- 1College of Traditional Chinese Medicine, Changchun University of Chinese Medicine, Changchun, China
- 2Research Center of Traditional Chinese Medicine, The First Affiliated Hospital of Changchun University of Chinese Medicine, Changchun, China
- 3Department of Endocrinology, The First Affiliated Hospital of Changchun University of Chinese Medicine, Changchun, China
- 4School of Life Sciences, Zhejiang Chinese Medical University, Hangzhou, China
- 5Northeast Asia Research Institute of Traditional Chinese Medicine, Key Laboratory of Active Substances and Biological Mechanisms of Ginseng Efficacy, Changchun University of Chinese Medicine, Changchun, China
Ferroptosis is characterized by the accumulation of iron and lipid peroxidation products, which regulates physiological and pathological processes in numerous organs and tissues. A growing body of research suggests that ferroptosis is a key causative factor in a variety of skeletal muscle diseases, including sarcopenia, rhabdomyolysis, rhabdomyosarcoma, and exhaustive exercise-induced fatigue. However, the relationship between ferroptosis and various skeletal muscle diseases has not been investigated systematically. This review’s objective is to provide a comprehensive summary of the mechanisms and signaling factors that regulate ferroptosis, including lipid peroxidation, iron/heme, amino acid metabolism, and autophagy. In addition, we tease out the role of ferroptosis in the progression of different skeletal muscle diseases and ferroptosis as a potential target for the treatment of multiple skeletal muscle diseases. This review can provide valuable reference for the research on the pathogenesis of skeletal muscle diseases, as well as for clinical prevention and treatment.
1 Introduction
As proposed by Dixon in 2012, ferroptosis is a novel method of regulatory cell death (RCD) (Dixon et al., 2012). Ferroptosis is characterized by the accumulation of iron ions and products of lipid peroxidation, which are different from the currently known mechanisms for cell death, such as apoptosis, pyroptosis, necrosis, and autophagy (Dixon et al., 2012; Latunde-Dada, 2017; Li J. et al., 2020). Ferroptosis, on the other hand, displays unique morphological changes, such as mitochondrial shrinkage, an increase in membrane density, and diminished or absent mitochondrial cristae (Dixon et al., 2012; Yu et al., 2017). As research advances, the interaction among amino acids, lipids, and iron metabolism is considered to be the key to ferroptosis, and a number of related signaling pathways, genes, proteins, and organelles have been identified (Chen et al., 2017; Badgley et al., 2020; Zhang H. et al., 2020; Venkatesh et al., 2020; Yu et al., 2020; Li Y. et al., 2021; Kong et al., 2021). Furthermore, genes associated with autophagy, such as nuclear receptor coactivator 4 (NCOA4) and fanconi anemia complementation group D2 (FANCD2), have also been discovered as key regulators of ferroptosis (Hou et al., 2016; Song et al., 2016). Meanwhile, numerous studies have demonstrated that ferroptosis plays a crucial role in cancer, diabetes mellitus, chronic kidney disease, heart failure, and other disease processes, offering a promising perspective for future clinical treatment (Chen X. et al., 2019; Li D. et al., 2020; Mao et al., 2021; Wang et al., 2022).
Skeletal muscle is the largest organ of the human body (accounting for about 40% of the body weight) (Janssen et al., 2000), which is crucial for maintaining body movements, posture and essential movements (such as swallowing and breathing), glucose intake and temperature regulation (Ferrannini et al., 1988; Meyer et al., 2002; Fluck and Hoppeler, 2003; Klaus et al., 2005; Shiozu et al., 2015; Kanezaki et al., 2021). Meanwhile, the storage of amino acids and the secretion of muscle cytokines are also the key functions of skeletal muscle (Wolfe, 2006; Argiles et al., 2016; Hoffmann and Weigert, 2017). The former can provide the substrate needed for the generation of energy and protein in the body (Garber et al., 1976; Perez-Sala et al., 1987; Perriello et al., 1997; Hyde et al., 2005), and the latter can participate in a versatile of physiological and pathological processes such as inflammation regulation, insulin sensitivity, tumor growth inhibition and cognitive improvement (Pedersen and Febbraio, 2008; Hong et al., 2009; Ellingsgaard et al., 2011; Hojman et al., 2011; Wrann et al., 2013). The importance of skeletal muscle bestows the life-threatening perniciousness on skeletal muscle diseases. Among the diseases, not only do sarcopenia, rhabdomyolysis (RML), rhabdomyosarcoma (RMS) and exhaustive exercise-induced fatigue (EEIF) and other skeletal muscle diseases seriously affect the quality of life and body function of patients, but they can also induce or develop into a variety of acute/chronic diseases, such as diabetes, fractures, hypercalcemia and acute kidney injury (AKI) (Kawasaki et al., 1998; Lima et al., 2008; Liu et al., 2014; Oliveira and Vaz, 2015; Tsekoura et al., 2017; Welch et al., 2020). Therefore, it is an urgent medical problem to clarify the pathogenesis of different skeletal muscle diseases and seek effective therapeutic targets. Recent studies have found that ferroptosis is an important RCD that induces skeletal muscle cell death and prevents skeletal muscle proliferation and differentiation (Guerrero-Hue et al., 2019; Ding et al., 2021; Huang et al., 2021). At the same time, ferroptosis is repeatedly reported to be associated with skeletal muscle disease processes such as sarcopenia, RML, RMS, and EEIF (Guerrero-Hue et al., 2019; Dachert et al., 2020; Huang et al., 2021; Xiao et al., 2022). The exact role of ferroptosis in these diseases, however, has not been systematically elucidated.
In this review, the biological mechanisms and key regulators of ferroptosis that have been published so far will be elaborated on. What will also be summarized is the research progress of ferroptosis in various skeletal muscle diseases and possible related therapeutic target strategies, aiming to provide valuable information and new directions for ferroptosis to participate in the pathogenesis and treatment of skeletal muscle diseases.
2 The regulatory mechanism of ferroptosis
2.1 Lipid peroxidation and ferroptosis
As shown in current research, ferroptosis is caused by the accumulation of lipid peroxides and their decomposition products (Dixon et al., 2012; Yang and Stockwell, 2016; Feng and Stockwell, 2018). It is therefore important to understand the mechanisms of lipid peroxide production and clearance for the purpose of comprehending ferroptosis regulation.
2.1.1 Lipid peroxide production
Reactive oxygen species (ROS) are a group of highly active chemicals containing oxygen, including superoxide anion, hydrogen peroxide (H2O2), hydroxyl radical and peroxyl radical (Bayir, 2005; Yang et al., 2013). ROS produced in physiological process can regulate tissue homeostasis and transduce cell signal transduction (Rhee et al., 2000; Zhang H. et al., 2013; Zhang et al., 2016; Ferreira et al., 2018). The intracellular antioxidant system strictly monitors the generation and elimination of the ROS to maintain balance (He et al., 2017). When the oxidation and antioxidant systems are out of balance, a large amount of ROS produced will react with polyunsaturated fatty acids (PUFAs) in membrane phospholipids to form lipid peroxidation, which will change the fluidity and permeability of cell membrane and eventually lead to cell death (Farmer and Mueller, 2013; Catala and Diaz, 2016; Su et al., 2019). Ferroptosis, named for its iron ion dependence, is a typical representative of this cell death mode (Dixon et al., 2012; Yang and Stockwell, 2016). Fenton reaction, a non-enzymatic reaction mediated by iron, can promote the lipid peroxidation of PUFAs in membrane lipids by producing highly toxic hydroxyl radicals, thereby inducing ferroptosis (He et al., 2020). Enzymatic reaction is another important way to cause lipid peroxidation of PUFAs (Yamamoto, 1991; Lee et al., 2021). During enzymatic reaction, the esterification of free PUFAs and the insertion of these molecules into membrane phospholipids are achieved by Acyl-CoA synthetase long-chain family member 4 (ACSL4) and Lysophosphatidylcholine acyltransferase 3 (LPCAT3) (Dixon et al., 2015; Yuan et al., 2016a; Doll et al., 2017). Subsequently, lipoxygenase catalyzes lipid peroxidation of membrane phospholipids, which results in ferroptosis (Yang et al., 2016). Meanwhile, malondialdehyde (MDA) and 4-hydroxy-nonenal (4-HNE), which are produced during lipid peroxide degradation, can have adverse effects on the structure and function of proteins and nucleic acids (Ayala et al., 2014). Reducing lipid peroxidation has thus become a core step in the regulation of ferroptosis.
2.1.2 Role of antioxidant system in ferroptosis
Lipid peroxidation mediated by ROS can lead to ferroptosis (Su et al., 2019). The three important intracellular antioxidant systems, GSH system, CoQ10 system and BH4 system, are essential for scavenging ROS and maintaining redox stability (Schafer and Buettner, 2001; James et al., 2004; Mugoni et al., 2013; Hatem et al., 2014; Jazvinscak Jembrek et al., 2014; Xue et al., 2017). In a variety of studies, they have also been proved to be a crucial defense to protect cells from lipid peroxidation induced ferroptosis (Bersuker et al., 2019; Kraft et al., 2020; Chen et al., 2022b). Hence, in the following discussion, we will focus on the role of these three antioxidant systems in ferroptosis.
2.1.2.1 Glutathione system and ferroptosis
Glutathione (GSH) is a tripeptide composed of glutamate, cysteine and glycine, and also a paramount antioxidant in cells (Meister and Anderson, 1983). Glutathione peroxidase 4 (GPX4) is a selenoprotein with selenocysteine as its active center (Flohe et al., 1973; Rotruck et al., 1973; Ingold et al., 2018). GPX4 can convert toxic lipid hydroperoxides (L-OOH) into non-toxic lipid alcohol (L-OH) to prevent the accumulation of Fe2+ dependent lipid ROS on membrane lipids, thus playing a strong role in inhibiting ferroptosis (Imai and Nakagawa, 2003; Ursini and Maiorino, 2020). The transformation relies on the electrons provided by the process of GSH conversion into glutathione disulfide (GSSG) (Deponte, 2013). Subsequently, GSSG regenerates GSH under the catalysis of glutathione reductase (GR) and cofactor NADPH/H+, so as to continuously and circularly play an antioxidant role (Lu, 2009). Cysteine is the rate limiting substrate for GSH synthesis, and its availability directly affects the intracellular GSH level (Lu, 2009). Heavy chain solute carrier family 3 member 2 (SLC3A2, also known as CD98hc) and light chain solute carrier family 7 member 11 (SLC7A11, also known as XCT) construct the crucial amino acid transporter known as the cysteine/glutamate antiporter (system Xc−) (Bridges et al., 2012). Under system Xc−, cystine is exchanged 1:1 with intracellular glutamate and quickly reduced to cysteine for GSH synthesis and antioxidant defense (Mandal et al., 2010; Lewerenz et al., 2013). As a result, inhibiting the impairment of cystine uptake caused by system Xc− can directly result in GSH depletion and GPX4 inactivation, which contributes to the induction of ferroptosis. Erastin is a representative of this ferroptosis trigger mechanism and has been widely used in ferroptosis induction experiments (Zhao Y. et al., 2020; Wang et al., 2020). Meanwhile, numerous researches results have proved that blocking System Xc− to induce ferroptosis of cancer cells is an important mechanism for sorafenib to play an anti-cancer role (Li Y. et al., 2020; Li Z. J. et al., 2021), which provides a promising direction for inhibiting tumor growth.
In addition, in some mammalian cells, another way to maintain the supply of cysteine is the activation of transsulfuration pathway (McBean, 2012; Eriksson et al., 2015). Specifically, methionine, a sulfur donor from the diet, is converted into homocysteine (Mosharov et al., 2000; Sbodio et al., 2019). Then, homocysteine is condensed with serine under the catalysis of cystathionine β-synthase (CBS) to produce cystathionine, which finally produces cysteine through the action of cystathionine gamma-lyase (CSE) (Sbodio et al., 2019). It was found that cells with cysteinyl tRNA synthatase deletion can resist ferroptosis induced by erastin by up regulating genes and metabolites (cystathionine) in the transsulfuration pathway (Hayano et al., 2016). It is suggested that the transsulfuration pathway may be an alternative in inhibiting ferroptosis during cysteine deprivation. Further, cysteine produced by trans-sulfuration or uptake pathway combines with glutamate through GSH synthesis rate limiting enzyme glutamate cysteine ligase (GCL) (Lu, 2009). After the combination, GSH is finally synthesized under the catalysis of glutamate synthase (GSS) (Lu, 2009). The study found that GCL inhibition can induce ferroptosis of cancer cells (Nishizawa et al., 2018; Qin et al., 2021), contributing a new strategy for the development of cancer drugs.
2.1.2.2 Coenzyme Q 10 system with ferroptosis
Coenzyme Q 10 (CoQ10, also known as ubiquinone) is a potent fat-soluble antioxidant that has been found in several recent studies to be involved in the regulation of ferroptosis (Laredj et al., 2014; Bersuker et al., 2019; Doll et al., 2019). Specifically, ferroptosis suppressor protein 1 (FSP1) is recruited to the plasma membrane by myristoylated and reduce CoQ10 to ubiquinol (CoQ10H2) by using NAD (P) H, thereby trapping lipid peroxidation free radicals to prevent lipid peroxidation (Bersuker et al., 2019). It is worth noting that although CoQ10 exists in almost all lipid membranes of cells, only CoQ10 outside mitochondria can inhibit ferroptosis under FSP1 dependent modification (Stockwell, 2019). Bersuker et al. found that FSP1 is a necessary factor to maintain tumor cell activity and growth under the condition of GPX4 knockout, and its expression is positively related to the resistance of cells to GPX4 inhibitors (Bersuker et al., 2019). It is indicated that FSP1/CoQ10/NAD(P)H pathway is a parallel and complementary regulatory mechanism of ferroptosis with GSH/GPX4 pathway.
The mevalonate pathway is essential for ferroptosis regulation because its metabolic intermediate, isopentenyl pyrophosphate, is indispensable for CoQ10 and GPX4 biosynthesis (Holstein and Hohl, 2004; Moosmann and Behl, 2004; Friedmann Angeli and Conrad, 2018). It was found that ferroptosis inducing 56 can induce ferroptosis by degrading GPX4 and consuming CoQ10, which is achieved by interfering with the mevalonate pathway (Shimada et al., 2016). Therefore, it is necessary to further explore the regulatory effect of mevalonate pathway in ferroptosis.
2.1.2.3 Tetrahydrobioterin system and ferroptosis
Tetrahydrobioterin (BH4) has been found in recent studies to be a potent radical-trapping antioxidant that protects cells from ferroptosis by blocking lipid peroxidation transmission and acts independently of the GSH-dependent GPX4 protective pathway (Kraft et al., 2020; Soula et al., 2020). Guanosine triphosphate cyclohydrolase-1 (GCH1), the rate-limiting enzyme of BH4 synthesis, regenerates BH4 by catalyzing guanosine triphosphate (Thony et al., 2000). It was found that up-regulation of GCH1 restored the resistance of BH4-deficient cells to RSL3, indicating that GCH1 expression is decisive in the effectiveness of BH4 (Soula et al., 2020). In addition, BH4 may increase CoQ10 levels by converting phenylalanine into tyrosine, which provides a new perspective for its involvement in ferroptosis regulation (Kraft et al., 2020). However, the exact mechanism of BH4 system involved in the regulation of ferroptosis is still unclear, and further research is needed.
2.2 Iron metabolism and ferroptosis
Iron is a trace element necessary to maintain human life and health, and plays many essential physiological functions, including metabolism, oxygen transport, antioxidant reactions, electron transport, and DNA synthesis (Dlouhy and Outten, 2013). To maintain iron homeostasis, the body tightly regulates iron metabolism (including iron acquisition, utilization, storage, and efflux) (Wang and Pantopoulos, 2011). However, the destruction of iron homeostasis can lead to unstable iron accumulation and catalyze Fenton reaction, thus inducing ferroptosis (Winterbourn, 1995; Dixon et al., 2012; Henning et al., 2022). Therefore, regulation of iron metabolism is vital for the ferroptosis process.
2.2.1 Cellular iron metabolism and ferroptosis
A complex formed by transferrin (TF) containing iron and the transferrin receptor (TFRC) on the cell membrane enters the endosome through endocytosis under physiological conditions (Andrews, 2000; Hentze et al., 2010). An endosome containing the complex is acidified to promote the release of Fe3+ from TF, which is then reduced to Fe2+ by six-transmembrane epithelial antigen of prostate 3 (Steap3) (Ohgami et al., 2005), and the iron is transported to the unstable labile iron pool in the cytoplasm by the divalent metal transporter 1 (DMT1) (Fleming et al., 1998). Studies have shown that TFRC and DMT1 overexpression can promote unstable iron accumulation and trigger lipid peroxidation, thus becoming key regulators of ferroptosis (Song et al., 2021a; Zhang H. et al., 2021; Guo et al., 2021; Lu et al., 2021). Additionally, the high expression of heat shock protein beta-1 (HSPB1) prevents ferroptosis by inhibiting TFRC-mediated iron absorption (Chen et al., 2006; Sun et al., 2015). Ferritin is the major cytoplasmic iron storage protein complex, which includes ferritin light chain (FTL) and ferritin heavy chain 1 (FTH1) (Knovich et al., 2009). Ferroptosis can be inhibited by the ferroxidase activity of FTH1, which converts Fe2+ captured by ferritin into Fe3+ in order to reduce the production of ROS (Theil, 2013). A number of studies have confirmed that high expression of FTH1 can reduce susceptibility to ferroptosis in vivo and in vitro, respectively (Tian et al., 2020; Kong et al., 2021). Mammalian cells release iron through ferroportin 1 (FPN1) (Nemeth et al., 2004; Ward and Kaplan, 2012). A high level of FPN1 expression can promote iron efflux and protect cells from ferroptosis (Zhao X. et al., 2020; Tian et al., 2021). The iron regulatory protein 1/2 (IRP1/2) can bind to the iron responsive element (IRE) to regulate the expression of various iron metabolism proteins (DMT1, TFRC, FPN, FTH1/FTL), which is responsible for regulating iron homeostasis in cells (Aziz and Munro, 1987; Koeller et al., 1989; Xu et al., 2018; Xu M. et al., 2022). By promoting the expression of IRP1/2, the ferroptosis inducers erastin and RSL3 increase the susceptibility of melanoma cells to ferroptosis (Yao et al., 2021).
In general, each regulatory link in cellular iron metabolism affects the intracellular iron content and therefore contributes to ferroptosis.
2.2.2 Mitochondrial iron metabolism and ferroptosis
Iron metabolism in mitochondria is crucial to the control of cellular iron homeostasis. Iron transported from endosomes, cytosols, or ferritin traverses the outer membrane and inner membrane of mitochondria to reach the mitochondrial matrix, where it is utilized for the biosynthesis of heme and iron sulfur clusters or stored by mitochondrial ferritin (FtMt) to maintain cellular iron homeostasis (Paul et al., 2017). Mitoferrin 1/2 (Mfrn1/2) is a crucial component in facilitating iron transport across the inner mitochondrial membrane during this process (Paradkar et al., 2009). Studies have shown that reduced Mfrn expression may minimize ferroptosis by ameliorating mitochondrial iron excess (An et al., 2022; Zhang T. et al., 2022). Not only does FtMt store free iron in mitochondria, but it can also transport cytoplasmic iron to mitochondria, which is essential for controlling mitochondrial iron metabolism (Corsi et al., 2002; Drysdale et al., 2002). In vivo and in vitro, overexpression of FtMt abolished erastin-induced ferroptosis (Wang et al., 2016). In addition, the mitochondrial outer membrane protein CDGSH iron sulfur domain 1 (CISD1) was reported to reduce the sensitivity of liver cancer cells to erastin by inhibiting mitochondrial iron overload mediated lipid peroxidation (Yuan et al., 2016b), providing a new target for cancer treatment.
2.3 Heme metabolism and ferroptosis
Heme is the primary source of functional iron in the human body and a crucial component of erythropoiesis (Chung et al., 2012; Hooda et al., 2014). Therefore, the maintenance of systemic iron homeostasis requires the maintenance of a normal heme metabolism. The up-regulation of heme oxygenase 1 (HO-1), a crucial enzyme that degrades heme to liberate iron, can participate in ferroptosis induction by increasing intracellular iron levels (Tenhunen et al., 1968; Han et al., 2022). HO-1 inhibitor zinc protoporphyrin IX was discovered to counteract erastin-induced ferroptosis in HT-1080 fibrosarcoma cells (Kwon et al., 2015). HO-1-deficient proximal tubular cells, however, are very vulnerable to ferroptosis produced by erastin and RAS-selective lethal 3 (RSL3), which may be correlated to elevated heme levels after HO-1 deletion (Adedoyin et al., 2018). Heme metabolism related genes such as ATP-binding cassette subfamily B member 6 (ABCB6), Feline leukemia virus subgroup C receptor 1 (FLVCR1) and ATP-binding cassette subfamily G member 2 (ABCG2) were also found to be associated with ferroptosis (Tang et al., 2020; Zhang et al., 2020; Kawai et al., 2022). ABCB6 can transport the coproporphyrinogen III (CPgenIII) precursor of heme synthesis from the cytosol to the mitochondria, which is a key gene for heme synthesis (Krishnamurthy et al., 2006). As is shown in a research, low expression of ABCB6 in hepatocellular carcinoma may reduce the sensitivity of cancer cells to ferroptosis by reducing heme production (Zhang et al., 2020). Another study found that ABCB6 and FLVCR1 (an important carrier of cell surface heme output) were strongly positive and synergetic in tumor tissue, and were significantly down regulated after erastin intervention (Quigley et al., 2004; Keel et al., 2008; Tang et al., 2020). These results suggest that the heme content in cancer cells may affect their sensitivity to ferroptosis. In addition, as another important executor of heme export, part of ABCG2’s heme output capacity was found to be related to ferroptosis in recent studies (Desuzinges-Mandon et al., 2010; Kawai et al., 2022). But there is still no substantive research to support this finding yet. In general, heme metabolism is a promising research direction in the regulation mechanism of ferroptosis, which needs more attention and research (Figure 1; Table 1).
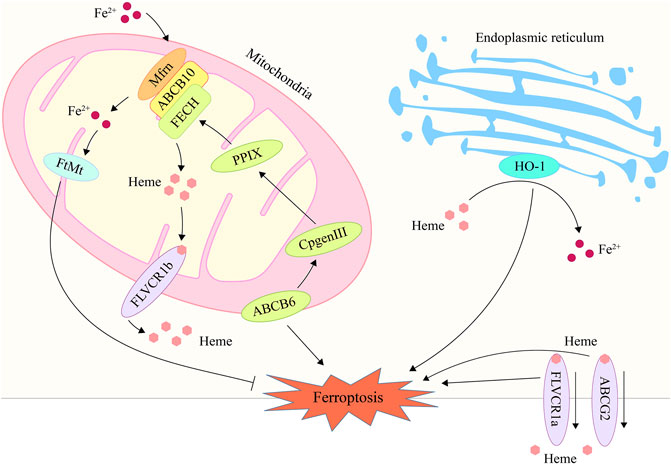
FIGURE 1. The processes of heme metabolism and mitochondrial iron metabolism are implicated in ferroptosis. In the endoplasmic reticulum, HO-1 degrades heme and releases iron ions, hence raising intracellular iron content and promoting ferroptosis. ABCB6 stimulates heme production by transferring CPgenIII from the cytosol to the mitochondrial membrane gap, a process that may play a role in ferroptosis. By exporting heme via the plasma membrane, ABCG2 and FLVCR1 contribute to the control of ferroptosis. Mfrn is capable of transporting iron through the inner membrane and into the mitochondrial matrix, hence elevating the iron concentration in mitochondria and inducing ferroptosis. FtMt may store free iron in mitochondria, hence reducing iron concentration and inhibiting ferroptosis. HO-1, heme oxygenase 1; ABCB6, ATP-binding cassette sub-family B member 6; ABCG2, ATP-binding cassette subfamily G member 2; Mfrn, mitoferrin; FtMt, mitochondrial ferritin.
2.4 Amino acid metabolism and ferroptosis
Amino acid metabolism is closely related to ferroptosis, one of the main reasons is that it participates in GSH synthesis (Te Braake et al., 2008; Sikalidis et al., 2014; Sun et al., 2018; Xu Y. et al., 2022). In addition to the above-mentioned cysteine, glutamate is another essential amino acid for GSH synthesis, and glutaminolysis is one of its sources (Whillier et al., 2011). By encouraging the conversion of glutamine to glutamate and boosting the synthesis of GSH, glutaminase 2 (GLS2) improves the antioxidant capacity of cells (Xiang et al., 2013). The ferroptosis resistance of cardiomyocytes can be significantly increased by targeted regulation of GLS2, according to studies (Zhou et al., 2021). However, a new regulatory mechanism for glutamate neurotoxicity is provided by the fact that high glutamate levels cause ferroptosis in neuronal cells by interfering with cystine uptake (Olney, 1971; Murphy et al., 1989; Jiang et al., 2020). Additionally, it has been demonstrated that the downstream metabolite of glutaminolysis, α-ketoglutarate, is involved in the production of lipid ROS, raising the possibility that ferroptosis influenced by glutaminolysis (Gao et al., 2015). Meanwhile, compound 968, a glutaminolysis inhibitor, can significantly reduce erastin sensitivity under cystine deficiency (Gao et al., 2015), further demonstrated the necessity of glutaminolysis for ferroptosis. It is interesting to note that the ferroptosis inhibitor ferrostatin-1 (Fer-1) completely restored the sharply decreased glutamine level under RSL3 intervention (Rodriguez-Graciani et al., 2022). Based on the aforementioned findings, it is possible that the amino acid environment in which cells are located plays a role in the bidirectional regulation of ferroptosis by glutaminolysis.
Other amino acids, such as branched chain amino acids, tryptophan and lysine, is pivotal in ferroptosis (Chepikova et al., 2020; Wang K. et al., 2021; Zeitler et al., 2021). It was shown that the branched-chain amino acid aminotransferase two and lysine oxidase can participate in the ferroptosis process by antagonizing the system Xc− inhibition and promoting H2O2 generation, respectively (Chepikova et al., 2020; Wang K. et al., 2021). Meanwhile, the tryptophan metabolite indole-3-pyruvate was recently found to negatively regulate ferroptosis through direct free radical depletion and upregulation of antioxidant genes (SLC7A11 and HO-1) (Zeitler et al., 2021). In addition, despite no relevant research report that arginine, serine and glycine participate in the regulation of ferroptosis, the three amino acids have shown the potential to fight ferroptosis in some studies (Possemato et al., 2011; Ye et al., 2014; Sen et al., 2018), which needs more exploration and excavation.
2.5 Other ferroptosis regulatory proteins
2.5.1 Nuclear factor erythroid 2-related factor 2
Nuclear factor erythroid 2-related factor 2 (Nrf2) is a crucial transcription factor for cell antioxidants and a crucial ferroptosis regulator (Dodson et al., 2019; Anandhan et al., 2020). To stop lipid peroxides-mediated ferroptosis, Nrf2 can directly control the expression level of the GPX4 protein and important genes for GSH synthesis, such as the catalytic and regulatory subunits of glutamate cysteine ligase (GCLC/GCLM), GSS, GR, and XCT (Chan and Kwong, 2000; Madduma Hewage et al., 2017; Feng et al., 2021; Scibior et al., 2021; Lu et al., 2022). Meanwhile, with the ability of targeting key genes (FTH1, FPN1) that regulate iron metabolism, Nrf2 can participate in the process of ferroptosis by affecting intracellular iron levels (Harada et al., 2011; Liu et al., 2020). In addition, Nrf2 also regulate the key genes of heme metabolism ABCB6, ABCG2, HO-1 and heme responsive gene-1 to participate in the process of ferroptosis (Hubner et al., 2009; Singh et al., 2010; Campbell et al., 2013; Dong et al., 2020). Among them, HO-1 is not only the key enzyme to decompose heme, but also an important antioxidant (Tenhunen et al., 1968; Niu et al., 2020). Multiple studies have shown that activating the antioxidant response axis Nrf2/HO-1 is a crucial means of inhibiting ferroptosis and ameliorating myocardial ischemia-reperfusion injury, ulcerative colitis, and acute lung injury (Chen et al., 2021b; Li J. et al., 2021; Lv et al., 2021). Kelch-like ECH-associated protein 1 (Keap1) is a substrate adaptor protein of E3 ubiquitin ligase, which tightly controls the activity of Nrf2 by way of the ubiquitin-proteasome system (Furukawa and Xiong, 2005; Yamamoto et al., 2018). In order to prevent Nrf2 degradation and to promote its nuclear translocation and maintain cellular redox homeostasis, p62 can bind to Keap1 in a competitive manner (Tan et al., 2021). Studies have shown that one of the effective ways to treat liver cancer, endometrial hyperplasia, and protect neurons is by mediating the p62/Keap1/Nrf2 pathway to regulate ferroptosis (Sun et al., 2016; Zhang M. et al., 2021; Li et al., 2022). Owing to that Nrf2 is extensive in the regulation of ferroptosis, targeting Nrf2 is of great significance in the treatment of ferroptosis related diseases.
2.5.2 P53
A crucial tumor suppressor gene called p53 is involved in the cell cycle, aging, apoptosis, and autophagy (Ong and Ramasamy, 2018). But more and more research has revealed that p53 also plays a significant part in controlling ferroptosis (Zhang Y. et al., 2021; Gao et al., 2021; Lei et al., 2021). Study has indicated that, as a direct target gene of p53, spermidine/spermine N1-acetyltransferase 1 (SAT1) can up-regulate arachidonate 15-lipoxygenase to promote ferroptosis due to accumulation of lipid peroxidation under ROS-induced stress (Ou et al., 2016). Other studies have shown that up-regulated p53 can affect GSH synthesis by inhibiting the expression of its downstream target gene SLC7A11, thereby inducing ferroptosis (Jiang et al., 2015; Guan et al., 2020). Meanwhile, by suppressing the expression of SLC7A11, p53 can indirectly activate the positive regulator of ferroptosis arachidonate 12-lipoxygenase (ALOX12) to promote ferroptosis (Chu et al., 2019). Additionally, p53 can facilitate dipeptidyl-peptidase 4’s (DPP4) nuclear translocation and join forces with it to form the DPP4-p53 complex, which lowers DPP4-dependent lipid peroxidation and inhibits ferroptosis in colorectal cancer cells (Xie et al., 2017). It has been suggested that p53 may mediate glutaminolysis to take part in the ferroptosis process because the essential enzyme for glutamine catabolism, GLS2, is also a direct target gene of p53 (Hu et al., 2010; Suzuki et al., 2010).
2.5.3 Nuclear receptor coactivator 4
One important mechanism for ferritin degradation is ferritinophagy, which can encourage the release of iron and provide a substrate for ferroptosis (Gao et al., 2016; Hou et al., 2016). A crucial regulator of ferritinophagy, NCOA4, can specifically bind to the surface arginine of FTH1 and promote ferritin degradation by lysosomes and autophagosomes (Mancias et al., 2014; Gryzik et al., 2017). Ferritinophagy also involves the traditional autophagy-related gene 3 (Atg3), Atg5, and Atg7, which are significant players (Hou et al., 2016). Knockout of Atg5, Atg7 or NCOA4 can effectively inhibited ferritin degradation to reduce erastin-induced ferroptosis (Hou et al., 2016). Furthermore, a new ferroptosis inhibitor compound 9a was found to block ferroptosis by disrupting the interaction of NCOA4-FTH1 (Fang et al., 2021), which opens up a new access for the development of ferroptosis inhibitors.
2.5.4 Fanconi anemia complementation group D2
As a nuclear protein involved in DNA damage repair, FANCD2 was discovered to be a key gene in autophagy-dependent ferroptosis (Nakanishi et al., 2002; Miao et al., 2022). Furthermore, FANCD2 mediates ferroptosis independently of autophagy (Song et al., 2016). The deletion of FANCD2 increases iron overload and lipid peroxidation in erastin-induced ferroptosis of bone marrow mesenchymal stem cells, which is associated with restricted expression of GPX4, FTH1, and upregulation of TFR1 (Song et al., 2016). It offers a novel approach to alleviating the side effects of bone marrow damage brought on by cancer treatment.
2.5.5 CDGSH iron sulfur domain 1
Recent research indicates that the outer mitochondrial membrane protein CDGSH iron sulfur domain 1 (CISD1) can inhibits mitochondrial iron uptake and lipid peroxidation, thereby negatively regulating erastin-induced ferroptosis (Yuan et al., 2016b). Meanwhile, pioglitazone inhibits iron-mediated mitochondrial lipid peroxidation and subsequent ferroptosis by binding CISD1 and stabilizing iron-sulfur clusters (Yuan et al., 2016b). Another study showed that the ferroptosis-related gene CISD1 is anticipated to become one of the novel biomarkers for predicting the prognosis of breast cancer patients, thereby providing a new target for cancer therapy (Wang D. et al., 2021) (Figure 2).
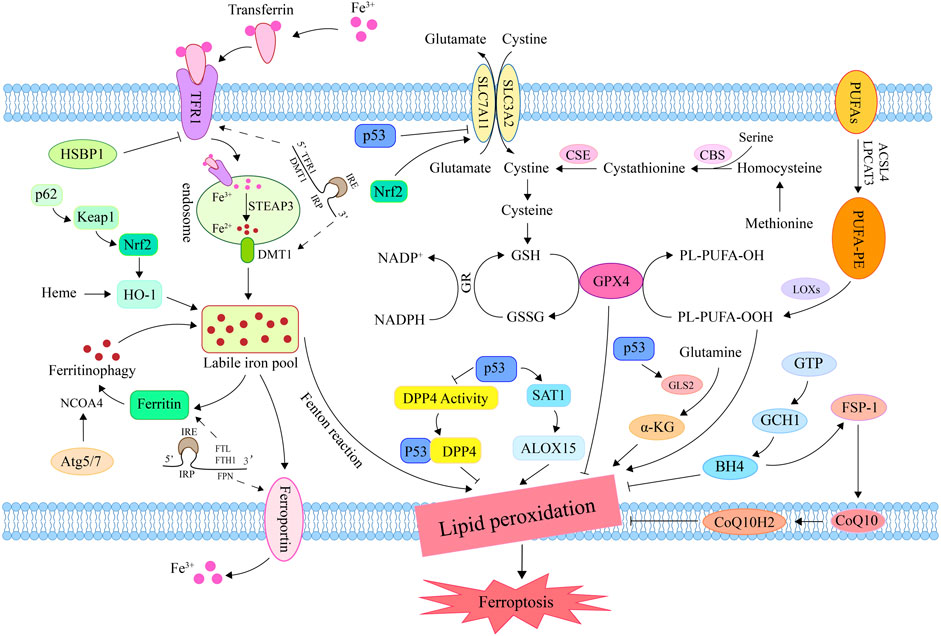
FIGURE 2. Mechanisms for regulating ferroptosis. Iron in circulation binds to TFR1 and enters the endosome via endocytosis; then, steap3 converts Fe3+ to Fe2+. DMT1 transports Fe2+ into the lip in order to promote unstable iron accumulation and induce ferroptosis via the Fenton reaction. Methionine generates cysteine under the action of CBS and CSE for GSH synthesis. Inhibition of system Xc− reduced GSH synthesis and inactivated GPX4, promoting lipid peroxide accumulation and inducing ferroptosis. Under the catalysis of ACSL4, LPCAT3, and LOX, PUFA lipid peroxidation resulted in ferroptosis. By promoting iron release, the ferritin autophagy-related genes Atg5, Atg7, and NCOA4 induce ferroptosis. By influencing genes related to iron/heme metabolism and amino acid metabolism, Nrf2 plays a significant role in the regulation of ferroptosis. By inhibiting DPP4 activity and systemic SLC7A11 expression, or by activating SAT1 and GLS2, P53 can induce ferroptosis. FSP1 prevents lipid peroxidation by converting CoQ10 to CoQ10H2, thereby inhibiting ferroptosis. GCH1 blocks the chain propagation of lipid peroxidation by catalyzing GTP to generate BH4. TFR1, transferrin receptor 1; DMT1, divalent metal transporter 1; CBS, cystathionine β-synthase; CSE, cystathionine gamma-lyase; GSH, glutathione; GPX4, glutathione peroxidase 4; ACSL4, Acyl-CoA synthetase long-chain family member 4; LPCAT3, lysophosphatidylcholine acyltransferase 3; LOX, lipoxygenase; PUFA, polyunsaturated fatty acid; Atg5, autophagy-related gene 3; Atg7, autophagy-related gene 7; NCOA4, nuclear receptor coactivator 4; Nrf2, nuclear factor erythroid 2-related factor 2; DPP4, dipeptidyl-peptidase 4; SLC7A11, solute carrier family 7 member 11; SAT1, spermidine/spermine N1-acetyltransferase 1; GLS2, glutaminase 2; FSP1, ferroptosis suppressor protein 1; CoQ10, ubiquinone; CoQ10H2, ubiquinol; GCH1, GTP cyclohydrolase-1; GTP, Guanosine triphosphate; BH4, tetrahydrobioterin.
3 The role of ferroptosis in skeletal muscle diseases
Ferroptosis has been widely concerned by researchers since its discovery, and has proved to play a pivotal role in the progress of human diseases in various systems, such as tumors, cardiovascular and cerebrovascular diseases, nervous system diseases, respiratory diseases and digestive diseases (Do Van et al., 2016; Fang et al., 2019; Badgley et al., 2020; Ma et al., 2020b; Guan et al., 2020; Bao et al., 2021; Wu et al., 2021; Zheng et al., 2021; Liu T. et al., 2022; Bao et al., 2022; Zhang Y. et al., 2022). In recent years, the functions of ferroptosis in a variety of skeletal muscle diseases has attracted the attention of researchers, and has been reported to be an overriding participant in the physiological and pathological processes such as sarcopenia, RML, RMS, and EEIF (Guerrero-Hue et al., 2019; Dachert et al., 2020; Huang et al., 2021; Xiao et al., 2022). Therefore, we summarized the functions of ferroptosis in the pathogenesis of these skeletal muscle diseases.
3.1 Ferroptosis and sarcopenia
Sarcopenia is an age-related degenerative loss of skeletal muscle strength and quality (Cruz-Jentoft et al., 2010). The main mechanism of its occurrence and development is the imbalance of muscle synthesis and degradation (Tan et al., 2020a), which is closely related to the decline of satellite cells (SCs) number/function (Brack et al., 2005; Budai et al., 2018). SCs are embryonic muscle stem cells located beneath the basal layer of muscle fibers and are in a quiescent state (Mauro, 1961; Yin et al., 2013). When muscle is damaged, SCs are activated and will proliferate into myoblasts, which will then differentiate and fuse to exert a powerful ability to promote muscle regeneration and repair damage (Aziz et al., 2012; Chen F. et al., 2019). However, some researches indicates that the number and function of SCs decline significantly with age, which would severely impair their capacity for self-renewal and regeneration and would lead to sarcopenia (Day et al., 2010; Jang et al., 2011; Sousa-Victor et al., 2014). Previous research has demonstrated that unstable iron accumulation existed in aging skeletal muscle and could promote muscle damage in mice by down-regulating SCs markers (paired box 7, myogenic differentiation antigen and myogenic factor 5) and inhibiting C2C12 myoblast differentiation (Ikeda et al., 2019). Another study found changes in ferroptosis related factors such as HO-1, SAT1 and prostaglandin-endoperoxide synthase 2 (Ptgs2) in muscle samples of elderly people with sarcopenia (Ding et al., 2021). It is suggested that SCs and C2C12 myoblasts may participate in sarcopenia disease through ferroptosis, which has been verified in several recent studies.
TFR1 is an important factor in the activation, proliferation, and maintenance of SCs, whereas the expression of TFR1 is significantly decreased in aging skeletal muscle (DeRuisseau et al., 2013; Ding et al., 2021). The research demonstrates that deletion of the TFR1 gene can promote zinc transporter Zip14 (Slc39a14) to absorb non-heme iron and down-regulate GPX4, Nrf2, and FTH1 to increase unstable iron accumulation and lipid peroxidation level, thereby inducing ferroptosis in skeletal muscle cells and impairing their regeneration (Ding et al., 2021). As is shown in another study, in animal models with sarcopenia, C2C12 myoblasts had age-related iron accumulation (Huang et al., 2021). Simultaneously, the activation of p53/SLC7A11 axis can induce ferroptosis of C2C12 myoblasts to hinder their differentiation into myotubes and to promote the progression of sarcopenia, which can be reversed by the ferroptosis inhibitors fer-1 and DFO (Huang et al., 2021). Targeted regulation of SCs/myoblast ferroptosis is therefore anticipated to become a new treatment strategy for sarcopenia. In addition, the CSE derivative hydrogen sulfide can inhibit the acetylation modification of ALOX12, prevent lipid peroxidation of phospholipid membrane, and protect myoblasts from ferroptosis (Wang Y. et al., 2021). It provides a new target for preventing ferroptosis in aging skeletal muscle, although the relationship to primary sarcopenia was not explored (Figure 3).
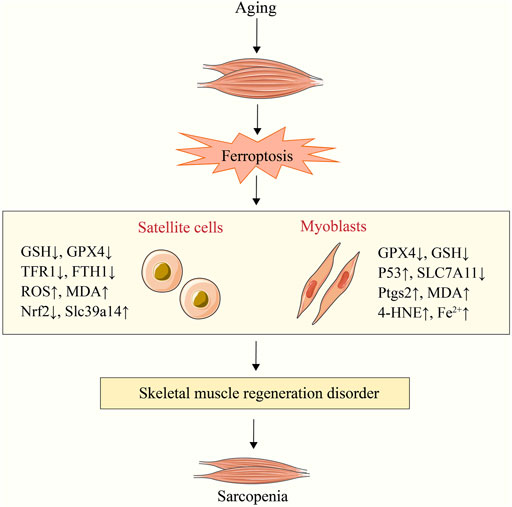
FIGURE 3. Sarcopenia caused by satellite cell and myoblast ferroptosis. Aging can result in satellite cell and myoblast ferroptosis. Sarcopenia is caused by the subsequent dysfunction of skeletal muscle regeneration. NADPH, nicotinamide adenine dinucleotide phosphate; Ptgs2, prostaglandin-endoperoxide synthase 2; 4-HNE, 4-hydroxynonenal; Slc39a14, zinc transporter Zip14; MDA, malondialdehyde; SLC7A11, solute carrier family 7 member 11; TFR1, transferrin receptor 1; GPX4, glutathione peroxidase 4; GSH, glutathione.
3.2 Ferroptosis and rhabdomyolysis
RML is an acute clinical syndrome caused by the injury of skeletal muscle cells and the release of intracellular components (such as myoglobin and creatine kinase) into the systemic circulation (Lindner and Zierz, 2003; Stahl et al., 2020). It is exhibited in study that ferroptosis of skeletal muscle cells induced by the inhibition of the antioxidant axis Nrf2-XCT/GPX4 is one of the potential mechanisms of atorvastatin-induced muscle-related symptoms (muscle weakness, pain, cramps, and RML) (Zhang Q. et al., 2022). It suggests that ferroptosis may be related to the disease progression of RML. AKI is a common complication of RML and one of the leading causes of death in RML patients (Ahmad et al., 2021). A study reported that iron deficiency could exacerbate RML-induced AKI by evoking lipid peroxidation through catalytic heme-iron (Zhao et al., 2021b). Another study found that the related characteristics of ferroptosis include decreased GSH levels and the accumulation of lipid peroxidation products MDA, 4-HNE and iron occurred in RML mice (Guerrero-Hue et al., 2019). Simultaneously, fer-1 has a significant improvement effect on muscle cell death, renal function and structure of RML mice compared to zVAD (an apoptosis inhibitor) and RIPK3-knockout mice (necroptosis pathway deficiency) (Guerrero-Hue et al., 2019). These results suggest that ferroptosis is a paramount pathogenic factor in RML and its associated renal injury. Furthermore, curcumin, a powerful antioxidant, has been reported to improve the AKI associated with RML by increasing the ferroptosis resistance of cells (Guerrero-Hue et al., 2019), which opens a new way for the treatment of RML syndrome.
RML is also one of the major types of exertional heat stroke (EHS)-related muscle damage (Epstein and Yanovich, 2019; Laitano et al., 2021). The experimental results revealed that high levels of iron content, ferroptosis markers (Ptgs2, MDA), and typical ferroptotic mitochondrial morphological changes would happen in the muscle tissue of RML mice after EHS (He et al., 2022). Through further study in vivo and in vitro, it is found that post-EHS-mediated ferroptosis of skeletal muscle cells depended on the up-regulation of ACSL4, the key gene for lipid modeling, and rosiglitazone (ACSL4 inhibitor) treatment could significantly reduce the skeletal muscle injury caused by EHS (He et al., 2022). Inhibition of ferroptosis by targeting ACSL4, therefore, may be a novel approach to prevent RML after EHS.
3.3 Ferroptosis and rhabdomyosarcoma
Characterized by poor survival and high recurrence, RMS is a malignant soft tissue tumor that occurs mostly in children and adolescents (Kramer et al., 1983; Ognjanovic et al., 2009; Dantonello et al., 2013; Egas-Bejar and Huh, 2014). Recent study has reported that RMS is sensitive to oxidative stress (Chen et al., 2013). Ferroptosis, as a novel mode of cell death induced by oxidative stress (Wu et al., 2018), has received attention in current RMS research. Research shows that erastin and RSL3, as common ferroptosis-inducing compounds, can cause ferroptosis of RMS cells through GSH consumption and GPX4 inactivation respectively (Codenotti et al., 2018; Dachert et al., 2020). Notably, inhibition of protein kinase C (PKC) isoform PKCα and its downstream target gene NADPH oxidases (NOX) isoforms (NOX1/4) can significantly protect RMS cells from erastin-induced ferroptosis, providing a new perspective for the treatment of RMS (Dachert et al., 2020). Activation of RAS-related signaling pathways is a key cause of RMS occurrence and recurrence (Zhang et al., 2013; Shern et al., 2014). It was found that ectopic expression of oncogenic RAS mutants (NRAS12V, KRAS12V and HRAS12V) significantly reduced the sensitivity of RMS13 cell line to erastin and RSL3 (Schott et al., 2015). This suggests that one of the pathways by which RAS drives the occurrence and progression of RMS is to confer ferroptosis resistance to RMS cells. In addition, a recent work found that tris (5-chloro-8-quinolinololato) gallium (III) complex had an active anti proliferation effect on RMS cells, and its efficacy decreased under the co incubation of fer-1 (Hreusova et al., 2022). Meanwhile, cells treated with tris (5-chloro-8-quinolinololato) gallium (III) complex presented typical characteristics of ferroptosis, such as down-regulation of GPX4 expression and accumulation of lipid peroxide (Hreusova et al., 2022). These results suggest that ferroptosis may be a potential mechanism for this compound to exert RMS therapeutic effect. In general, targeting RMS cell ferroptosis is a promising researching direction in clinical treatment. However, at present, the research on ferroptosis and RMS is mainly conducted in vitro, and further intervention on ferroptosis in vivo is needed to fully investigate the relationship between ferroptosis and RMS (Figure 4).
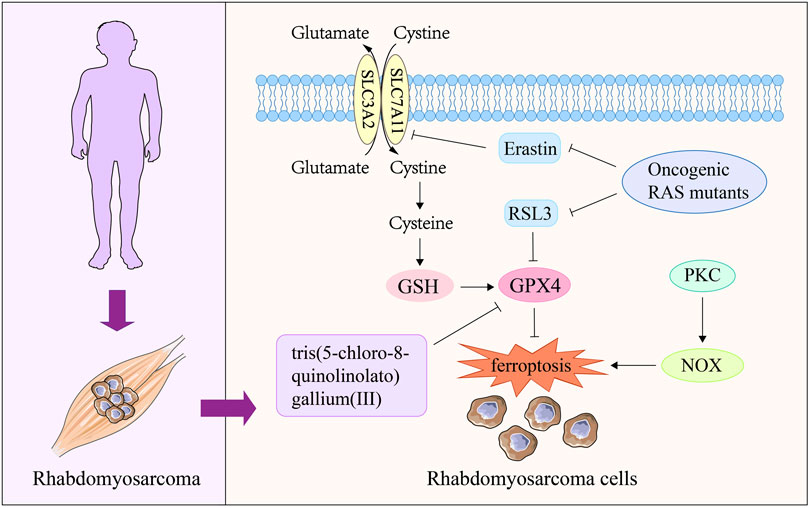
FIGURE 4. The role of ferroptosis in rhabdomyosarcoma. Oncogenic RAS mutants are the key mediator of RMS disease, which can block the ferroptosis of RMS cells induced by erastin and RSL3. The complex tris(5-chloro-8-quinolinolato) gallium (III) can induce ferroptosis in RMS cells by reducing GPX4 expression, thereby exerting therapeutic effects on RMS. The activation of PKC-NOX pathway can participate in the process of RMS disease by increasing the ferroptosis resistance of RMS cells. PKC, protein kinase C; NOX, NADPH oxidases; GPX4, glutathione peroxidase 4; GSH, glutathione.
3.4 Ferroptosis and exhaustive exercise-induced fatigue
EEIF refers to the inability of muscles to generate force due to prolonged and/or strenuous exercise, which not only reduces quality of life, but also promotes the development of fatigue itself, and even causes organic changes (Dalsgaard et al., 2004; da Rocha et al., 2018; Hou et al., 2020). Under physiological conditions, skeletal muscle fibers continuously produce ROS at a slow rate and increase during muscle contraction, which will be offset by the antioxidant system to maintain the balance of its production and removal (Reid, 2008). However, it is found in many research that long and/or strenuous exercise will cause a sharp ROS increase in skeletal muscle (Davies et al., 1982; Reid et al., 1992). Meanwhile, this activity dependent increase of ROS will induce lipid peroxidation of cell membrane and damage the healthy tissue, thus aggravating muscle fatigue during strenuous exercise (Dillard et al., 1978; Alessio et al., 1988). Therefore, ferroptosis may be a new type of cell death that participates in the physiological and pathological process of EEIF except apoptosis (Sun Y. et al., 2016; Liu S. et al., 2022). Excitingly, a recent report explored the relationship between ferroptosis and EEIF (Xiao et al., 2022). The experimental data showed that there were characteristics related to ferroptosis in skeletal muscle of EEIF mice, such as accumulation of iron, lipid peroxide, decreased expression of GPX4 and GSH, suggesting that ferroptosis may participate in the process of EEIF disease (Xiao et al., 2022). Trilobatin, as a natural food additive, has been reported to be anti-fatigue role by reducing the production of ROS and MDA, increasing the activity of GPX4 and the level of GSH, which is related to the inhibition of ferroptosis by the activation of Nrf2/ARE signaling pathway (Xiao et al., 2022). Based on the above, the high-level ROS produced in skeletal muscle after prolonged and/or strenuous exercise may increase the loss of muscle cells by activating ferroptosis, thereby promoting EEIF. Although there is limited evidence to support this view, it provides a new direction for the treatment of EEIF, which is worthy of more in-depth exploration from researchers in related fields.
3.5 Ferroptosis and idiopathic inflammatory myopathies
IIMs are a group of autoimmune diseases characterized by muscle inflammation (Parkes et al., 2015; Lundberg et al., 2016). Polymyositis (PM) and dermatomyositis (DM) are the two most common types of IIMs with high mortality (Yang et al., 2020). However, the role of ferroptosis in IIMs remains unclear. Vitamin E and selenium are important antioxidants that prevent lipid peroxidation with the effect of resisting ferroptosis (Conrad and Proneth, 2020; Hu et al., 2021; Tuo et al., 2021). It was found that a patient with chronic malabsorption and selective IgA deficiency lacking vitamin E and selenium appeared PM when receiving iron glucan treatment, which was related to lipid peroxidation caused by free iron activated free radicals (Foulkes et al., 1991). Ferritin is the main site of iron storage in the body, and elevated levels indicate iron accumulation in the body (Cook et al., 1974; Harrison, 1977; Worwood, 1987). Many population surveys based on PM/DM have found that the severity and prognosis of PM/DM and its complications (interstitial lung disease) were related to ferritinemia (Gono et al., 2010; Kawasumi et al., 2014; Ishizuka et al., 2016). In addition, mitochondrial dysfunction, as a landmark event of termination of ferroptosis and the main cause of ROS accumulation, has been reported as an important pathogenic mechanism of IIMs (Meyer et al., 2017; Boehler et al., 2019). Based on this, it is logical to speculate that ferroptosis is involved in the occurrence and development of IIM, and further exploration is needed to clarify the exact role ferroptosis plays in IIM (Table 2).
4 Conclusion and prospect
This review summarizes the regulatory mechanism of ferroptosis and its role in the progression of different skeletal muscle diseases. As mentioned above, in addition to the three classical regulatory pathways for ferroptosis in lipid, iron, and amino acid metabolism, a number of signal regulators and autophagy-related genes, such as Nrf2, p53, NCOA4, FANCD2, and CISD1, are also essential ferroptosis players. With the deepening of research, ferroptosis has been proved to be overriding in some muscle mass and dysfunction diseases, including sarcopenia, RML and EEIF. Skeletal muscle, composed of skeletal muscle cells, is an important organ to maintain human posture, exercise, energy metabolism and secretion of muscle cytokines. Therefore, it is of great significance to inhibit the ferroptosis of skeletal muscle cells in the treatment of sarcopenia, RML, EEIF and other diseases. However, in terms of RMS and other malignant tumor tissues, the key to prevent cancer occurrence and recurrence is to actively promote the ferroptosis of cancer cells. It is concluded that unstable iron accumulation, increase of lipid peroxide, inactivation of GPX4, inhibition of system Xc− and depletion of GSH are common causes of ferroptosis in skeletal muscle diseases. The regulatory mechanisms and targets involved include P53/SLC7A11 axis, Nrf2-xCT/GPX4 axis, TFR1, ACSL4, PKCα and NOX1/4. These ferroptosis related targets were found to be an important way in distinct skeletal muscle disease treatment drugs to inhibit ferroptosis (Trilobatin and Rosiglitazone) or induce ferroptosis [tris (5-chloro-8-quinolinolato) gallium (III) and atorvastatin]. Their effects were similar to those of known ferroptosis interventions such as erastin, RSL3, and fer-1. However, it is not clear whether these regulatory factors are specific targets of therapeutic drugs for skeletal muscle diseases.
Due to the limitation of current literature, our review of ferroptosis and skeletal muscle disease is not very comprehensive. However, there are still some exploratory suggestions worth putting forward. Ferroptosis is a regulatory mechanism that is promising for the treatment of sarcopenia, RML, RMS and EEIF. Whereas the exploration of the correlation between these diseases and ferroptosis is still in its infancy. Meanwhile, ferroptosis may also be a breakthrough for us to overcome the problems in treatment skeletal muscle-related intractable diseases such as IIMs. Of note, in the treatment of RMS and other skeletal muscle diseases, it is necessary to properly promote and inhibit ferroptosis to intervene the disease process. Therefore, when treating cancer and skeletal muscle related diseases with ferroptosis as a target, we should pay attention to balancing the two-way effects of ferroptosis treatment drugs on cancer tissues and healthy tissues. This is especially critical for the treatment of cancer patients with skeletal muscle disease, and it is also the focus and difficulty in drug development. Moreover, several genes associated with heme metabolism, such as ABCB6, FLVCR1, and ABCG2, appear to significantly influence ferroptosis, providing profound reference for the study on ferroptosis regulatory targets in skeletal muscle diseases, which needs more exploration and excavation.
Author contributions
YW and ZZ conceived the framework of the review and wrote the manuscript. WJ, YW, XW, YZ, XF, and LT added and checked references. XL and JM reviewed and improved the manuscript. All authors contributed to the article and approved the submitted version.
Funding
This work was supported by the National Natural Science Foundation of China (No: 82205039), the Innovation Team and Talents Cultivation Program of National Administration of Traditional Chinese Medicine (No: ZYYCXTD-D-202001), the Jilin Scientific and Technological Development Program (20200201305JC, 20190101010JH) and the Education Department of Jilin Province (JJKH20200882KJ).
Conflict of interest
The authors declare that the research was conducted in the absence of any commercial or financial relationships that could be construed as a potential conflict of interest.
Publisher’s note
All claims expressed in this article are solely those of the authors and do not necessarily represent those of their affiliated organizations, or those of the publisher, the editors and the reviewers. Any product that may be evaluated in this article, or claim that may be made by its manufacturer, is not guaranteed or endorsed by the publisher.
References
Adedoyin, O., Boddu, R., Traylor, A., Lever, J. M., Bolisetty, S., George, J. F., et al. (2018). Heme oxygenase-1 mitigates ferroptosis in renal proximal tubule cells. Am. J. Physiol. Ren. Physiol. 314 (5), F702–F714. doi:10.1152/ajprenal.00044.2017
Ahmad, S., Anees, M., Elahi, I., and Fazal, E. M. (2021). Rhabdomyolysis leading to acute kidney injury. J. Coll. Physicians Surg. Pak. 31 (2), 235–237. doi:10.29271/jcpsp.2021.02.235
Alessio, H. M., Goldfarb, A. H., and Cutler, R. G. (1988). MDA content increases in fast- and slow-twitch skeletal muscle with intensity of exercise in a rat. Am. J. Physiol. 255 (1), C874–C877. doi:10.1152/ajpcell.1988.255.6.C874
An, J. R., Su, J. N., Sun, G. Y., Wang, Q. F., Fan, Y. D., Jiang, N., et al. (2022). Liraglutide alleviates cognitive deficit in db/db mice: Involvement in oxidative stress, iron overload, and ferroptosis. Neurochem. Res. 47 (2), 279–294. doi:10.1007/s11064-021-03442-7
Anandhan, A., Dodson, M., Schmidlin, C. J., Liu, P., and Zhang, D. D. (2020). Breakdown of an ironclad defense system: The critical role of NRF2 in mediating ferroptosis. Cell Chem. Biol. 27 (4), 436–447. doi:10.1016/j.chembiol.2020.03.011
Andrews, N. C. (2000). Iron homeostasis: Insights from genetics and animal models. Nat. Rev. Genet. 1 (3), 208–217. doi:10.1038/35042073
Argiles, J. M., Campos, N., Lopez-Pedrosa, J. M., Rueda, R., and Rodriguez-Manas, L. (2016). Skeletal muscle regulates metabolism via interorgan crosstalk: Roles in health and disease. J. Am. Med. Dir. Assoc. 17 (9), 789–796. doi:10.1016/j.jamda.2016.04.019
Ayala, A., Munoz, M. F., and Arguelles, S. (2014). Lipid peroxidation: Production, metabolism, and signaling mechanisms of malondialdehyde and 4-hydroxy-2-nonenal. Oxid. Med. Cell. Longev. 2014, 360438. doi:10.1155/2014/360438
Aziz, A., Sebastian, S., and Dilworth, F. J. (2012). The origin and fate of muscle satellite cells. Stem Cell Rev. Rep. 8 (2), 609–622. doi:10.1007/s12015-012-9352-0
Aziz, N., and Munro, H. N. (1987). Iron regulates ferritin mRNA translation through a segment of its 5' untranslated region. Proc. Natl. Acad. Sci. U. S. A. 84 (23), 8478–8482. doi:10.1073/pnas.84.23.8478
Badgley, M. A., Kremer, D. M., Maurer, H. C., DelGiorno, K. E., Lee, H. J., Purohit, V., et al. (2020). Cysteine depletion induces pancreatic tumor ferroptosis in mice. Science 368 (6486), 85–89. doi:10.1126/science.aaw9872
Bao, C., Liu, C., Liu, Q., Hua, L., Hu, J., Li, Z., et al. (2022). Liproxstatin-1 alleviates LPS/IL-13-induced bronchial epithelial cell injury and neutrophilic asthma in mice by inhibiting ferroptosis. Int. Immunopharmacol. 109, 108770. doi:10.1016/j.intimp.2022.108770
Bao, W. D., Pang, P., Zhou, X. T., Hu, F., Xiong, W., Chen, K., et al. (2021). Loss of ferroportin induces memory impairment by promoting ferroptosis in Alzheimer's disease. Cell Death Differ. 28 (5), 1548–1562. doi:10.1038/s41418-020-00685-9
Bayir, H. (2005). Reactive oxygen species. Crit. Care Med. 33 (12), S498–S501. doi:10.1097/01.ccm.0000186787.64500.12
Bersuker, K., Hendricks, J. M., Li, Z., Magtanong, L., Ford, B., Tang, P. H., et al. (2019). The CoQ oxidoreductase FSP1 acts parallel to GPX4 to inhibit ferroptosis. Nature 575 (7784), 688–692. doi:10.1038/s41586-019-1705-2
Boehler, J. F., Horn, A., Novak, J. S., Li, N., Ghimbovschi, S., Lundberg, I. E., et al. (2019). Mitochondrial dysfunction and role of harakiri in the pathogenesis of myositis. J. Pathol. 249 (2), 215–226. doi:10.1002/path.5309
Brack, A. S., Bildsoe, H., and Hughes, S. M. (2005). Evidence that satellite cell decrement contributes to preferential decline in nuclear number from large fibres during murine age-related muscle atrophy. J. Cell Sci. 118 (20), 4813–4821. doi:10.1242/jcs.02602
Bridges, R. J., Natale, N. R., and Patel, S. A. (2012). System xc(-) cystine/glutamate antiporter: An update on molecular pharmacology and roles within the CNS. Br. J. Pharmacol. 165 (1), 20–34. doi:10.1111/j.1476-5381.2011.01480.x
Budai, Z., Balogh, L., and Sarang, Z. (2018). Altered gene expression of muscle satellite cells contributes to agerelated sarcopenia in mice. Curr. Aging Sci. 11 (3), 165–172. doi:10.2174/1874609811666180925104241
Campbell, M. R., Karaca, M., Adamski, K. N., Chorley, B. N., Wang, X., and Bell, D. A. (2013). Novel hematopoietic target genes in the NRF2-mediated transcriptional pathway. Oxid. Med. Cell. Longev. 2013, 120305. doi:10.1155/2013/120305
Catala, A., and Diaz, M. (2016). Editorial: Impact of lipid peroxidation on the physiology and pathophysiology of cell membranes. Front. Physiol. 7, 423. doi:10.3389/fphys.2016.00423
Chan, J. Y., and Kwong, M. (2000). Impaired expression of glutathione synthetic enzyme genes in mice with targeted deletion of the Nrf2 basic-leucine zipper protein. Biochim. Biophys. Acta 1517 (1), 19–26. doi:10.1016/s0167-4781(00)00238-4
Chen, F., Zhou, J., Li, Y., Zhao, Y., Yuan, J., Cao, Y., et al. (2019a). YY1 regulates skeletal muscle regeneration through controlling metabolic reprogramming of satellite cells. EMBO J. 38 (10), e99727. doi:10.15252/embj.201899727
Chen, H., Cao, L., Han, K., Zhang, H., Cui, J., Ma, X., et al. (2022b). Patulin disrupts SLC7A11-cystine-cysteine-GSH antioxidant system and promotes renal cell ferroptosis both in vitro and in vivo. Food Chem. Toxicol. 166, 113255. doi:10.1016/j.fct.2022.113255
Chen, H., Zheng, C., Zhang, Y., Chang, Y. Z., Qian, Z. M., and Shen, X. (2006). Heat shock protein 27 downregulates the transferrin receptor 1-mediated iron uptake. Int. J. Biochem. Cell Biol. 38 (8), 1402–1416. doi:10.1016/j.biocel.2006.02.006
Chen, M. S., Wang, S. F., Hsu, C. Y., Yin, P. H., Yeh, T. S., Lee, H. C., et al. (2017). CHAC1 degradation of glutathione enhances cystine-starvation-induced necroptosis and ferroptosis in human triple negative breast cancer cells via the GCN2-eIF2α-ATF4 pathway. Oncotarget 8 (70), 114588–114602. doi:10.18632/oncotarget.23055
Chen, X., Stewart, E., Shelat, A. A., Qu, C., Bahrami, A., Hatley, M., et al. (2013). Targeting oxidative stress in embryonal rhabdomyosarcoma. Cancer Cell 24 (6), 710–724. doi:10.1016/j.ccr.2013.11.002
Chen, X., Xu, S., Zhao, C., and Liu, B. (2019b). Role of TLR4/NADPH oxidase 4 pathway in promoting cell death through autophagy and ferroptosis during heart failure. Biochem. Biophys. Res. Commun. 516 (1), 37–43. doi:10.1016/j.bbrc.2019.06.015
Chen, Y., Wang, J., Li, J., Zhu, J., Wang, R., Xi, Q., et al. (2021b). Astragalus polysaccharide prevents ferroptosis in a murine model of experimental colitis and human Caco-2 cells via inhibiting NRF2/HO-1 pathway. Eur. J. Pharmacol. 911, 174518. doi:10.1016/j.ejphar.2021.174518
Chepikova, O. E., Malin, D., Strekalova, E., Lukasheva, E. V., Zamyatnin, A. A., and Cryns, V. L. (2020). Lysine oxidase exposes a dependency on the thioredoxin antioxidant pathway in triple-negative breast cancer cells. Breast Cancer Res. Treat. 183 (3), 549–564. doi:10.1007/s10549-020-05801-4
Chu, B., Kon, N., Chen, D., Li, T., Liu, T., Jiang, L., et al. (2019). ALOX12 is required for p53-mediated tumour suppression through a distinct ferroptosis pathway. Nat. Cell Biol. 21 (5), 579–591. doi:10.1038/s41556-019-0305-6
Chung, J., Chen, C., and Paw, B. H. (2012). Heme metabolism and erythropoiesis. Curr. Opin. Hematol. 19 (3), 156–162. doi:10.1097/MOH.0b013e328351c48b
Codenotti, S., Poli, M., Asperti, M., Zizioli, D., Marampon, F., and Fanzani, A. (2018). Cell growth potential drives ferroptosis susceptibility in rhabdomyosarcoma and myoblast cell lines. J. Cancer Res. Clin. Oncol. 144 (9), 1717–1730. doi:10.1007/s00432-018-2699-0
Conrad, M., and Proneth, B. (2020). Selenium: Tracing another essential element of ferroptotic cell death. Cell Chem. Biol. 27 (4), 409–419. doi:10.1016/j.chembiol.2020.03.012
Cook, J. D., Lipschitz, D. A., Miles, L. E., and Finch, C. A. (1974). Serum ferritin as a measure of iron stores in normal subjects. Am. J. Clin. Nutr. 27 (7), 681–687. doi:10.1093/ajcn/27.7.681
Corsi, B., Cozzi, A., Arosio, P., Drysdale, J., Santambrogio, P., Campanella, A., et al. (2002). Human mitochondrial ferritin expressed in HeLa cells incorporates iron and affects cellular iron metabolism. J. Biol. Chem. 277 (25), 22430–22437. doi:10.1074/jbc.M105372200
Cruz-Jentoft, A. J., Baeyens, J. P., Bauer, J. M., Boirie, Y., Cederholm, T., Landi, F., et al. (2010). Sarcopenia: European consensus on definition and diagnosis: Report of the European working group on sarcopenia in older people. Age Ageing 39 (4), 412–423. doi:10.1093/ageing/afq034
da Rocha, A. L., Teixeira, G. R., Pinto, A. P., de Morais, G. P., Oliveira, L. D. C., de Vicente, L. G., et al. (2018). Excessive training induces molecular signs of pathologic cardiac hypertrophy. J. Cell. Physiol. 233 (11), 8850–8861. doi:10.1002/jcp.26799
Dachert, J., Ehrenfeld, V., Habermann, K., Dolgikh, N., and Fulda, S. (2020). Targeting ferroptosis in rhabdomyosarcoma cells. Int. J. Cancer 146 (2), 510–520. doi:10.1002/ijc.32496
Dalsgaard, M. K., Ott, P., Dela, F., Juul, A., Pedersen, B. K., Warberg, J., et al. (2004). The CSF and arterial to internal jugular venous hormonal differences during exercise in humans. Exp. Physiol. 89 (3), 271–277. doi:10.1113/expphysiol.2003.026922
Dantonello, T. M., Int-Veen, C., Schuck, A., Seitz, G., Leuschner, I., Nathrath, M., et al. (2013). Survival following disease recurrence of primary localized alveolar rhabdomyosarcoma. Pediatr. Blood Cancer 60 (8), 1267–1273. doi:10.1002/pbc.24488
Davies, K. J., Quintanilha, A. T., Brooks, G. A., and Packer, L. (1982). Free radicals and tissue damage produced by exercise. Biochem. Biophys. Res. Commun. 107 (4), 1198–1205. doi:10.1016/s0006-291x(82)80124-1
Day, K., Shefer, G., Shearer, A., and Yablonka-Reuveni, Z. (2010). The depletion of skeletal muscle satellite cells with age is concomitant with reduced capacity of single progenitors to produce reserve progeny. Dev. Biol. 340 (2), 330–343. doi:10.1016/j.ydbio.2010.01.006
Deponte, M. (2013). Glutathione catalysis and the reaction mechanisms of glutathione-dependent enzymes. Biochim. Biophys. Acta 1830 (5), 3217–3266. doi:10.1016/j.bbagen.2012.09.018
DeRuisseau, K. C., Park, Y. M., DeRuisseau, L. R., Cowley, P. M., Fazen, C. H., and Doyle, R. P. (2013). Aging-related changes in the iron status of skeletal muscle. Exp. Gerontol. 48 (11), 1294–1302. doi:10.1016/j.exger.2013.08.011
Desuzinges-Mandon, E., Arnaud, O., Martinez, L., Huche, F., Di Pietro, A., and Falson, P. (2010). ABCG2 transports and transfers heme to albumin through its large extracellular loop. J. Biol. Chem. 285 (43), 33123–33133. doi:10.1074/jbc.M110.139170
Dillard, C. J., Litov, R. E., Savin, W. M., Dumelin, E. E., and Tappel, A. L. (1978). Effects of exercise, vitamin E, and ozone on pulmonary function and lipid peroxidation. J. Appl. Physiol. Respir. Environ. Exerc. Physiol. 45 (6), 927–932. doi:10.1152/jappl.1978.45.6.927
Ding, H., Chen, S., Pan, X., Dai, X., Pan, G., Li, Z., et al. (2021). Transferrin receptor 1 ablation in satellite cells impedes skeletal muscle regeneration through activation of ferroptosis. J. Cachexia Sarcopenia Muscle 12 (3), 746–768. doi:10.1002/jcsm.12700
Dixon, S. J., Lemberg, K. M., Lamprecht, M. R., Skouta, R., Zaitsev, E. M., Gleason, C. E., et al. (2012). Ferroptosis: An iron-dependent form of nonapoptotic cell death. Cell 149 (5), 1060–1072. doi:10.1016/j.cell.2012.03.042
Dixon, S. J., Winter, G. E., Musavi, L. S., Lee, E. D., Snijder, B., Rebsamen, M., et al. (2015). Human haploid cell genetics reveals roles for lipid metabolism genes in nonapoptotic cell death. ACS Chem. Biol. 10 (7), 1604–1609. doi:10.1021/acschembio.5b00245
Dlouhy, A. C., and Outten, C. E. (2013). The iron metallome in eukaryotic organisms. Mater. Ions Life Sci. 12, 241–278. doi:10.1007/978-94-007-5561-1_8
Do Van, B., Gouel, F., Jonneaux, A., Timmerman, K., Gele, P., Petrault, M., et al. (2016). Ferroptosis, a newly characterized form of cell death in Parkinson's disease that is regulated by PKC. Neurobiol. Dis. 94, 169–178. doi:10.1016/j.nbd.2016.05.011
Dodson, M., Castro-Portuguez, R., and Zhang, D. D. (2019). NRF2 plays a critical role in mitigating lipid peroxidation and ferroptosis. Redox Biol. 23, 101107. doi:10.1016/j.redox.2019.101107
Doll, S., Freitas, F. P., Shah, R., Aldrovandi, M., da Silva, M. C., Ingold, I., et al. (2019). FSP1 is a glutathione-independent ferroptosis suppressor. Nature 575 (7784), 693–698. doi:10.1038/s41586-019-1707-0
Doll, S., Proneth, B., Tyurina, Y. Y., Panzilius, E., Kobayashi, S., Ingold, I., et al. (2017). ACSL4 dictates ferroptosis sensitivity by shaping cellular lipid composition. Nat. Chem. Biol. 13 (1), 91–98. doi:10.1038/nchembio.2239
Dong, H., Qiang, Z., Chai, D., Peng, J., Xia, Y., Hu, R., et al. (2020). Nrf2 inhibits ferroptosis and protects against acute lung injury due to intestinal ischemia reperfusion via regulating SLC7A11 and HO-1. Aging (Albany NY) 12 (13), 12943–12959. doi:10.18632/aging.103378
Drysdale, J., Arosio, P., Invernizzi, R., Cazzola, M., Volz, A., Corsi, B., et al. (2002). Mitochondrial ferritin: A new player in iron metabolism. Blood Cells Mol. Dis. 29 (3), 376–383. doi:10.1006/bcmd.2002.0577
Egas-Bejar, D., and Huh, W. W. (2014). Rhabdomyosarcoma in adolescent and young adult patients: Current perspectives. Adolesc. Health Med. Ther. 5, 115–125. doi:10.2147/AHMT.S44582
Ellingsgaard, H., Hauselmann, I., Schuler, B., Habib, A. M., Baggio, L. L., Meier, D. T., et al. (2011). Interleukin-6 enhances insulin secretion by increasing glucagon-like peptide-1 secretion from L cells and alpha cells. Nat. Med. 17 (11), 1481–1489. doi:10.1038/nm.2513
Epstein, Y., and Yanovich, R. (2019). N. Engl. J. Med. 380 (25), 2449–2459. doi:10.1056/NEJMra1810762
Eriksson, S., Prigge, J. R., Talago, E. A., Arner, E. S., and Schmidt, E. E. (2015). Dietary methionine can sustain cytosolic redox homeostasis in the mouse liver. Nat. Commun. 6, 6479. doi:10.1038/ncomms7479
Fang, X., Wang, H., Han, D., Xie, E., Yang, X., Wei, J., et al. (2019). Ferroptosis as a target for protection against cardiomyopathy. Proc. Natl. Acad. Sci. U. S. A. 116 (7), 2672–2680. doi:10.1073/pnas.1821022116
Fang, Y., Chen, X., Tan, Q., Zhou, H., Xu, J., and Gu, Q. (2021). Inhibiting ferroptosis through disrupting the NCOA4-FTH1 interaction: A new mechanism of action. ACS Cent. Sci. 7 (6), 980–989. doi:10.1021/acscentsci.0c01592
Farmer, E. E., and Mueller, M. J. (2013). ROS-mediated lipid peroxidation and RES-activated signaling. Annu. Rev. Plant Biol. 64, 429–450. doi:10.1146/annurev-arplant-050312-120132
Feng, H., and Stockwell, B. R. (2018). Unsolved mysteries: How does lipid peroxidation cause ferroptosis? PLoS Biol. 16 (5), e2006203. doi:10.1371/journal.pbio.2006203
Feng, L., Zhao, K., Sun, L., Yin, X., Zhang, J., Liu, C., et al. (2021). SLC7A11 regulated by NRF2 modulates esophageal squamous cell carcinoma radiosensitivity by inhibiting ferroptosis. J. Transl. Med. 19 (1), 367. doi:10.1186/s12967-021-03042-7
Ferrannini, E., Simonson, D. C., Katz, L. D., Reichard, G., Bevilacqua, S., Barrett, E. J., et al. (1988). The disposal of an oral glucose load in patients with non-insulin-dependent diabetes. Metabolism. 37 (1), 79–85. doi:10.1016/0026-0495(88)90033-9
Ferreira, C. A., Ni, D., Rosenkrans, Z. T., and Cai, W. (2018). Scavenging of reactive oxygen and nitrogen species with nanomaterials. Nano Res. 11 (10), 4955–4984. doi:10.1007/s12274-018-2092-y
Fleming, M. D., Romano, M. A., Su, M. A., Garrick, L. M., Garrick, M. D., and Andrews, N. C. (1998). Nramp2 is mutated in the anemic belgrade (b) rat: Evidence of a role for Nramp2 in endosomal iron transport. Proc. Natl. Acad. Sci. U. S. A. 95 (3), 1148–1153. doi:10.1073/pnas.95.3.1148
Flohe, L., Gunzler, W. A., and Schock, H. H. (1973). Glutathione peroxidase: A selenoenzyme. FEBS Lett. 32 (1), 132–134. doi:10.1016/0014-5793(73)80755-0
Fluck, M., and Hoppeler, H. (2003). Molecular basis of skeletal muscle plasticity--from gene to form and function. Rev. Physiol. Biochem. Pharmacol. 146, 159–216. doi:10.1007/s10254-002-0004-7
Foulkes, W. D., Sewry, C., Calam, J., and Hodgson, H. J. (1991). Rhabdomyolysis after intramuscular iron-dextran in malabsorption. Ann. Rheum. Dis. 50 (3), 184–186. doi:10.1136/ard.50.3.184
Friedmann Angeli, J. P., and Conrad, M. (2018). Selenium and GPX4, a vital symbiosis. Free Radic. Biol. Med. 127, 153–159. doi:10.1016/j.freeradbiomed.2018.03.001
Furukawa, M., and Xiong, Y. (2005). BTB protein Keap1 targets antioxidant transcription factor Nrf2 for ubiquitination by the Cullin 3-Roc1 ligase. Mol. Cell. Biol. 25 (1), 162–171. doi:10.1128/MCB.25.1.162-171.2005
Gao, J., Li, Y., and Song, R. (2021). SIRT2 inhibition exacerbates p53-mediated ferroptosis in mice following experimental traumatic brain injury. Neuroreport 32 (12), 1001–1008. doi:10.1097/WNR.0000000000001679
Gao, M., Monian, P., Pan, Q., Zhang, W., Xiang, J., and Jiang, X. (2016). Ferroptosis is an autophagic cell death process. Cell Res. 26 (9), 1021–1032. doi:10.1038/cr.2016.95
Gao, M., Monian, P., Quadri, N., Ramasamy, R., and Jiang, X. (2015). Glutaminolysis and transferrin regulate ferroptosis. Mol. Cell 59 (2), 298–308. doi:10.1016/j.molcel.2015.06.011
Garber, A. J., Karl, I. E., and Kipnis, D. M. (1976). Alanine and glutamine synthesis and release from skeletal muscle. I. Glycolysis and amino acid release. J. Biol. Chem. 251 (3), 826–835. doi:10.1016/s0021-9258(17)33859-0
Gono, T., Kawaguchi, Y., Hara, M., Masuda, I., Katsumata, Y., Shinozaki, M., et al. (2010). Increased ferritin predicts development and severity of acute interstitial lung disease as a complication of dermatomyositis. Rheumatol. Oxf. 49 (7), 1354–1360. doi:10.1093/rheumatology/keq073
Gryzik, M., Srivastava, A., Longhi, G., Bertuzzi, M., Gianoncelli, A., Carmona, F., et al. (2017). Expression and characterization of the ferritin binding domain of Nuclear Receptor Coactivator-4 (NCOA4). Biochim. Biophys. Acta. Gen. Subj. 1861, 2710–2716. doi:10.1016/j.bbagen.2017.07.015
Guan, Z., Chen, J., Li, X., and Dong, N. (2020). Tanshinone IIA induces ferroptosis in gastric cancer cells through p53-mediated SLC7A11 down-regulation. Biosci. Rep. 40 (8), BSR20201807. doi:10.1042/BSR20201807
Guerrero-Hue, M., Garcia-Caballero, C., Palomino-Antolin, A., Rubio-Navarro, A., Vazquez-Carballo, C., Herencia, C., et al. (2019). Curcumin reduces renal damage associated with rhabdomyolysis by decreasing ferroptosis-mediated cell death. FASEB J. 33 (8), 8961–8975. doi:10.1096/fj.201900077R
Guo, S., Chen, Y., Xue, X., Yang, Y., Wang, Y., Qiu, S., et al. (2021). TRIB2 desensitizes ferroptosis via βTrCP-mediated TFRC ubiquitiantion in liver cancer cells. Cell Death Discov. 7 (1), 196. doi:10.1038/s41420-021-00574-1
Han, S., Lin, F., Qi, Y., Liu, C., Zhou, L., Xia, Y., et al. (2022). HO-1 contributes to luteolin-triggered ferroptosis in clear cell renal cell carcinoma via increasing the labile iron pool and promoting lipid peroxidation. Oxid. Med. Cell. Longev. 2022, 3846217. doi:10.1155/2022/3846217
Harada, N., Kanayama, M., Maruyama, A., Yoshida, A., Tazumi, K., Hosoya, T., et al. (2011). Nrf2 regulates ferroportin 1-mediated iron efflux and counteracts lipopolysaccharide-induced ferroportin 1 mRNA suppression in macrophages. Arch. Biochem. Biophys. 508 (1), 101–109. doi:10.1016/j.abb.2011.02.001
Hatem, E., Berthonaud, V., Dardalhon, M., Lagniel, G., Baudouin-Cornu, P., Huang, M. E., et al. (2014). Glutathione is essential to preserve nuclear function and cell survival under oxidative stress. Free Radic. Biol. Med. 75 (1), S25–S26. doi:10.1016/j.freeradbiomed.2014.10.746
Hayano, M., Yang, W. S., Corn, C. K., Pagano, N. C., and Stockwell, B. R. (2016). Loss of cysteinyl-tRNA synthetase (CARS) induces the transsulfuration pathway and inhibits ferroptosis induced by cystine deprivation. Cell Death Differ. 23 (2), 270–278. doi:10.1038/cdd.2015.93
He, L., He, T., Farrar, S., Ji, L., Liu, T., and Ma, X. (2017). Antioxidants maintain cellular redox homeostasis by elimination of reactive oxygen species. Cell. Physiol. biochem. 44 (2), 532–553. doi:10.1159/000485089
He, S., Li, R., Peng, Y., Wang, Z., Huang, J., Meng, H., et al. (2022). ACSL4 contributes to ferroptosis-mediated rhabdomyolysis in exertional heat stroke. J. Cachexia Sarcopenia Muscle 13 (3), 1717–1730. doi:10.1002/jcsm.12953
He, Y. J., Liu, X. Y., Xing, L., Wan, X., Chang, X., and Jiang, H. L. (2020). Fenton reaction-independent ferroptosis therapy via glutathione and iron redox couple sequentially triggered lipid peroxide generator. Biomaterials 241, 119911. doi:10.1016/j.biomaterials.2020.119911
Henning, Y., Blind, U. S., Larafa, S., Matschke, J., and Fandrey, J. (2022). Hypoxia aggravates ferroptosis in RPE cells by promoting the Fenton reaction. Cell Death Dis. 13 (7), 662. doi:10.1038/s41419-022-05121-z
Hentze, M. W., Muckenthaler, M. U., Galy, B., and Camaschella, C. (2010). Two to tango: Regulation of mammalian iron metabolism. Cell 142 (1), 24–38. doi:10.1016/j.cell.2010.06.028
Hoffmann, C., and Weigert, C. (2017). Skeletal muscle as an endocrine organ: The role of myokines in exercise adaptations. Cold Spring Harb. Perspect. Med. 7 (11), a029793. doi:10.1101/cshperspect.a029793
Hojman, P., Dethlefsen, C., Brandt, C., Hansen, J., Pedersen, L., and Pedersen, B. K. (2011). Exercise-induced muscle-derived cytokines inhibit mammary cancer cell growth. Am. J. Physiol. Endocrinol. Metab. 301 (3), E504–E510. doi:10.1152/ajpendo.00520.2010
Holstein, S. A., and Hohl, R. J. (2004). Isoprenoids: Remarkable diversity of form and function. Lipids 39 (4), 293–309. doi:10.1007/s11745-004-1233-3
Hong, E. G., Ko, H. J., Cho, Y. R., Kim, H. J., Ma, Z., Yu, T. Y., et al. (2009). Interleukin-10 prevents diet-induced insulin resistance by attenuating macrophage and cytokine response in skeletal muscle. Diabetes 58 (11), 2525–2535. doi:10.2337/db08-1261
Hooda, J., Shah, A., and Zhang, L. (2014). Heme, an essential nutrient from dietary proteins, critically impacts diverse physiological and pathological processes. Nutrients 6 (3), 1080–1102. doi:10.3390/nu6031080
Hou, W., Xie, Y., Song, X., Sun, X., Lotze, M. T., Zeh, H. J., et al. (2016). Autophagy promotes ferroptosis by degradation of ferritin. Autophagy 12 (8), 1425–1428. doi:10.1080/15548627.2016.1187366
Hou, Y., Tang, Y., Wang, X., Ai, X., Wang, H., Li, X., et al. (2020). Rhodiola Crenulata ameliorates exhaustive exercise-induced fatigue in mice by suppressing mitophagy in skeletal muscle. Exp. Ther. Med. 20 (4), 3161–3173. doi:10.3892/etm.2020.9072
Hreusova, M., Novohradsky, V., Markova, L., Kostrhunova, H., Potocnak, I., Brabec, V., et al. (2022). Gallium(III) complex with cloxyquin ligands induces ferroptosis in cancer cells and is a potent agent against both differentiated and tumorigenic cancer stem rhabdomyosarcoma cells. Bioinorg. Chem. Appl. 2022, 3095749. doi:10.1155/2022/3095749
Hu, Q., Zhang, Y., Lou, H., Ou, Z., Liu, J., Duan, W., et al. (2021). GPX4 and vitamin E cooperatively protect hematopoietic stem and progenitor cells from lipid peroxidation and ferroptosis. Cell Death Dis. 12 (7), 706. doi:10.1038/s41419-021-04008-9
Hu, W., Zhang, C., Wu, R., Sun, Y., Levine, A., and Feng, Z. (2010). Glutaminase 2, a novel p53 target gene regulating energy metabolism and antioxidant function. Proc. Natl. Acad. Sci. U. S. A. 107 (16), 7455–7460. doi:10.1073/pnas.1001006107
Huang, Y., Wu, B., Shen, D., Chen, J., Yu, Z., and Chen, C. (2021). Ferroptosis in a sarcopenia model of senescence accelerated mouse prone 8 (SAMP8). Int. J. Biol. Sci. 17 (1), 151–162. doi:10.7150/ijbs.53126
Hubner, R. H., Schwartz, J. D., De Bishnu, P., Ferris, B., Omberg, L., Mezey, J. G., et al. (2009). Coordinate control of expression of Nrf2-modulated genes in the human small airway epithelium is highly responsive to cigarette smoking. Mol. Med. 15 (7-8), 203–219. doi:10.2119/molmed.2008.00130
Hyde, R., Hajduch, E., Powell, D. J., Taylor, P. M., and Hundal, H. S. (2005). Ceramide down-regulates System A amino acid transport and protein synthesis in rat skeletal muscle cells. FASEB J. 19 (3), 461–463. doi:10.1096/fj.04-2284fje
Ikeda, Y., Satoh, A., Horinouchi, Y., Hamano, H., Watanabe, H., Imao, M., et al. (2019). Iron accumulation causes impaired myogenesis correlated with MAPK signaling pathway inhibition by oxidative stress. FASEB J. 33 (8), 9551–9564. doi:10.1096/fj.201802724RR
Imai, H., and Nakagawa, Y. (2003). Biological significance of phospholipid hydroperoxide glutathione peroxidase (PHGPx, GPx4) in mammalian cells. Free Radic. Biol. Med. 34 (2), 145–169. doi:10.1016/s0891-5849(02)01197-8
Ingold, I., Berndt, C., Schmitt, S., Doll, S., Poschmann, G., Buday, K., et al. (2018). Selenium utilization by GPX4 is required to prevent hydroperoxide-induced ferroptosis. Cell 172 (3), 409–422. doi:10.1016/j.cell.2017.11.048
Ishizuka, M., Watanabe, R., Ishii, T., Machiyama, T., Akita, K., Fujita, Y., et al. (2016). Long-term follow-up of 124 patients with polymyositis and dermatomyositis: Statistical analysis of prognostic factors. Mod. Rheumatol. 26 (1), 115–120. doi:10.3109/14397595.2015.1054081
James, A. M., Smith, R. A., and Murphy, M. P. (2004). Antioxidant and prooxidant properties of mitochondrial Coenzyme Q. Arch. Biochem. Biophys. 423 (1), 47–56. doi:10.1016/j.abb.2003.12.025
Jang, Y. C., Sinha, M., Cerletti, M., Dall'Osso, C., and Wagers, A. J. (2011). Skeletal muscle stem cells: Effects of aging and metabolism on muscle regenerative function. Cold Spring Harb. Symp. Quant. Biol. 76, 101–111. doi:10.1101/sqb.2011.76.010652
Janssen, I., Heymsfield, S. B., Wang, Z. M., and Ross, R. (2000). Skeletal muscle mass and distribution in 468 men and women aged 18-88 yr. J. Appl. Physiol. 89 (1), 81–88. doi:10.1152/jappl.2000.89.1.81
Jazvinscak Jembrek, M., Vlainic, J., Radovanovic, V., Erhardt, J., and Orsolic, N. (2014). Effects of copper overload in P19 neurons: Impairment of glutathione redox homeostasis and crosstalk between caspase and calpain protease systems in ROS-induced apoptosis. Biometals 27 (6), 1303–1322. doi:10.1007/s10534-014-9792-x
Jiang, L., Kon, N., Li, T., Wang, S. J., Su, T., Hibshoosh, H., et al. (2015). Ferroptosis as a p53-mediated activity during tumour suppression. Nature 520 (7545), 57–62. doi:10.1038/nature14344
Jiang, T., Cheng, H., Su, J., Wang, X., Wang, Q., Chu, J., et al. (2020). Gastrodin protects against glutamate-induced ferroptosis in HT-22 cells through Nrf2/HO-1 signaling pathway. Toxicol. Vitro 62, 104715. doi:10.1016/j.tiv.2019.104715
Kanezaki, M., Terada, K., Tanabe, N., Shima, H., Hamakawa, Y., and Sato, S. (2021). Effects of sarcopenia on ventilatory behavior and the multidimensional nature of dyspnea in patients with chronic obstructive pulmonary disease. J. Am. Med. Dir. Assoc. 22 (4), 827–833. doi:10.1016/j.jamda.2021.01.081
Kawai, K., Hirayama, T., Imai, H., Murakami, T., Inden, M., Hozumi, I., et al. (2022). Molecular imaging of labile heme in living cells using a small molecule fluorescent probe. J. Am. Chem. Soc. 144 (9), 3793–3803. doi:10.1021/jacs.1c08485
Kawasaki, H., Takayama, J., Nagasaki, K., Yamaguchi, K., and Ohira, M. (1998). Hypercalcemia in children with rhabdomyosarcoma. J. Pediatr. Hematol. Oncol. 20 (4), 327–329. doi:10.1097/00043426-199807000-00009
Kawasumi, H., Gono, T., Kawaguchi, Y., Kaneko, H., Katsumata, Y., Hanaoka, M., et al. (2014). IL-6, IL-8, and IL-10 are associated with hyperferritinemia in rapidly progressive interstitial lung disease with polymyositis/dermatomyositis. Biomed. Res. Int. 2014, 815245. doi:10.1155/2014/815245
Keel, S. B., Doty, R. T., Yang, Z., Quigley, J. G., Chen, J., Knoblaugh, S., et al. (2008). A heme export protein is required for red blood cell differentiation and iron homeostasis. Science 319 (5864), 825–828. doi:10.1126/science.1151133
Klaus, S., Rudolph, B., Dohrmann, C., and Wehr, R. (2005). Expression of uncoupling protein 1 in skeletal muscle decreases muscle energy efficiency and affects thermoregulation and substrate oxidation. Physiol. Genomics 21 (2), 193–200. doi:10.1152/physiolgenomics.00299.2004
Knovich, M. A., Storey, J. A., Coffman, L. G., Torti, S. V., and Torti, F. M. (2009). Ferritin for the clinician. Blood Rev. 23 (3), 95–104. doi:10.1016/j.blre.2008.08.001
Koeller, D. M., Casey, J. L., Hentze, M. W., Gerhardt, E. M., Chan, L. N., Klausner, R. D., et al. (1989). A cytosolic protein binds to structural elements within the iron regulatory region of the transferrin receptor mRNA. Proc. Natl. Acad. Sci. U. S. A. 86 (10), 3574–3578. doi:10.1073/pnas.86.10.3574
Kong, N., Chen, X., Feng, J., Duan, T., Liu, S., Sun, X., et al. (2021). Baicalin induces ferroptosis in bladder cancer cells by downregulating FTH1. Acta Pharm. Sin. B 11 (12), 4045–4054. doi:10.1016/j.apsb.2021.03.036
Kraft, V. A. N., Bezjian, C. T., Pfeiffer, S., Ringelstetter, L., Muller, C., Zandkarimi, F., et al. (2020). GTP cyclohydrolase 1/tetrahydrobiopterin counteract ferroptosis through lipid remodeling. ACS Cent. Sci. 6 (1), 41–53. doi:10.1021/acscentsci.9b01063
Kramer, S., Meadows, A. T., Jarrett, P., and Evans, A. E. (1983). Incidence of childhood cancer: Experience of a decade in a population-based registry. J. Natl. Cancer Inst. 70 (1), 49–55.
Krishnamurthy, P. C., Du, G., Fukuda, Y., Sun, D., Sampath, J., Mercer, K. E., et al. (2006). Identification of a mammalian mitochondrial porphyrin transporter. Nature 443 (7111), 586–589. doi:10.1038/nature05125
Kwon, M. Y., Park, E., Lee, S. J., and Chung, S. W. (2015). Heme oxygenase-1 accelerates erastin-induced ferroptotic cell death. Oncotarget 6 (27), 24393–24403. doi:10.18632/oncotarget.5162
Laitano, O., Oki, K., and Leon, L. R. (2021). The role of skeletal muscles in exertional heat stroke pathophysiology. Int. J. Sports Med. 42 (8), 673–681. doi:10.1055/a-1400-9754
Laredj, L. N., Licitra, F., and Puccio, H. M. (2014). The molecular genetics of coenzyme Q biosynthesis in health and disease. Biochimie 100, 78–87. doi:10.1016/j.biochi.2013.12.006
Latunde-Dada, G. O. (2017). Ferroptosis: Role of lipid peroxidation, iron and ferritinophagy. Biochim. Biophys. Acta. Gen. Subj. 1861 (8), 1893–1900. doi:10.1016/j.bbagen.2017.05.019
Lee, J. Y., Kim, W. K., Bae, K. H., Lee, S. C., and Lee, E. W. (2021). Lipid metabolism and ferroptosis. Biol. (Basel) 10 (3), 184. doi:10.3390/biology10030184
Lei, G., Zhang, Y., Hong, T., Zhang, X., Liu, X., Mao, C., et al. (2021). Ferroptosis as a mechanism to mediate p53 function in tumor radiosensitivity. Oncogene 40 (20), 3533–3547. doi:10.1038/s41388-021-01790-w
Lewerenz, J., Hewett, S. J., Huang, Y., Lambros, M., Gout, P. W., Kalivas, P. W., et al. (2013). The cystine/glutamate antiporter system x(c)(-) in health and disease: From molecular mechanisms to novel therapeutic opportunities. Antioxid. Redox Signal. 18 (5), 522–555. doi:10.1089/ars.2011.4391
Li, D., Jiang, C., Mei, G., Zhao, Y., Chen, L., Liu, J., et al. (2020b). Quercetin alleviates ferroptosis of pancreatic beta cells in type 2 diabetes. Nutrients 12 (10), E2954. doi:10.3390/nu12102954
Li, J., Cao, F., Yin, H. L., Huang, Z. J., Lin, Z. T., Mao, N., et al. (2020a). Ferroptosis: Past, present and future. Cell Death Dis. 11 (2), 88. doi:10.1038/s41419-020-2298-2
Li, J., Lu, K., Sun, F., Tan, S., Zhang, X., Sheng, W., et al. (2021b). Panaxydol attenuates ferroptosis against LPS-induced acute lung injury in mice by Keap1-Nrf2/HO-1 pathway. J. Transl. Med. 19 (1), 96. doi:10.1186/s12967-021-02745-1
Li, M. Y., Dai, X. H., Yu, X. P., Zou, W., Teng, W., Liu, P., et al. (2022). Scalp acupuncture protects against neuronal ferroptosis by activating the p62-keap1-nrf2 pathway in rat models of intracranial haemorrhage. J. Mol. Neurosci. 72 (1), 82–96. doi:10.1007/s12031-021-01890-y
Li, Y., Yan, H., Xu, X., Liu, H., Wu, C., and Zhao, L. (2020c). Erastin/sorafenib induces cisplatin-resistant non-small cell lung cancer cell ferroptosis through inhibition of the Nrf2/xCT pathway. Oncol. Lett. 19 (1), 323–333. doi:10.3892/ol.2019.11066
Li, Y., Zeng, X., Lu, D., Yin, M., Shan, M., and Gao, Y. (2021a). Erastin induces ferroptosis via ferroportin-mediated iron accumulation in endometriosis. Hum. Reprod. 36 (4), 951–964. doi:10.1093/humrep/deaa363
Li, Z. J., Dai, H. Q., Huang, X. W., Feng, J., Deng, J. H., Wang, Z. X., et al. (2021c). Artesunate synergizes with sorafenib to induce ferroptosis in hepatocellular carcinoma. Acta Pharmacol. Sin. 42 (2), 301–310. doi:10.1038/s41401-020-0478-3
Lima, R. S., da Silva Junior, G. B., Liborio, A. B., and Daher Ede, F. (2008). Acute kidney injury due to rhabdomyolysis. Saudi J. Kidney Dis. Transpl. 19 (5), 721–729.
Lindner, A., and Zierz, S. (2003). Rhabdomyolysis and myoglobinuria. Nervenarzt 74 (6), 505–515. doi:10.1007/s00115-003-1518-1
Liu, C. K., Leng, X., Hsu, F. C., Kritchevsky, S. B., Ding, J., Earnest, C. P., et al. (2014). The impact of sarcopenia on a physical activity intervention: The lifestyle interventions and independence for elders pilot study (LIFE-P). J. Nutr. Health Aging 18 (1), 59–64. doi:10.1007/s12603-013-0369-0
Liu, S., Meng, F., Zhang, D., Shi, D., Zhou, J., Guo, S., et al. (2022b). Lonicera caerulea berry polyphenols extract alleviates exercise fatigue in mice by reducing oxidative stress, inflammation, skeletal muscle cell apoptosis, and by increasing cell proliferation. Front. Nutr. 9, 853225. doi:10.3389/fnut.2022.853225
Liu, T., Xu, P., Ke, S., Dong, H., Zhan, M., Hu, Q., et al. (2022a). Histone methyltransferase SETDB1 inhibits TGF-beta-induced epithelial-mesenchymal transition in pulmonary fibrosis by regulating SNAI1 expression and the ferroptosis signaling pathway. Arch. Biochem. Biophys. 715, 109087. doi:10.1016/j.abb.2021.109087
Liu, Z., Lv, X., Song, E., and Song, Y. (2020). Fostered Nrf2 expression antagonizes iron overload and glutathione depletion to promote resistance of neuron-like cells to ferroptosis. Toxicol. Appl. Pharmacol. 407, 115241. doi:10.1016/j.taap.2020.115241
Lu, H., Xiao, H., Dai, M., Xue, Y., and Zhao, R. (2022). Britanin relieves ferroptosis-mediated myocardial ischaemia/reperfusion damage by upregulating GPX4 through activation of AMPK/GSK3β/Nrf2 signalling. Pharm. Biol. 60 (1), 38–45. doi:10.1080/13880209.2021.2007269
Lu, S. C. (2009). Regulation of glutathione synthesis. Mol. Asp. Med. 30 (1-2), 42–59. doi:10.1016/j.mam.2008.05.005
Lu, Y., Yang, Q., Su, Y., Ji, Y., Li, G., Yang, X., et al. (2021). MYCN mediates TFRC-dependent ferroptosis and reveals vulnerabilities in neuroblastoma. Cell Death Dis. 12 (6), 511. doi:10.1038/s41419-021-03790-w
Lundberg, I. E., Miller, F. W., Tjarnlund, A., and Bottai, M. (2016). Diagnosis and classification of idiopathic inflammatory myopathies. J. Intern. Med. 280 (1), 39–51. doi:10.1111/joim.12524
Lv, Z., Wang, F., Zhang, X., Zhang, X., Zhang, J., and Liu, R. (2021). Etomidate attenuates the ferroptosis in myocardial ischemia/reperfusion rat model via Nrf2/HO-1 pathway. Shock 56 (3), 440–449. doi:10.1097/SHK.0000000000001751
Ma, S., Sun, L., Wu, W., Wu, J., Sun, Z., and Ren, J. (2020b). USP22 protects against myocardial ischemia-reperfusion injury via the SIRT1-p53/slc7a11-dependent inhibition of ferroptosis-induced cardiomyocyte death. Front. Physiol. 11, 551318. doi:10.3389/fphys.2020.551318
Madduma Hewage, S. R. K., Piao, M. J., Kang, K. A., Ryu, Y. S., Fernando, P., Oh, M. C., et al. (2017). Galangin activates the ERK/AKT-Driven Nrf2 signaling pathway to increase the level of reduced glutathione in human keratinocytes. Biomol. Ther. 25 (4), 427–433. doi:10.4062/biomolther.2016.112
Mancias, J. D., Wang, X., Gygi, S. P., Harper, J. W., and Kimmelman, A. C. (2014). Quantitative proteomics identifies NCOA4 as the cargo receptor mediating ferritinophagy. Nature 509 (7498), 105–109. doi:10.1038/nature13148
Mandal, P. K., Seiler, A., Perisic, T., Kolle, P., Banjac Canak, A., Forster, H., et al. (2010). System x(c)- and thioredoxin reductase 1 cooperatively rescue glutathione deficiency. J. Biol. Chem. 285 (29), 22244–22253. doi:10.1074/jbc.M110.121327
Mao, C., Liu, X., Zhang, Y., Lei, G., Yan, Y., Lee, H., et al. (2021). DHODH-mediated ferroptosis defence is a targetable vulnerability in cancer. Nature 593 (7860), 586–590. doi:10.1038/s41586-021-03539-7
Mauro, A. (1961). Satellite cell of skeletal muscle fibers. J. Biophys. Biochem. Cytol. 9, 493–495. doi:10.1083/jcb.9.2.493
McBean, G. J. (2012). The transsulfuration pathway: A source of cysteine for glutathione in astrocytes. Amino Acids 42 (1), 199–205. doi:10.1007/s00726-011-0864-8
Meister, A., and Anderson, M. E. (1983). Glutathione. Annu. Rev. Biochem. 52, 711–760. doi:10.1146/annurev.bi.52.070183.003431
Meyer, A., Laverny, G., Allenbach, Y., Grelet, E., Ueberschlag, V., Echaniz-Laguna, A., et al. (2017). IFN-beta-induced reactive oxygen species and mitochondrial damage contribute to muscle impairment and inflammation maintenance in dermatomyositis. Acta Neuropathol. 134 (4), 655–666. doi:10.1007/s00401-017-1731-9
Meyer, C., Dostou, J. M., Welle, S. L., and Gerich, J. E. (2002). Role of human liver, kidney, and skeletal muscle in postprandial glucose homeostasis. Am. J. Physiol. Endocrinol. Metab. 282 (2), E419–E427. doi:10.1152/ajpendo.00032.2001
Miao, H., Ren, Q., Li, H., Zeng, M., Chen, D., Xu, C., et al. (2022). Comprehensive analysis of the autophagy-dependent ferroptosis-related gene FANCD2 in lung adenocarcinoma. BMC Cancer 22 (1), 225. doi:10.1186/s12885-022-09314-9
Moosmann, B., and Behl, C. (2004). Selenoproteins, cholesterol-lowering drugs, and the consequences: Revisiting of the mevalonate pathway. Trends cardiovasc. Med. 14 (7), 273–281. doi:10.1016/j.tcm.2004.08.003
Mosharov, E., Cranford, M. R., and Banerjee, R. (2000). The quantitatively important relationship between homocysteine metabolism and glutathione synthesis by the transsulfuration pathway and its regulation by redox changes. Biochemistry 39 (42), 13005–13011. doi:10.1021/bi001088w
Mugoni, V., Postel, R., Catanzaro, V., De Luca, E., Turco, E., Digilio, G., et al. (2013). Ubiad1 is an antioxidant enzyme that regulates eNOS activity by CoQ10 synthesis. Cell 152 (3), 504–518. doi:10.1016/j.cell.2013.01.013
Murphy, T. H., Miyamoto, M., Sastre, A., Schnaar, R. L., and Coyle, J. T. (1989). Glutamate toxicity in a neuronal cell line involves inhibition of cystine transport leading to oxidative stress. Neuron 2 (6), 1547–1558. doi:10.1016/0896-6273(89)90043-3
Nakanishi, K., Taniguchi, T., Ranganathan, V., New, H. V., Moreau, L. A., Stotsky, M., et al. (2002). Interaction of FANCD2 and NBS1 in the DNA damage response. Nat. Cell Biol. 4 (12), 913–920. doi:10.1038/ncb879
Nemeth, E., Tuttle, M. S., Powelson, J., Vaughn, M. B., Donovan, A., Ward, D. M., et al. (2004). Hepcidin regulates cellular iron efflux by binding to ferroportin and inducing its internalization. Science 306 (5704), 2090–2093. doi:10.1126/science.1104742
Nishizawa, S., Araki, H., Ishikawa, Y., Kitazawa, S., Hata, A., Soga, T., et al. (2018). Low tumor glutathione level as a sensitivity marker for glutamate-cysteine ligase inhibitors. Oncol. Lett. 15 (6), 8735–8743. doi:10.3892/ol.2018.8447
Niu, T., Fu, G., Zhou, J., Han, H., Chen, J., Wu, W., et al. (2020). Floridoside exhibits antioxidant properties by activating Ho-1 expression via P38/erk mapk pathway. Mar. Drugs 18 (2), E105. doi:10.3390/md18020105
Ognjanovic, S., Linabery, A. M., Charbonneau, B., and Ross, J. A. (2009). Trends in childhood rhabdomyosarcoma incidence and survival in the United States, 1975-2005. Cancer 115 (18), 4218–4226. doi:10.1002/cncr.24465
Ohgami, R. S., Campagna, D. R., Greer, E. L., Antiochos, B., McDonald, A., Chen, J., et al. (2005). Identification of a ferrireductase required for efficient transferrin-dependent iron uptake in erythroid cells. Nat. Genet. 37 (11), 1264–1269. doi:10.1038/ng1658
Oliveira, A., and Vaz, C. (2015). The role of sarcopenia in the risk of osteoporotic hip fracture. Clin. Rheumatol. 34 (10), 1673–1680. doi:10.1007/s10067-015-2943-9
Olney, J. W. (1971). Glutamate-induced neuronal necrosis in the infant mouse hypothalamus. An electron microscopic study. J. Neuropathol. Exp. Neurol. 30 (1), 75–90. doi:10.1097/00005072-197101000-00008
Ong, A. L. C., and Ramasamy, T. S. (2018). Role of Sirtuin1-p53 regulatory axis in aging, cancer and cellular reprogramming. Ageing Res. Rev. 43, 64–80. doi:10.1016/j.arr.2018.02.004
Ou, Y., Wang, S. J., Li, D., Chu, B., and Gu, W. (2016). Activation of SAT1 engages polyamine metabolism with p53-mediated ferroptotic responses. Proc. Natl. Acad. Sci. U. S. A. 113 (44), E6806–E6812. doi:10.1073/pnas.1607152113
Paradkar, P. N., Zumbrennen, K. B., Paw, B. H., Ward, D. M., and Kaplan, J. (2009). Regulation of mitochondrial iron import through differential turnover of mitoferrin 1 and mitoferrin 2. Mol. Cell. Biol. 29 (4), 1007–1016. doi:10.1128/MCB.01685-08
Parkes, J. E., Day, P. J., Chinoy, H., and Lamb, J. A. (2015). The role of microRNAs in the idiopathic inflammatory myopathies. Curr. Opin. Rheumatol. 27 (6), 608–615. doi:10.1097/BOR.0000000000000225
Paul, B. T., Manz, D. H., Torti, F. M., and Torti, S. V. (2017). Mitochondria and iron: Current questions. Expert Rev. Hematol. 10 (1), 65–79. doi:10.1080/17474086.2016.1268047
Pedersen, B. K., and Febbraio, M. A. (2008). Muscle as an endocrine organ: Focus on muscle-derived interleukin-6. Physiol. Rev. 88 (4), 1379–1406. doi:10.1152/physrev.90100.2007
Perez-Sala, D., Parrilla, R., and Ayuso, M. S. (1987). Key role of L-alanine in the control of hepatic protein synthesis. Biochem. J. 241 (2), 491–498. doi:10.1042/bj2410491
Perriello, G., Nurjhan, N., Stumvoll, M., Bucci, A., Welle, S., Dailey, G., et al. (1997). Regulation of gluconeogenesis by glutamine in normal postabsorptive humans. Am. J. Physiol. 272, E437–E445. doi:10.1152/ajpendo.1997.272.3.E437
Possemato, R., Marks, K. M., Shaul, Y. D., Pacold, M. E., Kim, D., Birsoy, K., et al. (2011). Functional genomics reveal that the serine synthesis pathway is essential in breast cancer. Nature 476 (7360), 346–350. doi:10.1038/nature10350
Qin, Z., Ou, S., Xu, L., Sorensen, K., Zhang, Y., Hu, D. P., et al. (2021). Design and synthesis of isothiocyanate-containing hybrid androgen receptor (AR) antagonist to downregulate AR and induce ferroptosis in GSH-Deficient prostate cancer cells. Chem. Biol. Drug Des. 97 (5), 1059–1078. doi:10.1111/cbdd.13826
Quigley, J. G., Yang, Z., Worthington, M. T., Phillips, J. D., Sabo, K. M., Sabath, D. E., et al. (2004). Identification of a human heme exporter that is essential for erythropoiesis. Cell 118 (6), 757–766. doi:10.1016/j.cell.2004.08.014
Reid, M. B. (2008). Free radicals and muscle fatigue: Of ROS, canaries, and the IOC. Free Radic. Biol. Med. 44 (2), 169–179. doi:10.1016/j.freeradbiomed.2007.03.002
Reid, M. B., Haack, K. E., Franchek, K. M., Valberg, P. A., Kobzik, L., and West, M. S. (1992). Reactive oxygen in skeletal muscle. I. Intracellular oxidant kinetics and fatigue in vitro. J. Appl. Physiol. 73 (5), 1797–1804. doi:10.1152/jappl.1992.73.5.1797
Rhee, S. G., Bae, Y. S., Lee, S. R., and Kwon, J. (2000). Hydrogen peroxide: A key messenger that modulates protein phosphorylation through cysteine oxidation. Sci. STKE 2000 (53), pe1. doi:10.1126/stke.2000.53.pe1
Rodriguez-Graciani, K. M., Chapa-Dubocq, X. R., Ayala-Arroyo, E. J., Chaves-Negron, I., Jang, S., Chorna, N., et al. (2022). Effects of ferroptosis on the metabolome in cardiac cells: The role of glutaminolysis. Antioxidants (Basel) 11 (2), 278. doi:10.3390/antiox11020278
Rotruck, J. T., Pope, A. L., Ganther, H. E., Swanson, A. B., Hafeman, D. G., and Hoekstra, W. G. (1973). Selenium: Biochemical role as a component of glutathione peroxidase. Science 179 (4073), 588–590. doi:10.1126/science.179.4073.588
Sbodio, J. I., Snyder, S. H., and Paul, B. D. (2019). Regulators of the transsulfuration pathway. Br. J. Pharmacol. 176 (4), 583–593. doi:10.1111/bph.14446
Schafer, F. Q., and Buettner, G. R. (2001). Redox environment of the cell as viewed through the redox state of the glutathione disulfide/glutathione couple. Free Radic. Biol. Med. 30 (11), 1191–1212. doi:10.1016/s0891-5849(01)00480-4
Schott, C., Graab, U., Cuvelier, N., Hahn, H., and Fulda, S. (2015). Oncogenic RAS mutants confer resistance of RMS13 rhabdomyosarcoma cells to oxidative stress-induced ferroptotic cell death. Front. Oncol. 5, 131. doi:10.3389/fonc.2015.00131
Scibior, A., Wojda, I., Wnuk, E., Pietrzyk, L., and Plewa, Z. (2021). Response of cytoprotective and detoxifying proteins to vanadate and/or magnesium in the rat liver: The nrf2-keap1 system. Oxid. Med. Cell. Longev. 2021, 8447456. doi:10.1155/2021/8447456
Sen, N., Cross, A. M., Lorenzi, P. L., Khan, J., Gryder, B. E., Kim, S., et al. (2018). EWS-FLI1 reprograms the metabolism of Ewing sarcoma cells via positive regulation of glutamine import and serine-glycine biosynthesis. Mol. Carcinog. 57 (10), 1342–1357. doi:10.1002/mc.22849
Shern, J. F., Chen, L., Chmielecki, J., Wei, J. S., Patidar, R., Rosenberg, M., et al. (2014). Comprehensive genomic analysis of rhabdomyosarcoma reveals a landscape of alterations affecting a common genetic axis in fusion-positive and fusion-negative tumors. Cancer Discov. 4 (2), 216–231. doi:10.1158/2159-8290.CD-13-0639
Shimada, K., Skouta, R., Kaplan, A., Yang, W. S., Hayano, M., Dixon, S. J., et al. (2016). Global survey of cell death mechanisms reveals metabolic regulation of ferroptosis. Nat. Chem. Biol. 12 (7), 497–503. doi:10.1038/nchembio.2079
Shiozu, H., Higashijima, M., and Koga, T. (2015). Association of sarcopenia with swallowing problems, related to nutrition and activities of daily living of elderly individuals. J. Phys. Ther. Sci. 27 (2), 393–396. doi:10.1589/jpts.27.393
Sikalidis, A. K., Mazor, K. M., Lee, J. I., Roman, H. B., Hirschberger, L. L., and Stipanuk, M. H. (2014). Upregulation of capacity for glutathione synthesis in response to amino acid deprivation: Regulation of glutamate-cysteine ligase subunits. Amino Acids 46 (5), 1285–1296. doi:10.1007/s00726-014-1687-1
Singh, A., Wu, H., Zhang, P., Happel, C., Ma, J., and Biswal, S. (2010). Expression of ABCG2 (BCRP) is regulated by Nrf2 in cancer cells that confers side population and chemoresistance phenotype. Mol. Cancer Ther. 9 (8), 2365–2376. doi:10.1158/1535-7163.MCT-10-0108
Song, Q., Peng, S., Sun, Z., Heng, X., and Zhu, X. (2021a). Temozolomide drives ferroptosis via a DMT1-dependent pathway in glioblastoma cells. Yonsei Med. J. 62 (9), 843–849. doi:10.3349/ymj.2021.62.9.843
Song, X., Xie, Y., Kang, R., Hou, W., Sun, X., Epperly, M. W., et al. (2016). FANCD2 protects against bone marrow injury from ferroptosis. Biochem. Biophys. Res. Commun. 480 (3), 443–449. doi:10.1016/j.bbrc.2016.10.068
Soula, M., Weber, R. A., Zilka, O., Alwaseem, H., La, K., Yen, F., et al. (2020). Metabolic determinants of cancer cell sensitivity to canonical ferroptosis inducers. Nat. Chem. Biol. 16 (12), 1351–1360. doi:10.1038/s41589-020-0613-y
Sousa-Victor, P., Gutarra, S., Garcia-Prat, L., Rodriguez-Ubreva, J., Ortet, L., Ruiz-Bonilla, V., et al. (2014). Geriatric muscle stem cells switch reversible quiescence into senescence. Nature 506 (7488), 316–321. doi:10.1038/nature13013
Stahl, K., Rastelli, E., and Schoser, B. (2020). A systematic review on the definition of rhabdomyolysis. J. Neurol. 267 (4), 877–882. doi:10.1007/s00415-019-09185-4
Stockwell, B. R. (2019). A powerful cell-protection system prevents cell death by ferroptosis. Nature 575 (7784), 597–598. doi:10.1038/d41586-019-03145-8
Su, L. J., Zhang, J. H., Gomez, H., Murugan, R., Hong, X., Xu, D., et al. (2019). Reactive oxygen species-induced lipid peroxidation in apoptosis, autophagy, and ferroptosis. Oxid. Med. Cell. Longev. 2019, 5080843. doi:10.1155/2019/5080843
Sun, X., Ou, Z., Chen, R., Niu, X., Chen, D., Kang, R., et al. (2016). Activation of the p62-Keap1-NRF2 pathway protects against ferroptosis in hepatocellular carcinoma cells. Hepatology 63 (1), 173–184. doi:10.1002/hep.28251
Sun, X., Ou, Z., Xie, M., Kang, R., Fan, Y., Niu, X., et al. (2015). HSPB1 as a novel regulator of ferroptotic cancer cell death. Oncogene 34 (45), 5617–5625. doi:10.1038/onc.2015.32
Sun, Y., Cui, D., Zhang, Z., Zhang, T., Shi, J., Jin, H., et al. (2016b). Attenuated oxidative stress following acute exhaustive swimming exercise was accompanied with modified gene expression profiles of apoptosis in the skeletal muscle of mice. Oxid. Med. Cell. Longev. 2016, 8381242. doi:10.1155/2016/8381242
Sun, Y., Zheng, Y., Wang, C., and Liu, Y. (2018). Glutathione depletion induces ferroptosis, autophagy, and premature cell senescence in retinal pigment epithelial cells. Cell Death Dis. 9 (7), 753. doi:10.1038/s41419-018-0794-4
Suzuki, S., Tanaka, T., Poyurovsky, M. V., Nagano, H., Mayama, T., Ohkubo, S., et al. (2010). Phosphate-activated glutaminase (GLS2), a p53-inducible regulator of glutamine metabolism and reactive oxygen species. Proc. Natl. Acad. Sci. U. S. A. 107 (16), 7461–7466. doi:10.1073/pnas.1002459107
Tan, C. T., Chang, H. C., Zhou, Q., Yu, C., Fu, N. Y., Sabapathy, K., et al. (2021). MOAP-1-mediated dissociation of p62/SQSTM1 bodies releases Keap1 and suppresses Nrf2 signaling. EMBO Rep. 22 (1), e50854. doi:10.15252/embr.202050854
Tan, K. T., Ang, S. J., and Tsai, S. Y. (2020a). Sarcopenia: Tilting the balance of protein homeostasis. Proteomics 20 (5-6), e1800411. doi:10.1002/pmic.201800411
Tang, B., Zhu, J., Li, J., Fan, K., Gao, Y., Cheng, S., et al. (2020). The ferroptosis and iron-metabolism signature robustly predicts clinical diagnosis, prognosis and immune microenvironment for hepatocellular carcinoma. Cell Commun. Signal. 18 (1), 174. doi:10.1186/s12964-020-00663-1
Te Braake, F. W., Schierbeek, H., de Groof, K., Vermes, A., Longini, M., Buonocore, G., et al. (2008). Glutathione synthesis rates after amino acid administration directly after birth in preterm infants. Am. J. Clin. Nutr. 88 (2), 333–339. doi:10.1093/ajcn/88.2.333
Tenhunen, R., Marver, H. S., and Schmid, R. (1968). The enzymatic conversion of heme to bilirubin by microsomal heme oxygenase. Proc. Natl. Acad. Sci. U. S. A. 61 (2), 748–755. doi:10.1073/pnas.61.2.748
Theil, E. C. (2013). Ferritin: The protein nanocage and iron biomineral in health and in disease. Inorg. Chem. 52 (21), 12223–12233. doi:10.1021/ic400484n
Thony, B., Auerbach, G., and Blau, N. (2000). Tetrahydrobiopterin biosynthesis, regeneration and functions. Biochem. J. 347 Pt 1, 1–16. doi:10.1042/bj3470001
Tian, H., Xiong, Y., Zhang, Y., Leng, Y., Tao, J., Li, L., et al. (2021). Activation of NRF2/FPN1 pathway attenuates myocardial ischemia-reperfusion injury in diabetic rats by regulating iron homeostasis and ferroptosis. Cell Stress Chaperones 27 (2), 149–164. doi:10.1007/s12192-022-01257-1
Tian, Y., Lu, J., Hao, X., Li, H., Zhang, G., Liu, X., et al. (2020). FTH1 inhibits ferroptosis through ferritinophagy in the 6-OHDA model of Parkinson's disease. Neurotherapeutics 17 (4), 1796–1812. doi:10.1007/s13311-020-00929-z
Tsekoura, M., Kastrinis, A., Katsoulaki, M., Billis, E., and Gliatis, J. (2017). Sarcopenia and its impact on quality of life. Adv. Exp. Med. Biol. 987, 213–218. doi:10.1007/978-3-319-57379-3_19
Tuo, Q. Z., Masaldan, S., Southon, A., Mawal, C., Ayton, S., Bush, A. I., et al. (2021). Characterization of selenium compounds for anti-ferroptotic activity in neuronal cells and after cerebral ischemia-reperfusion injury. Neurotherapeutics 18 (4), 2682–2691. doi:10.1007/s13311-021-01111-9
Ursini, F., and Maiorino, M. (2020). Lipid peroxidation and ferroptosis: The role of GSH and GPx4. Free Radic. Biol. Med. 152, 175–185. doi:10.1016/j.freeradbiomed.2020.02.027
Venkatesh, D., O'Brien, N. A., Zandkarimi, F., Tong, D. R., Stokes, M. E., Dunn, D. E., et al. (2020). MDM2 and MDMX promote ferroptosis by PPARα-mediated lipid remodeling. Genes Dev. 34 (7-8), 526–543. doi:10.1101/gad.334219.119
Wang, D., Wei, G., Ma, J., Cheng, S., Jia, L., Song, X., et al. (2021b). Identification of the prognostic value of ferroptosis-related gene signature in breast cancer patients. BMC Cancer 21 (1), 645. doi:10.1186/s12885-021-08341-2
Wang, J., and Pantopoulos, K. (2011). Regulation of cellular iron metabolism. Biochem. J. 434 (3), 365–381. doi:10.1042/BJ20101825
Wang, J., Wang, Y., Liu, Y., Cai, X., Huang, X., Fu, W., et al. (2022). Ferroptosis, a new target for treatment of renal injury and fibrosis in a 5/6 nephrectomy-induced CKD rat model. Cell Death Discov. 8 (1), 127. doi:10.1038/s41420-022-00931-8
Wang, K., Zhang, Z., Tsai, H. I., Liu, Y., Gao, J., Wang, M., et al. (2021d). Branched-chain amino acid aminotransferase 2 regulates ferroptotic cell death in cancer cells. Cell Death Differ. 28 (4), 1222–1236. doi:10.1038/s41418-020-00644-4
Wang, L., Liu, Y., Du, T., Yang, H., Lei, L., Guo, M., et al. (2020). ATF3 promotes erastin-induced ferroptosis by suppressing system Xc(.). Cell Death Differ. 27 (2), 662–675. doi:10.1038/s41418-019-0380-z
Wang, Y. Q., Chang, S. Y., Wu, Q., Gou, Y. J., Jia, L., Cui, Y. M., et al. (2016). The protective role of mitochondrial ferritin on erastin-induced ferroptosis. Front. Aging Neurosci. 8, 308. doi:10.3389/fnagi.2016.00308
Wang, Y., Yu, R., Wu, L., and Yang, G. (2021c). Hydrogen sulfide guards myoblasts from ferroptosis by inhibiting ALOX12 acetylation. Cell. Signal. 78, 109870. doi:10.1016/j.cellsig.2020.109870
Ward, D. M., and Kaplan, J. (2012). Ferroportin-mediated iron transport: Expression and regulation. Biochim. Biophys. Acta 1823 (9), 1426–1433. doi:10.1016/j.bbamcr.2012.03.004
Welch, A. A., Hayhoe, R. P. G., and Cameron, D. (2020). The relationships between sarcopenic skeletal muscle loss during ageing and macronutrient metabolism, obesity and onset of diabetes. Proc. Nutr. Soc. 79 (1), 158–169. doi:10.1017/S0029665119001150
Whillier, S., Garcia, B., Chapman, B. E., Kuchel, P. W., and Raftos, J. E. (2011). Glutamine and alpha-ketoglutarate as glutamate sources for glutathione synthesis in human erythrocytes. FEBS J. 278 (17), 3152–3163. doi:10.1111/j.1742-4658.2011.08241.x
Winterbourn, C. C. (1995). Toxicity of iron and hydrogen peroxide: The Fenton reaction. Toxicol. Lett. 82-83, 969–974. doi:10.1016/0378-4274(95)03532-x
Wolfe, R. R. (2006). The underappreciated role of muscle in health and disease. Am. J. Clin. Nutr. 84 (3), 475–482. doi:10.1093/ajcn/84.3.475
Worwood, M. (1987). The diagnostic value of serum ferritin determinations for assessing iron status. Haematol. (Budap) 20 (4), 229–235.
Wrann, C. D., White, J. P., Salogiannnis, J., Laznik-Bogoslavski, D., Wu, J., Ma, D., et al. (2013). Exercise induces hippocampal BDNF through a PGC-1α/FNDC5 pathway. Cell Metab. 18 (5), 649–659. doi:10.1016/j.cmet.2013.09.008
Wu, A., Feng, B., Yu, J., Yan, L., Che, L., Zhuo, Y., et al. (2021). Fibroblast growth factor 21 attenuates iron overload-induced liver injury and fibrosis by inhibiting ferroptosis. Redox Biol. 46, 102131. doi:10.1016/j.redox.2021.102131
Wu, C., Zhao, W., Yu, J., Li, S., Lin, L., and Chen, X. (2018). Induction of ferroptosis and mitochondrial dysfunction by oxidative stress in PC12 cells. Sci. Rep. 8 (1), 574. doi:10.1038/s41598-017-18935-1
Xiang, L., Xie, G., Liu, C., Zhou, J., Chen, J., Yu, S., et al. (2013). Knock-down of glutaminase 2 expression decreases glutathione, NADH, and sensitizes cervical cancer to ionizing radiation. Biochim. Biophys. Acta 1833 (12), 2996–3005. doi:10.1016/j.bbamcr.2013.08.003
Xiao, R., Wei, Y., Zhang, Y., Xu, F., Ma, C., Gong, Q., et al. (2022). Trilobatin, a naturally occurring food additive, ameliorates exhaustive exercise-induced fatigue in mice: Involvement of Nrf2/ARE/ferroptosis signaling pathway. Front. Pharmacol. 13, 913367. doi:10.3389/fphar.2022.913367
Xie, Y., Zhu, S., Song, X., Sun, X., Fan, Y., Liu, J., et al. (2017). The tumor suppressor p53 limits ferroptosis by blocking DPP4 activity. Cell Rep. 20 (7), 1692–1704. doi:10.1016/j.celrep.2017.07.055
Xu, H., Liu, X., Xia, J., Yu, T., Qu, Y., Jiang, H., et al. (2018). Activation of NMDA receptors mediated iron accumulation via modulating iron transporters in Parkinson's disease. FASEB J. 32, 6100–6111. doi:10.1096/fj.201800060RR
Xu, M., Li, Y., Meng, D., Zhang, D., Wang, B., Xie, J., et al. (2022b). 6-Hydroxydopamine induces abnormal iron sequestration in BV2 microglia by activating iron regulatory protein 1 and inhibiting hepcidin release. Biomolecules 12 (2), 266. doi:10.3390/biom12020266
Xu, Y., Li, Y., Li, J., and Chen, W. (2022a). Ethyl carbamate triggers ferroptosis in liver through inhibiting GSH synthesis and suppressing Nrf2 activation. Redox Biol. 53, 102349. doi:10.1016/j.redox.2022.102349
Xue, J., Yu, C., Sheng, W., Zhu, W., Luo, J., Zhang, Q., et al. (2017). The nrf2/GCH1/BH4 Axis Ameliorates radiation-induced skin injury by modulating the ROS cascade. J. Invest. Dermatol. 137 (10), 2059–2068. doi:10.1016/j.jid.2017.05.019
Yamamoto, M., Kensler, T. W., and Motohashi, H. (2018). The KEAP1-NRF2 system: A thiol-based sensor-effector apparatus for maintaining redox homeostasis. Physiol. Rev. 98 (3), 1169–1203. doi:10.1152/physrev.00023.2017
Yamamoto, S. (1991). Enzymatic" lipid peroxidation: Reactions of mammalian lipoxygenases. Free Radic. Biol. Med. 10 (2), 149–159. doi:10.1016/0891-5849(91)90008-q
Yang, W. S., Kim, K. J., Gaschler, M. M., Patel, M., Shchepinov, M. S., and Stockwell, B. R. (2016). Peroxidation of polyunsaturated fatty acids by lipoxygenases drives ferroptosis. Proc. Natl. Acad. Sci. U. S. A. 113 (34), E4966–E4975. doi:10.1073/pnas.1603244113
Yang, W. S., and Stockwell, B. R. (2016). Ferroptosis: Death by lipid peroxidation. Trends Cell Biol. 26 (3), 165–176. doi:10.1016/j.tcb.2015.10.014
Yang, X., Hao, Y., Zhang, X., Geng, Y., Ji, L., Li, G., et al. (2020). Mortality of Chinese patients with polymyositis and dermatomyositis. Clin. Rheumatol. 39 (5), 1569–1579. doi:10.1007/s10067-019-04910-w
Yang, Y., Bazhin, A. V., Werner, J., and Karakhanova, S. (2013). Reactive oxygen species in the immune system. Int. Rev. Immunol. 32 (3), 249–270. doi:10.3109/08830185.2012.755176
Yao, F., Cui, X., Zhang, Y., Bei, Z., Wang, H., Zhao, D., et al. (2021). Iron regulatory protein 1 promotes ferroptosis by sustaining cellular iron homeostasis in melanoma. Oncol. Lett. 22 (3), 657. doi:10.3892/ol.2021.12918
Ye, J., Fan, J., Venneti, S., Wan, Y. W., Pawel, B. R., Zhang, J., et al. (2014). Serine catabolism regulates mitochondrial redox control during hypoxia. Cancer Discov. 4 (12), 1406–1417. doi:10.1158/2159-8290.CD-14-0250
Yin, H., Price, F., and Rudnicki, M. A. (2013). Satellite cells and the muscle stem cell niche. Physiol. Rev. 93 (1), 23–67. doi:10.1152/physrev.00043.2011
Yu, H., Guo, P., Xie, X., Wang, Y., and Chen, G. (2017). Ferroptosis, a new form of cell death, and its relationships with tumourous diseases. J. Cell. Mol. Med. 21 (4), 648–657. doi:10.1111/jcmm.13008
Yu, Y., Jiang, L., Wang, H., Shen, Z., Cheng, Q., Zhang, P., et al. (2020). Hepatic transferrin plays a role in systemic iron homeostasis and liver ferroptosis. Blood 136 (6), 726–739. doi:10.1182/blood.2019002907
Yuan, H., Li, X., Zhang, X., Kang, R., and Tang, D. (2016b). CISD1 inhibits ferroptosis by protection against mitochondrial lipid peroxidation. Biochem. Biophys. Res. Commun. 478 (2), 838–844. doi:10.1016/j.bbrc.2016.08.034
Yuan, H., Li, X., Zhang, X., Kang, R., and Tang, D. (2016a). Identification of ACSL4 as a biomarker and contributor of ferroptosis. Biochem. Biophys. Res. Commun. 478 (3), 1338–1343. doi:10.1016/j.bbrc.2016.08.124
Zeitler, L., Fiore, A., Meyer, C., Russier, M., Zanella, G., Suppmann, S., et al. (2021). Anti-ferroptotic mechanism of IL4i1-mediated amino acid metabolism. Elife 10, e64806. doi:10.7554/eLife.64806
Zhang, H., Deng, T., Liu, R., Ning, T., Yang, H., Liu, D., et al. (2020b). CAF secreted miR-522 suppresses ferroptosis and promotes acquired chemo-resistance in gastric cancer. Mol. Cancer 19 (1), 43. doi:10.1186/s12943-020-01168-8
Zhang, H., Gomez, A. M., Wang, X., Yan, Y., Zheng, M., and Cheng, H. (2013b). ROS regulation of microdomain Ca(2+) signalling at the dyads. Cardiovasc. Res. 98 (2), 248–258. doi:10.1093/cvr/cvt050
Zhang, H., Ostrowski, R., Jiang, D., Zhao, Q., Liang, Y., Che, X., et al. (2021a). Hepcidin promoted ferroptosis through iron metabolism which is associated with DMT1 signaling activation in early brain injury following subarachnoid hemorrhage. Oxid. Med. Cell. Longev. 2021, 9800794. doi:10.1155/2021/9800794
Zhang, J., Wang, X., Vikash, V., Ye, Q., Wu, D., Liu, Y., et al. (2016). ROS and ROS-mediated cellular signaling. Oxid. Med. Cell. Longev. 2016, 4350965. doi:10.1155/2016/4350965
Zhang, J., Zhang, X., Li, J., and Song, Z. (2020). Systematic analysis of the ABC transporter family in hepatocellular carcinoma reveals the importance of ABCB6 in regulating ferroptosis. Life Sci. 257, 118131. doi:10.1016/j.lfs.2020.118131
Zhang, M., Linardic, C. M., and Kirsch, D. G. (2013). RAS and ROS in rhabdomyosarcoma. Cancer Cell 24 (6), 689–691. doi:10.1016/j.ccr.2013.11.015
Zhang, M., Zhang, T., Song, C., Qu, J., Gu, Y., Liu, S., et al. (2021c). Guizhi Fuling Capsule ameliorates endometrial hyperplasia through promoting p62-Keap1-NRF2-mediated ferroptosis. J. Ethnopharmacol. 274, 114064. doi:10.1016/j.jep.2021.114064
Zhang, Q., Qu, H., Chen, Y., Luo, X., Chen, C., Xiao, B., et al. (2022e). Atorvastatin induces mitochondria-dependent ferroptosis via the modulation of nrf2-xCT/GPx4 Axis. Front. Cell Dev. Biol. 10, 806081. doi:10.3389/fcell.2022.806081
Zhang, T., Sun, L., Hao, Y., Suo, C., Shen, S., Wei, H., et al. (2022a). ENO1 suppresses cancer cell ferroptosis by degrading the mRNA of iron regulatory protein 1. Nat. Cancer 3 (1), 75–89. doi:10.1038/s43018-021-00299-1
Zhang, Y., Xia, M., Zhou, Z., Hu, X., Wang, J., Zhang, M., et al. (2021d). p53 promoted ferroptosis in ovarian cancer cells treated with human serum incubated-superparamagnetic iron oxides. Int. J. Nanomedicine 16, 283–296. doi:10.2147/IJN.S282489
Zhang, Y., Zheng, L., Deng, H., Feng, D., Hu, S., Zhu, L., et al. (2022d). Electroacupuncture alleviates LPS-induced ARDS through α7 nicotinic acetylcholine receptor-mediated inhibition of ferroptosis. Front. Immunol. 13, 832432. doi:10.3389/fimmu.2022.832432
Zhao, S., Wang, X., Zheng, X., Liang, X., Wang, Z., Zhang, J., et al. (2021b). Iron deficiency exacerbates cisplatin- or rhabdomyolysis-induced acute kidney injury through promoting iron-catalyzed oxidative damage. Free Radic. Biol. Med. 173, 81–96. doi:10.1016/j.freeradbiomed.2021.07.025
Zhao, X., Liu, Z., Gao, J., Li, H., Wang, X., Li, Y., et al. (2020a). Inhibition of ferroptosis attenuates busulfan-induced oligospermia in mice. Toxicology 440, 152489. doi:10.1016/j.tox.2020.152489
Zhao, Y., Li, Y., Zhang, R., Wang, F., Wang, T., and Jiao, Y. (2020b). The role of erastin in ferroptosis and its prospects in cancer therapy. Onco. Targets. Ther. 13, 5429–5441. doi:10.2147/OTT.S254995
Zheng, H., Shi, L., Tong, C., Liu, Y., and Hou, M. (2021). circSnx12 is involved in ferroptosis during heart failure by targeting miR-224-5p. Front. Cardiovasc. Med. 8, 656093. doi:10.3389/fcvm.2021.656093
Keywords: ferroptosis, mechanism, sarcopenia, rhabdomyolysis, rhabdomyosarcoma, fatigue, myositis
Citation: Wang Y, Zhang Z, Jiao W, Wang Y, Wang X, Zhao Y, Fan X, Tian L, Li X and Mi J (2022) Ferroptosis and its role in skeletal muscle diseases. Front. Mol. Biosci. 9:1051866. doi: 10.3389/fmolb.2022.1051866
Received: 23 September 2022; Accepted: 20 October 2022;
Published: 03 November 2022.
Edited by:
Xin Wang, National Institutes of Health (NIH), United StatesReviewed by:
Min Wan, Cornell University, United StatesEnchao Qiu, Thomas Jefferson University, United States
Xianyi Cai, Huazhong University of Science and Technology, China
Copyright © 2022 Wang, Zhang, Jiao, Wang, Wang, Zhao, Fan, Tian, Li and Mi. This is an open-access article distributed under the terms of the Creative Commons Attribution License (CC BY). The use, distribution or reproduction in other forums is permitted, provided the original author(s) and the copyright owner(s) are credited and that the original publication in this journal is cited, in accordance with accepted academic practice. No use, distribution or reproduction is permitted which does not comply with these terms.
*Correspondence: Xiangyan Li, eGlhbmd5YW5fbGkxOTgxQDE2My5jb20=; Jia Mi, bWlqaWE4MjAxQDEyNi5jb20=
†These authors have contributed equally to this work