- 1Department of Nutrition and Food Hygiene, Guangdong Provincial Key Laboratory of Tropical Disease Research, National Medical Products Administration Key Laboratory of Cosmetic Safety Evaluation, School of Public Health, Southern Medical University, Guangzhou, Guangdong, China
- 2School of Physical Education, Hubei Minzu University, Enshi, China
- 3Key Laboratory of Antibody Engineering of Guangdong Higher Education Institutes, School of Laboratory Medicine and Biotechnology, Southern Medical University, Guangzhou, Guangdong, China
Noncommunicable diseases (NCDs), such as diabetes and related neurological disorders, are considered to not be directly transmissible from one person to another. However, NCDs may be transmissible in vivo through extracellular vesicles (EVs). A long-term high-fat diet (HFD) can induce a series of health issues like hyperlipidemia, type 2 diabetes mellitus (T2DM), and diabetic peripheral neuropathy (DPN) due to insulin resistance. Multiple molecular signaling changes can stimulate insulin resistance, especially blocking insulin signaling by increased insulin resistance inducer (phosphorylation of negative regulatory sites of insulin receptor substrate (IRS) proteins) and decreased tyrosine phosphorylation of insulin receptor substrate (phosphorylation of positive regulatory sites of IRS), thus leading to reduced phosphorylation of AKT enzymes. Current efforts to treat T2DM and prevent its complications mainly focus on improving insulin sensitivity, enhancing insulin secretion, or supplementing exogenous insulin based on a common assumption that insulin resistance is noncommunicable. However, insulin resistance is transmissible within multiple tissues or organs throughout the body. Exploring the regulatory roles of EVs in developing insulin resistance may provide novel and effective preventive and therapeutic strategies.
Introduction
The balance between energy consumption and intake is crucial to human health. Excessive energy intake can result in multiple metabolic diseases, including type 2 diabetes mellitus (T2DM) (Anderson et al., 2003), due to high-level blood glucose, deficient insulin secretion, and insulin resistance. Insulin resistance also can be induced by multiple factors, such as heredity, aging, and inflammation. The progression from insulin resistance to T2DM may take decades. Current treatments of insulin resistance or T2DM focus on promoting insulin sensitivity, improving islet β-cell function to enhance insulin secretion, or supplementing exogenous insulin. It is generally recognized that obesity can develop into T2DM with a long-term process of chronic excessive energy intake–obesity–insulin resistance–T2DM (Weyer et al., 1999; Leitner et al., 2017). The transmission of insulin resistance is also involved and critical in the development course of T2DM (Wang et al., 2017; Ying et al., 2017). Long-term hyperglycemia can induce chronic debilitating complications due to the toxicity of high-level glucose (Van den Berghe, 2004). Therefore, maintaining blood glucose levels is crucial to health. Usually, obesity is a common inducer of early T2DM, resulting from high caloric consumption and an irregular diet. Binge eating disorder is characterized by repeated gluttony, leading to a high incidence of chronic hyperglycemia among early diabetic patients (Allison et al., 2007; Abbott et al., 2018). To protect the body from glucose toxicity, the pancreas can produce a large amount of insulin to reduce blood glucose levels. A vicious cycle resulting from binge eating can lead to hyperinsulinemia and high serum insulin levels can cause a sense of hunger, thereby promoting the generation and accumulation of adipose tissue. During this process, insulin resistance gradually develops, as initially reflected in impaired glucose tolerance, then relative insulin deficiency, and eventually fasting hyperglycemia (Weyer et al., 1999). However, the exact mechanism for transmitting insulin resistance from tissues and organs to the whole body is still unclear. Recent evidence suggests that the factors for suppressing insulin signaling can be transmitted between cells via extracellular vesicles (EVs), thereby changing the definition of insulin resistance from a noncommunicable preclinical health condition to a transmissible pathological status (Dibaba et al., 2017; Ying et al., 2017).
EVs are particles delimited by a lipid bilayer and naturally released by cells (Théry et al., 2018). EVs can be classified into two major categories: microvesicles and exosomes. Microvesicles are formed by the budding of the plasma membrane, with particle sizes ranging from 50 nm to 1,000 nm. Exosomes are released from multivesicular endosomes (MVEs) after fusion with the plasma membrane. Functional studies of EVs have been spurred by their ability to transport various types of biomolecules, such as RNA, proteins, and DNA, to their recipient cells (Kapogiannis et al., 2015). Due to the similar biochemical properties and the overlapping size, it is often difficult to distinguish the functions of EVs (Kowal et al., 2016; Jeppesen et al., 2019). Currently, there is a lack of purification methods for the quantitative separation of various EV subclasses, making the assignment of functional properties to specific EV subtypes challenging. Therefore, there are no systematic studies on the roles and corresponding mechanisms of EVs in the development and transmission of insulin resistance. Recent studies have preliminarily confirmed that EVs can regulate the progression of insulin resistance (Choi et al., 2015). EVs from different cells may have opposite effects due to their different cargoes. Neutral ceramidase-enriched exosomes can prevent insulin resistance induced by palmitic acid, while the injection of exosomes from obese mice into healthy mice can lead to the development of insulin resistance (Deng et al., 2009; Zhu et al., 2016). Hence, if insulin resistance is treated as a transmissible pathological condition that can spread from cell to cell, the progression of T2DM could be inhibited by blocking its transmission.
In this article, we discuss the mechanisms that drive cell–EV–cell axis formation, thus causing insulin resistance, a point that has rarely been discussed in previous studies. Various factors may lead to insulin resistance, such as phosphorylation of most serine sites of insulin receptor substrate (IRS) proteins. It has been demonstrated that the phosphorylation of different serine residues has the opposite effect on insulin signaling (Copps and White, 2012). Here, we aim to discuss the function of the cell–EV–cell axis in the development of noncommunicable diseases (NCDs). Thus, all the “p-s-IRS” in this paper represent the phosphorylation of negative regulatory sites of IRS proteins. The word “exosome” has been used in the article, but it is noted that the term small extracellular vesicle (EV) is more precise and consistent with the latest findings in the EV field.
Insulin resistance can be transmitted via the cell–EV–cell axis to protect against stress-induced cellular damage
Insulin resistance is a cellular protective mechanism against glucose-induced ROS
The lesser sensitivity or complete nonresponsivity of cells to insulin is termed insulin resistance, a typical symptom at the early stage of T2DM. Insulin resistance can occur in multiple tissues and organs throughout the body and can be induced by a variety of inducers such as inflammation and obesity. Physiological stress can lead to a variety of cell dysfunctions, and some of these changes are protective mechanisms for improving cell survival through insulin resistance (Ye, 2013). Chronic exposure to hyperglycemia can lead to cellular dysfunction, termed glucotoxicity, which may become irreversible over time (Robertson et al., 2003; Staels, 2017). In addition to being an energy source, blood glucose can also result in side effects due to its toxicity. Glucose toxicity is reflected in its capacity to induce protein glycosylation (Burd, 2019). Glucose as a polyhydroxy aldehyde can react with amino residues of proteins to form fructosamine bonds and eventually become advanced glycation end products (AGEs) after a series of reactions. AGEs are associated with various diabetic complications such as diabetic retinopathy, kidney disease, and neurological diseases (Beisswenger, 2012). Therefore, the body can execute corresponding responses immediately by secreting insulin to reduce blood glucose levels and protect the body from glucose toxicity upon the high-level glucose stimulation.
Glucose is metabolized in cells into glyceric acid, glyceraldehyde, and acetone, thereby subsequently entering the tricarboxylic acid cycle and generating ATP through oxidative phosphorylation to provide energy for various physiological activities of cells. However, when a large amount of glucose enters cells, glycolysis is not able to consume enough glucose, and the glyceraldehyde metabolism is inhibited, thus eventually leading to the activation of nicotinamide adenine dinucleotide phosphate (NADPH) oxidases (NOX) (Han et al., 2012). NOX is a membrane protein widely distributed in tissues and organs of the body and includes multiple isoforms, such as NOX1, NOX2, NOX3, NOX4, and NOX5. NADPH is reduced after being utilized as the substrate of NOX2. Under the catalysis of NOX2, the electron can be transferred to O2 from NADPH on the cytosolic side of the phagosomal membrane, thereby resulting in the increase of O2−. Thus, NOX enzymes are the major source of ROS (Spencer and Engelhardt, 2014). NADPH is consumed during this process as the major antioxidant factor in cells and can reduce H2O2 to promote resistance to oxidative stress. Therefore, excessive glucose intake leads to the generation and accumulation of ROS (Han, 2016; Jiang et al., 2018), which can induce pathological oxidative damage in many tissues and cause the retrogradation of redox signaling in cells (Murphy, 2008). Oxygen free radicals, a type of ROS, are chemical species with an unpaired electron produced from molecular oxygen (Turrens, 2003). Both endogenous and exogenous free radicals can negatively impact bioactive factors such as nucleic acids, lipids, and proteins by altering the normal redox status, thus increasing oxidative stress. Free radical-dependent oxidative stress is involved in diabetes (Phaniendra et al., 2015).
The function of the cell membrane depends on the fluidity and physical state of the membrane, which is determined by the membrane lipid acyl chain profile. The acyl chains of lipids can be peroxidized by ROS, thereby reducing the fluidity of the cell membrane and leading to abnormal membrane function (Watanabe et al., 1990; Eze, 1992). ROS interferes with the normal physiological activities of cells and leads to the decreased expression of insulin-related genes and proteins, such as PDX-1 and MafA (Matsuoka et al., 1997; Bensellam et al., 2012). Hence, in the case of excessive glucose intake-induced insulin resistance, the cells must have a strong requirement to reduce the absorption of glucose to protect the normal function of the membrane (Ye, 2013). Insulin resistance is the hallmark of T2DM, associated with obesity induced by excessive energy intake (Veech, 2004). At the molecular level, insulin resistance is a complex pathological condition consisting of serious pathological phenomena, such as suppressed insulin receptor (IR), down-regulated p-AKT, or up-regulated p-s-IRS (Tanti and Jager, 2009; Tonks et al., 2013). It has been widely accepted that excessive generation and accumulation of ROS will significantly induce insulin resistance (Houstis et al., 2006). High uric acid-induced ROS can significantly inhibit the phosphorylation of AKT, promote the activation of p-s-IRS, and stimulate insulin resistance in differentiated 3T3L1 adipocytes and mice (Zhu et al., 2014; D'Apolito et al., 2010). Nonetheless, the mechanism of how ROS blocks insulin signaling is still unclear. Recent studies have demonstrated that ROS is an induction factor for EV secretion (Hedlund et al., 2011; Aswad et al., 2014; Atienzar-Aroca et al., 2016). O2− and H2O2 are two major stimulators of cell damage. EV secretion promotes a significant increase against H2O2-induced stress in Jurkat and Raji cells (Hedlund et al., 2011). Therefore, ROS may induce insulin resistance and stimulate the secretion of EVs in a feedback manner (Figure 1).
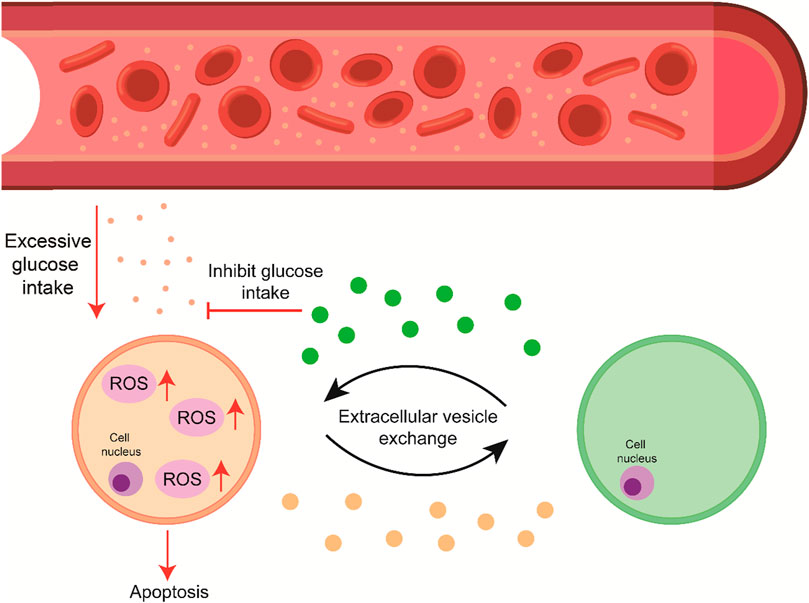
FIGURE 1. High blood glucose levels lead to ROS generation and accumulation, and the signal exchange through the cell–EV–cell axis promotes cell survival by suppressing apoptosis.
EVs form a transport axis between cells to spread insulin resistance
It has been shown that cells will form a signal axis through EVs due to stress-induced cellular damage. Donor cells can secrete EVs in response to the signaling of EVs from recipient cells (Crewe et al., 2018). In response to the signals received from EVs, endothelial cells secrete Caveolin1-containing EVs that are believed to be transported to specific adipocytes lacking the Caveolin1 protein. Furthermore, the process of Caveolin1 transfer relies mainly on EV transmission. When GW4869 inhibits EV secretion, the transmission of Caveolin1 can be significantly reduced (Crewe et al., 2018). However, a similar EV-dependent signaling axis can be formed under a state of excessive glucose intake. Excessive glucose not only promotes the generation and accumulation of ROS but also leads to the synthesis of lipids in cells (Parekh and Anania, 2007), thereby enhancing the secretion and transportation of adiponectin. Moreover, fatty acid-induced adiponectin transportation can be transmitted via EVs (DeClercq et al., 2015). Adiponectin is an adipokine involved in regulating the balance of glucose and lipid metabolism. It can reduce the oxidized LDL-induced ROS in endothelial cells (Ouedraogo et al., 2006; Tao et al., 2014). It has been demonstrated that exosomes with abundant adiponectin promote the release of interleukin-6 (IL-6) and tumor necrosis factor-α (TNF-α) from adipocytes (Zhang et al., 2016). IL-6 can regulate the inflammatory response and impair insulin signaling. TNF-α is a pro-inflammatory cytokine involved in systemic inflammation. TNF-α gene knockout mice have a higher sensitivity to insulin in an obese state (Hotamisligil, 1999). The secretion of IL-6 and TNF-α will reduce the translocation of IRS-1 and GLUT4, thus leading to insulin resistance (Uysal et al., 1997; Rotter et al., 2003). Furthermore, adipocyte-derived EVs have been proven to be the major immunomodulatory effectors for the secretion of insulin resistance factors (Kranendonk et al., 2014). Thus, excessive glucose-induced ROS may activate a cell–EV–cell signal axis to help cells under ROS stress gain insulin resistance factors.
EVs play a critical role in the development of diabetic complications such as DPN
evere complications often accompany diabetes, and neuropathy is the most common (Feldman et al., 2019), leading to many patient deaths. The treatment of DPN can significantly improve patient quality of life. The mechanism of DPN progression is associated with a variety of signaling pathways. Recent studies have shown that EVs have a significant influence on DPN, either positive or negative. Mesenchymal stromal cell (MSC)-derived exosomes significantly increased nerve conduction and inhibited the Toll-like receptor (TLR)4/NF-κB signaling pathway in diabetic mice with DPN (Fan et al., 2020). Exosomes enriched with miR-146a enhanced the therapeutic efficacy of DPN in diabetic mice (Fan et al., 2021). However, as we discussed previously, the function of EVs depends on the cargo they carry. An example is provided by the interaction of Schwann cells with nerve cells. Schwann cells significantly impact nerve cells, such as helping axons form typical large-caliber axons via controlling the number of neurofilaments and elevating the phosphorylation state of neurofilaments. EVs mediate intercellular communication between Schwann cells and nerve cells by exchanging their biomaterials. Exosomes derived from high-glucose-stimulated Schwann cells contain high levels of miR-28, miR-31a, and miR-130a, which may contribute to the development of DPN (Jia et al., 2018). Thus, nerve cells may also form a cell–EV–cell axis in response to the stimulation of multiple physiological changes.
Factors inhibiting insulin signaling can be transmitted via EVs
HFD-induced insulin resistance is due to down-regulated p-y-IRS and up-regulated p-s-IRS
In addition to obesity and other causes, at the molecular level, the abnormality of key proteins in the insulin signal pathway may affect cell sensitivity to insulin, such as the decrease in phosphorylated AKT, the up-regulation of phosphorylated IRS at the serine site (p-s-IRS), and the down-regulation of phosphorylated insulin receptor substrate at the tyrosine site (p-y-IRS) (Gao et al., 2002). Under normal circumstances, a cascade of reactions is activated after insulin binds to its cell surface receptors and causes receptor autophosphorylation. Phosphorylated insulin receptors will recruit their corresponding substrates to accomplish the phosphorylation at the tyrosine site, thereby further activating PI3K and leading to the phosphorylation of AKT. In contrast, p-s-IRS can activate subsequent signal pathways and inhibit p-y-IRS, thereby resulting in insulin resistance (Zhu et al., 2011). Multiple factors for inducing p-s-IRS can result in the increase of free fatty acids, cytokines, angiotensin II, endothelin-1, amino acids, cellular stress, and hyperinsulinemia (Gual et al., 2005). In addition, p-s-IRS can promote a decrease in tyrosine kinase activity (Schmelzle et al., 2006). Recent studies have shown that EVs can transmit these factors that block the insulin signal pathway between cells (Kapogiannis et al., 2015).
Ubiquitinated IR and IRS packed into EVs can be released into the extracellular environment
Many cells secrete EVs in an evolutionarily conserved manner. There is a wide range of EVs, including classical exosomes, nonclassical exosomes, classical microvesicles, large oncosomes, apoptotic vesicles, and autophagic extracellular vesicles (Jeppesen et al., 2019). Although the biogenesis of microvesicles and exosomes involves different pathways, they have similar morphology, compositions, and functions (Van Niel et al., 2018). As the medium for information transfers between cells, EVs secreted by different cells carry different substances and have multiple targets to cause different effects on recipient cells. Exosomes secreted by renal carcinoma cells will spread to other renal carcinoma cells and eventually cause resistance to sunitinib via transporting lncARSR (Qu et al., 2016). However, exosomes containing inflammasomes after central nervous system (CNS) injury can execute the protection of CNS from injury by activating the innate immune response of peripheral tissue (de Rivero Vaccari et al., 2016). Thus, to explore the functions of EVs, the substances in EVs must be studied. Exosomes secreted by T2DM patients have been found to contain p-s-IRS acting as an inhibitor to the insulin signal pathway (Kapogiannis et al., 2015). The most immediate factor for inducing EV formation is the abnormal expression or modification of ubiquitinated proteins. Recent studies have shown that the phosphorylation of IRS-1 at the serine site can lead to its degradation, followed by ubiquitination (Kim et al., 2012; Yoneyama et al., 2018). However, proteins monoubiquitinated on the cell surface are often transferred to multivesicular bodies (MVBs) (Buschow et al., 2005; Gual et al., 2005). Sorting machineries, such as transmembrane proteins and the endosomal sorting complex required for transport (ESCRT), can generate both microvesicles and exosomes (Akers et al., 2013). Exosomes are present in MVBs as intraluminal vesicles (ILVs) before release into the extracellular environment (Kowal et al., 2014; Wang et al., 2020). The MVBs from the early-stage endosomes and the formation of ILVs are involved in specific sorting machineries. These sorting machineries can separate the cargoes into a specific area of the MVE as the microdomain and germinate small membrane vesicles containing isolated cargoes (Kalra et al., 2016). The ESCRT complex is the driver of membrane invagination and budding to accomplish exosome formation in a certain order (Meldolesi, 2018). The ubiquitinated transmembrane cargoes are confined to the microdomain of the MVE by ESCRT-0 and ESCRT-I. Then, the ESCRT-III complex is recruited by ESCRT-II to conduct microdomain formation (Hurley, 2008). Transmembrane proteins are involved in sorting ESCRT-dependent and ESCRT-independent vesicle contents, such as transmembrane 4 superfamily (TM4SF) (Gual et al., 2005). When MVBs are formed, some are transported to lysosomes for degradation rather than fusion with the plasma membrane (Davies et al., 2009). As a calcium-dependent phospholipid-binding protein, Annexin A2 (Anxa2) can be involved in diverse cellular processes. Anxa2-containing MVBs can fuse directly with plasma membranes rather than be degraded by lysosomes (Valapala and Vishwanatha, 2011), and AnxA2 is highly expressed in diabetic patients (Bin et al., 2012). IR and IRS labeled with ubiquitin may be transported to MVBs rather than be degraded (Song et al., 2013; Zhao et al., 2018) (Figure 2). Thus, obesity-induced p-s-IRS or p-s-IR may be released to the extracellular microenvironment by EVs, which makes insulin resistance a transmissible pathological condition.
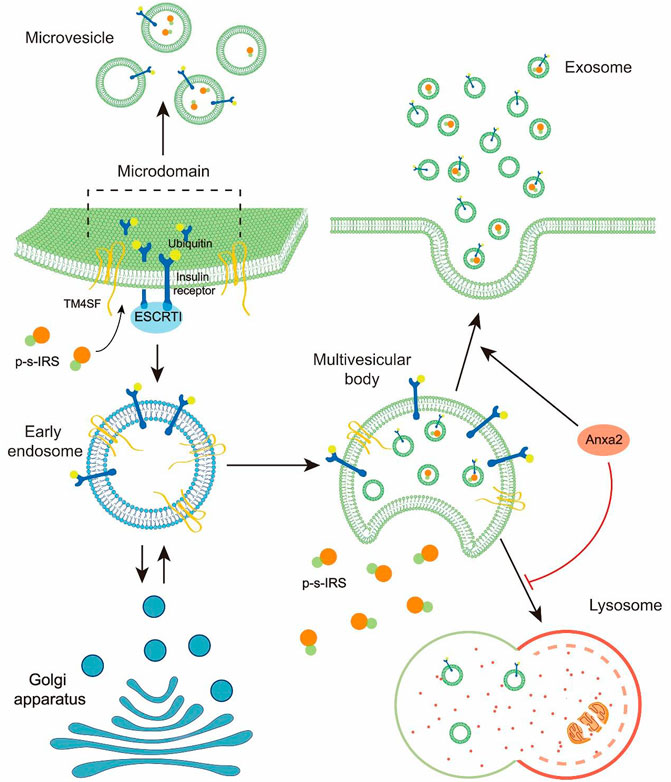
FIGURE 2. Diagram depicts that p-s-IRS is selectively loaded into EVs and released to the extracellular environment.
The transmission of insulin resistance can be prevented by altered EV cargoes induced by exercise and meditation
T2DM is a metabolic disease caused by excessive energy intake-induced obesity and insulin resistance. It is widely accepted that exercise can promote body weight loss by accelerating excessive energy consumption and increasing the insulin sensitivity of cells (Kjøbsted et al., 2017). However, exercise also can accomplish health promotion or disease rehabilitation via EVs (Bertoldi et al., 2018). Multiple tissues release EVs following exercise (D’Souza et al., 2018), and exercise can up-regulate p-y-IRS (Heled et al., 2003; Wrann et al., 2013). Recent studies have demonstrated a significant increase in the amount of EVs during exercise, which restores to pre-exercise level after 4 h. Moreover, EVs induced by exercise tend to be transported to the liver (Whitham et al., 2018; Li et al., 2022). Insulin resistance in the liver is a critical inducement of T2DM (Perry et al., 2014), and pharmacological intervention of glucose metabolism in the liver is an important treatment strategy for T2DM (Lin et al., 2000; Shaw et al., 2005). Hyperinsulinemia is a common symptom of T2DM, leading to decreased insulin signaling in the liver and skeletal muscle by increasing the p-s-IRS level, thereby resulting in insulin resistance (Ueno et al., 2005). Exercise can reduce serum insulin, improve p-y-IRS, and reduce p-s-IRS (Ngo et al., 2002; Heled et al., 2003; Ropelle et al., 2006). Furthermore, exercise-derived exosomes can improve the symptoms of T2DM (Houmard et al., 2004; Safdar et al., 2016). Thus, exercise may block the EV-dependent transmission of insulin resistance and reverse its spread. Similarly, pioglitazone (PIO), a common T2DM drug, can reverse insulin resistance by altering exosome cargo compositions (Kubota et al., 2006; Lopez and Pratley, 2018). The level of p-y-IRS can be improved upon PIO treatment (Hammarstedt et al., 2005), with a similar effect as exercise intervention (Figure 3).
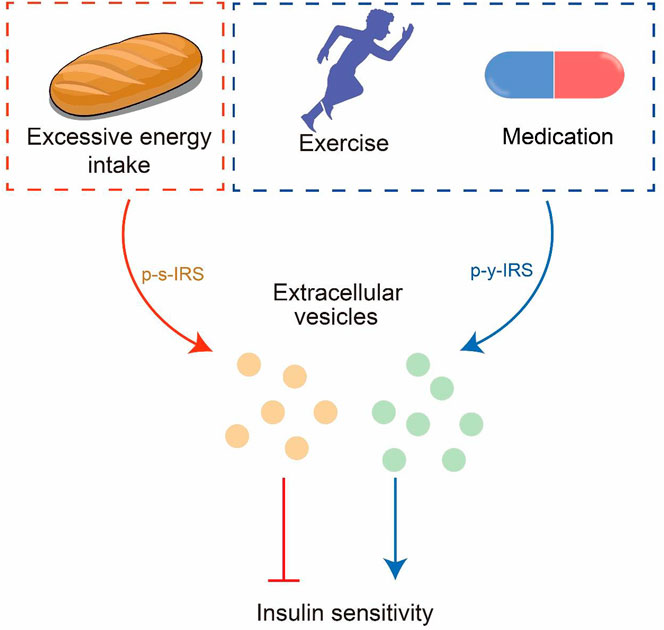
FIGURE 3. EVs are the information carriers between cells. Excessive energy intake will lead to insulin resistance transmitted through EVs; exercise and meditation can reverse insulin resistance.
While there has been increased research on EV-related NCD, it is important to note that different sample types have been used in various studies, as summarized in Table 1. As indicated in Table 1, most studies have used either cells or animal samples. Fewer studies have used clinical samples, highlighting the need for greater efforts to analyze clinical samples in future work.
Conclusion
The current studies on the development of insulin resistance have mainly focused on rescuing insulin resistance rather than suppressing its transmission in the body. Although studies on EVs for regulating the development and progression of insulin resistance have been initiated, the accurate regulatory roles of EVs in the transmission of insulin resistance and underlying mechanisms are still unclear. EVs, the information-exchanging carriers between cells, are involved in multiple pathological signal pathways. Exploring the regulatory roles of EVs in the development and progression of insulin resistance can not only help us understand the mechanisms for blocking the transmission of insulin signaling but also provide us with potentially effective EV-based preventive and therapeutic strategies. However, the functions of EVs depend on their compositions, such as p-s-IRS and p-y-IRS; therefore, exercise or medication interventions may reverse insulin resistance by blocking the transmission of insulin signaling by altering the cargoes of EVs (Figure 3).
Author contributions
LZ designed and supervised the review. BL and WL searched the references. BL, WL, and TL analyzed the references, and BL created the pictures and graphs. LZ and TL revised the final manuscript. All the authors have read and approved the manuscript.
Funding
The work was financially supported by grants from the National Natural Science Foundation of China (Grant Nos 82073532 and 21575058) and the National High Technology Research and Development Program (“863” program) of China (Grant No. 2014AA020904).
Conflict of interest
The authors declare that the research was conducted in the absence of any commercial or financial relationships that could be construed as a potential conflict of interest.
Publisher’s note
All claims expressed in this article are solely those of the authors and do not necessarily represent those of their affiliated organizations, or those of the publisher, the editors, and the reviewers. Any product that may be evaluated in this article, or claim that may be made by its manufacturer, is not guaranteed or endorsed by the publisher.
References
Abbott, S., Dindol, N., Tahrani, A. A., and Piya, M. K. (2018). Binge eating disorder and night eating syndrome in adults with type 2 diabetes: A systematic review. J. Eat. Disord. 6, 36. doi:10.1186/s40337-018-0223-1
Akers, J. C., Gonda, D., Kim, R., Carter, B. S., and Chen, C. C. (2013). Biogenesis of extracellular vesicles (EV): Exosomes, microvesicles, retrovirus-like vesicles, and apoptotic bodies. J. neuro-oncology 113, 1–11. doi:10.1007/s11060-013-1084-8
Allison, K. C., Crow, S. J., Reeves, R. R., West, D. S., Foreyt, J. P., Dilillo, V. G., et al. (2007). Binge eating disorder and night eating syndrome in adults with type 2 diabetes. Obesity 15, 1287–1293. doi:10.1038/oby.2007.150
Anderson, J. W., Kendall, C. W., and Jenkins, D. J. (2003). Importance of weight management in type 2 diabetes: Review with meta-analysis of clinical studies. J. Am. Coll. Nutr. 22, 331–339. doi:10.1080/07315724.2003.10719316
Aswad, H., Forterre, A., Wiklander, O. P., Vial, G., Danty-Berger, E., Jalabert, A., et al. (2014). Exosomes participate in the alteration of muscle homeostasis during lipid-induced insulin resistance in mice. Diabetologia 57, 2155–2164. doi:10.1007/s00125-014-3337-2
Atienzar-Aroca, S., Flores-Bellver, M., Serrano-Heras, G., Martinez-Gil, N., Barcia, J. M., Aparicio, S., et al. (2016). Oxidative stress in retinal pigment epithelium cells increases exosome secretion and promotes angiogenesis in endothelial cells. J. Cell. Mol. Med. 20, 1457–1466. doi:10.1111/jcmm.12834
Beisswenger, P. J. (2012). Glycation and biomarkers of vascular complications of diabetes. Amino acids 42, 1171–1183. doi:10.1007/s00726-010-0784-z
Bensellam, M., Laybutt, D. R., and Jonas, J.-C. (2012). The molecular mechanisms of pancreatic β-cell glucotoxicity: Recent findings and future research directions. Mol. Cell. Endocrinol. 364, 1–27. doi:10.1016/j.mce.2012.08.003
Bertoldi, K., Cechinel, L. R., Schallenberger, B., Corssac, G. B., Davies, S., Guerreiro, I. C. K., et al. (2018). Circulating extracellular vesicles in the aging process: Impact of aerobic exercise. Mol. Cell Biochem. 440, 115–125. doi:10.1007/s11010-017-3160-4
Bin, L., Yun, X., Courtney, V., Fang-hua, Q., Ming-zhe, Z., Yong-dong, L., et al. (2012). Altered protein expression in gestational diabetes mellitus placentas provides insight into insulin resistance and coagulation/fibrinolysis pathways. PloS one 7, e44701. doi:10.1371/journal.pone.0044701
Buschow, S. I., Liefhebber, J. M., Wubbolts, R., and Stoorvogel, W. (2005). Exosomes contain ubiquitinated proteins. Blood Cells, Mol. Dis. 35, 398–403. doi:10.1016/j.bcmd.2005.08.005
Choi, Y., Kwon, Y., Kim, D.-K., Jeon, J., Jang, S. C., Wang, T., et al. (2015). Gut microbe-derived extracellular vesicles induce insulin resistance, thereby impairing glucose metabolism in skeletal muscle. Sci. Rep. 5, 15878. doi:10.1038/srep15878
Copps, K. D., and White, M. F. (2012). Regulation of insulin sensitivity by serine/threonine phosphorylation of insulin receptor substrate proteins IRS1 and IRS2. Diabetologia 55, 2565–2582. doi:10.1007/s00125-012-2644-8
Crewe, C., Joffin, N., Rutkowski, J. M., Kim, M., Zhang, F., Towler, D. A., et al. (2018). An endothelial-to-adipocyte extracellular vesicle Axis governed by metabolic state. Cell 175, 695–708. doi:10.1016/j.cell.2018.09.005
D'Apolito, M., Du, X., Zong, H., Catucci, A., Maiuri, L., Trivisano, T., et al. (2010). Urea-induced ROS generation causes insulin resistance in mice with chronic renal failure. J. Clin. investigation 120, 203–213. doi:10.1172/JCI37672
Davies, B. A., Lee, J. R., Oestreich, A. J., and Katzmann, D. J. (2009). Membrane protein targeting to the MVB/lysosome. Chem. Rev. 109, 1575–1586. doi:10.1021/cr800473s
de Rivero Vaccari, J. P., Brand, F., Adamczak, S., Lee, S. W., Perez-Barcena, J., Wang, M. Y., et al. (2016). Exosome-mediated inflammasome signaling after central nervous system injury. J. Neurochem. 136 (1), 39–48. doi:10.1111/jnc.13036
DeClercq, V., d'Eon, B., and McLeod, R. S. (2015). Fatty acids increase adiponectin secretion through both classical and exosome pathways. Biochimica Biophysica Acta (BBA)-Molecular Cell Biol. Lipids 1851, 1123–1133. doi:10.1016/j.bbalip.2015.04.005
Deng, Z. B., Poliakov, A., Hardy, R. W., Clements, R., Liu, C., Liu, Y., et al. (2009). Adipose tissue exosome-like vesicles mediate activation of macrophage-induced insulin resistance. Diabetes 58, 2498–2505. doi:10.2337/db09-0216
Dibaba, D. T., Xun, P., Song, Y., Rosanoff, A., Shechter, M., and He, K. (2017). The effect of magnesium supplementation on blood pressure in individuals with insulin resistance, prediabetes, or noncommunicable chronic diseases: A meta-analysis of randomized controlled trials. Am. J. Clin. Nutr. 106, 921–929. doi:10.3945/ajcn.117.155291
D’Souza, R. F., Woodhead, J. S., Zeng, N., Blenkiron, C., Merry, T. L., Cameron-Smith, D., et al. (2018). Circulatory exosomal miRNA following intense exercise is unrelated to muscle and plasma miRNA abundances. Am. J. Physiology-Endocrinology Metabolism 315, E723–E733. doi:10.1152/ajpendo.00138.2018
Eguchi, A., Lazic, M., Armando, A. M., Phillips, S. A., Katebian, R., Maraka, S., et al. (2016). Circulating adipocyte-derived extracellular vesicles are novel markers of metabolic stress. J. Mol. Med. 94, 1241–1253. doi:10.1007/s00109-016-1446-8
Eze, M. O. (1992). Membrane fluidity, reactive oxygen species, and cell-mediated immunity: Implications in nutrition and disease. Med. hypotheses 37, 220–224. doi:10.1016/0306-9877(92)90191-e
Fan, B., Chopp, M., Zhang, Z. G., and Liu, X. S. (2021). Treatment of diabetic peripheral neuropathy with engineered mesenchymal stromal cell-derived exosomes enriched with microRNA-146a provide amplified therapeutic efficacy. Exp. Neurol. 341, 113694. doi:10.1016/j.expneurol.2021.113694
Fan, B., Li, C., Szalad, A., Wang, L., Pan, W., Zhang, R., et al. (2020). Mesenchymal stromal cell-derived exosomes ameliorate peripheral neuropathy in a mouse model of diabetes. Diabetologia 63, 431–443. doi:10.1007/s00125-019-05043-0
Feldman, E. L., Callaghan, B. C., Pop-Busui, R., Zochodne, D. W., Wright, D. E., Bennett, D. L., et al. (2019). Diabetic neuropathy. Nat. Rev. Dis. Prim. 5, 41. doi:10.1038/s41572-019-0092-1
Gao, Z., Hwang, D., Bataille, F., Lefevre, M., York, D., Quon, M. J., et al. (2002). Serine phosphorylation of insulin receptor substrate 1 by inhibitor κB kinase complex. J. Biol. Chem. 277, 48115–48121. doi:10.1074/jbc.M209459200
Gual, P., Le Marchand-Brustel, Y., and Tanti, J.-F. (2005). Positive and negative regulation of insulin signaling through IRS-1 phosphorylation. Biochimie 87, 99–109. doi:10.1016/j.biochi.2004.10.019
Hammarstedt, A., Sopasakis, V. R., Gogg, S., Jansson, P.-A., and Smith, U. (2005). Improved insulin sensitivity and adipose tissue dysregulation after short-term treatment with pioglitazone in non-diabetic, insulin-resistant subjects. Diabetologia 48, 96–104. doi:10.1007/s00125-004-1612-3
Han, C. Y. (2016). Roles of reactive oxygen species on insulin resistance in adipose tissue. Diabetes & metabolism J. 40, 272–279. doi:10.4093/dmj.2016.40.4.272
Han, C. Y., Umemoto, T., Omer, M., Den Hartigh, L. J., Chiba, T., LeBoeuf, R., et al. (2012). NADPH oxidase-derived reactive oxygen species increases expression of monocyte chemotactic factor genes in cultured adipocytes. J. Biol. Chem. 287, 10379–10393. doi:10.1074/jbc.M111.304998
Hedlund, M., Nagaeva, O., Kargl, D., Baranov, V., and Mincheva-Nilsson, L. (2011). Thermal- and oxidative stress causes enhanced release of NKG2D ligand-bearing immunosuppressive exosomes in leukemia/lymphoma T and B cells. PloS one 6, e16899. doi:10.1371/journal.pone.0016899
Heled, Y., Shapiro, Y., Shani, Y., Moran, D. S., Langzam, L., Braiman, L., et al. (2003). Physical exercise enhances protein kinase C δ activity and insulin receptor tyrosine phosphorylation in diabetes-prone Psammomys obesus. Metabolism Clin. Exp. 52, 1028–1033. doi:10.1016/s0026-0495(03)00154-9
Hotamisligil, G. (1999). Mechanisms of TNF-α-induced insulin resistance. Exp. Clin. Endocrinol. diabetes 107, 119–125. doi:10.1055/s-0029-1212086
Houmard, J. A., Tanner, C. J., Slentz, C. A., Duscha, B. D., McCartney, J. S., and Kraus, W. E. (2004). Effect of the volume and intensity of exercise training on insulin sensitivity. J. Appl. physiology 96, 101–106. doi:10.1152/japplphysiol.00707.2003
Houstis, N., Rosen, E. D., and Lander, E. S. (2006). Reactive oxygen species have a causal role in multiple forms of insulin resistance. Nature 440, 944–948. doi:10.1038/nature04634
Hurley, J. H. (2008). ESCRT complexes and the biogenesis of multivesicular bodies. Curr. Opin. Cell Biol. 20, 4–11. doi:10.1016/j.ceb.2007.12.002
Jeppesen, D. K., Fenix, A. M., Franklin, J. L., Higginbotham, J. N., Zhang, Q., Zimmerman, L. J., et al. (2019). Reassessment of exosome composition. Cell 177, 428–445. doi:10.1016/j.cell.2019.02.029
Jia, L., Chopp, M., Wang, L., Lu, X., Szalad, A., and Zhang, Z. G. (2018). Exosomes derived from high-glucose-stimulated Schwann cells promote development of diabetic peripheral neuropathy. FASEB J. 32, fj201800597R. doi:10.1096/fj.201800597R
Jiang, Z., Lu, W., Zeng, Q., Li, D., Ding, L., and Wu, J. (2018). High glucose-induced excessive reactive oxygen species promote apoptosis through mitochondrial damage in rat cartilage endplate cells. J. Orthop. Research® 36, 2476–2483. doi:10.1002/jor.24016
Kalra, H., Drummen, G. P., and Mathivanan, S. (2016). Focus on extracellular vesicles: Introducing the next small big thing. Int. J. Mol. Sci. 17, 170. doi:10.3390/ijms17020170
Kapogiannis, D., Boxer, A., Schwartz, J. B., Abner, E. L., Biragyn, A., Masharani, U., et al. (2015). Dysfunctionally phosphorylated type 1 insulin receptor substrate in neural-derived blood exosomes of preclinical Alzheimer's disease. FASEB J. official Publ. Fed. Am. Soc. Exp. Biol. 29, 589–596. doi:10.1096/fj.14-262048
Keryer-Bibens, C., Pioche-Durieu, C., Villemant, C., Souquère, S., Nishi, N., Hirashima, M., et al. (2006). Exosomes released by EBV-infected nasopharyngeal carcinoma cells convey the viral latent membrane protein 1 and the immunomodulatory protein galectin 9. BMC cancer 6, 283. doi:10.1186/1471-2407-6-283
Kim, S. J., DeStefano, M. A., Oh, W. J., Wu, C. C., Vega-Cotto, N. M., Finlan, M., et al. (2012). mTOR complex 2 regulates proper turnover of insulin receptor substrate-1 via the ubiquitin ligase subunit Fbw8. Mol. Cell 48, 875–887. doi:10.1016/j.molcel.2012.09.029
Kjøbsted, R., Munk-Hansen, N., Birk, J. B., Foretz, M., Viollet, B., Björnholm, M., et al. (2017). Enhanced muscle insulin sensitivity after contraction/exercise is mediated by AMPK. Diabetes 66, 598–612. doi:10.2337/db16-0530
Kowal, J., Arras, G., Colombo, M., Jouve, M., Morath, J. P., Primdal-Bengtson, B., et al. (2016). Proteomic comparison defines novel markers to characterize heterogeneous populations of extracellular vesicle subtypes. Proc. Natl. Acad. Sci. 113, E968–E977. doi:10.1073/pnas.1521230113
Kowal, J., Tkach, M., and Théry, C. (2014). Biogenesis and secretion of exosomes. Curr. Opin. Cell Biol. 29, 116–125. doi:10.1016/j.ceb.2014.05.004
Kranendonk, M. E., Visseren, F. L., van Balkom, B. W., Nolte-'t Hoen, E. N., van Herwaarden, J. A., de Jager, W., et al. (2014). Human adipocyte extracellular vesicles in reciprocal signaling between adipocytes and macrophages. Obesity 22, 1296–1308. doi:10.1002/oby.20679
Kubota, N., Terauchi, Y., Kubota, T., Kumagai, H., Itoh, S., Satoh, H., et al. (2006). Pioglitazone ameliorates insulin resistance and diabetes by both adiponectin-dependent and-independent pathways. J. Biol. Chem. 281, 8748–8755. doi:10.1074/jbc.M505649200
Leitner, D. R., Frühbeck, G., Yumuk, V., Schindler, K., Micic, D., Woodward, E., et al. (2017). Obesity and type 2 diabetes: Two diseases with a need for combined treatment strategies-EASO can lead the way. Obes. facts 10, 483–492. doi:10.1159/000480525
Li, T., Han, X., Chen, S., Wang, B., Teng, Y., Cheng, W., et al. (2022). Effects of exercise on extracellular vesicles in patients with metabolic dysfunction: A systematic review. J. Cardiovasc Transl. Res. doi:10.1007/s12265-022-10282-5
Lin, H. Z., Yang, S. Q., Chuckaree, C., Kuhajda, F., Ronnet, G., and Diehl, A. M. (2000). Metformin reverses fatty liver disease in obese, leptin-deficient mice. Nat. Med. 6, 998–1003. doi:10.1038/79697
Matsuoka, T., Kajimoto, Y., Watada, H., Kaneto, H., Kishimoto, M., Umayahara, Y., et al. (1997). Glycation-dependent, reactive oxygen species-mediated suppression of the insulin gene promoter activity in HIT cells. J. Clin. investigation 99, 144–150. doi:10.1172/JCI119126
Meldolesi, J. (2018). Exosomes and ectosomes in intercellular communication. Curr. Biol. CB 28, R435–R444. doi:10.1016/j.cub.2018.01.059
Murphy, M. P. (2008). How mitochondria produce reactive oxygen species. Biochem. J. 417, 1–13. doi:10.1042/BJ20081386
Ngo, T. H., Barnard, R. J., Tymchuk, C. N., Cohen, P., and Aronson, W. J. (2002). Effect of diet and exercise on serum insulin, IGF-I, and IGFBP-1 levels and growth of LNCaP cells in vitro (United States). Cancer Causes Control 13, 929–935. doi:10.1023/a:1021911517010
Ouedraogo, R., Wu, X., Xu, S.-Q., Fuchsel, L., Motoshima, H., Mahadev, K., et al. (2006). Adiponectin suppression of high-glucose–induced reactive oxygen species in vascular endothelial cells: Evidence for involvement of a cAMP signaling pathway. Diabetes 55, 1840–1846. doi:10.2337/db05-1174
Parekh, S., and Anania, F. A. (2007). Abnormal lipid and glucose metabolism in obesity: Implications for nonalcoholic fatty liver disease. Gastroenterology 132, 2191–2207. doi:10.1053/j.gastro.2007.03.055
Perry, R. J., Samuel, V. T., Petersen, K. F., and Shulman, G. I. (2014). The role of hepatic lipids in hepatic insulin resistance and type 2 diabetes. Nature 510, 84–91. doi:10.1038/nature13478
Phaniendra, A., Jestadi, D. B., and Periyasamy, L. (2015). Free radicals: Properties, sources, targets, and their implication in various diseases. Indian J. Clin. Biochem. 30, 11–26. doi:10.1007/s12291-014-0446-0
Qu, L., Ding, J., Chen, C., Wu, Z. J., Liu, B., Gao, Y., et al. (2016). Exosome-transmitted lncARSR promotes sunitinib resistance in renal cancer by acting as a competing endogenous RNA. Cancer Cell 29, 653–668. doi:10.1016/j.ccell.2016.03.004
Robertson, R. P., Harmon, J., Tran, P. O., Tanaka, Y., and Takahashi, H. (2003). Glucose toxicity in beta-cells: Type 2 diabetes, good radicals gone bad, and the glutathione connection. Diabetes 52, 581–587. doi:10.2337/diabetes.52.3.581
Ropelle, E. R., Pauli, J. R., Prada, P. O., De Souza, C. T., Picardi, P. K., Faria, M. C., et al. (2006). Reversal of diet-induced insulin resistance with a single bout of exercise in the rat: The role of PTP1B and IRS-1 serine phosphorylation. J. physiology 577, 997–1007. doi:10.1113/jphysiol.2006.120006
Rotter, V., Nagaev, I., and Smith, U. (2003). Interleukin-6 (IL-6) induces insulin resistance in 3T3-L1 adipocytes and is, like IL-8 and tumor necrosis factor-α, overexpressed in human fat cells from insulin-resistant subjects. J. Biol. Chem. 278, 45777–45784. doi:10.1074/jbc.M301977200
Safdar, A., Saleem, A., and Tarnopolsky, M. A. (2016). The potential of endurance exercise-derived exosomes to treat metabolic diseases. Nat. Rev. Endocrinol. 12, 504–517. doi:10.1038/nrendo.2016.76
Schmelzle, K., Kane, S., Gridley, S., Lienhard, G. E., and White, F. M. (2006). Temporal dynamics of tyrosine phosphorylation in insulin signaling. Diabetes 55, 2171–2179. doi:10.2337/db06-0148
Shaw, R. J., Lamia, K. A., Vasquez, D., Koo, S.-H., Bardeesy, N., DePinho, R. A., et al. (2005). The kinase LKB1 mediates glucose homeostasis in liver and therapeutic effects of metformin. Science 310, 1642–1646. doi:10.1126/science.1120781
Song, R., Peng, W., Zhang, Y., Lv, F., Wu, H. K., Guo, J., et al. (2013). Central role of E3 ubiquitin ligase MG53 in insulin resistance and metabolic disorders. Nature 494, 375–379. doi:10.1038/nature11834
Spencer, N. Y., and Engelhardt, J. F. (2014). The basic biology of redoxosomes in cytokine-mediated signal transduction and implications for disease-specific therapies. Biochemistry 53, 1551–1564. doi:10.1021/bi401719r
Staels, B. (2017). Cardiovascular protection by sodium glucose cotransporter 2 inhibitors: Potential mechanisms. Am. J. Cardiol. 120, S28–S36. doi:10.1016/j.amjcard.2017.05.013
Tanti, J.-F., and Jager, J. (2009). Cellular mechanisms of insulin resistance: Role of stress-regulated serine kinases and insulin receptor substrates (IRS) serine phosphorylation. Curr. Opin. Pharmacol. 9, 753–762. doi:10.1016/j.coph.2009.07.004
Tao, C., Sifuentes, A., and Holland, W. L. (2014). Regulation of glucose and lipid homeostasis by adiponectin: Effects on hepatocytes, pancreatic β cells and adipocytes. Best Pract. Res. Clin. Endocrinol. Metabolism 28, 43–58. doi:10.1016/j.beem.2013.11.003
Théry, C., Witwer, K. W., Aikawa, E., Alcaraz, M. J., Anderson, J. D., Andriantsitohaina, R., et al. (2018). Minimal information for studies of extracellular vesicles 2018 (MISEV2018): A position statement of the international society for extracellular vesicles and update of the MISEV2014 guidelines. J. Extracell. vesicles 7, 1535750. doi:10.1080/20013078.2018.1535750
Tonks, K., Ng, Y., Miller, S., Coster, A., Samocha-Bonet, D., Iseli, T., et al. (2013). Impaired Akt phosphorylation in insulin-resistant human muscle is accompanied by selective and heterogeneous downstream defects. Diabetologia 56, 875–885. doi:10.1007/s00125-012-2811-y
Turrens, J. F. (2003). Mitochondrial formation of reactive oxygen species. J. physiology 552, 335–344. doi:10.1113/jphysiol.2003.049478
Ueno, M., Carvalheira, J., Tambascia, R., Bezerra, R., Amaral, M., Carneiro, E., et al. (2005). Regulation of insulin signalling by hyperinsulinaemia: Role of IRS-1/2 serine phosphorylation and the mTOR/p70 S6K pathway. Diabetologia 48, 506–518. doi:10.1007/s00125-004-1662-6
Uysal, K. T., Wiesbrock, S. M., Marino, M. W., and Hotamisligil, G. S. (1997). Protection from obesity-induced insulin resistance in mice lacking TNF-α function. Nature 389, 610–614. doi:10.1038/39335
Valapala, M., and Vishwanatha, J. K. (2011). Lipid raft endocytosis and exosomal transport facilitate extracellular trafficking of Annexin A2. J. Biol. Chem. 286, 30911–30925. doi:10.1074/jbc.M111.271155
Van den Berghe, G. (2004). How does blood glucose control with insulin save lives in intensive care? J. Clin. investigation 114, 1187–1195. doi:10.1172/JCI23506
Van Niel, G., D'Angelo, G., and Raposo, G. (2018). Shedding light on the cell biology of extracellular vesicles. Nat. Rev. Mol. Cell Biol. 19, 213–228. doi:10.1038/nrm.2017.125
Veech, R. L. (2004). The therapeutic implications of ketone bodies: The effects of ketone bodies in pathological conditions: Ketosis, ketogenic diet, redox states, insulin resistance, and mitochondrial metabolism. Prostagl. Leukot. Essent. Fat. acids 70, 309–319. doi:10.1016/j.plefa.2003.09.007
Wang, J., Bonacquisti, E. E., Brown, A. D., and Nguyen, J. (2020). Boosting the biogenesis and secretion of mesenchymal stem cell-derived exosomes. Cells 9, 660. doi:10.3390/cells9030660
Wang, L., Zhang, B., Zheng, W., Kang, M., Chen, Q., Qin, W., et al. (2017). Exosomes derived from pancreatic cancer cells induce insulin resistance in C2C12 myotube cells through the PI3K/Akt/FoxO1 pathway. Sci. Rep. 7, 5384. doi:10.1038/s41598-017-05541-4
Watanabe, H., Kobayashi, A., Yamamoto, T., Suzuki, S., Hayashi, H., and Yamazaki, N. (1990). Alterations of human erythrocyte membrane fluidity by oxygen-derived free radicals and calcium. Free Radic. Biol. Med. 8, 507–514. doi:10.1016/0891-5849(90)90150-h
Weyer, C., Bogardus, C., Mott, D. M., and Pratley, R. E. (1999). The natural history of insulin secretory dysfunction and insulin resistance in the pathogenesis of type 2 diabetes mellitus. J. Clin. investigation 104, 787–794. doi:10.1172/JCI7231
Whitham, M., Parker, B. L., Friedrichsen, M., Hingst, J. R., Hjorth, M., Hughes, W. E., et al. (2018). Extracellular vesicles provide a means for tissue crosstalk during exercise. Cell metab. 27, 237e234–251. doi:10.1016/j.cmet.2017.12.001
Wrann, C. D., White, J. P., Salogiannnis, J., Laznik-Bogoslavski, D., Wu, J., Ma, D., et al. (2013). Exercise induces hippocampal BDNF through a PGC-1α/FNDC5 pathway. Cell metab. 18, 649–659. doi:10.1016/j.cmet.2013.09.008
Ye, J. (2013). Mechanisms of insulin resistance in obesity. Front. Med. 7, 14–24. doi:10.1007/s11684-013-0262-6
Ying, W., Riopel, M., Bandyopadhyay, G., Dong, Y., Birmingham, A., Seo, J. B., et al. (2017). Adipose tissue macrophage-derived exosomal miRNAs can modulate in vivo and in vitro insulin sensitivity. Cell 171, 372e312–384. doi:10.1016/j.cell.2017.08.035
Yoneyama, Y., Inamitsu, T., Chida, K., Iemura, S.-I., Natsume, T., Maeda, T., et al. (2018). Serine phosphorylation by mTORC1 promotes IRS-1 degradation through SCFβ-TRCP E3 ubiquitin ligase. iScience 5, 1–18. doi:10.1016/j.isci.2018.06.006
Zhang, Y., Yu, M., and Tian, W. (2016). Physiological and pathological impact of exosomes of adipose tissue. Cell Prolif. 49, 3–13. doi:10.1111/cpr.12233
Zhao, Y., Gao, L., Xu, L., Tong, R., Lin, N., Su, Y., et al. (2018). Ubiquitin-specific protease 4 is an endogenous negative regulator of metabolic dysfunctions in nonalcoholic fatty liver disease in mice. Hepatology 68, 897–917. doi:10.1002/hep.29889
Zhu, Q., Zhu, R., and Jin, J. (2016). Neutral ceramidase-enriched exosomes prevent palmitic acid-induced insulin resistance in H4IIEC3 hepatocytes. FEBS open bio 6, 1078–1084. doi:10.1002/2211-5463.12125
Zhu, S., Sun, F., Li, W., Cao, Y., Wang, C., Wang, Y., et al. (2011). Apelin stimulates glucose uptake through the PI3K/Akt pathway and improves insulin resistance in 3T3-L1 adipocytes. Mol. Cell. Biochem. 353, 305–313. doi:10.1007/s11010-011-0799-0
Keywords: insulin resistance, extracellular vesicle, insulin receptor substrate, T2DM, diabetes
Citation: Li B, Li W, Liu T and Zha L (2023) Extracellular vesicles regulate the transmission of insulin resistance and redefine noncommunicable diseases. Front. Mol. Biosci. 9:1024786. doi: 10.3389/fmolb.2022.1024786
Received: 22 August 2022; Accepted: 19 December 2022;
Published: 09 January 2023.
Edited by:
Ramin M. Hakami, George Mason University, United StatesReviewed by:
Fatah Kashanchi, George Mason University, United StatesCopyright © 2023 Li, Li, Liu and Zha. This is an open-access article distributed under the terms of the Creative Commons Attribution License (CC BY). The use, distribution or reproduction in other forums is permitted, provided the original author(s) and the copyright owner(s) are credited and that the original publication in this journal is cited, in accordance with accepted academic practice. No use, distribution or reproduction is permitted which does not comply with these terms.
*Correspondence: Tiancai Liu, bGl1dGNAc211LmVkdS5jbg==
†These authors have contributed equally to this work