- 1Department of Biochemistry, College of Medicine and Health Sciences, Debre Tabor University, Debre Tabor, Ethiopia
- 2Department of Physiology, College of Medicine and Health Sciences, Debre Tabor University, Debre Tabor, Ethiopia
- 3Department of Anatomy, College of Medicine and Health Sciences, Debre Tabor University, Debre Tabor, Ethiopia
Diabetes is a common metabolic illness characterized by hyperglycemia and is linked to long-term vascular problems that can impair the kidney, eyes, nerves, and blood vessels. By increasing protein glycation and gradually accumulating advanced glycation end products in the tissues, hyperglycemia plays a significant role in the pathogenesis of diabetic complications. Advanced glycation end products are heterogeneous molecules generated from non-enzymatic interactions of sugars with proteins, lipids, or nucleic acids via the glycation process. Protein glycation and the buildup of advanced glycation end products are important in the etiology of diabetes sequelae such as retinopathy, nephropathy, neuropathy, and atherosclerosis. Their contribution to diabetes complications occurs via a receptor-mediated signaling cascade or direct extracellular matrix destruction. According to recent research, the interaction of advanced glycation end products with their transmembrane receptor results in intracellular signaling, gene expression, the release of pro-inflammatory molecules, and the production of free radicals, all of which contribute to the pathology of diabetes complications. The primary aim of this paper was to discuss the chemical reactions and formation of advanced glycation end products, the interaction of advanced glycation end products with their receptor and downstream signaling cascade, and molecular mechanisms triggered by advanced glycation end products in the pathogenesis of both micro and macrovascular complications of diabetes mellitus.
Introduction
Diabetes mellitus (DM) is a chronic metabolic condition marked by hyperglycemia caused by abnormalities in insulin production, action, or both (Punthakee et al., 2018). It is associated with both acute and long-term vascular complications affecting the eye, kidney, nerves, and blood vessels which are the major causes of mortality and morbidity (Forbes and Cooper, 2013). Exposure to a hyperglycemic environment is the underlying cause of the pathogenesis of diabetic complications by activating or raising the rate of several metabolic pathways such as; the polyol pathway, the Protein Kinase C (PKC) pathway, and the production of advanced glycation end products (AGEs) (Park et al., 2019).
AGEs are heterogeneous substances formed by irreversible non-enzymatic interactions between reducing sugars and proteins, lipids, or nucleic acids, a process known as glycation (Perrone et al., 2020). AGEs can be exogenous (derived from food) or synthesized in the body endogenously (Vadakedath and Kandi, 2018). Exogenous AGEs can be found in meals (especially in western diets) as a result of cooking or food processing. Cooking circumstances (high temperatures for an extended period of time, low hydration, and high pH) generate huge numbers of several classes of AGEs (Mastrocola et al., 2020). Endogenous AGEs are majorly produced via the complex Maillard reaction, in which aldehyde groups on reducing sugars such as glucose, fructose, and ribose undergo a series of non-enzymatic reactions to the terminal amino groups of proteins, nucleic acids, and phospholipids, resulting in the formation of reactive carbonyl compounds (Fishman et al., 2018).
Glycation is a spontaneous and slow process under physiological conditions because it does not involve an enzyme catalyst; it requires several days or weeks to get completed (Rabbani and Ahn, 2019). AGEs, on the other hand, are actively generated and accumulate in the circulating blood and numerous tissues in the case of chronic hyperglycemia (Ahmad et al., 2014; Vadakedath and Kandi, 2018). Recently, both exogenous and endogenous AGEs have been implicated in the development of diabetic vascular problems in several investigations (Uribarri et al., 2015; Rhee and Kim, 2018). One of the potential mechanisms linking inflammation-related diabetes consequences such as atherosclerosis is exogenous AGE-induced extracellular matrix (ECM) alteration (Garay-Sevilla et al., 2021). Aside from atherosclerosis, AGE accumulation in ECM is thought to be important in other DM complications, as it has been linked to an increased risk of retinopathy and renal failure (Koska et al., 2018). However, the scope of this paper is on endogenous AGEs and their contribution to diabetic complications.
Clinical studies have revealed a link between the accumulation of endogenous AGEs and the occurrence of vascular problems in diabetes individuals (Koska et al., 2017). As indicated in Table 1, a significant association between AGEs and diabetic complications has been demonstrated. Furthermore, the measurement of AGEs in the tissue or circulation might be considered a promising biomarker and suggested as a predictor of diabetic complications (Simó-Servat et al., 2018). However, the precise biochemical processes underlying the pathophysiology of AGE-induced diabetic complications need to be elucidated. Hence, the focus of this paper was to compressively discuss; the chemical reactions and formation of AGEs, the interaction between AGE with their receptor and downstream signaling cascade, and molecular pathways induced by AGEs in the pathogenesis of both micro and macro-vascular complications of DM.
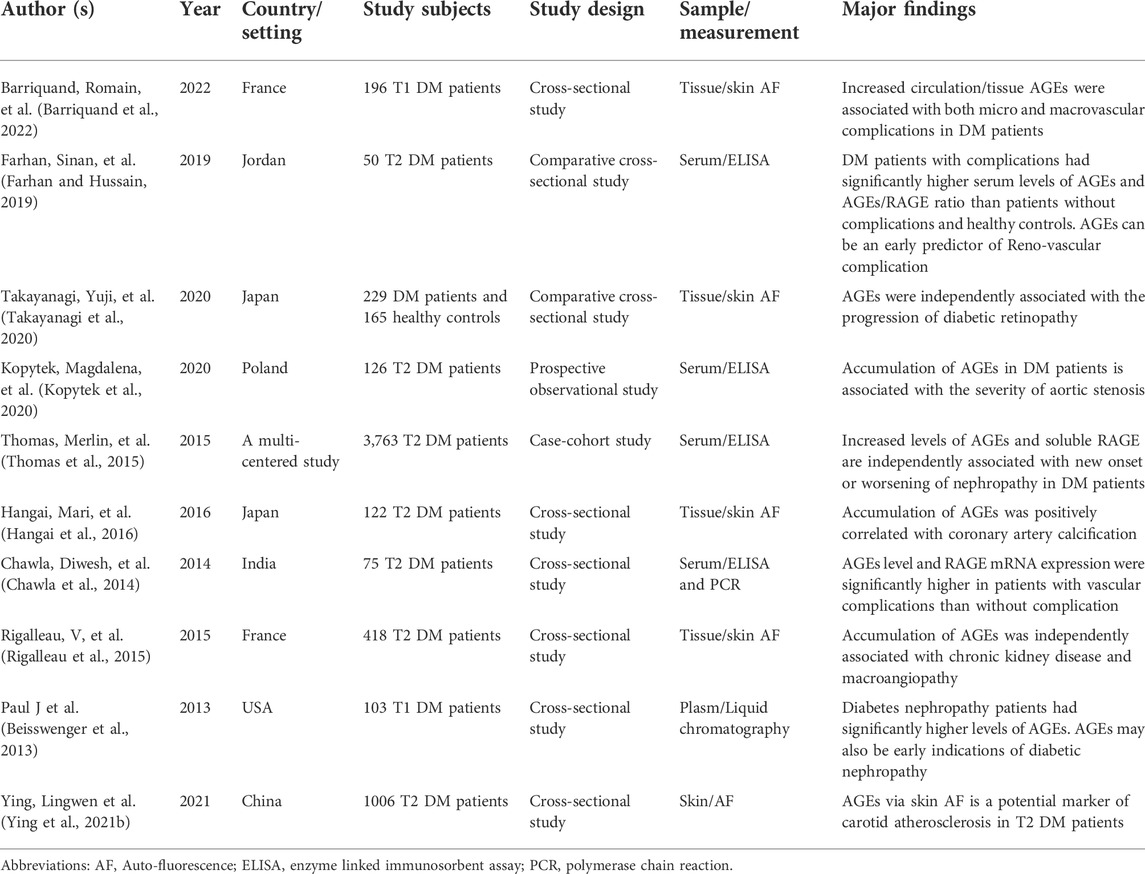
TABLE 1. Clinical and observational studies demonstrating the association between tissue or circulating AGEs and/or RAGE with diabetic chronic complications.
Biochemistry of AGEs
Biosynthesis of AGEs
The synthesis of AGEs is a complicated molecular process involving a multistep reaction. The whole process in the formation of AGE is called the Maillard reaction (Popova et al., 2010). Louis-Camille Maillard (1878–1936) developed the process in 1912, reporting that mixes of amino acids and sugars become intensely brown at high temperatures. After 40 years, chemist John E. Hodge discovered the mechanism of the Maillard reaction (Hellwig and Henle, 2014). It is a series of cascade reactions that occurs between free amino groups of proteins, peptides, and amino acids with carbonyl groups of reducing sugars (Perrone et al., 2020). This reaction has three distinct stages: early, intermediate, and late. A reducing sugar, such as glucose, combines non-enzymatically with the free amino group of protein to create the Schiff base in the early stages of the Maillard process. The Schiff base is then subjected to a rearrangement process, yielding a more stable molecule known as the Amadori product. In an intermediary stage, dehydration, oxidation, and other chemical events progressively degrade the Amadori product (keto-amine) to a variety of reactive carbonyl and dicarbonyl molecules such as glyoxal, methylglyoxal (MGO), and deoxyglucosones (Zhang et al., 2010; Tsekovska et al., 2016; Kim et al., 2017). Dicarbonyl compounds are the primary precursors for the synthesis of several key flavor products, heterocyclic compounds, and polymers. The highly electrophilic nature of these dicarbonyl compounds makes them react relatively faster with guanidine, arginine, lysine, and sulfhydryl functional moieties of proteins to produce different irreversible adducts (Allaman et al., 2015). Through polymerization, oxidation, dehydration, and cyclization events, nearly irreversible compounds known as AGEs are generated in the late or final stage of the Maillard reaction (Tsekovska et al., 2016). The properties of the participating reactants are one of the factors that affect the Maillard reaction. For instance, the monosaccharide fructose is more reactive in vitro than glucose (Yu et al., 2018). AGE molecules have the capacity to interact with certain proteins, leading to cross-links that impair the function of the body’s cells and tissues (Indyk et al., 2021).
Although the Maillard reaction is the most common process for the synthesis of AGEs, there are also other minor pathways, such as the polyol pathway and lipid peroxidation (Kuzan, 2021). The polyol pathway contains two enzymatic reactions catalyzed by aldose reductase (AR) and sorbitol dehydrogenase. Aldose reductase, the rate-limiting enzyme, reduces glucose to sorbitol at the expense of reduced nicotinamide adenine dinucleotide phosphate (NADPH), while, sorbitol dehydrogenase converts sorbitol to fructose at the expense of NAD+ leading to reduced nicotinamide adenine dinucleotide (NADH) production (Tang et al., 2012; Mathebula, 2015). Then the accumulated fructose can then be transformed into 3-deoxyglucose and fructose-3-phosphate, both of which are extremely powerful non-enzymatic glycation agents (Gugliucci, 2017). However, not only the polyol pathway (endogenous fructose), but consumption of fructose-rich diets (exogenous fructose) can also result in the formation of fructose-mediated AGEs (Sotokawauchi et al., 2019). Another process implicated in the synthesis of endogenous AGEs and protein cross-linking is lipid peroxidation. Lipid peroxidation is a process in which oxidants such as free radicals deteriorate lipids that contain a carbon-carbon double bond (Ayala et al., 2014). Oxidative stress-induced increased production of reactive aldehydes can occur because of lipid peroxidation and glycoxidation. Consequently, protein cross-linking, oligomerization, and the production of protein oxidation adducts occur (Moldogazieva et al., 2019).
The normal physiological rate of AGEs synthesis is proportional to the rate of protein turnover and oxidative stress. Long-lived proteins (such as collagen and elastin) are more vulnerable to glycation (Guo and Xu, 2017). The extent of AGEs synthesis in vivo is also increased by substrate availability (i.e., monosaccharides). As a result, their rate of production is accelerated in hyperglycemic conditions (Khalid et al., 2022). Although there are, several AGEs compounds that have not been well characterized, carboxymethyl-lysine (CML) and pentosidine have been the most described and studied AGEs so far (Ng et al., 2013a; Ashraf et al., 2014; Ashraf et al., 2015). They are insoluble, yellow-brown in appearance, and have fluorescent features that allow them to be detected in circulation and tissues. In general, AGEs can be classified as fluorescent or non-fluorescent based on their features (Haddad et al., 2016).
AGE and its receptor (RAGE) interaction
AGE receptors are known as receptor advanced glycation end-products (RAGEs), which are signal transduction receptors. It is a multi-ligand trans-membrane protein belonging to an immunoglobulin superfamily that is encoded by a gene on chromosome 6 and has 394 amino acids (Chawla and Kumar Tripathi, 2019). RAGE gene expression is abundant in many tissues and cells, including the vasculature, lung, heart, brain tissue, smooth muscle cells, monocytes/macrophages, and endothelial cells (Perrone et al., 2020). The full length of RAGE is the most well-studied isoform and has three domains; extracellular, transmembrane, and cytosolic domains (Chuah et al., 2013). Alternative splicing of mRNA of RAGE also leads to an additional RAGE isoform called soluble or secretary RAGE (sRAGE) which is a freely circulating form lacking a trans-membrane domain and not involved in the pathogenesis effect of AGEs (Ng et al., 2013b). Rather, they bind to AGEs to ensure endocytosis and degradation for the removal of AGEs (Kay et al., 2016).
The interaction of AGE and RAGE is critical in the pathophysiology of many diseases, including diabetes complications. The activation of RAGEs by AGEs transduces various signals, including those from the mitogen-activated protein kinases (MAPKs), extracellular signal-regulated kinases (ERKs), and Janus kinase, resulting in inflammatory, angiogenic, proliferative, and apoptotic responses (Chawla and Kumar Tripathi, 2019). The most frequently researched AGE receptor isoform is full-length RAGE, which is abundantly found on vascular endothelial cells. Through the activation of nuclear factor (NF-κB), the recognition of AGE by RAGE in endothelial cells accelerates the production of oxidative stress, different cytokines, and growth factors, culminating in the generation of inflammation (Rhee and Kim, 2018). The AGE/RAGE signaling cascade also increases the formation of reactive oxygen species (ROS) by activating certain signaling pathways such as TGF-α and NADPH-oxidase 1 (Nox-1). ROS-induced oxidative stress can alter a variety of intracellular components, including cellular membrane proteins, lipids, and DNA (Bongarzone et al., 2017). NF-κB is a universal transcription factor normally found in an inactive form in the cytoplasm bound to its inhibitor protein called IκB (Sivandzade et al., 2019). As illustrated in Figure 1, phosphorylation of IκB by a kinase protein called IκB kinas (Ikk), results in the separation of IκB from NF-κB. Then NF-κB trans-locates to the nucleus and binds to a specific region of DNA, triggering the development of numerous cytokines, chemokines, cell adhesion molecules, interleukins, TGF, pro-inflammatory proteins, and pro-apoptotic genes (Huang and Hung, 2013; Hinz and Scheidereit, 2014; Suryavanshi and Kulkarni, 2017).
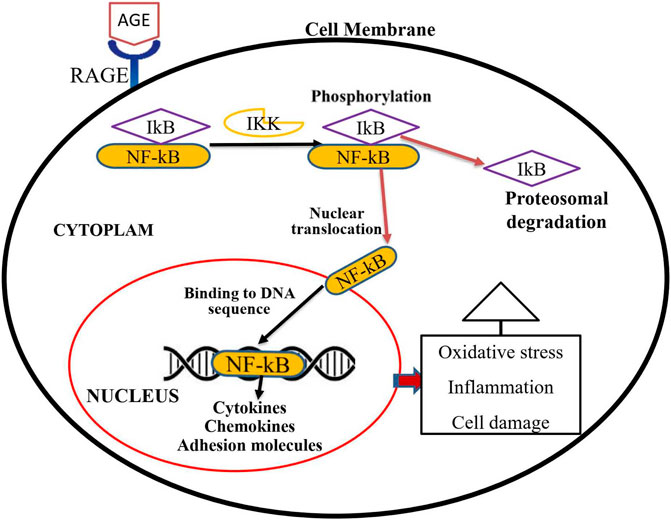
FIGURE 1. Activation process of NF-κB transcription factor through an AGE-RAGE signaling pathway. Activation of RAGE through AGE interaction transduces a signal for the phosphorylation of IkB by IKK. Phosphorylated IkB then could be detached from the cytosolic NF-kB transcription factor. Multiple genes such as cytokines, chemokines, and adhesion molecules are activated when active and free NF-kB translocate into the nucleus. These proteins trigger oxidative stress, inflammation, and cellular damage, all of which contribute to diabetic complications.
AGEs in the pathogenesis of diabetic complication
The chief causes of mortality and morbidity in diabetes are vascular complications, particularly microvascular and cardiovascular complications (Barrett et al., 2017). As previously stated, AGE cross-links are permanent and irreversible complexes generated when glucose binds to target proteins. As a result, once generated, AGEs will remain and continue to harm the tissue until the proteins involved are destroyed (Guo and Xu, 2017). AGEs can interact with the RAGE receptor to cause a variety of adverse outcomes including oxidative stress, apoptosis, and inflammation, as well as build the so-called “hyperglycemia memory” (Khalid et al., 2022). Although additional mechanisms, such as oxidative stress and epigenetic modifications, are implicated in the pathophysiology of hyperglycemic memory (currently metabolic memory), AGEs are the key contributors to metabolic memory (Zhan et al., 2022). Glycation and oxidative stress are inextricably related processes that are frequently referred to as glycoxidation. AGEs enhance the generation of reactive oxygen species (ROS) and impede antioxidant mechanisms; nonetheless, certain AGEs are formed naturally under oxidative conditions (Nowotny et al., 2015). Furthermore, AGE appears to be the primary driver of microvascular problems in DM for at least two reasons: First, AGE has various intra- and extracellular targets. Second, regardless of hyperglycemia level, Age-related intracellular glycation of mitochondrial respiratory chain proteins has been shown to produce additional reactive oxygen species, creating a vicious cycle that promotes AGE synthesis (Chilelli et al., 2013). As shown in the Figure 2 the general mechanisms by which AGEs contribute to all types of diabetic complications are either; receptor-mediated signaling cascade (AGE/RAGE cell surface interaction) or damage to the extracellular matrix through their cross-linking nature (Singh et al., 2014). Hence, understanding the mechanisms underlying accelerated diabetic complications is essential to uncover so that targeted therapeutic strategies might be developed.
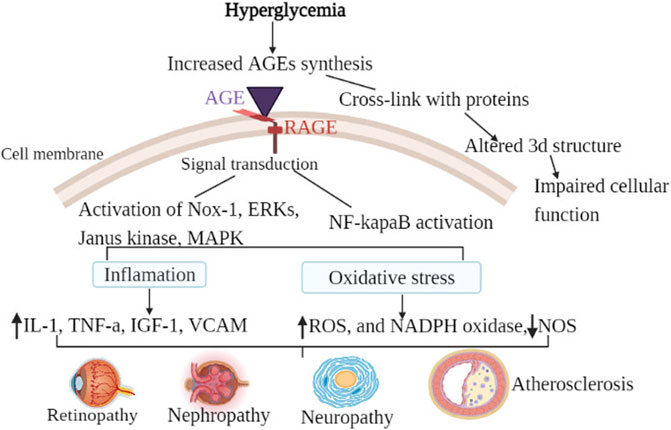
FIGURE 2. General mechanism of AGEs in diabetic chronic complications. Hyperglycemia is the most common cause of the synthesis of endogenous advanced glycation products in diabetic patients. The general mechanism of advanced glycation end-products in diabetic vascular complications is due to the activation of multiple signal transduction pathways as a result of RAGE/AGE interaction or through cross-link formation with cellular proteins. Activation of RAGE leads to the activation of Nox-1, ERKs, Janus kinase, MAPK, and activation of NF-κB. When those pathways are activated, oxidative stress (decreased NOS, increased ROS, and increased NADPH oxidase) and inflammatory factors are activated. Cross-link (adduct) formation on the other hand altered the three-dimensional structure of protein consequently impairing cellular function. As a result of the combined effect, diabetic vascular complications such as retinopathy, neuropathy, nephropathy, and atherosclerosis develop.
AGEs in diabetic retinopathy
Diabetic retinopathy (DR) is the most prevalent cause of blindness in people with DM, and it is characterized by lesions within the retina caused by changes in vascular permeability, capillary micro-aneurysm, loss of pericytes, and excessive development of new blood vessels (angiogenesis) (Shin et al., 2014). Recent epidemiological investigations have demonstrated that AGEs are linked to both the prevalence and severity of DR in diabetic patients (Beisswenger et al., 2013; Ying et al., 2021a). A significant association between skin AGEs and DR staging in T2DM patients was also reported, and skin AGE levels could substantially predict the degree of DR (Zhang et al., 2022). Chronic hyperglycemia exposure causes AGE buildup in the retina (Alan, 2018). Accumulation of AGEs in the retinal endothelial microcirculation contributes to premature closure (occlusion) of capillaries (Xu et al., 2018). Furthermore, they generate an increase in intracellular cell adhesion molecules (ICAM), which mediates retinal capillary leukocyte adherence and the collapse of the inner blood-retinal barrier, culminating in retinal injury (Moore et al., 2003; Alan, 2018).
Increasing pieces of evidence suggested that mRNA of RAGE is highly expressed in retinal cells of diabetic patients due to increased AGEs (Rujman Khan, 2016). Circulating AGE levels are positively linked with RAGE mRNA expression and oxidative indicators in patients with type 2 DM (Chawla et al., 2014). This means that RAGE expression could be enhanced in settings where ligands and inflammatory mediators accumulate (Yaw et al., 2013). It has been demonstrated that in a ligand-enriched environment (AGE accumulation), RAGE expression may rise and proinflammatory processes may be exacerbated (Senatus and Schmidt, 2017). The binding of AGE to RAGE triggers critical signaling pathways such as tyrosine phosphorylation of Janus kinase (JAK)/signal transducers and activators of transcription (STAT), activation of protein kinase C, and oxidative stress via NF-κB (Ott et al., 2014). Finally, it increases the expression of adhesion molecules and the production of cytokines including tumor necrosis factor-alpha (TNF-α) and vascular endothelial growth factor (VEGF) (Safi et al., 2014). Cytokines such as IL-α, IL-β, and IL-6 are mediators of inflammation in the retina whereas; VEGF is involved in the formation of new blood vessels (angiogenesis) in the retinal endothelium, which contributes to proliferative retinopathy (Gui et al., 2020). AGEs, on the other hand, independently promote the secretion of IL-6 from retinal cells, which can lead to neovascularization by boosting VEGF expression (Alan, 2018). It was also demonstrated that AGEs could enhance mRNA expression of TNF-α but reduce eNOS mRNA expression in human endothelial cells, which may contribute to vascular dysfunction in DM (Rashid et al., 2004).
Recently, RAGE gene variants (gene polymorphism) have been associated with DR by altering RAGE gene expression, albeit there are some contradictory studies (Tao et al., 2017). For instance, 2245 G/A RAGE gene polymorphisms were associated with the development of DR in the Malaysian population (Ng et al., 2012a). 374 T/A RAGE gene polymorphism might be also a risk factor for DR among Pakistani T2 DM patients (Qayyum et al., 2021). On the other hand, another study conducted on Malaysian DM patients reported that -429 T/C and -374 T/A gene polymorphism in the promoter region of the RAGE gene were not associated with DR (Ng et al., 2012b). There was also no association between 1704 G/T and 2184 A/G RAGE gene polymorphism and retinopathy susceptibility in DM patients (Ng et al., 2012c). Hence, more research is required to determine the relationship between RAGE gene polymorphism and DR.
AGEs also play an important part in the loss of lens transparency (cataract formation) (Hashim and Zarina, 2017). A cataract is one of the most common causes of vision impairment in diabetics. Glycation of lens proteins (crystallins) has been identified as one of the mechanisms causing diabetes cataracts. AGEs cause permanent alterations in structural proteins, causing lens protein aggregation and the creation of high-molecular-weight aggregates, which causes light dispersion and impairs vision (Rujman Khan, 2016). In vitro investigations also revealed that AGEs in pericytes promote apoptosis by increasing the activity of caspase-3 due to a reduction in the Bcl/Bax ratio (Safi et al., 2014). Because pericytes play a vital role in the maintenance of microvascular homeostasis, their loss can cause endothelial cell damage and angiogenesis in the retinal blood vessels, resulting in diabetic retinopathy (Huang, 2020).
AGEs in diabetic nephropathy
Diabetic nephropathy (DN) is the leading cause of end-stage renal failure in diabetic people (Lin et al., 2018). Clinically, it is defined by the development of proteinuria, followed by a gradual reduction in the glomerular filtration rate over time. It is also a substantial risk factor for macrovascular problems if left untreated (Parwani, 2017; Lin et al., 2018). AGE levels in renal tissue have been found to correlate with DN. AGEs disrupt the balance between synthesis and degradation of glomerular basement membrane extracellular matrix (ECM) components, particularly collagen (Anil Kumar et al., 2014). The cross-linking of AGE with collagen in the basement membrane will contribute to membrane thickening, impaired filtration, and eventually loss of glomerular function (Pasupulati et al., 2016).
AGE-RAGE axis also plays an important role in DN. TGF-β expression is stimulated by AGE-RAGE signaling in podocytes, tubular cells, and mesangial cells (Ott et al., 2014). TGF-β is expressed via the JAK/STATA signaling pathway and is a pro-fibrotic factor that increases the production of type IV collagen, laminin, and fibronectin, causing basement membrane thickening (Singh et al., 2014). The other mechanism of DN is through cross-talk between AGEs and Rennin-Angiotensin-Aldosterone System (RAAS). RAS components include renin, angiotensin I, angiotensin-converting enzyme (ACE), and angiotensin II, which is predominantly recognized to regulate fluid balance (Lin et al., 2018). Angiotensin II causes mesangial and tubular epithelial cell hypertrophy and acts via the angiotensin II type 1 receptor (AT1R). AGEs, on the other hand, increase AT1R expression, which increases Angiotensin II activity (Parwani, 2017).
Furthermore, AGE also induces renal inflammation and fibrosis. Activation of RAGE induces the expression of various cytokines in kidney cells as well. These cytokines, in turn, promote monocyte chemoattractant protein-1 (MCP-1) production in renal cells, which is related to monocyte/macrophage infiltration into the cell (Nowotny et al., 2015). In addition, AGEs also induce podocytopathy. Glomerular podocytes are specialized cells that act as a size-selective filtration barrier, regulating the entry of plasma proteins into the urine from circulation. RAGE activation in podocytes promotes NF-κB signaling, which causes zinc finger protein production called homeobox-2 E-box binding (ZEB2) (Pasupulati et al., 2016). ZEB2 is a transcription factor that regulates the epithelial-mesenchymal transition by inhibiting E-cadherin (an epithelial marker) and activating N-cadherin (a mesenchymal marker). Podocytes may undergo epithelial-mesenchymal transition and detach from the basement membrane, resulting in a drop in podocyte count per glomerulus and proteinuria (Loeffler and Wolf, 2015). A significant association between AGEs, particularly CML, with podocyte injury and proteinuria was also observed in DM patients, which contributed to impaired kidney function (Nishad et al., 2021). However, the precise signaling mechanisms of NF-κB/ZEB2 in podocytes still need to be explored.
AGEs in diabetic neuropathy
Diabetic neuropathy is a phenomenon characterized by segmental demyelination and axonal degradation in both the somatic and autonomic divisions of the peripheral nervous system (Salahuddin et al., 2014). It causes functional abnormalities such as decreased nerve transmission and blood flow, which increases the risk of lower extremity amputations in diabetics throughout the course of their lives (Amin and Doupis, 2016). The generation of AGEs in peripheral nerves has recently been identified as an additional risk factor for diabetic neuropathy development. The glycation of myelin is increased in diabetes. Glycated myelin is vulnerable to macrophage phagocytosis and induces macrophages to release proteases, which may contribute to nerve demyelination (Khangholi et al., 2015). It has been also observed that AGE modification of important axonal cytoskeletal proteins such as tubulin, neurofilament, and actin results in axonal atrophy/degeneration and decreased axonal transport (Sugimoto et al., 2008). Furthermore, in vitro studies revealed that oxidative stress increases the glycation of the Na+/K+ ATPase protein. Glycation of Na+/K+ ATPase may cause it to lose activity, resulting in a decrease in motor nerve conduction velocity (Singh et al., 2014). AGEs are elevated in the presence of distal sensorimotor polyneuropathy (DSPN) among T2 DM patients, and AGEs are linked with the severity of DSPN, according to observational studies (Papachristou et al., 2021). Decreased relative muscle strength was also observed in patients with T2 DM with elevated serum levels of AGEs (Wu et al., 2022).
AGEs in diabetic atherosclerosis
Atherosclerosis is the most serious long-term diabetes complication, defined by the deposition of atherosclerotic plaque on the interior of artery walls, leading to blockage of blood flow and, finally, myocardial infarction/cardiomyopathy (Nowotny et al., 2015). Increased glycation of Apolipoprotein-B and phospholipid components of low-density lipoprotein (LDL) particle occurs in diabetes. Apo B, a surface protein of LDL, is glycated at a positively charged lysine residue in the receptor-binding domain. Glycated LDL is thus not recognized by the LDL receptor, yet its absorption by macrophages is increased. This could hasten the production of foam cells seen in diabetics (Knott et al., 2019). Glycation, on the other hand, increases the turnover of high-density lipoprotein (HDL) and lowers its efficiency during reverse cholesterol transfer. Furthermore, glycation of HDL decreases the activity of paraoxonase, an HDL-associated enzyme that prevents LDL oxidation and monocyte adherence to endothelial cells. Both of which are critical early stages in the production of atherosclerotic plaques (Komplikacija and Tipa, 2015).
It has been reported that AGE-RAGE signaling generates oxidative stress and inhibits endothelial cell-derived nitric oxide (NO). Endothelial dysfunction, an early hallmark of atherosclerosis, could be exacerbated by impaired endothelial cell-derived NO production and/or bioavailability (Yamagishi and Matsui, 2018). The cross-linking of AGEs with extracellular matrix proteins like collagen and elastin has also been linked to arterial stiffness. Through their interaction with RAGE, AGEs also increase the synthesis of vascular endothelial growth factor (VEGF) in endothelial cells. VEGF then promotes pathologic angiogenesis in the atheroma and exacerbates plaque inflammation (Rhee and Kim, 2018).
When AGE binds to receptors, it causes oxidative stress, nuclear factor (NF-κB) activation, and the synthesis of adhesive molecules and vascular cell adhesion molecules (VCAM-1), resulting in greater permeability of endothelial cells and a more intense invasion of lipids in the sub-endothelium (Milstone et al., 2016). AGEs in DM also activate the RAGE/toll-like receptor 4 (TLR4) pathway in plaque macrophages. Delta-like ligand 4 (DLL4) expression is promoted by activated RAGE/TLR4 signaling. Dll4 expression on macrophage-treated vascular smooth muscle cells (VSMCs) results in contractile-phenotypic conversion via the Notch pathway, which contributes to atherosclerosis (Xing et al., 2022). Additionally, AGE-RAGE interaction in monocytes-macrophages increases the synthesis of the following mediators: interleukin-1 (Il-1), tumor necrosis factor (TNF-a), platelet-derived growth factor (PDGF), and insulin-like growth factor-1 (IGF-1), all of which play a role in the pathogenesis of atherosclerosis (Komplikacija and Tipa, 2015). Furthermore, oxidative stress induced by AGE-RAGE signaling has been demonstrated to reduce the expression levels of Adenosine triphosphate Binding membrane Cassette transporter A1 (ABCA1) in cultured macrophages and eventually suppress cholesterol efflux from macrophages to Apo-A1 of High-Density Lipoprotein (HDL), ultimately impairing the reverse cholesterol transport system (Yamagishi and Matsui, 2018).
Given the detrimental effects of AGEs, ongoing studies are being carried out to develop molecules that can suppress the AGE-RAGE signaling pathway (Hong Sheng et al., 2017a). Drugs clinically approved for other indications such as statins, anti-hypertensive medicines, and anti-diabetic therapies are the most recent promising anti-AGEs-RAGE signaling agents (Nenna et al., 2015). Nevertheless, the clinical evidence on the anti-AGE-RAGE antagonizing action of these medications is still limited to certain drug classes (Jud and Sourij, 2019). Statins have been demonstrated in studies to increase sRAGE levels by triggering RAGE shedding. Hence they suppress pro-inflammatory disease-promoting ligand/RAGE pathways (Quade-Lyssy et al., 2013). Although its exact molecular mechanism is not clearly elucidated, atorvastatin has been shown to have a direct inhibitory effect on AGE-RAGE expression in the aorta of rats, indicating that it may protect against diabetes-related atherosclerosis (Xu et al., 2014). It was observed that in DR patients on antihyperglycemic and antihypertensive medications, both NF-κB p65 and circulating MCP-1 levels, which are pro-inflammatory markers, were significantly reduced (Ng et al., 2013c). It is hypothesized that inhibiting the AGE-RAGE axis may be more advantageous in the early stages of diabetes, delaying the advancement of related vascular complications (Hong Sheng et al., 2017b). Furthermore, in recent years, natural compounds rich in bioactive elements (phytochemicals) have been shown to interact with AGEs and their related mediators via multiple signaling cascades, thereby limiting and inhibiting the course of diabetes (Parveen et al., 2021). The results of ongoing and future clinical trials may aid in defining the best therapeutic target for AGEs in diabetes complications.
Conclusion
Hyperglycemia promotes the development of AGEs in diabetic patients. Increased glycation and AGE accumulation in tissues and serum contribute significantly to the pathophysiology of diabetic vascular problems such as retinopathy, nephropathy, neuropathy, and atherosclerosis. Recent evidence focused on the molecular mechanism of AGE-RAGE axis cellular signaling in diabetic complications besides their impact on long-lived extracellular proteins through non-receptor-mediated mechanisms. Several chemical compounds are produced that appear to stimulate intracellular signal transduction pathways for the production of pro-inflammatory and pro-sclerotic cytokines, thereby increasing oxidative stress and gene expression and leading to the development and progression of diabetic vascular complications. In the future, these mechanisms and molecular pathways may be the source of new therapeutic targets to prevent vascular complications in diabetes mellitus patients.
Author contributions
MM developed the concept of the review. The manuscript of the protocol was drafted by EC, AB, and AT, then critically revised by MT, MA, EZ, and AA. MM developed and provided feedback for all sections of the review protocol and approved the final manuscript. All authors have approved the final version of the manuscript to be published.
Conflict of interest
The authors declare that the research was conducted in the absence of any commercial or financial relationships that could be construed as a potential conflict of interest.
Publisher’s note
All claims expressed in this article are solely those of the authors and do not necessarily represent those of their affiliated organizations, or those of the publisher, the editors and the reviewers. Any product that may be evaluated in this article, or claim that may be made by its manufacturer, is not guaranteed or endorsed by the publisher.
References
Ahmad, S., Khan, M. S., Akhter, F., Khan, M. S., Khan, A., Ashraf, J. M., et al. (2014). Glycoxidation of biological macromolecules: a critical approach to halt the menace of glycation. Glycobiology 24 (11), 979–990. doi:10.1093/glycob/cwu057 | |
Allaman, I., Bélanger, M., and Magistretti, P. J. (2015). Methylglyoxal, the dark side of glycolysis. Front. Neurosci. 9 (3), 23–12. doi:10.3389/fnins.2015.00023 | |
Amin, N., and Doupis, J. (2016). Diabetic foot disease: From the evaluation of the “foot at risk” to the novel diabetic ulcer treatment modalities. World J. Diabetes 7 (7), 153–164. doi:10.4239/wjd.v7.i7.153 | |
Anil Kumar, P., Welsh, G. I., Saleem, M. A., and Menon, R. K. (2014). Molecular and cellular events mediating glomerular podocyte dysfunction and depletion in diabetes mellitus. Front. Endocrinol. 5 (151), 151–210. doi:10.3389/fendo.2014.00151 |
Ashraf, J. M., Ahmad, S., Rabbani, G., Jan, A. T., Lee, E. J., Khan, R. H., et al. (2014). Physicochemical analysis of structural alteration and advanced glycation end products generation during glycation of H2A histone by 3-deoxyglucosone. IUBMB Life 66 (10), 686–693. doi:10.1002/iub.1318 | |
Ashraf, J. M., Ahmad, S., Rabbani, G., Hasan, Q., Jan, A. T., Lee, E. J., et al. (2015). 3-Deoxyglucosone: A potential glycating agent accountable for structural alteration in H3 histone protein through generation of different AGEs. PLoS One 10 (2), e0116804–10. doi:10.1371/journal.pone.0116804 | |
Ayala, A., Muñoz, M. F., and Argüelles, S. (2014). Lipid peroxidation: Production, metabolism, and signaling mechanisms of malondialdehyde and 4-hydroxy-2-nonenal. Oxid. Med. Cell. Longev. 2014, 360438–360534. doi:10.1155/2014/360438 | |
Barrett, E. J., Liu, Z., Khamaisi, M., King, G. L., Klein, R., Klein, B. E. K., et al. (2017). Diabetic microvascular disease: An endocrine society scientific statement. J. Clin. Endocrinol. Metab. 102 (12), 4343–4410. doi:10.1210/jc.2017-01922 | |
Barriquand, R., Francois, M., Ly, S., Mahmoudi, R., Arndt, C., Nazeyrollas, P., et al. (2022). Association between the tissue and circulating advanced glycation end-products and the micro- and macrovascular complications in type 1 diabetes. DIABAGE Study 13, 1531–1546.doi:10.1007/s13300-022-01285-1 |
Beisswenger, P. J., Howell, S. K., Russell, G. B., Miller, M. E., Rich, S. S., and Mauer, M. (2013). Early progression of diabetic nephropathy correlates with methylglyoxal-derived advanced glycation end products. Diabetes Care 36 (10), 3234–3239. doi:10.2337/dc12-2689 | |
Bongarzone, S., Savickas, V., Luzi, F., and Gee, A. D. (2017). Targeting the receptor for advanced glycation endproducts (RAGE): A medicinal chemistry perspective. J. Med. Chem. 60 (17), 7213–7232. doi:10.1021/acs.jmedchem.7b00058 | |
Chawla, D., and Kumar Tripathi, A. (2019). Role of advanced glycation end products (AGEs) and its receptor (RAGE)-mediated diabetic vascular complications. Integr. Food Nutr. Metab. 6 (5), 1–6. doi:10.15761/ifnm.1000267 |
Chawla, D., Bansal, S., Banerjee, B. D., Madhu, S. V., Kalra, O. P., and Tripathi, A. K. (2014). Role of advanced glycation end product (AGE)-induced receptor (RAGE) expression in diabetic vascular complications. Microvasc. Res. 95 (1), 1–6. doi:10.1016/j.mvr.2014.06.010 | |
Chilelli, N. C., Burlina, S., and Lapolla, A. (2013). AGEs, rather than hyperglycemia, are responsible formicrovascular complications in diabetes: A"glycoxidation-centric" point of view. Nutr. Metab. Cardiovasc. Dis. 23 (10), 913–919. doi:10.1016/j.numecd.2013.04.004 | |
Chuah, Y. K., Basir, R., Talib, H., Tie, T. H., and Nordin, N. (2013). Receptor for advanced glycation end products and its involvement in inflammatory diseases. Int. J. Inflam. 2013, 403460–403515. doi:10.1155/2013/403460 | |
Farhan, S. S., and Hussain, S. A. (2019). Advanced glycation end products (AGEs) and their soluble receptors (sRAGE) as early predictors of reno-vascular complications in patients with uncontrolled type 2 diabetes mellitus. Diabetes Metab. Syndr. 13 (4), 2457–2461. doi:10.1016/j.dsx.2019.06.019 | |
Fishman, S. L., Sonmez, H., Basman, C., Singh, V., and Poretsky, L. (2018). The role of advanced glycation end-products in the development of coronary artery disease in patients with and without diabetes mellitus: A review. Mol. Med. 24 (1), 59–12. doi:10.1186/s10020-018-0060-3 | |
Forbes, J. M., and Cooper, M. E. (2013). Mechanisms of diabetic complications. Physiol. Rev. 93 (1), 137–188. doi:10.1152/physrev.00045.2011 | |
Garay-Sevilla, M. E., Rojas, A., Portero-Otin, M., and Uribarri, J. (2021). Dietary ages as exogenous boosters of inflammation. Nutrients 13 (8), 2802–2815. doi:10.3390/nu13082802 | |
Gugliucci, A. (2017). Formation of fructose-mediated advanced glycation end products and their roles in metabolic and inflammatory diseases. Adv. Nutr. 8 (1), 54–62. doi:10.3945/an.116.013912 | |
Gui, F., You, Z., Fu, S., Wu, H., and Zhang, Y. (2020). Endothelial dysfunction in diabetic retinopathy. Front. Endocrinol. 11 (591), 591–616. doi:10.3389/fendo.2020.00591 | |
Guo, H., and Xu, Y. (2017). Role of advanced glycation end products in the progression of diabetes mellitus. Glob. J. Obes. Diabetes Metab. Syndr. 4 (6), 024–035. doi:10.17352/2455-8583.000019 | |
Haddad, M., Knani, I., Bouzidi, H., Berriche, O., Hammami, M., and Kerkeni, M. (2016). Plasma levels of pentosidine, carboxymethyl-lysine, soluble receptor for advanced glycation end products, and metabolic syndrome: The metformin effect. Dis. Markers 2016, 6248264. doi:10.1155/2016/6248264 | |
Hangai, M., Takebe, N., Honma, H., Sasaki, A., Chida, A., Nakano, R., et al. (2016). Association of advanced glycation end products with coronary artery calcification in Japanese subjects with type 2 diabetes as assessed by skin autofluorescence. J. Atheroscler. Thromb. 23, 1178–1187. doi:10.5551/jat.30155 | |
Hashim, Z., and Zarina, S. (2017). Advanced glycation end products in diabetic and non-diabetic human subjects suffering from cataract. Age 33 (3), 377–384. doi:10.1007/s11357-010-9177-1 | |
Hellwig, M., and Henle, T. (2014). Baking, ageing, diabetes: A short history of the maillard reaction. Angew. Chem. Int. Ed. Engl. 53 (39), 10316–10329. doi:10.1002/anie.201308808 | |
Hinz, M., and Scheidereit, C. (2014). The IκB kinase complex in NF-κB regulation and beyond. EMBO Rep. 15 (1), 46–61. doi:10.1002/embr.201337983 | |
Hong Sheng, C., So Ha, T., and Khalid Abdul, K. (2017). Therapeutic agents targeting at AGE-RAGE Axis for the treatment of diabetes and cardiovascular disease: A review of clinical evidence. Clin. Diabetes Res. 1 (1), 16–34. doi:10.36959/647/490 |
Hong Sheng, C., So Ha, T., and Khalid Abdul, K. (2017). Therapeutic agents targeting at AGE-RAGE Axis for the treatment of diabetes and cardiovascular disease: A review of clinical evidence. Clin. Diabetes Res. 1 (1), 1–17. doi:10.36959/647/490 |
Huang, W. C., and Hung, M. C. (2013). Beyond NF-κB activation: nuclear functions of IκB kinase α. J. Biomed. Sci. 20 (3). doi:10.1186/1423-0127-20-3 | |
Huang, H. (2020). Pericyte-endothelial interactions in the retinal microvasculature. Int. J. Mol. Sci. 21 (19), E7413–E7418. doi:10.3390/ijms21197413 | |
Indyk, D., Bronowicka-Szydełko, A., Gamian, A., and Kuzan, A. (2021). Advanced glycation end products and their receptors in serum of patients with type 2 diabetes. Sci. Rep. 11 (1), 13264–13314. doi:10.1038/s41598-021-92630-0 | |
Jud, P., and Sourij, H. (2019). Therapeutic options to reduce advanced glycation end products in patients with diabetes mellitus: A review. Diabetes Res. Clin. Pract. 148, 54–63. doi:10.1016/j.diabres.2018.11.016 | |
Kay, A. M., Simpson, C. L., and Stewart, J. A. (2016). The role of AGE/RAGE signaling in diabetes-mediated vascular calcification. J. Diabetes Res. 8 (5), 6809703–6809756. doi:10.1155/2016/6809703 | |
Khalid, M., Petroianu, G., and Adem, A. (2022). Advanced glycation end products and diabetes mellitus: Mechanisms and perspectives. Biomolecules 12 (4), 542. doi:10.3390/biom12040542 | |
Khangholi, S., Majid, F. A. A., Berwary, N. J. A., Ahmad, F., and Aziz, R. B. A. (2015). The mechanisms of inhibition of advanced glycation end products formation through polyphenols in hyperglycemic condition. Planta Med. 82 (2), 32–45. doi:10.1055/s-0035-1558086 | |
Kim, C-S., Park, S., and Kim, J. (2017). The role of glycation in the pathogenesis of aging and its prevention through herbal products and physical exercise. J. Exerc. Nutr. Biochem. 21 (3), 55–61. doi:10.20463/jenb.2017.0027 | |
Knott, H. M., Brown, B. E., Davies, M. J., and Dean, R. T. (2019). Glycation and glycoxidation of low-density lipoproteins by glucose and low-molecular mass aldehydes. Formation of modified and oxidized particles. Eur. J. Biochem. 270 (17), 3572–3582. doi:10.1046/j.1432-1033.2003.03742.x | |
Komplikacija, V., and Tipa, U. D. (2015). The effect of hyperglycemia and oxidative stress on the development and progress of vascular complications in type 2 diabetes. JMB 28 (2), 63–71.
Kopytek, M., Za¸bczyk, M., Mazur, P., Undas, A., and Natorska, J. (2020). Accumulation of advanced glycation end products (AGEs) is associated with the severity of aortic stenosis in patients with concomitant type 2 diabetes. Cardiovasc Diabetol. 19, 1–12. [Internet]. doi:10.1186/s12933-020-01068-7 | |
Koska, J., Saremi, A., Courten, B. D., Ginsberg, H., Reaven, P. D., Howell, S., et al. (2017). Advanced glycation end products, oxidation products, and incident cardiovascular events in patients with type 2 diabetes. Diabetes Care 41 (6), 570–576. doi:10.2337/dc17-1740 | |
Koska, J., Saremi, A., Howell, S., Bahn, G., De Courten, B., Ginsberg, H., et al. (2018). Advanced glycation end products, oxidation products, and incident cardiovascular events in patients with type 2 diabetes. Diabetes Care 41 (3), 570–576. doi:10.2337/dc17-1740 | |
Kuzan, A. (2021). Toxicity of advanced glycation end products (Review). Biomed. Rep. 14 (5), 46–48. doi:10.3892/br.2021.1422 | |
Lin, Y. C., Chang, Y. H., Yang, S. Y., Wu, K. D., and Chu, T. S. (2018). Update of pathophysiology and management of diabetic kidney disease. J. Formos. Med. Assoc. 117 (8), 662–675. [Internet]. doi:10.1016/j.jfma.2018.02.007 | |
Loeffler, I., and Wolf, G. (2015). Epithelial-to-Mesenchymal transition in diabetic nephropathy: Fact or fiction? Cells 4 (4), 631–652. doi:10.3390/cells4040631 | |
Mastrocola, R., Collotta, D., Gaudioso, G., Le Berre, M., Cento, A. S., Ferreira, G. A., et al. (2020). Effects of exogenous dietary advanced glycation end products on the cross-talk mechanisms linking microbiota to metabolic inflammation. Nutrients 12 (9), 24977–E2520. doi:10.3390/nu12092497 |
Mathebula, S. D. (2015). Polyol pathway: A possible mechanism of diabetes complications in the eye. Afr. Vis. Eye Health 74 (1), 1–5. doi:10.4102/aveh.v74i1.13 |
Milstone, D. S., Ilyama, M., Chen, M., Donnell, P. O., Davis, V. M., Plutzky, J., et al. (2016). Differential role of a NF-kappaB transcriptional response element in endothelial versus intimal cell VCAM-1 expression david. HHS Public Access 117 (2), 166–177. doi:10.1161/CIRCRESAHA.117.306666 | |
Moldogazieva, N. T., Mokhosoev, I. M., Mel’Nikova, T. I., Porozov, Y. B., and Terentiev, A. A. (2019). Oxidative stress and advanced lipoxidation and glycation end products (ALEs and AGEs) in aging and age-related diseases. Oxid. Med. Cell. Longev. 2019, 3085756–3085814. doi:10.1155/2019/3085756 | |
Moore, T. C. B., Moore, J. E., Kaji, Y., Frizzell, N., Usui, T., Poulaki, V., et al. (2003). The role of advanced glycation end products in retinal microvascular leukostasis. Invest. Ophthalmol. Vis. Sci. 44 (10), 4457–4464. doi:10.1167/iovs.02-1063 | |
Nenna, A., Nappi, F., Avtaar Singh, S., Sutherland, F., Di Domenico, F., Chello, M., et al. (2015). Pharmacologic approaches against advanced glycation end products (AGEs) in diabetic cardiovascular disease. Res. Cardiovasc. Med. 4 (2), e26949. doi:10.5812/cardiovascmed.4(2)2015.26949 | |
Ng, Z. X., Kuppusamy, U. R., Tajunisah, I., Fong, K. C. S., Koay, A. C. A., and Chua, K. H. (2012). 2245G/A polymorphism of the receptor for advanced glycation end-products (RAGE) gene is associated with diabetic retinopathy in the Malaysian population. Br. J. Ophthalmol. 96 (2), 289–292. doi:10.1136/bjophthalmol-2011-300658 | |
Ng, Z. X., Kuppusamy, U. R., Tajunisah, I., Fong, K. C. S., and Chua, K. H. (2012). Association analysis of -429T/C and -374T/A polymorphisms of receptor of advanced glycation end products (RAGE) gene in Malaysian with type 2 diabetic retinopathy. Diabetes Res. Clin. Pract. 95 (3), 372–377. doi:10.1016/j.diabres.2011.11.005 | |
Ng, Z. X., Kuppusamy, U. R., Poh, R., Tajunisah, I., Koay, A. C., Fong, K. C., et al. (2012). Lack of association between Gly82Ser, 1704G/T and 2184A/G of RAGE gene polymorphisms and retinopathy susceptibility in Malaysian diabetic patients. Genet. Mol. Res. 11 (1), 455–461. doi:10.4238/2012.March.1.2 | |
Ng, Z. X., Chua, K. H., Iqbal, T., and Kuppusamy, U. R. (2013). Soluble receptor for advanced glycation end-product (sRAGE)/pentosidine ratio: A potential risk factor determinant for type 2 diabetic retinopathy. Int. J. Mol. Sci. 14 (4), 7480–7491. doi:10.3390/ijms14047480 | |
Ng, Z. X., Kuppusamy, U. R., Iqbal, T., and Chua, K. H. (2013). Receptor for advanced glycation end-product (RAGE) gene polymorphism 2245G/A is associated with pro-inflammatory, oxidative-glycation markers and sRAGE in diabetic retinopathy. Gene 521 (2), 227–233. doi:10.1016/j.gene.2013.03.062 | |
Ng, Z. X., Chua, K. H., Tajunisah, I., Pendek, R., and Kuppusamy, U. R. (2013). Attenuated levels of pro-inflammatory markers in diabetic retinopathy patients undergoing treatment with antihyperglycemic and antihypertensive drugs. Clinics 68 (2), 185–193. doi:10.6061/clinics/2013(02)oa11 | |
Nishad, R., Tahaseen, V., Kavvuri, R., Motrapu, M., Singh, A. K., Peddi, K., et al. (2021). Advanced-glycation end-products induce podocyte injury and contribute to proteinuria. Front. Med. 8, 685447–685511. doi:10.3389/fmed.2021.685447 |
Nowotny, K., Jung, T., Höhn, A., Weber, D., and Grune, T. (2015). Advanced glycation end products and oxidative stress in type 2 diabetes mellitus. Biomolecules 5 (1), 194–222. doi:10.3390/biom5010194 | |
Ott, C., Jacobs, K., Haucke, E., Navarrete Santos, A., Grune, T., and Simm, A. (2014). Role of advanced glycation end products in cellular signaling. Redox Biol. 2 (1), 411–429. doi:10.1016/j.redox.2013.12.016 | |
Papachristou, S., Pafili, K., Trypsianis, G., Papazoglou, D., Vadikolias, K., and Papanas, N. (2021). Skin advanced glycation end products among subjects with type 2 diabetes mellitus with or without distal sensorimotor polyneuropathy. J. Diabetes Res. 2021, 1–7. doi:10.1155/2021/6045677 |
Park, S., Kang, H. J., Jeon, J. H., Kim, M. J., and Lee, I. K. (2019). Recent advances in the pathogenesis of microvascular complications in diabetes. Arch. Pharm. Res. 42 (3), 252–262. doi:10.1007/s12272-019-01130-3 | |
Parveen, A., Sultana, R., Lee, S. M., Kim, T. H., and Kim, S. Y. (2021). Phytochemicals against anti-diabetic complications: targeting the advanced glycation end product signaling pathway. Arch. Pharm. Res. 44 (4), 378–401. doi:10.1007/s12272-021-01323-9 | |
Parwani, K. (2017). Association of advanced glycation end products ( AGEs ) with diabetic nephropathy and alcohol consumption. J. Alcohol Drug Depend. 5 (6), 232–488. doi:10.4172/2329-6488.1000290 | |
Pasupulati, A. K., Chitra, P. S., and Reddy, G. B. (2016). Advanced glycation end products mediated cellular and molecular events in the pathology of diabetic nephropathy. Biomol. Concepts 7 (6), 293–309. doi:10.1515/bmc-2016-0021 | |
Perrone, A., Giovino, A., Benny, J., and Martinelli, F. (2020). Advanced glycation end products (AGEs): Biochemistry, signaling, analytical methods, and epigenetic effects. Oxid. Med. Cell. Longev. 20 (6), 3818196–3818213. doi:10.1155/2020/3818196 | |
Popova, E. A., Mironova, R. S., and Odjakova, M. K. (2010). Non-enzymatic glycosylation and deglycating enzymes. Biotechnol. Biotechnol. Equip. 24 (3), 1928–1935. doi:10.2478/v10133-010-0066-7 |
Punthakee, Z., Goldenberg, R., and Katz, P. (2018). Definition, classification and diagnosis of diabetes, prediabetes and metabolic syndrome. Can. J. Diabetes 42, S10–S15. doi:10.1016/j.jcjd.2017.10.003 | |
Qayyum, S., Afzal, M., and Naveed, A. K. (2021). Association analysis of -429T/C receptor for advanced glycation end products (RAGE) gene polymorphism with type 2 diabetic retinopathy and serum soluble RAGE levels in Pakistani patients. J. Pak. Med. Assoc. 71 (4), 1175–1180. doi:10.47391/JPMA.01-184 | |
Quade-Lyssy, P., Kanarek, A. M., Baiersdörfer, M., Postina, R., and Kojro, E. (2013). Statins stimulate the production of a soluble form of the receptor for advanced glycation end products. J. Lipid Res. 54 (11), 3052–3061. doi:10.1194/jlr.M038968 | |
Rabbani, G., and Ahn, S. N. (2019). Structure, enzymatic activities, glycation and therapeutic potential of human serum albumin: A natural cargo. Int. J. Biol. Macromol. 123, 979–990. doi:10.1016/j.ijbiomac.2018.11.053 | |
Rashid, G., Benchetrit, S., Fishman, D., and Bernheim, J. (2004). Effect of advanced glycation end-products on gene expression and synthesis of TNF-α and endothelial nitric oxide synthase by endothelial cells. Kidney Int. 66 (3), 1099–1106. doi:10.1111/j.1523-1755.2004.00860.x | |
Rhee, S. Y., and Kim, Y. S. (2018). The role of advanced glycation end products in diabetic vascular complications. Diabetes Metab. J. 42 (3), 188–195. doi:10.4093/dmj.2017.0105 | |
Rigalleau, V., Cougnard-Gregoire, A., Nov, S., Gonzalez, C., Maury, E., Lorrain, S., et al. (2015). Association of advanced glycation end products and chronic kidney disease with macroangiopathy in type 2 diabetes. J. Diabetes Complicat. 29 (2), 270–274. doi:10.1016/j.jdiacomp.2014.10.011 | |
Rujman Khan, M. P. (2016). Advanced glycation end products: Formation, role in diabetic complications. Intech 1 (6), 13–26. [Internet]Available from: https://www.intechopen.com/books/advanced-biometric-technologies/liveness-detection-in-biometrics.
Safi, S. Z., Qvist, R., Kumar, S., Batumalaie, K., Shah, I., and Ismail, B. (2014). Molecular mechanisms of diabetic retinopathy , general preventive strategies , and novel therapeutic targets. Biomed. Res. Int. 2014 (8), 801269–801329. doi:10.1155/2014/801269 | |
Salahuddin, P., Rabbani, G., and Khan, R. H. (2014). The role of advanced glycation end products in various types of neurodegenerative disease: a therapeutic approach. Cell. Mol. Biol. Lett. 19 (3), 407–437. doi:10.2478/s11658-014-0205-5 | |
Senatus, L. M., and Schmidt, A. M. (2017). The AGE-RAGE axis: Implications for age-associated arterial diseases. Front. Genet. 8, 187–210. doi:10.3389/fgene.2017.00187 | |
Shin, E. S., Sorenson, C. M., and Sheibani, N. (2014). Diabetes and retinal vascular dysfunction. J. Ophthalmic Vis. Res. 9 (3), 362–373. doi:10.4103/2008-322X.143378 | |
Simó-Servat, O., Planas, A., Ciudin, A., Simó, R., and Hernández, C. (2018). Assessment of advanced glycation end-products as a biomarker of diabetic outcomes. Endocrinol. Diabetes Nutr. 65 (9), 540–545. [Internet]. doi:10.1016/j.endinu.2018.06.003 |
Singh, V. P., Bali, A., Singh, N., and Jaggi, A. S. (2014). Advanced glycation end products and diabetic complications. Korean J. Physiol. Pharmacol. 18 (5), 1–14. doi:10.4196/kjpp.2014.18.1.1 | |
Sivandzade, F., Prasad, S., Bhalerao, A., and Cucullo, L. (2019). NRF2 and NF-қB interplay in cerebrovascular and neurodegenerative disorders: Molecular mechanisms and possible therapeutic approaches. Redox Biol. 21 (9), 101059–59. doi:10.1016/j.redox.2018.11.017 | |
Sotokawauchi, A., Matsui, T., Higashimoto, Y., and Yamagishi, S. I. (2019). Fructose causes endothelial cell damage via activation of advanced glycation end products–receptor system. Diab. Vasc. Dis. Res. 16 (6), 556–561. doi:10.1177/1479164119866390 | |
Sugimoto, K., Yasujima, M., and Yagihashi, S. (2008). Role of advanced glycation end products in diabetic neuropathy. Curr. Pharm. Des. 14, 953–961. doi:10.2174/138161208784139774 | |
Suryavanshi, S. V., and Kulkarni, Y. A. (2017). NF-κβ: A potential target in the management of vascular complications of diabetes. Front. Pharmacol. 8 (7), 1–12. doi:10.3389/fphar.2017.00798 | |
Takayanagi, Y., Yamanaka, M., Fujihara, J., Matsuoka, Y., Gohto, Y., Obana, A., et al. (2020). Evaluation of relevance between advanced glycation end products and diabetic retinopathy stages using skin autofluorescence. Antioxidants 9 (11), E1100–E1112. doi:10.3390/antiox9111100 | |
Tang, W. H., Martin, K. A., and Hwa, J. (2012). Aldose reductase, oxidative stress, and diabetic mellitus. Front. Pharmacol. 3, 87–88. doi:10.3389/fphar.2012.00087 | |
Tao, D., Mai, X., Zhang, T., and Mei, Y. (2017). Association between the RAGE (receptor for advanced glycation end-products)-374T/A gene polymorphism and diabetic retinopathy in T2DM. Rev. Assoc. Med. Bras. 63 (11), 971–977. doi:10.1590/1806-9282.63.11.971 | |
Thomas, M. C., Woodward, M., Neal, B., Li, Q., Pickering, R., Marre, M., et al. (2015). Relationship between levels of advanced glycation end products and their soluble receptor and adverse outcomes in adults with type 2 diabetes. Diabetes Care 38 (10), 1891–1897. doi:10.2337/dc15-0925 | |
Tsekovska, R., Sredovska-Bozhinov, A., Niwa, T., Ivanov, I., and Mironova, R. (2016). Maillard reaction and immunogenicity of protein therapeutics. World J. Immunol. 6 (1), 19. doi:10.5411/wji.v6.i1.19 |
Uribarri, J., del Castillo, M. D., de la Maza, M. P., Filip, R., Gugliucci, A., Luevano-Contreras, C., et al. (2015). Dietary advanced glycation end products and their role in health and disease. Adv. Nutr. 6 (4), 461–473. doi:10.3945/an.115.008433 | |
Vadakedath, S., and Kandi, V. (2018). Role of advanced glycation end products ( AGE ) in health and disease : An overview. Biochem. Physio. 7 (4), 246–252. doi:10.4172/2168-9652.1000246 |
Wu, T. H., Tsai, S. C., Lin, H. W., Chen, C. N., and Hwu, C. M. (2022). Increased serum levels of advanced glycation end products are negatively associated with relative muscle strength in patients with type 2 diabetes mellitus. BMC Endocr. Disord. 22 (1), 118–119. doi:10.1186/s12902-022-01035-1 | |
Xing, Y., Pan, S., Zhu, L., Cui, Q., Tang, Z., Liu, Z., et al. (2022). Advanced glycation end products induce atherosclerosis via RAGE/TLR4 signaling mediated-M1 macrophage polarization-dependent vascular smooth muscle cell phenotypic conversion. Oxid. Med. Cell. Longev. 2022, 9763377–9763411. doi:10.1155/2022/9763377 | |
Xu, L., Zang, P., Feng, B., and Qian, Q. (2014). Atorvastatin inhibits the expression of RAGE induced by advanced glycation end products on aortas in healthy Sprague-Dawley rats. Diabetol. Metab. Syndr. 6 (1), 102–110. doi:10.1186/1758-5996-6-102 | |
Xu, J., Chen, L. J., Yu, J., Wang, H. J., Zhang, F., Liu, Q., et al. (2018). Involvement of advanced glycation end products in the pathogenesis of diabetic retinopathy. Cell. Physiol. biochem. 48 (2), 705–717. doi:10.1159/000491897 | |
Yamagishi, S., and Matsui, T. (2018). Role of hyperglycemia-induced advanced glycation end product ( AGE ) accumulation in atherosclerosis. Ann. Vasc. Dis. 11 (3), 253–258. doi:10.3400/avd.ra.18-00070 | |
Yaw, K. C., Basir, R., Talib, H., Tung, H. T., and Nordin, N. (2013). Receptor for advanced glycation end products and its involvement in inflammatory diseases. Int. J. Inflam. 2013, 1. [Internet]Available from: http://www.ncbi.nlm.nih.gov/pubmed/24102034%0Ahttp://www.pubmedcentral.nih.gov/articlerender.fcgi?artid=PMC3786507%0Ahttp://www.ncbi.nlm.nih.gov/pubmed/24506934.
Ying, L., Shen, Y., Zhang, Y., Wang, Y., Liu, Y., Yin, J., et al. (2021). Association of advanced glycation end products with diabetic retinopathy in type 2 diabetes mellitus. Diabetes Res. Clin. Pract. 177, 108880. doi:10.1016/j.diabres.2021.108880 | |
Ying, L., Shen, Y., Zhang, Y., Wang, Y., Liu, Y., Yin, J., et al. (2021). Advanced glycation end products via skin autofluorescence as potential marker of carotid atherosclerosis in patients with type 2 diabetes. Nutr. Metab. Cardiovasc. Dis. 31 (12), 3449–3456. doi:10.1016/j.numecd.2021.09.005 | |
Yu, H., Seow, Y. X., Ong, P. K. C., and Zhou, W. (2018). Kinetic study of high-intensity ultrasound-assisted Maillard reaction in a model system of D-glucose and glycine. Food Chem. 269, 628–637. doi:10.1016/j.foodchem.2018.07.053 | |
Zhan, J., Chen, C., Wang, D. W., and Li, H. (2022). Hyperglycemic memory in diabetic cardiomyopathy. Front. Med. 16 (1), 25–38. doi:10.1007/s11684-021-0881-2 | |
Zhang, Q., Ames, J. M., Smith, R. D., Baynes, J. W., and Metz, T. O. (2010). A perspective on the maillard reaction and the analysis of protein glycation by mass spectrometry: Probing the pathogenesis of chronic disease. J. Proteome Res. 8 (2), 754–769. doi:10.1021/pr800858h | |
Zhang, S., Ma, P., and Chen, Q. (2022). The correlation between the level of skin advanced glycation end products in type 2 diabetes mellitus and the stages of diabetic retinopathy and the types of traditional Chinese medicine syndrome. Evidence-Based Complement. Altern. Med. 2022, 5193944. doi:10.1155/2022/5193944 |
Keywords: glycation, advanced glycation end products, diabetes complication, hyperglycemia, receptor advanced glycation end products
Citation: Mengstie MA, Chekol Abebe E, Behaile Teklemariam A, Tilahun Mulu A, Agidew MM, Teshome Azezew M, Zewde EA and Agegnehu Teshome A (2022) Endogenous advanced glycation end products in the pathogenesis of chronic diabetic complications. Front. Mol. Biosci. 9:1002710. doi: 10.3389/fmolb.2022.1002710
Received: 25 July 2022; Accepted: 01 September 2022;
Published: 15 September 2022.
Edited by:
Saurabh Awasthi, Université de Fribourg, SwitzerlandReviewed by:
Palash Mandal, Charotar University of Science and Technology, IndiaZhi Xiang Ng, University of Nottingham Malaysia Campus, Malaysia
Savita Bansal, University of Delhi, India
Copyright © 2022 Mengstie, Chekol Abebe, Behaile Teklemariam, Tilahun Mulu, Agidew, Teshome Azezew, Zewde and Agegnehu Teshome. This is an open-access article distributed under the terms of the Creative Commons Attribution License (CC BY). The use, distribution or reproduction in other forums is permitted, provided the original author(s) and the copyright owner(s) are credited and that the original publication in this journal is cited, in accordance with accepted academic practice. No use, distribution or reproduction is permitted which does not comply with these terms.
*Correspondence: Misganaw Asmamaw Mengstie, bWlzZ2FuYXcxMThAZ21haWwuY29t,