- Human Metabolomics, North-West University, Potchefstroom, South Africa
The HIV/AIDS (human immunodeficiency virus/acquired immunodeficiency syndrome) and tuberculosis (TB) pandemics are perpetuated by a significant global burden of HIV/TB co-infection. The synergy between HIV and Mycobacterium tuberculosis (Mtb) during co-infection of a host is well established. While this synergy is known to be driven by immunological deterioration, the metabolic mechanisms thereof remain poorly understood. Metabolomics has been applied to study various aspects of HIV and Mtb infection separately, yielding insights into infection- and treatment-induced metabolic adaptations experienced by the host. Despite the contributions that metabolomics has made to the field, this approach has not yet been systematically applied to characterize the HIV/TB co-infected state. Considering that limited HIV/TB co-infection metabolomics studies have been published to date, this review briefly summarizes what is known regarding the HIV/TB co-infection synergism from a conventional and metabolomics perspective. It then explores metabolomics as a tool for the improved characterization of HIV/TB co-infection in the context of previously published human-related HIV infection and TB investigations, respectively as well as for addressing the gaps in existing knowledge based on the similarities and deviating trends reported in these HIV infection and TB studies.
Introduction
Tuberculosis (TB) ranks as the top cause of death due to a single infectious pathogen, ranking above the human immunodeficiency virus (HIV), the causative agent of acquired immunodeficiency syndrome (AIDS) (World Health Organization, 2019). These diseases are pandemics with high morbidity and mortality rates (World Health Organization, 2020). Although HIV-positive individuals suffer from many serious secondary infections, TB is the most common disease, especially in sub-Saharan Africa. Due to the immunosuppressive effects of HIV on the host, HIV-positive individuals have a 26–31 times greater risk of developing active TB compared to HIV-negative individuals (Wood et al., 2000; World Health Organization, 2012). This increased risk, along with various other factors, contributes to the significant global burden of HIV/TB co-infection. Although pulmonary TB is the most common form of active TB in HIV/TB co-infected individuals, this population is also more prone to the development of independent or concurrent extrapulmonary TB, especially when CD4 T cell counts are low (Chaisson et al., 1987; Elliott et al., 1995; Schutz et al., 2010). When co-infection with these pathogens is established, they act synergistically to increase the disease burden in the host (Kwan and Ernst, 2011). As expected, the pathophysiology of Mycobacterium tuberculosis (Mtb) infection differs in HIV-positive individuals and even more so in those who have progressed to severe immunosuppression, i.e., AIDS, typically showing an accelerated clinical course (Badri et al., 2001; Schutz et al., 2010). In general, HIV/TB co-infected patients present with characteristic symptoms of active pulmonary TB (Burman and Jones, 2003; Luies and du Preez, 2020) but often have more severe systemic symptoms including fevers and weight loss. A mucus-producing cough is less common, which is linked to HIV-induced immunosuppression, as a less vigorous immune response results in fewer pulmonary complications (Selwyn et al., 1998; Garcia et al., 2007; Schutz et al., 2010). In 2019, 208,000 of the approximately 1.4 million TB-related deaths were among HIV-positive people (World Health Organization, 2020).
The incidence of smear-negative (culture-negative, symptomatic) and subclinical (culture-positive, asymptomatic active) TB is also higher among co-infected patients. This leads to decreased sensitivity in screening tests and subsequent diagnostic delays, as the most widely applied TB diagnostic tests are based on sputum examination (Schutz et al., 2010; Tiberi et al., 2017). This manifests as higher mortality rates for smear-negative HIV-positive TB patients, the discovery of subclinical TB only after the initiation of antiretroviral therapy (ART) (Sterling et al., 2010), and poor treatment outcomes for these patients. Therefore, it is recommended that all HIV-positive individuals are screened for Mtb infection, latent or otherwise (Tiberi et al., 2017). TB can be cured in these individuals, although treatment is complicated by various factors (Tiberi et al., 2017). Co-infected individuals also have an increased rate of recurrence, mainly due to reinfection as opposed to relapse (Sonnenberg et al., 2001; Charalambous et al., 2008).
Various aspects of the pathology of HIV infection, TB, and HIV/TB co-infection remain incompletely understood, which hampers the development of improved or novel diagnostic and management strategies. There is a need to better understand and characterize the complex metabolic changes induced by co-infection since many deaths can be prevented with timely diagnosis and treatment. Metabolomics is a promising tool in this regard and refers to the analysis of small molecules (i.e. metabolites) present in a biological system, to infer biological functions or changes in response to specific internal or external stimuli (Dunn et al., 2005).
Metabolomics has yielded new insights into HIV and Mtb infection- and treatment-induced metabolic alterations experienced by the host. Despite the contributions that metabolomics has made to date, this approach has not yet been systematically applied to characterize the HIV/TB co-infected state. Considering this, we briefly review the HIV/TB co-infection synergism from a conventional and metabolomics perspective. We then evaluate the role of metabolomics as a tool to investigate HIV/TB co-infection in the context of previously published human-related HIV infection (specifically relating to HIV-1) and TB investigations, respectively. This will inform the design of future experiments and allow for a better understanding of the contributions that HIV and Mtb make toward host metabolic adaptations during co-infection. Due to the complexity of the synergism between HIV and Mtb these metabolic changes might be similar, exacerbated, or altered, compared to HIV infection or TB alone.
Human Immunodeficiency Virus/Tuberculosis Co-infection: What do We Know and What are the Current Gaps?
HIV and Mtb display the same outcome in their interactions with the host: effective replication without killing the host for as long as possible, to improve the chances of transmission to a new host and perpetuation of this process. During co-infection, the core of the synergism, which amplifies the disease burden, lies in the deterioration of immunological functions (Pawlowski et al., 2012; Sharan et al., 2020). The overall immune activation is worse in patients with HIV/TB co-infection relative to those with TB only (Vanham et al., 1996). A detailed discussion of the immunology concerned is outside the scope of this review, however, several publications relating to this topic are available (Toossi, 2003; Diedrich and Flynn, 2011; Kwan and Ernst, 2011; Bell and Noursadeghi, 2018; Sharan et al., 2020).
Considering that immunological deterioration has profound effects on the host metabolism (Cheng et al., 2014; Li et al., 2017), the field of immunometabolism has emerged to better characterize these changes. Immunometabolism refers to the convergence of bioenergetic pathways and the specific functions of immune cells (Shi et al., 2016). To explain briefly, during HIV and Mtb infections, the collective actions of the innate and adaptive immune responses result in a concentrated effort to eliminate the pathogenic threats (Kwan and Ernst, 2011). A state of chronic inflammation is established (Semba et al., 2010; Aounallah et al., 2016) and the balance between the immune system and metabolism disrupted. This is mainly due to the significant energy demands and biosynthesis required to mount and sustain an immune response (Aounallah et al., 2016). As such, the infected host experiences anorexia via effects on the hypothalamus (Gautron and Laye, 2010), which collectively results in a negative energy balance, and the consequent utilization of stored fat and eventually protein (from muscle tissue) (Powanda and Beisel, 2003) for energy. This often culminates in malnutrition and the condition known as cachexia (Moldawer and Sattler, 1998), an established feature of both HIV infection and TB. Consequently, there is an inability to control infection, resulting in increased mortality (Zachariah et al., 2002; Paton et al., 2006).
To date, metabolic changes during HIV infection, TB, and HIV/TB co-infection have typically been measured by conventional assays [such as resting energy expenditure measurement by indirect calorimetry (Melchior et al., 1991; Schwenk et al., 2003), body composition measurement by dual-energy X-ray absorptiometry (Meininger et al., 2002; Schwenk et al., 2003) and nitrogen flux by the administration of, for example, a glycine isotope (Paton et al., 2003)]. Using such techniques, HIV infection was characterized by alterations in energy, glucose, lipid, and protein metabolism (Salas-Salvado and Garcia-Lorda, 2001), while active TB was characterized by altered protein metabolism (MacAllan et al., 1998), low serum cholesterol (Taylor and Bamgboye, 1979), and glucose intolerance (Oluboyo and Erasmus, 1990). In HIV/TB co-infected patients, protein metabolism was mainly affected. The net protein balance in the fed state was shown to be impaired, compared to a strongly anabolic protein balance in those with HIV infection or TB only (Paton et al., 2003). Serum albumin levels serve as indicators of protein status. HIV/TB co-infected patients were shown to suffer from hypoalbuminemia relative to TB patients (Van Lettow et al., 2003). The distribution and magnitude of body composition changes were not altered in HIV/TB co-infection relative to that of TB patients (Paton and Ng, 2006).
Although informative, conventional techniques allow for only one specific aspect of metabolism to be investigated at a time (Sitole et al., 2013). This complicates the process of forming a holistic, coherent view of infection- and treatment-induced metabolic alterations. Since metabolomics allows for the measurement of multiple metabolites at a time, it has aided in better understanding HIV infection and TB, respectively. In the case of HIV infection, additional changes in other carbohydrates, amino acids (as well as proteins), lipids, and nucleotides (and/or DNA), has been established by untargeted (Munshi et al., 2013; Williams et al., 2014; Sitole et al., 2015; Li et al., 2018; Sitole et al., 2019) and targeted (Riddler et al., 2008; Williams et al., 2011; Scarpellini et al., 2016; Peltenburg et al., 2018; Sitole et al., 2019) metabolomics studies in an untreated, treated, treatment over time, treatment response and/or comorbidity context (Rodriguez-Gallego et al., 2018; Babu et al., 2019; Rosado-Sanchez et al., 2019). Metabolomics has also been extensively applied to various aspects of TB research, i.e. metabolic mechanisms associated with latent Mtb infection and conversion to active disease, Mtb infection at the cellular level, active TB, comorbidities, as well as TB treatment (Luies et al., 2017; Vrieling et al., 2019; Albors-Vaquer et al., 2020; Vrieling et al., 2020). The approach has thus contributed, among others, to a better understanding of the pathogen, its interaction with the host, and its effect on the physiology of the host. Many studies have focused on the discovery of biomarkers for specific TB-related applications, but the results are often not extensively interpreted in a biological context. Metabolomics, however, has not yet been systematically applied to characterize the co-infected state.
It is worth mentioning that the metabolic characteristics of HIV/TB co-infection derived from conventional methods mainly focused on the synergistic effects of HIV infection, TB, and malnutrition, which together have been termed “triple trouble” with reference to the public health term “double trouble” when referring to HIV/TB co-infection (Van Lettow et al., 2003). The risk of malnutrition (Swaminathan et al., 2008) and the severity of wasting (Lucas et al., 1994; Villamor et al., 2006) are exacerbated during HIV/TB co-infection relative to either disease state alone, although the mechanisms underlying this are not fully understood. HIV infection is associated with the loss of gut mucosal integrity. The CD4 T cells of the gut mucosa are preferentially depleted, leading to impaired immune function in the gut (Ibarrondo et al., 2013; Sharan et al., 2020). This leads to a “leaky gut” and the translocation of gut microbial products into the blood, which exacerbates systemic immune activation (Brenchley et al., 2006; Sharan et al., 2020). Furthermore, it is well established that HIV induces changes in the gut microbiota composition (Vujkovic-Cvijin and Somsouk, 2019). How these processes are affected by Mtb during HIV/TB co-infection are not yet understood (Waters et al., 2020), although it is known that patients with TB also have an altered gut microbiota composition (Winglee et al., 2014; Luo et al., 2017; Hu et al., 2019).
The effects of HIV infection on the gut and the high energy requirement during chronic infection culminates in micronutrient deficiencies [reviewed in detail by Semba et al. (2010)] and alterations to the macronutrient status (e.g., increased utilization of protein and fat for energy). Micronutrients have major roles in maintaining homeostasis. Deficiencies have far-reaching complications for the immune system and metabolism (Katona and Katona-Apte, 2008), of which tracing the source might be extremely difficult due to the confounding effect of the inflammatory state and the inherent interconnectedness of metabolism.
Although aspects of metabolism and nutrition improve by treating HIV-positive or TB patients with ART and anti-TB drugs, respectively (Kennedy et al., 1996; Drain et al., 2007), these treatments do not restore patients to a healthy state. HIV-positive individuals often still suffer from wasting even after the initiation of ART (Tang et al., 2002). ART also induces metabolic alterations separate from HIV infection (van der Valk et al., 2002; Jain et al., 2005), while anti-TB treatment fails to completely recover protein reserves (Onwubalili, 1988; Schwenk et al., 2004) and induces oxidative stress (OS) (Sodhi et al., 1997). These effects are reflected in the metabolomics literature for HIV infection (Hewer et al., 2006; Li et al., 2018; Babu et al., 2019) and TB (Luies et al., 2017; Combrink et al., 2019; Opperman et al., 2021). The causes of malnutrition and wasting in HIV-positive and TB patients are multifactorial and are influenced by disease progression. It is known that the development and degree of HIV-induced wasting is influenced by factors such as CD4 T cell count (Forrester et al., 2001), viral load (Lyles et al., 1999; Zackin et al., 1999), inflammatory markers [reviewed by Chang et al. (1998)], and nutrition [reviewed by Koethe and Heimburger. (2010)]. The role of these factors and the HIV-induced gut complications mentioned above, in the co-infected individual is not well understood.
The signaling pathways involved in pathogenic control are closely involved in the regulation of nutrients and metabolic homeostasis (Aounallah et al., 2016) and are mediated by cytokines, chemokines, and various reactive oxygen species (ROS). Besides directly damaging the invading microbe, ROS stimulates the production of pro-inflammatory cytokines, which exacerbates the inflammatory state and perpetuates immunometabolic alterations (Zhang et al., 2018). Increased levels of ROS and pro-inflammatory cytokines are the hallmarks of chronic inflammation (Awodele et al., 2012), and have been studied in HIV infection (Williams et al., 2013), TB (Yao et al., 2017), and co-infection (Verma et al., 2018). Although these interactions have been linked to various metabolic changes and are more pronounced during co-infection (Madebo et al., 2003; Awodele et al., 2012), these are complex and not yet fully understood (Zhang et al., 2018).
No single factor can be attributed to the cause of the co-infected phenotype as the interactions between the host and the two pathogens are multidirectional: either oppositional or synergistic and to various degrees. Given that conventional assays are less sensitive, less specific, and laborious to perform (Williams et al., 2011), researchers have turned to newer, high-throughput omics techniques to better match the dimensionality of the problem to the data obtained and thus allow for a more comprehensive profile of host metabolism, as it relates to HIV/TB co-infection.
Human Immunodeficiency Virus/Tuberculosis Co-Infection and Metabolomics
Only three metabolomics investigations that study a very specific aspect of HIV/TB co-infection have been published to date (Table 1). To the best of our knowledge, no metabolomics study has hitherto characterized the HIV/TB co-infected metabolic profile relative to healthy individuals or either HIV-positive or TB-positive individuals, or in terms of a treatment-associated profile.
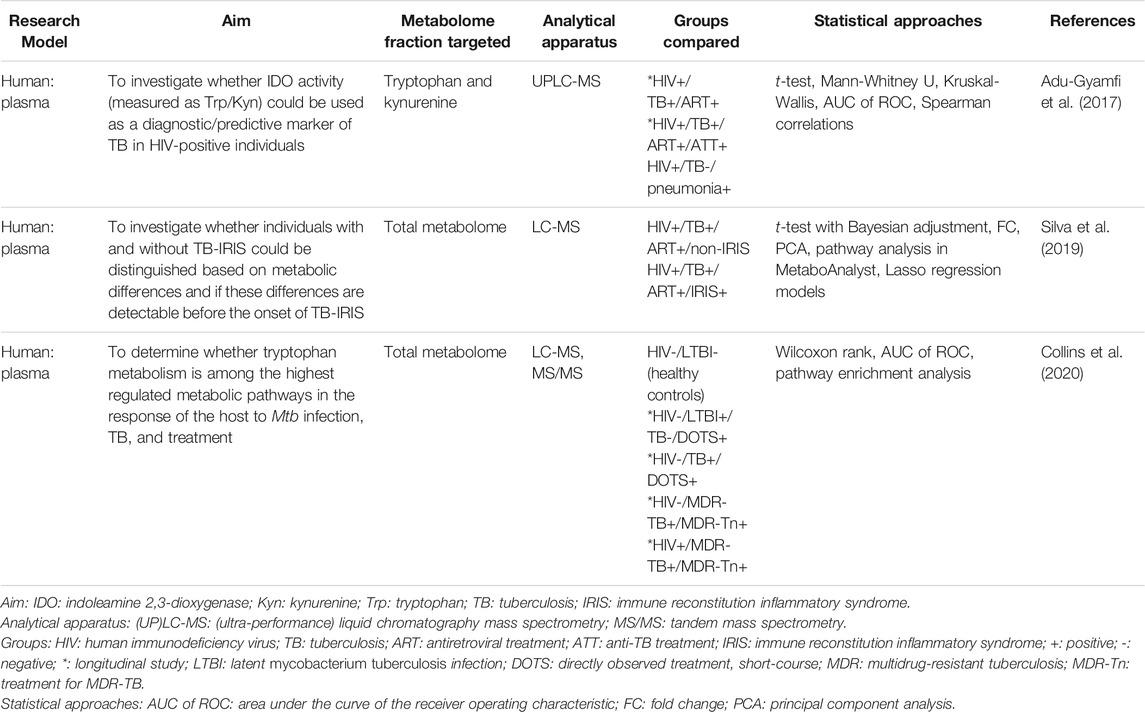
TABLE 1. Metabolomics studies published to date investigating a specific aspect of HIV/TB co-infection.
The optimal physiological function is reliant on a balanced tryptophan metabolism (Wang et al., 2021), as the immune, central nervous, and reproductive systems respond to alterations in its catabolic flux (Ball et al., 2014). The enzymes tryptophan 2,3-dioxygenase and indoleamine 2,3-dioxygenase 1 (IDO1) catabolize tryptophan via the kynurenine pathway, producing a range of immunomodulatory and neuroactive catabolites. IDO1 activity in particular has been widely investigated for its role in immune tolerance and suppression (Wang et al., 2021). Increased IDO1 activity, induced by interferon-γ (Katz et al., 2008), and the resulting alterations to the kynurenine/tryptophan (K/T) ratio have been investigated as biomarkers for various disease states (Sucher et al., 2010; Mancuso et al., 2015; Pett et al., 2017; Li et al., 2019), including HIV infection and TB measured conventionally (Almeida et al., 2009; Jenabian et al., 2013; Gostner et al., 2015; Dagenais-Lussier et al., 2016; Gautam et al., 2018; van Laarhoven et al., 2018) and via metabolomics (Weiner et al., 2012; Luies and Loots, 2016; Isa et al., 2018; Li et al., 2018; Peltenburg et al., 2018; Sitole et al., 2019).
Of the three studies mentioned, two investigated the effects of IDO1 activity on host metabolism during HIV/TB co-infection. Adu-Gyamfi et al. (2017) monitored HIV-positive individuals longitudinally for the development of active TB. At the time of diagnosis, IDO1 activity was higher in those individuals who developed TB compared to those who did not, an effect that was reversed after 6 months of anti-TB treatment. The IDO1 activity of HIV/TB co-infected individuals was also four-fold higher when compared to that of HIV-positive individuals with confirmed pneumonia. Collins et al. (2020) confirmed that individuals with HIV/TB co-infection have higher IDO1 activity compared to those with a single disease. Collins et al. (2020) further found that HIV-positive individuals with multidrug-resistant (MDR) TB also had an increased K/T ratio relative to those with TB alone. After receiving treatment for MDR-TB for 2 years, there was no significant change in the K/T ratio, which is in concurrence with a slower bacterial clearance rate in MDR-TB patients (Collins et al., 2020). Indoleamine 2,3-dioxygenase 2 (IDO2) plays a smaller role in tryptophan catabolism and has been suggested to promote pro-inflammatory B cell responses, as opposed to the immunosuppressive effects of IDO1, through the induction of T regulatory cell responses (Manches et al., 2008) and other mechanisms (Munn et al., 1999; Mellor et al., 2002; Merlo et al., 2020). The levels of IDO2 transcripts is higher during co-infection compared to individuals with TB alone, suggesting that HIV infection or the co-infected state causes increased production of IDO2 in addition to IDO1 (Collins et al., 2020). These studies indicate that increased IDO activity is significantly more pronounced in the HIV/TB co-infected state and that the K/T ratio may be a suitable biomarker for TB in HIV-positive patients (Adu-Gyamfi et al., 2017) and a possible target for host-directed therapies (Collins et al., 2020). Studies are needed to evaluate whether the significantly increased IDO1 activity contributes to the increased TB progression risk in HIV-positive individuals (Pawlowski et al., 2012; Collins et al., 2020) and those studies comparing HIV-positive individuals with other secondary (specifically respiratory) diseases as diseased controls to validate this are also warranted. Diagnostically, it would be ideal to include both HIV-negative TB-positive patients and HIV-positive TB-negative patients as controls in a single study.
Within the context of co-infection, ART is typically initiated based on a patient’s immunological status. Patients with CD4 <50 cells/mm3 or significantly advanced disease are prescribed to start ART within 2 weeks of starting anti-TB treatment, while those with an improved immunological profile (CD4 >50 cells/mm3) start ART two to 8 weeks after anti-TB treatment initiation. These guidelines have been proposed in response to the development of an aberrant immune response (immune reconstitution inflammatory syndrome; IRIS) in HIV/TB co-infected patients when ART is initiated early in the course of anti-TB treatment (Török et al., 2011; Manosuthi et al., 2012; World Health Organization, 2016b). IRIS is characterized by a severe inflammatory response that is triggered against a pre-existing condition in HIV-positive individuals shortly after the initiation of ART. When this reaction is directed against Mtb, it is referred to as TB-IRIS. It may be transient and resolve of its own, but it may also be fatal. Since there are currently no diagnostic tests for TB-IRIS, its diagnosis is based on clinical presentation and medical history (Pozniak et al., 2011; Tiberi et al., 2017). Considering this, Silva et al. (2019) collected samples from HIV/TB co-infected individuals after the initiation of ART and as close as possible to the onset of IRIS (at a time point compared to this for those who did not develop IRIS). Those individuals who developed IRIS experienced increased arachidonic acid (most notably increased hydroxyeicosatetraenoic acids) and glycerophospholipid metabolism relative to the non-IRIS group at the start of the study, whereas altered sphingolipid and linolenic acid metabolism distinguished the groups during the IRIS window. This study showed that altered polyunsaturated fatty acid metabolism distinguished patients who develop IRIS from those who do not. Since IRIS involves an acute inflammatory response these results are expected, as polyunsaturated fatty acids give rise to various inflammatory mediators. This study again underscores the reflection of immunological changes in the metabolism and its potential prognostic application.
The studies reviewed here stress the many gaps that exist in our knowledge of HIV/TB co-infection, including an incomplete understanding of the mechanisms underlying diagnostic problems, the effects of ART and anti-TB treatment on the host, recurrent TB, and the effect of Mtb on the gut mucosal damage induced by HIV. These studies also highlight the potential of metabolomics to address such gaps. Comparing the existing HIV infection and TB metabolomics profiles (Tables 2–4) in the absence of a comprehensive HIV/TB profile may yield insight into the mechanisms of what is known about host metabolism during HIV/TB co-infection and inform the design of future metabolomics studies.
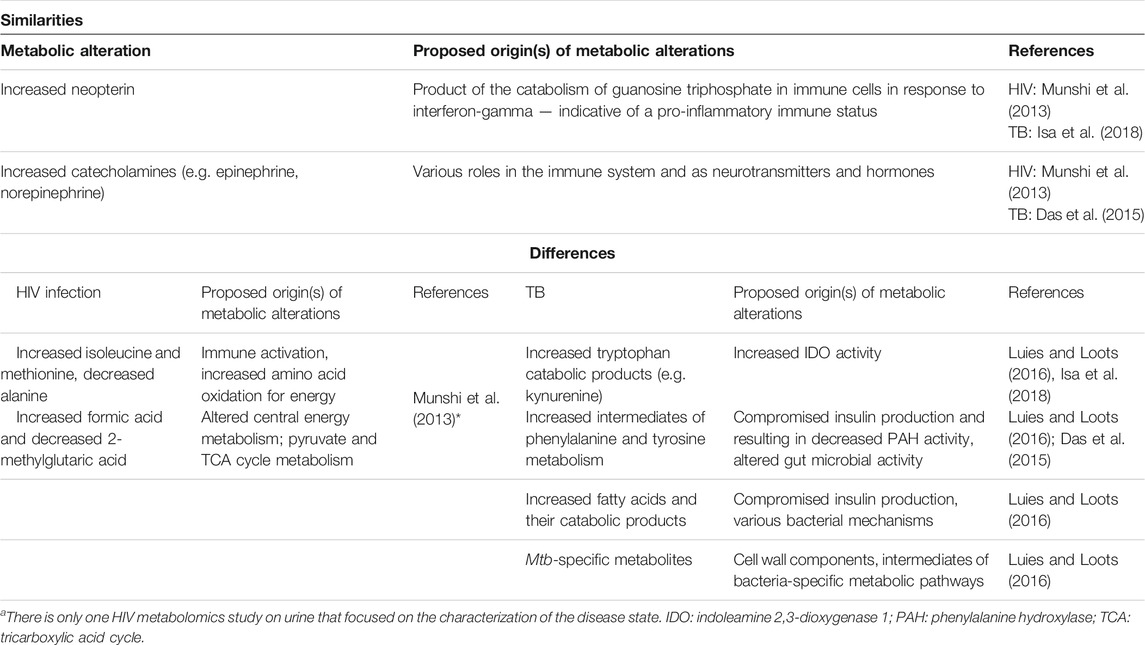
TABLE 4. A comparison of the findings of HIV infection and TB urinary metabolomics investigationsa.
Comparing Human Immunodeficiency Virus Infection and Tuberculosis Metabolic Profiles
To date, metabolomics has been used to investigate various aspects of HIV infection and TB, using various sample matrices, which can be used as a guide in terms of what to expect during co-infection. HIV infection metabolomics studies have often been concerned with characterizing the disease state, prognosis, and the effect of ART, using blood-based (serum or plasma), cerebrospinal fluid (CSF), cell culture, urine, bronchoalveolar lavage fluid (BALF), oral wash/saliva, and tissue samples (Williams et al., 2017). Within the TB metabolomics literature, blood-based, urine, sputum, tissue, pleural effusion, and cell culture samples have been used, with a focus on biomarker discovery for diagnosis and treatment outcome. As Mtb has its own metabolism, unlike HIV, bacterial culture metabolomics has also been extensively used to characterize the mycobacterium’s metabolic adaptations (Du Preez et al., 2019).
The normal course of the interactions between the metabolic, immune and endocrine systems is distorted due to the chronic nature of immune activation and inflammation during HIV and Mtb infection. At the time of diagnosis, when treatment-naïve samples are typically collected, overlapping biochemical processes have already been set in motion and become so interconnected that no single measure of causality can be attributed. This makes it difficult to interpret metabolomics data. Despite this, and although the trends are not always consistent, an overlapping pattern of metabolites frequently altered during HIV infection and TB can be identified and linked to known effects of these infections. Tables 2–4 compare the serum, plasma and urine metabolomics profiles of untreated HIV-positive and TB patients (where these patients were compared to healthy controls), respectively. Other sample matrices were not compared in this way as there is very little overlap in the use of matrices other than blood and urine between the two disease states. In cases where a metabolite was found to be significantly altered during HIV infection and/or TB, but where the trend was inconsistent, the metabolite is marked as “altered,” instead of “increased” or “decreased.”
Despite the potential for metabolic differences between serum and plasma (Liu et al., 2018), the blood-based metabolomics investigations reviewed here show that there is significant overlap between the metabolic alterations, as induced by HIV infection and TB. For example, alterations to tryptophan and its catabolic products, glutamine and/or glutamate, aspartate, as well as alanine are commonly reported for HIV infection and TB in both serum and plasma (see “similarities” in Tables 2 and 3). Alterations to the following metabolites are similar (being frequently cited as statistically significant and, in some cases, trending in the same direction) between HIV infection and TB but occur only in either serum or plasma: indole-3-acetic acid, phenylalanine and tyrosine, pyroglutamic acid (also called 5-oxoproline), phospholipids, fatty acids, and creatine in serum (Table 2), as well as urea cycle intermediates (other than aspartate), histidine, choline, and sarcosine in plasma (Table 3).
Amino acids are central to metabolism and the function of the immune system, especially during infection. As an infection becomes chronic, these alterations become more complex as various other areas of metabolism become impaired, and amino acids may antagonize each other’s effects (Li et al., 2007). Besides the specific functions of amino acids in the immune system, free amino acids, as well as stored amino acids released from muscle tissue, are used as a last resort to produce energy via the tricarboxylic acid cycle (TCA) cycle during extended periods of high energy expenditure, such as chronic infection. Glutaminolysis has been postulated as a mechanism by which the TCA cycle is replenished during viral infection (Plaza et al., 2016). Continual oxidation of amino acids combined with malnutrition and HIV-associated malabsorption (Grinspoon and Mulligan, 2003) further contributes to alterations in amino acid metabolism. Cachexia is associated with progressive HIV infection and TB and is characterized by the loss of muscle mass. As such, the mobilization of amino acids for energy production may contribute to the observed amino acid changes.
The interconversion of glutamine and glutamate (Tables 2 and 3) is important in metabolic reactions essential to cellular function (Newsholme et al., 2003). Although alterations to both these metabolic partners are not always statistically significant (likely due to the untargeted nature of studies), one or both of these metabolites are a common feature of HIV infection (Munshi et al., 2013; Sitole et al., 2019; Tarancon-Diez et al., 2019) and TB metabolic profiles (Zhou et al., 2013; Vrieling et al., 2018; Vrieling et al., 2019; Cho et al., 2020). Where these partners are found together, increased levels of glutamate with a concurrent decrease in glutamine results in a decreased glutamine/glutamate ratio (Cho et al., 2020; Ding et al., 2020). The alterations to glutamine and glutamate have been attributed to nitrogen balance, redox homeostasis and survival mechanisms of Mtb (Frediani et al., 2014; Cho et al., 2020; Thandi et al., 2020). It has also been associated with immune activation, stress on the host, and has been indicated as an early metabolic alteration during HIV (Tarancon-Diez et al., 2019) and Mtb (Albors-Vaquer et al., 2020) infection.
The balance of glutamine and glutamate, as well as asparagine and aspartate, have various roles in the immune system. An imbalance in these ratios affects the immune-competence of T cells and macrophages (Yoneda et al., 2009). Increased serum and plasma aspartate was reported for HIV (Munshi et al., 2013; Scarpellini et al., 2016; Sitole et al., 2019). An increased aspartate/asparagine ratio was also observed in the serum (Table 2) and plasma (Table 3) of TB patients (Cho et al., 2020; Ding et al., 2020), confirming the finding of increased aspartate by Weiner et al. (2012).
Aspartate forms part of the urea cycle, where it is combined with citrulline to form argininosuccinate by argininosuccinate synthase. In the liver mitochondria, aspartate is also produced from oxaloacetate by aspartate aminotransferase, with the concurrent conversion of glutamate to α-ketoglutarate (Watford, 2003). This highlights the interconnected nature of the observed alterations to glutamine/glutamate and aspartate in HIV infection and TB metabolomics. Since part of the urea cycle occurs in mitochondria, these alterations relate to mitochondrial dysfunction that has previously been associated with HIV infection (Pinti et al., 2010) and TB (Luies et al., 2017; Ramond et al., 2019). Alterations to the urea cycle were only reported for TB in serum (Table 2) but not for HIV infection. Similar urea cycle alterations were reported for both HIV infection and TB in plasma (Table 3). Decreased urea (Weiner et al., 2012), ornithine (Vrieling et al., 2019), and citrulline, with concurrently increased aspartate (Weiner et al., 2012; Vrieling et al., 2019; Ding et al., 2020) are indicative of urea cycle dysregulation in TB patients. Although the exact mechanisms underlying these alterations are still unclear, they may be related to antimicrobial mechanisms of immune cells. For example, Thandi et al. (2020) showed that ornithine inhibits Mtb growth and enhances autophagy in mouse alveolar macrophages. Reduced levels of ornithine and putrescine suggest a decreased urea cycle and reduced ammonia clearance in HIV-positive individuals (Peltenburg et al., 2018).
Alterations to plasma phenylalanine are more commonly reported for TB than for HIV infection [see “similarities” (Table 2) and “differences” (Table 3)], with only one HIV serum investigation reporting on this metabolite (Table 2). These changes have been postulated to be related to decreased activity of phenylalanine hydroxylase, which converts phenylalanine to tyrosine (Ghannoum et al., 2013), as a consequence of compromised insulin secretion (Luies and Loots, 2016). Inflammatory diseases (such as TB) are often associated with insulin resistance, hence diabetes mellitus (DM) is a known risk factor for TB. Evidence for insulin resistance has also been found in TB patients without a clinical diagnosis of DM, indicating that the condition is caused by the diseased state (Philips et al., 2017). Two recent metabolomics studies by Vrieling et al. (2018); Vrieling et al. (2019) characterized the metabolic alterations underlying TB and DM, as well as how these change when DM presents as a comorbidity in TB patients. These studies confirmed previous reports of increased serum (Weiner et al., 2012; Zhou et al., 2013) and urinary (Luies and Loots, 2016) phenylalanine levels in TB patients. One serum-based study (Che et al., 2013), however, reported decreased phenylalanine. Altered energy regulation through the endocrine system may also contribute to the other metabolic alterations discussed here, as various enzymes are influenced by insulin (Dashty, 2013). Furthermore, mitochondrial metabolism, which is modulated by HIV and Mtb, is central to coupling amino acids (such as glutamine, glutamate, and alanine) to insulin secretion (Newsholme et al., 2007).
During gluconeogenesis, non-carbohydrate molecules are converted to glucose, an essential energy substrate for leukocytes (Newsholme and Newsholme, 1989) during times of decreased energy availability. In the liver, the glucose-alanine cycle functions as a gluconeogenesis pathway, using alanine as a substrate. The amino group of alanine is transaminated to a keto acid, and the resulting carbon skeleton used in gluconeogenesis. This process contributes to the decreased levels of alanine (see “similarities” in Tables 2 and 3) that have been reported in both HIV infection (Peltenburg et al., 2018; Sitole et al., 2019) and TB (Zhou et al., 2013; Vrieling et al., 2018; Vrieling et al., 2019). The decrease in alanine relates to cachexia in HIV-positive and TB patients, as a study that included lung cancer patients related aberrations in glucose and alanine metabolism to the degree of weight loss, rather than the presence of cancer (Leij-Halfwerk et al., 2000). In other tissues, the carbon skeletons of amino acids (except for leucine and lysine) are also used for gluconeogenesis. Such oxidation of amino acids results in the need for increased ammonia secretion through the urea cycle (Dashty, 2013), previously mentioned to be dysfunctional (due to mitochondrial dysfunction or other mechanisms), as per the urea cycle alterations discussed above.
Histidine is associated with anti-inflammatory and anti-oxidative processes (Li et al., 2018), mainly through the actions of or effects on cytokines (Son et al., 2005; Hasegawa et al., 2012). As such, decreased levels of this essential amino acid have been associated with inflammatory diseases (Hisamatsu et al., 2012; Yu et al., 2015) including HIV infection (Li et al., 2018) and TB (Weiner et al., 2012; Vrieling et al., 2018; Vrieling et al., 2019) (see “similarities” in Table 3). The levels of histidine were found to be significantly correlated with CD4 T cell count, which implies a role for this amino acid in CD4 T cell regeneration (Li et al., 2018).
A metabolite directly associated with OS, which was common amongst HIV infection (Williams et al., 2011) and TB (Weiner et al., 2012; Che et al., 2013) metabolomics studies, was pyroglutamic acid (also referred to as 5-oxoproline) (see “similarities” in Table 2). The gamma-glutamyl cycle (γ-GC) forms an integral part of glutathione recycling. Gamma-glutamyl dipeptides, by-products of glutathione synthesis, have been reported to be lower in the serum of TB patients compared to healthy controls (Weiner et al., 2012; Vrieling et al., 2019). Similarly, 5-oxoproline, a metabolite directly downstream from the gamma-glutamyl amino acid in the γ-GC, shows the same trend (Che et al., 2013; Combrink et al., 2019) and correlates with pathological lung damage (Che et al., 2013). The imbalance of the γ-GC during active TB suggests decreased antioxidant capacity because of the prolonged OS. The alterations observed for other amino acids in HIV infection, such as glutamate and aspartate, have also been associated with OS (Sitole et al., 2019). In serum, decreased methionine and increased oxidative products of this amino acid (sulfoxymethionine) have been reported for TB (Suzuki et al., 2016; Ding et al., 2020), but not HIV infection (“differences,” Table 2). Decreased methionine has been reported for HIV infection in plasma (Peltenburg et al., 2018) but this is an inconsistent finding. Based on previous reports (using conventional techniques), OS-associated alterations are worse during the co-infected state (Madebo et al., 2003; Awodele et al., 2012). However, the mechanisms that give rise to these alterations may interact in more complex ways than that which would result in simple compounding of effects.
Immune cells remodel energetically and structurally upon immune activation to accomplish the appropriate differentiation. This results in metabolic changes associated with increased energetic and biosynthetic demands as the immune system responds to the increased viral load (Plaza et al., 2016). Mitochondrial dysfunction affects multiple systems (Nunnari and Suomalainen, 2012) contributing to the loss of lipid homeostasis, as this organelle plays key roles in the biosynthesis of phospholipids for membranes, as well as the catabolism of fatty acids (Tilokani et al., 2018). Immune cell function is thus impacted, as phospholipids are essential in the structural remodeling [especially glycerophospholipids and phosphatidylcholines (Sonnino et al., 2018)], differentiation, and cell signaling of immune cells, all of which are crucial to mounting an effective immune response (O'Donnell et al., 2018). As such, it is not surprising that alterations to phospholipids are a common finding in HIV infection and TB metabolic profiles (“similarities,” Table 2). Increased cholesterol esters and decreased phospholipids in TB patients have also been associated with the utilization of host lipids by Mtb as a carbon source and in various immune evasion mechanisms. Furthermore, the combination of two phosphatidylcholines, a cholesterol ester, and sphingomyelin, was able to distinguish TB patients from those with other lung conditions (Han et al., 2020).
Phosphatidylcholines (phospholipids, Table 2) have also been implicated as markers of a more progressive/worsened outcome in HIV infection and TB. In a cohort of HIV-positive individuals on ART, a decrease in phosphatidylcholines predicted poor immunological recovery (Rosado-Sanchez et al., 2019). Interestingly, these lipids were restored after 1 year of ART (Peltenburg et al., 2018). Huang et al. (2020) searched for serum metabolic biomarkers of MDR-TB, as alternative diagnostic options in future. Decreased NADH metabolites, phosphatidylcholines, caprylic acid, and increased D-xylulose in serum distinguished MDR-TB patients from drug-susceptible TB patients and healthy controls. The authors speculated that these alterations may represent mechanisms of drug resistance, especially relating to cell membrane remodeling in resistant strains of Mtb.
Alterations to fatty acids were common between HIV infection and TB in serum (see “similarities” in Table 2). Viral infections are associated with fatty acid synthesis (Plaza et al., 2016), which, in addition to various other factors such as mitochondrial dysfunction, contribute to a profile of increased unsaturated triglycerides and decreased sphingomyelin species, as observed by Peltenburg et al. (2018). Alterations to acylcarnitine metabolism, which is linked to fatty acid metabolism, during HIV infection are suggestive of mitochondrial dysfunction (Li et al., 2018). The importance of fatty acids as indicators of TB is exemplified by Che et al. (2018) and Cao et al. (2021). These studies showed that increased levels of tryptophan catabolic products, decreased fatty acids, as well as increased acylcarnitines and bile acids could distinguish TB pleural effusion (TPE) from pleural effusion caused by lung cancer (malignant pleural effusion; MPE), a condition which is also characterized by inflammation and altered energy metabolism. A combination of four acylcarnitines and two fatty acids discriminated TPE from MPE with the highest sensitivity and specificity (Cao et al., 2021). Fatty acids have already been proven to be important in the context of HIV/TB co-infection, as polyunsaturated fatty acids, glycerophospholipids, and sphingomyelins distinguished individuals who developed IRIS from those who did not (Silva et al., 2019).
The metabolism of choline, sarcosine and creatinine is closely linked, as all three these metabolites are intermediates in pathways related to glycine metabolism. While the plasma levels of choline were increased during HIV infection (Munshi et al., 2013), they were lower for TB patients and even more so in TB patients with DM (Vrieling et al., 2019) (see “similarities” in Table 3). Choline is a major component of phospholipids, and as such alterations in the levels of this metabolite is associated with membrane turnover (Munshi et al., 2013), which is high during immune activation. Furthermore, choline is also a precursor for acetylcholine, a neurotransmitter, and has been suggested as a biomarker for the AIDS dementia complex (Munshi et al., 2013). A gut microbe-related choline metabolite has also been related to carotid atherosclerosis progression in HIV-positive individuals (Shan et al., 2018). Decreased levels of choline have been associated with non-alcoholic fatty liver disease due to decreased intake of choline or gut microbe dysbiosis (Leermakers et al., 2015; Sharpton et al., 2019). This trend had not been reported in TB patients before the study of Vrieling et al. (2019), who advised caution in the interpretation of this finding. Sarcosine is a downstream metabolite of choline and is converted to glycine. It is an important intermediate in one-carbon metabolism, which is involved in many physiological processes, including redox defense (notably through glutathione). Increased levels of sarcosine (see “similarities” in Table 3) have been associated with folate deficiencies and muscle wasting during HIV infection (Munshi et al., 2013), but this metabolic alteration has not been interpreted in the context of TB. However, as sarcosine is a product of muscle degradation and cachexia is a characteristic feature of TB (Semba et al., 2010), there may be a similar mechanism driving the increased levels of sarcosine. Glycine and arginine are precursors for creatine. The majority of all creatine in the body is in muscle, where it serves as the primary fuel to maintain ATP availability for cellular metabolism, especially during periods of high energy demand (Kreider and Stout, 2021). As such, the observed serum creatine in HIV infection (Sitole et al., 2019) and TB (Weiner et al., 2018) relates to the prolonged period of high energy expenditure and cachexia associated with these diseases (see “similarities” in Table 2). Another contributor to this alteration may be mitochondrial dysfunction, as creatine crosses the mitochondrial membrane as part of the creatine phosphate shuttle, which facilitates the replenishment of ATP (Kreider and Stout, 2021).
Considering the comparisons (Tables 2 and 3), the HIV/TB co-infected metabolic profile obtained from blood is likely to be characterized primarily by exacerbated amino acid alterations (especially the essential amino acids and those non-essential amino acids involved in immune function and redox balance such as glutamate and glutamine). There may also be lipid alterations (deduced from similar metabolic changes highlighted in Tables 2 and 3). Figure 1 represents the overlapping metabolic alterations and maps these changes and their interpretations, which have been identified by HIV infection and TB metabolomics, separately, onto what is known about host metabolism during the co-infected state (dark purple arrows). It shows that HIV infection and TB (to the left and right of the Venn diagram, respectively) is associated with constant immune activation and inflammation as well as alterations to the gut microbiome (metabolites 1 and 2), which increases the host energy expenditure as immune cells differentiate (reflected by metabolites 3–14) and pathogens carry out biosynthetic processes (metabolites 3 and 10). This adds to the oxidative profile (metabolites 2–4, 6, 7 and 14), impacting mitochondrial structure and function (metabolites 5, 9, 11 and 14). Part of the urea cycle occurs in mitochondria, and since this organelle’s structure and function is impacted, there is reduced clearance of ammonia, which translates to urea cycle alterations. In this chronic state of immune activation and inflammation, the individual experiences malaise and anorexia, leaving a malnourished individual who eventually suffers from cachexia (metabolites 7, 13 and 14). Dysfunctional mitochondria impact lipid metabolism and is reflected as changes in phospholipids, choline, sarcosine and fatty acids—all with interrelated and sometimes compounding effects on the immune system, inflammation and biosynthetic processes. Given that these changes occur during HIV infection and TB respectively, it is highly likely that they will be detected in co-infected samples (represented by the overlapping Venn diagram) and that more quantitative versus qualitative differences will reflect.
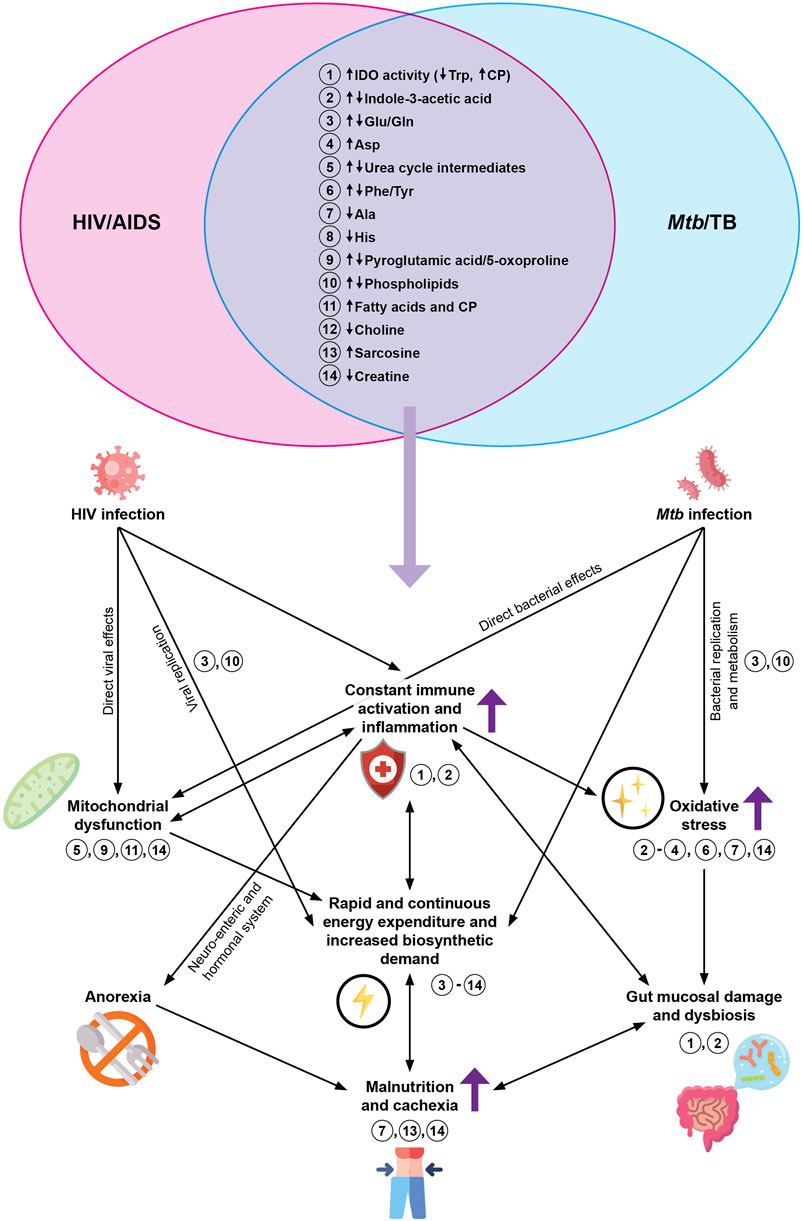
FIGURE 1. The proposed metabolic effects of HIV/TB co-infection, based on the overlapping findings of prior blood-based HIV infection and TB metabolomics studies (as indicated by the Venn diagram at the top). The dark purple arrows represent effects that are known to be exacerbated during HIV/TB co-infection. The light purple arrow represents metabolites hypothesized to be altered during HIV/TB co-infection, based on published literature. The numbers indicate the overlapping metabolites and where they play a role in what is known about host metabolism during HIV/TB co-infection. Abbreviations: HIV, human immunodeficiency virus; AIDS, acquired immunodeficiency syndrome; Mtb, Mycobacterium tuberculosis; TB, tuberculosis; IDO, indoleamine-2,3-dioxygenase; Trp, tryptophan; CP, catabolic products; Glu, glutamate; Gln, glutamine; Phe, phenylalanine; Tyr, tyrosine; Asp, aspartate; Ala, alanine; His, histidine.
Although the lipids did not overlap between the studies compared, the classes affected were similar, and their numerous roles in HIV infection, TB, and the immune response are already established. The exacerbated immune dysfunction during co-infection (Sharan et al., 2020) is likely to be reflected in the metabolic profile in terms of the overlapping HIV infection and TB metabolites (Figure 1 and “similarities” in Tables 2–4). Furthermore, those altered metabolites which have to date only been associated with either HIV infection or TB may also be significant during co-infection (“differences” in Tables 2–4) and metabolites that have not previously been associated with HIV infection or TB may also be significant in the co-infection metabolic profile.
Features unique to TB pathology have also been reported, such as fibrinopeptides, proposed to originate from the granuloma (Weiner et al., 2012) (see “differences,” Table 2). Within plasma, alterations to the branched-chain amino acids were more commonly reported for HIV infection than for TB, although alterations to these amino acids are commonly attributed to immune activation and wasting, which are also features of TB (see “differences,” Table 3). Given the limited number of metabolomics studies that focused on HIV/TB co-infection to date, the metabolic mechanisms underlying HIV/TB co-infection remains incompletely characterized. The comparison made here yields insight into how the metabolic changes during HIV infection and TB may interact during the co-infected state and could serve to inform future study design. Compared to blood, urine is used less frequently for characterization or diagnostic purposes of either HIV infection or TB, although it has been popular for TB drug metabolism elucidation (see Du Preez et al., 2019). As such, the extensive comparison made for blood could not be made for urine (and other sample matrices), and hence a large piece of the puzzle is still missing.
Given the advantages of urine as a clinical specimen (Du Preez et al., 2019), it has been considered an excellent alternative to sputum for TB diagnostics. Characterizing the urine metabolic profile of HIV-positive individuals will be a necessary step if urine were to be developed as a robust TB diagnostic matrix. The HIV infection and TB urine metabolic profiles overlap to a smaller degree than the blood-based profiles. While the urinary metabolic profile of HIV-positive patients indicates altered energy metabolism and the utilization of amino acids for energy, the urinary TB metabolic profile indicates increased inflammation and compromised insulin production in addition to reflecting Mtb-related alterations (see “differences,” Table 4). This relatively smaller degree of overlap may be due to the limited number of studies eligible for the comparison (in which participants were untreated for either condition; see Table 4). Nevertheless, increased levels of neopterin (which has been detected using conventional assays for both diseases) and catecholamines were points of similarity in the urine metabolic profiles of HIV infection and TB (Table 4).
The study of Munshi et al. (2013) provides unique insights into HIV infection since it relied on the use of various sample types, namely plasma, urine, and saliva. The only other urinary HIV-related metabolomics study is that of Chetwynd et al. (2017), which does not concur with Munshi et al. (2013) on all results. For instance, the authors’ observations regarding the extent to which immunosuppression is reflected in urine differs. Chetwynd et al. (2017) found that no separation could be achieved within the HIV-positive treatment-naïve group upon stratification by CD4 T cell count (point of division at 250 cells/mm3), which led them to suggest that metabolic markers of immunosuppression are not visible in urine. However, conventional methodologies established that urinary neopterin has an inverse correlation with CD4 T cell count (Immanuel et al., 2005). Increased levels of urinary neopterin have been recognized as a marker of immune activation, a trend which has been observed in urinary HIV infection (Munshi et al., 2013) and TB metabolomics studies, as well as in a small group of co-infected patients included in the study of Isa et al. (2018), in which the elevation was more pronounced. These results confirm previous findings [reviewed by Siawaya et al. (2007)] and suggest that urinary neopterin may be useful as part of the HIV/TB co-infection biosignature. There have been observations where urinary neopterin decreased in response to ART (Munshi et al., 2013) as well as in serum upon anti-TB treatment. This suggests the additional usefulness of neopterin as a prognostic marker. In co-infected patients, consistently high neopterin levels have been associated with HIV disease progression and poor outcomes (Immanuel et al., 2005). As co-infection metabolic profiles have not yet been systematically studied, this remains a viable avenue for future investigation.
There are a few studies not relevant to the comparisons in Tables 2–4, but which are important to mention in the context of the co-infected state. Alterations to the human microbiome have been associated with both HIV infection and TB, but it remains unclear exactly how the microbiome is affected during co-infection. The composition of the microbiome of the upper respiratory tract of HIV-positive treatment-naïve individuals was similar to those of HIV/TB co-infected patients except for a lower abundance of species belonging to the Veillonellaceae family of bacteria. This family was also less abundant in the treatment-naïve TB group relative to the treated TB group. This suggests such an alteration to the microbiome of HIV-positive patients to be indicative of TB, but this requires further investigation (Htun et al., 2018).
Two metabolomics studies focused on lung health in HIV-positive individuals, which provides the opportunity to evaluate the state of the lungs before Mtb infection in HIV-positive individuals from a metabolomics perspective. Cribbs et al. (2014) studied the BALF metabolic profiles of HIV-positive individuals compared to healthy controls and found that the primary differences reflected in metabolites have their origin in the lung microbiota, and could further indicate a subclinical infection in the HIV-positive lung. This study established a specific metabolic profile of the HIV-positive lung environment, which gives an indication of lung immune function and could contribute to improving diagnostic and prognostic techniques. In another study, HIV-positive individuals could not be distinguished from healthy controls based on lung microbial population composition (determined by 16S rRNA gene sequencing); however, a distinction could be made based on the BALF metabolic profile (Cribbs et al., 2016). These studies indicate that the interaction of the host and the microbiota contribute to a functional difference in the HIV-positive lung, rather than the species present. Given that HIV-induced metabolic alterations have their origin in immune dysfunction, it is the most likely cause for the alterations observed by the authors. Such an altered environment may change the way that the HIV-positive host interacts with Mtb, and vice versa, contributing to the altered clinical course and phenotype of co-infected individuals.
Metabolomics as a Tool to Investigate Human Immunodeficiency Virus/Tuberculosis Co-Infection
There are several aspects in the HIV/TB co-infection field that remain unanswered. These gaps were reinforced/emphasized in our analysis of the HIV infection and TB metabolomics literature on informing potential changes during co-infection. The overlap in metabolites from the respective HIV infection and TB studies which confirm what is already known about co-infection indicates the potential use of metabolomics in further characterizing the co-infected state.
The diagnostic problems experienced in HIV/TB co-infected individuals are related to the diagnosis of TB rather than the diagnosis of HIV infection, as mentioned above, due to the limitations of sputum smear and culture-based tests [reviewed by Schutz et al. (2010); Tiberi et al. (2017)]. Co-infected individuals are also often unable to provide a sufficient sputum sample for diagnostic testing, especially when severely immunocompromised (World Health Organization, 2015). Molecular tests for TB diagnosis (GeneXpert MTB/RIF assay and the improved version, GeneXpert MTB/RIF Ultra) are highly effective in diagnosing TB in HIV-negative individuals, has a better turn-around time, and can give an early indication of drug resistance. However, access to such tests in high burden areas is limited by cost and there are concerns about the specificity (false-positives) of this test in HIV-negative individuals (World Health Organization, 2019). Similarly, a urinary point-of-care diagnostic assay based on the detection of an Mtb cell wall component, lipoarabinomannan (LAM), has not found widespread application partly due to its inapplicability to HIV-negative samples. It is recommended that this test not be used for screening or diagnosis in HIV-negative individuals nor HIV-positive individuals with relatively high CD4 T cell counts (World Health Organization, 2015). Given the low sensitivity of this test, an improved version (Fujifilm SILVAMP TB LAM) has been developed which retained specificity with improved diagnostic sensitivity (Broger et al., 2019). Despite high efficacy when this test is applied to immunocompromised individuals, a difficulty remains in assigning diagnostic categories [such as definite TB, possibly TB, or not TB, as used by Broger et al. (2019)] based on the results of various indicators of TB. For example, several patients could not be diagnostically classified, but tested positive using Fuji-LAM. Consequently, these patients were not started on anti-TB drugs and died within 3 months of follow-up (Broger et al., 2019).
Considering the sputum diagnostic limitations, the focus for the development of new TB diagnostic techniques should be on the identification of non-sputum biomarkers. Metabolomics can aid in identifying markers with diagnostic application or be combined with existing tests to increase sensitivity and specificity. Urine is an ideal sample in this sense as the procedures for collection and storage are simple and require minimal biosafety considerations (World Health Organization, 2015). This makes identifying markers to be used in conjunction with the LAM assay an attractive avenue of research to improve the early detection of TB and timely intervention in HIV-positive individuals. However, the criteria by which such a marker is selected must be carefully considered, particularly amenability to accurate and sensitive detection by an inexpensive point-of-care platform (Walzl et al., 2018) and specificity to TB (as opposed to a general inflammatory marker). One must also consider the increased incidence of extrapulmonary TB in this regard. Often, extrapulmonary TB is not included in the TB diagnostic testing procedure, and even if it was, sample collection for diagnosis remains difficult (Denkinger et al., 2014). If biomarkers specific to extrapulmonary disease are identified, including these in the standard TB diagnostic repertoire may significantly improve the early diagnosis of TB in HIV-positive individuals.
Metabolomics has been applied to identify metabolic determinants that indicate conversion of latent Mtb infection to clinical disease in the studies of Weiner et al. (2018) and Albors-Vaquer et al. (2020). The efficacy of such a profile has not yet been tested in HIV-positive individuals, for whom the risk of conversion to clinical disease is much greater than for HIV-negative individuals. Untreated profiles aid in understanding the underlying mechanisms that drive conversion of latent Mtb infection to active TB in HIV-positive individuals and provide early prognostic information, especially regarding TB-IRIS and treatment response. However, this might prove difficult considering sample availability, given the recommendation for early treatment of latent Mtb infection (World Health Organization, 2016a) as well as the “Test and Treat” policy for HIV infection, recommended by the World Health Organization (World Health Organization, 2016b). Nonetheless, such basic understanding is necessary for the development of robust and sustainable diagnostic and prognostic procedures.
Even if a highly effective diagnostic technique for TB in HIV-positive persons is developed, its success will depend on the integration of HIV infection and TB services on the ground, especially in high-burden areas, which are unfortunately also most often resource-limited. Once the difficult diagnosis is made, various factors complicating the management of HIV/TB co-infection come into play. The management of HIV/TB co-infection is challenging and is usually associated with unfavorable treatment outcomes due to various factors, reviewed by Tiberi et al. (2017) and Letang et al. (2020). Pharmacometabolomics is a branch of metabolomics wherein the effects of a drug or combination of drugs underlie the observed metabolic alterations (Combrink et al., 2019) and have been applied to HIV infection (Chetwynd et al., 2017; Li et al., 2018; Babu et al., 2019) and TB (reviewed by Du Preez and Loots. (2018), see Table 3). The interactions between ART and anti-TB drugs, and their interactions with enzymes, have been characterized by high-performance LC-MS (Wenning et al., 2009) and LC-MS/MS (Varshneya et al., 2019), demonstrating how metabolomics aids the understanding of in vivo drug metabolism and efficacy, which is essential to the development of better treatment strategies for co-infected patients.
Besides the interactions of the drugs themselves, variations on an individual level, identified as one of the factors contributing to TB treatment failure (De Villiers and Loots, 2013) will also affect the efficacy of drug regimens. The rate of recurrent TB (relapse and reinfection) is greater among HIV-positive compared to HIV-negative individuals (Sonnenberg et al., 2001; Charalambous et al., 2008). The only way to prevent reinfection would be an effective vaccine. Also, by better understanding the mechanisms underlying treatment failure in co-infected patients, the cases of relapse will be minimized by optimizing treatment regimens. Metabolomics has been used to identify the host factors that contribute to TB treatment failure in HIV-negative TB patients (Luies et al., 2017). Such an approach could provide valuable insights, as co-infection is associated with poor treatment outcomes and susceptibility to infection in general, due to the additional immunosuppressive effects of HIV (Guerra-Assuncao et al., 2015). Another aspect of interest from a metabolomics perspective is how the effect of the second Mtb co-infection event on the HIV-positive host would compare to that of the first.
Given that much of the HIV and Mtb infection-induced metabolic changes are driven by immune activation, inflammation and OS with subsequent impact on the mitochondria and the gut (Figure 1), metabolomics could aid in elucidating the effect that Mtb has on the gut mucosal damage as induced by HIV. The metabolic markers obtained are not unique and have been reported in the metabolomics investigations of other inflammatory conditions (Leermakers et al., 2015; Pett et al., 2017). As such, more longitudinal studies, especially in the treated context, with the inclusion of more disease controls to get more specific markers associated with each condition are thus needed.
Concluding Remarks
For the rational design of novel or improved diagnostic, treatment, and monitoring techniques, the research must first focus on better characterizing a disease state so that the origin of identified markers is understood, as opposed to unqualifiedly advocating statistically significant markers that have no true specificity for the disease state. It is of extreme importance to develop and validate highly specific biomarkers for HIV/TB co-infection—although biosignatures may be more practical considering the overlapping pathologies of HIV infection and TB. Furthermore, when developing these metabolic signatures, it should be kept in mind that the ultimate goal is point-of-care, individualized assessments that should be accessible even in resource-limited settings, as these are commonly the areas of high incidence and spread. This further requires the complete integration of HIV infection and TB services and for this, a greater awareness of co-infection should be brought among clinicians and built into infrastructure to ensure that even those with minimal education could apply it.
Author Contributions
All authors listed have made a substantial, direct, and intellectual contribution to the work and approved it for publication.
Funding
This work is based wholly/in part on the research supported by the National Research Foundation of South Africa (Grant nos: 129871 and 122116).
Conflict of Interest
The authors declare that the research was conducted in the absence of any commercial or financial relationships that could be construed as a potential conflict of interest.
Publisher’s Note
All claims expressed in this article are solely those of the authors and do not necessarily represent those of their affiliated organizations, or those of the publisher, the editors and the reviewers. Any product that may be evaluated in this article, or claim that may be made by its manufacturer, is not guaranteed or endorsed by the publisher.
References
Adu-Gyamfi, C. G., Snyman, T., Hoffmann, C. J., Martinson, N. A., Chaisson, R. E., George, J. A., et al. (2017). Plasma Indoleamine 2, 3-dioxygenase, a Biomarker for Tuberculosis in Human Immunodeficiency Virus-Infected Patients. Clin. Infect. Dis. 65 (8), 1356–1363. doi:10.1093/cid/cix550
Albors-Vaquer, A., Rizvi, A., Matzapetakis, M., Lamosa, P., Coelho, A. V., Patel, A. B., et al. (2020). Active and Prospective Latent Tuberculosis Are Associated with Different Metabolomic Profiles: Clinical Potential for the Identification of Rapid and Non-invasive Biomarkers. Emerging Microbes & Infections 9 (1), 1131–1139. doi:10.1080/22221751.2020.1760734
Almeida, A. S., Lago, P. M., Boechat, N., Huard, R. C., Lazzarini, L. C. O., Santos, A. R., et al. (2009). Tuberculosis Is Associated with a Down-Modulatory Lung Immune Response that Impairs Th1-type Immunity. J. Immunol. 183 (1), 718–731. doi:10.4049/jimmunol.0801212
Aounallah, M., Dagenais-Lussier, X., El-Far, M., Mehraj, V., Jenabian, M.-A., Routy, J.-P., et al. (2016). Current Topics in HIV Pathogenesis, Part 2: Inflammation Drives a Warburg-like Effect on the Metabolism of HIV-Infected Subjects. Cytokine Growth Factor. Rev. 28, 1–10. doi:10.1016/j.cytogfr.2016.01.001
Awodele, O., Olayemi, S. O., Nwite, J. A., and Adeyemo, T. A. (2012). Investigation of the Levels of Oxidative Stress Parameters in HIV and HIV-TB Co-infected Patients. J. Infect. Dev. Ctries 6 (1), 79–85. doi:10.3855/jidc.1906
Babu, H., Sperk, M., Ambikan, A. T., Rachel, G., Viswanathan, V. K., Tripathy, S. P., et al. (2019). Plasma Metabolic Signature and Abnormalities in HIV-Infected Individuals on Long-Term Successful Antiretroviral Therapy. Metabolites 9 (10), 210. doi:10.3390/metabo9100210
Badri, M., Ehrlich, R., Wood, R., Pulerwitz, T., and Maartens, G. (2001). Association between Tuberculosis and HIV Disease Progression in a High Tuberculosis Prevalence Area. Int. J. Tuberc. Lung Dis. 5 (3), 225–232.
Ball, H. J., Fedelis, F. F., Bakmiwewa, S. M., Hunt, N. H., and Yuasa, H. J. (2014). Tryptophan-Catabolizing Enzymes — Party of Three. Front. Immunol. 5 (485). doi:10.3389/fimmu.2014.00485
Bell, L. C. K., and Noursadeghi, M. (2018). Pathogenesis of HIV-1 and Mycobacterium tuberculosis Co-infection. Nat. Rev. Microbiol. 16 (2), 80–90. doi:10.1038/nrmicro.2017.128
Brenchley, J. M., Price, D. A., Schacker, T. W., Asher, T. E., Silvestri, G., Rao, S., et al. (2006). Microbial Translocation Is a Cause of Systemic Immune Activation in Chronic HIV Infection. Nat. Med. 12 (12), 1365–1371. doi:10.1038/nm1511
Broger, T., Sossen, B., Du Toit, E., Kerkhoff, A. D., Schutz, C., Ivanova Reipold, E., et al. (2019). Novel Lipoarabinomannan point-of-care Tuberculosis Test for People with HIV: a Diagnostic Accuracy Study. Lancet Infect. Dis. 19 (8), 852–861. doi:10.1016/S1473-3099(19)30001-5
Burman, W., and Jones, B. E. (2003). Clinical and Radiographic Features of HIV-Related Tuberculosis1. Semin. Respir. infections 18 (4), 263–271. doi:10.1053/S0882-0546(03)00072-0
Cao, G., Song, Z., Yang, Z., Chen, Z., Hong, Y., and Cai, Z. (2021). Database-assisted Global Metabolomics Profiling of Pleural Effusion Induced by Tuberculosis and Malignancy. Chin. Chem. Lett. doi:10.1016/j.cclet.2021.03.052
Chaisson, R. E., Schecter, G. F., Theuer, C. P., Rutherford, G. W., Echenberg, D. F., and Hopewell, P. C. (1987). Tuberculosis in Patients with the Acquired Immunodeficiency Syndrome: Clinical Features, Response to Therapy, and Survival. Am. Rev. Respir. Dis. 136 (3), 570–574. doi:10.1164/ajrccm/136.3.570
Chang, H. R., Dulloo, A. G., and Bistrian, B. R. (1998). Role of Cytokines in AIDS Wasting. Nutrition 14 (11-12), 853–863. doi:10.1016/s0899-9007(98)00108-7
Charalambous, S., Grant, A. D., Moloi, V., Warren, R., Day, J. H., van Helden, P., et al. (2008). Contribution of Reinfection to Recurrent Tuberculosis in South African Gold Miners. Int. J. Tuberc. Lung Dis. 12 (8), 942–948.
Che, N., Cheng, J., Li, H., Zhang, Z., Zhang, X., Ding, Z., et al. (2013). Decreased Serum 5-oxoproline in TB Patients Is Associated with Pathological Damage of the Lung. Clinica Chim. Acta 423, 5–9. doi:10.1016/j.cca.2013.04.010
Che, N., Ma, Y., Ruan, H., Xu, L., Wang, X., Yang, X., et al. (2018). Integrated Semi-targeted Metabolomics Analysis Reveals Distinct Metabolic Dysregulation in Pleural Effusion Caused by Tuberculosis and Malignancy. Clinica Chim. Acta 477, 81–88. doi:10.1016/j.cca.2017.12.003
Cheng, S.-C., Joosten, L. A. B., and Netea, M. G. (2014). The Interplay between central Metabolism and Innate Immune Responses. Cytokine Growth Factor. Rev. 25 (6), 707–713. doi:10.1016/j.cytogfr.2014.06.008
Chetwynd, A. J., Samarawickrama, A., Vera, J. H., Bremner, S. A., Abdul-Sada, A., Gilleece, Y., et al. (2017). Nanoflow-nanospray Mass Spectrometry Metabolomics Reveals Disruption of the Urinary Metabolite Profiles of HIV-Positive Patients on Combination Antiretroviral Therapy. JAIDS J. Acquired Immune Deficiency Syndromes 74 (2), e45–e53. doi:10.1097/qai.0000000000001159
Cho, Y., Park, Y., Sim, B., Kim, J., Lee, H., Cho, S.-N., et al. (2020). Identification of Serum Biomarkers for Active Pulmonary Tuberculosis Using a Targeted Metabolomics Approach. Sci. Rep. 10 (1), 3825. doi:10.1038/s41598-020-60669-0
Collins, J. M., Siddiqa, A., Jones, D. P., Liu, K., Kempker, R. R., Nizam, A., et al. (2020). Tryptophan Catabolism Reflects Disease Activity in Human Tuberculosis. JCI Insight 5 (10). doi:10.1172/jci.insight.137131
Combrink, M., Du Preez, I., Ronacher, K., Walzl, G., and Loots, D. T. (2019). Time-dependent Changes in Urinary Metabolome before and after Intensive Phase Tuberculosis Therapy: a Pharmacometabolomics Study. OMICS: A J. Integr. Biol. 23 (11), 560–572. doi:10.1089/omi.2019.0140
Cribbs, S. K., Park, Y., Guidot, D. M., Martin, G. S., Brown, L. A., Lennox, J., et al. (2014). Metabolomics of Bronchoalveolar Lavage Differentiate Healthy HIV-1-Infected Subjects from Controls. AIDS Res. Hum. retroviruses 30 (6), 579–585. doi:10.1089/AID.2013.0198
Cribbs, S. K., Uppal, K., Li, S., Jones, D. P., Huang, L., Tipton, L., et al. (2016). Correlation of the Lung Microbiota with Metabolic Profiles in Bronchoalveolar Lavage Fluid in HIV Infection. Microbiome 4 (3). doi:10.1186/s40168-016-0147-4
Dagenais-Lussier, X., Aounallah, M., Mehraj, V., El-Far, M., Tremblay, C., Sekaly, R.-P., et al. (2016). Kynurenine Reduces Memory CD4 T-Cell Survival by Interfering with Interleukin-2 Signaling Early during HIV-1 Infection. J. Virol. 90 (17), 7967–7979. doi:10.1128/JVI.00994-16
Das, M. K., Bishwal, S. C., Das, A., Dabral, D., Badireddy, V. K., Pandit, B., et al. (2015). Deregulated Tyrosine-Phenylalanine Metabolism in Pulmonary Tuberculosis Patients. J. Proteome Res. 14 (4), 1947–1956. doi:10.1021/acs.jproteome.5b00016
Dashty, M. (2013). A Quick Look at Biochemistry: Carbohydrate Metabolism. Clin. Biochem. 46, 1339–1352. doi:10.1016/j.clinbiochem.2013.04.027
De Villiers, L., and Loots, D. T. (2013). Using Metabolomics for Elucidating the Mechanisms Related to Tuberculosis Treatment Failure. Curr. metabolomics 1 (4), 306–317.
Denkinger, C. M., Schumacher, S. G., Boehme, C. C., Dendukuri, N., Pai, M., and Steingart, K. R. (2014). Xpert MTB/RIF Assay for the Diagnosis of Extrapulmonary Tuberculosis: a Systematic Review and Meta-Analysis. Eur. Respir. J. 44 (2), 435–446. doi:10.1183/09031936.00007814
Diedrich, C. R., and Flynn, J. L. (2011). HIV-1/Mycobacterium tuberculosis Coinfection Immunology: How Does HIV-1 Exacerbate Tuberculosis? Infect. Immun. 79 (4), 1407–1417. doi:10.1128/IAI.01126-10
Ding, Y., Raterink, R.-J., Marín-Juez, R., Veneman, W. J., Egbers, K., van den Eeden, S., et al. (2020). Tuberculosis Causes Highly Conserved Metabolic Changes in Human Patients, Mycobacteria-Infected Mice and Zebrafish Larvae. Sci. Rep. 10 (1), 11635. doi:10.1038/s41598-020-68443-y
Djoba Siawaya, J. F., Ruhwald, M., Eugen-Olsen, J., and Walzl, G. (2007). Correlates for Disease Progression and Prognosis during Concurrent HIV/TB Infection. Int. J. Infect. Dis. 11 (4), 289–299. doi:10.1016/j.ijid.2007.02.001
Drain, P. K., Kupka, R., Mugusi, F., and Fawzi, W. W. (2007). Micronutrients in HIV-Positive Persons Receiving Highly Active Antiretroviral Therapy. Am. J. Clin. Nutr. 85 (2), 333–345. doi:10.1093/ajcn/85.2.333
Du Preez, I., and Loots, D. T. (2018). Novel Insights into the Pharmacometabonomics of First-Line Tuberculosis Drugs Relating to Metabolism, Mechanism of Action and Drug-Resistance. Drug Metab. Rev. 50 (4), 466–481. doi:10.1080/03602532.2018.1559184
Du Preez, I., Luies, L., and Loots, D. T. (2019). The Application of Metabolomics toward Pulmonary Tuberculosis Research. Tuberculosis 115, 126–139. doi:10.1016/j.tube.2019.03.003
Dunn, W. B., Bailey, N. J. C., and Johnson, H. E. (2005). Measuring the Metabolome: Current Analytical Technologies. Analyst 130 (5), 606–625. doi:10.1039/B418288J
Elliott, A. M., Halwiindi, B., Hayes, R. J., Luo, N., Mwinga, A. G., Tembo, G., et al. (1995). The Impact of Human Immunodeficiency Virus on Mortality of Patients Treated for Tuberculosis in a Cohort Study in Zambia. Trans. R. Soc. Trop. Med. Hyg. 89 (1), 78–82. doi:10.1016/0035-9203(95)90668-1
Forrester, J., Spiegelman, D., Woods, M., Knox, T., Fauntleroy, J., and Gorbach, S. (2001). Weight and Body Composition in a Cohort of HIV-Positive Men and Women. Public Health Nutr. 4 (3), 743–747. doi:10.1079/PHN200099
Frediani, J. K., Jones, D. P., Tukvadze, N., Uppal, K., Sanikidze, E., Kipiani, M., et al. (2014). Plasma Metabolomics in Human Pulmonary Tuberculosis Disease: a Pilot Study. PLoS One 9 (10), e108854. doi:10.1371/journal.pone.0108854
Garcia, G. F., Moura, A. S., Ferreira, C. S., and Rocha, M. O. d. C. (2007). Clinical and Radiographic Features of HIV-Related Pulmonary Tuberculosis According to the Level of Immunosuppression. Rev. Soc. Bras. Med. Trop. 40, 622–626. doi:10.1590/S0037-86822007000600004
Gautam, U. S., Foreman, T. W., Bucsan, A. N., Veatch, A. V., Alvarez, X., Adekambi, T., et al. (2018). In Vivo inhibition of Tryptophan Catabolism Reorganizes the Tuberculoma and Augments Immune-Mediated Control of Mycobacterium tuberculosis. Proc. Natl. Acad. Sci. USA 115 (1), E62–E71. doi:10.1073/pnas.1711373114
Gautron, L., and Laye, S. (2009). Neurobiology of Inflammation-Associated Anorexia. Front. Neurosci. 3 (3). doi:10.3389/neuro.23.003.2009
Ghannoum, M. A., Mukherjee, P. K., Jurevic, R. J., Retuerto, M., Brown, R. E., Sikaroodi, M., et al. (2013). Metabolomics Reveals Differential Levels of Oral Metabolites in HIV-Infected Patients: toward Novel Diagnostic Targets. OMICS: A J. Integr. Biol. 17 (1), 5–15. doi:10.1089/omi.2011.0035
González Plaza, J. J., Hulak, N., Kausova, G., Zhumadilov, Z., and Akilzhanova, A. (2016). Role of Metabolism during Viral Infections, and Crosstalk with the Innate Immune System. Irdr 5 (2), 90–96. doi:10.5582/irdr.2016.01008
Gostner, J. M., Becker, K., Kurz, K., and Fuchs, D. (2015). Disturbed Amino Acid Metabolism in HIV: Association with Neuropsychiatric Symptoms. Front. Psychiatry 6, 97. doi:10.3389/fpsyt.2015.00097
Grinspoon, S., and Mulligan, K. (2003). Weight Loss and Wasting in Patients Infected with Human Immunodeficiency Virus. Clin. Infect. Dis. 36 (36), S69–S78. doi:10.1086/367561
Guerra-Assunção, J. A., Houben, R. M. G. J., Crampin, A. C., Mzembe, T., Mallard, K., Coll, F., et al. (2015). Recurrence Due to Relapse or Reinfection WithMycobacterium Tuberculosis: A Whole-Genome Sequencing Approach in a Large, Population-Based Cohort with a High HIV Infection Prevalence and Active Follow-Up. J. Infect. Dis. 211 (7), 1154–1163. doi:10.1093/infdis/jiu574
Han, Y.-S., Chen, J.-X., Li, Z.-B., Chen, J., Yi, W.-J., Huang, H., et al. (2020). Identification of Potential Lipid Biomarkers for Active Pulmonary Tuberculosis Using Ultra-high-performance Liquid Chromatography-Tandem Mass Spectrometry. Exp. Biol. Med. (Maywood) 246 (4), 387–399. doi:10.1177/1535370220968058
Hasegawa, S., Ichiyama, T., Sonaka, I., Ohsaki, A., Okada, S., Wakiguchi, H., et al. (2012). Cysteine, Histidine and glycine Exhibit Anti-inflammatory Effects in Human Coronary Arterial Endothelial Cells. Clin. Exp. Immunol. 167 (2), 269–274. doi:10.1111/j.1365-2249.2011.04519.x
Hewer, R., Vorster, J., Steffens, F. E., and Meyer, D. (2006). Applying Biofluid 1H NMR-Based Metabonomic Techniques to Distinguish between HIV-1 Positive/AIDS Patients on Antiretroviral Treatment and HIV-1 Negative Individuals. J. Pharm. Biomed. Anal. 41 (4), 1442–1446. doi:10.1016/j.jpba.2006.03.006
Hisamatsu, T., Okamoto, S., Hashimoto, M., Muramatsu, T., Andou, A., Uo, M., et al. (2012). Novel, Objective, Multivariate Biomarkers Composed of Plasma Amino Acid Profiles for the Diagnosis and Assessment of Inflammatory Bowel Disease. PloS one 7 (1), e31131. doi:10.1371/journal.pone.0031131
Htun, K. S., Fong, Y., Kyaw, A. A., Aung, S. T., Oo, K. Z., Zaw, T., et al. (2018). Microbiome Dataset from the Upper Respiratory Tract of Patients Living with HIV, HIV/TB and TB from Myanmar. Data in Brief 21, 354–357. doi:10.1016/j.dib.2018.10.003
Hu, Y., Feng, Y., Wu, J., Liu, F., Zhang, Z., Hao, Y., et al. (2019). The Gut Microbiome Signatures Discriminate Healthy From Pulmonary Tuberculosis Patients. Front. Cel. Infect. Microbiol. 9, 90. doi:10.3389/fcimb.2019.00090
Huang, H., Han, Y.-S., Chen, J., Shi, L.-Y., Wei, L.-L., Jiang, T.-T., et al. (2020). The Novel Potential Biomarkers for Multidrug-Resistance Tuberculosis Using UPLC-Q-TOF-MS. Exp. Biol. Med. (Maywood) 245 (6), 501–511. doi:10.1177/1535370220903464
Ibarrondo, F. J., Wilson, S. B., Hultin, L. E., Shih, R., Hausner, M. A., Hultin, P. M., et al. (2013). Preferential Depletion of Gut CD4-Expressing iNKT Cells Contributes to Systemic Immune Activation in HIV-1 Infection. Mucosal Immunol. 6 (3), 591–600. doi:10.1038/mi.2012.101
Immanuel, C., Victor, L., Chelvi, K. S., Padmapriyadarsini, C., Rehman, F., Iliayas, S., et al. (2005). Serum Neopterin Levels in HIV Infected Patients with & without Tuberculosis. Indian J. Med. Res. 121 (4), 220–225.
Isa, F., Collins, S., Lee, M. H., Decome, D., Dorvil, N., Joseph, P., et al. (2018). Mass Spectrometric Identification of Urinary Biomarkers of Pulmonary Tuberculosis. EBioMedicine 31, 157–165. doi:10.1016/j.ebiom.2018.04.014
Jain, R. G., Furfine, E. S., Pedneault, L., White, A. J., and Lenhard, J. M. (2005). Metabolic Complications Associated with Antiretroviral Therapy. Antivir. Res 51 (3), 151–177. doi:10.1016/s0166-3542(01)00148-6
Jenabian, M.-A., Patel, M., Kema, I., Kanagaratham, C., Radzioch, D., Thébault, P., et al. (2013). Distinct Tryptophan Catabolism and Th17/Treg Balance in HIV Progressors and Elite Controllers. PLoS One 8 (10), e78146. doi:10.1371/journal.pone.0078146
Katona, P., and Katona‐Apte, J. (2008). The Interaction between Nutrition and Infection. Clin. Infect. Dis. 46 (10), 1582–1588. doi:10.1086/587658
Katz, J. B., Muller, A. J., and Prendergast, G. C. (2008). Indoleamine 2,3-dioxygenase in T-Cell Tolerance and Tumoral Immune Escape. Immunol. Rev. 222, 206–221. doi:10.1111/j.1600-065X.2008.00610.x
Kennedy, N., Ramsay, A., Uiso, L., Gutmann, J., Ngowi, F. I., and Gillespie, S. H. (1996). Nutritional Status and Weight Gain in Patients with Pulmonary Tuberculosis in Tanzania. Trans. R. Soc. Trop. Med. Hyg. 90 (2), 162–166. doi:10.1016/S0035-9203(96)90123-6
Koethe, J. R., and Heimburger, D. C. (2010). Nutritional Aspects of HIV-Associated Wasting in Sub-saharan Africa. Am. J. Clin. Nutr. 91 (4), 1138S–1142S. doi:10.3945/ajcn.2010.28608D
Kreider, R. B., and Stout, J. R. (2021). Creatine in Health and Disease. Nutrients 13 (2), 447. doi:10.3390/nu13020447
Kwan, C. K., and Ernst, J. D. (2011). HIV and Tuberculosis: a Deadly Human Syndemic. Clin. Microbiol. Rev. 24 (2), 351–376. doi:10.1128/cmr.00042-10
Leermakers, E. T. M., Moreira, E. M., Kiefte-de Jong, J. C., Darweesh, S. K. L., Visser, T., Voortman, T., et al. (2015). Effects of Choline on Health across the Life Course: a Systematic Review. Nutr. Rev. 73 (8), 500–522. doi:10.1093/nutrit/nuv010
Leij-Halfwerk, S., Dagnelie, P. C., van den Berg, J. W. O., Wattimena, J. D. L., Hordijk-Luijk, C. H., and Wilson, J. P. (2000). Weight Loss and Elevated Gluconeogenesis from Alanine in Lung Cancer Patients. Am. J. Clin. Nutr. 71 (2), 583–589. doi:10.1093/ajcn/71.2.583
Letang, E., Ellis, J., Naidoo, K., Casas, E. C., Sánchez, P., Hassan-Moosa, R., et al. (2020). Tuberculosis-HIV Co-infection: Progress and Challenges after Two Decades of Global Antiretroviral Treatment Roll-Out. Archivos de Bronconeumología 56 (7), 446–454. doi:10.1016/j.arbres.2019.11.015
Li, M., Kwok, M. K., Fong, S. S. M., and Schooling, C. M. (2019). Indoleamine 2,3-dioxygenase and Ischemic Heart Disease: a Mendelian Randomization Study. Sci. Rep. 9 (1), 8491. doi:10.1038/s41598-019-44819-7
Li, P., Yin, Y.-L., Li, D., Woo Kim, S., and Wu, G. (2007). Amino Acids and Immune Function. Br. J. Nutr. 98 (2), 237–252. doi:10.1017/S000711450769936X
Li, S., Sullivan, N. L., Rouphael, N., Yu, T., Banton, S., Maddur, M. S., et al. (2017). Metabolic Phenotypes of Response to Vaccination in Humans. Cell 169 (5), 862–877. e817. doi:10.1016/j.cell.2017.04.026
Li, X., Wu, T., Jiang, Y., Zhang, Z., Han, X., Geng, W., et al. (2018). Plasma Metabolic Changes in Chinese HIV-Infected Patients Receiving Lopinavir/ritonavir Based Treatment: Implications for HIV Precision Therapy. Cytokine 110, 204–212. doi:10.1016/j.cyto.2018.05.001
Liu, X., Hoene, M., Wang, X., Yin, P., Häring, H.-U., Xu, G., et al. (2018). Serum or Plasma, what Is the Difference? Investigations to Facilitate the Sample Material Selection Decision Making Process for Metabolomics Studies and beyond. Analytica Chim. Acta 1037, 293–300. doi:10.1016/j.aca.2018.03.009
Lucas, S. B., De Cock, K. M., Hounnou, A., Peacock, C., Diomande, M., Hondé, M., et al. (1994). Contribution of Tuberculosis to Slim Disease in Africa. Bmj 308 (6943), 1531–1533. doi:10.1136/bmj.308.6943.1531
Luies, L., and du Preez, I. (2020). The echo of Pulmonary Tuberculosis: Mechanisms of Clinical Symptoms and Other Disease-Induced Systemic Complications. Clin. Microbiol. Rev. 33 (4), e00036–00020. doi:10.1128/CMR.00036-20
Luies, L., and Loots, D. T. (2016). Tuberculosis Metabolomics Reveals Adaptations of Man and Microbe to Outcompete and Survive. Metabolomics 12 (40). doi:10.1007/s11306-016-0979-8
Luies, L., Mienie, J., Motshwane, C., Ronacher, K., Walzl, G., and Loots, D. T. (2017). Urinary Metabolite Markers Characterizing Tuberculosis Treatment Failure. Metabolomics 13 (10). doi:10.1007/s11306-017-1261-4
Luo, M., Liu, Y., Wu, P., Luo, D.-X., Sun, Q., Zheng, H., et al. (2017). Alternation of Gut Microbiota in Patients with Pulmonary Tuberculosis. Front. Physiol. 8, 822. doi:10.3389/fphys.2017.00822
Lyles, R. H., Tang, A. M., Smit, E., Mellors, J. W., Margolick, J. B., Visscher, B. R., et al. (1999). Virologic, Immunologic, and Immune Activation Markers as Predictors of HIV-Associated Weight Loss Prior to AIDS. Multicenter AIDS Cohort Study. J. Acquir Immune Defic Syndr. 22 (4), 386–394. doi:10.1097/00126334-199912010-00010
MacAllan, D. C., McNurlan, M. A., Kurpad, A. V., De Souza, G., Shetty, P. S., Calder, A. G., et al. (1998). Whole Body Protein Metabolism in Human Pulmonary Tuberculosis and Undernutrition: Evidence for Anabolic Block in Tuberculosis. Clin. Sci. 94 (3), 321–331. doi:10.1042/cs0940321
Madebo, T., Lindtjørn, B., Aukrust, P., and Berge, R. K. (2003). Circulating Antioxidants and Lipid Peroxidation Products in Untreated Tuberculosis Patients in Ethiopia. Am. J. Clin. Nutr. 78 (1), 117–122. doi:10.1093/ajcn/78.1.117
Manches, O., Munn, D., Fallahi, A., Lifson, J., Chaperot, L., Plumas, J., et al. (2008). HIV-activated Human Plasmacytoid DCs Induce Tregs through an Indoleamine 2,3-dioxygenase-dependent Mechanism. J. Clin. Invest. 118 (10), 3431–3439. doi:10.1172/JCI34823
Mancuso, R., Hernis, A., Agostini, S., Rovaris, M., Caputo, D., Fuchs, D., et al. (2015). Indoleamine 2,3 Dioxygenase (IDO) Expression and Activity in Relapsing- Remitting Multiple Sclerosis. PLOS ONE 10 (6), e0130715. doi:10.1371/journal.pone.0130715
Manosuthi, W., Mankatitham, W., Lueangniyomkul, A., Thongyen, S., Likanonsakul, S., Suwanvattana, P., et al. (2012). Time to Initiate Antiretroviral Therapy between 4 Weeks and 12 Weeks of Tuberculosis Treatment in HIV-Infected Patients: Results from the TIME Study. J. Acquir Immune Defic Syndr. 60 (4), 377–383. doi:10.1097/QAI.0b013e31825b5e06
Meininger, G., Hadigan, C., Laposata, M., Brown, J., Rabe, J., Louca, J., et al. (2002). Elevated Concentrations of Free Fatty Acids Are Associated with Increased Insulin Response to Standard Glucose challenge in Human Immunodeficiency Virus-Infected Subjects with Fat Redistribution. Metabolism 51 (2), 260–266. doi:10.1053/meta.2002.29999
Melchior, J. C., Salmon, D., Rigaud, D., Leport, C., Bouvet, E., Detruchis, P., et al. (1991). Resting Energy Expenditure Is Increased in Stable, Malnourished HIV-Infected Patients. Am. J. Clin. Nutr. 53 (2), 437–441. doi:10.1093/ajcn/53.2.437
Mellor, A. L., Keskin, D. B., Johnson, T., Chandler, P., and Munn, D. H. (2002). Cells Expressing Indoleamine 2,3-dioxygenase Inhibit T Cell Responses. J. Immunol. 168 (8), 3771–3776. doi:10.4049/jimmunol.168.8.3771
Merlo, L. M. F., DuHadaway, J. B., Montgomery, J. D., Peng, W.-D., Murray, P. J., Prendergast, G. C., et al. (2020). Differential Roles of IDO1 and IDO2 in T and B Cell Inflammatory Immune Responses. Front. Immunol. 11 (1861). doi:10.3389/fimmu.2020.01861
Moldawer, L. L., and Sattler, F. R. (1998). Human Immunodeficiency Virus-Associated Wasting and Mechanisms of Cachexia Associated with Inflammation. Semin. Oncol. 25, 73–81.
Munn, D. H., Shafizadeh, E., Attwood, J. T., Bondarev, I., Pashine, A., and Mellor, A. L. (1999). Inhibition of T Cell Proliferation by Macrophage Tryptophan Catabolism. J. Exp. Med. 189 (9), 1363–1372. doi:10.1084/jem.189.9.1363
Munshi, S. U., Rewari, B. B., Bhavesh, N. S., and Jameel, S. (2013). Nuclear Magnetic Resonance Based Profiling of Biofluids Reveals Metabolic Dysregulation in HIV-Infected Persons and Those on Anti-retroviral Therapy. PLoS One 8 (5), e64298. doi:10.1371/journal.pone.0064298
Newsholme, P., Bender, K., Kiely, A., and Brennan, L. (2007). Amino Acid Metabolism, Insulin Secretion and Diabetes. Biochem. Soc. Trans. 35, 1180–1186. doi:10.1042/BST0351180
Newsholme, P., and Newsholme, E. A. (1989). Rates of Utilization of Glucose, Glutamine and Oleate and Formation of End-Products by Mouse Peritoneal Macrophages in Culture. Biochem. J. 261 (1), 211–218. doi:10.1042/bj2610211
Newsholme, P., Procopio, J., Lima, M. M. R., Pithon-Curi, T. C., and Curi, R. (2003). Glutamine and Glutamate?their central Role in Cell Metabolism and Function. Cell Biochem. Funct. 21 (1), 1–9. doi:10.1002/cbf.1003
Nunnari, J., and Suomalainen, A. (2012). Mitochondria: in Sickness and in Health. Cell 148 (6), 1145–1159. doi:10.1016/j.cell.2012.02.035
O’Donnell, V. B., Rossjohn, J., and Wakelam, M. J. O. (2018). Phospholipid Signaling in Innate Immune Cells. J. Clin. Invest. 128 (7), 2670–2679. doi:10.1172/JCI97944
Oluboyo, P. O., and Erasmus, R. T. (1990). The Significance of Glucose Intolerance in Pulmonary Tuberculosis. Tubercle 71 (2), 135–138. doi:10.1016/0041-3879(90)90010-6
Onwubalili, J. K. (1988). Malnutrition Among Tuberculosis Patients in Harrow, England. Eur. J. Clin. Nutr. 42 (4), 363–366.
Opperman, M., Loots, D. T., van Reenen, M., Ronacher, K., Walzl, G., and du Preez, I. (2021). Chronological Metabolic Response to Intensive Phase TB Therapy in Patients with Cured and Failed Treatment Outcomes. ACS Infect. Dis. 7 (6), 1859–1869. doi:10.1021/acsinfecdis.1c00162
Paton, N. I., and Ng, Y.-M. (2006). Body Composition Studies in Patients with Wasting Associated with Tuberculosis. Nutrition 22 (3), 245–251. doi:10.1016/j.nut.2005.06.009
Paton, N. I., Ng, Y.-M., Chee, C. B., Persaud, C., and Jackson, A. A. (2003). Effects of Tuberculosis and HIV Infection on Whole-Body Protein Metabolism during Feeding, Measured by the [15N]glycine Method. Am. J. clincal Nutr. 78, 319–325. doi:10.1093/ajcn/78.2.319
Paton, N., Sangeetha, S., Earnest, A., and Bellamy, R. (2006). The Impact of Malnutrition on Survival and the CD4 Count Response in HIV-Infected Patients Starting Antiretroviral Therapy. HIV Med. 7 (5), 323–330. doi:10.1111/j.1468-1293.2006.00383.x
Pawlowski, A., Jansson, M., Sköld, M., Rottenberg, M. E., and Källenius, G. (2012). Tuberculosis and HIV Co-infection. Plos Pathog. 8 (2), e1002464. doi:10.1371/journal.ppat.1002464
Peltenburg, N. C., Schoeman, J. C., Hou, J., Mora, F., Harms, A. C., Lowe, S. H., et al. (2018). Persistent Metabolic Changes in HIV-Infected Patients during the First Year of Combination Antiretroviral Therapy. Sci. Rep. 8 (1), 16947. doi:10.1038/s41598-018-35271-0
Pett, S. L., Kunisaki, K. M., Wentworth, D., Griffin, T. J., Kalomenidis, I., Nahra, R., et al. (2017). Increased Indoleamine-2,3-Dioxygenase Activity Is Associated with Poor Clinical Outcome in Adults Hospitalized with Influenza in the INSIGHT FLU003Plus Study. Open Forum Infect. Dis. 5 (1), ofx228. doi:10.1093/ofid/ofx228
Philips, L., Visser, J., Nel, D., and Blaauw, R. (2017). The Association between Tuberculosis and the Development of Insulin Resistance in Adults with Pulmonary Tuberculosis in the Western Sub-district of the Cape Metropole Region, South Africa: a Combined Cross-Sectional, Cohort Study. BMC Infect. Dis. 17 (1), 570. doi:10.1186/s12879-017-2657-5
Pinti, M., Nasi, M., Gibellini, L., Roat, E., De Biasi, S., Bertoncelli, L., et al. (2010). The Role of Mitochondria in HIV Infection and its Treatment. J. Exp. Clin. Med. 2 (4), 145–155. doi:10.1016/s1878-3317(10)60024-1
Powanda, M. C., and Beisel, W. R. (2003). Metabolic Effects of Infection on Protein and Energy Status. J. Nutr. 133 (1), 322S–327S. doi:10.1093/jn/133.1.322S
Pozniak, A., Coyne, K., Miller, R., Lipman, M., Freedman, A., Ormerod, L., et al. (2011). British HIV Association Guidelines for the Treatment of TB/HIV Coinfection 2011. HIV Med. 12 (9), 517–524. doi:10.1111/j.1468-1293.2011.00954.x
Ramond, E., Jamet, A., Coureuil, M., and Charbit, A. (2019). Pivotal Role of Mitochondria in Macrophage Response to Bacterial Pathogens. Front. Immunol. 10 (2461). doi:10.3389/fimmu.2019.02461
Rodríguez-Gallego, E., Gómez, J., Domingo, P., Ferrando-Martínez, S., Peraire, J., Viladés, C., et al. (2018). Circulating Metabolomic Profile Can Predict Dyslipidemia in HIV Patients Undergoing Antiretroviral Therapy. Atherosclerosis 273, 28–36. doi:10.1016/j.atherosclerosis.2018.04.008
Rosado-Sánchez, I., Rodríguez-Gallego, E., Peraire, J., Viladés, C., Herrero, P., Fanjul, F., et al. (2019). Glutaminolysis and Lipoproteins Are Key Factors in Late Immune Recovery in Successfully Treated HIV-Infected Patients. Clin. Sci. 133 (8), 997–1010. doi:10.1042/CS20190111
Scarpelini, B., Zanoni, M., Sucupira, M. C. A., Truong, H.-H. M., Janini, L. M. R., Segurado, I. D. C., et al. (2016). Plasma Metabolomics Biosignature According to HIV Stage of Infection, Pace of Disease Progression, Viremia Level and Immunological Response to Treatment. PLoS One 11 (12), e0161920. doi:10.1371/journal.pone.0161920
Schutz, C., Meintjes, G., Almajid, F., Wilkinson, R. J., and Pozniak, A. (2010). Clinical Management of Tuberculosis and HIV-1 Co-infection. Eur. Respir. J. 36 (6), 1460–1481. doi:10.1183/09031936.00110210
Schwenk, A., Hodgson, L., Rayner, C. F., Griffin, G. E., and Macallan, D. C. (2003). Leptin and Energy Metabolism in Pulmonary Tuberculosis. Am. J. Clin. Nutr. 77, 392–398. doi:10.1093/ajcn/77.2.392
Schwenk, A., Hodgson, L., Wright, A., Ward, L. C., Rayner, C. F., Grubnic, S., et al. (2004). Nutrient Partitioning during Treatment of Tuberculosis: Gain in Body Fat Mass but Not in Protein Mass. Am. J. Clin. Nutr. 79 (6), 1006–1012. doi:10.1093/ajcn/79.6.1006
Selwyn, P. A., Pumerantz, A. S., Durante, A., Alcabes, P. G., Gourevitch, M. N., Boiselle, P. M., et al. (1998). Clinical Predictors of Pneumocystis Carinii Pneumonia, Bacterial Pneumonia and Tuberculosis in HIV-Infected Patients. AIDS 12 (8), 885–893. doi:10.1097/00002030-199808000-00011
Semba, R. D., Darnton-Hill, I., and de Pee, S. (2010). Addressing Tuberculosis in the Context of Malnutrition and HIV Coinfection. Food Nutr. Bull. 31 (4), S345–S364. doi:10.1177/15648265100314s404
Shan, Z., Clish, C. B., Hua, S., Scott, J. M., Hanna, D. B., Burk, R. D., et al. (2018). Gut Microbial-Related Choline Metabolite Trimethylamine-N-Oxide Is Associated with Progression of Carotid Artery Atherosclerosis in HIV Infection. J. Infect. Dis. 218 (9), 1474–1479. doi:10.1093/infdis/jiy356
Sharan, R., Bucşan, A. N., Ganatra, S., Paiardini, M., Mohan, M., Mehra, S., et al. (2020). Chronic Immune Activation in TB/HIV Co-infection. Trends Microbiology 28 (8), 619–632. doi:10.1016/j.tim.2020.03.015
Sharpton, S. R., Ajmera, V., and Loomba, R. (2019). Emerging Role of the Gut Microbiome in Nonalcoholic Fatty Liver Disease: from Composition to Function. Clin. Gastroenterol. Hepatol. 17 (2), 296–306. doi:10.1016/j.cgh.2018.08.065
Shi, L., Eugenin, E. A., and Subbian, S. (2016). Immunometabolism in Tuberculosis. Front. Immunol. 7, 150. doi:10.3389/fimmu.2016.00150
Silva, C. A. M., Graham, B., Webb, K., Ashton, L. V., Harton, M., Luetkemeyer, A. F., et al. (2019). A Pilot Metabolomics Study of Tuberculosis Immune Reconstitution Inflammatory Syndrome. Int. J. Infect. Dis. 84, 30–38. doi:10.1016/j.ijid.2019.04.015
Sitole, L. J., Tugizimana, F., and Meyer, D. (2019). Multi-platform Metabonomics Unravel Amino Acids as Markers of HIV/combination Antiretroviral Therapy-Induced Oxidative Stress. J. Pharm. Biomed. Anal. 176, 112796. doi:10.1016/j.jpba.2019.112796
Sitole, L. J., Williams, A. A., and Meyer, D. (2013). Metabonomic Analysis of HIV-Infected Biofluids. Mol. Biosyst. 9 (1), 18–28. doi:10.1039/c2mb25318f
Sodhi, C. P., Rana, S. V., Mehta, S. K., Vaiphei, K., Attri, S., Thakur, S., et al. (1997). Study of Oxidative Stress in Isoniazid-Induced Hepatic Injury in Young Rats with and without Protein-Energy Malnutrition. J. Biochem. Toxicol. 11 (3), 139–146. doi:10.1002/(SICI)1522-7146(1996)11:3<139:AID-JBT6>3.0.CO;2-L
Son, D. O., Satsu, H., and Shimizu, M. (2005). Histidine Inhibits Oxidative Stress- and TNF-α-Induced Interleukin-8 Secretion in Intestinal Epithelial Cells. FEBS Lett. 579 (21), 4671–4677. doi:10.1016/j.febslet.2005.07.038
Sonnenberg, P., Murray, J., Glynn, J. R., Shearer, S., Kambashi, B., and Godfrey-Faussett, P. (2001). HIV-1 and Recurrence, Relapse, and Reinfection of Tuberculosis after Cure: a Cohort Study in South African Mineworkers. The Lancet 358 (9294), 1687–1693. doi:10.1016/s0140-6736(01)06712-5
Sonnino, S., Chiricozzi, E., Grassi, S., Mauri, L., Prioni, S., and Prinetti, A. (2018). “Gangliosides in Membrane Organization,” in Progress in Molecular Biology and Translational Science. Editors R. L. Schnaar, and P. H. H. Lopez (Academic Press), 83–120. doi:10.1016/bs.pmbts.2017.12.007
Sterling, T. R., Pham, P. A., and Chaisson, R. E. (2010). HIV Infection-Related Tuberculosis: Clinical Manifestations and Treatment. Clin. Infect. Dis. 50, S223–S230. doi:10.1086/651495
Sucher, R., Kurz, K., Weiss, G., Margreiter, R., Fuchs, D., and Brandacher, G. (2010). IDO-mediated Tryptophan Degradation in the Pathogenesis of Malignant Tumor Disease. Int. J. Tryptophan Res. 3, IJTR.S4157–120. doi:10.4137/ijtr.s4157
Suzuki, S., Kodera, Y., Saito, T., Fujimoto, K., Momozono, A., Hayashi, A., et al. (2016). Methionine Sulfoxides in Serum Proteins as Potential Clinical Biomarkers of Oxidative Stress. Sci. Rep. 6, 38299. doi:10.1038/srep38299
Swaminathan, S., Padmapriyadarsini, C., Sukumar, B., Iliayas, S., Kumar, S. R., Triveni, C., et al. (2008). Nutritional Status of Persons with HIV Infection, Persons with HIV Infection and Tuberculosis, and HIV-Negative Individuals from Southern India. Clin. Infect. Dis. 46 (6), 946–949. doi:10.1086/528860
Tang, A. M., Forrester, J., Spiegelman, D., Knox, T. A., Tchetgen, E., and Gorbach, S. L. (2002). Weight Loss and Survival in HIV-Positive Patients in the Era of Highly Active Antiretroviral Therapy. J. Acquir Immune Defic Syndr. 31 (2), 230–236. doi:10.1097/00126334-200210010-00014
Tarancon-Diez, L., Rodríguez-Gallego, E., Rull, A., Peraire, J., Viladés, C., Portilla, I., et al. (2019). Immunometabolism Is a Key Factor for the Persistent Spontaneous Elite Control of HIV-1 Infection. EBioMedicine 42, 86–96. doi:10.1016/j.ebiom.2019.03.004
Taylor, G. O., and Bamgboye, A. E. (1979). Serum Cholesterol and Diseases in Nigerians. Am. J. Clin. Nutr. 32 (12), 2540–2545. doi:10.1093/ajcn/32.12.2540
Thandi, R. S., Radhakrishnan, R. K., Tripathi, D., Paidipally, P., Azad, A. K., Schlesinger, L. S., et al. (2020). Ornithine-A Urea Cycle Metabolite Enhances Autophagy and Controls Mycobacterium tuberculosis Infection. Nat. Commun. 11 (1), 3535. doi:10.1038/s41467-020-17310-5
Tiberi, S., Carvalho, A. C. C., Sulis, G., Vaghela, D., Rendon, A., Mello, F. C. d. Q., et al. (2017). The Cursed Duet Today: Tuberculosis and HIV-Coinfection. La Presse Médicale 46 (2), e23–e39. doi:10.1016/j.lpm.2017.01.017
Tilokani, L., Nagashima, S., Paupe, V., and Prudent, J. (2018). Mitochondrial Dynamics: Overview of Molecular Mechanisms. Essays Biochem. 62 (3), 341–360. doi:10.1042/EBC20170104
Toossi, Z. (2003). Virological and Immunological Impact of Tuberculosis on Human Immunodeficiency Virus Type 1 Disease. J. Infect. Dis. 188 (8), 1146–1155. doi:10.1086/378676
Török, M. E., Yen, N. T. B., Chau, T. T. H., Mai, N. T. H., Phu, N. H., Mai, P. P., et al. (2011). Timing of Initiation of Antiretroviral Therapy in Human Immunodeficiency Virus (HIV)-Associated Tuberculous Meningitis. Clin. Infect. Dis. 52 (11), 1374–1383. doi:10.1093/cid/cir230
van der Valk, M., Reiss, P., van Leth, F. C., Ackermans, M. T., Endert, E., Romijn, J. A., et al. (2002). Highly Active Antiretroviral Therapy-Induced Lipodystrophy Has Minor Effects on Human Immunodeficiency Virus-Induced Changes in Lipolysis, but Normalizes Resting Energy Expenditure. J. Clin. Endocrinol. Metab. 87 (11), 5066–5071. doi:10.1210/jc.2002-020892
van Laarhoven, A., Dian, S., Aguirre-Gamboa, R., Avila-Pacheco, J., Ricaño-Ponce, I., Ruesen, C., et al. (2018). Cerebral Tryptophan Metabolism and Outcome of Tuberculous Meningitis: an Observational Cohort Study. Lancet Infect. Dis. 18 (5), 526–535. doi:10.1016/S1473-3099(18)30053-7
Van Lettow, M., Fawzi, W. W., Semba, P. H., and Semba, R. D. (2003). Triple Trouble: the Role of Malnutrition in Tuberculosis and Human Immunodeficiency Virus Co-infection. Nutr. Rev. 61 (3), 81–90. doi:10.131/nr.2003.marr.81-9010.1301/nr.2003.marr.81-90
Vanham, G., Edmonds, K., Qing, L., Hom, D., Toossi, Z., Jones, B., et al. (1996). Generalized Immune Activation in Pulmonary Tuberculosis: Co-activation with HIV Infection. Clin. Exp. Immunol. 103 (1), 30–34. doi:10.1046/j.1365-2249.1996.907600.x
Varshney, E., Tandon, M., Saha, N., and Ali, S. (2019). In Vivo phenotyping of Cytochrome 450 Isoforms Involved in the Metabolism of Anti-HIV and Anti-tubercular Drugs in Human Using Cocktail Approach: An LC-MS/MS Analysis. J. Pharm. Biomed. Anal. 164, 698–705. doi:10.1016/j.jpba.2018.11.026
Verma, S., Du, P., Nakanjako, D., Hermans, S., Briggs, J., Nakiyingi, L., et al. (2018). "Tuberculosis in Advanced HIV Infection Is Associated with Increased Expression of IFNγ and its Downstream Targets". BMC Infect. Dis. 18 (1), 220. doi:10.1186/s12879-018-3127-4
Villamor, E., Saathoff, E., Mugusi, F., Bosch, R. J., Urassa, W., and Fawzi, W. W. (2006). Wasting and Body Composition of Adults with Pulmonary Tuberculosis in Relation to HIV-1 Coinfection, Socioeconomic Status, and Severity of Tuberculosis. Eur. J. Clin. Nutr. 60 (2), 163–171. doi:10.1038/sj.ejcn.1602281
Vrieling, F., Alisjahbana, B., Sahiratmadja, E., van Crevel, R., Harms, A. C., Hankemeier, T., et al. (2019). Plasma Metabolomics in Tuberculosis Patients with and without Concurrent Type 2 Diabetes at Diagnosis and during Antibiotic Treatment. Sci. Rep. 9 (1), 18669. doi:10.1038/s41598-019-54983-5
Vrieling, F., Kostidis, S., Spaink, H. P., Haks, M. C., Mayboroda, O. A., Ottenhoff, T. H. M., et al. (2020). Analyzing the Impact of Mycobacterium tuberculosis Infection on Primary Human Macrophages by Combined Exploratory and Targeted Metabolomics. Sci. Rep. 10 (1), 7085. doi:10.1038/s41598-020-62911-1
Vrieling, F., Ronacher, K., Kleynhans, L., van den Akker, E., Walzl, G., Ottenhoff, T. H. M., et al. (2018). Patients with Concurrent Tuberculosis and Diabetes Have a Pro-atherogenic Plasma Lipid Profile. EBioMedicine 32, 192–200. doi:10.1016/j.ebiom.2018.05.011
Vujkovic-Cvijin, I., and Somsouk, M. (2019). HIV and the Gut Microbiota: Composition, Consequences, and Avenues for Amelioration. Curr. Hiv/aids Rep. 16 (3), 204–213. doi:10.1007/s11904-019-00441-w
Walzl, G., McNerney, R., du Plessis, N., Bates, M., McHugh, T. D., Chegou, N. N., et al. (2018). Tuberculosis: Advances and Challenges in Development of New Diagnostics and Biomarkers. Lancet Infect. Dis. 18 (7), e199–e210. doi:10.1016/s1473-3099(18)30111-7
Wang, X., Mehra, S., Kaushal, D., Veazey, R. S., and Xu, H. (2021). Abnormal Tryptophan Metabolism in HIV and Mycobacterium tuberculosis Infection. Front. Microbiol. 12, 666227. doi:10.3389/fmicb.2021.666227
Waters, R., Ndengane, M., Abrahams, M.-R., Diedrich, C. R., Wilkinson, R. J., and Coussens, A. K. (2020). The Mtb-HIV Syndemic Interaction: Why Treating M. tuberculosis Infection May Be Crucial for HIV-1 Eradication. Future Virol. 15 (2), 101–126. doi:10.2217/fvl-2019-0069
Watford, M. (2003). The Urea Cycle: Teaching Intermediary Metabolism in a Physiological Setting. Biochem. Mol. Biol. Educ. 31 (5), 289–297. doi:10.1002/bmb.2003.494031050249
Weiner, J., Maertzdorf, J., Maertzdorf, J., Sutherland, J. S., Duffy, F. J., Thompson, E., et al. (2018). Metabolite Changes in Blood Predict the Onset of Tuberculosis. Nat. Commun. 9 (1). doi:10.1038/s41467-018-07635-7
Weiner, J., Parida, S. K., Maertzdorf, J., Black, G. F., Repsilber, D., Telaar, A., et al. (2012). Biomarkers of Inflammation, Immunosuppression and Stress Are Revealed by Metabolomic Profiling of Tuberculosis Patients. PLoS ONE 7 (7), e40221. doi:10.1371/journal.pone.0040221
Wenning, L. A., Hanley, W. D., Brainard, D. M., Petry, A. S., Ghosh, K., Jin, B., et al. (2009). Effect of Rifampin, a Potent Inducer of Drug-Metabolizing Enzymes, on the Pharmacokinetics of Raltegravir. Antimicrob. Agents Chemother. 53 (7), 2852–2856. doi:10.1128/AAC.01468-08
Williams, A. A., Sitole, L. J., and Meyer, D. (2017). HIV/HAART-associated Oxidative Stress Is Detectable by Metabonomics. Mol. Biosyst. 13 (11), 2202–2217. doi:10.1039/c7mb00336f
Williams, A., Koekemoer, G., Lindeque, Z., Reinecke, C., and Meyer, D. (2011). Qualitative Serum Organic Acid Profiles of HIV-Infected Individuals Not on Antiretroviral Treatment. Metabolomics 8 (5), 804–818. doi:10.1007/s11306-011-0376-2
Williams, A., Steffens, F., Reinecke, C., and Meyer, D. (2013). The Th1/Th2/Th17 Cytokine Profile of HIV-Infected Individuals: a Multivariate Cytokinomics Approach. Cytokine 61 (2), 521–526. doi:10.1016/j.cyto.2012.11.006
Winglee, K., Eloe-Fadrosh, E., Gupta, S., Guo, H., Fraser, C., and Bishai, W. (2014). Aerosol Mycobacterium tuberculosis Infection Causes Rapid Loss of Diversity in Gut Microbiota. PLOS ONE 9 (5), e97048. doi:10.1371/journal.pone.0097048
Wood, R., Maartens, G., and Lombard, C. J. (2000). Risk Factors for Developing Tuberculosis in HIV-1-Infected Adults From Communities with a Low or Very High Incidence of Tuberculosis. J. Acquir Immune Defic Syndr. 23 (1), 75–80. doi:10.1097/00126334-200001010-00010
World Health Organization(2016a). Consolidated Guidelines on HIV Prevention, Diagnosis, Treatment and Care for Key Populations 2016. Geneva: World Health Organization.
World Health Organization (2016b). Consolidated Guidelines on the Use of Antiretroviral Drugs for Treating and Preventing HIV Infection: Recommendations for a Public Health Approach. Geneva: World Health Organization.
World Health Organization (2019). Global Tuberculosis Report 2019. Geneva: World Health Organization.
World Health Organization (2020). Global Tuberculosis Report 2020. Geneva: World Health Organization.
World Health Organization (2015). The Use of Lateral Flow Urine Lipoarabinomannan Assay (LF-LAM) for the Diagnosis and Screening of Active Tuberculosis in People Living with HIV. Geneva: World Health Organization.
World Health Organization (2012). WHO Policy on Collaborative TB/HIV Activities: Guidelines for National Programmes and Other Stakeholders. Geneva: World Health Organization.
Yao, X., Liu, Y., Liu, Y., Liu, W., Ye, Z., Zheng, C., et al. (2017). Multiplex Analysis of Plasma Cytokines/chemokines Showing Different Immune Responses in Active TB Patients, Latent TB Infection and Healthy Participants. Tuberculosis 107, 88–94. doi:10.1016/j.tube.2017.07.013
Yoneda, J., Andou, A., and Takehana, K. (2009). Regulatory Roles of Amino Acids in Immune Response. Crr 5 (4), 252–258. doi:10.2174/157339709790192567
Yu, B., Li, A. H., Muzny, D., Veeraraghavan, N., de Vries, P. S., Bis, J. C., et al. (2015). Association of Rare Loss-Of-Function Alleles in HAL, Serum Histidine. Circ. Cardiovasc. Genet. 8 (2), 351–355. doi:10.1161/CIRCGENETICS.114.000697
Zachariah, R., Spielmann, M. P., Harries, A. D., and Salaniponi, F. M. L. (2002). Moderate to Severe Malnutrition in Patients with Tuberculosis Is a Risk Factor Associated with Early Death. Trans. R. Soc. Trop. Med. Hyg. 96 (3), 291–294. doi:10.1016/s0035-9203(02)90103-3
Zackin, R. A., Clark, R. A., Currier, J. S., and Mildvan, D. (1999). Predictive Markers of HIV-Related Weight Loss and Determination of Differences Between Populations with Weight Loss Stratified by Opportunistic Processes. J. Acquir Immune Defic Syndr. 22 (2), 189–193. doi:10.1097/00126334-199910010-00012
Zhang, S., Carriere, J., Lin, X., Xie, N., and Feng, P. (2018). Interplay between Cellular Metabolism and Cytokine Responses during Viral Infection. Viruses 10 (10), 521. doi:10.3390/v10100521
Keywords: HIV/AIDS, tuberculosis, HIV/TB co-infection, metabolomics, metabolism, treatment
Citation: Liebenberg C, Luies L and Williams AA (2021) Metabolomics as a Tool to Investigate HIV/TB Co-Infection. Front. Mol. Biosci. 8:692823. doi: 10.3389/fmolb.2021.692823
Received: 09 April 2021; Accepted: 04 October 2021;
Published: 20 October 2021.
Edited by:
Mahbuba Rahman, Qatar Biomedical Research Institute, QatarReviewed by:
Oleg Mayboroda, Leiden University Medical Center, NetherlandsGrant R. Campbell, University of California, San Diego, United States
Copyright © 2021 Liebenberg, Luies and Williams. This is an open-access article distributed under the terms of the Creative Commons Attribution License (CC BY). The use, distribution or reproduction in other forums is permitted, provided the original author(s) and the copyright owner(s) are credited and that the original publication in this journal is cited, in accordance with accepted academic practice. No use, distribution or reproduction is permitted which does not comply with these terms.
*Correspondence: Aurelia A. Williams, YXVyZWxpYS53aWxsaWFtc0Bud3UuYWMuemE=