- 1School of Medicine, Shanxi Datong University, Datong, China
- 2Key Laboratory of Molecular Target and Clinical Pharmacology and the State Key Laboratory of Respiratory Disease, School of Pharmaceutical Sciences and the Fifth Affiliated Hospital, Guangzhou Medical University, Guangzhou, China
- 3Xinjiang Institute of Materia Medica, Urumqi, China
- 4Department of Biomedical Sciences, School of Medicine, Nazarbayev University, Nur-Sultan, Kazakhstan
- 5School of Medicine, Shahid Beheshti University of Medical Sciences, Tehran, Iran
SARS-CoV-2 infection has become an urgent public health concern worldwide, severely affecting our society and economy due to the long incubation time and high prevalence. People spare no effort on the rapid development of vaccine and treatment all over the world. Amongst the numerous ways of tackling this pandemic, some approaches using extracellular vesicles (EVs) are emerging. In this review, we summarize current prevalence and pathogenesis of COVID-19, involving the combination of SARS-CoV-2 and virus receptor ACE2, endothelial dysfunction and micro thrombosis, together with cytokine storm. We also discuss the ongoing EVs-based strategies for the treatment of COVID-19, including mesenchymal stem cell (MSC)-EVs, drug-EVs, vaccine-EVs, platelet-EVs, and others. This manuscript provides the foundation for the development of targeted drugs and vaccines for SARS-CoV-2 infections.
Overview of COVID-19
Current Prevalence of COVID-19
COVID-19, named Coronavirus Infectious Disease 2019 by the World Health Organization (Zhou, et al., 2020a; Zhu, et al., 2020), is an infectious disease caused by the severe acute respiratory syndrome coronavirus type 2 (SARS-CoV-2), which was first reported in Wuhan, China, in December 2019. COVID-19 infection is now a pressing global public health problem. As of today, 23 March 2021, more than 124 million people have been infected with SARS-CoV-2 and more than 2.7 million have died. In addition to having an unprecedented impact on global health care systems, COVID-19 has profound socioeconomic consequences (Nicola, et al., 2020).
Clinically, COVID-19 mainly affects the lungs. Although the majority of COVID-19 victims may show asymptomatic or mild symptoms, interstitial pneumonia (IP) and acute respiratory distress syndrome (ARDS) requiring mechanical ventilation in the intensive care units can occur in approximately 15% of cases (Cascella, et al., 2021), especially in the elderly and individuals with underlying diseases. COVID-19 also has systemic manifestations, affecting multiple organ systems containing cardiovascular, gastrointestinal, hematopoietic, renal, and immune systems (Yi, et al., 2020). In severe cases, COVID-19 can lead to severe cytokine storm or cytokine release syndrome (CRS) (Cascella, Rajnik, 2021), sepsis, multiple organ failure and even death.
SARS-CoV-2 is by far the seventh human coronavirus discovered to date. Four viruses (HCoV-NL63, HCoV-229E, HCoV-OC43, and HKU1) persist in human population and cause mild common cold symptoms (Fung,and Liu, 2019). The other two are similar to SARS-CoV-2, named SARS-CoV and Middle East Respiratory Syndrome (MERS)-CoV, and both of them lead to acute respiratory disease (Cui, et al., 2019). SARS-CoV-2 is a spherical or pleomorphic enveloped virus particle with a typical diameter range of 80–120 nm. This virus contains a 30 kB positive single-stranded RNA which surrounded by a membrane embedded with a variety of viral proteins, especially the Spike (S) protein (Mousavizadeh, and Ghasemi, 2020). Studies shown that the Spike protein in SARS-CoV-2 shared a high degree of structural homology with that in SARS-CoV (Li, et al., 2005; Xu, et al., 2020b). SARS-CoV-2 virus infect into human cells by recognizing the angiotensin converting enzyme 2 (ACE2) receptor of host cells. A recent study by Wan et al. reported that SARS-CoV-2 had a stronger binding ability with ACE2 than with SARS-CoV, promoting the infection and transmission capacity of the virus (Wan, et al., 2020).
Pathogenesis of COVID-19
ACE2 Plays a Key Role in SARS-CoV-2 Invasion
In 2020, using cryopreserved electron microscopy, Zhou Qiang Laboratory of Westlake University successfully analyzed the full-length structure of ACE2 (Yan, et al., 2020), the receptor protein of SARS-CoV-2. This is the first time of the world that the full-length structure of ACE2 has been resolved. As SARS-CoV-2 invades the body, ACE2 acts like a “doorknob,” the virus grabs it and opens the door to the recipient cells. In addition, interestingly, it has recently been demonstrated that transmembrane protease serine 2 (TMPRSS2) incises the Spike protein during the internalization of SARS-CoV-2 which fuses with host cell membrane (Scheller, et al., 2019). This process is necessary for SARS-CoV-2 to enter into recipient cells (Devaux, et al., 2020). After fusing with the human cell membrane, the virus genome enters into the recipient/host cell. The virus then begins to replicate, mature and leave the host cells to infect new healthy cells. SARS-CoV-2 enters the respiratory system from the upper respiratory tract and eventually infects alveolar cells, causing angiectasis of alveolar cells, increased capillary permeability, decreased pulmonary surface active substances with infiltration of lymphocytes and monocytes. As ACE2 and TMPRSS2 are abundant in type II alveolar and endothelial cells (Hamming, et al., 2004), pulmonary vessels are susceptible to SARS-CoV-2-induced inflammation and injury (Zhao, et al., 2020). Other than the respiratory tract, SARS-CoV-2 infection can cause parts of the body damage, such as the cardiovascular system. Therefore, COVID-19 patients may present with severe forms of myocarditis and endocarditis besides respiratory dysfunction (Guzik, et al., 2020).
ACE2 is located in various types of epithelial cells (lung, kidney, heart, intestinal) and endothelial cells. In recent years, studies have shown that ACE2 played roles in the cardiovascular, renal and respiratory system, and was associated with hypertension and diabetes. ACE2 has a protective effect on a variety of lung diseases, such as acute lung injury, asthma, ARDS, pulmonary hypertension and chronic obstructive pulmonary disease (Jia, 2016). More importantly, ACE2 was identified as a SARS-CoV and SARS-CoV-2 receptor, which played a protective role in the pathogenesis of SARS and COVID-19. As the first homolog of ACE, ACE2 regulates the renin angiotensin system (RAS) by balancing the ACE activity. The binding complex of Spike protein with ACE2 can induce the cell membrane ACE2 down-regulation (Glowacka, et al., 2010), contributing to the imbalance of ACE and ACE2 activity, and therefore leading to acute lung injury (Zhang, et al., 2020a).
COVID-19 Is Associated With Endothelial Dysfunction and Micro-thrombosis
Patients with COVID-19 have a high incidence of thrombotic events (Berger, et al., 2020). About the mechanism of thrombosis in COVID-19, there are a lot of speculations, such as coagulation and platelet activation, endothelial cell activation, inflammation and complement system activation, etc (Mackman, et al., 2020). Varga and colleagues demonstrated that SARS-CoV-2 presented in the endothelial cells of various human organs (Varga, et al., 2020). In addition, evidence of alveolar capillary micro-thrombosis and endothelial injury associated with intracellular viruses has been noted in autopsy analyses of infected lungs (Ackermann, et al., 2020). Due to ACE2 show high-expression in vascular endothelial cells, viral infection of the circulatory system directly leads to excessive coagulation in COVID-19 patients (Varga, Flammer, 2020). In addition, the number of peripheral blood mononuclear cells decreased significantly in patients with respiratory failure 7–14 days after the onset of SARS-CoV-2 infection (Yi, Lagniton, 2020). High level of D-dimer was found to presence for the moment, which was associated with severe hypercoagulation and indicated a poor prognosis (Yi, Lagniton, 2020). As a major activator of the coagulation cascade (Grover, and Mackman, 2018), several studies have speculated that the induction of tissue factor (TF) might play an important role in the COVID-19-related thrombosis (Bautista-Vargas, et al., 2020; Grover and Mackman, 2018; Mackman, Antoniak, 2020). Axel Rosell et al. developed a method to determine the activity of TF in plasma EVs in 100 patients with moderate to severe COVID-19. The results showed that the level of EVs-TF activity was significantly higher in COVID-19 patients than in the normal individuals. In addition, the level of EVs-TF activity was associated with disease severity, mortality, and several plasma markers, including D-dimer. These findings suggest that SARS-CoV-2 infection induces the release of TF-positive EVs into the circulation, and possibly cause thrombosis in patients infected with COVID-19 (Rosell, et al., 2020).
Cytokine Storm: Immune Response to SARS-CoV-2 Is a Major Driving Force of Disease Severity
Because SARS-CoV-2 entry into cells depend on binding to its receptor, ACE2, the RAS and various inflammatory cascades are connected with the pathobiology of COVID-19 (Bourgonje, et al., 2020). The SARS-CoV-2 can also activate the innate and adaptive immune response in patients with COVID-19 (Yang, et al., 2020a). The immune effector cells release a large amount of proinflammatory cytokines and chemokines (Tukmechi, et al., 2014), such as tumor necrosis factor (TNF), interleukin 1 (IL-1), interleukin 6 (IL-6), interleukin 7 (IL-7) and granulocyte colony stimulating factor (Pedersen and Ho, 2020), inducing an uncontrolled CRS, and leading to various clinical manifestations, such as high fever, hepatosplenomegaly, cytopenia, central nervous system abnormalities, hypoalbuminaemia and capillary leakage (England, et al., 2021; Gao, et al., 2021). A previously published study showed that identifying circulating protein biomarkers in COVID-19 patients used an ultra-high-throughput serum and plasma proteomics technology (Messner, et al., 2020). Recently, Balaji Krishnamachary et al. indicated that EVs from patients affected by the SARS-CoV-2 might alter the pro-inflammatory response, blood coagulation disorders, and endothelium damage. They found that EVs of serious cases of COVID-19 carried higher levels of cytokines, including the IL-6 family, TNF superfamily, chemokines (MCP-1 and CXCL16), and proteases and peptidases (Cathepsin L1), compared with that of patients with moderate COVID-19 or asymptomatic individuals (Krishnamachary, et al., 2020). Therefore, the use of appropriate immunosuppressive and immunomodulatory agents to address the potential inflammatory complications of COVID-19 is currently being explored, which might improve clinical outcomes and ultimately reduce COVID-19 mortality (Stebbing, et al., 2020). Because ACE2 receptors are widely distributed in human alveolar type II cells and capillary endothelial cells (Hamming, Timens, 2004), the lungs are exceptionally sensitive to SARS-CoV-2 infection. In fact, recently published researches have shown that a substantial portion of the lungs were impacted by this disease, leading to an extensive damage that could subsequently result in permanent change of lung function. A recent MRI study in a 59-year-old man who diagnosed with COVID-19, suggested that this disease was not restricted to any specific area in the lung, but spread to the entire lung. In fact, this symptom is not composed directly arouse the SARS-CoV-2 virus, but a host immune response that causes a resistless cytokine storm in lungs. Overexpression of cytokines such as the interleukin family (IL-2, IL-6, IL-7), GSCF, IP10, MCP1, MIP1A, and TNF-α leads to edema and impairs oxygen exchange, which may lead to ARDS with potential acute cardiac injury, secondary infection and death (Huang, et al., 2020a).
EVs Are the Promising New Therapeutic Means of COVID-19
There is currently no specific therapeutic for COVID-19. Conventional treatment includes infection prevention, supportive care that invloving supplement of oxygen and mechanical ventilation support (Grasselli, et al., 2020; Marini, and Gattinoni., 2020). Currently, drugs against COVID-19 are evaluated worldwide, including antiviral drugs, anti-malarial drugs and anti-inflammatory drugs, such as radecivir (Beigel, et al., 2020), chloroquine (Zhou, et al., 2020b) and hydroxychloroquine (HCQ) (Soy, et al., 2020), anthropoized anti-IL-6 receptor antibody tocilizumab (Perrone, et al., 2020; Toniati, et al., 2020; Xu, et al., 2020c), recombinant human IL-1 receptor antagonist Anakinra (Cavalli, et al., 2020; Huet, et al., 2020), etc. Although these treatment strategies improved patient recovery and survival, they do not definitively restore lung damage caused by the virus. In addition, a range of anti-inflammatory drugs have been tested to inhibit the cytokine storm and multiple organ failure caused by the worsening immune response in severe patients, but the effect has not been significant. In recent years, increasing researches reported that the role of EVs in the treatment of inflammation (Lasser, et al., 2016; Martinez-Bravo, et al., 2017), injury (Lanyu, and Feilong, 2019), and lung and respiratory viral infection (Scheller, Herold, 2019; van Dongen, et al., 2016; Yoshikawa, et al., 2019). It was reported that EVs promoted the pathogenesis of diseases such as in infectious diseases and cancers (Fleming, et al., 2014; Han, et al., 2019). The interesting interaction between EVs and the virus provides a new perspective on the treatment of COVID-19 (Dogrammatzis, et al., 2020). Viral infection may affect the exosomal-loading mechanisms of the host cells, resulting in changes in protein and nucleic acid content of EVs. It means the infected cells release modified EVs, not rely on virus contents. Therefore, compared with EVs without infected cells, these modified EVs may modulate the host immune response. Besides, EVs may act as a negatively regulatory element in the transmission of viral infection, and induce the immune system to respond to the virus. There are three types of EVs, including exosomes (20–150 nm), microvesicles (MVs) (100–1,000 nm in diameter), and apoptotic vesicles (1,000–5,000 nm) (Maione, et al., 2020; Raposo and Stoorvogel, 2013). EVs, as a carrier for cell-to-cell transfer of biomolecules, is an important mode of cell-to-cell communication (Huang, et al., 2020b). EVs are present in a variety of biological fluids, such as blood, tissue fluid, pleural fluid, bronchoalveolar lavage fluid (BAL), peritoneal fluid, saliva, urine, breast milk, cerebrospinal fluid, amniotic fluid and so on (Kowal, et al., 2014; Rezaie, et al., 2019). EVs can be transported or accumulated not only in biological liquids but also in solid tissues. In solid tissue, EVs deliver their contents, such as proteins, miRNAs, mRNAs, and lncRNAs, to adjacent or distant cells and reprogram the target cells in fate, function, and morphology, resulting in physiological or pathological effects (Kowal, Tkach, 2014; Maas, et al., 2017; Statello, et al., 2018).
Exosomes, also referred to as intraluminal vesicles (ILVs), are a subtype of EV formed by an endosomal route, which are surrounded by a phospholipid bilayer, and have been found in biological liquids (Raposo and Stoorvogel, 2013). Exosomal vesicles form through inward budding of the limiting membrane of early endosomes, which mature into multivesicular bodies (MVBs) (Huang, Yan, 2020b). MVBs play a significant role in the endocytic and trafficking functions of the cell material, such as protein sorting, recycling, storage, transport, and release (Babaei, and Rezaie, 2021). Release of exosomes into the extracellular space is facilitated by the fusion of the MVB limiting membrane with the plasma membrane. Researches showed that exosomes participated in cell communication, cell maintenance, and tumor progression (Rezaie, et al., 2021). Balaji Krishnamachary et al. explored EVs isolated from plasma of patients with COVID-19 to identify some potential biomarkers which influence the disease severity and to analyze its role in the pathogenesis of this disease (Krishnamachary, Cook, 2020). Plasma-derived EVs were separated from 53 COVID-19 patients in hospital and compared depending on the severity of their disease. Analysis of inflammation and cardiovascular protein loading in large EVs suggested significant differences in protein expression across disease subgroups. Prominently, the TNF superfamily and IL-6 family members were upregulated in severe and moderate disease patients who need oxygen supplement. EVs in severe patients also shown enhancement of prothrombotic or endothelial injury factors (TF, t-PA, and VWF) and cardiovascular pathology-related proteins (MB, PRSS8, REN, and HGF). There were significantly higher levels of TF, CD163, and EN-RAGE has been observed in EVs from patients with severe disease compared to moderate disease. EVs also play a key role in transmitting viral infection (Owczarek, et al., 2018). It was found that EVs and viruses shared similar physicochemical properties. They are small in size and have common biogenesis and cell entry mechanisms (van Dongen, Masoumi, 2016). The virus enters the uninfected cell via the endocytosis pathway and then exits the host cell by budding directly through the cell membrane. A new study found that EVs acted as the tool of virus export to cell, and the EVs depend virus entry mechanisms for cargo transport. EVs are the delivery vectors of viral contents. EVs released from virus-infected cells can infect healthy cells by transferring viral components, for example, virus-derived miRNAs and viral proteins, etc. (Ali, et al., 2010; Arenaccio, et al., 2015; Nolte-'t Hoen, et al., 2016). EVs separated from infected cells may also activate humoral and cellular immune responses by transferring viruses and autoantigens in the host (Fleming, Sampey, 2014; Gunasekaran, et al., 2017). In addition, EVs release from virus-infected cells can also transfer viral receptors and pro-inflammatory factors to the recipient cells, leading to the transmitting of viral infection and worsening of tissue damage (Mack, et al., 2000). Studies have shown that the number of EVs secreted from the SARS-CoV-2 infected cells increased significantly during the virus infection, and EVs still played an important role in the pathogenesis of diseases (Yoshikawa, Teixeira, 2019). Lanyu et al. emphasized the role of EVs play which secreted by lung cells and alveolar epithelial cells in lung injury and inflammation (Lanyu and Feilong, 2019). According to report, EVs secreted from broncho alveolar lavage fluid are involved in the pathogenesis of idiopathic pulmonary fibrosis through the signaling regulation mediators such as Wnt5a (Martin-Medina, et al., 2018). The latest research showed EVs derived from epithelial cells which transduced by lentiviral overexpressing SARS-CoV-2 gene could transfer viral genes to recipient cardiomyocytes, leading to increased expression of inflammatory genes (Kwon, et al., 2020).
EVs may contribute to the infection, internalization and transmission of SARS-CoV-2 virus. Some components such as miRNAs, viral proteins and viral receptor ACE2 could be packed into EVs (Wang, et al., 2020), that render the recipient cells sensitive to viral invasion (Figure 1). In this review, we discuss the current EVs based COVID-19 treatment strategies, including MSC and its EVs, EVs based drug delivery systems, EVs based vaccine, platelet EVs and inhibition of EVs intake, etc.
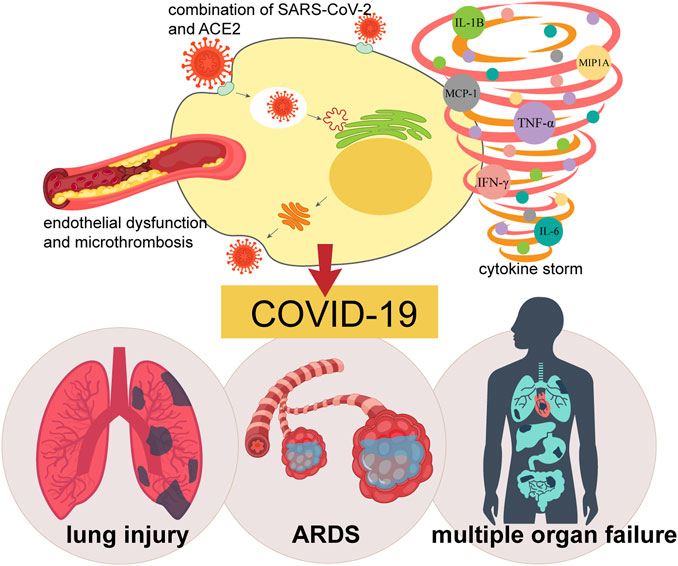
FIGURE 1. The role of EVs in SARS-CoV-2 virus internalization and infection. EVs may contribute to the transmission of SARS-CoV-2 virus. The virus may enter the recipient cells through internalization. Virus components, such as miRNAs, viral proteins and viral receptor ACE2, are packaged into the EVs and make the healthy cells sensitive to viral invasion.
EVs-based COVID-19 Therapy
MSC and MSC-EVs
MSCs are heterogeneous cells, including stromal cells, progenitor cells, fibroblasts, and stem cells (Dominici, et al., 2006; Galderisi and Giordano, 2014). The MSCs can be isolated from many tissues, such as bone marrow, placenta, adipose tissue, and umbilical cord blood. They are currently used as therapeutics at the present time, and meanwhile being tested in multiple clinical trials across the world (Meng, et al., 2020). MSCs are safe and possess immunomodulatory and tissue regeneration capabilities (Gao, et al., 2016; Thompson, et al., 2020). Studies suggested that the prospective therapeutic products based on MSCs were success, despite the apparent heterogeneity origin and lacking in specific biomarkers to predict, once implanted, MSCs could show strong regulate ability in immunomodulatory, antioxidant, and angiogenesis. MSCs and MSC-EVs have emerged as promising novel therapies that can not only reduce inflammation, but also regenerate and repair lung damage, and may therefore be used alone or in combination with other therapeutic agents to benefit patients with COVID-19.
Due to MSCs lack the expression of membrane bound molecules involved in immune rejection which enable their allogenic transplantation, the clinical applications of MSC-based therapy have witnessed an outstanding achievement (Ahmadi and Rezaie, 2021). Nevertheless, in the long-term follow up, safety issues regarding MSCs-based therapy are still a matter of debate. It is worth noting that MSCs have the capacity to differentiate into endothelial cells and to create a capillary network, and the inhibitory effect of MSCs in anti-tumor immune response results an increased tumor growth, which promotes tumor growth and metastasis (Kolios and Moodley, 2013). In addition, it was reported that local microenvironment in which MSCs engraft contained factors that induced unwanted differentiation of transplanted MSCs in vivo. Therefore, several factors and signaling pathways regarding MSCs therapy after their in vivo administration should be focused. Medical staff should abide by moral and ethical norms, so that the public and government administrators can clearly comprehend the nature and functions of MSCs, and understand the potential risks of treatment, and avoid deviating by non-standard clinical trials (Volarevic, et al., 2018).
The membrane of MSC-EVs is rich in cholesterol, sphingomyelin, ceramide and lipid raft proteins, which enables membrane fusion with target cells. After fusion, MSC-EVs may trigger signaling pathways through the receptor ligand interaction, or be internalized by endocytosis to deliver contents, such as mRNAs, miRNAs, enzymes, cytokines, etc. (Harrell, et al., 2019). Compared with homocellular transport, MSC-EVs overcome safety concerns regarding the long-term survival of engrafted MSCs due to its composition (Rezaie, et al., 2018). Thus, MSC-EVs therapy is a new remedy in cell-free treatment of autoimmune and inflammatory diseases.
Application of MSCs in Pulmonary Diseases
Inflammatory cytokines play a leading role in the development of COVID-19-caused lung injury (Figure 2). Immunotherapy that reduce the cytokine storm could be the key treatment option. However, traditional immunotherapy usually targeted one or two factors and may not produce enough response. MSCs have been shown to have powerful and extensive immunomodulatory and anti-inflammatory capabilities (Abdi, et al., 2008; Wada, et al., 2000).
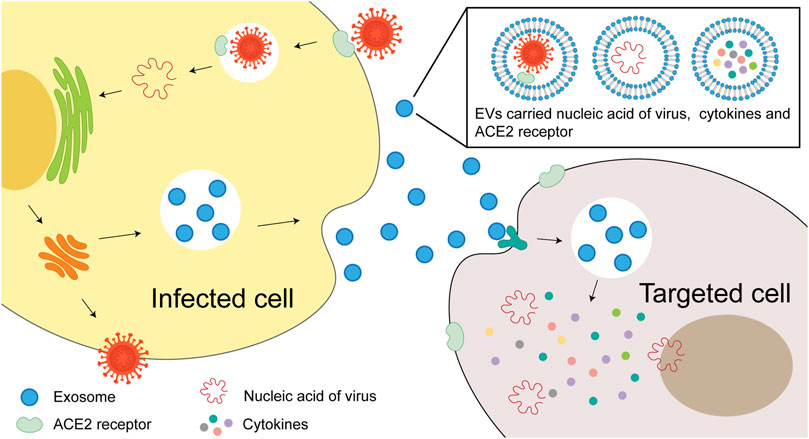
FIGURE 2. Pathogenesis of COVID-19. The combination of SARS-CoV-2 and virus receptor ACE2, endothelial dysfunction and micro thrombosis, together with cytokine storm leads to COVID-19, causing a series of clinical manifestations such as lung injury, ARDS, and even systemic multiple organ failure.
Bone marrow MSCs therapy has evolved from preclinical trials to clinical trials for different diseases states in recent years. In animal models, MSCs significantly improve lung pathological change (Cruz and Rocco, 2020) and inhibit immune cell-mediated inflammation induced by influenza virus (Khatri, et al., 2018). Bhattacharya et al. demonstrated that bone marrow MSCs could stabilize endothelial cells and maintain alveolar-capillary barrier function, which is essential for maintaining or reducing inflammation-induced lung permeability, thereby mitigating the development of interstitial pulmonary edema (Bhattacharya and Matthay, 2013). Li et al. reported that MSCs could alleviate acute lung injury caused by H9N2 and H5N1 viruses in mice by reducing the secretion of pro-inflammatory chemokines and cytokines, and inhibiting migration of inflammatory cells to the lungs (Li, et al., 2016). Currently, there are several ongoing clinical trials using MSCs for a variety of pulmonary diseases, such as obstructive bronchiolitis idiopathic pulmonary fibrosis (NCT02013700), chronic obstructive pulmonary disease (NCT01849159), and bronchial dysplasia (NCT01175655) (Wecht and Rojas, 2016), as well as for ARDS (NCT02097641) (Matthay, et al., 2019) and septic shock (NCT02421484) (McIntyre, et al., 2018). Specifically, Leng et al. found that there were no ACE2 and TMPRSS2 receptors express in MSCs, which indicated that the virus should not infect this cell population (Leng, et al., 2020). In a rat model of hyperoxia-induced lung injury, MSCs reduced overexpression of hyperoxia-induced angiotensin and angiotensin type 1 receptor (AT1R), and reduced ACE to normal level (Chen and Chou, 2018). Recently, Simonson et al. reported a long-term follow-up study on two severe ARDS patients, who required ECMO support and combined mechanical ventilation during the acute phase (Simonson, et al., 2020). After receiving a single systemic infusion of allogeneic MSCs, both patients fully recovered their physical and mental abilities. Remarkably, a dual-energy CT scan showed no signs of pulmonary fibrosis after 5 yr of MSCs treatment.
Application of MSCs in the Treatment of COVID-19
MSCs therapy is considered a promising strategy for the treatment of COVID-19. The potential of MSCs for the treatment of COVID-19 is based on two benefits: 1) induction of the regenerative program of lung epithelial and endothelial cells, and 2) synchronous modulation of the inflammatory response. MSCs secrete multiple kinds of cytokines and paracrine factors which interact with immune cells directly, including T cells, B cells, dendritic cells, macrophages, and natural killer cells. This combined effect enables MSCs have immunomodulatory capability, which contributes to suppressing overactivation of the immune system. By producing and recruiting different growth factors such as vascular endothelial growth factor (VEGF), epidermal growth factor (EGF) and transforming growth factor (TGF), MSCs promote tissue regeneration and thus improve the microenvironment (Atluri, et al., 2020). It prevents uncontrolled inflammatory cascades while reducing pulmonary fibrosis and pulmonary dysfunction following COVID-19 infection (Mo, et al., 2020; Sun, et al., 2020; Thille, et al., 2013). Particularly, the lacking of ACE2 ensures the injected MSCs achieve immunomodulatory effects free from being destroyed by virus. MSCs from human umbilical cord origin were reported in a recent clinical trial, compared to the placebo group, they were associated with the increase of peripheral lymphocyte count and the decrease of systemic inflammatory biomarkers (ChiCTR2000029990) (Leng, et al., 2020). MSC infusion alleviated cytokine storm syndrome and significantly improved outcomes in patients with severe COVID-19.
Application of MSC-EVs in Lung Diseases
Most of the therapeutic progress of MSCs are achieved through paracrine mechanisms, including EVs secretion that contain cell protective factors such as keratinocyte growth factor (KGF), anti-inflammatory mediators (PGE2 or lipid A4), anti-osmotic factors (Ang1) and others (Doorn, et al., 2012; Gnecchi, et al., 2008; Li, et al., 2014; Silini, et al., 2017). There have been reports of some limitations of using MSCs. For instance, intravenous administration of MSCs may induce particle aggregation leading to embolism; and some MSCs, especially those from embryonic tissues, may carry a risk of mutagenicity and tumorigenicity. Therefore, MSC derivatives such as SECRETOME (Bari, et al., 2019) and EVs (Tsiapalis and O’Driscoll, 2020) have been suggested as alternatives to MSC therapy. In addition, account of the low immunogenicity and tumorigenicity, simplicity of operation, and low cost, MSC-EVs therapy has significant advantages over MSC therapy. According to the report recently published, MSC-EVs could be administered by inhalation or injection (Bari, Ferrarotti, 2019). Preliminary studies have shown that MSC-EVs might also be effective against COVID-19 (Bari, et al., 2020). MSC-derived EVs have been demonstrated to induce similar influence to parent cells. In animal models, they can be store for a longer time safely without losing their biological function, on the other hand, they have been indicated similar or better indications than MSCs (Tsiapalis and O'Driscoll, 2020).
Some researchers have demonstrated the immunomodulatory effects of MSC-derived EVs in vitro (Budoni, et al., 2013; Del Fattore, et al., 2015; Di Trapani, et al., 2016; Henao Agudelo, et al., 2017; Liu, et al., 2012), and have shown significant anti-inflammatory and regenerative abilities in several diseased animal models (Fierabracci, et al., 2015; Phinney and Pittenger, 2017). Specifically, researchers showed demonstration that in animal models of lung injury, the efficacy of MSC-EVs was effective like in hyperoxia (Braun, et al., 2018; Porzionato, et al., 2019; Willis, et al., 2018), severe bacterial pneumonia (Monsel, et al., 2015), and viral pneumonia (Khatri, Richardson, 2018). In addition, MSC-EVs has beneficial effects on lung perfusion ex vivo with severe E. coli pneumonia (Park, et al., 2011). Finally, MSC-EVs can effectively repair marginal donor lungs through several methods, the first one is dose-dependently increasing the clearance of alveolar fluid; the second one is reducing lung weight after perfusion and ventilation; and the third one is improving airway and hemodynamic parameters (Ragni, et al., 2017). On the basic of these useful and promising results, the role of MSC-EVs in alleviating and repairing ARDS lung injury is gaining increasing attention (Lee, et al., 2019; Li, Huang, 2014; Monsel, Zhu, 2015; Shah, et al., 2019). Notably, fibrosis sequelae with reduced pulmonary function have been reported in patients recovering from COVID-19 pneumonia. Indeed, there is an increased risk of idiopathic pulmonary fibrosis following viral infection (Sheng, et al., 2020), as a long-term complication, it has been reported in parts of SARS infected patients (Zhang, et al., 2020b). MSC-EVs prevent fibrosis following experimental lung injury, which is similar to the cells of their origin (Cargnoni, et al., 2009; Mansouri, et al., 2019). In fact, there is a growing interest in the new potential application of EV as a disease treatment strategy. EVs are considered safe, easy and cheap to produce, isolate, store and manage (Muraca, et al., 2017), which could reduce costs and improve product availability. It is worth mentioning that EVs seem to be a very generic product that it has application diversity and can be modified by various technologies, for example, manipulating their parent cells use genetic engineering, and then integrated into the secreted EVs by introducing exogenous substances (Armstrong, et al., 2017; Vader, et al., 2016; Wiklander, et al., 2019). A similar method has been demonstrated to deliver exogenous miRNA-Let-7c to alleviate renal fibrosis via MSC-EVs in the mice model of unilateral ureteral obstruction (Wang, et al., 2016). On the other hand, studies also suggested that EVs could be loaded with therapeutic molecules form a polymer complex to improve its targeting activity (Cappariello, et al., 2018; Pascucci, et al., 2014; Vader, Mol, 2016; van der Meel, et al., 2014).
The pulmonary pathology of COVID-19 critically ill patients includes the exudation and proliferation phase of diffuse alveolar injury, and microvascular thrombosis that suggest early ARDS (Wichmann, et al., 2020; Xu, et al., 2020d). The pathogenesis includes change of alveolar permeability and neutrophils infiltration (Zemans and Matthay, 2017). Several studies showed that MSC-EVs could reduce alveolar permeability and increase alveolar fluid clearance, in an vitro model of human perfusion lung injury with severe E. coli pneumonia (Gennai, et al., 2015; Lee, et al., 2009; Park, et al., 2019). Interestingly, there were antibacterial substances in the culture medium of the bacteria-stimulated MSCs (Krasnodembskaya, et al., 2010). In a mouse sepsis model, treatment with MSCs could increase bacterial clearance, part of the reason is it increased phagocytic activity of host immune cells (Mei, et al., 2010). In addition, MSC-EVs demonstrated antiviral activity in vitro by inhibiting replication of influenza virus in lung epithelial cells, meanwhile by reducing viral load in an influenza-induced lung injury model of pigs (Khatri, Richardson, 2018).
Application of MSC-EVs in the Treatment of COVID-19
Recently, Sengupta, et al. published the safety and efficacy of allogeneic bone marrow MSC-derived EVs in 24 patients with COVID-19 severe pneumonia that they have been treated with hydroxychloroquine and azithromycin (Sengupta, et al., 2020). It was interesting to note that inflammatory biomarkers and absolute neutrophilic counts were decreased prominently, while the counts of total lymphocyte and CD8+ were increased prominently within 5 days after EVs injection. In addition, D-dimer was significantly reduced. Although this EVs treatment resulted about 71% of patients recovery to health, it is not clear whether the recovery was attributable to EVs treatment, because of the lack of a matched control group and the lack of reported protein or microRNA composition of EVs used in the study. In summary, we would like to underline that there has been currently no approved MSC-based approach in preventing and treating the COVID-19, either with MSCs or MSCs-derived EVs. Clinical trials using EVs should be carried out with great caution, as their cargo determines the functional impact. Consequently, before MSC-derived EVs used in clinic, standardized protocols need to be developed, including mass production, isolation, functional evaluation, and batch-to-batch consistency. Some clinical trials using MSC-EVs in COVID-19 is currently underway and are summarized in Table 1.
Preliminary studies demonstrated that intravenous injection of MSCs and MSC-EVs possessed an enormous potential in treatment for COVID-19 patients. So far, no adverse events were found during MSCs and MSC-EVs treatment and follow-up period, suggesting that this therapy is safe and effective for COVID-19 patients (Akbari and Rezaie, 2020). However, MSCs express tissue factors and accumulate mainly in pulmonary capillaries, which may increase the risk of pulmonary embolism and other thromboembolic events. The effects of administration time, dose, frequency and route of MSCs and MSC-EVs need further study. Though clinical outcomes are promising, the limited literature still warrants more studies to establish safety and efficacy of MSCs and MSC-EVs to treat and manage symptoms associated with COVID-19 infection (Hamdan, et al., 2021).
Drug-EVs
One interesting approach of using EVs as therapeutic agents is its drug delivery potential (Gnecchi, et al., 2006; Lamichhane, et al., 2016; Lv, et al., 2012). EVs have a cellular origin, such as MSCs, and therefore offer greater safety and stability than other delivery systems such as liposomes (Malhotra, et al., 2016). Selectable compounds or genetically engineered molecules may loading with EVs in the nanocarriers to enhance the targeting ability for tissue/cell infection, suggesting that EVs-based nanocarriers can be used to treat infectious diseases. Some studies have used EV as an effective carrier of tumor-targeted anticancer drugs curcumin, doxorubicin, and paclitaxel (Kim, et al., 2016). Therefore, an EV-based drug delivery system has great potential to increase drug loading in targeting cells and inhibit off-target effects. EVs may be used to deliver therapeutic drugs or biomodulators to inhibiting the spread and replication of virus in the recipient cells (Romagnoli, et al., 2014). The development of safe and efficient nanocarriers is the main goal of nanomedicine. Therefore, the development of EVs-based nanocarriers offers a promising opportunity for therapeutic drug delivery. However, most of the studies have been conducted in vitro and in vivo experimental models, but it remains a mystery of the safety, specificity, and efficiency of the method in clinical trials.
Another attractive treatment option is to use the blood products of convalescent patients, such as the whole blood, plasma or serum. The EVs containing neutralizing antibodies in convalescent blood products were transferred into patients, and we benefit tremendously with this treatment by promoting immune regulation and lung tissue wound healing. Kesimer et al. proved that EVs derived from culture medium of human tracheobronchial epithelial cells showed a neutralizing effect on human influenza virus (Kesimer, et al., 2009). Studies have proved that plasma-derived EVs also carry an amount of cell growth factors, and these factors could induce the activation of cellular signaling pathways, change vascular reactivity, induce angiogenesis and promote tissue repair (Guo, et al., 2017; Tao, et al., 2017; Torreggiani, et al., 2014).
EVs Vaccines
The use of EVs as immunogenicity factors in the treatment of SARS coronavirus infection has been studied. EV might be used as a vaccine with its advantages of high stability, low toxicity and immunogenicity in circulation (Zhou, et al., 2020c). Kuate et al. analyzed exosome-based vaccines which containing the S protein of SARS-CoV-2. The S proteins-containing exosomes were procured by replacing the transmembrane and cytoplasmic domains of the S protein with those of VSV-G, and its immunogenicity and efficacy were tested in mice. After comparing it to an adenoviral vector vaccine expressing the S protein, it has been proved that both of the exosomes and the vaccine could induce neutralizing antibody titers. After priming with the SARS-S protein exosomal vaccine and boosting with the adenoviral vector the neutralizing antibody titers of using SARS-S protein exosomal vaccine exceeded those observed in the convalescent serum of SARS patients (Kuate, et al., 2007). In addition, the treatment of EVs has been shown to be more effective than that of soluble protein subunit vaccines, which might be due to the fact that the expression of multiple copies of the same viral protein exposed on the EVs surface promotes the cross-linking between EVs and the B cell receptor (Gyorgy, et al., 2015).
Platelet-derived EVs
Platelets are small (2–4 µm), and they are the anuclear cellular fragments of megakaryocytes from bone marrow and lung (Lefrancais, et al., 2017; Machlus, and Italiano, 2013). There are almost one trillion platelets patrol in the blood to hold the vascular system integrity. Once blood vessels are damaged, platelets will form a thrombus to prevent subsequent hemorrhage (Davi and Patrono, 2007). Platelet mediated thrombosis may also involve EVs (micro particles or micro vesicles), that provide anionic phospholipids such as phosphatidylserine to support the blood coagulation cascade (Ridger, et al., 2017). In addition to playing a role in hemostasis and thrombosis, platelets contribute to inducing the immune and inflammatory response (Kapur, et al., 2015; Li, et al., 2012; Morrell, et al., 2014; Semple, et al., 2011). The exosmosis and invasion to inflammatory tissues of neutrophils require interaction with activated platelets (Imhof, et al., 2016; Sreeramkumar, et al., 2014). The release of extracellular DNA (NETosis) by neutrophils was observed in patients with COVID-19 (Barnes, et al., 2020; Middleton, et al., 2020; Zuo, et al., 2020). NETosis requires platelets and may result in thrombosis (Constantinescu-Bercu, et al., 2020; Martinod, and Wagner, 2014). Some immune and inflammatory molecules have been found to express in platelets, such as IL-1; besides some immune receptors have been detected, such as CD40L, Toll-like receptors (TLR), and Fc receptors (Karas, et al., 1982; Lindemann, et al., 2001; Semple, Italiano, 2011).
Serological findings in patients with symptomatic COVID-19 include severe leukopenia and lymphocytopenia (Arentz, et al., 2020; Connors and Levy, 2020; Tang, et al., 2020). Low platelet count is closely associated with an increased risk of death for the in-hospital COVID-19 patients, although platelet levels are not generally considered clinically relevant. This finding suggests that SARS-CoV-2 infection may decrease platelet production and/or increase the damage of platelet. Patients with COVID-19 are more likely increase platelet consumption because of the platelet activation and thrombosis (Al-Samkari, et al., 2020; Liao, et al., 2020; Lippi and Favalora, 2020a; Lippi, et al., 2020b; Liu, et al., 2020; Xu, et al., 2020a; Yang, et al., 2020b).
Since thrombus and clotting are mainly controlled by platelets (Roberts, et al., 2006), it is critical to determine the status of platelets in COVID-19. Platelet-EVs delivers molecules from the mother platelet. They transport platelet-derived cytokines and other pro-inflammatory molecules, such as damage-associated molecular patterns (DAMPs) (Boudreau, et al., 2014; Maugeri, et al., 2018; Melki, et al., 2017). SARS-CoV-2 enters the ACE2-expressed endothelial cells. The loss of endothelial integrity may facilitate the recruitment of circulating platelets to the infected site, causing platelet activation and degranulation. Through analysis of platelet degranulation and cytokine release, Zaid et al. observed an evident increase in platelet-EVs firstly in platelet-EVs in patients with COVID-19 (Zaid, et al., 2020). This increase may be due to increased production of megakaryocytes or platelets, reduced clearance, or a combination of the two. Surprisingly, the level of platelet-EVs was significantly lower in severely ill patients than in non-critically ill patients. In severe COVID-19 cases, the number of platelets decreases. They reported that platelets were at the forefront of COVID-19 because they release various molecules at different stages of the disease. Thus, it is possible that platelets, which are associated with SARS-CoV-2 RNA and are highly activated in COVID-19, may be involved in the overwhelming thrombosis of COVID-19. Platelet overactivation may be involved in the systemic inflammatory response and thrombosis events observed in this disease. Therefore, inhibition of pathways associated with platelet activation may improve prognosis during COVID-19.
Giuseppe Cappellano et al. (Cappellano, et al., 2021) showed that platelet-EVs counts were higher in SARS-CoV-2 positive patients compared with SARS-CoV-2-negative patients, while platelet counts were not changed. What is particularly interesting is that the level of PLT-EVs was strongly associated with SARS-CoV-2 infection by a multivariate analysis, which independent from any confounding factors (age, sex, comorbidity, etc.). There was a very good diagnostic performance in ROC curve analysis, with sensitivity of 75% and specificity of 74%.
Platelet-derived EVs can be used to develop novel treatment strategies for COVID-19 patients. A study showed that engineered platelet-derived EVs loaded with TPCA-1 were effective in treating pneumonia (Ma, et al., 2020). In mouse models that selectively target inflammatory sites, platelet-derived EVs suppressed inflammation and reduced local cytokine storms.
Reduces EVs Secreted by Virus-infected Cells Into Receptor Cells
EVs secreted from virus-infected cells contribute to promoting viral infection and inhibiting immune cell response. Therefore, it may be a new useful method to overcome viral transmission by inhibiting the uptake of EVs (Li, et al., 2019; Schneider, et al., 2017).
Opportunities and Challenges
Understanding the roles of EVs in COVID-19 infection could increase our knowledge of the dynamics of the virus and help developing effective prevention and treatment modalities. The above-mentioned findings provide a basis for future research on the dynamics of SARS-CoV-2 virus infection and its inhibition. However, it is worth noting that no EVs-based treatment has been approved to date. Significant barriers remain for the development of MSC-EVs as a therapeutic tool (Tsiapalis and O'Driscoll, 2020). Currently, we do not have any information on the possible side effects of EVs treatment against COVID-19. First, there are the similarities between EVs and viruses, both of them interact with the endosomal system which is responsible for synthesis of EVs. Some viruses may use this mechanism to pack viral proteins and RNA into vesicles in infected cells, subsequently released into the extracellular space, then facilitate the spread of the virus to uninfected cells (Gould, et al., 2003). This process has been demonstrated in HIV infection, but has not been elucidated in coronavirus infection (Arenaccio, Anticoli, 2015; Giannessi, et al., 2020). Second, the heterogeneity of EVs is a big challenge (Tkach, et al., 2018). Up to now, all published studies using heterogeneous EVs in some cases using the part size (<200 nm “small” EVs). Because the origin, size, composition and functional characteristics of the EVs are different, the source selection should be carefully described when using them for treatment. It is important to note that different sizes EVs of dendritic cells can induce the activation of T cells with different polarization mode (Kowal, et al., 2016). Third, due to the lack of standardization for accurate counting EV, as well as the current equipment does not differentiate between vesicles and non-vesicular granules, the quantitation of EV preparation is also an unsolved problems (Tkach, Kowal, 2018). For example, Campanella et al. reported that autologous exosomes were safer than allogeneic plasma exosomes in regenerative medicine (Campanella, et al., 2019). Soni et al. demonstrated that alveolar macrophages produce EVs might have a proinflammatory or anti-inflammatory effects, depending on the separation time of EVs in the process of acute lung injury (Soni, et al., 2016). Fourth, variability of tissue of origin and culture conditions (Patel, et al., 2018; Pittenger, et al., 2019). Although the MSCs from different sources have different immunosuppression and differentiation ability, but the optimal source of immunomodulation has not yet been determined (Gao, Chiu, 2016). Chance et al. reported that MSC-EVs had higher thrombotic activity than BMMMSC-EVs (Chance, et al., 2019). Recently, Inal reported a specific EVs subgroup, especially the EVs-TF positive might be associated with venous thromboembolism in COVID-19 patients with hypertension and diabetes (Inal, 2020). Taken together, it is critical to emphasize that care should be taken in using EVs for disease treatment (Borger, et al., 2020). To ensure the curative effect and safety of using EVs for COVID-19, enormous efforts are required to improve the technology for the separation and identification of EVs.
Author Contributions
Y-yY, W-mZ, and Y-qW wrote the first draft of manuscript, Q-rG, F-xZ, and Z-yZ designed the figures, Y-xX and H-yZ finished the table, MA and AMJ amended the text and figures, BM and J-yZ designed and revised the manuscript. All authors read and approved the final manuscript.
Funding
This work was supported by the National Natural Science Foundation of China (81902152, U1903126, 81773888 and 81903467), Open Founding of Key Laboratory Ethnomedicine Ministry of Education (KLEM-KF2019Y03), the Key Medical Science and Technology Project of Shanxi Province (2020 XM33-“Four Batches” of Science and Technology Medical Innovation Project of Shanxi Province), and the Fund of Shanxi Province Higher Education Technology Innovation Project (2019L0753).
Conflict of Interest
The authors declare that the research was conducted in the absence of any commercial or financial relationships that could be construed as a potential conflict of interest.
References
Abdi, R., Fiorina, P., Adra, C. N., Atkinson, M., and Sayegh, M. H. (2008). Immunomodulation by Mesenchymal Stem Cells: A Potential Therapeutic Strategy for Type 1 Diabetes. Diabetes 57, 1759–1767. doi:10.2337/db08-0180
Ackermann, M., Verleden, S. E., Kuehnel, M., Haverich, A., Welte, T., Laenger, F., et al. (2020). Pulmonary Vascular Endothelialitis, Thrombosis, and Angiogenesis in Covid-19. N. Engl. J. Med. 383, 120–128. doi:10.1056/NEJMoa2015432
Ahmadi, M., and Rezaie, J. (2021). Ageing and Mesenchymal Stem Cells Derived Exosomes: Molecular Insight and Challenges. Cell Biochem Funct 39, 60–66. doi:10.1002/cbf.3602
Akbari, A., and Rezaie, J. (2020). Potential Therapeutic Application of Mesenchymal Stem Cell-Derived Exosomes in SARS-CoV-2 Pneumonia. Stem Cell Res. Ther. 11, 356. doi:10.1186/s13287-020-01866-6
Al-Samkari, H., Karp Leaf, R. S., Dzik, W. H., Carlson, J. C. T., Fogerty, A. E., Waheed, A., et al. (2020). COVID-19 and Coagulation: Bleeding and Thrombotic Manifestations of SARS-CoV-2 Infection. Blood 136, 489–500. doi:10.1182/blood.2020006520
Ali, S. A., Huang, M.-B., Campbell, P. E., Roth, W. W., Campbell, T., Khan, M., et al. (2010). Genetic Characterization of HIV Type 1 Nef-Induced Vesicle Secretion. AIDS Res. Hum. Retroviruses 26, 173–192. doi:10.1089/aid.2009.0068
Arenaccio, C., Anticoli, S., Manfredi, F., Chiozzini, C., Olivetta, E., and Federico, M. (2015). Latent HIV-1 Is Activated by Exosomes from Cells Infected with Either Replication-Competent or Defective HIV-1. Retrovirology 12, 87. doi:10.1186/s12977-015-0216-y
Arentz, M., Yim, E., Klaff, L., Lokhandwala, S., Riedo, F. X., Chong, M., et al. (2020). Characteristics and Outcomes of 21 Critically Ill Patients with COVID-19 in Washington State. JAMA 323, 1612–1614. doi:10.1001/jama.2020.4326
Armstrong, J. P. K., Holme, M. N., and Stevens, M. M. (2017). Re-Engineering Extracellular Vesicles as Smart Nanoscale Therapeutics. ACS Nano 11, 69–83. doi:10.1021/acsnano.6b07607
Atluri, S., Manchikanti, L., and Hirsch, J. A. (2020). Expanded Umbilical Cord Mesenchymal Stem Cells (UC-MSCs) as a Therapeutic Strategy in Managing Critically Ill COVID-19 Patients: The Case for Compassionate Use. Pain Physician 23, E71–E83. doi:10.36076/ppj.2020/23/e71
Babaei, M., and Rezaie, J. (2021). Application of Stem Cell-Derived Exosomes in Ischemic Diseases: Opportunity and Limitations. J. Transl Med. 19, 196. doi:10.1186/s12967-021-02863-w
Bari, E., Ferrarotti, I., Saracino, L., Perteghella, S., Torre, M. L., and Corsico, A. G. (2020). Mesenchymal Stromal Cell Secretome for Severe COVID-19 Infections: Premises for the Therapeutic Use. Cells 9, 924. doi:10.3390/cells9040924
Bari, E., Ferrarotti, I., Torre, M. L., Corsico, A. G., and Perteghella, S. (2019). Mesenchymal Stem/stromal Cell Secretome for Lung Regeneration: The Long Way through "pharmaceuticalization" for the Best Formulation. J. Control. Release 309, 11–24. doi:10.1016/j.jconrel.2019.07.022
Barnes, B. J., Adrover, J. M., Baxter-Stoltzfus, A., Borczuk, A., Cools-Lartigue, J., Crawford, J. M., et al. (2020). Targeting Potential Drivers of COVID-19: Neutrophil Extracellular Traps. J. Exp. Med. 217, e20200652. doi:10.1084/jem.20200652
Bautista-Vargas, M., Bonilla-Abadía, F., and Cañas, C. A. (2020). Potential Role for Tissue Factor in the Pathogenesis of Hypercoagulability Associated with in COVID-19. J. Thromb. Thrombolysis 50, 479–483. doi:10.1007/s11239-020-02172-x
Beigel, J. H., Tomashek, K. M., Dodd, L. E., Mehta, A. K., Zingman, B. S., Kalil, A. C., et al. (2020). Remdesivir for the Treatment of Covid-19 - Final Report. N. Engl. J. Med. 383, 1813–1826. doi:10.1056/NEJMoa2007764
Berger, J. S., Kunichoff, D., Adhikari, S., Ahuja, T., Amoroso, N., Aphinyanaphongs, Y., et al. (2020). Prevalence and Outcomes of D-Dimer Elevation in Hospitalized Patients with COVID-19. Arterioscler. Thromb. Vasc. Biol. 40, 2539–2547. doi:10.1161/ATVBAHA.120.314872
Bhattacharya, J., and Matthay, M. A. (2013). Regulation and Repair of the Alveolar-Capillary Barrier in Acute Lung Injury. Annu. Rev. Physiol. 75, 593–615. doi:10.1146/annurev-physiol-030212-183756
Börger, V., Weiss, D. J., Anderson, J. D., Borràs, F. E., Bussolati, B., Carter, D. R. F., et al. (2020). International Society for Extracellular Vesicles and International Society for Cell and Gene Therapy Statement on Extracellular Vesicles from Mesenchymal Stromal Cells and Other Cells: Considerations for Potential Therapeutic Agents to Suppress Coronavirus Disease-19. Cytotherapy 22, 482–485. doi:10.1016/j.jcyt.2020.05.002
Boudreau, L. H., Duchez, A.-C., Cloutier, N., Soulet, D., Martin, N., Bollinger, J., et al. (2014). Platelets Release Mitochondria Serving as Substrate for Bactericidal Group IIA-Secreted Phospholipase A2 to Promote Inflammation. Blood 124, 2173–2183. doi:10.1182/blood-2014-05-573543
Bourgonje, A. R., Abdulle, A. E., Timens, W., Hillebrands, J. L., Navis, G. J., Gordijn, S. J., et al. (2020). Angiotensin‐converting Enzyme 2 ( ACE2 ), SARS‐CoV ‐2 and the Pathophysiology of Coronavirus Disease 2019 ( COVID ‐19). J. Pathol. 251, 228–248. doi:10.1002/path.5471
Braun, R. K., Chetty, C., Balasubramaniam, V., Centanni, R., Haraldsdottir, K., Hematti, P., et al. (2018). Intraperitoneal Injection of MSC-Derived Exosomes Prevent Experimental Bronchopulmonary Dysplasia. Biochem. Biophys. Res. Commun. 503, 2653–2658. doi:10.1016/j.bbrc.2018.08.019
Budoni, M., Fierabracci, A., Luciano, R., Petrini, S., Di Ciommo, V., and Muraca, M. (2013). The Immunosuppressive Effect of Mesenchymal Stromal Cells on B Lymphocytes Is Mediated by Membrane Vesicles. Cell Transplant. 22, 369–379. doi:10.3727/096368911X58276910.3727/096368911x582769b
Campanella, C., Caruso Bavisotto, C., Logozzi, M., Marino Gammazza, A., Mizzoni, D., Cappello, F., et al. (2019). On the Choice of the Extracellular Vesicles for Therapeutic Purposes. Int. J. Mol. Sci. 20, 236. doi:10.3390/ijms20020236
Cappariello, A., Loftus, A., Muraca, M., Maurizi, A., Rucci, N., and Teti, A. (2018). Osteoblast-Derived Extracellular Vesicles Are Biological Tools for the Delivery of Active Molecules to Bone. J. Bone Miner Res. 33, 517–533. doi:10.1002/jbmr.3332
Cappellano, G., Raineri, D., Rolla, R., Giordano, M., Puricelli, C., Vilardo, B., et al. (2021). Circulating Platelet-Derived Extracellular Vesicles Are a Hallmark of Sars-Cov-2 Infection. Cells 10, 85. doi:10.3390/cells10010085
Cargnoni, A., Gibelli, L., Tosini, A., Signoroni, P. B., Nassuato, C., Arienti, D., et al. (2009). Transplantation of Allogeneic and Xenogeneic Placenta-Derived Cells Reduces Bleomycin-Induced Lung Fibrosis. Cell Transplant. 18, 405–422. doi:10.3727/096368909788809857
Cascella, M., Rajnik, M., Cuomo, A., Dulebohn, S. C., and Di Napoli, R. (2021). Features, Evaluation, and Treatment of Coronavirus (COVID-19). Treasure Island, FL: StatPearls.
Cavalli, G., De Luca, G., Campochiaro, C., Della-Torre, E., Ripa, M., Canetti, D., et al. (2020). Interleukin-1 Blockade with High-Dose Anakinra in Patients with COVID-19, Acute Respiratory Distress Syndrome, and Hyperinflammation: A Retrospective Cohort Study. Lancet Rheumatol. 2, e325–e331. doi:10.1016/S2665-9913(20)30127-2
Chance, T. C., Rathbone, C. R., Kamucheka, R. M., Peltier, G. C., Cap, A. P., and Bynum, J. A. (2019). The Effects of Cell Type and Culture Condition on the Procoagulant Activity of Human Mesenchymal Stromal Cell-Derived Extracellular Vesicles. J. Trauma Acute Care Surg. 87, S74–S82. doi:10.1097/TA.0000000000002225
Chen, C. M., and Chou, H. C. (2018). Human Mesenchymal Stem Cells Attenuate Hyperoxia-Induced Lung Injury through Inhibition of the Renin-Angiotensin System in Newborn Rats. Am. J. Transl Res. 10, 2628–2635.
Connors, J. M., and Levy, J. H. (2020). COVID-19 and its Implications for Thrombosis and Anticoagulation. Blood 135, 2033–2040. doi:10.1182/blood.2020006000
Constantinescu-Bercu, A., Grassi, L., Frontini, M., Salles-Crawley, I. I., Woollard, K., and Crawley, J. T. (2020). Activated αIIbβ3 on Platelets Mediates Flow-dependent NETosis via SLC44A2. Elife 9, e53353. doi:10.7554/eLife.53353
Cruz, F. F., and Rocco, P. R. M. (2020). The Potential of Mesenchymal Stem Cell Therapy for Chronic Lung Disease. Expert Rev. Respir. Med. 14, 31–39. doi:10.1080/17476348.2020.1679628
Cui, J., Li, F., and Shi, Z.-L. (2019). Origin and Evolution of Pathogenic Coronaviruses. Nat. Rev. Microbiol. 17, 181–192. doi:10.1038/s41579-018-0118-9
Davì, G., and Patrono, C. (2007). Platelet Activation and Atherothrombosis. N. Engl. J. Med. 357, 2482–2494. doi:10.1056/NEJMra071014
Del Fattore, A., Luciano, R., Pascucci, L., Goffredo, B. M., Giorda, E., Scapaticci, M., et al. (2015). Immunoregulatory Effects of Mesenchymal Stem Cell-Derived Extracellular Vesicles on T Lymphocytes. Cell Transplant. 24, 2615–2627. doi:10.3727/096368915X687543
Devaux, C. A., Rolain, J.-M., and Raoult, D. (2020). ACE2 Receptor Polymorphism: Susceptibility to SARS-CoV-2, Hypertension, Multi-Organ Failure, and COVID-19 Disease Outcome. J. Microbiol. Immunol. Infect. 53, 425–435. doi:10.1016/j.jmii.2020.04.015
Di Trapani, M., Bassi, G., Midolo, M., Gatti, A., Takam Kamga, P., Cassaro, A., et al. (2016). Differential and Transferable Modulatory Effects of Mesenchymal Stromal Cell-Derived Extracellular Vesicles on T, B and NK Cell Functions. Sci. Rep. 6, 24120. doi:10.1038/srep24120
Dogrammatzis, C., Waisner, H., and Kalamvoki, M. (2020). Cloaked Viruses and Viral Factors in Cutting Edge Exosome-Based Therapies. Front. Cell Dev. Biol. 8, 376. doi:10.3389/fcell.2020.00376
Dominici, M., Le Blanc, K., Mueller, I., Slaper-Cortenbach, I., Marini, F. C., Krause, D. S., et al. (2006). Minimal Criteria for Defining Multipotent Mesenchymal Stromal Cells. The International Society for Cellular Therapy Position Statement. Cytotherapy 8, 315–317. doi:10.1080/14653240600855905
Doorn, J., Moll, G., Le Blanc, K., van Blitterswijk, C., and de Boer, J. (2012). Therapeutic Applications of Mesenchymal Stromal Cells: Paracrine Effects and Potential Improvements. Tissue Eng. Part B Rev. 18, 101–115. doi:10.1089/ten.TEB.2011.0488
England, J. T., Abdulla, A., Biggs, C. M., Lee, A. Y. Y., Hay, K. A., Hoiland, R. L., et al. (2021). Weathering the COVID-19 Storm: Lessons from Hematologic Cytokine Syndromes. Blood Rev. 45, 100707. doi:10.1016/j.blre.2020.100707
Fierabracci, A., Del Fattore, A., Luciano, R., Muraca, M., Teti, A., and Muraca, M. (2015). Recent Advances in Mesenchymal Stem Cell Immunomodulation: the Role of Microvesicles. Cell Transplant. 24, 133–149. doi:10.3727/096368913X675728
Fleming, A., Sampey, G., Chung, M.-C., Bailey, C., van Hoek, M. L., Kashanchi, F., et al. (2014). The Carrying Pigeons of the Cell: Exosomes and Their Role in Infectious Diseases Caused by Human Pathogens. Pathog. Dis. 71, 109–120. doi:10.1111/2049-632X.12135
Fung, T. S., and Liu, D. X. (2019). Human Coronavirus: Host-Pathogen Interaction. Annu. Rev. Microbiol. 73, 529–557. doi:10.1146/annurev-micro-020518-115759
Galderisi, U., and Giordano, A. (2014). The Gap Between the Physiological and Therapeutic Roles of Mesenchymal Stem Cells. Med. Res. Rev. 34, 1100–1126. doi:10.1002/med.21322
Gao, F., Chiu, S. M., Motan, D. A. L., Zhang, Z., Chen, L., Ji, H.-L., et al. (2016). Mesenchymal Stem Cells and Immunomodulation: Current Status and Future Prospects. Cell Death Dis 7, e2062. doi:10.1038/cddis.2015.327
Gao, Y. M., Xu, G., Wang, B., and Liu, B. C. (2021). Cytokine Storm Syndrome in Coronavirus Disease 2019: A Narrative Review. J. Intern. Med. 289, 147–161. doi:10.1111/joim.13144
Gennai, S., Monsel, A., Hao, Q., Park, J., Matthay, M. A., and Lee, J. W. (2015). Microvesicles Derived from Human Mesenchymal Stem Cells Restore Alveolar Fluid Clearance in Human Lungs Rejected for Transplantation. Am. J. Transplant. 15, 2404–2412. doi:10.1111/ajt.13271
Giannessi, F., Aiello, A., Franchi, F., Percario, Z. A., and Affabris, E. (2020). The Role of Extracellular Vesicles as Allies of HIV, HCV and SARS Viruses. Viruses 12, 571. doi:10.3390/v12050571
Glowacka, I., Bertram, S., Herzog, P., Pfefferle, S., Steffen, I., Muench, M. O., et al. (2010). Differential Downregulation of ACE2 by the Spike Proteins of Severe Acute Respiratory Syndrome Coronavirus and Human Coronavirus NL63. J. Virol. 84, 1198–1205. doi:10.1128/JVI.01248-09
Gnecchi, M., He, H., Noiseux, N., Liang, O. D., Zhang, L., Morello, F., et al. (2006). Evidence Supporting Paracrine Hypothesis for Akt‐modified Mesenchymal Stem Cell‐mediated Cardiac protection and Functional Improvement. FASEB J. 20, 661–669. doi:10.1096/fj.05-5211com
Gnecchi, M., Zhang, Z., Ni, A., and Dzau, V. J. (2008). Paracrine Mechanisms in Adult Stem Cell Signaling and Therapy. Circ. Res. 103, 1204–1219. doi:10.1161/CIRCRESAHA.108.176826
Gould, S. J., Booth, A. M., and Hildreth, J. E. K. (2003). The Trojan Exosome Hypothesis. Proc. Natl. Acad. Sci. USA 100, 10592–10597. doi:10.1073/pnas.1831413100
Grasselli, G., Zangrillo, A., Zanella, A., Antonelli, M., Cabrini, L., Castelli, A., et al. (2020). Baseline Characteristics and Outcomes of 1591 Patients Infected with SARS-CoV-2 Admitted to ICUs of the Lombardy Region. Italy. JAMA. 323, 1574–1581. doi:10.1001/jama.2020.539410.1001/jama.2020.4031
Grover, S. P., and Mackman, N. (2018). Tissue Factor: An Essential Mediator of Hemostasis and Trigger of Thrombosis. Arterioscler. Thromb. Vasc. Biol. 38, 709–725. doi:10.1161/ATVBAHA.117.309846
Gunasekaran, M., Xu, Z., Nayak, D. K., Sharma, M., Hachem, R., Walia, R., et al. (2017). Donor-Derived Exosomes with Lung Self-Antigens in Human Lung Allograft Rejection. Am. J. Transplant. 17, 474–484. doi:10.1111/ajt.13915
Guo, S.-C., Tao, S.-C., Yin, W.-J., Qi, X., Yuan, T., and Zhang, C.-Q. (2017). Exosomes Derived from Platelet-Rich Plasma Promote the Re-epithelization of Chronic Cutaneous Wounds via Activation of YAP in a Diabetic Rat Model. Theranostics 7, 81–96. doi:10.7150/thno.16803
Guzik, T. J., Mohiddin, S. A., Dimarco, A., Patel, V., Savvatis, K., Marelli-Berg, F. M., et al. (2020). COVID-19 and the Cardiovascular System: Implications for Risk Assessment, Diagnosis, and Treatment Options. Cardiovasc. Res. 116, 1666–1687. doi:10.1093/cvr/cvaa106
György, B., Hung, M. E., Breakefield, X. O., and Leonard, J. N. (2015). Therapeutic Applications of Extracellular Vesicles: Clinical Promise and Open Questions. Annu. Rev. Pharmacol. Toxicol. 55, 439–464. doi:10.1146/annurev-pharmtox-010814-124630
Hamdan, H., Hashmi, S. K., Lazarus, H., Gale, R. P., Qu, W., and El Fakih, R. (2021). Promising Role for Mesenchymal Stromal Cells in Coronavirus Infectious Disease-19 (COVID-19)-Related Severe Acute Respiratory Syndrome? Blood Rev. 46, 100742. doi:10.1016/j.blre.2020.100742
Hamming, I., Timens, W., Bulthuis, M., Lely, A., Navis, G., and van Goor, H. (2004). Tissue Distribution of ACE2 Protein, the Functional Receptor for SARS Coronavirus. A First Step in Understanding SARS Pathogenesis. J. Pathol. 203, 631–637. doi:10.1002/path.1570
Han, L., Lam, E. W.-F., and Sun, Y. (2019). Extracellular Vesicles in the Tumor Microenvironment: Old Stories, but New Tales. Mol. Cancer 18, 59. doi:10.1186/s12943-019-0980-8
Harrell, C. R., Jovicic, N., Djonov, V., Arsenijevic, N., and Volarevic, V. (2019). Mesenchymal Stem Cell-Derived Exosomes and Other Extracellular Vesicles as New Remedies in the Therapy of Inflammatory Diseases. Cells 8, 1605. doi:10.3390/cells8121605
Henao Agudelo, J. S., Braga, T. T., Amano, M. T., Cenedeze, M. A., Cavinato, R. A., Peixoto-Santos, A. R., et al. (2017). Mesenchymal Stromal Cell-Derived Microvesicles Regulate an Internal Pro-inflammatory Program in Activated Macrophages. Front. Immunol. 8, 881. doi:10.3389/fimmu.2017.00881
Huang, C., Wang, Y., Li, X., Ren, L., Zhao, J., Hu, Y., et al. (2020a). Clinical Features of Patients Infected with 2019 Novel Coronavirus in Wuhan, China. Lancet 395, 497–506. doi:10.1016/S0140-6736(20)30183-5
Huang, W., Yan, Y., Liu, Y., Lin, M., Ma, J., Zhang, W., et al. (2020b). Exosomes with Low miR-34c-3p Expression Promote Invasion and Migration of Non-small Cell Lung Cancer by Upregulating Integrin α2β1. Signal Transduct. Target. Ther. 5, 39. doi:10.1038/s41392-020-0133-y
Huet, T., Beaussier, H., Voisin, O., Jouveshomme, S., Dauriat, G., Lazareth, I., et al. (2020). Anakinra for Severe Forms of COVID-19: A Cohort Study. Lancet Rheumatol. 2, e393–e400. doi:10.1016/S2665-9913(20)30164-8
Imhof, B. A., Jemelin, S., Ballet, R., Vesin, C., Schapira, M., Karaca, M., et al. (2016). CCN1/CYR61-mediated Meticulous Patrolling by Ly6Clow Monocytes Fuels Vascular Inflammation. Proc. Natl. Acad. Sci. USA 113, E4847–E4856. doi:10.1073/pnas.1607710113
Inal, J. (2020). COVID‐19 Comorbidities, Associated Procoagulant Extracellular Vesicles and Venous Thromboembolisms: A Possible Link with Ethnicity? Br. J. Haematol. 190, e218–e220. doi:10.1111/bjh.17011
Jia, H. (2016). Pulmonary Angiotensin-Converting Enzyme 2 (ACE2) and Inflammatory Lung Disease. Shock 46, 239–248. doi:10.1097/SHK.0000000000000633
Kapur, R., Zufferey, A., Boilard, E., and Semple, J. W. (2015). Nouvelle Cuisine: Platelets Served with Inflammation. J. Immunol. 194, 5579–5587. doi:10.4049/jimmunol.1500259
Karas, S., Rosse, W., and Kurlander, R. (1982). Characterization of the IgG-Fc Receptor on Human Platelets. Blood 60, 1277–1282. doi:10.1182/blood.v60.6.1277.bloodjournal6061277
Kesimer, M., Scull, M., Brighton, B., DeMaria, G., Burns, K., O’Neal, W., et al. (2009). Characterization of Exosome‐like Vesicles Released from Human Tracheobronchial Ciliated Epithelium: A Possible Role in Innate Defense. FASEB J. 23, 1858–1868. doi:10.1096/fj.08-119131
Khatri, M., Richardson, L. A., and Meulia, T. (2018). Mesenchymal Stem Cell-Derived Extracellular Vesicles Attenuate Influenza Virus-Induced Acute Lung Injury in a Pig Model. Stem Cell Res. Ther. 9, 17. doi:10.1186/s13287-018-0774-8
Kim, M. S., Haney, M. J., Zhao, Y., Mahajan, V., Deygen, I., Klyachko, N. L., et al. (2016). Development of Exosome-Encapsulated Paclitaxel to Overcome MDR in Cancer Cells. Nanomedicine 12, 655–664. doi:10.1016/j.nano.2015.10.012
Kolios, G., and Moodley, Y. (2013). Introduction to Stem Cells and Regenerative Medicine. Respiration 85, 3–10. doi:10.1159/000345615
Kowal, J., Arras, G., Colombo, M., Jouve, M., Morath, J. P., Primdal-Bengtson, B., et al. (2016). Proteomic Comparison Defines Novel Markers to Characterize Heterogeneous Populations of Extracellular Vesicle Subtypes. Proc. Natl. Acad. Sci. USA 113, E968–E977. doi:10.1073/pnas.1521230113
Kowal, J., Tkach, M., and Théry, C. (2014). Biogenesis and Secretion of Exosomes. Curr. Opin. Cell Biol. 29, 116–125. doi:10.1016/j.ceb.2014.05.004
Krasnodembskaya, A., Song, Y., Fang, X., Gupta, N., Serikov, V., Lee, J.-W., et al. (2010). Antibacterial Effect of Human Mesenchymal Stem Cells Is Mediated in Part from Secretion of the Antimicrobial Peptide LL-37. Stem Cells 28, 2229–2238. doi:10.1002/stem.544
Krishnamachary, B., Cook, C., Spikes, L., Chalise, P., and Dhillon, N. K. (2020). The Potential Role of Extracellular Vesicles in COVID-19 Associated Endothelial Injury and Pro-inflammation. medRxiv [Preprint]. Available at: https://www.ncbi.nlm.nih.gov/pmc/articles/PMC7480053/ (Accessed September 1, 2020). doi:10.1101/2020.08.27.20182808
Kuate, S., Cinatl, J., Doerr, H. W., and Überla, K. (2007). Exosomal Vaccines Containing the S Protein of the SARS Coronavirus Induce High Levels of Neutralizing Antibodies. Virology 362, 26–37. doi:10.1016/j.virol.2006.12.011
Kwon, Y., Nukala, S. B., Srivastava, S., Miyamoto, H., Ismail, N. I., Rehman, J., et al. (2020). Detection of Viral RNA Fragments in Human iPSC-Cardiomyocytes Following Treatment with Extracellular Vesicles from SARS-CoV-2 Coding-Sequence-Overexpressing Lung Epithelial Cells. bioRxiv [Preprint]. Available at: https://pubmed.ncbi.nlm.nih.gov/32637965/ (Accessed July 1, 2020). doi:10.1101/2020.05.14.093583
Lamichhane, T. N., Jeyaram, A., Patel, D. B., Parajuli, B., Livingston, N. K., Arumugasaamy, N., et al. (2016). Oncogene Knockdown via Active Loading of Small RNAs into Extracellular Vesicles by Sonication. Cell. Mol. Bioeng. 9, 315–324. doi:10.1007/s12195-016-0457-4
Lanyu, Z., and Feilong, H. (2019). Emerging Role of Extracellular Vesicles in Lung Injury and Inflammation. Biomed. Pharmacother. 113, 108748. doi:10.1016/j.biopha.2019.108748
Lässer, C., O’Neil, S. E., Shelke, G. V., Sihlbom, C., Hansson, S. F., Gho, Y. S., et al. (2016). Exosomes in the Nose Induce Immune Cell Trafficking and Harbour an Altered Protein Cargo in Chronic Airway Inflammation. J. Transl Med. 14, 181. doi:10.1186/s12967-016-0927-4
Lee, J. H., Park, J., and Lee, J.-W. (2019). Therapeutic Use of Mesenchymal Stem Cell-Derived Extracellular Vesicles in Acute Lung Injury. Transfusion 59, 876–883. doi:10.1111/trf.14838
Lee, R. H., Pulin, A. A., Seo, M. J., Kota, D. J., Ylostalo, J., Larson, B. L., et al. (2009). Intravenous hMSCs Improve Myocardial Infarction in Mice Because Cells Embolized in Lung Are Activated to Secrete the Anti-inflammatory Protein TSG-6. Cell Stem Cell 5, 54–63. doi:10.1016/j.stem.2009.05.003
Lefrançais, E., Ortiz-Muñoz, G., Caudrillier, A., Mallavia, B., Liu, F., Sayah, D. M., et al. (2017). The Lung Is a Site of Platelet Biogenesis and a Reservoir for Haematopoietic Progenitors. Nature 544, 105–109. doi:10.1038/nature21706
Leng, Z., Zhu, R., Hou, W., Feng, Y., Yang, Y., Han, Q., et al. (2020). Transplantation of ACE2- Mesenchymal Stem Cells Improves the Outcome of Patients with COVID-19 Pneumonia. Aging Dis. 11, 216–228. doi:10.14336/AD.2020.0228
Li, C., Li, J., Li, Y., Lang, S., Yougbare, I., Zhu, G., et al. (2012). Crosstalk Between Platelets and the Immune System: Old Systems with New Discoveries. Adv. Hematol. 2012, 1–14. doi:10.1155/2012/384685
Li, F., Li, W., Farzan, M., and Harrison, S. C. (2005). Structure of SARS Coronavirus Spike Receptor-Binding Domain Complexed with Receptor. Science 309, 1864–1868. doi:10.1126/science.1116480
Li, J., Huang, S., Wu, Y., Gu, C., Gao, D., Feng, C., et al. (2014). Paracrine Factors from Mesenchymal Stem Cells: A Proposed Therapeutic Tool for Acute Lung Injury and Acute Respiratory Distress Syndrome. Int. Wound J. 11, 114–121. doi:10.1111/iwj.12202
Li, S., Li, S., Wu, S., and Chen, L. (2019). Exosomes Modulate the Viral Replication and Host Immune Responses in HBV Infection. Biomed. Res. Int. 2019, 1–9. doi:10.1155/2019/2103943
Li, Y., Xu, J., Shi, W., Chen, C., Shao, Y., Zhu, L., et al. (2016). Mesenchymal Stromal Cell Treatment Prevents H9N2 Avian Influenza Virus-Induced Acute Lung Injury in Mice. Stem Cell Res. Ther. 7, 159. doi:10.1186/s13287-016-0395-z
Liao, D., Zhou, F., Luo, L., Xu, M., Wang, H., Xia, J., et al. (2020). Haematological Characteristics and Risk Factors in the Classification and Prognosis Evaluation of COVID-19: A Retrospective Cohort Study. Lancet Haematol. 7, e671–e678. doi:10.1016/S2352-3026(20)30217-9
Lindemann, S., Tolley, N. D., Dixon, D. A., McIntyre, T. M., Prescott, S. M., Zimmerman, G. A., et al. (2001). Activated Platelets Mediate Inflammatory Signaling by Regulated Interleukin 1β Synthesis. J. Cell Biol. 154, 485–490. doi:10.1083/jcb.200105058
Lippi, G., and Favaloro, E. J. (2020a). D-dimer Is Associated with Severity of Coronavirus Disease 2019: A Pooled Analysis. Thromb. Haemost. 120, 876–878. doi:10.1055/s-0040-1709650
Lippi, G., Plebani, M., and Henry, B. M. (2020b). Thrombocytopenia Is Associated with Severe Coronavirus Disease 2019 (COVID-19) Infections: A Meta-Analysis. Clin. Chim. Acta 506, 145–148. doi:10.1016/j.cca.2020.03.022
Liu, F. P., Dong, J. J., Sun, S. J., Gao, W. Y., Zhang, Z. W., Zhou, X. J., et al. (2012). Autologous Bone Marrow Stem Cell Transplantation in Critical Limb Ischemia: A Meta-Analysis of Randomized Controlled Trials. Chin. Med. J. (Engl) 125, 4296–4300. doi:10.3760/cma.j.issn.0366-6999.2012.23.024
Liu, Y., Sun, W., Guo, Y., Chen, L., Zhang, L., Zhao, S., et al. (2020). Association between Platelet Parameters and Mortality in Coronavirus Disease 2019: Retrospective Cohort Study. Platelets 31, 490–496. doi:10.1080/09537104.2020.1754383
Lv, L.-H., Wan, Y.-L., Lin, Y., Zhang, W., Yang, M., Li, G.-L., et al. (2012). Anticancer Drugs Cause Release of Exosomes with Heat Shock Proteins from Human Hepatocellular Carcinoma Cells that Elicit Effective Natural Killer Cell Antitumor Responses In Vitro. J. Biol. Chem. 287, 15874–15885. doi:10.1074/jbc.M112.340588
Ma, Q., Fan, Q., Xu, J., Bai, J., Han, X., Dong, Z., et al. (2020). Calming Cytokine Storm in Pneumonia by Targeted Delivery of TPCA-1 Using Platelet-Derived Extracellular Vesicles. Matter 3, 287–301. doi:10.1016/j.matt.2020.05.017
Maas, S. L. N., Breakefield, X. O., and Weaver, A. M. (2017). Extracellular Vesicles: Unique Intercellular Delivery Vehicles. Trends Cell Biol. 27, 172–188. doi:10.1016/j.tcb.2016.11.003
Machlus, K. R., and Italiano, J. E. (2013). The Incredible Journey: From Megakaryocyte Development to Platelet Formation. J. Cell Biol. 201, 785–796. doi:10.1083/jcb.201304054
Mack, M., Kleinschmidt, A., Brühl, H., Klier, C., Nelson, P. J., Cihak, J., et al. (2000). Transfer of the Chemokine Receptor CCR5 between Cells by Membrane-Derived Microparticles: A Mechanism for Cellular Human Immunodeficiency Virus 1 Infection. Nat. Med. 6, 769–775. doi:10.1038/77498
Mackman, N., Antoniak, S., Wolberg, A. S., Kasthuri, R., and Key, N. S. (2020). Coagulation Abnormalities and Thrombosis in Patients Infected with SARS-CoV-2 and Other Pandemic Viruses. Arterioscler. Thromb. Vasc. Biol. 40, 2033–2044. doi:10.1161/ATVBAHA.120.314514
Maione, F., Cappellano, G., Bellan, M., Raineri, D., and Chiocchetti, A. (2020). Chicken‐or‐egg Question: Which Came First, Extracellular Vesicles or Autoimmune Diseases? J. Leukoc. Biol. 108, 601–616. doi:10.1002/JLB.3MR0120-232R
Malhotra, H., Sheokand, N., Kumar, S., Chauhan, A. S., Kumar, M., Jakhar, P., et al. (2016). Exosomes: Tunable Nano Vehicles for Macromolecular Delivery of Transferrin and Lactoferrin to Specific Intracellular Compartment. J. Biomed. Nanotechnol 12, 1101–1114. doi:10.1166/jbn.2016.2229
Mansouri, N., Willis, G. R., Fernandez-Gonzalez, A., Reis, M., Nassiri, S., Mitsialis, S. A., et al. (2019). Mesenchymal Stromal Cell Exosomes Prevent and Revert Experimental Pulmonary Fibrosis through Modulation of Monocyte Phenotypes. JCI Insight 4, e128060. doi:10.1172/jci.insight.128060
Marini, J. J., and Gattinoni, L. (2020). Management of COVID-19 Respiratory Distress. JAMA 323, 2329–2330. doi:10.1001/jama.2020.6825
Martin-Medina, A., Lehmann, M., Burgy, O., Hermann, S., Baarsma, H. A., Wagner, D. E., et al. (2018). Increased Extracellular Vesicles Mediate WNT5A Signaling in Idiopathic Pulmonary Fibrosis. Am. J. Respir. Crit. Care Med. 198, 1527–1538. doi:10.1164/rccm.201708-1580OC
Martinez-Bravo, M.-J., Wahlund, C. J. E., Qazi, K. R., Moulder, R., Lukic, A., Rådmark, O., et al. (2017). Pulmonary Sarcoidosis Is Associated with Exosomal Vitamin D-Binding Protein and Inflammatory Molecules. J. Allergy Clin. Immunol. 139, 1186–1194. doi:10.1016/j.jaci.2016.05.051
Martinod, K., and Wagner, D. D. (2014). Thrombosis: Tangled up in NETs. Blood 123, 2768–2776. doi:10.1182/blood-2013-10-463646
Matthay, M. A., Calfee, C. S., Zhuo, H., Thompson, B. T., Wilson, J. G., Levitt, J. E., et al. (2019). Treatment with Allogeneic Mesenchymal Stromal Cells for Moderate to Severe Acute Respiratory Distress Syndrome (START Study): A Randomised Phase 2a Safety Trial. Lancet Respir. Med. 7, 154–162. doi:10.1016/S2213-2600(18)30418-1
Maugeri, N., Capobianco, A., Rovere-Querini, P., Ramirez, G. A., Tombetti, E., Valle, P. D., et al. (2018). Platelet Microparticles Sustain Autophagy-Associated Activation of Neutrophils in Systemic Sclerosis. Sci. Transl. Med. 10, eaao3089. doi:10.1126/scitranslmed.aao3089
McIntyre, L. A., Stewart, D. J., Mei, S. H. J., Courtman, D., Watpool, I., Granton, J., et al. (2018). Cellular Immunotherapy for Septic Shock. A Phase I Clinical Trial. Am. J. Respir. Crit. Care Med. 197, 337–347. doi:10.1164/rccm.201705-1006OC
Mei, S. H. J., Haitsma, J. J., Dos Santos, C. C., Deng, Y., Lai, P. F. H., Slutsky, A. S., et al. (2010). Mesenchymal Stem Cells Reduce Inflammation while Enhancing Bacterial Clearance and Improving Survival in Sepsis. Am. J. Respir. Crit. Care Med. 182, 1047–1057. doi:10.1164/rccm.201001-0010OC
Melki, I., Tessandier, N., Zufferey, A., and Boilard, E. (2017). Platelet Microvesicles in Health and Disease. Platelets 28, 214–221. doi:10.1080/09537104.2016.1265924
Meng, F., Xu, R., Wang, S., Xu, Z., Zhang, C., Li, Y., et al. (2020). Human Umbilical Cord-Derived Mesenchymal Stem Cell Therapy in Patients with COVID-19: A Phase 1 Clinical Trial. Signal Transduct. Target. Ther. 5, 172. doi:10.1038/s41392-020-00286-5
Messner, C. B., Demichev, V., Wendisch, D., Michalick, L., White, M., Freiwald, A., et al. (2020). Ultra-High-Throughput Clinical Proteomics Reveals Classifiers of COVID-19 Infection. Cell Syst. 11, 11–24. doi:10.1016/j.cels.2020.05.012
Middleton, E. A., He, X.-Y., Denorme, F., Campbell, R. A., Ng, D., Salvatore, S. P., et al. (2020). Neutrophil Extracellular Traps Contribute to Immunothrombosis in COVID-19 Acute Respiratory Distress Syndrome. Blood 136, 1169–1179. doi:10.1182/blood.2020007008
Mo, X., Jian, W., Su, Z., Chen, M., Peng, H., Peng, P., et al. (2020). Abnormal Pulmonary Function in COVID-19 Patients at Time of Hospital Discharge. Eur. Respir. J. 55, 2001217. doi:10.1183/13993003.01217-2020
Monsel, A., Zhu, Y.-g., Gennai, S., Hao, Q., Hu, S., Rouby, J.-J., et al. (2015). Therapeutic Effects of Human Mesenchymal Stem Cell-Derived Microvesicles in Severe Pneumonia in Mice. Am. J. Respir. Crit. Care Med. 192, 324–336. doi:10.1164/rccm.201410-1765OC
Morrell, C. N., Aggrey, A. A., Chapman, L. M., and Modjeski, K. L. (2014). Emerging Roles for Platelets as Immune and Inflammatory Cells. Blood 123, 2759–2767. doi:10.1182/blood-2013-11-462432
Mousavizadeh, L., and Ghasemi, S. (2021). Genotype and Phenotype of COVID-19: Their Roles in Pathogenesis. J. Microbiol. Immunol. Infect. 54, 159–163. doi:10.1016/j.jmii.2020.03.022
Muraca, M., Piccoli, M., Franzin, C., Tolomeo, A., Jurga, M., Pozzobon, M., et al. (2017). Diverging Concepts and Novel Perspectives in Regenerative Medicine. Int. J. Mol. Sci. 18, 1021. doi:10.3390/ijms18051021
Nicola, M., Alsafi, Z., Sohrabi, C., Kerwan, A., Al-Jabir, A., Iosifidis, C., et al. (2020). The Socio-Economic Implications of the Coronavirus Pandemic (COVID-19): A Review. Int. J. Surg. 78, 185–193. doi:10.1016/j.ijsu.2020.04.018
Nolte-’t Hoen, E., Cremer, T., Gallo, R. C., and Margolis, L. B. (2016). Extracellular Vesicles and Viruses: Are They Close Relatives? Proc. Natl. Acad. Sci. U S A. 113, 9155–9161. doi:10.1073/pnas.1605146113
Owczarek, K., Szczepanski, A., Milewska, A., Baster, Z., Rajfur, Z., Sarna, M., et al. (2018). Early Events During Human Coronavirus OC43 Entry to the Cell. Sci. Rep. 8, 7124. doi:10.1038/s41598-018-25640-0
Park, J., Kim, S., Lim, H., Liu, A., Hu, S., Lee, J., et al. (2019). Therapeutic Effects of Human Mesenchymal Stem Cell Microvesicles in an Ex Vivo Perfused Human Lung Injured with Severe E. coli Pneumonia. Thorax 74, 43–50. doi:10.1136/thoraxjnl-2018-211576
Park, J. S., Shim, M.-S., Shim, S. H., Yang, H. N., Jeon, S. Y., Woo, D. G., et al. (2011). Chondrogenic Potential of Stem Cells Derived from Amniotic Fluid, Adipose Tissue, or Bone Marrow Encapsulated in Fibrin Gels Containing TGF-Β3. Biomaterials 32, 8139–8149. doi:10.1016/j.biomaterials.2011.07.043
Pascucci, L., Coccè, V., Bonomi, A., Ami, D., Ceccarelli, P., Ciusani, E., et al. (2014). Paclitaxel Is Incorporated by Mesenchymal Stromal Cells and Released in Exosomes that Inhibit In Vitro Tumor Growth: A New Approach for Drug Delivery. J. Control. Release 192, 262–270. doi:10.1016/j.jconrel.2014.07.042
Patel, D. B., Santoro, M., Born, L. J., Fisher, J. P., and Jay, S. M. (2018). Towards Rationally Designed Biomanufacturing of Therapeutic Extracellular Vesicles: Impact of the Bioproduction Microenvironment. Biotechnol. Adv. 36, 2051–2059. doi:10.1016/j.biotechadv.2018.09.001
Pedersen, S. F., and Ho, Y.-C. (2020). SARS-CoV-2: A Storm Is Raging. J. Clin. Invest. 130, 2202–2205. doi:10.1172/JCI137647
Perrone, F., Piccirillo, M. C., Piccirillo, M. C., Ascierto, P. A., Salvarani, C., Parrella, R., et al. (2020). Tocilizumab for Patients with COVID-19 Pneumonia. The Single-Arm TOCIVID-19 Prospective Trial. J. Transl Med. 18, 405. doi:10.1186/s12967-020-02573-9
Phinney, D. G., and Pittenger, M. F. (2017). Concise Review: MSC-Derived Exosomes for Cell-free Therapy. Stem Cells 35, 851–858. doi:10.1002/stem.2575
Pittenger, M. F., Discher, D. E., Péault, B. M., Phinney, D. G., Hare, J. M., and Caplan, A. I. (2019). Mesenchymal Stem Cell Perspective: Cell Biology to Clinical Progress. NPJ Regen. Med. 4, 22. doi:10.1038/s41536-019-0083-6
Porzionato, A., Zaramella, P., Dedja, A., Guidolin, D., Van Wemmel, K., Macchi, V., et al. (2019). Intratracheal Administration of Clinical-Grade Mesenchymal Stem Cell-Derived Extracellular Vesicles Reduces Lung Injury in a Rat Model of Bronchopulmonary Dysplasia. Am. J. Physiol. Lung Cell Mol. Physiol. 316, L6–L19. doi:10.1152/ajplung.00109.2018
Ragni, E., Banfi, F., Barilani, M., Cherubini, A., Parazzi, V., Larghi, P., et al. (2017). Extracellular Vesicle-Shuttled mRNA in Mesenchymal Stem Cell Communication. Stem Cells 35, 1093–1105. doi:10.1002/stem.2557
Raposo, G., and Stoorvogel, W. (2013). Extracellular Vesicles: Exosomes, Microvesicles, and Friends. J. Cell Biol. 200, 373–383. doi:10.1083/jcb.201211138
Rezaie, J., Aslan, C., Ahmadi, M., Zolbanin, N. M., Kashanchi, F., and Jafari, R. (2021). The Versatile Role of Exosomes in Human Retroviral Infections: From Immunopathogenesis to Clinical Application. Cell Biosci 11, 19. doi:10.1186/s13578-021-00537-0
Rezaie, J., Mehranjani, M. S., Rahbarghazi, R., and Shariatzadeh, M. A. (2018). Angiogenic and Restorative Abilities of Human Mesenchymal Stem Cells Were Reduced Following Treatment with Serum from Diabetes Mellitus Type 2 Patients. J. Cell. Biochem. 119, 524–535. doi:10.1002/jcb.26211
Rezaie, J., Rahbarghazi, R., Pezeshki, M., Mazhar, M., Yekani, F., Khaksar, M., et al. (2019). Cardioprotective Role of Extracellular Vesicles: A Highlight on Exosome Beneficial Effects in Cardiovascular Diseases. J. Cell. Physiol. 234, 21732–21745. doi:10.1002/jcp.28894
Ridger, V. C., Boulanger, C. M., Angelillo-Scherrer, A., Badimon, L., Blanc-Brude, O., Bochaton-Piallat, M.-L., et al. (2017). Microvesicles in Vascular Homeostasis and Diseases. Thromb. Haemost. 117, 1296–1316. doi:10.1160/TH16-12-0943
Roberts, H., Hoffman, M., and Monroe, D. (2006). A Cell-Based Model of Thrombin Generation. Semin. Thromb. Hemost. 32 (Suppl. 1), 32–38. doi:10.1055/s-2006-939552
Romagnoli, G. G., Zelante, B. B., Toniolo, P. c. A., Migliori, I. K., and Barbuto, J. A. M. (2014). Dendritic Cell-Derived Exosomes May Be a Tool for Cancer Immunotherapy by Converting Tumor Cells into Immunogenic Targets. Front. Immunol. 5, 692. doi:10.3389/fimmu.2014.00692
Rosell, A., Havervall, S., von Meijenfeldt, F., Hisada, Y., Aguilera, K., Grover, S. P., et al. (2021). Patients with COVID-19 Have Elevated Levels of Circulating Extracellular Vesicle Tissue Factor Activity that Is Associated with Severity and Mortality-Brief Report. Arterioscler. Thromb. Vasc. Biol. 41, 878–882. doi:10.1161/ATVBAHA.120.315547
Scheller, N., Herold, S., Kellner, R., Bertrams, W., Jung, A. L., Janga, H., et al. (2019). Proviral MicroRNAs Detected in Extracellular Vesicles from Bronchoalveolar Lavage Fluid of Patients with Influenza Virus-Induced Acute Respiratory Distress Syndrome. J. Infect. Dis. 219, 540–543. doi:10.1093/infdis/jiy554
Schneider, D. J., Speth, J. M., Penke, L. R., Wettlaufer, S. H., Swanson, J. A., and Peters-Golden, M. (2017). Mechanisms and Modulation of Microvesicle Uptake in a Model of Alveolar Cell Communication. J. Biol. Chem. 292, 20897–20910. doi:10.1074/jbc.M117.792416
Semple, J. W., Italiano, J. E., and Freedman, J. (2011). Platelets and the Immune Continuum. Nat. Rev. Immunol. 11, 264–274. doi:10.1038/nri2956
Sengupta, V., Sengupta, S., Lazo, A., Woods, P., Nolan, A., and Bremer, N. (2020). Exosomes Derived from Bone Marrow Mesenchymal Stem Cells as Treatment for Severe COVID-19. Stem Cells Dev. 29, 747–754. doi:10.1089/scd.2020.0080
Shah, T. G., Predescu, D., and Predescu, S. (2019). Mesenchymal Stem Cells‐derived Extracellular Vesicles in Acute Respiratory Distress Syndrome: A Review of Current Literature and Potential Future Treatment Options. Clin. Transl. Med. 8, 25. doi:10.1186/s40169-019-0242-9
Sheng, G., Chen, P., Wei, Y., Yue, H., Chu, J., Zhao, J., et al. (2020). Viral Infection Increases the Risk of Idiopathic Pulmonary Fibrosis. Chest 157, 1175–1187. doi:10.1016/j.chest.2019.10.032
Silini, A. R., Magatti, M., Cargnoni, A., and Parolini, O. (2017). Is Immune Modulation the Mechanism Underlying the Beneficial Effects of Amniotic Cells and Their Derivatives in Regenerative Medicine? Cell Transplant. 26, 531–539. doi:10.3727/096368916X693699
Simonson, O. E., Ståhle, E., Hansen, T., Wedin, J. O., Larsson, A., Mattsson, M., et al. (2020). Five-Year Follow-Up After Mesenchymal Stromal Cell-Based Treatment of Severe Acute Respiratory Distress Syndrome. Am. J. Respir. Crit. Care Med. 202, 1051–1055. doi:10.1164/rccm.202003-0544LE
Soni, S., Wilson, M. R., O'Dea, K. P., Yoshida, M., Katbeh, U., Woods, S. J., et al. (2016). Alveolar Macrophage-Derived Microvesicles Mediate Acute Lung Injury. Thorax 71, 1020–1029. doi:10.1136/thoraxjnl-2015-208032
Soy, M., Keser, G., Atagündüz, P., Tabak, F., Atagündüz, I., and Kayhan, S. (2020). Cytokine Storm in COVID-19: Pathogenesis and Overview of Anti-inflammatory Agents Used in Treatment. Clin. Rheumatol. 39, 2085–2094. doi:10.1007/s10067-020-05190-5
Sreeramkumar, V., Adrover, J. M., Ballesteros, I., Cuartero, M. I., Rossaint, J., Bilbao, I., et al. (2014). Neutrophils Scan for Activated Platelets to Initiate Inflammation. Science 346, 1234–1238. doi:10.1126/science.1256478
Statello, L., Maugeri, M., Garre, E., Nawaz, M., Wahlgren, J., Papadimitriou, A., et al. (2018). Identification of RNA-Binding Proteins in Exosomes Capable of Interacting with Different Types of RNA: RBP-Facilitated Transport of RNAs into Exosomes. PLoS One 13, e0195969. doi:10.1371/journal.pone.0195969
Stebbing, J., Phelan, A., Griffin, I., Tucker, C., Oechsle, O., Smith, D., et al. (2020). COVID-19: Combining Antiviral and Anti-inflammatory Treatments. Lancet Infect. Dis. 20, 400–402. doi:10.1016/S1473-3099(20)30132-8
Sun, P., Qie, S., Liu, Z., Ren, J., Li, K., and Xi, J. (2020). Clinical Characteristics of Hospitalized Patients with SARS‐CoV‐2 Infection: A Single Arm Meta‐analysis. J. Med. Virol. 92, 612–617. doi:10.1002/jmv.25735
Tang, N., Li, D., Wang, X., and Sun, Z. (2020). Abnormal Coagulation Parameters Are Associated with Poor Prognosis in Patients with Novel Coronavirus Pneumonia. J. Thromb. Haemost. 18, 844–847. doi:10.1111/jth.14768
Tao, S.-C., Guo, S.-C., and Zhang, C.-Q. (2017). Platelet-derived Extracellular Vesicles: An Emerging Therapeutic Approach. Int. J. Biol. Sci. 13, 828–834. doi:10.7150/ijbs.19776
Thille, A. W., Esteban, A., Fernández-Segoviano, P., Rodriguez, J.-M., Aramburu, J.-A., Vargas-Errázuriz, P., et al. (2013). Chronology of Histological Lesions in Acute Respiratory Distress Syndrome with Diffuse Alveolar Damage: A Prospective Cohort Study of Clinical Autopsies. Lancet Respir. Med. 1, 395–401. doi:10.1016/S2213-2600(13)70053-5
Thompson, M., Mei, S. H. J., Wolfe, D., Champagne, J., Fergusson, D., Stewart, D. J., et al. (2020). Cell Therapy with Intravascular Administration of Mesenchymal Stromal Cells Continues to Appear Safe: An Updated Systematic Review and Meta-Analysis. EClinicalMedicine 19, 100249. doi:10.1016/j.eclinm.2019.100249
Tkach, M., Kowal, J., and Théry, C. (2018). Why the Need and How to Approach the Functional Diversity of Extracellular Vesicles. Philos. Trans. R. Soc. Lond. B Biol. Sci. 373, 20160479. doi:10.1098/rstb.2016.0479
Toniati, P., Piva, S., Cattalini, M., Garrafa, E., Regola, F., Castelli, F., et al. (2020). Tocilizumab for the Treatment of Severe COVID-19 Pneumonia with Hyperinflammatory Syndrome and Acute Respiratory Failure: A Single center Study of 100 Patients in Brescia, Italy. Autoimmun. Rev. 19, 102568. doi:10.1016/j.autrev.2020.102568
Torreggiani, E., Perut, F., Perut, F., Roncuzzi, L., Zini, N., Baglìo, S., et al. (2014). Exosomes: Novel Effectors of Human Platelet Lysate Activity. Eur. Cell Mater. 28, 137–151. doi:10.22203/ecm.v028a11
Tsiapalis, D., and O’Driscoll, L. (2020). Mesenchymal Stem Cell Derived Extracellular Vesicles for Tissue Engineering and Regenerative Medicine Applications. Cells 9, 991. doi:10.3390/cells9040991
Tukmechi, A., Rezaee, J., Nejati, V., and Sheikhzadeh, N. (2013). Effect of Acute and Chronic Toxicity of Paraquat on Immune System and Growth Performance in Rainbow trout, Oncorhynchus mykiss. Aquac. Res. 45, 1737–1743. doi:10.1111/are.12118
Vader, P., Mol, E. A., Pasterkamp, G., and Schiffelers, R. M. (2016). Extracellular Vesicles for Drug Delivery. Adv. Drug Deliv. Rev. 106, 148–156. doi:10.1016/j.addr.2016.02.006
van der Meel, R., Fens, M. H. A. M., Vader, P., van Solinge, W. W., Eniola-Adefeso, O., and Schiffelers, R. M. (2014). Extracellular Vesicles as Drug Delivery Systems: Lessons from the Liposome Field. J. Control. Release 195, 72–85. doi:10.1016/j.jconrel.2014.07.049
van Dongen, H. M., Masoumi, N., Witwer, K. W., and Pegtel, D. M. (2016). Extracellular Vesicles Exploit Viral Entry Routes for Cargo Delivery. Microbiol. Mol. Biol. Rev. 80, 369–386. doi:10.1128/MMBR.00063-15
Varga, Z., Flammer, A. J., Steiger, P., Haberecker, M., Andermatt, R., Zinkernagel, A. S., et al. (2020). Endothelial Cell Infection and Endotheliitis in COVID-19. Lancet 395, 1417–1418. doi:10.1016/S0140-6736(20)30937-5
Volarevic, V., Markovic, B. S., Gazdic, M., Volarevic, A., Jovicic, N., Arsenijevic, N., et al. (2018). Ethical and Safety Issues of Stem Cell-Based Therapy. Int. J. Med. Sci. 15, 36–45. doi:10.7150/ijms.21666
Wada, N., Gronthos, S., and Bartold, P. M. (2013). Immunomodulatory Effects of Stem Cells. Periodontol. 2000 63, 198–216. doi:10.1111/prd.120242013
Wan, Y., Shang, J., Graham, R., Baric, R. S., and Li, F. (2020). Receptor Recognition by the Novel Coronavirus from Wuhan: An Analysis Based on Decade-Long Structural Studies of SARS Coronavirus. J. Virol. 94, e00127-20. doi:10.1128/JVI.00127-20
Wang, B., Yao, K., Huuskes, B. M., Shen, H.-H., Zhuang, J., Godson, C., et al. (2016). Mesenchymal Stem Cells Deliver Exogenous MicroRNA-Let7c via Exosomes to Attenuate Renal Fibrosis. Mol. Ther. 24, 1290–1301. doi:10.1038/mt.2016.90
Wang, J., Chen, S., and Bihl, J. (2020). Exosome-Mediated Transfer of ACE2 (Angiotensin-Converting Enzyme 2) from Endothelial Progenitor Cells Promotes Survival and Function of Endothelial Cell. Oxid. Med. Cell Longev. 2020, 1–11. doi:10.1155/2020/4213541
Wecht, S., and Rojas, M. (2016). Mesenchymal Stem Cells in the Treatment of Chronic Lung Disease. Respirology 21, 1366–1375. doi:10.1111/resp.12911
Wichmann, D., Sperhake, J.-P., Lütgehetmann, M., Steurer, S., Edler, C., Heinemann, A., et al. (2020). Autopsy Findings and Venous Thromboembolism in Patients with COVID-19. Ann. Intern. Med. 173, 268–277. doi:10.7326/M20-2003
Wiklander, O. P. B., Brennan, M. Á., Lötvall, J., Breakefield, X. O., and El Andaloussi, S. (2019). Advances in Therapeutic Applications of Extracellular Vesicles. Sci. Transl. Med. 11, eaav8521. doi:10.1126/scitranslmed.aav8521
Willis, G. R., Fernandez-Gonzalez, A., Anastas, J., Vitali, S. H., Liu, X., Ericsson, M., et al. (2018). Mesenchymal Stromal Cell Exosomes Ameliorate Experimental Bronchopulmonary Dysplasia and Restore Lung Function through Macrophage Immunomodulation. Am. J. Respir. Crit. Care Med. 197, 104–116. doi:10.1164/rccm.201705-0925OC
Xu, P., Zhou, Q., and Xu, J. (2020a). Mechanism of Thrombocytopenia in COVID-19 Patients. Ann. Hematol. 99, 1205–1208. doi:10.1007/s00277-020-04019-0
Xu, X., Chen, P., Wang, J., Feng, J., Zhou, H., Li, X., et al. (2020b). Evolution of the Novel Coronavirus from the Ongoing Wuhan Outbreak and Modeling of its Spike Protein for Risk of Human Transmission. Sci. China Life Sci. 63, 457–460. doi:10.1007/s11427-020-1637-5
Xu, X., Han, M., Li, T., Sun, W., Wang, D., Fu, B., et al. (2020c). Effective Treatment of Severe COVID-19 Patients with Tocilizumab. Proc. Natl. Acad. Sci. USA 117, 10970–10975. doi:10.1073/pnas.2005615117
Xu, Z., Shi, L., Wang, Y., Zhang, J., Huang, L., Zhang, C., et al. (2020d). Pathological Findings of COVID-19 Associated with Acute Respiratory Distress Syndrome. Lancet Respir. Med. 8, 420–422. doi:10.1016/S2213-2600(20)30076-X
Yan, R., Zhang, Y., Li, Y., Xia, L., Guo, Y., and Zhou, Q. (2020). Structural Basis for the Recognition of SARS-CoV-2 by Full-Length Human ACE2. Science 367, 1444–1448. doi:10.1126/science.abb2762
Yang, L., Liu, S., Liu, J., Zhang, Z., Wan, X., Huang, B., et al. (2020a). COVID-19: Immunopathogenesis and Immunotherapeutics. Signal Transduct. Target. Ther. 5, 128. doi:10.1038/s41392-020-00243-2
Yang, X., Yang, Q., Wang, Y., Wu, Y., Xu, J., Yu, Y., et al. (2020b). Thrombocytopenia and its Association with Mortality in Patients with COVID‐19. J. Thromb. Haemost. 18, 1469–1472. doi:10.1111/jth.14848
Yi, Y., Lagniton, P. N. P., Ye, S., Li, E., and Xu, R.-H. (2020). COVID-19: What Has Been Learned and to Be Learned about the Novel Coronavirus Disease. Int. J. Biol. Sci. 16, 1753–1766. doi:10.7150/ijbs.45134
Yoshikawa, F. S. Y., Teixeira, F. M. E., Sato, M. N., and Oliveira, L. M. d. S. (2019). Delivery of MicroRNAs by Extracellular Vesicles in Viral Infections: Could the News Be Packaged? Cells 8, 611. doi:10.3390/cells8060611
Zaid, Y., Puhm, F., Allaeys, I., Naya, A., Oudghiri, M., Khalki, L., et al. (2020). Platelets Can Associate with SARS-Cov-2 RNA and Are Hyperactivated in COVID-19. Circ. Res. 127, 1404–1418. doi:10.1161/CIRCRESAHA.120.317703
Zemans, R. L., and Matthay, M. A. (2017). What Drives Neutrophils to the Alveoli in ARDS? Thorax 72, 1–3. doi:10.1136/thoraxjnl-2016-209170
Zhang, H., Penninger, J. M., Li, Y., Zhong, N., and Slutsky, A. S. (2020a). Angiotensin-converting Enzyme 2 (ACE2) as a SARS-CoV-2 Receptor: Molecular Mechanisms and Potential Therapeutic Target. Intensive Care Med. 46, 586–590. doi:10.1007/s00134-020-05985-9
Zhang, P., Li, J., Liu, H., Han, N., Ju, J., Kou, Y., et al. (2020b). Long-term Bone and Lung Consequences Associated with Hospital-Acquired Severe Acute Respiratory Syndrome: A 15-year Follow-Up from a Prospective Cohort Study. Bone Res. 8, 8. doi:10.1038/s41413-020-0084-5
Zhao, Y., Zhao, Z., Wang, Y., Zhou, Y., Ma, Y., and Zuo, W. (2020). Single-Cell RNA Expression Profiling of ACE2, the Receptor of SARS-CoV-2. Am. J. Respir. Crit. Care Med. 202, 756–759. doi:10.1164/rccm.202001-0179LE
Zhou, P., Yang, X.-L., Wang, X.-G., Hu, B., Zhang, L., Zhang, W., et al. (2020a). A Pneumonia Outbreak Associated with a New Coronavirus of Probable Bat Origin. Nature 579, 270–273. doi:10.1038/s41586-020-2012-7
Zhou, W., Wang, H., Yang, Y., Chen, Z.-S., Zou, C., and Zhang, J. (2020b). Chloroquine Against Malaria, Cancers and Viral Diseases. Drug Discov. Today 25, 2012–2022. doi:10.1016/j.drudis.2020.09.010
Zhou, X., Xie, F., Wang, L., Zhang, L., Zhang, S., Fang, M., et al. (2020c). The Function and Clinical Application of Extracellular Vesicles in Innate Immune Regulation. Cell Mol Immunol 17, 323–334. doi:10.1038/s41423-020-0391-1
Zhu, F.-C., Guan, X.-H., Li, Y.-H., Huang, J.-Y., Jiang, T., Hou, L.-H., et al. (2020). Immunogenicity and Safety of a Recombinant Adenovirus Type-5-Vectored COVID-19 Vaccine in Healthy Adults Aged 18 Years or Older: A Randomised, Double-Blind, Placebo-Controlled, Phase 2 Trial. Lancet 396, 479–488. doi:10.1016/S0140-6736(20)31605-6
Keywords: COVID-19, SARS-CoV-2, extracellular vesicles (EVs), mesenchymal stem cell (MSC), immunomodulation, ACE2
Citation: Yan Y-y, Zhou W-m, Wang Y-q, Guo Q-r, Zhao F-x, Zhu Z-y, Xing Y-x, Zhang H-y, Aljofan M, Jarrahi AM, Makabel B and Zhang J-y (2021) The Potential Role of Extracellular Vesicles in COVID-19 Treatment: Opportunity and Challenge. Front. Mol. Biosci. 8:699929. doi: 10.3389/fmolb.2021.699929
Received: 24 April 2021; Accepted: 08 July 2021;
Published: 21 July 2021.
Edited by:
Wen Li, Zhejiang University, ChinaReviewed by:
Jafar Rezaie, Urmia University of Medical Sciences, IranLanlan Wei, Shenzhen Third People’s Hospital, China
Copyright © 2021 Yan, Zhou, Wang, Guo, Zhao, Zhu, Xing, Zhang, Aljofan, Jarrahi, Makabel and Zhang. This is an open-access article distributed under the terms of the Creative Commons Attribution License (CC BY). The use, distribution or reproduction in other forums is permitted, provided the original author(s) and the copyright owner(s) are credited and that the original publication in this journal is cited, in accordance with accepted academic practice. No use, distribution or reproduction is permitted which does not comply with these terms.
*Correspondence: Bolat Makabel, a3prdEAxNjMuY29t; Jian-ye Zhang, amlhbnllekAxNjMuY29t
†These authors have contributed equally to this work and share first authorship.