- 1Department of Biological and Environmental Sciences, Alabama A&M University, Huntsville, AL, United States
- 2Department of Microbiology, College of Medicine, Howard University, Washington, DC, United States
- 3Department of Biology, Howard University, Washington, DC, United States
RseA is the critical central regulator of the σE-dependent stress response in E. coli and other related bacteria. The synthesis of RseA is controlled at the transcriptional level by several promoters and transcriptional regulators, including σE itself at two σE-dependent promoters: rpoEP and rseAP3. The presence of these two independent polycistrons encoding rseA is potentially redundant. We hypothesized that post-transcriptional control of the rseAP3 transcript was necessary to overcome this redundancy. However, to date, nothing is known about the post-transcriptional control of the rseAP3 transcript. We executed a targeted genetic screen to identify small RNA regulators of the rseAP3 transcript and identified RyhB and FnrS as small RNA activators of the RseA P3 transcript. Through genetic analysis, we confirmed that a direct interaction occurs between RyhB and RseA. We also identified sequences within the 5′ untranslated region (UTR) of RseA that were inhibitory for RseA expression. Point mutations predicted to prevent an interaction between RyhB and RseA resulted in increased RseA expression. Taken together, this suggests that the 5’ UTR of the RseAP3 transcript prevents optimal expression of RseA, preventing redundancy due to RseA expression from the σE-dependent rpoEP, and this is overcome by the stimulatory activity of RyhB and FnrS.
Introduction
The cell envelope promotes structural integrity of bacteria under dynamic conditions. The cell envelope can be perturbed via internal or external cues. Envelope stress occurs as a result of excess or mis-folded outer membrane proteins (OMPs), subsequently resulting in the activation of the extracytoplasmic function (ECF) sigma factor, σE (Erickson et al., 1987; Mecsas et al., 1993; Walsh et al., 2003). Over-expression or mis-folding of OMPs can compromise the integrity of the bacterial cell envelope and therefore bacterial cell survival (Walsh et al., 2003).
E. coli and other bacterial species have developed an envelope stress response (ESR) that restores homeostasis following the onset of envelope stress. The ESR can be mediated by several key regulators in E. coli, most notably CpxR and σE (Ravio, 1999; Ravio et al., 1999). In this work, we focused on the σE-dependent ESR. Activation of σE-dependent promoters results in the transcriptional initiation of at least 60 genes, many of which encode proteins necessary for the resolution of the ESR (Dartigalongue et al., 2001; Johansen et al., 2006; Rhodius et al., 2006; Thompson et al., 2007). Effectors of the ESR include small RNAs and periplasmic proteases that act to repress OMP levels (Douchin et al., 2006; Johansen et al., 2006; Rhodius et al., 2006; Thompson et al., 2007; Vogt et al., 2014). The stabilization of OMP levels restores cell envelope homeostasis. This also results in decreased σE activity. It is critically important to ensure that the steady-state levels of σE do not increase indefinitely as σE over-expression results in cell lysis and death (Nitta et al., 2000; Kabir et al., 2005). The small RNAs that act as σE-dependent ESR effectors include MicA, RybB, and MicL; all of which have σE-dependent promoters, repress expression of outer membrane proteins, and regulate LPS composition (Johansen et al., 2006; Thompson et al., 2007; Udekwu and Wagner, 2007; Klein et al., 2011; Klein and Raina, 2017).
The σE-dependent ESR is tightly controlled via regulation of the rpoE operon (rseD-rpoE-rseA-rseB-rseC) at transcriptional and post-transcriptional levels (Klein et al., 2016; Yakhnin et al., 2017). There are several promoters driving expression of this operon in concert with multiple transcription and sigma factors (Klein et al., 2016; Klein and Raina, 2017; Yakhnin et al., 2017). Transcriptional control of the σE-dependent promoters is also controlled by guanosine 3′,5′-bispyrophosphate (ppGpp) and promoter architecture (Costanzo and Ades, 2006; Thompson et al., 2007; Costanzo et al., 2008; Balbontin et al., 2010). Only two of the promoters driving expression of the rpoE operon are σE-dependent, while the others are cognate partners to σ54, σs, σ70, or other transcriptional regulators (Klein et al., 2016). All promoters driving expression of the rpoE operon are localized upstream of, or within, the rpoE leader peptide (RseD) (Figure 1A). The remaining σE-dependent promoter (rseAP3) is located within the rpoE coding region, over 230 nucleotides upstream of the rseA start codon (Rhodius et al., 2006). The σE-dependent rseAP3 promoter drives the synthesis of an rseA-rseB-rseC transcript, while the σE-dependent promoter rpoEP drives the synthesis of the entire rseD-rpoE-rseA-rseB-rseC transcript (Rhodius et al., 2006). The activity of the σE-dependent stress response is also regulated at the transcriptional level by the activity of RseA.
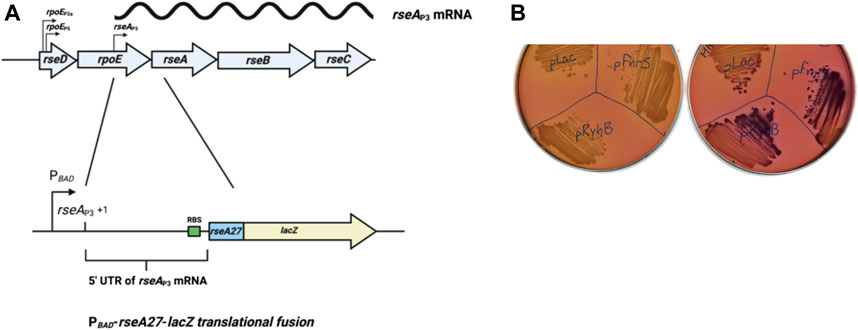
FIGURE 1. Genetic screen for small RNA regulators (A). Schematic of the rpoE operon with selected promoters, including the rseAP3 promoter, and the PBAD-rseA27-lacZ translational fusion utilized for the small RNA library screen. The rseA fusion consists of the rseA P3 transcript, and the first nine codons of the rseA gene fused in the frame to the ninth codon of lacZ, downstream and controlled by the araBAD promoter (PBAD-rseA27-lacZ translational fusion) (B). PBAD-rseA27-lacZ translational fusion strains transformed with an empty vector control (pBR-pLac), pBR-ryhB, or pBR-fnrS grown overnight at 37°C on MacConkey-Lactose agar plates. One plate was supplemented with 0.0002% arabinose to stimulate basal transcription of the PBAD-rseA27-lacZ translational fusion.
RseA is the anti-sigma factor for σE and plays a central role in envelope stress signal transduction (De Las Penans et al., 1997; Missiakas et al., 1997; Ades et al., 1999). RseA spans the inner membrane of the cell envelope and utilizes its cytoplasmic domain to interact with σE (Campbell et al., 2003). RseA sequesters σE to the inner member under vegetative growth conditions and thereby prevents access of σE to its cognate promoters (Campbell et al., 2003). Envelope stress stimulates a signal transduction cascade culminating in regulated intramembrane proteolysis (RIP) of RseA by periplasmic proteases DegS and RseP (De Las Penans et al., 1997; Missiakas et al., 1997; Alba et al., 2002; Kanehara et al., 2002). Following this, the N-terminus of RseA enters the cytoplasm in complex with σE, whereby the RseA N-terminus is then cleaved by the adenosine 5′-triphosphate-dependent protease ClpXP (Flynn et al., 2004).
The synthesis of RseA from σE-dependent rseAP3 and rpoEP promoters has the potential to be physiologically redundant. It is likely that post-transcriptional regulation of rpoE and rseABC operons removes any physiological redundancy. While post-transcriptional regulatory mechanisms have been identified for the rpoE operon, little is known about post-transcriptional regulation of the rseABC operon. The rseABC operon has a 228 nucleotide 5′ untranslated region (UTR) (Rhodius et al., 2006). Due to the presence of the relatively long 5′ UTR for the rseABC operon, we hypothesized that cis-acting RNA structures and trans-acting small RNAs regulate the expression of rseABC operon. To test this hypothesis, we constructed an arabinose-inducible rseA-lacZ translational fusion and screened it with a small RNA library. We identified RyhB and FnrS as factors that stimulate post-transcriptional expression of RseA.
Materials and Methods
Media and Growth Conditions
All strains were grown in Luria Bertani (Lennox) liquid media (LB) at 37°C, with the exception of λ–Red-based recombineering strains using mini-λ:tet lysogens. These strains were grown in LB at 30°C and then shifted 43.5°C to induce expression of λ-Red proteins. Transformants were grown on LB agar plates supplemented with ampicillin, to a final concentration of 100 μg/ml. Zeomycin-resistant recombinants or transductants were selected on LB agar plates supplemented with Zeocin™ (or zeomycin) to a final concentration of 25 μg/ml. Small RNA screens were executed on MacConkey-Lactose (Mac-Lac) agar plates supplemented with ampicillin, to a final concentration of 100 μg/ml, and arabinose to a final concentration of 0.02% or 0.00002%. All gene fusions were created as previously described using recombineering and selecting for recombinant fusions on M63 minimal salt agar plates supplemented with glycerol, 6% sucrose, and 80 μg/ml of X-gal at 30°C (Mandin and Gottesman, 2009). For iron starvation experiments, cultures were grown in LB media in a shaking water bath at 37°C to an OD600 of 0.3 and then treated with 2′2-dipyridyl (Sigma Aldrich) to a final concentration of 250 μM for 30 min. For RNA stability assays, rifampicin was added to bacterial cultures to a final concentration of 250 μg/ml.
Bacterial Strains, Plasmids, and Genetic Constructs
All strains used for experiments conducted in this study were derivatives of Escherichia coli K-12 MG1655. Cloning reactions were executed in MC1061 or NEB5α (New England Biolabs). All strains are listed in Table 1. All plasmids used in this study are listed in Table 2. All oligonucleotides used for polymerase chain reaction (PCR)-mediated genetic engineering, PCR screening, or Northern blot analysis are listed in Table 3. λ-Red recombineering reactions to gene fusions were executed in strain PM1800. PM1800 has a cat-sacB cassette inserted in the lac locus and encodes the λ-Red proteins (gam, exo, and beta) on a partial lambda vector marked with tetracycline resistance (mini-λ:tet). Plasmids from the small RNA library or their respective mutants were transformed into the PBAD-rseA27-lacZ translational fusion strain using TSS transformation (Chung et al., 1989). Mutations were transduced into reporter fusion strains using Bacteriophage P1 transduction.
Construction of PBAD-rseA27-lacZ and Translational Fusion and PBAD-rseA-3XFLAG Strains
In order to execute our screen for small RNA regulation of the rseABC operon, we first created an arabinose-inducible in-frame translational fusion of the first nine codons of the rseA gene (rseA27) to the ninth codon of lacZ via recombineering into strain PM1800 as previously described (Mandin and Gottesman, 2009). We also created a 3XFLAG-tagged allele of the entire rseA gene at the lac locus using the same recombineering method (Mandin and Gottesman, 2009). All the fusions contained the entire 5′ UTR of the rseABC transcript (Figure 1A) immediately downstream from the arabinose-inducible araBAD promoter (PBAD). To create the allelic exchange substrates for either the PBAD-rseA27-lacZ, PBAD-rseA27cm1-lacZ, PBAD-rseA27cm3-lacZ, or PBAD-rseA-3XFLAG, or PBAD-rseAcm1-3XFLAG fusions, we amplified synthetic DNA gBlocks (IDT DNA) corresponding to each fusion using oligonucleotide primers KT902 and KT903. All synthetic DNA sequences used for genetic engineering of gene fusions are listed in Table 4. All oligonucleotide primers used for PCR reactions are listed in Table 3. We confirmed the presence of the lacZ translational fusion inserts by PCR using oligonucleotide primers KT940 and KT903 and DNA sequencing. We confirmed the presence of the 3XFLAG tagged alleles by PCR using oligonucleotide primers KT902 and KT1136 and DNA sequencing.
Site-Directed Mutagenesis of pBR-pLac-ryhB
We used the QuikChange® Site-Directed Mutagenesis Kit (Stratagene), according to the manufacturer’s recommendations to create ryhB point mutants. Mutagenic primers used for the PCR reaction are listed in Table 3. Point mutants were verified by DNA sequencing.
β-Galactosidase Assays
Overnight cultures were grown in Lennox Broth (LB) supplemented with ampicillin and glucose to a final concentration of 100 μg/ml and 0.2%, respectively, at 37°C. The cultures were diluted 1:1000 in fresh Lennox Broth (LB) supplemented with ampicillin and arabinose to a final concentration of 100 μg/ml and 0.02%, respectively. Once the culture reached an OD600 of 0.4–0.5, a 100 μL aliquot was taken for the β-galactosidase assay as previously described (Miller, 1992). Alternatively, β-galactosidase assays were executed in 96-well plates as previously described (Zhou and Gottesman, 1998).
Western Blot Analysis
Cell lysates were created as previous described (Ezemaduka et al., 2014). Briefly, cells were harvested and resuspended in 300 ul of 20 mM Tris-HCl, pH 8.0, and lysed by sonication. The resulting cell lysates were centrifuged at 800 × g for 5-min to remove cell debris and unbroken cells. The supernatants for the respective samples were then transferred to new 1.5 ml tubes and subjected to centrifugation at 1,000 × g for 30 min. The resulting supernatant (soluble proteins) and precipitate (aggregated proteins) from the second centrifugation were then quantified using a Lowry assay and placed on ice. Equivalent amounts of total protein were prepared and subjected to electrophoresis using a Bolt™ 12%, Bis-Tris Protein Gel (Invitrogen), according to the manufacturer’s instructions. The total proteins were then transferred to a 0.45 μm pore-size nitrocellulose membrane using a Trans-Blot® Turbo™ System (BIO-RAD) for 10 min at 2.5 A and 25 V. After the successful transfer, the membrane was washed briefly in a phosphate-buffered saline and Tween 20 (PBST) solution and then blocked at room temperature for 30 min in 0.5% Blotting-Grade nonfat milk dissolved in PBST. The membrane was incubated overnight with gentle shaking at 4°C in a 1:50,000 dilution of a primary antibody and 0.5% Blotting-Grade nonfat milk blocking solution. The membrane was washed with PBST three times for 5 min each and incubated with gentle shaking in a 1:50,000 dilution of a secondary antibody and 0.5% Blotting-Grade nonfat milk blocking solution for 2 h. The signal was developed using a Novex AP Chemiluminescent Kit according to the manufacturer’s recommendations (Thermofisher Scientific). The protein signals were visualized using the FluorChem R imager (Protein Simple).
Northern Blot Analysis
Total RNA was isolated using the hot acid phenol method as previously described (Aiba et al., 1981). RNA stability assays were performed as previously described using hot phenol following culture treatment with rifampicin to a final concentration of 250 µg/ml (Masse et al., 2003). Total RNA from each sample was mixed with the 10X RNA gel loading dye (National Diagnostics), 10X MOPS buffer, 100% formaldehyde, and 100% formamide and heated at 65°C for 15 min. The samples were loaded onto a 1% agarose gel and subjected to electrophoresis at 100 V for 40 min. The agarose gel was subjected to a nylon membrane capillary transfer. Following UV cross-linking, the membrane was pre-hybridized for 2 h using PerfectHyb™ Plus Hybridization Buffer (Sigma Aldrich). The membrane was then hybridized with a biotinylated DNA probe against RseA or 16S rRNA transcripts. (IDT DNA) for 4 h (Table 3). The membrane was washed with high, medium, and low stringency buffers and processed using a Chemiluminescent Detection Kit (Lifetechnologies) according to the manufacturer’s recommendation. The chemiluminescent signal was detected using Fluorochem E (Protein Simple).
Statistical Analysis
All statistical analyses were executed using GraphPad Prism version 9 (GraphPad).
Results
RyhB and FnrS were Picked up in a Genetic Screen for Small RNA Regulators of RseA Expression
The start site of the rseAP3 promoter is 228 nucleotides upstream from the rseA start codon. The 228 nucleotide 5′ UTR likely forms a secondary structure that influences translational initiation in concert with small RNAs. We therefore hypothesized that the rseABC operon, driven by the rseAP3 promoter, was regulated at the post-transcriptional level by a small RNA. In order to determine if the rseAP3 transcript was regulated by a small RNA, we constructed an arabinose-inducible rseA-lacZ translational gene fusion (Figure 1A). We then transformed the PBAD-rseA27-lacZ translational fusion strain with a plasmid based small RNA library as previously described (Mandin and Gottesman, 2009). This small RNA library contains 30 of the most extensively characterized E. coli small RNAs cloned downstream of an isopropyl β-d-1-thiogalactopyranoside (IPTG)-inducible promoter (Mandin and Gottesman, 2009). We executed the small RNA library screen on MacConkey-Lactose (Mac-Lac) agar plates supplemented with ampicillin to a final concentration of 100 μg/ml and arabinose to a final concentration of 0.0002%. This arabinose concentration was sufficient to induce basal transcription from the PBAD promoter without producing a strong Lac+ phenotype. The concentration of lactose in the Mac-Lac plates was sufficient to induce small RNA expression from the IPTG-inducible promoter. We hypothesized that this screening condition was ideal for the possible identification of stimulatory small RNAs. Two of the 30 plasmids, carrying RyhB or FnrS, resulted in an increased Lac phenotype (Figure 1B), suggesting that RyhB and FnrS promote post-transcriptional expression of RseA.
RyhB and FnrS Point Mutants are Defective in Stimulating RseA Expression
To validate the results of our genetic screen, we executed computational analysis to identify regions of potential complementary base pairing between RyhB or FnrS and the RseA leader region (Figures 2A,B). Specifically, we screened the RyhB and FnrS sequences against the RseAP3 5′ UTR sequence using IntaRNA 2.0. For RyhB, we identified a semi-continuous stretch of nucleotides between C14 and C37 with complementarity to RseAP3 G128 to G155 (Figure 2A). The longest stretch of complementarity was seven base pairs between RyhB C14 to RyhB C20 and RseA G148 to G155. For FnrS, we identified a semi-continuous stretch of nucleotides between U44 and U57 with complementarity to RseAP3 A152 and A167 (Figure 2B). The longest stretch of which was a seven base pair region of complementarity between FnrS C51 to U57 and RseA A152 to G158 (Figure 2B). We then created nucleotide point mutants in the plasmid-based RyhB construct that would disrupt the predicted complimentary base pairing between RyhB and RseA (Figure 2A). The RyhB point mutants consist of the following changes: m1 (C14G, C15G, C16G), m2 (C18G, G19C, C20G, and G21C), and m3 (G21C, G22C, A23C, G24A, A25C, and A26C) (Figure 2A). The FnrS point mutants were previously described and consist of the following changes: fnrS-I (U57A, U58G, U59A), fnrS-II (C47A U48A U49G), and fnrS-III (G4C, G5U) (Durand and Storz, 2010).
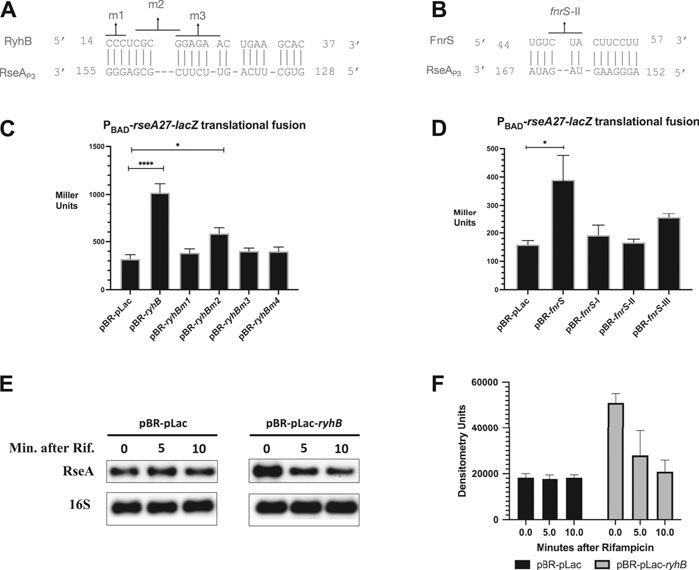
FIGURE 2. RyhB/FnrS pairing with RseA and β-galactosidase assays (A). Regions of predicted base pairing between the RyhB and RseA sequences were determined using the online computational tool IntaRNA 2.0. Point mutants in the small RNA used for interaction analysis were denoted as m1 (C14G, C15G, C16G), m2 (C18G, G19C, C20G, and G21C), or m3 (G21C, G22C, A23C, G24A, A25C, and A26C) (B). ΔryhB ΔfnrS PBAD-rseA27-lacZ translational fusions containing pBR-pLac, pBR-ryhB, pBR-pLac-ryhBm1, pBR-pLac-ryhBm2, or pBR-pLac-ryhBm3 were grown in rich media to an OD600 of 0.5, and aliquots were isolated for the β-galactosidase assay (C). Regions of predicted base pairing between FnrS small RNA and the 5′ UTR of RseA were determined using the online computational tool IntaRNA 2.0. One of the fnrS point mutants tested occurs in the region of pairing shown (B). ΔryhB ΔfnrS PBAD-rseA27-lacZ translational fusions containing pBR-pLac, pBR-ryhB, pBR-pLac-ryhBm1, pBR-pLac-ryhBm2, or pBR-pLac-ryhBm3 were grown in rich media to an OD600 of 0.5, and aliquots were isolated for the β-galactosidase assay (D). ΔryhB ΔfnrS PBAD-rseA27-lacZ translational fusions containing pBR-pLac, pBR-fnrS, pBR-pLac-fnrS-I, pBR-pLac-fnrS-II, or pBR-pLac-fnrS-III were grown in rich media to an OD600 of 0.5, and aliquots were isolated for the β-galactosidase assay (E). PBAD-rseA27-lacZ translational fusions were grown to the mid-exponential phase and induced with arabinose and IPTG to a final concentration of 0.2% and 1 mM, respectively. Cultures were then treated with rifampicin, and total RNA was isolated at 5 and 10 min following rifampicin treatment. RNA was subjected to northern blot analysis using a biotinylated RseA probe (F). Densitometric analysis of northern blot is shown in Panel E. All β-galactosidase assays and densitometry assays were executed in triplicate and are represented as averages ± the standard error of the mean (SEM). Statistical significance was assessed by one-way ANOVA with Dunnett’s post hoc test (*p < 0.05, ****p < 0.0001).
In order to determine if the RyhB nucleotides predicted to pair with RseA are necessary for RseA stimulation, we determined the activity of the PBAD-rseA27-lacZ translational fusion upon over-expression of wild type and mutant alleles of RyhB. We executed these assays in a ryhB− fnrS− genetic background to ensure that the plasmids were the only source of RyhB or FnrS expression. We grew all strains in LB (Lennox) supplemented with ampicillin and arabinose to final concentrations of 100 μg/ml and 0.02%, respectively. We then obtained 100 μL aliquots of each culture in the mid-log phase of growth (OD600 of 0.5) and measured β-galactosidase activity. As expected, plasmid-based RyhB induced PBAD-rseA27-lacZ activity by approximately 3-fold in comparison to the vector control (Figure 2C), while all RyhB point mutants were defective for stimulation of PBAD-rseA27-lacZ (Figure 2C). This confirms the results of our genetic screen and suggests that RyhB stimulates post-transcriptional expression of RseA. Further, it suggests that RyhB nucleotides predicted to pair with nucleotides in the RseA leader region are necessary for RyhB post-transcriptional stimulation of RseA expression (Figure 2C). We executed a similar experiment using FnrS and a series of FnrS point mutants to determine if FnrS may stimulate the post-transcriptional expression of RseA. FnrS expression results in a 2-fold increase in PBAD-rseA27-lacZ. Each of the FnrS point mutants were also defective for stimulation of PBAD-rseA27-lacZ. To determine if the RyhB stimulatory effect on RseAP3 expression was due to an increase in RseA mRNA stability, we tested the stability of the RseA-LacZ following over-expression of RyhB (Figures 2E,F). We observed a 2-fold increase in RseA-LacZ mRNA levels prior to the initiation of mRNA stability measures. The change in RseA-LacZ mRNA levels is similar to the change in PBAD-rseA27-lacZ activity in the presence of RyhB. This suggests that RyhB stimulation of RseA post-transcriptional expression could occur at the level of transcription termination as previously described (Chen et al., 2019).
Compensatory Mutations in RseA Suggest that RyhB Interacts with the RseA Leader Region
In order to test the hypothesis that RyhB stimulates RseA through the direct interaction between complementary nucleotides, we designed putative compensatory nucleotide point mutations in the 5′UTR of PBAD-rseA27-lacZ translational fusions that were predicted to restore the ability of RyhB m1 or RyhB m3 mutants to stimulate PBAD-rseA27-lacZ activity (Figure 3C). The PBAD-rseA27(cm1)-lacZ translational fusion contains G153C G154C G155C nucleotide point mutants within the RseA 5′ UTR regions of the fusion, which were predicted to interact with the RyhB m1 allele (Figure 3C). The PBAD-rseA27 (cm3)-lacZ allele contains U139G U141G C142U U143G U144G C145G nucleotide point mutants within the RseA 5′ UTR regions of the fusion, which were predicted to interact with the RyhBm3 allele (Figure 3C). RyhB over-expression does not result in the stimulation of cm1 or cm3 mutants of the PBAD-rseA27-lacZ fusion (Figures 3A,B). However, the expression of the RyhBm1 mutant was able to stimulate the PBAD-rseA27(cm1)-lacZ fusion, approximately 2-fold in comparison to the vector control (Figure 3A). In addition, the RyhBm3 allele was able to stimulate the PBAD-rseA27(cm3)-lacZ fusion, approximately 2-fold in comparison to the vector control (Figure 3B). These results suggest that RyhB activates post-transcriptional expression of RseA because of a direct interaction between RyhB and the RseA 5′ UTR. Furthermore, this interaction requires RyhB C14 C15 C16 nucleotides and the RseA 5′ UTR G153 G154 G155 nucleotides, as well as RyhB nucleotides G21, G22, A23, G24, A25, A26 and RseA nucleotides U139, U141, C142, U143, U144, C145 (Figure 3A).
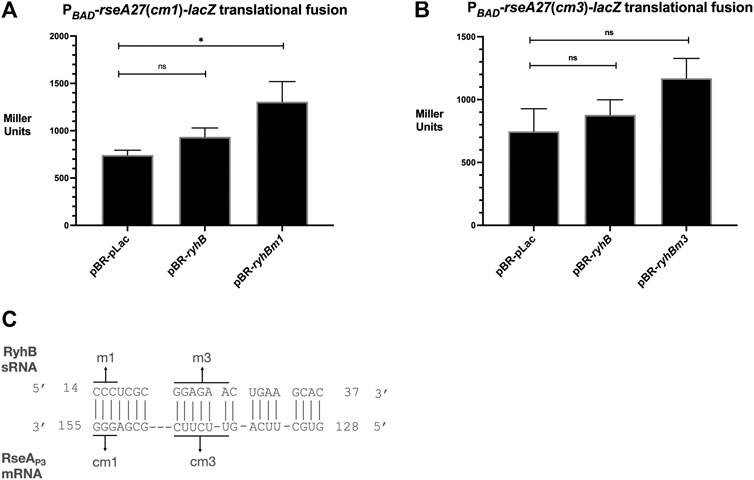
FIGURE 3. RyhB-RseA compensatory mutational analysis (A). The PBAD-rseA27cm1-lacZ translational fusion strain transformed with pBR-pLac, pBR-pLac-ryhB, or pBR-pLac-ryhBm1 was grown to the mid-log phase (OD600 of 0.5) in LB supplemented with ampicillin and arabinose to final concentrations of 100 μg/ml and 0.02%, respectively, and 100 μL aliquots were isolated for the β-galactosidase assay (B). The PBAD-rseA27cm3-lacZ translational fusion strain transformed with pBR-pLac, pBR-pLac-ryhB, or pBR-pLac-ryhBm3 was grown to the mid-log phase (OD600 of 0.5) in LB supplemented with ampicillin and arabinose to final concentrations of 100 μg/ml and 0.02%, respectively, and 100 μL aliquots were isolated for the β-galactosidase assay (C). Putative nucleotide interactions between RyhB and RseA tested with RyhB mutants and RseA compensatory mutants. All β-galactosidase assays were executed in triplicate and are represented as averages ± SEM. Statistical significance was assessed by one-way ANOVA with Dunnett’s post hoc test (*p < 0.05).
Optimal Post-Transcriptional Expression of RseAP3 is Inhibited by 5′ UTR Sequences
Since the RseAP3 transcript is stimulated by RyhB and FnrS and RseA mRNA stability is not affected by RyhB over-expression, it is logical to assume that translation is repressed in the absence of the stimulatory small RNAs. Since the compensatory mutants in the RseA 5′ UTR subdued the ability of RyhB to stimulate RseA translation, we hypothesized that the cm1 and cm3 mutations would result in increased post-transcriptional expression of RseA in the absence of stimulatory RNAs RyhB and FnrS (Figures 2, 3A,B). The 5′ UTR sequence of the RseAP3 transcript was analyzed using the RNAStructure program. The predicted secondary structure contains several hairpins that may affect translation efficiency and accessibility of the RseA Shine Delgado sequence (Figure 4A). To test the hypothesis that the RseA compensatory mutant alleles have higher expression than wild-type alleles, we measured the activity of wild-type, cm1, and cm3 alleles of the PBAD-rseA27-lacZ translational fusion in the mid-log phase in rich media (Figure 4B). Both cm1 and cm3 alleles had higher β-galactosidase activity than the wild-type fusion (Figure 4B). The activity of the PBAD-rseA27(cm3)-lacZ translational fusion was approximately 3-fold higher than the activity of the wild-type PBAD-rseA27-lacZ translational fusion (Figure 4B). The activity of the PBAD-rseA27 (cm3)-lacZ translational fusion was approximately 1.5–2-fold higher than the activity of the wild-type PBAD-rseA27-lacZ translational fusion (Figure 4B). We also created a chromosomal 3XFLAG epitope-tagged allele of rseA, driven by an arabinose inducible promoter, to measure post-transcriptional expression of the RseAP3 transcript in a manner that uncouples its synthesis from envelope stress (PBAD-rseA-3XFLAG at the lac locus–Figure 4C). We also created a cm1 allele of the PBAD-rseA-3XFLAG allele. This would allow us to determine if the RseA 5′ UTR mutations that increase PBAD-rseA27-lacZ activity correspond to increased RseA protein levels. We then grew (ryhB+ and ryhB−) wild-type and cm1 5′ UTR alleles of PBAD-rseA-3XFLAG in rich media supplemented with 0.002% arabinose to induce RseA-3XFLAG expression. At the mid-logarithmic phase, cultures were treated with 2,2-dipyridyl for 30 min to deplete iron and induce RyhB expression. We then isolated total protein and measured RseA-FLAG expression. RseA-FLAG levels were increased by at least 3-fold in the cm1 5′ UTR genetic background compared to the wild-type 5′ UTR genetic background (Figure 4D, lane 3 vs lane 1), consistent with our β-galactosidase assay results in Figure 4B. This further supports the idea that the wild-type sequence of the RseAP3 5′ UTR contains secondary structures that prevent optimal translation. Also, the absence of ryhB in the cm1 5′ UTR genetic background resulted in a 3-fold decrease in RseA-FLAG protein levels from cells grown in rich media without 2,2-dipyridyl supplementation (Figure 4D, lane 4 vs lane 3). This suggests that the cm1 mutation is not sufficient for complete inhibition of RyhB stimulation of RseA expression. Unexpectedly, we did not see this difference in RseA-FLAG protein levels isolated from cells grown in rich media supplemented with 2,2-dipyridyl. In addition, the absence of ryhB did not change RseA-FLAG protein levels in the wild-type 5’ UTR genetic background for cells grown in rich media without 2,2-dipyridyl, whereby RyhB levels are not expected to be repressed by Fur. (Figure 4D, lanes 1 and 2). Unexpectedly, for reasons that are not clear, the absence of ryhB had no noticeable effect on the stimulation of RseA-FLAG protein levels under iron starvation conditions (Figure 4D, lanes 5 and 6).
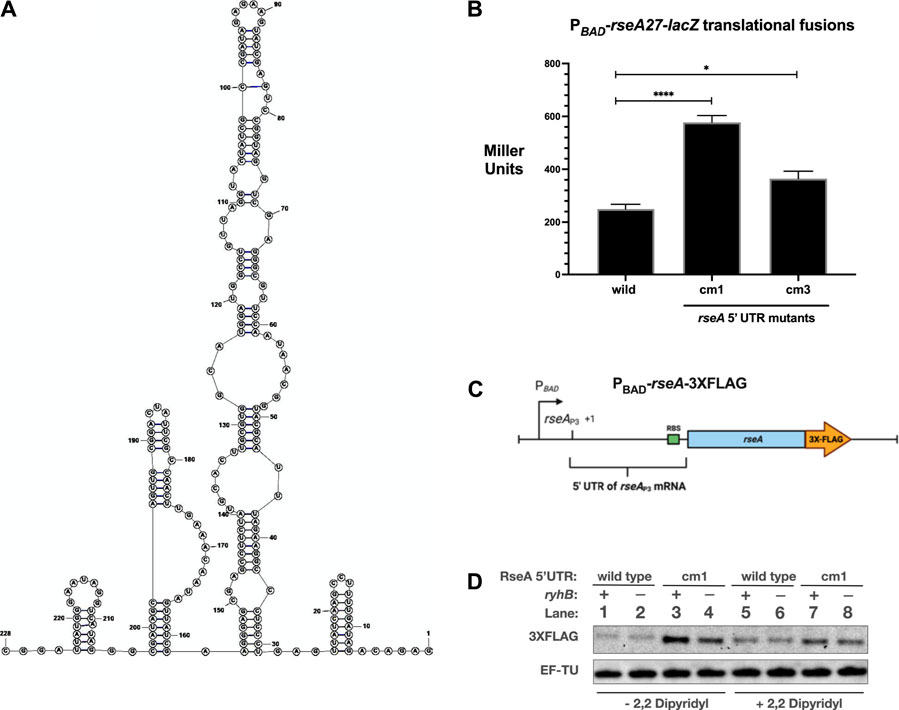
FIGURE 4. 5′ UTR mutations stimulate RseA translation (A). Predicted structure of RseAP3 5′ UTR using RNAStructure (B). The wild-type, cm1, and cm3 alleles of the PBAD-rseA27-lacZ translational fusion strains were grown in rich media to the mid-log phase (OD600 of 0.5), and aliquots were isolated for β-galactosidase activity (C). Schematic of the PBAD-rseA-3XFLAG allele utilized for the subsequent RseA-FLAG western blot (D). RseA-FLAG western blot. The wild-type and cm1 PBAD-rseA-3XFLAG strains were grown in LB supplemented with arabinose to a final concentration of 0.002% to the mid-log phase and then treated with 2,2-dipyridyl to a final concentration of 250 μM. Total proteins were isolated and subjected to western blot analysis using α-FLAG. All β-galactosidase assays were executed in triplicate and are represented as averages ± SEM. Statistical significance was assessed by one-way ANOVA with Dunnett’s post hoc test (*p < 0.05, ****p < 0.0001).
Discussion
Optimal levels of σE are critically important for a functional ESR. The absence of σE precludes the initiation of the ESR. Excess σE results in aberrant cell physiology and ultimately cell death (Nitta et al., 2000; Kabir et al., 2005). Fine tuning the synthesis and activity of σE is the major tool that the cell uses in order to achieve this goal. The rpoE operon includes several negative regulators of σE activity: rseA, rseB, and rseC, in addition to the rpoE leader peptide rseD (Klein et al., 2016). This particular operon has several promoters controlled by several transcriptional regulators and sigma factors, responding to a multitude of conditions (Klein et al., 2016). Since the negative regulators of σE are cistronic to rpoE, a negative feedback loop for σE activity is built into the synthesis of rpoE. The rseAP3 promoter drives the transcription of the rseA-rseB-rseC operon (Rhodius et al., 2006). Since this operon differs from the rpoE operon only in the absence of the rseD and rpoE genes, it suggests that secondary synthesis of the negative regulators of σE activity are necessary under conditions that are unique and separate from conditions driving the synthesis of the rpoE operon. The rseAP3 promoter and one of the several promoters of the rpoE operon are σE-dependent. This highlights the regulatory redundancy in the σE-dependent synthesis of RseA at the level of transcriptional initiation (Rhodius et al., 2006; Klein et al., 2016). This redundancy is likely reconciled through post-transcriptional regulation of rpoEP and rseAP3 transcripts via mechanisms described in this work and additional regulatory switches that are undiscovered (Yakhnin et al., 2017). Genetic analysis of the PBAD-rseA27-lacZ translational fusion, RseA-FLAG protein levels from a PBAD-rseA-3XFLAG epitope-tagged allele, suggests that the wild-type sequence of the RseAP3 5′ UTR prevents optimal RseA expression (Figure 3), presumably through RNA secondary structures that prevent efficient translation or promoting degradation of the RseAP3 transcript. This likely promotes tight control of RseA levels, specifically preventing excess of RseA levels in response to σE activity. However, the existence of the rseAP3 transcript driven by σE-dependent promoters suggests that under specific conditions in concern with the σE-dependent ESR, the cell requires the synthesis of additional RseA to prevent excess activity of σE, which is deleterious in nature. The presence of a relatively long 5′ UTR, with a secondary structure suboptimal for the promotion of post-transcriptional expression, is an ideal cognate partner for one or more small regulatory RNAs. While a direct interaction with RseA is necessary for the post-transcriptional regulatory effect of RyhB, its precise molecular mechanism is not clear but a part of an ongoing investigations in our lab. The difference in RyhB-dependent RseA-LacZ mRNA decay rates suggests that a complicated mechanism of action may occur. Possible pathways for the RyhB regulatory effect on RseA include modulation of translation initiation or transcription termination.
We identified RyhB and FnrS as small RNAs with positive regulatory effects on RseA synthesis using a targeted genetic screen of a small RNA library. FnrS expression is induced in response to oxygen limitation (Durand and Storz, 2010). RyhB is expressed under iron-limiting conditions upon de-activation of the Fur repressesor (Masse and Gottesman, 2002). RyhB was also previously identified as a binding partner for RseA using the RNA interaction by ligation and sequencing (RIL-seq) (Melamed et al., 2016). RyhB is known to repress post-transcriptional expression of a host of genes, including iron sulfur cluster proteins involved in the tricarboxylic acid cycles, in response to iron limitation (Masse and Gottesman, 2002; Masse et al., 2003; Semsey et al., 2006; Desnoyers and Masse, 2012; Wright et al., 2013). There is at least one positive regulatory target for RyhB, the shikimate permease gene ShiA (Prevost et al., 2007). FnrS has several regulatory targets, all of which are negative regulatory targets (Boysen et al., 2010; Durand and Storz, 2010; Wright et al., 2013). The observations in this work expand the RyhB regulon by adding a second positive regulatory target. This work also expands the FnrS regulon by uncovering the first positive regulatory target of FnrS. RyhB and FnrS share three unique targets, SodA, SodB, and MarA (Masse and Gottesman, 2002; Afonyushkin et al., 2005; Boysen et al., 2010; Durand and Storz, 2010; Argaman et al., 2012; Wright et al., 2013). In addition, both RyhB and FnrS have post-transcriptional regulatory targets in the iscR-iscS-iscU-iscA operon (Desnoyers et al., 2009; Wright et al., 2013). Our observation of both RyhB and FnrS regulating RseA is consistent with previously reported overlapping targets for RyhB and FnrS. RyhB and RseA are highly conserved in Gram-negative bacteria. It is possible that RyhB homologues or other small RNAs in these bacterial species regulate the post-transcriptional synthesis of RseA homologues.
For reasons that are not clear, there may be an increased requirement for RseA synthesis when iron, or oxygen, limitation occurs simultaneously with envelope stress. In our studies, we observed an increase in RseA-FLAG protein levels under iron-limiting conditions, implicating iron starvation in the post-transcriptional expression of the RseAP3 transcript. In the cm1 genetic background, RseA-FLAG protein levels decrease in the absence of RyhB (Figure 4D, lanes 3 vs 4). This supports our over-expression studies and implicate RyhB in the post-transcriptional stimulation of the RseAP3 expression. However, it is puzzling that the ryhB mutant did not prevent an increase in RseA levels under iron-limiting conditions (Figure 4D, Lanes 1/2 vs Lanes 5/6). It is possible that the change in RseA protein levels is transient in nature. Further investigated is needed to fully ascertain the physiological link between iron limitation, RyhB, and RseA expression. However, it is clear that both iron limitation and chromosomal RyhB levels affect post-transcriptional expression of the RseAP3 transcript. This also further suggests that excess σE activity may be especially deleterious to the cell under iron limitation. There have been some studies executed in E. coli and Vibrio sp. that support a link between envelope stress and iron limitation. Under iron-limiting conditions, suboptimal secretion of the siderophore enterobacterin results in the induction of the Cpx-dependent ESR (Guest et al., 2019). The Cpx-dependent ESR links iron sensing and adaptation in Vibrio cholerae (Acosta et al., 2015). The treatment of Vibrio vulnificus with the broad-spectrum antibiotic tropodithietic acid (TDA) simultaneously induced the expression of genes involved in cell envelope biogenesis, oxidative stress, and iron limitation (Dittman et al., 2019). Treatment of V. cholerae with polymyxin B results in induction of the σE-dependent stress response and iron metabolism changes (Sikora et al., 2009). From studies in these systems, there appears to be a link between the iron metabolism and envelope stress. The precise mechanisms linking the iron metabolism and envelope stress are the subject of ongoing investigations in our lab.
Data Availability Statement
The raw data supporting the conclusion of this article will be made available by the authors, without undue reservation.
Author Contributions
LL, JA, and JN executed experiments associated with data in the article and also contributed to the writing and editing of the article. KT created the hypotheses driving the experiments in the study, designed the experiments associated with the data in the article, and contributed to the writing and editing of the article.
Funding
This work was supported by a grant (SC2GM105419) from the National Institute of General Medical Sciences (NIGMS) of the National Institutes of Health (United States), Howard University College of Medicine Bridge Fund and Pilot Study Award, Howard University Medical Alumni Association Basic Science Chair Award, and the Small Equipment and Research Resource Award from the Howard University Office of the Provost to KT. In addition, this work was also supported by a grant (1011634) from the National Institute of Food and Agriculture (NIFA) of the United States Department of Agriculture (USDA) to LL.
Conflict of Interest
The authors declare that the research was conducted in the absence of any commercial or financial relationships that could be construed as a potential conflict of interest.
Publisher’s Note
All claims expressed in this article are solely those of the authors and do not necessarily represent those of their affiliated organizations, or those of the publisher, the editors, and the reviewers. Any product that may be evaluated in this article, or claim that may be made by its manufacturer, is not guaranteed or endorsed by the publisher.
Acknowledgments
We would like to thank members of the Thompson Lab for their comments and critique of this article. We would also like to thank Gisela Storz for generously donating plasmids pBR-fnrS-I, pBR-fnrS-II, and pBR-fnrS-III for this study.
References
Acosta, N., Pukatzki, S., and Raivio, T. L. (2015). The Vibrio cholerae Cpx Envelope Stress Response Senses and Mediates Adaptation to Low Iron. J. Bacteriol. 197, 262–276. doi:10.1128/jb.01957-14
Ades, S. E., Connolly, L. E., Alba, B. M., and Gross, C. A. (1999). The Escherichia coli Sigma E-Dependent Extracytoplasmic Stress Response Is Controlled by the Regulated Proteolysis of an Anti-Sigma Factor. Genes Develop. 13, 2449–2461. doi:10.1101/gad.13.18.2449
Afonyushkin, T., Vecerek, B., Moll, I., Blasi, U., and Kaberdin, V. R. (2005). Both RNase E and RNase III Control the Stability of sodB mRNA upon Translational Inhibition by the Small Regulatory RNA RyhB. Nucleic Acids Res. 33, 1678–1689. doi:10.1093/nar/gki313
Aiba, H., Adhya, S., and De Crombrugghe, B. (1981). Evidence for Two Functional Gal Promoters in Intact Escherichia coli Cells. J. Biol. Chem. 256, 11905–11910. doi:10.1016/s0021-9258(19)68491-7
Alba, B. M., Leeds, J. A., Onufryk, C., Lu, C. Z., and Gross, C. A. (2002). DegS and YaeL Participate Sequentially in the Cleavage of RseA to Activate the Sigma E-Dependent Extracytoplasmic Stress Response. Genes Dev. 16, 2156–2168. doi:10.1101/gad.1008902
Argaman, L., Elgrably-Weiss, M., Hershko, T., Vogel, J., and Altuvia, S. (2012). RelA Protein Stimulates the Activity of RyhB Small RNA by Acting on RNA-Binding Protein Hfq. Proc. Natl. Acad. Sci. 109, 4621–4626. doi:10.1073/pnas.1113113109
Balbontín, R., Fiorini, F., Figueroa-Bossi, N., Casadesús, J., and Bossi, L. (2010). Recognition of Heptameric Seed Sequence Underlies Multi-Target Regulation by RybB Small RNA in Salmonella enterica. Mol. Microbiol. 78, 380–394. doi:10.1111/j.1365-2958.2010.07342.x
Boysen, A., Møller-Jensen, J., Kallipolitis, B., Valentin-Hansen, P., and Overgaard, M. (2010). Translational Regulation of Gene Expression by an Anaerobically Induced Small Non-Coding RNA in Escherichia coli. J. Biol. Chem. 285, 10690–10702. doi:10.1074/jbc.m109.089755
Campbell, E. A., Tupy, J. L., Gruber, T. M., Wang, S., Sharp, M. M., Gross, C. A., et al. (2003). Crystal Structure of Escherichia coli σE with the Cytoplasmic Domain of its Anti-σ RseA. Mol. Cel 11, 1067–1078. doi:10.1016/s1097-2765(03)00148-5
Chen, J., Morita, T., and Gottesman, S. (2019). Regulation of Transcription Termination of Small RNAs and by Small RNAs: Molecular Mechanisms and Biological Functions. Front Cel Infect Microbiol 9, 201. doi:10.3389/fcimb.2019.00201
Chung, C. T., Niemela, S. L., and Miller, R. H. (1989). One-step Preparation of Competent Escherichia coli: Transformation and Storage of Bacterial Cells in the Same Solution. Proc. Natl. Acad. Sci. 86, 2172–2175. doi:10.1073/pnas.86.7.2172
Costanzo, A., and Ades, S. E. (2006). Growth Phase-Dependent Regulation of the Extracytoplasmic Stress Factor, σ E , by Guanosine 3′,5′-Bispyrophosphate (ppGpp). J. Bacteriol. 188, 4627–4634. doi:10.1128/jb.01981-05
Costanzo, A., Nicoloff, H., Barchinger, S. E., Banta, A. B., Gourse, R. L., and Ades, S. E. (2008). ppGpp and DksA Likely Regulate the Activity of the Extracytoplasmic Stress Factor σEinEscherichia Coliby Both Direct and Indirect Mechanisms. Mol. Microbiol. 67, 619–632. doi:10.1111/j.1365-2958.2007.06072.x
Dartigalongue, C., Missiakas, D., and Raina, S. (2001). Characterization of the Escherichia coliςE Regulon. J. Biol. Chem. 276, 20866–20875. doi:10.1074/jbc.m100464200
De Las Penans, A., Connolly, L., and Gross, C. A. (1997). The sE-Mediated Response to Extracytoplasmic Stress in Escherichia coli Is Transduced by RseA and RseB, Two Negative Regulators of sE. Mol. Microbiol. 24, 373–385.
Desnoyers, G., and Masse, E. (2012). Noncanonical Repression of Translation Initiation through Small RNA Recruitment of the RNA Chaperone Hfq. Genes Develop. 26, 726–739. doi:10.1101/gad.182493.111
Desnoyers, G., Morissette, A., Prévost, K., and Massé, E. (2009). Small RNA-Induced Differential Degradation of the Polycistronic mRNA iscRSUA. EMBO J. 28, 1551–1561. doi:10.1038/emboj.2009.116
Dittmann, K. K., Porsby, C. H., Goncalves, P., Mateiu, R. V., Sonnenschein, E. C., Bentzon‐Tilia, M., et al. (2019). Tropodithietic Acid Induces Oxidative Stress Response, Cell Envelope Biogenesis and Iron Uptake in Vibrio Vulnificus. Environ. Microbiol. Rep. 11, 581–588. doi:10.1111/1758-2229.12771
Douchin, V., Bohn, C., and Bouloc, P. (2006). Down-Regulation of Porins by a Small RNA Bypasses the Essentiality of the Regulated Intramembrane Proteolysis Protease RseP in Escherichia coli. J. Biol. Chem. 281, 12253–12259. doi:10.1074/jbc.m600819200
Durand, S., and Storz, G. (2010). Reprogramming of Anaerobic Metabolism by the FnrS Small RNA. Mol. Microbiol. 75, 1215–1231. doi:10.1111/j.1365-2958.2010.07044.x
Erickson, J. W., Vaughn, V., Walter, W. A., Neidhardt, F. C., and Gross, C. A. (1987). Regulation of the Promoters and Transcripts of rpoH, the Escherichia coli Heat Shock Regulatory Gene. Genes Develop. 1, 419–432. doi:10.1101/gad.1.5.419
Ezemaduka, A. N., Yu, J., Shi, X., Zhang, K., Yin, C.-C., Fu, X., et al. (2014). A Small Heat Shock Protein Enables Escherichia coli to Grow at a Lethal Temperature of 50 C Conceivably by Maintaining Cell Envelope Integrity. J. Bacteriol. 196, 2004–2011. doi:10.1128/jb.01473-14
Flynn, J. M., Levchenko, I., Sauer, R. T., and Baker, T. A. (2004). Modulating Substrate Choice: The SspB Adaptor Delivers a Regulator of the Extracytoplasmic-Stress Response to the AAA+ Protease ClpXP for Degradation. Genes Develop. 18, 2292–2301. doi:10.1101/gad.1240104
Guest, R. L., Court, E. A., Waldon, J. L., Schock, K. A., and Raivio, T. L. (2019). Impaired Efflux of the Siderophore Enterobactin Induces Envelope Stress in Escherichia coli. Front. Microbiol. 10, 2776. doi:10.3389/fmicb.2019.02776
Johansen, J., Rasmussen, A. A., Overgaard, M., and Valentin-Hansen, P. (2006). Conserved Small Non-Coding RNAs that Belong to the σE Regulon: Role in Down-Regulation of Outer Membrane Proteins. J. Mol. Biol. 364, 1–8. doi:10.1016/j.jmb.2006.09.004
Kabir, M. S., Yamashita, D., Koyama, S., Oshima, T., Kurokawa, K., Maeda, M., et al. (2005). Cell Lysis Directed by σ E in Early Stationary Phase and Effect of Induction of the rpoE Gene on Global Gene Expression in Escherichia coli. Microbiology 151, 2721–2735. doi:10.1099/mic.0.28004-0
Kanehara, K., Ito, K., and Akiyama, Y. (2002). YaeL (EcfE) Activates the Sigma E Pathway of Stress Response through a Site-2 Cleavage of Anti-Sigma E, RseA. Genes Dev. 16, 2147–2155. doi:10.1101/gad.1002302
Klein, G., Lindner, B., Brade, H., and Raina, S. (2011). Molecular Basis of Lipopolysaccharide Heterogeneity in Escherichia coli. J. Biol. Chem. 286, 42787–42807. doi:10.1074/jbc.m111.291799
Klein, G., and Raina, S. (2017). Small Regulatory Bacterial RNAs Regulating the Envelope Stress Response. Biochem. Soc. Trans. 45, 417–425. doi:10.1042/bst20160367
Klein, G., Stupak, A., Biernacka, D., Wojtkiewicz, P., Lindner, B., and Raina, S. (2016). Multiple Transcriptional Factors Regulate Transcription of the rpoE Gene in Escherichia coli under Different Growth Conditions and when the Lipopolysaccharide Biosynthesis Is Defective. J. Biol. Chem. 291, 22999–23019. doi:10.1074/jbc.m116.748954
Mandin, P., and Gottesman, S. (2009). A Genetic Approach for Finding Small RNAs Regulators of Genes of Interest Identifies RybC as Regulating the DpiA/DpiB Two-Component System. Mol. Microbiol. 72, 551–565. doi:10.1111/j.1365-2958.2009.06665.x
Masse, E., Escorcia, F. E., and Gottesman, S. (2003). Coupled Degradation of a Small Regulatory RNA and its mRNA Targets in Escherichia coli. Genes Develop. 17, 2374–2383. doi:10.1101/gad.1127103
Masse, E., and Gottesman, S. (2002). A Small RNA Regulates the Expression of Genes Involved in Iron Metabolism in Escherichia coli. Proc. Natl. Acad. Sci. 99, 4620–4625. doi:10.1073/pnas.032066599
Mecsas, J., Rouviere, P. E., Erickson, J. W., Donohue, T. J., and Gross, C. A. (1993). The Activity of Sigma E, an Escherichia coli Heat-Inducible Sigma-Factor, Is Modulated by Expression of Outer Membrane Proteins. Genes Dev. 7, 2618–2628. doi:10.1101/gad.7.12b.2618
Melamed, S., Peer, A., Faigenbaum-Romm, R., Gatt, Y. E., Reiss, N., Bar, A., et al. (2016). Global Mapping of Small RNA-Target Interactions in Bacteria. Mol. Cel 63, 884–897. doi:10.1016/j.molcel.2016.07.026
Miller, J. H. (1992). A Short Course in Bacterial Genetics. Plainview, N. Y.: Cold Spring Harbor Laboratory Press.
Missiakas, D., Mayer, M. P., Lemaire, M., Georgopoulos, C., and Raina, S. (1997). Modulation of the Escherichia coli σ E (RpoE) Heat‐Shock Transcription‐Factor Activity by the RseA, RseB and RseC Proteins. Mol. Microbiol. 24, 355–371. doi:10.1046/j.1365-2958.1997.3601713.x
Nitta, T., Nagamitsu, H., Murata, M., Izu, H., and Yamada, M. (2000). Function of the ς E Regulon in Dead-Cell Lysis in Stationary-Phase Escherichia coli. J. Bacteriol. 182, 5231–5237. doi:10.1128/jb.182.18.5231-5237.2000
Prévost, K., Salvail, H., Desnoyers, G., Jacques, J.-F., Phaneuf, É., and Massé, E. (2007). The Small RNA RyhB Activates the Translation of shiA mRNA Encoding a Permease of Shikimate, a Compound Involved in Siderophore Synthesis. Mol. Microbiol. 64, 1260–1273. doi:10.1111/j.1365-2958.2007.05733.x
Ravio, T. L., Popkin, D. L., and Silhavy, T. J. (1999). The Cpx Envelope Stress Response Is Controlled by Amplification and Feedback Inhibition. J. Bacteriol. 18, 5263–5272.
Ravio, T. L. (1999). The sE and Cpx Regulatory Pathways: Overlapping but Distinct Envelope Stress Responses. Curr. Opin. Microbiol. 2, 159–165.
Rhodius, V. A., Suh, W. C., Nonaka, G., West, J., and Gross, C. A. (2006). Conserved and Variable Functions of the SigmaE Stress Response in Related Genomes. Plos Biol. 4, e2. doi:10.1371/journal.pbio.0040002
Semsey, S., Andersson, A. M. C., Krishna, S., Jensen, M. H., Massé, E., and Sneppen, K. (2006). Genetic Regulation of Fluxes: Iron Homeostasis of Escherichia coli. Nucleic Acids Res. 34, 4960–4967. doi:10.1093/nar/gkl627
Sikora, A. E., Beyhan, S., Bagdasarian, M., Yildiz, F. H., and Sandkvist, M. (2009). Cell Envelope Perturbation Induces Oxidative Stress and Changes in Iron Homeostasis in Vibrio cholerae. J. Bacteriol. 191, 5398–5408. doi:10.1128/jb.00092-09
Thompson, K. M., Rhodius, V. A., and Gottesman, S. (2007). σ E Regulates and Is Regulated by a Small RNA in Escherichia coli. J. Bacteriol. 189, 4243–4256. doi:10.1128/jb.00020-07
Udekwu, K. I., and Wagner, E. G. (2007). Sigma E Controls Biogenesis of the Antisense RNA MicA. Nucleic Acids Res. 35, 1279–1288. doi:10.1093/nar/gkl1154
Vogt, S. L., Evans, A. D., Guest, R. L., and Raivio, T. L. (2014). The Cpx Envelope Stress Response Regulates and Is Regulated by Small Noncoding RNAs. J. Bacteriol. 196, 4229–4238. doi:10.1128/jb.02138-14
Walsh, N. P., Alba, B. M., Bose, B., Gross, C. A., and Sauer, R. T. (2003). OMP Peptide Signals Initiate the Envelope-Stress Response by Activating DegS Protease via Relief of Inhibition Mediated by its PDZ Domain. Cell 113, 61–71. doi:10.1016/s0092-8674(03)00203-4
Wright, P. R., Richter, A. S., Papenfort, K., Mann, M., Vogel, J., Hess, W. R., et al. (2013). Comparative Genomics Boosts Target Prediction for Bacterial Small RNAs. Proc. Natl. Acad. Sci. 110, E3487–E3496. doi:10.1073/pnas.1303248110
Yakhnin, H., Aichele, R., Ades, S. E., Romeo, T., and Babitzke, P. (2017). Circuitry Linking the Global Csr- and σE-Dependent Cell Envelope Stress Response Systems. J. Bacteriol. 199, e00484–00417. doi:10.1128/JB.00484-17
Keywords: small RNA, RyhB, FnrS, Escherichia coli, envelope stress response
Citation: London LY, Aubee JI, Nurse J and Thompson KM (2021) Post-Transcriptional Regulation of RseA by Small RNAs RyhB and FnrS in Escherichia coli. Front. Mol. Biosci. 8:668613. doi: 10.3389/fmolb.2021.668613
Received: 16 February 2021; Accepted: 03 September 2021;
Published: 03 November 2021.
Edited by:
Olga N. Ozoline, Institute of Cell Biophysics (RAS), RussiaReviewed by:
Satish Raina, Gdansk University of Technology, PolandTracy Raivio, University of Alberta, Canada
Andrey Shadrin, Institute of Biochemistry and Physiology of Microorganisms (RAS), Russia
Copyright © 2021 London, Aubee, Nurse and Thompson. This is an open-access article distributed under the terms of the Creative Commons Attribution License (CC BY). The use, distribution or reproduction in other forums is permitted, provided the original author(s) and the copyright owner(s) are credited and that the original publication in this journal is cited, in accordance with accepted academic practice. No use, distribution or reproduction is permitted which does not comply with these terms.
*Correspondence: Karl M Thompson, a2FybC50aG9tcHNvbkBob3dhcmQuZWR1