- 1Kuang Yaming Honors School and Institute for Brain Sciences, Nanjing University, Nanjing, China
- 2State Key Laboratory of Nonlinear Mechanics and Beijing Key Laboratory of Engineered Construction and Mechanobiology, Institute of Mechanics, Chinese Academy of Sciences, Beijing, China
- 3Institute of Physics, Polish Academy of Sciences, Warsaw, Poland
- 4Key Laboratory of Biomechanics and Mechanobiology (Beihang University), Ministry of Education Beijing Advanced Innovation Center for Biomedical Engineering School of Biological Science and Medical Engineering, Beihang University, Beijing, China
- 5School of Engineering Science, University of Chinese Academy of Sciences, Beijing, China
Cell adhesion involved in biological processes such as cell migration, immune responses, and cancer metastasis, is mediated by the specific binding of receptor and ligand proteins. Some of these proteins exhibit affinity for nanoscale lipid clusters in cell membranes. A key question is how these nanoscale lipid clusters influence and react to the receptor-ligand binding during cell adhesion. In this article, we review recent computational studies that shed new light on the interplay of the receptor-ligand binding and the formation of lipid domains in adhering membranes. These studies indicate that the receptor-ligand binding promotes coalescence of lipid clusters into mesoscale domains, which, in turn, enhances both the affinity and cooperativity of the receptor-ligand binding in cell-cell adhesion with mobile ligands. In contrast, in the case of cell-extracellular matrix adhesion with immobile ligands, the receptor-ligand binding and the lipid cluster coalescence can be correlated or anti-correlated, depending strongly on the ligand distribution. These findings deepen our understanding of correlations between cell adhesion and membrane heterogeneities.
Introduction
The processes of cell-cell adhesion and cell-extracellular matrix adhesion are fundamental for numerous biological functions of cells, including immune responses, cell locomotion, tissue formation and cancer metastasis (Abraham and Miao, 2015; Micalizzi et al., 2021; Singh et al., 2021). The adhesion is mediated by the specific binding of receptor and ligand proteins that are anchored in the two apposing surfaces (Rozycki and Weikl, 2021). In cell-cell adhesion, both receptors and ligands are mobile. Whereas, in cell-extracellular matrix adhesion, the ligand molecules in matrix are immobile (Zhu et al., 2007; Sackmann and Smith, 2014). A key property quantifying the receptor-ligand binding is their binding affinity
The receptor-ligand binding is often studied using model membranes, in which the membrane lipids exhibit uniform distribution. However, cumulative evidence suggests that cell membranes are heterogeneous and contain nanoscale domains, or lipid clusters, enriched in saturated phospholipids and cholesterol (Michel and Bakovic, 2007; Lingwood and Simons, 2010; Mollinedo and Gajate, 2015; Sezgin et al., 2017; Levental et al., 2020). These liquid-ordered-type nanodomains, often termed as lipid rafts, exhibit larger rigidity and smaller fluidity than the surrounding liquid-disordered-type membrane matrix (Pralle et al., 2000; Pierce, 2002; Fan et al., 2010; Simons and Sampaio, 2011). One of the most fascinating properties of lipid rafts is their ability to selectively recruit or exclude specific proteins to variable extents (i.e. raft affinity for membrane proteins), inducing a heterogeneous protein distribution and contributing to protein sorting (Simons and Toomre, 2000). The length, palmitoylation, and surface area of protein transmembrane domains have been identified as determinants of protein affinity for raft domains (Lorent et al., 2017). Many studies have demonstrated the preferred localization of diverse adhesion and signaling proteins (e.g., CD44, T cell receptor (TCR), and peptide major histocompatibility complex (pMHC)) within lipid rafts (Murai et al., 2013; Stone et al., 2017). Multiple separate lipid rafts can assemble and merge into large-scale domains by the virtue of, e.g., protein-lipid interactions, protein-protein interactions, and actin cytoskeleton rearrangements (Douglass and Vale, 2005; Viola and Gupta, 2007; Destainville et al., 2018), thereby functioning as platforms that facilitate specific protein-protein interactions within one membrane (so-called cis-interactions) as well as signal transduction cascades (Simons and Toomre, 2000; Mollinedo and Gajate, 2015). Heterogeneities in cell membranes are thus thought to be crucial for cell biological functions and have been shown to be closely related to cancer, neurodegenerative and cardiovascular diseases (Simons and Ehehalt, 2002; Michel and Bakovic, 2007). Targeting lipid rafts and membrane heterogeneities has provided novel strategies and routes for disease therapies (Murai, 2015; Yang et al., 2018; Sorice et al., 2021).
How does the receptor-ligand binding affect the distribution of lipid rafts and the heterogeneity of the cell membranes? And how do the properties of lipid rafts influence the receptor-ligand binding affinity and cooperativity? Answering these questions will help us to understand the molecular mechanisms underlying such biological processes as cell adhesion and signaling, and can contribute to drug design and biomedical applications. Experimental studies on cell-cell adhesion and cell-extracellular matrix adhesion have led to contradictory conclusions on the interplay of the receptor-ligand binding and the lipid domain formation during cell adhesion (see below for more details) (Huang et al., 2010; Zhao et al., 2013; Anderson and Roche, 2015; Evani and Ramasubramanian, 2016; Son et al., 2017). Here, we review recent theoretical and simulation results that provide new insights into relationships between the receptor-ligand binding and the lipid distribution heterogeneities in the adhering membranes. These results indicate significant differences in the interplay of the receptor-ligand binding and the lipid domain formation during the cell-cell adhesion and the cell-extracellular matrix adhesions. The differences are determined mainly by the mobility and distribution of the ligand molecules. These results together not only deepen our understanding of the mechanisms underlying the adhesion-induced redistribution of lipid components in cell membranes but also help to clarify the contradictory experimental observations reported for different adhesion systems.
Cell-cell adhesion
In vitro experiments indicate that the adhesion of T cells to antigen presenting cells results in coalescence of lipid rafts and clustering of raft-associated MHC class II molecules at the immunological synapse, which enhances the T cell signaling and immune response mediated by TCR-pMHC interactions (Anderson et al., 2000; Anderson and Roche, 2015). Disrupting the lipid raft integrity and signaling protein clustering by treatment with cholesterol depletion agents [e.g., methyl-β-cyclodextrin (MβCD)] has been shown to reduce the TCR-pMHC binding affinity (Huang et al., 2010). Similarly, biomimetic experiments show that the adhesion of giant unilamellar vesicles (GUV) to supported lipid bilayer (SLB) stabilizes the membrane heterogeneity in both synthesized and isolated GUVs, and facilitates the protein accumulation within the adhered region. Disrupting the clusters of rafts and proteins in vesicles with MβCD weakens the stable adhesion mediated by the streptavidin-biotin interaction (Zhao et al., 2013). These experimental results indicate that receptor-ligand binding and lipid distribution heterogeneity positively affect each other.
To further elucidate the mechanisms underlying the interplay between the receptor-ligand binding and the lipid cluster coalescence during the adhesion of cell membranes, mean-field theories and Monte Carlo simulation models have been developed (Li et al., 2020). In these statistical-mechanical models, the two apposing membranes are discretized into small patches that undergo transverse movements to mimic thermal fluctuations of the adhering membranes (Li et al., 2018b). The receptors, ligands, and lipid rafts diffuse in the membranes through hopping processes. The receptors and ligands anchored in the apposing membranes can bind together to form receptor-ligand complexes. The processes of binding and unbinding of the receptors and ligands are determined by the local distance between the binding sites. Further, to describe the affinity of the adhesion proteins for the lipid rafts, an energy of coupling between the proteins and rafts is introduced. In addition, the pairs of lipid rafts at nearest-neighbor membrane patches are subject to cis-attractive interactions (as quantified by contact energy
In the absence of the receptors and ligands, the phase behavior of the model system is given by the exact solution of the Ising model on the two-dimensional square lattice. For small values of inter-raft contact energy
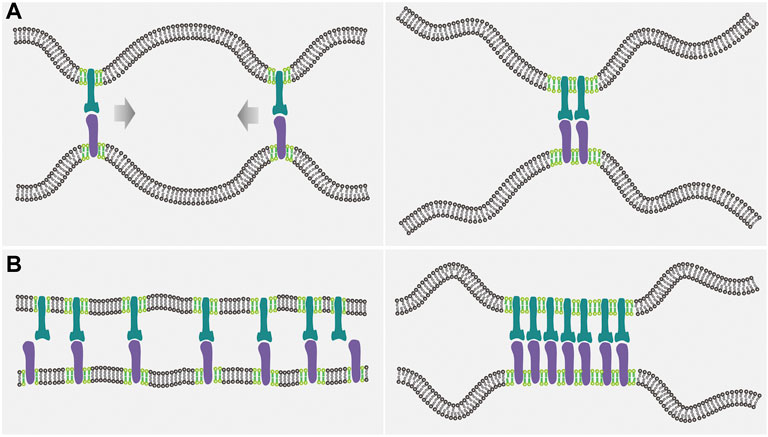
FIGURE 1. Illustration of the interplay between the receptor-ligand binding and the lipid domain formation in the case of mobile receptors and ligands. (A) Since the flexible membranes can adopt more configurations when the receptor-ligand complexes are close together (left panel) than far apart (right panel), thermal undulations of the membranes induce an effective, entropic attraction between the receptor-ligand complexes, which in turn promotes the coalescence of lipid rafts (light green) by means of the protein-raft association. (B) Raft coalescence leads to aggregation of the raft-associated receptors (dark green) and ligands (purple), and therefore decreases the configurational entropy loss of the flexible membranes upon the receptor-ligand binding, enhancing the receptor-ligand binding affinity and cooperativity.
The mesoscopic model for membrane adhesion has been used also to explore the influence of lipid raft properties on the receptor-ligand binding. It has been shown, in particular, that the binding affinity of the receptors and ligands that preferentially associate with raft domains increases with the enhancement of raft coalescence as achieved by increasing the raft-raft contact energy (Li et al., 2021c), which is in agreement with the experimental observations (Huang et al., 2010). Importantly, a dramatic increase in the binding affinity has been found to coincide with the spontaneous separation of membrane components, regardless of the particular values of system parameters. Therefore, the receptor-ligand binding affinity can serve as a general sign for the transition from a homogeneous membrane state to a phase-separated state in the cell-cell adhesion system. The dependence of the receptor-ligand binding cooperativity on the coalescence propensity of lipid rafts can be quantified in the Hill plot, wherein the Hill coefficient
In the case of homogeneous and flexible membranes, thermal fluctuations of the local intermembrane separation act to adversely affect the receptor-ligand binding (Hu et al., 2013; An et al., 2022). On the contrary, in the case of inhomogeneous membranes containing lipid rafts, thermal fluctuations of the intermembrane separation can actually function as a positive regulator of the intermembrane receptor-ligand binding when the receptor and ligand proteins associate with lipid rafts (Li et al., 2017). The influence of raft domain formation on the intermembrane receptor-ligand binding has been shown to be sensitive to such characteristics of lipid rafts as their area fraction, bending rigidity, and affinity for association with the adhesion proteins. For example, the contrast in the bending rigidity between lipid rafts and the non-raft membrane matrix contributes significantly to the receptor-ligand binding affinity and cooperativity by increasing the raft coalescence propensity and by suppressing the local membrane fluctuations within raft-type domains. Taken together, all these theoretical and simulation results are consistent with experimental observations, showing that, in cell-cell adhesion system, receptor-ligand binding and lipid cluster coalescence are mutually beneficial. This interplay can be additionally altered by ligand-ligand cis-attraction or cis-repulsion, which adds to the effective, fluctuation-induced attraction between the receptor-ligand complexes (Li et al., 2021d).
Cell-extracellular matrix adhesion
For the adhesion of cells to extracellular matrix with immobile ligands, experimental studies of cell-substrate adhesion mimicking cell-extracellular matrix adhesion have led to contradictory conclusions regarding the relationship between the receptor-ligand binding and the lipid domain formation (Mitchell et al., 2009; Norman et al., 2010; Wang et al., 2010; Evani and Ramasubramanian, 2016). Some experimental results show that the adhesion of a cell to a flat substrate functionalized with immobile ligands increases the propensity for raft domain formation and drive protein clustering, and disruption of lipid raft integrity and binding protein clustering weakens the cell-substrate adhesion that is mediated by the binding of receptors and ligands (Son et al., 2017). Conversely, other experimental studies show that the receptor-ligand binding and the cell-substrate adhesion are enhanced because of the increased uniformity of distribution of both lipid raft and raft-associated protein (Norman et al., 2010; Murai et al., 2013). These studies give rise to the question of what accounts for the discrepancy regarding the interplay between the receptor-ligand binding and the lipid cluster coalescence in cell-extracellular matrix adhesion.
To this end, we adopt the mesoscale model described above, but consider ligands immobilized 1) in form of clusters, or 2) uniformly, or 3) randomly on the substrate. Our results suggest that the relationship between the receptor-ligand binding and the coalescence of lipid clusters is regulated by the ligand distribution (Li et al., 2021e). For clustered ligand distribution, the ligand molecules can be immobilized on substrate in the form of one or multiple clusters. For multiple ligand clusters, it is found that the binding of receptors to immobile ligands leads to a greater value of the raft-raft contact energy at which the phase separation takes place in the cell-substrate adhesion system. This indicates that the receptor-ligand binding hinders the coalescence of lipid rafts into large domains. Such inhibition results from the fact that the lipid raft coalescence needs to pay an energetic penalty (receptor-raft coupling energy and receptor-ligand binding energy) for releasing the raft-associated and ligand-bounded receptors (Figure 2A) (Li et al., 2021e). This result is contrary to the findings for mobile ligands in cell-cell adhesion system, wherein the raft coalescence is promoted upon the receptor-ligand binding. In contrast, for one single ligand cluster, the receptor-ligand binding causes the phase separation to occur at smaller values of
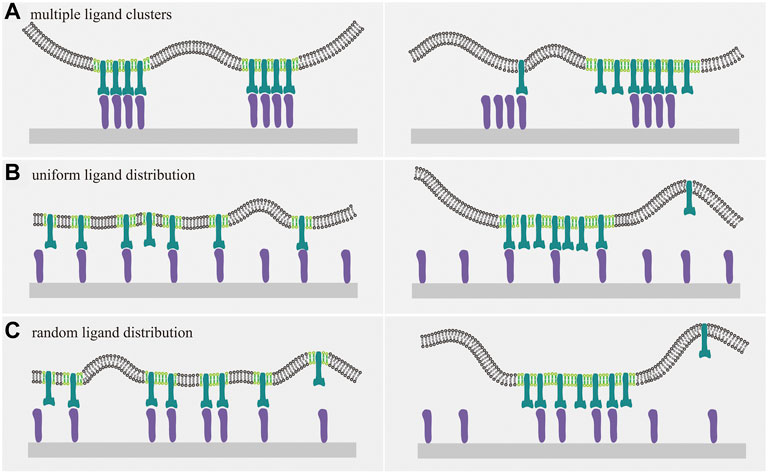
FIGURE 2. Cartoons of membrane receptors binding to ligands immobilized on a planar substrate. The interplay between receptor-ligand binding and raft domain formation depends on the distribution of the immobilized ligands. Receptors, ligands, and lipid rafts are indicated with the same color as in Figure 1. (A) For multiple ligand clusters, the receptor-ligand binding inhibits the coalescence of rafts into large domains, since the release of raft-associated and ligand-bounded receptors is required for the formation of large raft domains and energetically unfavorable due to the receptor-raft coupling energy and receptor-ligand binding energy, as evident from the comparison between the right and left panel. For uniform (B) and random (C) distribution of immobile ligands, receptor-ligands binding disfavors the coalescence of lipid rafts and vice versa. This interplay can be understood by considering the energetic penalty as discussed in (A).
Results for the substrate ligands immobilized uniformly or randomly show that the binding of raft-associated receptors to immobile ligands increases the raft-raft contact energy
Experimental findings from both in situ and mimetic model systems with mobile and immobile ligands indicate that there exists a discrepancy with regard to the relationship between the receptor-ligand binding and the lipid cluster coalescence, which may result from the ligand mobility. It is found that the receptor-ligand binding and the lipid domain formation reinforce each other for mobile ligands, in agreement with the experimental observations (Huang et al., 2010; Anderson and Roche, 2015). In contrast, the receptor-ligand binding and the lipid cluster coalescence can be correlated positively or negatively, depending strongly on the distribution of immobilized ligands. It has been recognized that the environmental conditions such as pH, temperature, ionic strength, composition of buffer solution, as well as the properties of both proteins and substrate have tremendous impact on the distribution of proteins on the substrate (Rabe et al., 2011; Landoulsi and Dupres, 2013). The difference in protein distribution can result in different outcome with regard to the interplay between the binding of receptors and ligands and the formation of raft domains.
Discussion and perspective
Separate studies on the receptor-ligand binding in cell adhesion processes, on the one hand, and on lipid rafts in cell membranes, on the other hand, have revealed their important roles in various biological functions and provided inspiring therapeutic targets for disease intervention and treatment. For example, the binding of CD47 proteins overexpressed in cancer cells to SIRPα receptors anchored in the macrophage plasma membrane enhances the phosphorylation of immunoreceptor tyrosine-based inhibitory motif (ITIM) and promotes the activation of phosphatases (e.g., SHP-1/2), which in turn assists cancer cells in escaping from the surveillance by immune cells and the elimination by macrophages (Zhang et al., 2020). Anti-cancer treatments (e.g., immunotherapy) by intervening the binding affinity of CD47 to SIRPα contribute to the adaptive immune response and tumour cells phagocytosis (Ring et al., 2017; Weiskopf, 2017; Zhang et al., 2020). On the other hand, disrupting raft integrity to regulate lipid and protein heterogeneity has been shown to be an effective way to control the protein-protein cis-interactions, signal pathways and cell fate, making lipid rafts a promising target for therapeutic interventions in various diseases (Mollinedo and Gajate, 2015; Sviridov et al., 2020). Studies on correlations between the receptor-ligand binding and lipid raft redistributions can further enrich the strategies for novel therapies. Take for example the syndrome coronavirus-2 (SARS-CoV-2) that currently ravages the world. Recent investigations suggest that lipid rafts promote aggregation of angiotensin-converting enzyme-2 (ACE-2), which in turn enhances the binding of ACE-2 on the host cell membrane to the spike protein on SARS-CoV-2 envelope. Disruption of lipid raft integrity and membrane heterogeneity adversely affects the ACE-2-spike binding, leading to inhibited coronavirus adhesion, entry, and infectivity (Sorice et al., 2021). These encouraging results introduce potential therapeutic approaches against coronavirus, and highlight the importance of elucidating the relationship between the receptor-ligand binding and the lipid cluster coalescence.
Existing experimental studies have led to contradictory conclusions with regard to the relation between the binding of receptors and ligands and the formation of raft domains for cell-cell and cell-extracellular matrix adhesion. Here, we reviewed recent results obtained from statistical-mechanical models and computer simulations that provide new insights into the relationships between the receptor-ligand binding and the lipid cluster coalescence. In the case of cell membrane adhesion mediated by mobile receptors and ligands, the binding of raft-associated receptors and ligands facilitates the coalescence of lipid rafts due to the fluctuation-induced lateral attraction between the receptor-ligand complexes. The raft coalescence and resultant protein aggregation, in turn, enhance the receptor-ligand binding due to entropic effects and elevated local concentrations of the receptor and ligand molecules. In contrast, in the case of adhesion mediated by membrane-anchored receptors binding to immobile ligands, the receptor-ligand binding and the lipid domain formation may be correlated positively or negatively, depending strongly on the ligand distribution. For uniform or random distributions of immobile ligands, their binding to receptors associated with lipid rafts disfavors the coalescence of lipid rafts, and vice versa. For ligands immobilized in clusters, however, the receptor-ligand binding and the propensity for lipid raft to coalesce can cooperate or not, depending on whether the ligands are immobilized in single or multiple clusters. This complexity of possibilities can be explained by considering the translational entropy of lipid rafts, the configurational entropy of the adhering membranes, and the energetic penalty due to excessive free receptors within lipid domains. All these findings together deepen our understanding of the interplay between the receptor-ligand binding and the formation of membrane heterogeneities, and help to explain the existing experimental discrepancies, wherein the ligand mobility and distribution should be carefully taken into account. In addition, these results suggest that caution should be exercised for drug design targeting the receptor-ligand binding as well as the lipid and protein heterogeneities to intervene the disease development that involves both the cell-cell adhesion and the cell-extracellular matrix adhesion.
An important direction of future studies seems to be the role of the cytoskeletal network beneath cell membranes. On the one hand, the cytoskeletal network can affect membrane fluctuation spectra due to the membrane-cytoskeleton anchorage and active cytoskeletal dynamics (Rodriguez-Garcia et al., 2015). On the other hand, cytoskeletal network is involved in regulating integrity and organization of lipid rafts by means of cytoskeleton remodeling and raft-cytoskeleton coupling (Head et al., 2014; Li et al., 2021a). Therefore, the cytoskeletal network is likely to play a pivotal role in the relationship between the binding of receptors and ligands and the formation of lipid domain as an internal factor that needs to be confirmed. A better understanding of the contribution of the cytoskeletal network to the receptor-ligand binding and raft reorganization will further extend our understanding of cell adhesion and has the potential to advance the drug design and disease treatment.
Author contributions
JH, BR, and JJ designed this research. LL, JH, BR, and JJ wrote the first draft of the manuscript. All authors read and contributed to the final manuscript.
Funding
LL, JH, and FS acknowledge support from the National Natural Science Foundation of China (Grant Nos. 11902327, 11972041, 21973040, 12272388, and 12232019), Youth Innovation Promotion Association CAS. This work was also supported jointly by the National Natural Science Foundation of China (Grant No. 22161132012 to JH) and the National Science Centre of Poland (Grant No. 2021/40/Q/NZ1/00017 to BR) within the framework of the international SHENG program.
Conflict of interest
The authors declare that the research was conducted in the absence of any commercial or financial relationships that could be construed as a potential conflict of interest.
Publisher’s note
All claims expressed in this article are solely those of the authors and do not necessarily represent those of their affiliated organizations, or those of the publisher, the editors and the reviewers. Any product that may be evaluated in this article, or claim that may be made by its manufacturer, is not guaranteed or endorsed by the publisher.
References
Abraham, S. N., and Miao, Y. X. (2015). The nature of immune responses to urinary tract infections. Nat. Rev. Immunol. 15, 655–663. doi:10.1038/nri3887
Alon, R., Hammer, D. A., and Springer, T. A. (1995). Lifetime of the P-selectin-carbohydrate bond and its response to tensile force in hydrodynamic flow. Nature 374, 539–542. doi:10.1038/374539a0
An, C., Wang, X., Song, F., Hu, J. L., and Li, L. (2022). Insights into intercellular receptor-ligand binding kinetics in cell communication. Front. Bioeng. Biotechnol. 10, 953353. doi:10.3389/fbioe.2022.953353
Anderson, H. A., Hiltbold, E. M., and Roche, P. A. (2000). Concentration of MHC class II molecules in lipid rafts facilitates antigen presentation. Nat. Immunol. 1, 156–162. doi:10.1038/77842
Anderson, H. A., and Roche, P. A. (2015). MHC class II association with lipid rafts on the antigen presenting cell surface. Biochim. Biophys. Acta 1853, 775–780. doi:10.1016/j.bbamcr.2014.09.019
Chen, Y. F., Ju, L. A., Zhou, F. Y., Liao, J. X., Xue, L. Z., Su, Q. P., et al. (2019). An integrin αIIbβ3 intermediate affinity state mediates biomechanical platelet aggregation. Nat. Mat. 18, 760–769. doi:10.1038/s41563-019-0323-6
Destainville, N., Manghi, M., and Cornet, J. (2018). A rationale for mesoscopic domain formation in biomembranes. Biomolecules 8, E104. doi:10.3390/biom8040104
Doan, T. L. L., Guerardel, Y., Loubiere, P., Mercier-Bonin, M., and Dague, E. (2011). Measuring kinetic dissociation/association constants between Lactococcus lactis bacteria and mucins using living cell probes. Biophys. J. 101, 2843–2853. doi:10.1016/j.bpj.2011.10.034
Douglass, A. D., and Vale, R. D. (2005). Single-molecule microscopy reveals plasma membrane microdomains created by protein-protein networks that exclude or trap signaling molecules in T cells. Cell 121, 937–950. doi:10.1016/j.cell.2005.04.009
Dustin, M. L., Ferguson, L. M., Chan, P. Y., Springer, T. A., and Golan, D. E. (1996). Visualization of CD2 interaction with LFA-3 and determination of the two-dimensional dissociation constant for adhesion receptors in a contact area. J. Cell Biol. 132, 465–474. doi:10.1083/jcb.132.3.465
Evani, S. J., and Ramasubramanian, A. K. (2016). Biophysical regulation of Chlamydia pneumoniae-infected monocyte recruitment to atherosclerotic foci. Sci. Rep. 6, 19058. doi:10.1038/srep19058
Fan, J., Sammalkorpi, M., and Haataja, M. (2010). Formation and regulation of lipid microdomains in cell membranes: Theory, modeling, and speculation. FEBS Lett. 584, 1678–1684. doi:10.1016/j.febslet.2009.10.051
Fenz, S. F., Bihr, T., Schmidt, D., Merkel, R., Seifert, U., Sengupta, K., et al. (2017). Membrane fluctuations mediate lateral interaction between cadherin bonds. Nat. Phys. 13, 906–913. doi:10.1038/nphys4138
He, K. J., Wei, Y. S., Zhang, Z. H., Chen, H. B., Yuan, B., Pang, H. B., et al. (2021). Membrane-curvature-mediated co-endocytosis of bystander and functional nanoparticles. Nanoscale 13, 9626–9633. doi:10.1039/d1nr01443a
Head, B. P., Patel, H. H., and Insel, P. A. (2014). Interaction of membrane/lipid rafts with the cytoskeleton: Impact on signaling and function membrane/lipid rafts, mediators of cytoskeletal arrangement and cell signaling. Biochim. Biophys. Acta 1838, 532–545. doi:10.1016/j.bbamem.2013.07.018
Hu, J. L., Lipowsky, R., and Weikl, T. R. (2013). Binding constants of membrane-anchored receptors and ligands depend strongly on the nanoscale roughness of membranes. Proc. Natl. Acad. Sci. U. S. A. 110, 15283–15288. doi:10.1073/pnas.1305766110
Hu, J. L., Xu, G. K., Lipowsky, R., and Weikl, T. R. (2015). Binding kinetics of membrane-anchored receptors and ligands: Molecular dynamics simulations and theory. J. Chem. Phys. 143, 243137. doi:10.1063/1.4936135
Huang, J., Zarnitsyna, V. I., Liu, B. Y., Edwards, L. J., Jiang, N., Evavold, B. D., et al. (2010). The kinetics of two-dimensional TCR and pMHC interactions determine T-cell responsiveness. Nature 464, 932–936. doi:10.1038/nature08944
Huppa, J. B., Axmann, M., Mortelmaier, M. A., Lillemeier, B. F., Newell, E. W., Brameshuber, M., et al. (2010). TCR-peptide-MHC interactions in situ show accelerated kinetics and increased affinity. Nature 463, 963–967. doi:10.1038/nature08746
Kav, B., Grafmuller, A., Schneck, E., and Weikl, T. R. (2020). Weak carbohydrate-carbohydrate interactions in membrane adhesion are fuzzy and generic. Nanoscale 12, 17342–17353. doi:10.1039/d0nr03696j
Krobath, H., Rozycki, B., Lipowsky, R., and Weikl, T. R. (2009). Binding cooperativity of membrane adhesion receptors. Soft Matter 5, 3354–3361. doi:10.1039/b902036e
Kuo, J. C. H., Gandhi, J. G., Zia, R. N., and Paszek, M. J. (2018). Physical biology of the cancer cell glycocalyx. Nat. Phys. 14, 658–669. doi:10.1038/s41567-018-0186-9
Landoulsi, J., and Dupres, V. (2013). Direct AFM force mapping of surface nanoscale organization and protein adsorption on an aluminum substrate. Phys. Chem. Chem. Phys. 15, 8429–8440. doi:10.1039/c3cp00137g
Levental, I., Levental, K. R., and Heberle, F. A. (2020). Lipid Rafts: Controversies resolved, mysteries remain. Trends Cell Biol. 30, 341–353. doi:10.1016/j.tcb.2020.01.009
Li, G. Y., Hu, X. W., Nie, P. P., Mang, D. Z., Jiao, S., Zhang, S. J., et al. (2021a). Lipid-raft-targeted molecular self-assembly inactivates YAP to treat ovarian cancer. Nano Lett. 21, 747–755. doi:10.1021/acs.nanolett.0c04435
Li, L., Hu, J. L., Li, L., and Song, F. (2019). Binding constant of membrane-anchored receptors and ligands that induce membrane curvatures. Soft Matter 15, 3507–3514. doi:10.1039/c8sm02504e
Li, L., Hu, J. L., Rozycki, B., and Song, F. (2020). Intercellular receptor-ligand binding and thermal fluctuations facilitate receptor aggregation in adhering membranes. Nano Lett. 20, 722–728. doi:10.1021/acs.nanolett.9b04596
Li, L., Hu, J. L., Rozycki, B., Wang, X. H., Wu, H. L., and Song, F. (2021b). Influence of lipid rafts on pattern formation during T-cell adhesion. New J. Phys. 23, 043052. doi:10.1088/1367-2630/abeacb
Li, L., Hu, J. L., Shi, X. H., Rozycki, B., and Song, F. (2021c). Interplay between cooperativity of intercellular receptor-ligand binding and coalescence of nanoscale lipid clusters in adhering membranes. Soft Matter 17, 1912–1920. doi:10.1039/d0sm01904f
Li, L., Hu, J. L., Shi, X. H., Shao, Y. F., and Song, F. (2017). Lipid rafts enhance the binding constant of membrane-anchored receptors and ligands. Soft Matter 13, 4294–4304. doi:10.1039/c7sm00572e
Li, L., Hu, J. L., Wu, H. P., and Song, F. (2021d). Cis-interaction of ligands on a supported lipid bilayer affects their binding to cell adhesion receptors. Sci. China Phys. Mech. Astron. 64, 108712. doi:10.1007/s11433-021-1752-0
Li, L., Hu, J. L., Xu, G. K., and Song, F. (2018a). Binding constant of cell adhesion receptors and substrate-immobilized ligands depends on the distribution of ligands. Phys. Rev. E 97, 012405. doi:10.1103/PhysRevE.97.012405
Li, L., Ji, J., Song, F., and Hu, J. (2022). Intercellular receptor-ligand binding: Effect of protein-membrane interaction. J. Mol. Biol., 167787. doi:10.1016/j.jmb.2022.167787
Li, L., Wang, X. H., Shao, Y. F., Li, W., and Song, F. (2018b). Entropic pressure between fluctuating membranes in multilayer systems. Sci. China Phys. Mech. Astron. 61, 128711. doi:10.1007/s11433-018-9264-x
Li, L., Wang, X. H., Wu, H. L., Shao, Y. F., Wu, H. P., and Song, F. (2021e). Interplay between receptor-ligand binding and lipid domain formation depends on the mobility of ligands in cell-substrate adhesion. Front. Mol. Biosci. 8, 655662. doi:10.3389/fmolb.2021.655662
Limozin, L., Bongrand, P., and Robert, P. (2016). A rough energy landscape to describe surface-linked antibody and antigen bond formation. Sci. Rep. 6, 35193. doi:10.1038/srep35193
Lingwood, D., and Simons, K. (2010). Lipid rafts as a membrane-organizing principle. Science 327, 46–50. doi:10.1126/science.1174621
Lorent, J. H., Diaz-Rohrer, B., Lin, X. B., Spring, K., Gorfe, A. A., Levental, K. R., et al. (2017). Structural determinants and functional consequences of protein affinity for membrane rafts. Nat. Commun. 8, 1219. doi:10.1038/s41467-017-01328-3
Micalizzi, D. S., Ebright, R. Y., Haber, D. A., and Maheswaran, S. (2021). Translational regulation of cancer metastasis. Cancer Res. 81, 517–524. doi:10.1158/0008-5472.can-20-2720
Michel, V., and Bakovic, M. (2007). Lipid rafts in health and disease. Biol. Cell 99, 129–140. doi:10.1042/bc20060051
Mitchell, J. S., Brown, W. S., Woodside, D. G., Vanderslice, P., and McIntyre, B. W. (2009). Clustering T-cell GM1 lipid rafts increases cellular resistance to shear on fibronectin through changes in integrin affinity and cytoskeletal dynamics. Immunol. Cell Biol. 87, 324–336. doi:10.1038/icb.2008.103
Mollinedo, F., and Gajate, C. (2015). Lipid rafts as major platforms for signaling regulation in cancer. Adv. Biol. Regul. 57, 130–146. doi:10.1016/j.jbior.2014.10.003
Murai, T. (2015). Lipid raft-mediated regulation of hyaluronan-CD44 interactions in inflammation and cancer. Front. Immunol. 6, 420. doi:10.3389/fimmu.2015.00420
Murai, T., Sato, C., Sato, M., Nishiyama, H., Suga, M., Mio, K., et al. (2013). Membrane cholesterol modulates the hyaluronan-binding ability of CD44 in T lymphocytes and controls rolling under shear flow. J. Cell Sci. 126, 3284–3294. doi:10.1242/jcs.120014
Norman, L. L., Oetama, R. J., Dembo, M., Byfield, F., Hammer, D. A., Levitan, I., et al. (2010). Modification of cellular cholesterol content affects traction force, adhesion and cell spreading. Cell. Mol. Bioeng. 3, 151–162. doi:10.1007/s12195-010-0119-x
O'Donoghue, G. P., Pielak, R. M., Smoligovets, A. A., Lin, J. J., and Groves, J. T. (2013). Direct single molecule measurement of TCR triggering by agonist pMHC in living primary T cells. Elife 2, e00778. doi:10.7554/eLife.00778
Paszek, M. J., DuFort, C. C., Rossier, O., Bainer, R., Mouw, J. K., Godula, K., et al. (2014). The cancer glycocalyx mechanically primes integrin-mediated growth and survival. Nature 511, 319–325. doi:10.1038/nature13535
Pierce, S. K. (2002). Lipid rafts and B-cell activation. Nat. Rev. Immunol. 2, 96–105. doi:10.1038/nri726
Pralle, A., Keller, P., Florin, E. L., Simons, K., and Horber, J. K. H. (2000). Sphingolipid-cholesterol rafts diffuse as small entities in the plasma membrane of mammalian cells. J. Cell Biol. 148, 997–1008. doi:10.1083/jcb.148.5.997
Rabe, M., Verdes, D., and Seeger, S. (2011). Understanding protein adsorption phenomena at solid surfaces. Adv. Colloid Interface Sci. 162, 87–106. doi:10.1016/j.cis.2010.12.007
Ring, N. G., Herndler-Brandstetter, D., Weiskopf, K., Shan, L., Volkmer, J. P., George, B. M., et al. (2017). Anti-SIRPα antibody immunotherapy enhances neutrophil and macrophage antitumor activity. Proc. Natl. Acad. Sci. U. S. A. 114, E10578–E10585. doi:10.1073/pnas.1710877114
Rodriguez-Garcia, R., Lopez-Montero, I., Mell, M., Egea, G., Gov, N. S., and Monroy, F. (2015). Direct cytoskeleton forces cause membrane softening in red blood cells. Biophys. J. 108, 2794–2806. doi:10.1016/j.bpj.2015.05.005
Rozycki, B., and Weikl, T. R. (2021). Cooperative stabilization of close-contact zones leads to sensitivity and selectivity in T-cell recognition. Cells 10, 1023. doi:10.3390/cells10051023
Sackmann, E., and Smith, A. S. (2014). Physics of cell adhesion: Some lessons from cell-mimetic systems. Soft Matter 10, 1644–1659. doi:10.1039/c3sm51910d
Sando, R., Jiang, X., and Südhof, T. C. (2019). Latrophilin GPCRs direct synapse specificity by coincident binding of FLRTs and teneurins. Science 363, eaav7969. doi:10.1126/science.aav7969
Scapin, G., Dandey, V. P., Zhang, Z. N., Prosise, W., Hruza, A., Kelly, T., et al. (2018). Structure of the insulin receptor-insulin complex by single-particle cryo-EM analysis. Nature 556, 122–125. doi:10.1038/nature26153
Sezgin, E., Levental, I., Mayor, S., and Eggeling, C. (2017). The mystery of membrane organization: Composition, regulation and roles of lipid rafts. Nat. Rev. Mol. Cell Biol. 18, 361–374. doi:10.1038/nrm.2017.16
Simons, K., and Ehehalt, R. (2002). Cholesterol, lipid rafts, and disease. J. Clin. Invest. 110, 597–603. doi:10.1172/JCI16390
Simons, K., and Sampaio, J. L. (2011). Membrane organization and lipid rafts. Cold Spring Harb. Perspect. Biol. 3, a004697. doi:10.1101/cshperspect.a004697
Simons, K., and Toomre, D. (2000). Lipid rafts and signal transduction. Nat. Rev. Mol. Cell Biol. 1, 31–39. doi:10.1038/35036052
Singh, J., Pagulayan, A., Camley, B. A., and Nain, A. S. (2021). Rules of contact inhibition of locomotion for cells on suspended nanofibers. Proc. Natl. Acad. Sci. U. S. A. 118, e2011815118. doi:10.1073/pnas.2011815118
Son, S., Moroney, G. J., and Butler, P. J. (2017). β1-integrin-mediated adhesion is lipid-bilayer dependent. Biophys. J. 113, 1080–1092. doi:10.1016/j.bpj.2017.07.010
Sorice, M., Misasi, R., Riitano, G., Manganelli, V., Martellucci, S., Longo, A., et al. (2021). Targeting lipid rafts as a strategy against coronavirus. Front. Cell Dev. Biol. 8, 618296. doi:10.3389/fcell.2020.618296
Steinkühler, J., Rozycki, B., Alvey, C., Lipowsky, R., Weikl, T. R., Dimova, R., et al. (2019). Membrane fluctuations and acidosis regulate cooperative binding of 'marker of self' protein CD47 with the macrophage checkpoint receptor SIRPα. J. Cell Sci. 132, jcs216770. doi:10.1242/jcs.216770
Stone, M. B., Shelby, S. A., Nunez, M. F., Wisser, K., and Veatch, S. L. (2017). Protein sorting by lipid phase-like domains supports emergent signaling function in B lymphocyte plasma membranes. Elife 6, e19891. doi:10.7554/eLife.19891
Sviridov, D., Mukhamedova, N., and Miller, Y. I. (2020). Lipid rafts as a therapeutic target. J. Lipid Res. 61, 687–695. doi:10.1194/jlr.TR120000658
Viola, A., and Gupta, N. (2007). Tether and trap: Regulation of membrane-raft dynamics by actin-binding proteins. Nat. Rev. Immunol. 7, 889–896. doi:10.1038/nri2193
Wang, C. R., Yoo, Y., Fan, H. P., Kim, E., Guan, K. L., and Guan, J. L. (2010). Regulation of integrin β1 recycling to lipid rafts by Rab1a to promote cell migration. J. Biol. Chem. 285, 29398–29405. doi:10.1074/jbc.M110.141440
Weikl, T. R., Hu, J. L., Xu, G. K., and Lipowsky, R. (2016). Binding equilibrium and kinetics of membrane-anchored receptors and ligands in cell adhesion: Insights from computational model systems and theory. Cell adh. Migr. 10, 576–589. doi:10.1080/19336918.2016.1180487
Weikl, T. R. (2018). Membrane-mediated cooperativity of proteins. Annu. Rev. Phys. Chem. 69, 521–539. doi:10.1146/annurev-physchem-052516-050637
Weiskopf, K. (2017). Cancer immunotherapy targeting the CD47/SIRPα axis. Eur. J. Cancer 76, 100–109. doi:10.1016/j.ejca.2017.02.013
Xiao, H., Woods, E. C., Vukojicic, P., and Bertozzi, C. R. (2016). Precision glycocalyx editing as a strategy for cancer immunotherapy. Proc. Natl. Acad. Sci. U. S. A. 113, 10304–10309. doi:10.1073/pnas.1608069113
Xu, G. K., Hu, J. L., Lipowsky, R., and Weikl, T. R. (2015). Binding constants of membrane-anchored receptors and ligands: A general theory corroborated by Monte Carlo simulations. J. Chem. Phys. 143, 243136. doi:10.1063/1.4936134
Xu, G. K., Qian, J., and Hu, J. L. (2016). The glycocalyx promotes cooperative binding and clustering of adhesion receptors. Soft Matter 12, 4572–4583. doi:10.1039/c5sm03139g
Yang, Z. S., Qin, W. H., Chen, Y., Yuan, B., Song, X. L., Wang, B. B., et al. (2018). Cholesterol inhibits hepatocellular carcinoma invasion and metastasis by promoting CD44 localization in lipid rafts. Cancer Lett. 429, 66–77. doi:10.1016/j.canlet.2018.04.038
Zhang, W. T., Huang, Q. H., Xiao, W. W., Zhao, Y., Pi, J., Xu, H., et al. (2020). Advances in anti-tumor treatments targeting the CD47/SIRPα axis. Front. Immunol. 11, 18. doi:10.3389/fimmu.2020.00018
Zhao, J., Wu, J., and Veatch, S. L. (2013). Adhesion stabilizes robust lipid heterogeneity in supercritical membranes at physiological temperature. Biophys. J. 104, 825–834. doi:10.1016/j.bpj.2012.12.047
Keywords: cell adhesion, receptor-ligand binding, lipid domain formation, binding affinity, binding cooperativity, phase separation
Citation: Li L, Hu J, Różycki B, Ji J and Song F (2022) Interplay of receptor-ligand binding and lipid domain formation during cell adhesion. Front. Mol. Biosci. 9:1019477. doi: 10.3389/fmolb.2022.1019477
Received: 15 August 2022; Accepted: 05 September 2022;
Published: 20 September 2022.
Edited by:
Shreyas Kaptan, University of Helsinki, FinlandReviewed by:
Giray Enkavi, University of Helsinki, FinlandAnna Duncan, University of Oxford, United Kingdom
Copyright © 2022 Li, Hu, Różycki, Ji and Song. This is an open-access article distributed under the terms of the Creative Commons Attribution License (CC BY). The use, distribution or reproduction in other forums is permitted, provided the original author(s) and the copyright owner(s) are credited and that the original publication in this journal is cited, in accordance with accepted academic practice. No use, distribution or reproduction is permitted which does not comply with these terms.
*Correspondence: Jinglei Hu, aHVqaW5nbGVpQG5qdS5lZHUuY24=; Bartosz Różycki, cm96eWNraUBpZnBhbi5lZHUucGw=; Jing Ji, MDk3MTRAYnVhYS5lZHUuY24=