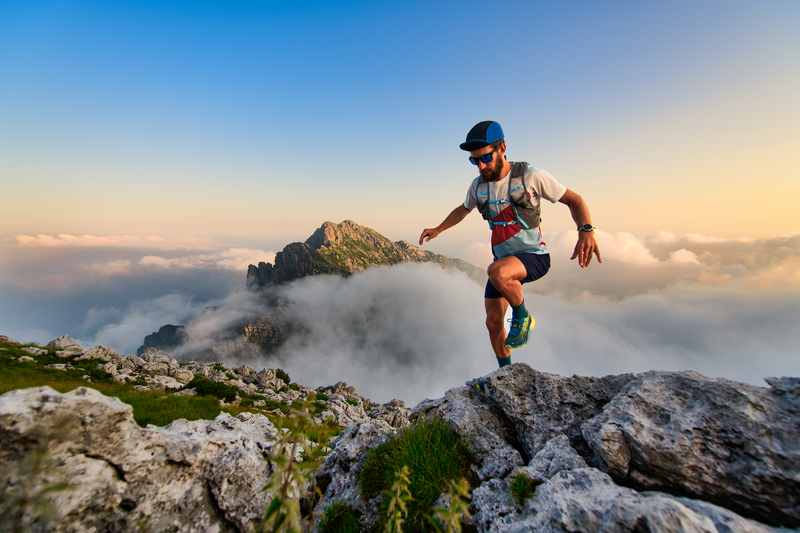
95% of researchers rate our articles as excellent or good
Learn more about the work of our research integrity team to safeguard the quality of each article we publish.
Find out more
MINI REVIEW article
Front. Mol. Biosci. , 08 April 2021
Sec. RNA Networks and Biology
Volume 8 - 2021 | https://doi.org/10.3389/fmolb.2021.658020
This article is part of the Research Topic The Role of Protein Post-Translational Modifications in Protein-RNA Interactions and RNP Assemblies View all 10 articles
RNA-binding proteins undergo regulated phase transitions in an array of cell types. The phase separation of RNA-binding proteins, and subsequent formation of RNP condensates or granules, occurs during physiological conditions and can also be induced by stress. Some RNP granules have roles in post-transcriptionally regulating mRNAs, and mutations that prevent the condensation of RNA-binding proteins can reduce an organism’s fitness. The reversible and multivalent interactions among RNP granule components can result in RNP complexes that transition among diffuse and condensed states, the latter of which can be pathological; for example, in neurons solid RNP aggregates contribute to disease states such as amyotrophic lateral sclerosis (ALS), and the dysregulation of RNP granules in human germ cells may be involved in Fragile X-associated primary ovarian insufficiency. Thus, regulating the assembly of mRNAs and RNA-binding proteins into discrete granules appears to provide important functions at both cellular and physiological levels. Here we review our current understanding of the role of post-translational modifications (PTMs) in regulating the condensation of RNA-binding proteins in the germ line. We compare and contrast the in vitro evidence that methylation inhibits phase separation of RNA binding proteins, with the extent to which these results apply to the in vivo germ line environment of several model systems. We also focus on the role of phosphorylation in modulating the dynamics of RNP granules in the germ line. Finally, we consider the gaps that exist in our understanding of the role of PTMs in regulating germ line RNP granules.
Phase separation is an important principle of cellular organization. Many types of membraneless organelles (MLOs) assemble through the process of liquid-liquid phase separation. Some of the best studied MLOs in the cytoplasm are ribonucleoprotein (RNP) granules composed of RNA and RNA binding proteins, such as stress granules and processing bodies. Much of our understanding of phase separation to date has come from in vitro studies. From such studies, we now understand that phase separation is driven mainly by weak interactions between multivalent protein interaction domains or intrinsically disordered low complexity domains (LCDs) (Kato et al., 2012; Li et al., 2012). Since multivalent interaction motifs and the short linear motifs in intrinsically disordered regions and LCDs are often post-translationally modified (Xie et al., 2007; Bah and Forman-Kay, 2016; Chong and Forman-Kay, 2016), in hindsight it is not surprising that post-translational modifications (PTMs) have been revealed as important regulators of phase separation (Itakura et al., 2018; Rhoads et al., 2018). While a diverse array of PTMs can modulate condensates, in this review we focus on the best-studied paradigms: methylation and phosphorylation.
PTMs can alter the chemical properties of amino acids, such as the steric properties, bulkiness, or charge state. For example, when Arginine (Arg) is methylated, bulkiness is increased, and the distribution of charge and hydrophobicity is altered which affects intermolecular interactions and phase separation. Phosphorylation of Tyr or Ser introduces a negative charge which can either promote or inhibit phase separation (Monahan et al., 2017; Wang et al., 2018); thus, PTMs can weaken or enhance multivalent interactions between phase-separated macromolecules. PTMs can also recruit protein into, or exclude protein from, the condensate (Hofweber and Dormann, 2019). Thus, PTMs can modulate the assembly and disassembly of liquid-like RNP granules, and transitions from the liquid state to gel- or solid-like states.
Condensation of RNA binding proteins and RNA in the germ line of many organisms results in germ granules (Voronina et al., 2011). A variety of terms are used to describe the array of germ granules found across different species which can be confusing but are described in several resources (Table 1 and Schisa, 2012). While some germ granule proteins exhibit liquid-like properties, such as the PGL-1 granules in C. elegans embryos (Brangwynne et al., 2009), other types of germ granules such as the Balbiani body in Xenopus oocytes have solid-like properties (Boke et al., 2016; Woodruff et al., 2018). Careful examination has revealed multiple phases within germ granules; for example, the germ granules of early C. elegans embryos include a liquid-like phase of PGL proteins, and a gel phase of MEG-3 protein that appears to have a scaffolding role in the assembly of germ granules (Putnam et al., 2019). An increasing number of examples of PTMs modulating the assembly of germ granules have been documented over the past two decades. Building upon our understanding from in vitro studies, these in vivo experiments are revealing both conserved and complex roles of PTMs in regulating condensates of RNA binding proteins in the germ line that are associated with critical germ line functions. This review will focus on our understanding of how methylation and phosphorylation regulate germ line RNP condensates across invertebrate and vertebrate model systems.
Methylation is a key regulator of phase transitions and RNP granule dynamics. Within many RNP granules are proteins with RGG or RG-rich motifs, and the Arginine residues in these motifs are often methylated by protein arginine methyltransferase (PRMT) enzymes (Bedford and Clarke, 2009). In general, Arg-methylation is considered a less dynamic modification, in how it impacts target proteins, than others such as phosphorylation and acetylation (Fackelmayer, 2005). In in vitro studies methylation of Arg weakens intermolecular interactions and thus inhibits phase separation of RNA binding proteins. For example, droplets of the N-terminal RGG-rich domain of the conserved nuage protein Ddx4/Vasa are destabilized by Arg-methylation (Nott et al., 2015). Methylation of recombinant or purified FUS protein, the protein that phase separates into granules in amyotrophic lateral sclerosis (ALS) mutations, similarly reduces liquid-liquid de-mixing (Qamar et al., 2018; Hofweber and Dormann, 2019). Since no examples have shown Arg-methylation to promote condensation in vitro, it has been suggested that this PTM is a general inhibitor of Arg-aromatic (π) interactions that reduces phase separations (Hofweber and Dormann, 2019). However, ex vivo studies reveal a more complex effect of methylation on phase separation (Figure 1A). Some experiments align with in vitro results showing Arg-methylation suppresses RNP granule formation. Treatments of cultured U2OS cells that increase the methylation of Ras-GAP SH3-binding protein (G3BP1) repress the assembly of stress granules (Tsai et al., 2016). However, there is also evidence for Arg-methylation promoting RNP granule assembly. When methylation of the Lsm4 protein, RAP55A, is decreased, the localization of RAP55A to P bodies is inhibited in cell culture (Matsumoto et al., 2012), and similarly, recruitment of unmethylated CIRP to stress granules is blocked (De Leeuw et al., 2007). Overall, many in vitro and ex vivo examples highlight a role for Arg-methylation in controlling the dynamics of RNP granules; however, these results do not address the extent to which this regulation occurs in vivo or in the germ line. To date, studies in three model systems all demonstrate a role for Arg-methylation in promoting phase separation of RNA binding proteins in the germ line, opposite of the role seen in vitro (Figure 1B).
Figure 1. Methylation promotes condensate assembly in the germ line. CH3 indicates the methylated form of the corresponding protein. Arrows indicate whether methylation promotes or inhibits a condensed state. (A) Methylation suppresses condensates in in vitro studies, but can sometimes promote condensation in cell culture. (B) Methylation promotes condensation of germ line proteins. Effects of methylation are shown for six distinct germ line RNP granules in three model systems.
In Drosophila, components of the methylosome regulate RNA binding proteins in multiple MLOs of the female germ line (Figure 1B). Capsuleen (Csul), also known as Dart5, is the homolog of the methyltransferase PRMT5. In dart5/csul mutant egg chambers the condensation of Tudor, Vasa, and Maelstrom into perinuclear granules of the nurse cell nuage is diminished, suggesting methylation normally promotes condensation of these proteins in the Drosophila female germ line (Gonsalvez et al., 2006; Anne et al., 2007). The Capsuleen-Valois methylosome complex also has a role in assembly of the pole plasm of Drosophila oocytes. The localization of Tudor and the Sm proteins, SmB and SD3, to the posterior pole plasm requires the methylation of Arg residues (Anne et al., 2007; Anne, 2010). The consequence of blocked methylation and disrupted nuage and pole plasm assembly in dart5/csul mutants is a grandchildless phenotype, where embryos of mutant females completely lack pole cells and develop into agametic, sterile adults (Gonsalvez et al., 2006; Anne et al., 2007). Csul also methylates the Piwi protein Aubergine (Aub), which is required for Aub to bind Tudor and to promote the assembly of pole plasm in the developing oocyte (Kirino et al., 2010).
A role for methylation has also been identified in Drosophila primary spermatocytes, where a novel condensate called the piRNA nuage giant body (piNG-body) is enriched for Vasa, Aub, Argonaute 3, and Tudor (Kibanov et al., 2011). In spermatocytes lacking Csul/PRMT5, unmethylated Vasa and Aub fail to condense into the piNG-body; only small Vasa-positive nuage granules, and unlocalized Aub signals are detected. At the same time, the piRNA pathway is disrupted, and male sterility occurs. Thus, methylation by PRMT5 appears to be essential for piNG-body assembly and normal development of the male germ line.
Methylation of Ddx4/Vasa is widely conserved from planar worms to humans (Rouhana et al., 2012); thus, it will be interesting to determine if Arg-methylation also promotes assembly of Vasa granules in systems beyond Drosophila. It is notable that these in vivo germ line studies show an opposite effect of methylation as compared to in vitro studies, where PRMT1-dependent methylation disrupts the phase separation of Ddx4 (Nott et al., 2015). This difference seems likely to be due to the fact in vitro studies generally involve only one or a few purified RBPs while the in vivo environment is much more complex.
Piwi-tudor domain protein interactions promote the assembly of germ granules not only in Drosophila, but also in mouse (Arkov and Ramos, 2010). In vitro studies show that Tudor proteins recognize methylarginine marks on mouse Piwi proteins to drive their localization to cytoplasmic foci (Vagin et al., 2009). In cell culture, treatment with an inhibitor of methyltransferases abolishes interactions between Tdrd1 and the mouse Piwi protein, MILI, suggesting that Arg-methylation and Tudor binding promote assembly of piRNA pathway components into nuage (Vagin et al., 2009). Moreover, immunoprecipitation studies show Tdrd6 interacts with the mouse Piwi proteins Miwi and Mili in vivo, and Miwi is methylated by PRMT5 and binds Tdrd6 in a symmetrical dimethylarginine methylation (sDMA)-dependent manner (Vasileva et al., 2009; Kirino et al., 2010). In tdrd6-/- testes the RNA binding proteins Mael, Miwi, and Mouse Vasa homolog (MVH)/Ddx4 fail to condense into the normal chromatoid bodies (the nuage in mouse spermatogenic cells) (Vasileva et al., 2009). The defects in condensation are accompanied by a lack of elongated spermatids and sperm. Given the proposed role for chromatoid bodies as storage sites during spermatid differentiation, their aberrant architecture and absence of condensed Mael, Miwi, and MVH in chromatoid bodies may directly impact spermatid differentiation (Vasileva et al., 2009). PRMT5 also methylates the SmB splicing protein in mouse spermatocytes. When Arg-methylation is abrogated via mutation of Tdrd6, the assembly of spliceosomes is impaired in primary spermatocytes, resulting in a decreased number of Cajal bodies, the nuclear membraneless condensates where spliceosome maturation occurs (Akpınar et al., 2017). Thus, methylation promotes condensation in both cytoplasmic and nuclear compartments of the mouse male germ line.
In the zebrafish model system methylation appears to promote the condensation of a solid-like germ granule, the Balbiani body. The germ plasm in early embryos originates from the Balbiani body in the oocyte (Kloc et al., 2004). A role for Tudor6 (Tdrd6) has been shown in modulating the aggregation of Buckyball (Buc), the organizer of the Balbiani body (Roovers et al., 2018). Tdrd6a and Tdrd6c interact with Buc via its three symmetrically dimethylated arginines. The three arginines of Buc are required for Buc to condense into a mature Balbiani body in oocytes, and to form germ plasm in embryos. The importance of Arg-methylation in promoting this phase transition to a solid condensate is further underscored by the observation that deleting the three arginines of Buc has a more severe phenotype than a tdrd6a mutant, with defects in germ cell formation and embryonic development (Roovers et al., 2018). It will be interesting to determine if methylation also modulates condensation of germ granule proteins in the adult germ cells or embryonic primordial germ cells where Tdrd7 has a role in maintaining the integrity of the germ granule protein Vasa (Strasser et al., 2008). The recent discovery of a role for PRMT5 in the methylation of Zili and Vasa in the zebrafish gonad should allow researchers to address whether methylation has any role in modulating condensation of Zili, Vasa, or other granule components (Zhu et al., 2019).
No studies to date have identified regulation of RNP condensates by methylation in the C. elegans germ line. However, Arg-methylation of the C. elegans RG proteins PGL-1/-3 by the PRMT1 homolog EPG-11 results in decreased phase separation in vitro (Zhang et al., 2018). In addition, in C. elegans embryos, where the primordial germ cells localize PGL-1/-3 to germ granules, EPG-11 destabilizes PGL-1/-3 aggregates from somatic blastomeres via methylation of the PGL-1/-3 RGG repeats (Li et al., 2013). It remains to be determined if this example of an inhibition of condensation by methylation will be extended to the worm germ line in future studies. In any event, the experiments in the fly, fish, and mouse germ lines clearly indicate differences from how methylation modulates phase separation in vitro and highlight the necessity of additional in vivo studies. Biochemical approaches to further study Ddx4/Vasa may be especially valuable due its broad conservation. Employing a high-resolution mass spectrometry approach to profile PRMT substrates may also be useful, as has been successful in other contexts (Shishkova et al., 2017).
Phosphorylation is a common PTM that is implicated in the regulation of RNP granule dynamics. Phosphorylation is a rapid and reversible process by which proteins acquire negatively charged PO4 groups that alter their intramolecular interactions and consequently impact phase separation (Hofweber and Dormann, 2019). Multivalent interactions among serine and tyrosine residues are especially prominent in the LCDs and intrinsically disordered regions (IDRs) of RNA binding proteins in granules. In in vitro studies phosphorylation sometimes promotes, and other times suppresses, phase separation of RNA binding proteins (Hofweber and Dormann, 2019). For example, phase separation of FUS is blocked when FUS is phosphorylated by the DNA-dependent protein kinase (Monahan et al., 2017; Murray et al., 2017; Luo et al., 2018). In contrast, phosphomimetic S48E substitution in the N-terminal domain of TDP-43 (TAR DNA– binding protein of 43 kDa) blocks the phosphorylation of TDP-43 by Casein Kinase 1 (CK1), and leads to reduced liquid-liquid phase separation of TDP-43 in vitro (Kametani et al., 2009; Wang et al., 2018; Hofweber and Dormann, 2019). Phosphorylation also promotes the condensation of Tau, a neuron-specific microtubule-associated protein (Ambadipudi et al., 2017). Similar to the varied effects of phosphorylation in in vitro studies (Figure 2A), cell culture experiments also reveal both inhibitory and stimulatory roles of phosphorylation on condensation of stress granule proteins (Hofweber and Dormann, 2019). It is not yet clear how well the in vitro and ex vivo studies translate to more complex environments of in vivo tissues and organisms. However, the studies highlighted below elucidate a growing role for phosphorylation in regulating the assembly and disassembly of germ granules in invertebrate and vertebrate systems.
Figure 2. Phosphorylation can suppress or promote condensate assembly. The red P indicates the phosphorylated form of the corresponding protein. Arrows indicate whether phosphorylation drives a dispersed or condensed state; kinase names are above each arrow. (A) Phosphorylation has varying effects on phase separation in in vitro studies. (B) Phosphorylation negatively and positively regulates RNP condensates in the germ line. The effects of phosphorylation are shown for proteins in six distinct germ line RNP granules in three model systems. Note: phosphorylation of the C. elegans P granule protein PGL-1 during heat stress conditions promotes condensates in somatic cells, outside of the germline.
Studies of C. elegans germ cells reveal insights into the complex roles of phosphorylation in regulating RNP granule assembly (Figure 2B). MBK-2, the C. elegans DYRK3 kinase homolog, and the PP2APPTR–1/2 phosphatase are key players in mediating the dynamics of PGL-1, an RGG protein in germ granules (Seydoux, 2018). Phosphorylation of MEG-1 and MEG-3 (maternal-effect germline defective) proteins by MBK-2 promotes disassembly of PGL-1 granules in zygotes (Wippich et al., 2013). This action is balanced by PPTR-1 and PPTR-2 which have redundant phosphatase functions to dephosphorylate MEG proteins and stabilize PGL-1 granule formation (Wang et al., 2014). In addition to contributing to P granule regulation, the MEG proteins are required for fertility; however, how regulated phosphorylation impacts fertility is not yet clear (Wang et al., 2014). A contrasting example of phosphorylation in C. elegans is the Ddx4/Vasa homolog GLH-1 which is phosphorylated by the KGB-1 MAP kinase (Orsborn et al., 2007). In the kgb-1 knockout the localization of GLH-1 to discrete P granules is partially disrupted, suggesting that KGB-1 normally promotes condensation of GLH-1 via phosphorylation. When GLH-1 is not phosphorylated by KGB-1, elevated levels of GLH-1 protein are detected in the gonad, as well as over-proliferation of germ cells, and a high level of sterility. A second example of phosphorylation promoting condensation of P granules has been described outside of germ cells. mTORC1-mediated phosphorylation of PGL-1/-3 during mild heat-stress promotes the assembly of PGL-1/-3 granules in somatic blastomeres of early embryos (Zhang et al., 2018).
In the Drosophila germ line, phosphorylation has been shown to inhibit protein condensation. Orb, the cytoplasmic polyadenylation element binding protein (CPEB) homolog, regulates the translation of target mRNAs in Drosophila ovaries and is phosphorylated by casein kinase II (ck2) (Wong et al., 2011). When ck2 activity is compromised, Orb transitions from a diffuse state in oocytes into condensed sponge body-like granules, implicating phosphorylation in inhibiting Orb condensation (Wong et al., 2011). Deducing the precise consequence of ectopic Orb condensates in ck2 mutants is not straightforward; however, the phenotype of both orb and ck2 mutants includes dorsal-ventral defects during oogenesis. Interestingly, several examples demonstrate that phosphorylation does not always affect protein condensation. The Drosophila Pan Gu (PNG) kinase directly phosphorylates two de-capping activator proteins Trailer hitch (TRAL)/RAP55 and Me31B/RCK; however, the dispersal of TRAL granules is not affected during in vitro egg activation (Hara et al., 2018). In addition, the phosphorylation of maelstrom (Mael) by polo kinase is not required for its localization to nuage puncta in ovaries (Pek et al., 2012).
Multiple examples in both female and male germ lines of vertebrates demonstrate a role for phosphorylation in regulating protein condensation. In the female mouse germ line, MSY2 is one of several RNA-binding proteins that condense into transient, RNA-containing aggregates in fully grown oocytes but later decondense during oocyte maturation (Flemr et al., 2010). Phosphorylation of MSY2 by CDC2A occurs during oocyte maturation concomitant with its de-condensation, suggesting that phosphorylation may inhibit MSY2 condensation (Medvedev et al., 2008). In contrast, in the male mouse germ line, when Gonadotropin-regulated testicular RNA helicase (GRTH) is not phosphorylated by Protein Kinase A (PKA), GRTH localization to the chromatoid body (CB) is impaired, the CB is reduced in size, testis size is reduced due to germ cell apoptosis, and spermatogenesis arrests at the round spermatid stage (Sheng et al., 2006; Kavarthapu et al., 2019). Phosphorylation of eIF2α by GCN2 also appears to promote its condensation into stress granules in male spermatids in response to temperature stress (Kim et al., 2012; Yoon et al., 2017). In Xenopus oocytes, Staufen proteins (XStau1 and XStau2) are phosphorylated via the MAP Kinase pathway during meiotic maturation (Allison et al., 2004). Phosphorylated XStau1 appears to transition from small aggregates concentrated locally in the vicinity of the ER, to larger aggregates less localized to the ER, suggesting phosphorylation promotes condensation of XStau1 which may impact its association with the ER network (Allison et al., 2004). It is not yet clear how phosphorylation modifies XStau1 function.
Overall, the role of phosphorylation in modulating phase transitions in the germ line has been understudied to date. Dozens of RNA binding proteins have been characterized as components of P granules in C. elegans, and of polar granules and nuage in Drosophila (Updike and Strome, 2010; Kato and Nakamura, 2012). It will be interesting to determine the extent to which phosphorylation regulates condensation of these proteins. For example, the C. elegans MPK-1 ERK (extracellular signal-regulated kinase) controls seven different processes in the adult germ line, including germ cell apoptosis and oocyte maturation (Lee et al., 2007). Genomic approaches have identified 30 ERK substrates including several RNA binding proteins, some of which are P granule proteins (Arur et al., 2009). Future studies should be able to address if ERK modulates condensation of any of its protein substrates.
The germ line has distinct functions from the soma, including the need to accurately transmit genetic information between generations and the requirement of pluripotency. The germ line may also have unique requirements in regulating gene expression, as large pools of maternal mRNAs accumulate in oocytes, many of which are non-translating until after fertilization. Germ line RNP condensates can facilitate post-transcriptional gene regulation of mRNA, and the prevailing theory is that such gene regulation is advantageous for rapid gene activation post-fertilization. PTMs afford some of the fastest changes to protein function that are also reversible and avoid de novo nuclear activities. Since RNP condensates are very dynamic complexes, these advantages may begin to answer why PTMs have evolved as an important regulator of germ line RNP condensates.
In comparison to the many examples of PTMs modulating phase transitions in vitro and in cell culture, there are notably fewer documented cases in the germ line. This discrepancy may simply reflect the challenge of studying PTMs in vivo, with the expectation that additional examples will be identified in the future. Alternatively, the relatively low number of examples may be due to alternative mechanisms regulating condensates in the germ line, with a smaller relative role for PTMs. This review highlights one major difference between in vitro studies that demonstrate a role for methylation in reducing phase separation, and germ line studies that show a role in promoting condensation of RNA binding proteins. In particular, the example of Ddx4/Vasa is striking. The formation of liquid droplets of the disordered N-terminal domain of Ddx4 is suppressed by asymmetric demethylation via PRMT1 expression in bacterial cells (Nott et al., 2015). In contrast, the methylation of Vasa by Dart5/Csul in Drosophila promotes the assembly of nuage granules in nurse cells and of piNG bodies in spermatocytes (Kibanov et al., 2011). The explanation for these opposite effects may simply be a more complex in vivo environment in the germ line, but probing this difference could be helpful in understanding the limitations of applying in vitro findings to the germ line. Another instance of context-specific effects of PTMs can be seen in the C. elegans embryo. MBK-2 phosphorylation of the MEG proteins drives disassembly of P granules, including the PGL-1 protein (Wang et al., 2014); however, phosphorylation of PGL-1 by mTORC1 stimulates the assembly of ectopic P granules in somatic blastomeres during heat stress (Zhang et al., 2018). These differences highlight our incomplete understanding of the role of PTMs in regulating phase separation.
One important consideration not yet discussed is the combinatorial nature of PTMs in modulating the assembly of granules. RNA binding proteins are often both phosphorylated and methylated, suggesting these two types of PTMs can be either synergistic or antagonistic (Bah and Forman-Kay, 2016). In addition, other PTMs such as O-linked GlcNAc modification and lysine acetylation have been shown to interact with phosphorylation in in vitro assays. For example, acetylation of Tau on Lys-321 prevents phosphorylation of a downstream Ser residue, and results in decreased aggregation of Tau filaments (Carlomagno et al., 2017). In regards to the germ line, few combinatorial PTMs of RNA binding proteins have been identified to date. However, the C. elegans PGL-1 protein is methylated by EPG-11 which inhibits PGL-1 aggregation into granules in vitro, and may act similarly in somatic blastomeres of the early embryo (Li et al., 2013; Zhang et al., 2018). The de-condensation of PGL-1 in somatic blastomeres can be balanced during heat stress when phosphorylation of PGL-1 by mTORC1 accelerates phase separations, resulting in ectopic somatic granules (Zhang et al., 2018). It remains to be seen if RNP condensates in the germ line proper are similarly modulated by combinations of PTMs.
Another interesting avenue to pursue is the extent to which stress conditions trigger PTMs that regulate RNP condensates in the germ line. Heat stress, extended meiotic arrest, starvation, hypoxia, and osmotic stress can induce the assembly of large RNP condensates in the germ line; however, PTMs have not been identified as regulators of any of these stress-induced granules to date (Schisa, 2014). In vitro studies, on the other hand, show that certain stresses lead to phosphorylation of FUS in addition to its constitutive Arg-methylation (Rhoads et al., 2018). During environmental stresses, stress granule formation is stimulated by a combination of phosphorylation and O-GlcNAcylation on Ser/Thr residues; however, the precise cause and effect relationship between these combinations of PTMs and phase transitions remains incompletely understood (Hofweber and Dormann, 2019).
Another unresolved question is whether RNP condensates in germ cells are bona fide phase-separated structures. To address this, time-lapse microscopy could be useful to determine the behavior of fusing droplets in vivo. FRAP studies may also be helpful; however, fast FRAP recovery is not sufficient to demonstrate phase separation (Alberti et al., 2019). The use of super-resolution microscopy is a relatively new tool that may be useful in mapping phase diagrams to determine concentration dependent thresholds for assembly (Patel et al., 2015; Rai et al., 2018). Another approach being developed to assess viscosity and porosity of condensates is the use of genetically encoded nanoparticles (GEMS) as microrheology probes (Alberti et al., 2019). One challenge to the field with investigations of the physical properties of in vivo germ line condensates is distinguishing whether genetic perturbations that affect function do so due to altered condensation or independently of condensation alterations.
We have a good appreciation that the dysregulation of protein folding and condensation can result in human disease, as is well-exemplified by multiple neurodegenerative diseases, for example, ectopic aggregates containing TDP-43 and FUS in ALS. Germ cells share several attributes with neurons such as being post-mitotic and differentiated, and relying on regulation of gene expression post-transcriptionally, e.g., the synaptic ends of axons are a distance from nuclei, and maturing oocytes have large stores of maternal mRNAs. Certain stresses and mutations cause increased condensation of RNA binding proteins in C. elegans and Drosophila germ lines. In some cases, the condensates are liquid-like and reversible and have been hypothesized to be protective (Jud et al., 2008; Shimada et al., 2011; Hubstenberger et al., 2013); while in other cases, such as the cgh-1 (tn691) germ line at restrictive temperature, RNA binding proteins condense into sheet-like structures with immobile pools of protein (Hubstenberger et al., 2013; Langerak et al., 2019). The cgh-1 (tn691) phenotype also includes oogenesis defects, increased germ line apoptosis, and embryonic lethality; therefore, the pleiotropic nature of the defects makes it impossible to assess if the condensation of RNA binding proteins into solid structures contributes directly to the infertility (Navarro et al., 2001; Audhya et al., 2005). It will be of interest for future studies to find approaches to address the cause and effect relationships between condensate regulation and gamete development/function in the germ line.
JS contributed writing and editing of the manuscript and prepared the figures. ME contributed writing of the manuscript. Both authors contributed to the article and approved the submitted version.
JS is funded by the National Institutes of General Medical Sciences 2R15GM109337.
The authors declare that the research was conducted in the absence of any commercial or financial relationships that could be construed as a potential conflict of interest.
The authors thank Dr. Xantha Karp and lab members for helpful feedback on the manuscript.
Akpınar, M., Lesche, M., Fanourgakis, G., Fu, J., Anasstasiadis, K., Dahl, A., et al. (2017). TDRD6 mediates early steps of spliceosome maturation in primary spermatocytes. PLoS Genet. 13:e1006660. doi: 10.1371/journal.pgen.1006660
Alberti, S., Gladfelter, A., and Mittag, T. (2019). Considerations and challenges in studying liquid-liquid phase separation and biomolecular condensates. Cell 176, 419–434. doi: 10.1016/j.cell.2018.12.035
Allison, R., Czaplinski, K., Git, A., Adegbenro, E., Stennard, F., Houliston, E., et al. (2004). Two distinct Staufen isoforms in Xenopus are vegetally localized during oogenesis. RNA 10, 1751–1763. doi: 10.1261/rna.7450204
Ambadipudi, S., Biernat, J., Riedel, D., Mandelkow, E., and Zweckstetter, M. (2017). Liquid-liquid phase separation of the microtubule-binding repeats of the Alzheimer-related protein Tau. Nat. Commun. 8:275. doi: 10.1038/s41467-017-00480-0
Anne, J. (2010). Arginine methylation of SmB is required for Drosophila germ cell development. Development 137, 2819–2828. doi: 10.1242/dev.052944
Anne, J., Ollo, R., Ephrussi, A., and Mechler, B. M. (2007). Arginine methyltransferase Capsuléen is essential for methylation of spliceosomal Sm proteins and germ cell formation in Drosophila. Development 134, 137–146. doi: 10.1242/dev.02687
Arkov, A. L., and Ramos, A. (2010). Building RNA-protein granules: insight from the germline. Trends Cell Biol. 20, 482–490. doi: 10.1016/j.tcb.2010.05.004
Arur, S., Ohmachi, M., Nayak, S., Hayes, M., Miranda, A., Hay, A., et al. (2009). Multiple ERK substrates execute single biological processes in Caenorhabditis elegans germ-line development. Proc. Natl. Acad. Sci. U.S.A. 106, 4776–4781. doi: 10.1073/pnas.0812285106
Audhya, A., Hyndman, F., McLeod, I. X., Maddox, A. S., Yates, J. R. III, Desai, A., et al. (2005). A complex containing the Sm protein CAR-1 and the RNA helicase CGH-1 is required for embryonic cytokinesis in Caenorhabditis elegans. J. Cell Biol. 171, 267–279. doi: 10.1083/jcb.200506124
Bah, A., and Forman-Kay, J. D. (2016). Modulation of intrinsically disordered protein function by post-translational modifications. J. Biol. Chem. 291, 6696–6705. doi: 10.1074/jbc.R115.695056
Bedford, M. T., and Clarke, S. G. (2009). Protein Arginine Methylation in Mammals: who. What, and why. Mol. Cell 33, 1–13. doi: 10.1016/j.molcel.2008.12.013
Boke, E., Ruer, M., Wühr, M., Coughlin, M., Lemaitre, R., Gygi, S. P., et al. (2016). Amyloid-like self-assembly of a cellular compartment. Cell 166, 637–650. doi: 10.1016/j.cell.2016.06.051
Brangwynne, C. P., Eckmann, C. R., Courson, D. S., Rybarska, A., Hoege, C., Gharakhani, J., et al. (2009). Germline P granules are liquid droplets that localize by controlled dissolution/condensation. Science 324, 1729–1732. doi: 10.1126/science.1172046
Carlomagno, Y., Chung, D. C., Yue, M., Castanedes-Casey, M., Madden, B. J., Dunmore, J., et al. (2017). An Acetylation–phosphorylation switch that regulates tau aggregation propensity and function. J. Biol. Chem. 292, 15277–15286. doi: 10.1074/jbc.M117.794602
Chong, P. A., and Forman-Kay, J. D. (2016). Liquid–liquid phase separation in cellular signaling systems. Curr. Opin. Struct. Biol. 41, 180–186. doi: 10.1016/j.sbi.2016.08.001
De Leeuw, F., Zhang, T., Wauquier, C., Huez, G., Kruys, V., and Gueydan, C. (2007). The cold-inducible RNA-binding protein migrates from the nucleus to cytoplasmic stress granules by a methylation-dependent mechanism and acts as a translational repressor. Exp. Cell Res. 313, 4130–4144. doi: 10.1016/j.yexcr.2007.09.017
Fackelmayer, F. O. (2005). Protein arginine methyltransferases: guardians of the arg? Trends Biochem. Sci. 30, 666–671. doi: 10.1016/j.tibs.2005.10.002
Flemr, M., Ma, J., Schultz, R. M., and Svoboda, P. (2010). P-body loss is concomitant with formation of a messenger RNA storage domain in mouse oocytes. Biol. Reprod. 82, 1008–1017. doi: 10.1095/biolreprod.109.082057
Gonsalvez, G. B., Rajendra, T. K., Tian, L., and Matera, A. G. (2006). The Sm-protein methyltransferase, Dart5, is essential for germ-cell specification and maintenance. Curr. Biol. 16, 1077–1089. doi: 10.1016/j.cub.2006.04.037
Hara, M., Lourido, S., Petrova, B., Lou, H. J., Von Stetina, J. R., Kashevsky, H., et al. (2018). Identification of PNG kinase substrates uncovers interactions with the translational repressor TRAL in the oocyte-to-embryo transition. ELife 7:e33150. doi: 10.7554/eLife.33150
Hofweber, M., and Dormann, D. (2019). Friend or foe-post-translational modifications as regulators of phase separation and RNP granule dynamics. J. Biol. Chem. 294, 7137–7150. doi: 10.1074/jbc.TM118.001189
Hubstenberger, A., Noble, S. L., Cameron, C., and Evans, T. C. (2013). Translation repressors, an RNA helicase, and developmental cues control RNP phase transitions during early development. Dev. Cell 27, 161–173. doi: 10.1016/j.devcel.2013.09.024
Itakura, A. K., Futia, R. A., and Jarosz, D. F. (2018). It pays to be in phase. Biochemistry 57, 2520–2529. doi: 10.1021/acs.biochem.8b00205
Jud, M. C., Czerwinski, M. J., Wood, M. P., Young, R. A., Gallo, C. M., Bickel, J. S., et al. (2008). Large P body-like RNPs form in C. elegans oocytes in response to arrested ovulation, heat shock, osmotic stress and anoxia and are regulated by the major sperm protein pathway. Dev. Biol. 318, 38–51. doi: 10.1016/j.ydbio.2008.02.059
Kametani, F., Nonaka, T., Suzuki, T., Arai, T., Dohmae, N., Akiyama, H., et al. (2009). Identification of casein kinase-1 phosphorylation sites on TDP-43. Biochem. Biophys. Res. Commun. 382, 405–409. doi: 10.1016/j.bbrc.2009.03.038
Kato, M., Han, T. W., Xie, S., Shi, K., Du, X., Wu, L. C., et al. (2012). Cell-free formation of RNA granules: low complexity sequence domains form dynamic fibers within hydrogels. Cell 149, 753–767. doi: 10.1016/j.cell.2012.04.017
Kato, Y., and Nakamura, A. (2012). Roles of cytoplasmic RNP granules in intracellular RNA localization and translational control in the Drosophila oocyte. Dev. Grow. Differ. 54, 19–31. doi: 10.1111/j.1440-169X.2011.01314.x
Kavarthapu, R., Anbazhagan, R., Raju, M., Morris, C. H. T., Pickel, J., and Dufau, M. L. (2019). Targeted knock-in mice with a human mutation in GRTH/DDX25 reveals the essential role of phosphorylated GRTH in spermatid development during spermatogenesis. Hum. Mol. Genet. 28, 2561–2572. doi: 10.1093/hmg/ddz079
Kibanov, M. V., Egorova, K. S., Ryazansky, S. S., Sokolova, O. A., Kotov, A. A., Olenkina, O. M., et al. (2011). A novel organelle, the piNG-body, in the nuage of Drosophila male germ cells is associated with piRNA-mediated gene silencing. Mol. Biol. Cell 22, 3410–3419. doi: 10.1091/mbc.E11-02-0168
Kim, B., Cooke, H. J., and Rhee, K. (2012). DAZL is essential for stress granule formation implicated in germ cell survival upon heat stress. Development 139, 568–578. doi: 10.1242/dev.075846
Kirino, Y., Vourekas, A., Sayed, N., De Lima Alves, F., Thomson, T., Lasko, P., et al. (2010). Arginine methylation of aubergine mediates tudor binding and germ plasm localization. RNA 16, 70–78. doi: 10.1261/rna.1869710
Kloc, M., Bilinski, S., and Etkin, L. D. (2004). The Balbiani body and germ cell determinants: 150 years later. Curr. Top. Dev. Biol. 59, 1–36. doi: 10.1016/S0070-2153(04)59001-4
Langerak, S., Trombley, A., Patterson, J. R., Leroux, D., Couch, A., Wood, M. P., et al. (2019). Remodeling of the endoplasmic reticulum in Caenorhabditis elegans oocytes is regulated by CGH-1. Genesis 57:e23267. doi: 10.1002/dvg.23267
Lee, M. H., Ohmachi, M., Arur, S., Nayak, S., Francis, R., Church, D., et al. (2007). Multiple functions and dynamic activation of MPK-1 extracellular signal-regulated kinase signaling in Caenorhabditis elegans germline development. Genetics 177, 2039–2062. doi: 10.1534/genetics.107.081356
Li, P., Banjade, S., Cheng, H. C., Kim, S., Chen, B., Guo, L., et al. (2012). Phase transitions in the assembly of multivalent signalling proteins. Nature 483, 336–340. doi: 10.1038/nature10879
Li, S., Yang, P., Tian, E., and Zhang, H. (2013). Arginine methylation modulates autophagic degradation of PGL granules in C.elegans. Mol. Cell 52, 421–433. doi: 10.1016/j.molcel.2013.09.014
Luo, F., Gui, X., Zhou, H., Gu, J., Li, Y., Liu, X., et al. (2018). Atomic structures of FUS LC domain segments reveal bases for reversible amyloid fibril formation. Nat. Struct. Mol. Biol. 25, 341–346. doi: 10.1038/s41594-018-0050-8
Matsumoto, K., Nakayama, H., Yoshimura, M., Masuda, A., Dohmae, N., Matsumoto, S., et al. (2012). PRMT1 is required for RAP55 to localize to processing bodies. RNA Biol. 9, 610–623. doi: 10.4161/rna.19527
Medvedev, S., Yang, J., Hecht, N. B., and Schultz, R. M. (2008). CDC2A (CDK1)-mediated phosphorylation of MSY2 triggers maternal mRNA degradation during mouse oocyte maturation. Dev. Biol. 321, 205–215. doi: 10.1016/j.ydbio.2008.06.016
Monahan, Z., Ryan, V. H., Janke, A. M., Burke, K. A., Rhoads, S. N., Zerze, G. H., et al. (2017). Phosphorylation of the FUS low−complexity domain disrupts phase separation, aggregation, and toxicity. EMBO J. 36, 2951–2967. doi: 10.15252/embj.201696394
Murray, D. T., Kato, M., Lin, Y., Thurber, K. R., Hung, I., McKnight, S. L., et al. (2017). Structure of FUS protein fibrils and its relevance to self-assembly and phase separation of low-complexity domains. Cell 171, 615–627.e16. doi: 10.1016/j.cell.2017.08.048
Navarro, R. E., Shim, E. Y., Kohara, Y., Singson, A., and Blackwell, T. K. (2001). Cgh-1, a conserved predicted RNA helicase required for gametogenesis and protection from physiological germline apoptosis in C. elegans. Development 128, 3221–3232.
Nott, T. J., Petsalaki, E., Farber, P., Jervis, D., Fussner, E., Plochowietz, A., et al. (2015). Phase transition of a disordered nuage protein generates environmentally responsive membraneless organelles. Mol. Cell 57, 936–947. doi: 10.1016/j.molcel.2015.01.013
Orsborn, A. M., Li, W., McEwen, T. J., Mizuno, T., Kuzmin, E., Matsumoto, K., et al. (2007). GLH-1, the C. elegans P granule protein, is controlled by the JNK KGB-1 and by the COP9 subunit CSN-5. Development 134, 3383–3392. doi: 10.1242/dev.005181
Patel, A., Lee, H. O., Jawerth, L., Maharana, S., Jahnel, M., Hein, M. Y., et al. (2015). A Liquid-to-Solid phase transition of the ALS protein FUS accelerated by disease mutation. Cell 162, 1066–1077. doi: 10.1016/j.cell.2015.07.047
Pek, J. W., Ng, B. F., and Kai, T. (2012). Polo-mediated phosphorylation of maelstrom regulates oocyte determination during oogenesis in drosophila. Development (Cambridge) 139, 4505–4513. doi: 10.1242/dev.082867
Putnam, A., Cassani, M., Smith, J., and Seydoux, G. (2019). A gel phase promotes condensation of liquid P granules in C. elegans embryos. Nat. Struct. Mol. Biol. 26, 220–226. doi: 10.1038/s41594-019-0193-2
Qamar, S., Wang, G. Z., Randle, S. J., Ruggeri, F. S., Varela, J. A., Lin, J. Q., et al. (2018). FUS phase separation is modulated by a molecular chaperone and methylation of arginine cation-π interactions. Cell 173, 720–734.e15. doi: 10.1016/j.cell.2018.03.056
Rai, D., Dey, S., and Ray, K. (2018). A method for estimating relative changes in the synaptic density in Drosophila central nervous system. BMC Neurosci. 19:30. doi: 10.1186/s12868-018-0430-3
Rhoads, S. N., Monahan, Z. T., Yee, D. S., and Shewmaker, F. P. (2018). The role of post-translational modifications on prion-like aggregation and liquid-phase separation of FUS. Int. J. Mol. Sci. 19:886. doi: 10.3390/ijms19030886
Roovers, E. F., Kaaij, L. J. T., Redl, S., Bronkhorst, A. W., Wiebrands, K., de Jesus Domingues, A. M., et al. (2018). Tdrd6a regulates the aggregation of Buc into functional subcellular compartments that drive germ cell specification. Dev. Cell 46, 285–301.e9. doi: 10.1016/j.devcel.2018.07.009
Rouhana, L., Vieira, A. P., Roberts-Galbrait, R. H., and Newmark, P. A. (2012). PRMT5 and the role of symmetrical dimethylarginine in chromatoid bodies of planarian stem cells. Development 139, 1083–1094. doi: 10.1242/dev.076182
Schisa, J. A. (2012). New insights into the regulation of RNP granule assembly in oocytes. Int. Rev. Cell Mol. Biol. 295, 233–289. doi: 10.1016/B978-0-12-394306-4.00013-7
Schisa, J. A. (2014). Effects of stress and aging on ribonucleoprotein assembly and function in the germ line. WIREs RNA 5, 231–246. doi: 10.1002/wrna.1204
Seydoux, G. (2018). The P granules of C. elegans: a genetic model for the study of RNA–protein condensates. J. Mol. Biol. 430, 4702–4710. doi: 10.1016/j.jmb.2018.08.007
Sheng, Y., Tsai-Morris, C. H., Gutti, R., Maeda, Y., and Dufau, M. L. (2006). Gonadotropin-regulated testicular RNA helicase (GRTH/Ddx25) is a transport protein involved in gene-specific mRNA export and protein translation during spermatogenesis. J. Biol. Chem. 281, 35048–35056. doi: 10.1074/jbc.M605086200
Shimada, Y., Burn, K. M., Niwa, R., and Cooley, L. (2011). Reversible response of protein localization and microtubule organization to nutrient stress during Drosophila early oogenesis. Dev. Biol. 355, 250–262. doi: 10.1016/j.ydbio.2011.04.022
Shishkova, E., Zeng, H., Liu, F., Kwiecien, N. W., Hebert, A. S., Coon, J. J., et al. (2017). Global mapping of CARM1 substrates defines enzyme specificity and substrate recognition. Nat. Commun. 24:15571. doi: 10.1038/ncomms15571
Strasser, M. J., Mackenzie, N. C., Dumstrei, K., Nakkrasae, L. I., Stebler, J., and Raz, E. (2008). Control over the morphology and segregation of Zebrafish germ cell granules during embryonic development. BMC Dev. Biol. 8:58. doi: 10.1186/1471-213X-8-58
Tsai, W. C., Gayatri, S., Reineke, L. C., Sbardella, G., Bedford, M. T., and Lloyd, R. E. (2016). Arginine demethylation of G3BP1 promotes stress granule assembly. J. Biol. Chem. 291, 22671–22685. doi: 10.1074/jbc.M116.739573
Updike, D., and Strome, S. (2010). P granule assembly and function in Caenorhabditis elegans germ cells. J. Androl. 31, 53–60. doi: 10.2164/jandrol.109.008292
Vagin, V. V., Wohlschlegel, J., Qu, J., Jonsson, Z., Huang, X., Chuma, S., et al. (2009). Proteomic analysis of murine Piwi proteins reveals a role for arginine methylation in specifying interaction with Tudor family members. Genes Dev. 23, 1749–1762. doi: 10.1101/gad.1814809
Vasileva, A., Tiedau, D., Firooznia, A., Müller-Reichert, T., and Jessberger, R. (2009). Tdrd6 is required for spermiogenesis, chromatoid body architecture, and regulation of miRNA expression. Curr. Biol. 19, 630–639. doi: 10.1016/j.cub.2009.02.047
Voronina, E., Seydoux, G., Sassone-Corsi, P., and Nagamori, I. (2011). RNA granules in germ cells. Cold Spring Harb. Perspect. Biol. 3:a002774. doi: 10.1101/cshperspect.a002774
Wang, A., Conicella, A. E., Schmidt, H. B., Martin, E. W., Rhoads, S. N., Reeb, A. N., et al. (2018). A single N-terminal phosphomimic disrupts TDP-43 polymerization, phase separation, and RNA splicing. EMBO J. 37, 1–18. doi: 10.15252/embj.201797452
Wang, J. T., Smith, J., Chen, B. C., Schmidt, H., Rasoloson, D., Paix, A., et al. (2014). Regulation of RNA granule dynamics by phosphorylation of serine-rich, intrinsically disordered proteins in C. elegans. ELife 3:e04591. doi: 10.7554/eLife.04591
Wippich, F., Bodenmiller, B., Trajkovska, M. G., Wanka, S., Aebersold, R., and Pelkmans, L. (2013). Dual specificity kinase DYRK3 couples stress granule condensation/dissolution to mTORC1 signaling. Cell 152, 791–805. doi: 10.1016/j.cell.2013.01.033
Wong, L. C., Costa, A., McLeod, I., Sarkeshik, A., Yates, J., Kyin, S., et al. (2011). The functioning of the drosophila CPEB protein orb is regulated by phosphorylation and requires casein kinase 2 activity. PLoS One 6:e24355. doi: 10.1371/journal.pone.0024355
Woodruff, J. B., Hyman, A. A., and Boke, E. (2018). Organization and function of non-dynamic biomolecular condensates. Trends Biochem. Sci. 43, 81–94. doi: 10.1016/j.tibs.2017.11.005
Xie, H., Vucetic, S., Iakoucheva, L. M., Oldfield, C. J., Dunker, A. K., Uversky, V. N., et al. (2007). Functional anthology of intrinsic disorder. 1. Biological processes and functions of proteins with long disordered regions. J. Proteome Res. 6, 1882–1898. doi: 10.1021/pr060392u
Yoon, J., Park, K., Hwang, D. S., and Rhee, K. (2017). Importance of eIF2α phosphorylation as a protective mechanism against heat stress in mouse male germ cells. Mol. Reprod. Dev. 84, 265–274. doi: 10.1002/mrd.22778
Zhang, G., Wang, Z., Du, Z., and Zhang, H. (2018). mTOR regulates phase separation of PGL granules to modulate their autophagic degradation. Cell 174, 1492–1506.e22. doi: 10.1016/j.cell.2018.08.006
Keywords: RNP granules, phase transition, germ line, methylation, phosphorylation, condensate
Citation: Schisa JA and Elaswad MT (2021) An Emerging Role for Post-translational Modifications in Regulating RNP Condensates in the Germ Line. Front. Mol. Biosci. 8:658020. doi: 10.3389/fmolb.2021.658020
Received: 24 January 2021; Accepted: 19 March 2021;
Published: 08 April 2021.
Edited by:
Nicolas Lux Fawzi, Brown University, United StatesReviewed by:
Dustin Updike, Mount Desert Island Biological Laboratory, United StatesCopyright © 2021 Schisa and Elaswad. This is an open-access article distributed under the terms of the Creative Commons Attribution License (CC BY). The use, distribution or reproduction in other forums is permitted, provided the original author(s) and the copyright owner(s) are credited and that the original publication in this journal is cited, in accordance with accepted academic practice. No use, distribution or reproduction is permitted which does not comply with these terms.
*Correspondence: Jennifer A. Schisa, c2NoaXMxakBjbWljaC5lZHU=
Disclaimer: All claims expressed in this article are solely those of the authors and do not necessarily represent those of their affiliated organizations, or those of the publisher, the editors and the reviewers. Any product that may be evaluated in this article or claim that may be made by its manufacturer is not guaranteed or endorsed by the publisher.
Research integrity at Frontiers
Learn more about the work of our research integrity team to safeguard the quality of each article we publish.