskeleton; this process is modulated by multiple actin-binding proteins.
BOX 1 Key points: Actin assembly in activated platelets
• Platelets are activated by extracellular ligands that bind to cell surface receptors.
• Activated platelets change shape during aggregation, adhesion and hemostasis.
• This shape change reaction requires efficient signal transduction between the plasma membrane and the actin cytoskeleton – mediated by actin-binding proteins.
Filamin A (FLNA)
Actin-binding proteins can be classified as monomer-sequestering, filament-severing, bundling, and cross-linking proteins (Bearer et al., 2002). Filamins are large actin crosslinking proteins that assemble actin filaments into orthogonal networks (Stossel et al., 2001) and exist as three paralogs (A, B, and C). Filamin A (FLNA), whose gene is located on the X chromosome, is the most abundantly expressed isoform (Stossel et al., 2001), including in platelets, which also express low levels of filamin B (FLNB) whose gene resides on chromosome 3 (Takafuta et al., 1998). Filamin C (FLNC) is encoded on chromosome 7 and is primarily expressed in skeletal and cardiac muscle cells (Maestrini et al., 1993; van der Flier et al., 2002).
While a primary function of FLNA is to crosslink actin, FLNA also binds >50 other proteins including cell surface receptors, and therefore serves as a signaling scaffold (Stossel et al., 2001; Zhou et al., 2010; Zhou et al., 2021). The known FLNA binding partners, including those specifically implicated in platelet function, are listed in Table 1.
Structure of FLNA
FLNA is a 280-kDa protein comprised of an N-terminal actin-binding domain followed by 24 immmunoglobulin (Ig)-like repeats of beta (β)-sheets containing seven β-strands (A-G) each (Figure 1A). The N-terminal spectrin-related actin-binding domain contains two calponin homology domains (CH1 and CH2), and another distal secondary actin binding domain (Robertson et al., 2003; Nakamura et al., 2007). The FLNA molecule is organized into rod one containing repeats 1–15; rod two containing repeats 16–23; and two calpain-sensitive flexible loops, called hinges, separating rods one and two and repeats 23 and 24, respectively (Figure 1A). While rod one is a 58 nm extended chain, interactions between repeats 16, 18, 20 with repeats 17, 19, and 21, respectively, lead to the compact propeller-like structure of rod 2 (Nakamura et al., 2007; Ruskamo et al., 2012; Tossavainen et al., 2012). FLNA forms a V-shape upon self-dimerization at the C-terminal repeat 24 (Figure 1A), and the two actin-binding domains bind and organize actin into an orthogonal network. Rod two mediates binding to multiple proteins (Table 1) thus contributing to the major scaffolding function of FLNA.
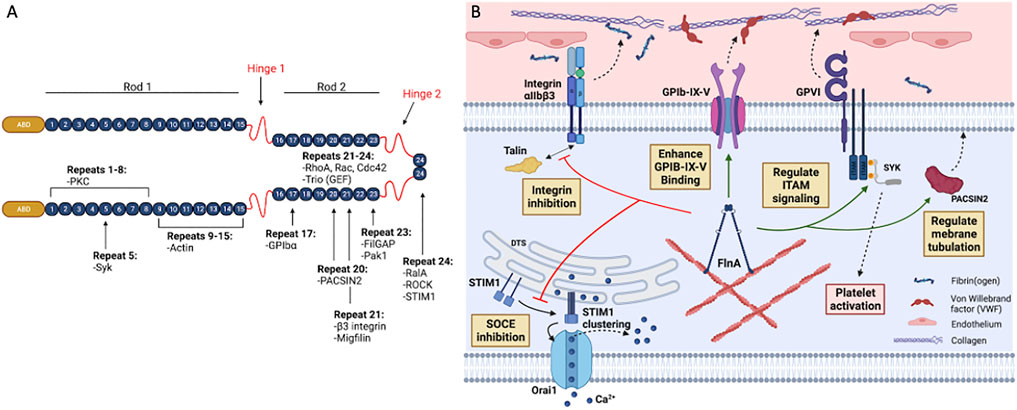
FIGURE 1. Structure and binding partners of FLNA. (A) FLNA is a 280 kDa homodimer consisting of an actin-binding domain (ABD) at the N-terminus, followed by 24 immunoglobulin (Ig)-like repeat domains folded into β-sheets (numbered 1–24). Two hinge domains, one at Ig repeat 15–16 and another at 23–24, separate the Ig domains into two different rod regions: rod one and rod 2. Rod one consists of Ig repeats 1–15, and rod two consists of Ig repeats 16–23. Dimerization occurs through the interaction of repeat 24. FLNA interacts with many receptors and signaling molecules through its 24 Ig repeats. (B) In platelets, the store-operated calcium entry (SOCE) is regulated by the interaction between STIM1 and Orai1. Upon depletion of Ca2+ storage, STIM1 undergoes a conformational change and multimerizes on the dense tubular system (DTS) membrane. Consequently, STIM1 clusters initiate the assembly of Orai1 subunits in the plasma membrane, forming a Ca2+ channel which leads to the influx of extracellular Ca2+. FLNA downregulates SOCE function by directly interacting with STIM1 in the actin cytoskeleton, thereby abolishing the STIM1-Orai1 interaction. In resting platelets, FLNA has been proposed to constitutively associate with integrin β3 cytoplasmic tail (CT) through its Ig repeat 21. This association blocks the interaction between the β3 CT and talin or kindlin-3, thereby inhibiting integrin αIIbβ3 activation. GPIb-IX-V mediates adhesion of platelets to von Willebrand factor (VWF) upon endothelial injuries. FLNA constitutively interacts with GPIb-IX-V and enhances its binding to VWF. This interaction involves FLNA Ig repeat 17 to the GPIbα CT of GPIb-IX-V. FLNA also positively regulates ITAM- and ITAM-like-containing receptor signaling in platelets by interacting with Spleen tyrosine kinase (Syk). This interaction is essential for GPVI receptor signaling, which is an important pathway for collagen-mediated platelet adhesion and activation. FLNA Ig repeat 20 interacts with the F-BAR protein PACSIN2 to regulate membrane tubulation and intracellular membrane architecture in platelets. This interaction also likely contributes to demarcation membrane system (DMS) formation in megakaryocytes. Figure created with BioRender.com.
Clinical implications of FLNA gene variants
Variants of the FLNA gene confer a group of clinical disorders collectively termed filaminopathies A (Nurden et al., 2011). The most prominent such disorder is X-linked periventricular heterotopia (PVNH), caused by a defect in neuronal migration during fetal development (Feng and Walsh, 2004; Robertson, 2005). PVNH manifests as cardiac and cerebral malformations and seizure disorders (Feng and Walsh, 2004). Frameshift, missense and nonsense mutations of FLNA underlie PVNH (Robertson, 2005). Other filaminopathies A include otopalatodigital syndrome, frontometaphyseal dysplasia and Melnick-Needles syndrome, which are characterized by skeletal dysplasia (Robertson, 2007). Multiple variants of the human FLNA gene are specifically associated with aberrant platelet function (Vassallo et al., 2020; Tanner et al., 2022). Consequently, patients with PVNH can exhibit hemorrhage, coagulopathy and thrombocytopenia (Nurden et al., 2011; Berrou et al., 2017).
FLNA is a critical determinant of platelet structural integrity and shape change
The most compelling direct evidence of the critical role of FLNA in platelet function has been obtained from studies with FLNA knockout mice. Because FLNA deficiency is an embryonic lethal trait, conditional knockouts were generated where FLNA expression is specifically deleted in megakaryocytes and platelets (Falet et al., 2010; Jurak Begonja et al., 2011). These conditional knockout mice display macrothrombocytopenia, which is characterized by morphologically large platelets that circulate in low numbers (Falet et al., 2010; Jurak Begonja et al., 2011). Electron micrographs of resting platelets showed that actin filaments are dissociated from the cell membranes in FLNA-deficient platelets (Falet et al., 2010), indicating that FLNA is essential for maintaining structural continuity between the plasma membrane and the actin cytoskeleton. Importantly, FLNA-null platelets fail to assemble actin normally in response to stimulation by either thrombin or collagen-related peptide (Falet et al., 2010). In a separate study, the stability of the platelet plasma membrane was monitored (Jurak Begonja et al., 2011). After 24 h of storage, FLNA-deficient platelets exhibited increased microvesiculation relative to control platelets (Jurak Begonja et al., 2011), which further reinforces the notion that FLNA underpins membrane stability. Collectively, the available evidence clearly implicates FLNA as an essential transducer of PAR- and GPVI-driven signals to the actin cytoskeleton. Less clearly defined are the exact protein/protein interactions between FLNA and the various plasma membrane and cytoplasmic proteins that regulate the actin cytoskeleton.
FLNA interactions with platelet plasma membrane proteins
Multiple receptors mediate the platelet response to vascular injury (Jurk and Kehrel, 2005); moreover, platelet function is directly contingent on the integrity of the actin cytoskeleton (Bearer et al., 2002; Sorrentino et al., 2015; Bender and Palankar, 2021). The receptors that drive platelet response to injury include the PARs which recognize thrombin (Coughlin, 2000), the glycoprotein Ib/V/IX complex which recognizes von Willebrand factor (vWF) (Shen et al., 2000; Zhang et al., 2022), and the glycoprotein VI (GPVI) receptor which recognizes exposed collagen following vascular injury (Jurk and Kehrel, 2005). FLNA is essential for normal PAR4-and GPVI-driven signal transduction (Falet et al., 2010) and also directly binds other receptors thus serving as a critical signaling conduit between the plasma membrane and the platelet cytoskeleton (Figure 1B).
FLNA interaction with GPIbα
The initial platelet adhesion to the damaged vascular wall requires the binding of von Willebrand factor (vWF) to the GPIb-IX-V receptor complex at the platelet surface (Varga-Szabo et al., 2008a). This quadripartite complex consists of GPIbα, GPIbβ, GPIX, and GPV (Zhang et al., 2022); notably, FLNA constitutively binds GPIbα, thus anchoring the receptor complex to the actin cytoskeleton (Nakamura et al., 2006). Specifically, the hydrophobic region of FLNA Ig repeat 17 binds the cytoplasmic tail of GPIbα at amino acids 563–571 (Meyer et al., 1997; Cranmer et al., 2005; Nakamura et al., 2006). Multiple evidences support the functional importance of the FLNA-GPIbα interaction. For example, FLNA-deficient platelets exhibit abnormal surface expression and distribution of GPIbα (Falet et al., 2010). Moreover, transgenic mice expressing a mutant, non-FLNA-binding GPIbα produce platelets that fragment under shear stress (Cranmer et al., 2011). Similarly, Chinese hamster ovary (CHO) cells transfected with a non-FLNA-binding GPIbα failed to generate contractile forces on VWF substrates (Feghhi et al., 2016). Conversely, a gain-of-function mutation in FLNA Ig repeat 16 was proposed to promote the GPIbα-FLNA interaction and platelet adhesion on a VWF surface (Berrou et al., 2013). Combined with data suggesting that FLNA is required for normal GPIbα trafficking to the cell surface (Kanaji et al., 2012), it is therefore clear that the GPIbα-FLNA association is of crucial importance in the context of platelet adhesion and hemostasis. A recently published report implicates the GPIbα-FLNA interaction as a determinant in GPIbα receptor shedding, which diminishes the function of stored platelets (Zhou et al., 2022). The authors report that GPIbα receptor shedding is directly related to the stability of the actin cytoskeleton and the integrity of GPIbα-FLNA binding (Zhou et al., 2022), thus further underscoring the role of FLNA in platelet signal transduction.
FLNA interaction with integrin αIIbβ3
Integrins are heterodimeric, transmembrane receptors that adopt a folded, closed conformation in the resting platelet. Integrins can be activated via their cytoplasmic domains (termed “inside-out signaling”) (Huang et al., 2019) or by ligand binding to their extracellular domain (“outside-in” signaling) (Shattil and Newman, 2004). FLNA interacts with integrin beta (β) subunits. This interaction requires FLNA Ig repeat 21 and a region located between two endocytic NPxY/F motifs on β subunits that also interact with talin-1 (Kiema et al., 2006) and kindlin-3 (Yates et al., 2012).
Megakaryocytes and platelets express both β1 and β3 integrins: the collagen receptor α2β1, the fibronectin receptor α5β1, the laminin receptor α6β1, the fibrinogen receptor αIIbβ3, and the vitronectin receptor αVβ3 [recently reviewed in (Yang et al., 2022)]. While the β3 subunit does not have an optimal FLNA-binding motif (unlike the β1 subunit), most studies on platelets have focused on the interaction between FLNA and αIIbβ3 and its role in fibrinogen binding and platelet aggregation. As the fibrinogen-binding receptor (Bennett et al., 2009; Nieswandt et al., 2009; Jackson and Schoenwaelder, 2010), the glycoprotein IIb/IIIa (αIIbβ3 integrin) receptor is critical for normal platelet function and transmits signals to/from the platelet actin cytoskeleton (Morse et al., 2014). Two cytoplasmic proteins, talin (Petrich et al., 2007a; Petrich et al., 2007b) and kindlin-3 (Moser et al., 2008) are identified as integrin “activators” that bind the cytoplasmic domain of the β3 integrin subunit, triggering conformational changes that expose the integrin’s extracellular ligand binding site (Ginsberg, 2014) to promote integrin activation (Ma et al., 2007). Notably, the Ig repeat 21 of FLNA also binds the β3 integrin at amino acids 747–755, thus competing with talin and kindlin-3 (Kiema et al., 2006; Liu et al., 2015; Rosa et al., 2019).
The prevailing theory regarding integrin activation is that FLNA binding to the β3 integrin serves primarily to retain the latter in a resting state (Liu et al., 2015), and that the dissociation of FLNA from the integrin promotes talin and kindlin binding to, and activation of, the integrin (Ithychanda et al., 2009). This contention is partially supported by a report of increased β3 integrin function conferred by a FLNA variant near the C-terminus (repeat 24) carried by a human subject (Berrou et al., 2017). The patient’s platelets exhibited increased aggregation, secretion, and αIIbβ3 integrin activity, as well as an increased association between talin and the β3 subunit (Berrou et al., 2017). The same research group recently generated a knock-in mouse that recapitulates the FLNA mutation; platelets from this knock-in mouse essentially replicate the gain-of-function phenotype observed in the human subject (Adam et al., 2022). These data clearly point to the functional importance of the FLNA-αIIbβ3 integrin association with regards to hemostasis and thrombosis. However, it should be noted that platelets completely devoid of FLNA exhibit comparable, yet not elevated, αIIbβ3 integrin activity relative to controls (Falet et al., 2010). This finding suggests that the regulation of integrin activity is perhaps more complex than is currently known, and that further research is required to fully validate the hypothesized role of FLNA as a strict suppressor of integrin activation.
FLNA interaction with PACSIN2
FLNA interacts with the adaptor protein PACSIN2, a member of the Bin/amphiphysin/Rvs (BAR) family of proteins that bind and tubulate membranes (Begonja et al., 2015). PACSIN2 has been implicated in receptor internalization, caveolae biogenesis, endosomal trafficking, and cell adhesion, spreading, and migration. In platelets, PACSIN2 colocalizes with GPIbα in membrane invaginations reminiscent of the open canalicular system (OCS), the membrane reservoir for platelet spreading and channels for granule secretion following platelet activation (Heijnen and van der Sluijs, 2015). In megakaryocytes, PACSIN2 colocalizes with the initiating demarcation membrane system (DMS), the highly invaginated membrane system that provides membrane for future platelets. This interaction requires FLNA Ig repeat 20 and the tip of PACSIN2 F-BAR domain, and regulates membrane tubulation in vitro, in platelets, and in megakaryocytes (Begonja et al., 2015). Single nucleotide polymorphisms in PACSIN2 have been associated with platelet count and size (Astle et al., 2016; Eicher et al., 2016; Chen et al., 2020; Vuckovic et al., 2020).
FLNA interactions with G-protein coupled receptors (GPCRs)
In addition to directly binding GPIbα and integrin αIIbβ3, FLNA also transduces signals from other platelet receptors. For example, thrombin is a serine protease and a potent physiological agonist that activates platelets at concentrations as low as 0.1 nM (Greco et al., 1995; Michelson, 2013). Thrombin signals via PARs (Vu et al., 1991), which are seven-transmembrane G-protein coupled receptors (GPCRs) (Michelson, 2013). Other platelet GPCRs include the P2Y12 receptor, which recognizes ADP, and the thromboxane receptor (TP receptor), which recognizes thromboxane A2 (Jurk and Kehrel, 2005; Dowal and Flaumenhaft, 2010). As mentioned in Section 1 above, PAR-driven signaling is thought to elicit changes in the actin cytoskeleton via Gq, PLCβ, rise in [Ca2+]i and PKC activation. However, a model has been proposed in which Ig repeat 21 of FLNA binds GPCRs with high affinity (Tirupula et al., 2015). Although this model has not been specifically validated in platelets, it does raise the interesting possibility that FLNA modulates thrombin-induced shape change via direct bridging of PARs with the actin cytoskeleton.
FLNA regulation of the platelet cytoskeleton via cytosolic proteins
FLNA regulation of Ca2+ signaling
The rise in intracellular calcium ([Ca2+]i) following platelet activation is essential for actin assembly. This is exemplified by data indicating that platelets fail to spread in the presence of the Ca2+-chelating agent BAPTA/AM (Mazharian et al., 2007). Accordingly, the [Ca2+]i rise is necessary for complete platelet aggregation and thrombus formation (Jardin et al., 2007; Varga-Szabo et al., 2008b). Interestingly, FLNA was recently found to regulate Ca2+ signaling in platelets. Lopez et al. reported that stromal interaction molecule 1 (STIM1), which serves as a calcium-sensing molecule at the endoplasmic reticulum (ER) (Lunz et al., 2019), co-immunoprecipitates with FLNA in thapsigargin-treated human platelets. Following activation-induced Ca2+ release from intracellular stores, ER-localized STIM1 interacts with Orai1, a Ca2+ release-activated channel on the plasma membrane (Galan et al., 2011). This interaction promotes store-operated calcium entry (SOCE) and the Ca2+-dependent platelet functions. Further, the authors reported that following siRNA knockdown of FLNA in platelets, the STIM1-Orai1 interaction (and the Ca2+) rise was accentuated. The authors conclude that FLNA regulates store-operated calcium entry (SOCE) process by restraining rises in [Ca2+]i thus avoiding Ca2+ overloading (Lopez et al., 2018). The finding of increased [Ca2+]i in the FLNA-knockdown platelets appears to stand in contrast with data obtained from FLNA-deficient mouse platelets, in which actin assembly is diminished (Falet et al., 2010). It is possible that FLNA’s modulation of [Ca2+]i fluxes is independent of its role in modulating shape change. Additional research is required to fully dissect the role(s) of FLNA in this complex biological system.
FLNA interactions with protein kinases
As noted in Table 1, FLNA interacts with multiple signaling molecules that modulate actin assembly. Syk is a tyrosine kinase that is a critical element of the GPVI-driven signaling pathway (Manne et al., 2015). Stimulation of ITAM- and hemITAM–containing receptors GPVI or CLEC2 lead to their phosphorylation and recruitment and activation of the tyrosine kinase Syk (Poole et al., 1997; Suzuki-Inoue et al., 2006). Syk then promotes activation of downstream signaling leading to increases in [Ca2+]i and inside-out activation of αIIbβ3 integrin (Ozaki et al., 2005). Notably, Syk was shown to be essential for lamellipodial formation and platelet spreading (Hughan et al., 2007). Moreover, FLNA Ig repeat 5 binds directly to Syk and regulates the ITAM-Syk signaling pathway by promoting Syk recruitment to the plasma membrane (Falet et al., 2010). Another kinase, protein kinase C (PKC), is activated by rises in [Ca2+]i and has been shown to regulate actin assembly in platelets (Harper and Poole, 2007; Harper and Poole, 2010). Interactions between FLNA and PKC have been documented in fibroblasts (Glogauer et al., 1998) and in HeLa cells (Tigges et al., 2003), and evidence also indicates that FLNA and PKC share a common function in regulating cell spreading (Kim et al., 2010) although this was not directly tested in platelets. Nevertheless, these data collectively support the notion that FLNA likely integrates multiple intracellular kinase pathways that regulate the actin cytoskeleton.
Other FLNA interacting proteins not documented in platelets
In addition to the proteins described above, there are multiple FLNA interacting proteins whose interactions with FLNA have not been specifically documented in platelets to date. Of particular relevance to actin cytoskeletal dynamics are the Rho GTPases. The formation of the cell membrane extensions characteristic of activated platelets (e.g. filopodia and lamellipodia) is catalyzed by the Rho GTPases Cdc42 and Rac1 (Nobes and Hall, 1995). Since Cdc42 and Rac1 reportedly bind FLNA Ig repeat 24 (Ohta et al., 1999), it is reasonable to speculate that FLNA directly modulates their activity since actin assembly is curtailed in FLNA-deficient platelets relative to controls (Falet et al., 2010). Conversely, another FLNA binding partner is FilGAP, which inactivates Rac1 (Ohta et al., 2006). FLNA binds FilGAP at the Ig repeat 23 and regulates FilGAP activity in cells (Ohta et al., 2006), possibly by approximating FilGAP and Rac1. These data suggest that FLNA may serve to constrain actin assembly by facilitating the inactivation of Rac1. Further work is clearly required to elucidate the precise determinants through which FLNA regulates actin dynamics in platelets.
BOX 2 Key points: FLNA as a plasma membrane-cytoskeleton scaffold
• Normal actin assembly in platelets is disrupted in the absence of FLNA.
• FLNA tethers platelet GPIbα, integrin αIIbβ3 (and possibly GPCRs) to the actin cytoskeleton.
• There exist a multitude of FLNA interacting proteins that regulate the cytoskeleton (although not all interactions with FLNA have been demonstrated in platelets).
Perspectives
Much is now known regarding the critical importance of FLNA in maintaining the integrity of both the plasma membrane and the cytoskeleton, and for relaying critical signals between these two structures. Data from platelets further underscore how FLNA integrates signaling pathways between the plasma membrane and the actin cytoskeleton, and also provide an explanation for the coagulopathies associated with FLNA gene variants.
Despite the advances in knowledge, open questions remain. For example, FLNA seemingly functions to suppress integrin αIIbβ3 function yet this integrin is not overactive in FLNA-null mouse platelets. Moreover, it is clear that FLNA is required for spreading of activated platelets although in some experiments, FLNA appears to constrain the [Ca2+]i rise that is normally required for actin assembly. Therefore, considerable additional research is still required to fully unravel the many facets of FLNA function in platelets and other cell types. This is particularly relevant given the large (and growing) number of proteins that interact with FLNA. Ultimately, an improved understanding of the FLNA-centric signaling networks, combined with detailed structural information on the specific protein-protein interactions, should identify viable therapeutic targets for managing coagulopathies and other diseases.
Author contributions
EDS wrote the first draft of the manuscript. FH, HF, and HK designed the concept and layout of the manuscript and/or wrote sections of the manuscript. All authors revised and approved the manuscript.
Funding
This work was supported by a Canadian Institutes of Health Research (CIHR) Project Grant (PJT-156341), a Michael Smith Foundation for Health Research (MSFHR) Scholar Award (to HK), and National Heart, Lung, and Blood Institute (NHLBI) R01 grant HL126743 (to HF). FH acknowledges support from a Research Training Studentship from the Canadian Venous Thromboembolism Research Network (CanVECTOR) and a Graduate Student Award (GAP) from the UBC Centre for Blood Research. EDS was supported by a Graduate Student Award from the UBC Centre for Blood Research and by a Training Graduate PhD Award from The Arthritis Society (19-0491).
Conflict of interest
The authors declare that the research was conducted in the absence of any commercial or financial relationships that could be construed as a potential conflict of interest.
Publisher’s note
All claims expressed in this article are solely those of the authors and do not necessarily represent those of their affiliated organizations, or those of the publisher, the editors and the reviewers. Any product that may be evaluated in this article, or claim that may be made by its manufacturer, is not guaranteed or endorsed by the publisher.
References
Adam, F., Kauskot, A., Lamrani, L., Solarz, J., Soukaseum, C., Reperant, C., et al. (2022). A gain-of-function filamin A mutation in mouse platelets induces thrombus instability. J. Thromb. Haemost. 20, 2666–2678. doi:10.1111/jth.15864
Aslan, J. E., Itakura, A., Haley, K. M., Tormoen, G. W., Loren, C. P., Baker, S. M., et al. (2013). p21 activated kinase signaling coordinates glycoprotein receptor VI-mediated platelet aggregation, lamellipodia formation, and aggregate stability under shear. Arterioscler. Thromb. Vasc. Biol. 33 (7), 1544–1551. doi:10.1161/ATVBAHA.112.301165
Aslan, J. E., and McCarty, O. J. (2013). Rho GTPases in platelet function. J. Thromb. Haemost. 11 (1), 35–46. doi:10.1111/jth.12051
Astle, W. J., Elding, H., Jiang, T., Allen, D., Ruklisa, D., Mann, A. L., et al. (2016). The allelic landscape of human blood cell trait variation and links to common complex disease. Cell 167 (5), 1415–1429. doi:10.1016/j.cell.2016.10.042
Azim, A. C., Barkalow, K., Chou, J., and Hartwig, J. H. (2000). Activation of the small GTPases, rac and cdc42, after ligation of the platelet PAR-1 receptor. Blood 95 (3), 959–964. doi:10.1182/blood.v95.3.959.003k22_959_964
Bearer, E. L., Prakash, J. M., and Li, Z. (2002). Actin dynamics in platelets. Int. Rev. Cytol. 217, 137–182. doi:10.1016/s0074-7696(02)17014-8
Begonja, A. J., Pluthero, F. G., Suphamungmee, W., Giannini, S., Christensen, H., Leung, R., et al. (2015). FlnA binding to PACSIN2 F-BAR domain regulates membrane tubulation in megakaryocytes and platelets. Blood 126 (1), 80–88. doi:10.1182/blood-2014-07-587600
Bellanger, J. M., Astier, C., Sardet, C., Ohta, Y., Stossel, T. P., and Debant, A. (2000). The Rac1- and RhoG-specific GEF domain of Trio targets filamin to remodel cytoskeletal actin. Nat. Cell Biol. 2 (12), 888–892. doi:10.1038/35046533
Bender, M., and Palankar, R. (2021). Platelet shape changes during thrombus formation: Role of actin-based protrusions. Hamostaseologie 41 (1), 14–21. doi:10.1055/a-1325-0993
Bennett, J. S., Berger, B. W., and Billings, P. C. (2009). The structure and function of platelet integrins. J. Thromb. Haemost. 7, 200–205. doi:10.1111/j.1538-7836.2009.03378.x
Berrou, E., Adam, F., Lebret, M., Fergelot, P., Kauskot, A., Coupry, I., et al. (2013). Heterogeneity of platelet functional alterations in patients with filamin A mutations. Arterioscler. Thromb. Vasc. Biol. 33 (1), e11–e18. doi:10.1161/ATVBAHA.112.300603
Berrou, E., Adam, F., Lebret, M., Planche, V., Fergelot, P., Issertial, O., et al. (2017). Gain-of-Function mutation in filamin A potentiates platelet integrin αIIbβ3 activation. Arterioscler. Thromb. Vasc. Biol. 37 (6), 1087–1097. doi:10.1161/ATVBAHA.117.309337
Bodie, S. L., Ford, I., Greaves, M., and Nixon, G. F. (2001). Thrombin-induced activation of RhoA in platelet shape change. Biochem. Biophys. Res. Commun. 287 (1), 71–76. doi:10.1006/bbrc.2001.5547
Chen, M., and Stracher, A. (1989). In situ phosphorylation of platelet actin-binding protein by cAMP-dependent protein kinase stabilizes it against proteolysis by calpain. J. Biol. Chem. 264 (24), 14282–14289. doi:10.1016/s0021-9258(18)71675-x
Chen, M. H., Raffield, L. M., Mousas, A., Sakaue, S., Huffman, J. E., Moscati, A., et al. (2020). Trans-ethnic and ancestry-specific blood-cell genetics in 746, 667 individuals from 5 global populations. Cell 182 (5), 1198–1213. doi:10.1016/j.cell.2020.06.045
Coughlin, S. R. (2000). Thrombin signalling and protease-activated receptors. Nature 407 (6801), 258–264. doi:10.1038/35025229
Cranmer, S. L., Ashworth, K. J., Yao, Y., Berndt, M. C., Ruggeri, Z. M., Andrews, R. K., et al. (2011). High shear-dependent loss of membrane integrity and defective platelet adhesion following disruption of the GPIbα-filamin interaction. Blood 117 (9), 2718–2727. doi:10.1182/blood-2010-07-296194
Cranmer, S. L., Pikovski, I., Mangin, P., Thompson, P. E., Domagala, T., Frazzetto, M., et al. (2005). Identification of a unique filamin A binding region within the cytoplasmic domain of glycoprotein Ibalpha. Biochem. J. 387, 849–858. doi:10.1042/BJ20041836
DeMali, K. A., Wennerberg, K., and Burridge, K. (2003). Integrin signaling to the actin cytoskeleton. Curr. Opin. Cell Biol. 15 (5), 572–582. doi:10.1016/s0955-0674(03)00109-1
Dowal, L., and Flaumenhaft, R. (2010). Targeting platelet G-protein coupled receptors (GPCRs): Looking beyond conventional GPCR antagonism. Curr. Vasc. Pharmacol. 8 (2), 140–154. doi:10.2174/157016110790886938
Dutting, S., Gaits-Iacovoni, F., Stegner, D., Popp, M., Antkowiak, A., van Eeuwijk, J. M. M., et al. (2017). A Cdc42/RhoA regulatory circuit downstream of glycoprotein Ib guides transendothelial platelet biogenesis. Nat. Commun. 8, 15838. doi:10.1038/ncomms15838
Ehrlicher, A. J., Nakamura, F., Hartwig, J. H., Weitz, D. A., and Stossel, T. P. (2011). Mechanical strain in actin networks regulates FilGAP and integrin binding to filamin A. Nature 478 (7368), 260–263. doi:10.1038/nature10430
Eicher, J. D., Chami, N., Kacprowski, T., Nomura, A., Chen, M. H., Yanek, L. R., et al. (2016). Platelet-related variants identified by exomechip meta-analysis in 157, 293 individuals. Am. J. Hum. Genet. 99 (1), 40–55. doi:10.1016/j.ajhg.2016.05.005
Etulain, J. (2018). Platelets in wound healing and regenerative medicine. Platelets 29 (6), 556–568. doi:10.1080/09537104.2018.1430357
Falet, H. (2017). “Anatomy of the platelet cytoskeleton,” in Platelets in thrombotic and non-thrombotic disorders. Editors P. Gresele, N. Kleiman, J. Lopez, and C. Page (Cham: Springer), 139–156.
Falet, H. (2013). New insights into the versatile roles of platelet FlnA. Platelets 24 (1), 1–5. doi:10.3109/09537104.2011.654004
Falet, H., Pollitt, A. Y., Begonja, A. J., Weber, S. E., Duerschmied, D., Wagner, D. D., et al. (2010). A novel interaction between FlnA and Syk regulates platelet ITAM-mediated receptor signaling and function. J. Exp. Med. 207 (9), 1967–1979. doi:10.1084/jem.20100222
Feghhi, S., Munday, A. D., Tooley, W. W., Rajsekar, S., Fura, A. M., Kulman, J. D., et al. (2016). Glycoprotein ib-IX-V complex transmits cytoskeletal forces that enhance platelet adhesion. Biophys. J. 111 (3), 601–608. doi:10.1016/j.bpj.2016.06.023
Feng, Y., and Walsh, C. A. (2004). The many faces of filamin: A versatile molecular scaffold for cell motility and signalling. Nat. Cell Biol. 6 (11), 1034–1038. doi:10.1038/ncb1104-1034
Galan, C., Dionisio, N., Smani, T., Salido, G. M., and Rosado, J. A. (2011). The cytoskeleton plays a modulatory role in the association between STIM1 and the Ca2+ channel subunits Orai1 and TRPC1. Biochem. Pharmacol. 82 (4), 400–410. doi:10.1016/j.bcp.2011.05.017
Ginsberg, M. H. (2014). Integrin activation. BMB Rep. 47 (12), 655–659. doi:10.5483/bmbrep.2014.47.12.241
Glogauer, M., Arora, P., Chou, D., Janmey, P. A., Downey, G. P., and McCulloch, C. A. (1998). The role of actin-binding protein 280 in integrin-dependent mechanoprotection. J. Biol. Chem. 273 (3), 1689–1698. doi:10.1074/jbc.273.3.1689
Greco, N. J., Tandon, N. N., and Jamieson, G. A. (1995). Thrombin binding to platelets defines functional receptors: Inhibition of thrombin-induced platelet activation by catalytically-inactivated thrombin. Platelets 6 (5), 270–274. doi:10.3109/09537109509023566
Harper, M. T., and Poole, A. W. (2010). Diverse functions of protein kinase C isoforms in platelet activation and thrombus formation. J. Thromb. Haemost. 8 (3), 454–462. doi:10.1111/j.1538-7836.2009.03722.x
Harper, M. T., and Poole, A. W. (2007). Isoform-specific functions of protein kinase C: The platelet paradigm. Biochem. Soc. Trans. 35, 1005–1008. doi:10.1042/BST0351005
Heijnen, H., and van der Sluijs, P. (2015). Platelet secretory behaviour: As diverse as the granules . or not? J. Thromb. Haemost. 13 (12), 2141–2151. doi:10.1111/jth.13147
Hou, Y., Carrim, N., Wang, Y., Gallant, R. C., Marshall, A., and Ni, H. (2015). Platelets in hemostasis and thrombosis: Novel mechanisms of fibrinogen-independent platelet aggregation and fibronectin-mediated protein wave of hemostasis. J. Biomed. Res. 29, 437–444. doi:10.7555/JBR.29.20150121
Huang, J., Li, X., Shi, X., Zhu, M., Wang, J., Huang, S., et al. (2019). Platelet integrin αIIbβ3: Signal transduction, regulation, and its therapeutic targeting. J. Hematol. Oncol. 12 (1), 26. doi:10.1186/s13045-019-0709-6
Hughan, S. C., Hughes, C. E., McCarty, O. J., Schweighoffer, E., Soultanova, I., Ware, J., et al. (2007). GPVI potentiation of platelet activation by thrombin and adhesion molecules independent of Src kinases and Syk. Arterioscler. Thromb. Vasc. Biol. 27 (2), 422–429. doi:10.1161/01.ATV.0000252826.96134.21
Ithychanda, S. S., Das, M., Ma, Y. Q., Ding, K., Wang, X., Gupta, S., et al. (2009). Migfilin, a molecular switch in regulation of integrin activation. J. Biol. Chem. 284 (7), 4713–4722. doi:10.1074/jbc.M807719200
Jackson, S. P., and Schoenwaelder, S. M. (2010). PI 3-Kinase p110β regulation of platelet integrin α(IIb)β3. Curr. Top. Microbiol. Immunol. 346, 203–224. doi:10.1007/82_2010_61
Jardin, I., Ben Amor, N., Hernandez-Cruz, J. M., Salido, G. M., and Rosado, J. A. (2007). Involvement of SNARE proteins in thrombin-induced platelet aggregation: Evidence for the relevance of Ca2+ entry. Arch. Biochem. Biophys. 465 (1), 16–25. doi:10.1016/j.abb.2007.04.038
Job, C., and Lagnado, L. (1998). Calcium and protein kinase C regulate the actin cytoskeleton in the synaptic terminal of retinal bipolar cells. J. Cell Biol. 143 (6), 1661–1672. doi:10.1083/jcb.143.6.1661
Jurak Begonja, A., Hoffmeister, K. M., Hartwig, J. H., and Falet, H. (2011). FlnA-null megakaryocytes prematurely release large and fragile platelets that circulate poorly. Blood 118 (8), 2285–2295. doi:10.1182/blood-2011-04-348482
Jurk, K., and Kehrel, B. E. (2005). Platelets: Physiology and biochemistry. Semin. Thromb. Hemost. 31 (4), 381–392. doi:10.1055/s-2005-916671
Kanaji, T., Ware, J., Okamura, T., and Newman, P. J. (2012). GPIbα regulates platelet size by controlling the subcellular localization of filamin. Blood 119 (12), 2906–2913. doi:10.1182/blood-2011-08-376566
Kiema, T., Lad, Y., Jiang, P., Oxley, C. L., Baldassarre, M., Wegener, K. L., et al. (2006). The molecular basis of filamin binding to integrins and competition with talin. Mol. Cell 21 (3), 337–347. doi:10.1016/j.molcel.2006.01.011
Kim, H., and McCulloch, C. A. (2011). Filamin A mediates interactions between cytoskeletal proteins that control cell adhesion. FEBS Lett. 585 (1), 18–22. doi:10.1016/j.febslet.2010.11.033
Kim, H., Nakamura, F., Lee, W., Shifrin, Y., Arora, P., and McCulloch, C. A. (2010). Filamin A is required for vimentin-mediated cell adhesion and spreading. Am. J. Physiol. Cell Physiol. 298 (2), C221–C236. doi:10.1152/ajpcell.00323.2009
Lad, Y., Jiang, P., Ruskamo, S., Harburger, D. S., Ylanne, J., Campbell, I. D., et al. (2008). Structural basis of the migfilin-filamin interaction and competition with integrin beta tails. J. Biol. Chem. 283 (50), 35154–35163. doi:10.1074/jbc.M802592200
Liu, J., Das, M., Yang, J., Ithychanda, S. S., Yakubenko, V. P., Plow, E. F., et al. (2015). Structural mechanism of integrin inactivation by filamin. Nat. Struct. Mol. Biol. 22 (5), 383–389. doi:10.1038/nsmb.2999
Lopez, J. J., Albarran, L., Jardin, I., Sanchez-Collado, J., Redondo, P. C., Bermejo, N., et al. (2018). Filamin A modulates store-operated Ca(2+) entry by regulating STIM1 (stromal interaction molecule 1)-orai1 association in human platelets. Arterioscler. Thromb. Vasc. Biol. 38 (2), 386–397. doi:10.1161/ATVBAHA.117.310139
Lunz, V., Romanin, C., and Frischauf, I. (2019). STIM1 activation of Orai1. Cell Calcium 77, 29–38. doi:10.1016/j.ceca.2018.11.009
Ma, Y. Q., Qin, J., and Plow, E. F. (2007). Platelet integrin alpha(IIb)beta(3): Activation mechanisms. J. Thromb. Haemost. 5 (7), 1345–1352. doi:10.1111/j.1538-7836.2007.02537.x
Maestrini, E., Patrosso, C., Mancini, M., Rivella, S., Rocchi, M., Repetto, M., et al. (1993). Mapping of two genes encoding isoforms of the actin binding protein ABP-280, a dystrophin like protein, to Xq28 and to chromosome 7. Hum. Mol. Genet. 2 (6), 761–766. doi:10.1093/hmg/2.6.761
Manne, B. K., Badolia, R., Dangelmaier, C., Eble, J. A., Ellmeier, W., Kahn, M., et al. (2015). Distinct pathways regulate Syk protein activation downstream of immune tyrosine activation motif (ITAM) and hemITAM receptors in platelets. J. Biol. Chem. 290 (18), 11557–11568. doi:10.1074/jbc.M114.629527
Mazharian, A., Roger, S., Berrou, E., Adam, F., Kauskot, A., Nurden, P., et al. (2007). Protease-activating receptor-4 induces full platelet spreading on a fibrinogen matrix: Involvement of ERK2 and p38 and Ca2+ mobilization. J. Biol. Chem. 282 (8), 5478–5487. doi:10.1074/jbc.M609881200
Meyer, S. C., Zuerbig, S., Cunningham, C. C., Hartwig, J. H., Bissell, T., Gardner, K., et al. (1997). Identification of the region in actin-binding protein that binds to the cytoplasmic domain of glycoprotein IBalpha. J. Biol. Chem. 272 (5), 2914–2919. doi:10.1074/jbc.272.5.2914
Morse, E. M., Brahme, N. N., and Calderwood, D. A. (2014). Integrin cytoplasmic tail interactions. Biochemistry 53 (5), 810–820. doi:10.1021/bi401596q
Moser, M., Nieswandt, B., Ussar, S., Pozgajova, M., and Fassler, R. (2008). Kindlin-3 is essential for integrin activation and platelet aggregation. Nat. Med. 14 (3), 325–330. doi:10.1038/nm1722
Nakamura, F., Osborn, T. M., Hartemink, C. A., Hartwig, J. H., and Stossel, T. P. (2007). Structural basis of filamin A functions. J. Cell Biol. 179 (5), 1011–1025. doi:10.1083/jcb.200707073
Nakamura, F., Pudas, R., Heikkinen, O., Permi, P., Kilpelainen, I., Munday, A. D., et al. (2006). The structure of the GPIb-filamin A complex. Blood 107 (5), 1925–1932. doi:10.1182/blood-2005-10-3964
Nieswandt, B., Varga-Szabo, D., and Elvers, M. (2009). Integrins in platelet activation. J. Thromb. Haemost. 7, 206–209. doi:10.1111/j.1538-7836.2009.03370.x
Nobes, C. D., and Hall, A. (1995). Rho, rac, and cdc42 GTPases regulate the assembly of multimolecular focal complexes associated with actin stress fibers, lamellipodia, and filopodia. Cell 81 (1), 53–62. doi:10.1016/0092-8674(95)90370-4
Nurden, P., Debili, N., Coupry, I., Bryckaert, M., Youlyouz-Marfak, I., Sole, G., et al. (2011). Thrombocytopenia resulting from mutations in filamin A can be expressed as an isolated syndrome. Blood 118 (22), 5928–5937. doi:10.1182/blood-2011-07-365601
Ohta, Y., Hartwig, J. H., and Stossel, T. P. (2006). FilGAP, a Rho- and ROCK-regulated GAP for Rac binds filamin A to control actin remodelling. Nat. Cell Biol. 8 (8), 803–814. doi:10.1038/ncb1437
Ohta, Y., Suzuki, N., Nakamura, S., Hartwig, J. H., and Stossel, T. P. (1999). The small GTPase RalA targets filamin to induce filopodia. Proc. Natl. Acad. Sci. U. S. A. 96 (5), 2122–2128. doi:10.1073/pnas.96.5.2122
Ozaki, Y., Asazuma, N., Suzuki-Inoue, K., and Berndt, M. C. (2005). Platelet GPIb-IX-V-dependent signaling. J. Thromb. Haemost. 3 (8), 1745–1751. doi:10.1111/j.1538-7836.2005.01379.x
Petrich, B. G., Fogelstrand, P., Partridge, A. W., Yousefi, N., Ablooglu, A. J., Shattil, S. J., et al. (2007). The antithrombotic potential of selective blockade of talin-dependent integrin alpha IIb beta 3 (platelet GPIIb-IIIa) activation. J. Clin. Invest. 117 (8), 2250–2259. doi:10.1172/JCI31024
Petrich, B. G., Marchese, P., Ruggeri, Z. M., Spiess, S., Weichert, R. A., Ye, F., et al. (2007). Talin is required for integrin-mediated platelet function in hemostasis and thrombosis. J. Exp. Med. 204 (13), 3103–3111. doi:10.1084/jem.20071800
Pollard, T. D. (2016). Actin and actin-binding proteins. Cold Spring Harb. Perspect. Biol. 8 (8), a018226. doi:10.1101/cshperspect.a018226
Poole, A., Gibbins, J. M., Turner, M., van Vugt, M. J., van de Winkel, J. G., Saito, T., et al. (1997). The Fc receptor gamma-chain and the tyrosine kinase Syk are essential for activation of mouse platelets by collagen. EMBO J. 16 (9), 2333–2341. doi:10.1093/emboj/16.9.2333
Robertson, S. P. (2005). Filamin A: Phenotypic diversity. Curr. Opin. Genet. Dev. 15 (3), 301–307. doi:10.1016/j.gde.2005.04.001
Robertson, S. P. (2007). Otopalatodigital syndrome spectrum disorders: Otopalatodigital syndrome types 1 and 2, frontometaphyseal dysplasia and melnick-needles syndrome. Eur. J. Hum. Genet. 15 (1), 3–9. doi:10.1038/sj.ejhg.5201654
Robertson, S. P., Twigg, S. R., Sutherland-Smith, A. J., Biancalana, V., Gorlin, R. J., Horn, D., et al. (2003). Localized mutations in the gene encoding the cytoskeletal protein filamin A cause diverse malformations in humans. Nat. Genet. 33 (4), 487–491. doi:10.1038/ng1119
Romero, S., Le Clainche, C., and Gautreau, A. M. (2020). Actin polymerization downstream of integrins: Signaling pathways and mechanotransduction. Biochem. J. 477 (1), 1–21. doi:10.1042/BCJ20170719
Rosa, J. P., Raslova, H., and Bryckaert, M. (2019). Filamin A: Key actor in platelet biology. Blood 134 (16), 1279–1288. doi:10.1182/blood.2019000014
Ruskamo, S., Gilbert, R., Hofmann, G., Jiang, P., Campbell, I. D., Ylanne, J., et al. (2012). The C-terminal rod 2 fragment of filamin A forms a compact structure that can be extended. Biochem. J. 446 (2), 261–269. doi:10.1042/BJ20120361
Sang, Y., Roest, M., de Laat, B., de Groot, P. G., and Huskens, D. (2021). Interplay between platelets and coagulation. Blood Rev. 46, 100733. doi:10.1016/j.blre.2020.100733
Savoy, R. M., and Ghosh, P. M. (2013). The dual role of filamin A in cancer: can't live with (too much of) it, can't live without it. Endocr. Relat. Cancer 20 (6), R341–R356. doi:10.1530/ERC-13-0364
Shattil, S. J., and Newman, P. J. (2004). Integrins: Dynamic scaffolds for adhesion and signaling in platelets. Blood 104 (6), 1606–1615. doi:10.1182/blood-2004-04-1257
Shen, Y., Romo, G. M., Dong, J. F., Schade, A., McIntire, L. V., Kenny, D., et al. (2000). Requirement of leucine-rich repeats of glycoprotein (GP) Ibα for shear-dependent and static binding of von Willebrand factor to the platelet membrane GP Ib–IX-V complex. Blood 95 (3), 903–910. doi:10.1182/blood.v95.3.903.003k37_903_910
Shifrin, Y., Arora, P. D., Ohta, Y., Calderwood, D. A., and McCulloch, C. A. (2009). The role of FilGAP-filamin A interactions in mechanoprotection. Mol. Biol. Cell 20 (5), 1269–1279. doi:10.1091/mbc.e08-08-0872
Sorrentino, S., Studt, J. D., Medalia, O., and Tanuj Sapra, K. (2015). Roll, adhere, spread and contract: Structural mechanics of platelet function. Eur. J. Cell Biol. 94 (3-4), 129–138. doi:10.1016/j.ejcb.2015.01.001
Stossel, T. P., Condeelis, J., Cooley, L., Hartwig, J. H., Noegel, A., Schleicher, M., et al. (2001). Filamins as integrators of cell mechanics and signalling. Nat. Rev. Mol. Cell Biol. 2 (2), 138–145. doi:10.1038/35052082
Sun, C., Forster, C., Nakamura, F., and Glogauer, M. (2013). Filamin-A regulates neutrophil uropod retraction through RhoA during chemotaxis. PLoS One 8 (10), e79009. doi:10.1371/journal.pone.0079009
Suzuki-Inoue, K., Fuller, G. L., García, A., Eble, J. A., Pöhlmann, S., Inoue, O., et al. (2006). A novel Syk-dependent mechanism of platelet activation by the C-type lectin receptor CLEC-2. Blood 107 (2), 542–549. doi:10.1182/blood-2005-05-1994
Takafuta, T., Wu, G., Murphy, G. F., and Shapiro, S. S. (1998). Human beta-filamin is a new protein that interacts with the cytoplasmic tail of glycoprotein Ibalpha. J. Biol. Chem. 273 (28), 17531–17538. doi:10.1074/jbc.273.28.17531
Tanner, L. M., Kunishima, S., Lehtinen, E., Helin, T., Volmonen, K., Lassila, R., et al. (2022). Platelet function and filamin A expression in two families with novel FLNA gene mutations associated with periventricular nodular heterotopia and panlobular emphysema. Am. J. Med. Genet. A 188 (6), 1716–1722. doi:10.1002/ajmg.a.62690
Tigges, U., Koch, B., Wissing, J., Jockusch, B. M., and Ziegler, W. H. (2003). The F-actin cross-linking and focal adhesion protein filamin A is a ligand and in vivo substrate for protein kinase C alpha. J. Biol. Chem. 278 (26), 23561–23569. doi:10.1074/jbc.M302302200
Tirupula, K. C., Ithychanda, S. S., Mohan, M. L., Naga Prasad, S. V., Qin, J., and Karnik, S. S. (2015). G protein-coupled receptors directly bind filamin A with high affinity and promote filamin phosphorylation. Biochemistry 54 (44), 6673–6683. doi:10.1021/acs.biochem.5b00975
Tossavainen, H., Koskela, O., Jiang, P., Ylanne, J., Campbell, I. D., Kilpelainen, I., et al. (2012). Model of a six immunoglobulin-like domain fragment of filamin A (16-21) built using residual dipolar couplings. J. Am. Chem. Soc. 134 (15), 6660–6672. doi:10.1021/ja2114882
Ueda, K., Ohta, Y., and Hosoya, H. (2003). The carboxy-terminal pleckstrin homology domain of ROCK interacts with filamin-A. Biochem. Biophys. Res. Commun. 301 (4), 886–890. doi:10.1016/s0006-291x(03)00048-2
Vadlamudi, R. K., Li, F., Adam, L., Nguyen, D., Ohta, Y., Stossel, T. P., et al. (2002). Filamin is essential in actin cytoskeletal assembly mediated by p21-activated kinase 1. Nat. Cell Biol. 4 (9), 681–690. doi:10.1038/ncb838
van der Flier, A., Kuikman, I., Kramer, D., Geerts, D., Kreft, M., Takafuta, T., et al. (2002). Different splice variants of filamin-B affect myogenesis, subcellular distribution, and determine binding to integrin [beta] subunits. J. Cell Biol. 156 (2), 361–376. doi:10.1083/jcb.200103037
Varga-Szabo, D., Braun, A., Kleinschnitz, C., Bender, M., Pleines, I., Pham, M., et al. (2008). The calcium sensor STIM1 is an essential mediator of arterial thrombosis and ischemic brain infarction. J. Exp. Med. 205 (7), 1583–1591. doi:10.1084/jem.20080302
Varga-Szabo, D., Pleines, I., and Nieswandt, B. (2008). Cell adhesion mechanisms in platelets. Arterioscler. Thromb. Vasc. Biol. 28 (3), 403–412. doi:10.1161/ATVBAHA.107.150474
Vassallo, P., Westbury, S. K., and Mumford, A. D. (2020). FLNA variants associated with disorders of platelet number or function. Platelets 31 (8), 1097–1100. doi:10.1080/09537104.2020.1748587
Vu, T. K., Hung, D. T., Wheaton, V. I., and Coughlin, S. R. (1991). Molecular cloning of a functional thrombin receptor reveals a novel proteolytic mechanism of receptor activation. Cell 64 (6), 1057–1068. doi:10.1016/0092-8674(91)90261-v
Vuckovic, D., Bao, E. L., Akbari, P., Lareau, C. A., Mousas, A., Jiang, T., et al. (2020). The polygenic and monogenic basis of blood traits and diseases. Cell 182 (5), 1214–1231. doi:10.1016/j.cell.2020.08.008
Wersall, A., Williams, C. M., Brown, E., Iannitti, T., Williams, N., and Poole, A. W. (2018). Mouse platelet ral GTPases control P-selectin surface expression, regulating platelet-leukocyte interaction. Arterioscler. Thromb. Vasc. Biol. 38 (4), 787–800. doi:10.1161/ATVBAHA.117.310294
Williamson, D., Pikovski, I., Cranmer, S. L., Mangin, P., Mistry, N., Domagala, T., et al. (2002). Interaction between platelet glycoprotein Ibalpha and filamin-1 is essential for glycoprotein Ib/IX receptor anchorage at high shear. J. Biol. Chem. 277 (3), 2151–2159. doi:10.1074/jbc.M109384200
Yang, X., Chitalia, S. V., Matsuura, S., and Ravid, K. (2022). Integrins and their role in megakaryocyte development and function. Exp. Hematol. 106, 31–39. doi:10.1016/j.exphem.2021.11.007
Yates, L. A., Fuzery, A. K., Bonet, R., Campbell, I. D., and Gilbert, R. J. (2012). Biophysical analysis of Kindlin-3 reveals an elongated conformation and maps integrin binding to the membrane-distal beta-subunit NPXY motif. J. Biol. Chem. 287 (45), 37715–37731. doi:10.1074/jbc.M112.415208
Zhang, Y., Ehrlich, S. M., Zhu, C., and Du, X. (2022). Signaling mechanisms of the platelet glycoprotein Ib-IX complex. Platelets 33 (6), 823–832. doi:10.1080/09537104.2022.2071852
Zhou, A. X., Hartwig, J. H., and Akyurek, L. M. (2010). Filamins in cell signaling, transcription and organ development. Trends Cell Biol. 20 (2), 113–123. doi:10.1016/j.tcb.2009.12.001
Zhou, J., Kang, X., An, H., Lv, Y., and Liu, X. (2021). The function and pathogenic mechanism of filamin A. Gene 784, 145575. doi:10.1016/j.gene.2021.145575
Zhou, K., Xia, Y., Yang, M., Xiao, W., Zhao, L., Hu, R., et al. (2022). Actin polymerization regulates glycoprotein Ibα shedding. Platelets 33 (3), 381–389. doi:10.1080/09537104.2021.1922882
Keywords: platelets, filamin A, cytoskeleton, cell signaling, actin
Citation: De Silva E, Hong F, Falet H and Kim H (2022) Filamin A in platelets: Bridging the (signaling) gap between the plasma membrane and the actin cytoskeleton. Front. Mol. Biosci. 9:1060361. doi: 10.3389/fmolb.2022.1060361
Received: 03 October 2022; Accepted: 30 November 2022;
Published: 20 December 2022.
Edited by:
Esther Garcia, University of Glasgow, United KingdomReviewed by:
Kabir H. Biswas, Hamad bin Khalifa University, QatarCopyright © 2022 De Silva, Hong, Falet and Kim. This is an open-access article distributed under the terms of the Creative Commons Attribution License (CC BY). The use, distribution or reproduction in other forums is permitted, provided the original author(s) and the copyright owner(s) are credited and that the original publication in this journal is cited, in accordance with accepted academic practice. No use, distribution or reproduction is permitted which does not comply with these terms.
*Correspondence: Hugh Kim, aHVnaGtpbUBkZW50aXN0cnkudWJjLmNh
†These authors have contributed equally to this work