- School of Molecular Sciences, Arizona State University, Tempe, AZ, United States
The current trend in atmospheric carbon dioxide concentrations is causing increasing concerns for its environmental impacts, and spurring the developments of sustainable methods to reduce CO2 to usable molecules. We report the light-driven CO2 reduction in water in mild conditions by artificial protein catalysts based on cytochrome b562 and incorporating cobalt protoporphyrin IX as cofactor. Incorporation into the protein scaffolds enhances the intrinsic reactivity of the cobalt porphyrin toward proton reduction and CO generation. Mutations around the binding site modulate the activity of the enzyme, pointing to the possibility of further improving catalytic activity through rational design or directed evolution.
Introduction
The ongoing use of fossil fuels has led to an increase in atmospheric CO2 concentrations, causing severe consequences for the environment (Lacis et al., 2010). Current research efforts are focused on developing energetic alternatives that can help curb CO2 emissions. Although nature aids in the removal of this greenhouse gas through photosynthesis (Sun et al., 2014; Kondo et al., 2018), negative emissions technologies are necessary to reduce the excess gas from the atmosphere (Smith et al., 2015). One possible path is through artificial photosynthesis, where light is utilized as the energy source to drive production of carbon-free or carbon-neutral fuels, mirroring the role of the enzyme RuBisCO in fixing CO2. In that vein, promising approaches interfacing photosensitizers and/or materials with molecular catalysts, enzymes, and microorganisms have been developed (Woo, 2017; Luan and Lu, 2018; Edwards and Bren, 2020; Ma et al., 2020).
Artificial metalloenzymes obtained by incorporating organometallic catalysts within a protein bridge traditional molecular catalysts and enzymes, with the advantage of expanding the range of reactivity encountered in natural enzymes. Protein scaffolds may aide the efficiency of the organometallic center by offering tunable primary and secondary coordination spheres, by facilitating reactant binding and product release to and from the active site, and by protecting the organometallic center from degradation (Lu et al., 2009; Alcala-Torano et al., 2016; Schwizer et al., 2018; Davis and Ward, 2019; Nastri et al., 2019; Oohora et al., 2019; Vornholt et al., 2020). This approach has been used to produce water-soluble catalysts that are capable of producing hydrogen from protons under mild conditions, repurposing a diverse group of organometallic catalysts (Sano et al., 2011; Roy et al., 2012; Kleingardner et al., 2014; Onoda et al., 2014; Sommer et al., 2014; Sommer et al., 2016; Firpo et al., 2018; Call et al., 2019a; Slater et al., 2019; Walsh et al., 2019; Alvarez-Hernandez et al., 2020; Laureanti et al., 2020; Le et al., 2020; Roy et al., 2020). Comparatively little work has been carried out on the reduction of CO2 by hybrid metalloenzymes: in one example nickel cyclam complexes anchored to azurin support catalytic CO2 reduction, with evidence of protein modulation of activity compared to the isolated cyclam (Schneider and Shafaat, 2016; Liu et al., 2018; Schneider et al., 2018).
Here, we investigate whether coordination of CoPPIX within a protein scaffold would support carbon dioxide reduction, motivated by recent reports of metalloporphyrins as molecular CO2 reduction electrocatalysts (Manbeck and Fujita, 2015; Mondal et al., 2015; Zhang et al., 2017; Birdja et al., 2018; Rao et al., 2018) and most recently photocatalysts (Call et al., 2019b; Fukuzumi et al., 2019). In these catalysts, tuning the reaction conditions or modifying the porphyrin framework in order to stabilize catalytic intermediates through hydrogen bonding resulted in increased activities of these catalysts toward CO2 reduction (Bhugun et al., 1996; Lee et al., 2011; Costentin et al., 2012; Azcarate et al., 2016a; b; Call et al., 2019b). Further, increased turnover numbers (TON) and product selectivity upon incorporation of cobalt porphyrins into supramolecular structures such as metal-organic frameworks (Kornienko et al., 2015; Lin et al., 2015; Zhang et al., 2016; Feng et al., 2020; Wu et al., 2020; Zhang et al., 2020) and polymers (Kramer and McCrory, 2016; Liu and McCrory, 2019) suggest that catalysis by cobalt porphyrins could be enhanced by incorporation into a protein environment. Our approach utilizes a natural protein scaffold, cytochrome b562 (cyt b562), in which the native heme has been swapped with its cobalt analog, cobalt protoporphyrin IX (CoPPIX). Our group and others have previously demonstrated that exchanging the metal ion to cobalt in heme-binding proteins or peptides results in metalloproteins that can catalyze proton reduction activity, and show an increase of H2 production compared to the cobalt porphyrin in solution. Moreover, altering the protein sequence results in fine modulation of the total activity, further supporting the crucial role of the protein environment throughout the catalytic cycle, beyond simple encapsulation (Kleingardner et al., 2014; Sommer et al., 2014; Sommer et al., 2016; Firpo et al., 2018; Le and Bren, 2019; Alvarez-Hernandez et al., 2020; Le et al., 2020). Compared with other supramolecular systems, proteins are easily modifiable by mutating the amino acid sequence, allowing the systematic exploration of the primary and secondary coordination sphere. Recent advances dramatically expand the range of chemical moieties available on the side chains through incorporation of noncanonical amino acids (Alcala-Torano et al., 2016; Burke et al., 2019; Drienovská et al., 2020; Drienovská and Roelfes, 2020; Zhou and Roelfes, 2020). Further, proteins can be optimized by directed evolution coupled with high-thrughput screening to identify favorable mutations (Liang et al., 2019; Chen and Arnold, 2020; Jeong et al., 2020; Markel et al., 2020).
Material and Methods
All chemicals were purchased from Sigma-Aldrich and used without further purification unless otherwise noted. Calibration gases were obtained from Matheson in 14 L lecture bottles. All aqueous solutions were prepared using deionized water with a resistivity greater or equal to 18 MΩ. Cobalt (III) protoporphyrin IX chloride was purchased from Sigma-Aldrich and used without further purification.
Protein Expression
Mutants were generated in a pET30c (+) vector encoding WT cyt b562 using mutagenic primers by Gibson assembly as described in Supplementary Information (Supplementary Table S1 and Supplementary Figure S8) (Sommer et al., 2016). The verified mutants were transformed into Escherichia coli BL21 (DE3) and grown in 1 L of 2xTY media at 37 °C with shaking at 300 rpm. Cells were induced with 1 mM IPTG at an OD600 of 0.6 and harvested after 4 h of expression. The cell pellets were suspended in 20 mM Tris-HCl, 1 mM DTT, 0.5 mM EDTA and lysed by multiple cycles of ultrasonication. The clarified lysate was brought to 75% saturation with solid ammonium sulfate, and precipitated proteins were removed by centrifugation. The supernatant, containing the cytochrome mutants, was dialyzed against two changes of 10 mM Tris pH 7.5 and one of water at 4 °C. Following dialysis, the protein solution was lyophilized and redissolved in 10 mM NaCl water for further purification via RP-HPLC, using a semi-preparatory scale C18 column with a linear 1% min−1 gradient from 100% solvent A (0.1% v/v TFA in water) to 100% solvent B (4.9% v/v water, 0.1% v/v TFA in acetonitrile). The fractions containing the desired protein were then lyophilized to yield the pure apo-protein. The protein identities were confirmed via MALDI-TOF-MS and their purity determined by C18 analytical analysis (Supplementary Information, Supplementary Figure S9). The purified protein was lyophilized and stored at −78 °C. Protein stocks were prepared by dissolving the protein in the appropriate phosphate buffer. The protein stock concentration was determined by UV spectroscopy in 6 M urea using an extinction coefficient of 2980 M−1 cm−1 at 280 nm.
Binding Assays
The dissociation constant (Kd) of each mutant was assessed by titrating a solution of CoPPIX in 200 mM potassium phosphate buffer at pH 7.5 with a solution of the apo protein, and monitoring the absorbance of the Soret peak corresponding to the holo protein (∼425 nm depending on the mutant) (Supplementary Figure S2). The initial CoPPIX concentration was determined using the observed absorbance value at the Soret peak of the free porphyrin (417 nm) and ε = 143,540 M−1 cm−1 (as determined by ICP-OES experiments). All titrations were carried out in an anaerobic chamber (Coy Laboratory Products) equipped with UV-Vis (Ocean Optics USB4000). Data were fitted to Eq. 1 using OriginLab as described in the Supplementary Information by adapting established procedures (Hulme and Trevethick, 2010; Kovacs et al., 2010; Thordarson, 2011).
ΔA and Δε are the differences in absorbance and in extinction coefficients, respectively, between the free porphyrin and the holo protein at the latter’s Soret peak (∼425 nm). [CoPPIX]T is the total CoPPIX concentration, and [Cyt]T is the total protein concentration after each addition.
Circular Dichroism Spectroscopy
CD spectra were recorded on a JASCO J-815 spectropolarimeter in the range of 200–280 nm using a 1 mm cuvette. Data points were recorded every 1 nm and averaged over three scans. The protein concentration was kept at 5 µM in 10 mM phosphate buffer pH 7.5 and the spectra was recorded at 20 °C. Holo protein measurements were carried out in excess CoPPIX (20 μM). Thermal denaturation was performed by heating samples from 4 to 90 °C at a rate of 1°C min−1, monitoring the loss of signal at 222 nm.
Photocatalytic Experiments
Reactions were carried out in sealed cuvettes. Stock buffer containing 200 mM potassium phosphate and 125 mM ascorbic acid at pH 6.0 was bubbled with either CO2 or Ar, and adjusted back to pH 6.0 with aqueous KOH if necessary. Finally, solid [Ru (bpy)3]Cl2·6 H2O was added to a 1.25 mM final concentration, flash frozen in aliquots, and stored at −80 °C. Before each assay, CoPPIX was dissolved in 100 mM KOH to make a saturated solution, and the stock concentration was determined by UV-Vis using the Soret peak at 417 nm with an extinction coefficient of ε = 143,540 M−1 cm−1 (as determined by ICP-OES measurements). The frozen buffer was thawed under an atmosphere of either CO2 or Ar, and CoPPIX and/or protein (in 200 mM potassium phosphate) added to obtain working solutions containing 20 µM CoPPIX, 30 µM protein, 100 mM ascorbic acid, and 1 mM [Ru(bpy)3]2+ in 200 mM potassium phosphate. The ratio of CoPPIX to apo protein was chosen from equilibrium calculations based on the highest observed Kd value to ensure ≥95% of porphyrin was bound to the protein.
For each experiment, 400 µL of sample was added to a 10 mm × 1 mm gas tight cuvette of known headspace volume, and the headspace sparged with gas (Ar or CO2) for 20 min. The cuvettes were then irradiated with a white light LED source for 8 h. All experiments were done in triplicate and the variation is reported as the standard deviation of the sample. The gaseous products, H2 and CO, were quantified by removing 100 μL from the headspace at time intervals, and analyzed with an SRI Instruments gas chromatograph equipped with a 3 ft × 1/8” molecular sieve 5 Å packed column. H2 and CO were detected and quantified simultaneously from the same injection using a Thermal Conductivity Detector (TCD) and a Flame ionization Detector (FID) with a methanizer connected in series. The analytes were eluted using Ar as a carrier gas with a temperature program starting at 60 °C for 1 min, ramping at 20 °C min−1 until 80°C, holding for 2 min, ramping at 50 °C min−1 to 250°C, and holding until the CO2 exited the instrument, with a retention time (tR) ca. 12 min. A peak corresponding to H2 was seen on the TCD channel at tR of 0.400 min, while the peak corresponding to CO appeared at tR = 3.42 min on the FID channel. Peak areas were converted to moles using a calibration curve (Supplementary Figures S4 and S5). After irradiation was stopped, the solution was frozen at −80 °C for future analysis. Formate was quantified by diluting the sample to 10% v/v D2O in water and 100 µM sodium 4,4-dimethyl-1-silapentane-1- sulfonate (DSS) as an internal standard. The samples were then analyzed by 1H NMR using a water suppression method with 64 scans and a 30 s relaxation delay. The formate concentration was determined by comparing the integration area of the singlet at 8.45 ppm to the DSS peak at 0.0 ppm (Boston et al., 2013; Yehezkeli et al., 2016).
Turnover numbers (TON) are reported as the ratio of moles of product produced by moles of total CoPPIX present in solution. Comparisons between the means of each experiment were assessed using a two-tailed t-test analysis for samples with equal or unequal variances as determined by Levene’s test. The null hypothesis (H0:
Results and Discussion
Protein Design
Cyt b562 is a small, water-soluble, four-helix bundle that natively binds a heme cofactor via bis-axial ligation from the side chains of residues His102 and Met7 (Figure 1), and can coordinate a wide range of metalloporphyrins (Pia et al., 2012; Sommer et al., 2016; Bowen et al., 2020). Swapping in CoPPIX for heme and assessing activity under photocatalytic conditions results in a 10 fold increase in hydrogen production compared to CoPPIX in solution (Sommer et al., 2016). In order to facilitate binding of carbon dioxide and conversion to product, we utilized mutants that either remove axial ligation sites (M7A and H102A) or alter axial ligation (M7H) (Kamiya et al., 2001; Hay and Wydrzynski, 2005; Sommer et al., 2016). We assessed the effect of these mutations on the efficiency and specificity of CO2 reduction in water by cobalt porphyrins, which yields formate, carbon monoxide, and hydrogen in photocatalytic conditions.
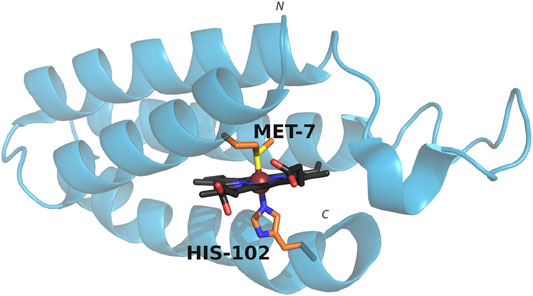
FIGURE 1. Structure of cyt b562 showing the coordinating axial ligands. PDB entry: 1QPU. Colors represent carbon (porphyrin: black, axial residues: orange), oxygen (red), nitrogen (blue), sulfur (yellow). The metal ion is shown as a sphere (brown). The N and C terminus are indicated.
We investigated the secondary structure and stability of the mutant proteins in the apo state and in response to binding to CoPPIX. The CD spectra of all apo mutants showed the typical signals of α-helical proteins, with local minima at 208 and 222 nm (Supplementary Information, Supplementary Figure S1). Upon reconstitution with CoPPIX, WT and M7A show minimal changes in this region, indicating that incorporation of the cofactor has little effect on the structure of the protein at room temperature. The signal for M7H increases upon addition of the porphyrin, showing that incorporation of the ligand stabilizes the secondary structure of the protein, possibly because coordination to the metal reduces the buried positive charges related to the two abutting histidines in the unoccupied active site. In contrast, H102A shows a decrease in signal upon addition of the porphyrin suggesting that this mutant adopts different apo and bound structures compared to the other mutants in the series (see discussion below).
More information on structural changes upon binding was gathered by comparing the stability of the mutants in the apo and holo state. We carried out unfolding experiments by monitoring the loss of intensity of the 222 nm peak in the CD spectrum at increasing temperature, obtaining thermal denaturation curves of the apo and holo mutants (SI, Supplementary Figure S1). The data presented in Table 1 shows that the apparent midpoint of the thermal denaturation, Tm, increases significantly upon binding of the porphyrin, with the exception of H102A, which showed a minimal change in its Tm value.
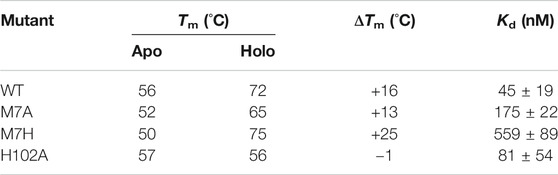
TABLE 1. CD and binding data for Co-cyt b562 mutants. CD spectra was obtained at 20 °C in 10 mM phosphate buffer pH 7.5 with a protein concentration of 5 μM and CoPPIX concentration of 20 μM. The Kd values were obtained using UV-Vis spectroscopy by titrating a stock solution of apo protein to a solution of CoPPIX of known concentration (see Methods and Supplementary Figures S1 and S2).
The stability of the apo state is strongly affected by mutations: compared to WT, the Tm of M7A is lowered 4 °C, possibly due to a decrease in core hydrophobic volume, balanced by the high helical propensity of alanine compared to methionine (Chakrabartty et al., 1991). M7H experiences a 6 °C destabilization. The solution structure of WT apo cyt b562 (Feng et al., 1994) shows that His7 is largely solvent accessible, suggesting a pKa close to neutrality and indicating that the side chain can be partially charged under the experimental conditions (Edgcomb and Murphy, 2002). The observed destabilization correlates to lower helical propensity of histidine—particularly when ionized—compared to methionine (Pace and Scholtz, 1998). In contrast, H102A displays stability slightly higher than WT, because of the high helical propensity of alanine. Binding to CoPPIX stabilizes the proteins to an extent that reflects the changes in axial ligation: M7A is stabilized by 13 °C, a lesser extent than observed in WT (16 °C) because of the loss of axial ligand, while M7H experiences much higher stabilization (25 °C) because of the strong double axial ligation afforded by histidine, and the loss of buried charges upon coordination. In H102A, however, binding to CoPPIX does not increase stability. We speculate that comparable helical content and stabilities between the apo forms of H102A and WT arise from the small contribution of the C-terminal α-helix to the folding of apo cyt b562, because the C-terminus is unfolded in solution (PDB 1APC) (Feng et al., 1994). This observation suggests that folding of the C-terminal α-helix in the holo state of WT is predominantly driven by ligation of His102 to the porphyrin, and abolishing this interaction in H102A prevents folding of the bundle. In contrast, mutations to the axial position M7 have minimal effect on the stability of the holo protein: M7A is slightly destabilized compared to WT, while M7H displays higher apparent Tm, reflecting the relative strength of the axial coordination.
All mutants bind CoPPIX with Kd values in the low to mid-nanomolar range, as assessed through UV-Vis titration of apo protein to a solution of CoPPIX (SI, Supplementary Figure S2 and Table 1). However, displacement of either one of the native axial ligands weakens affinity, and all mutants bind CoPPIX with higher Kd values than WT. Among the mutants, H102A displays the highest affinity, similar to WT, followed by M7A; M7H registers the weakest affinity by far. This observation suggests that binding of the cofactor requires a pre-structured binding pocket in the apo state, as the affinity trend mirrors the trend observed for the stability of the apo forms.
Photocatalytic Activity
The Co-cyt b562 mutants were assayed to investigate their ability to reduce CO2 under photoinduced conditions by using [Ru (bpy)3]2+ as photosensitizer and ascorbic acid as sacrificial electron donor. The excited state of [Ru(bpy)3]2+ is reduced by ascorbic acid to [Ru(bpy)3]+, which in turns transfers electrons to the catalyst resulting in substrate reduction (Morris et al., 2009). The Co-cyt b562 mutants produced carbon monoxide and formate, in addition to H2 produced both in the presence and absence of CO2. Control experiments lacking CoPPIX showed little H2 was produced and no CO or HCO2− were detected, indicating that these species were produced by the cobalt catalyst. The reactivity observed is in line with previous mechanistic studies of cobalt porphyrins used as catalysts for these reactions (SI, Supplementary Figure S3) (Dhanasekaran et al., 1999; Morris et al., 2009; Leung et al., 2010; Nielsen and Leung, 2010; Shen et al., 2016; Call et al., 2019b).
The time-dependent results of the experiments are presented in Figure 2 and the final turnover numbers are shown on Table 2 and Figure 3. When assayed under a CO2 atmosphere at pH 6.0, all mutants showed a two-fold increase in production of CO equivalent compared to the porphyrin alone, indicating that activity is increased by the interaction of the protein with the porphyrin (Table 2; Figures 2, 3). In contrast, activity toward formate production was similar to free porphyrin in all cases, with a slight decrease for M7A at pH 6.0. Observed H2 production activity at pH 6.0 was in all cases higher than CoPPIX, however with significant variation between mutants: M7A showed a (20 ± 5)% increase in H2 compared to the porphyrin alone, followed by M7H ((45 ± 7)%) and WT ((72 ± 7)%, p = 0.64 for the M7H and WT comparison), and finally H102A with the highest increase in activity [(113 ± 5)%]. The assay was repeated in the absence of CO2 (Figure 3 and SI, Supplementary Figure S6), and yielded similar TON values for H2, indicating that overall H2 production is not affected by the presence of CO2. Methionine coordination at position seven results in a higher overall activity, as mutants bearing this residue (WT, H102A) resulted in the highest TON values. A possible explanation of these observations is that as the metal center becomes more electron rich, a softer ligand—such as methionine’s thioether side chain—is capable of better stabilizing the reduced intermediates that are formed throughout the catalytic cycle. Finally, the difference between the activities of H102A and WT could be due to the opening of a coordination site on one side of the porphyrin, thus increasing the chance of substrate binding to the active site. The near identical TONs observed for CO production as opposed to the variability observed for proton reduction suggests that the protein scaffold may provide proton relay pathways toward the active site. The rate limiting step of CO2 reduction to CO does not involve protonation steps, thus not benefiting in the same manner from the different proton environments as the H2-production cycle (Supplementary Figure S3).
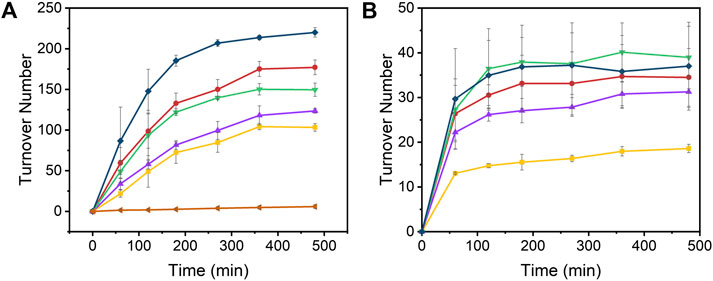
FIGURE 2. Turnover number values of (A) H2 and (B) CO over time from the photoinduced reduction of protons by [Ru(bpy)3]2+, CoPPIX, and Co-cyt b562 mutants at pH 6.0 under 1 atm CO2. The experiments were carried out in 100 mM ascorbic acid, 1 mM [Ru(bpy)3]2+, 200 mM potassium phosphate, 20 µM CoPPIX (except in the negative control), and 30 µM of corresponding apo cyt b562 (except for free CoPPIX; apo WT cyt b562 was used for the negative control) under white LED light irradiation. Colors represent [Ru(bpy)3]2+ (negative control, orange side triangles), free CoPPIX (yellow squares), WT (red circles), M7A (purple up triangles), M7H (green down triangles), and H102A (dark blue diamonds). The TON for the negative control was calculated assuming a hypothetical catalyst content identical to the other samples (8 nmol) for direct comparison. The experiments were run on triplicate and error bars represent the standard deviation of the sample.

TABLE 2. Turnover numbers for the observed products under light irradiation in 200 mM potassium phosphate, 100 mM ascorbic acid, 1 mM [Ru(bpy)3]2+, and 1 atm of gas.
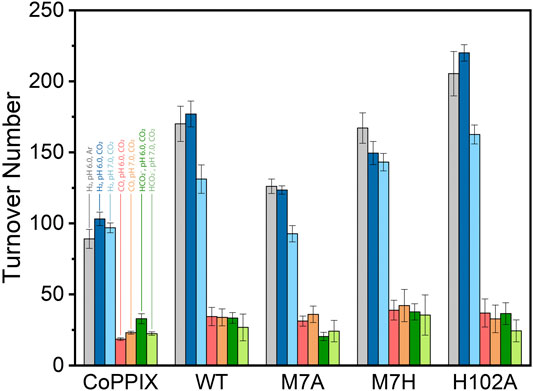
FIGURE 3. Turnover number values obtained for CoPPIX and cobalt cyt b562 mutants under irradiation with light for 8 h in 100 mM ascorbic acid, 1 mM [Ru(bpy)3]2+, and 200 mM potassium phosphate. The bars represent the TON of each catalyst at pH 6.0 in absence (H2: gray) and presence of CO2 (H2: dark blue, CO: red, HCO2−: dark green); and in the presence of CO2 at pH 7 (H2: light blue, CO: orange, HCO2−: light green). The experiments were run on triplicate and error bars represent the standard deviation of the sample.
The turnover numbers relative to CO production measured at pH 7.0 were generally unchanged for the holoprotein, while a slight increase was recorded for CoPPIX. Unsurprisingly the H2 yield for WT, M7A, and H102A decreased by 20%, as proton concentration is decreased 10-fold. However, no statistically significant change was observed for the H2 TON of the free porphyrin or the M7H mutant. Finally, a decrease in formate production was observed for the free porphyrin, consistent with the fact that its formation is dependent on proton concentration. The formate TON values for the holo proteins were not statistically different to those obtained at pH 6.0, suggesting that the protein environment might serve as a proton source throughout this particular catalytic route, thus mitigating the effect of the lower proton concentration in solution.
Conclusion
We have investigated the effect of the primary coordination sphere provided by the protein environment of cobalt cytochrome b562 in the light-promoted CO2 reduction pathway in mild aqueous conditions. Our results indicate that the native CO2 reduction activity of CoPPIX is increased when incorporated into the protein, whereas HCO2− production is not affected by the protein scaffold. Additionally, proton reduction showed a difference in activities between the mutants, suggesting that both electronic and steric effects play an important role throughout the catalytic cycle that leads to H2 production. Three of our protein scaffolds (WT, M7A, H102A) displayed pH dependance of proton reduction activity as expected, whereas M7H and CoPPIX did not show any noticeable change in activity at the examined pH values. No pH dependence of CO2 reduction activity was observed between the catalysts, indicating that other factors may come into play in the mechanism of CO2 reduction by these catalysts.
This work illustrates the first example of light driven carbon dioxide reduction by a protein-cobalt porphyrin catalyst, and demonstrates that hybrid metalloenzymes can be generated by incorporating CoPPIX into cyt b562; in water, the novel enzyme yields CO, formate, and hydrogen. Hydrogen production activity is modulated by mutating residues involved in the first coordination sphere of the porphyrin; in contrast, the mutations investigated here do not affect significantly CO2 reduction. Further work explanding mutations in the first and second coordination sphere could provide insight into the mechanism(s) of photoinduced reduction of H+ and CO2, which in turn could result in better catalysts for renewable energy and green chemistry.
Data Availability Statement
The original contributions presented in the study are included in the article/Supplementary Material, further inquiries can be directed to the corresponding author.
Author Contributions
RA-T, DS, and GG conceived the idea and designed the experiments. RA-T, DS, and NG performed the experiments. RA-T, NH, and GG analyzed the data. RA-T and GG wrote the article.
Funding
This work was supported by NSF SusChEM under Award 1508301 to GG.
Conflict of Interest
The authors declare that the research was conducted in the absence of any commercial or financial relationships that could be construed as a potential conflict of interest.
Acknowledgments
The authors would like to thank Brian Cherry (ASU) for help with setting up the NMR quantification of formate, and Dr. Kirtland J. Robinson for helpful discussions. The content of this manuscript has been published in part as part of the thesis of RA-T (Alcala-Torano, 2019).
Supplementary Material
The Supplementary Material for this article can be found online at: https://www.frontiersin.org/articles/10.3389/fmolb.2021.609654/full#supplementary-material.
References
Alcala-Torano, R. (2019). Rational metalloprotein design for energy conversion applications. Dissertation, Tempe (AZ): Arizona State University.
Alcala-Torano, R., Sommer, D. J., Bahrami Dizicheh, Z., and Ghirlanda, G. (2016). in Chapter seventeen—design strategies for redox active metalloenzymes: applications in hydrogen production. Methods in Enzymology. Editor V. L. Pecoraro (Academic Press), 389–416.
Alvarez-Hernandez, J. L., Sopchak, A. E., and Bren, K. L. (2020). Buffer pKa impacts the mechanism of hydrogen evolution catalyzed by a cobalt porphyrin-peptide. Inorg. Chem. 59 (12), 8061–8069. doi:10.1021/acs.inorgchem.0c00362
Azcarate, I., Costentin, C., Robert, M., and Savéant, J.-M. (2016a). Dissection of electronic substituent effects in multielectron-multistep molecular catalysis. Electrochemical CO2-to-CO conversion catalyzed by iron porphyrins. J. Phys. Chem. C. 120 (51), 28951–28960. doi:10.1021/acs.jpcc.6b09947
Azcarate, I., Costentin, C., Robert, M., and Savéant, J.-M. (2016b). Through-space charge interaction substituent effects in molecular catalysis leading to the design of the most efficient catalyst of CO2-to-CO electrochemical conversion. J. Am. Chem. Soc. 138 (51), 16639–16644. doi:10.1021/jacs.6b07014
Bhugun, I., Lexa, D., and Savéant, J.-M. (1996). Catalysis of the electrochemical reduction of carbon dioxide by iron(0) porphyrins: synergystic effect of weak brönsted acids. J. Am. Chem. Soc. 118 (7), 1769–1776. doi:10.1021/ja9534462
Birdja, Y. Y., Vos, R. E., Wezendonk, T. A., Jiang, L., Kapteijn, F., and Koper, M. T. M. (2018). Effects of substrate and polymer encapsulation on CO2 electroreduction by immobilized indium(III) protoporphyrin. ACS Catal. 8 (5), 4420–4428. doi:10.1021/acscatal.7b03386
Boston, D. J., Xu, C., Armstrong, D. W., and MacDonnell, F. M. (2013). Photochemical reduction of carbon dioxide to methanol and formate in a homogeneous system with pyridinium catalysts. J. Am. Chem. Soc. 135 (44), 16252–16255. doi:10.1021/ja406074w
Bowen, B. J., McGarrity, A. R., Szeto, J.-Y. A., Pudney, C. R., and Jones, D. D. (2020). Switching protein metalloporphyrin binding specificity by design from iron to fluorogenic zinc. Chemical Commun. 56 (31), 4308–4311. doi:10.1039/D0CC00596G
Burke, A. J., Lovelock, S. L., Frese, A., Crawshaw, R., Ortmayer, M., Dunstan, M., et al. (2019). Design and evolution of an enzyme with a non-canonical organocatalytic mechanism. Nature 570 (7760), 219–223. doi:10.1038/s41586-019-1262-8
Call, A., Casadevall, C., Romero-Rivera, A., Martin-Diaconescu, V., Sommer, D. J., Osuna, S., et al. (2019a). Improved electro- and photocatalytic water reduction by confined cobalt catalysts in streptavidin. ACS Catal. 9 (7), 5837–5846. doi:10.1021/acscatal.8b04981
Call, A., Cibian, M., Yamamoto, K., Nakazono, T., Yamauchi, K., and Sakai, K. (2019b). Highly efficient and selective photocatalytic CO2 reduction to CO in water by a cobalt porphyrin molecular catalyst. ACS Catal. 9 (6), 4867–4874. doi:10.1021/acscatal.8b04975
Chakrabartty, A., Schellman, J. A., and Baldwin, R. L. (1991). Large differences in the helix propensities of alanine and glycine. Nature 351 (6327), 586–588. doi:10.1038/351586a0
Chen, K., and Arnold, F. H. (2020). Engineering new catalytic activities in enzymes. Nature Catal. 3 (3), 203–213. doi:10.1038/s41929-019-0385-5
Costentin, C., Drouet, S., Robert, M., and Savéant, J.-M. (2012). A local proton source enhances CO2; electroreduction to CO by a molecular Fe catalyst. Science 338 (6103), 90. doi:10.1126/science.1224581
Davis, H. J., and Ward, T. R. (2019). Artificial metalloenzymes: challenges and opportunities. ACS Cent. Sci. 5 (7), 1120–1136. doi:10.1021/acscentsci.9b00397
Dhanasekaran, T., Grodkowski, J., Neta, P., Hambright, P., and Fujita, E. (1999). p-Terphenyl-Sensitized photoreduction of CO2 with cobalt and iron porphyrins. interaction between CO and reduced metalloporphyrins. J. Phys. Chem. 103 (38), 7742–7748. doi:10.1021/jp991423u
Drienovská, I., and Roelfes, G. (2020). Expanding the enzyme universe with genetically encoded unnatural amino acids. Nature Catalysis. 3 (3), 193–202. doi:10.1038/s41929-019-0410-8
Drienovská, I., Scheele, R. A., Gutiérrez de Souza, C., and Roelfes, G. (2020). A hydroxyquinoline-based unnatural amino acid for the design of novel artificial metalloenzymes. Chembiochem. 21, 3077–3081. doi:10.1002/cbic.202000306
Edgcomb, S. P., and Murphy, K. P. (2002). Variability in the pKa of histidine side-chains correlates with burial within proteins. Proteins: Structure, Function, and Bioinformatics. 49 (1), 1–6. 10.1002/prot.10177
Edwards, E. H., and Bren, K. L. (2020). Light-driven catalysis with engineered enzymes and biomimetic systems. Biotechnol. Appl. Biochem. 67, 463–483. doi:10.1002/bab1976
Feng, L., Wang, K.-Y., Joseph, E., and Zhou, H.-C. (2020). Catalytic porphyrin framework compounds. Trends Chem. 2 (6), 555–568. doi:10.1016/j.trechm.2020.01.003
Feng, Y., Sligar, S. G., and Wand, A. J. (1994). Solution structure of apocytochrome b562. Nat. Struct. Biol. 1 (1), 30–35. doi:10.1038/nsb0194-30
Firpo, V., Le, J. M., Pavone, V., Lombardi, A., and Bren, K. L. (2018). Hydrogen evolution from water catalyzed by cobalt-monochrome VI*a, a synthetic mini-protein. Chem. Sci. 9 (45), 8582–8589. doi:10.1039/C8SC01948G
Fukuzumi, S., Lee, Y.-M., and Nam, W. (2019). Photocatalytic redox reactions with metalloporphyrins. J. Porphyr. Phthalocyanines 24 (01n03), 21–32. doi:10.1142/S1088424619300131
Hay, S., and Wydrzynski, T. (2005). Conversion of the Escherichia coli cytochrome b562 to an archetype cytochrome b: A mutant with bis-histidine ligation of heme iron. Biochemistry 44 (1), 431–439. doi:10.1021/bi0492298
Hulme, E. C., and Trevethick, M. A. (2010). Ligand binding assays at equilibrium: validation and interpretation. Br. J. Pharmacol. 161 (6), 1219–1237. doi:10.1111/j.1476-5381.2009.00604.x
Jeong, W. J., Yu, J., and Song, W. J. (2020). Proteins as diverse, efficient, and evolvable scaffolds for artificial metalloenzymes. Chemical Commun. 56 (67), 9586–9599. doi:10.1039/D0CC03137B
Kamiya, N., Okimoto, Y., Ding, Z., Ohtomo, H., Shimizu, M., Kitayama, A., et al. (2001). How does heme axial ligand deletion affect the structure and the function of cytochrome b562? Protein Eng. Des. Sel. 14 (6), 415–419. doi:10.1093/protein/14.6.415
Kleingardner, J. G., Kandemir, B., and Bren, K. L. (2014). Hydrogen evolution from neutral water under aerobic conditions catalyzed by cobalt microperoxidase-11. J. Am. Chem. Soc. 136 (1), 4–7. doi:10.1021/ja406818h
Kondo, M., Ichii, K., Patra, P. K., Poulter, B., Calle, L., Koven, C., et al. (2018). Plant regrowth as a driver of recent enhancement of terrestrial CO2 uptake. Geophys. Res. Lett. 45 (10), 4820–4830. doi:10.1029/2018GL077633
Kornienko, N., Zhao, Y., Kley, C. S., Zhu, C., Kim, D., Lin, S., et al. (2015). Metal-organic frameworks for electrocatalytic reduction of carbon dioxide. J. Am. Chem. Soc. 137 (44), 14129–14135. doi:10.1021/jacs.5b08212
Kovacs, E., Tóth, J., Vértessy, B. G., and Liliom, K. (2010). Dissociation of calmodulin-target peptide complexes by the lipid mediator sphingosylphosphorylcholine: implications in calcium signaling. J. Biol. Chem. 285 (3), 1799–1808. doi:10.1074/jbc.M109.053116
Kramer, W. W., and McCrory, C. C. L. (2016). Polymer coordination promotes selective CO2 reduction by cobalt phthalocyanine. Chem. Sci. 7 (4), 2506–2515. doi:10.1039/C5SC04015A
Lacis, A. A., Schmidt, G. A., Rind, D., and Ruedy, R. A. (2010). Atmospheric CO2: principal control knob governing earth's temperature. Science 330 (6002), 356. doi:10.1126/science.1190653
Laureanti, J. A., Ginovska, B., Buchko, G. W., Schenter, G. K., Hebert, M., Zadvornyy, O. A., et al. (2020). A positive charge in the outer coordination sphere of an artificial enzyme increases CO2 hydrogenation. Organometallics 39 (9), 1532–1544. doi:10.1021/acs.organomet.9b00843
Le, J. M., Alachouzos, G., Chino, M., Frontier, A. J., Lombardi, A., and Bren, K. L. (2020). Tuning mechanism through buffer dependence of hydrogen evolution catalyzed by a cobalt mini-enzyme. Biochemistry 59 (12), 1289–1297. doi:10.1021/acs.biochem.0c00060
Le, J. M., and Bren, K. L. (2019). Engineered enzymes and bioinspired catalysts for energy conversion. ACS Energy Letters. 4 (9), 2168–2180. doi:10.1021/acsenergylett.9b01308
Lee, C. H., Dogutan, D. K., and Nocera, D. G. (2011). Hydrogen generation by hangman metalloporphyrins. J. Am. Chem. Soc. 133 (23), 8775–8777. doi:10.1021/ja202136y
Leung, K., Nielsen, I. M. B., Sai, N., Medforth, C., and Shelnutt, J. A. (2010). Cobalt-porphyrin catalyzed electrochemical reduction of carbon dioxide in water. 2. mechanism from first principles. J. Phys. Chem. 114 (37), 10174–10184. doi:10.1021/jp1012335
Liang, A. D., Serrano-Plana, J., Peterson, R. L., and Ward, T. R. (2019). Artificial metalloenzymes based on the biotin–streptavidin technology: enzymatic cascades and directed evolution. Acc. Chem. Res. 52 (3), 585–595. doi:10.1021/acs.accounts.8b00618
Lin, S., Diercks, C. S., Zhang, Y.-B., Kornienko, N., Nichols, E. M., Zhao, Y., et al. (2015). Covalent organic frameworks comprising cobalt porphyrins for catalytic CO2 reduction in water. Science 349 (6253), 1208. doi:10.1126/science.aac8343
Liu, X., Kang, F., Hu, C., Wang, L., Xu, Z., Zheng, D., et al. (2018). A genetically encoded photosensitizer protein facilitates the rational design of a miniature photocatalytic CO2-reducing enzyme. Nat. Chem. 10 (12), 1201–1206. doi:10.1038/s41557-018-0150-4
Liu, Y., and McCrory, C. C. L. (2019). Modulating the mechanism of electrocatalytic CO2 reduction by cobalt phthalocyanine through polymer coordination and encapsulation. Nat. Commun. 10 (1), 1683. doi:10.1038/s41467-019-09626-8
Lu, Y., Yeung, N., Sieracki, N., and Marshall, N. M. (2009). Design of functional metalloproteins. Nature 460, 855. doi:10.1038/nature08304
Luan, G., and Lu, X. (2018). Tailoring cyanobacterial cell factory for improved industrial properties. Biotechnol. Adv. 36 (2), 430–442. doi:10.1016/j.biotechadv.2018.01.005
Ma, B., Chen, G., Fave, C., Chen, L., Kuriki, R., Maeda, K., et al. (2020). Efficient visible-light-driven CO2 reduction by a cobalt molecular catalyst covalently linked to mesoporous carbon nitride. J. Am. Chem. Soc. 142 (13), 6188–6195. doi:10.1021/jacs.9b13930
Manbeck, G. F., and Fujita, E. (2015). A review of iron and cobalt porphyrins, phthalocyanines and related complexes for electrochemical and photochemical reduction of carbon dioxide. J. Porphyr. Phthalocyanines 19 (01-03), 45–64. doi:10.1142/S1088424615300013
Markel, U., Essani, K. D., Besirlioglu, V., Schiffels, J., Streit, W. R., and Schwaneberg, U. (2020). Advances in ultrahigh-throughput screening for directed enzyme evolution. Chem. Soc. Rev. 49 (1), 233–262. doi:10.1039/C8CS00981C
Mondal, B., Rana, A., Sen, P., and Dey, A. (2015). Intermediates involved in the 2e−/2H+ reduction of CO2 to CO by iron(0) porphyrin. J. Am. Chem. Soc. 137 (35), 11214–11217. doi:10.1021/jacs.5b05992
Morris, A. J., Meyer, G. J., and Fujita, E. (2009). Molecular approaches to the photocatalytic reduction of carbon dioxide for solar fuels. Acc. Chem. Res. 42 (12), 1983–1994. doi:10.1021/ar9001679
Nastri, F., D’Alonzo, D., Leone, L., Zambrano, G., Pavone, V., and Lombardi, A. (2019). Engineering metalloprotein functions in designed and native scaffolds. Trends Biochem. Sci. 44 (12), 1022–1040. doi:10.1016/j.tibs.2019.06.006
Nielsen, I. M. B., and Leung, K. (2010). Cobalt-porphyrin catalyzed electrochemical reduction of carbon dioxide in water. 1. A density functional study of intermediates. J. Phys. Chem. 114 (37), 10166–10173. doi:10.1021/jp101180m
Onoda, A., Kihara, Y., Fukumoto, K., Sano, Y., and Hayashi, T. (2014). Photoinduced hydrogen evolution catalyzed by a synthetic diiron dithiolate complex embedded within a protein matrix. ACS Catal. 4 (8), 2645–2648. doi:10.1021/cs500392e
Oohora, K., Onoda, A., and Hayashi, T. (2019). Hemoproteins reconstituted with artificial metal complexes as biohybrid catalysts. Acc. Chem. Res. 52 (4), 945–954. doi:10.1021/acs.accounts.8b00676
Pace, C. N., and Scholtz, J. M. (1998). A helix propensity scale based on experimental studies of peptides and proteins. Biophys. J. 75 (1), 422–427. 10.1016/S0006-3495(98)77529-0
Pia, E. A. D., Chi, Q., Elliott, M., Emyr Macdonald, J., Ulstrup, J., and Dafydd Jones, D. (2012). Redox tuning of cytochrome b562 through facile metal porphyrin substitution. Chemical Communications 48 (86), 10624–10626. doi:10.1039/C2CC34302A
Rao, H., Lim, C.-H., Bonin, J., Miyake, G. M., and Robert, M. (2018). Visible-light-driven conversion of CO2 to CH4 with an organic sensitizer and an iron porphyrin catalyst. J. Am. Chem. Soc. 140 (51), 17830–17834. doi:10.1021/jacs.8b09740
Roy, A., Madden, C., and Ghirlanda, G. (2012). Photo-induced hydrogen production in a helical peptide incorporating a [FeFe] hydrogenase active site mimic. Chemical Commun. 48 (79), 9816–9818. doi:10.1039/C2CC34470J
Roy, A., Vaughn, M. D., Tomlin, J., Booher, G. J., Kodis, G., Simmons, C. R., et al. (2020). Enhanced photocatalytic hydrogen production by hybrid streptavidin-diiron catalysts. Chemistry 26 (28), 6240–6246. doi:10.1002/chem.202000204
Sano, Y., Onoda, A., and Hayashi, T. (2011). A hydrogenase model system based on the sequence of cytochrome c: photochemical hydrogen evolution in aqueous media. Chemical Commun. 47 (29), 8229–8231. doi:10.1039/C1CC11157D
Schneider, C. R., Manesis, A. C., Stevenson, M. J., and Shafaat, H. S. (2018). A photoactive semisynthetic metalloenzyme exhibits complete selectivity for CO2 reduction in water. Chemical Commun. 54 (37), 4681–4684. doi:10.1039/C8CC01297K
Schneider, C. R., and Shafaat, H. S. (2016). An internal electron reservoir enhances catalytic CO2 reduction by a semisynthetic enzyme. Chemical Commun. 52 (64), 9889–9892. doi:10.1039/C6CC03901D
Schwizer, F., Okamoto, Y., Heinisch, T., Gu, Y., Pellizzoni, M. M., Lebrun, V., et al. (2018). Artificial metalloenzymes: reaction scope and optimization strategies. Chem. Rev. 118 (1), 142–231. doi:10.1021/acs.chemrev.7b00014
Shen, J., Kolb, M. J., Göttle, A. J., and Koper, M. T. M. (2016). DFT study on the mechanism of the electrochemical reduction of CO2 catalyzed by cobalt porphyrins. J. Phys. Chem. C 120 (29), 15714–15721. doi:10.1021/acs.jpcc.5b10763
Slater, J. W., Marguet, S. C., Gray, M. E., Monaco, H. A., Sotomayor, M., and Shafaat, H. S. (2019). Power of the secondary sphere: modulating hydrogenase activity in nickel-substituted rubredoxin. ACS Catal. 8928–8942. doi:10.1021/acscatal.9b01720
Smith, P., Davis, S. J., Creutzig, F., Fuss, S., Minx, J., Gabrielle, B., et al. (2015). Biophysical and economic limits to negative CO2 emissions. Nat. Clim. Change 6, 42. doi:10.1038/nclimate2870
Sommer, D. J., Vaughn, M. D., Clark, B. C., Tomlin, J., Roy, A., and Ghirlanda, G. (2016). Reengineering cyt b562 for hydrogen production: a facile route to artificial hydrogenases. Biochim. Biophys. Acta Bioenerg. 1857 (5), 598–603. doi:10.1016/j.bbabio.2015.09.001
Sommer, D. J., Vaughn, M. D., and Ghirlanda, G. (2014). Protein secondary-shell interactions enhance the photoinduced hydrogen production of cobalt protoporphyrin IX. Chemical Commun. 50 (100), 15852–15855. doi:10.1039/C4CC06700B
Sun, Y., Gu, L., Dickinson, R. E., Norby, R. J., Pallardy, S. G., and Hoffman, F. M. (2014). Impact of mesophyll diffusion on estimated global land CO2 fertilization. Proc. Natl. Acad. Sci. Unit. States Am. 111, 15774–15779. doi:10.1073/pnas.1418075111
Thordarson, P. (2011). Determining association constants from titration experiments in supramolecular chemistry. Chem. Soc. Rev. 40 (3), 1305–1323. doi:10.1039/C0CS00062K
Vornholt, T., Christoffel, F., Pellizzoni, M. M., Panke, S., Ward, T. R., and Jeschek, M. (2020). Systematic engineering of artificial metalloenzymes for new-to-nature reactions, bioRxiv. 2007, 204206. doi:10.1101/2020.07.15.204206
Walsh, A. P., Laureanti, J. A., Katipamula, S., Chambers, Geoffrey. M., Priyadarshani, N., Lense, S., et al. (2019). Evaluating the impacts of amino acids in the second and outer coordination spheres of Rh-bis(diphosphine) complexes for CO2 hydrogenation. Faraday Discuss 215 (0), 123–140. doi:10.1039/C8FD00164B
Woo, H. M. (2017). Solar-to-chemical and solar-to-fuel production from CO2 by metabolically engineered microorganisms. Curr. Opin. Biotechnol. 45, 1–7. doi:10.1016/j.copbio.2016.11.017
Wu, Q., Xie, R.-K., Mao, M.-J., Chai, G.-L., Yi, J.-D., Zhao, S.-S., et al. (2020). Integration of strong electron transporter tetrathiafulvalene into metalloporphyrin-based covalent organic framework for highly efficient electroreduction of CO2. ACS Energy Letters 5 (3), 1005–1012. doi:10.1021/acsenergylett.9b02756
Yehezkeli, O., Bedford, N. M., Park, E., Ma, K., and Cha, J. N. (2016). Semiconductor-based, solar-driven photochemical cells for fuel generation from carbon dioxide in aqueous solutions. ChemSusChem. 9 (22), 3188–3195. doi:10.1002/cssc.201601105
Zhang, H., Wei, J., Dong, J., Liu, G., Shi, L., An, P., et al. (2016). Efficient visible-light-driven carbon dioxide reduction by a single-atom implanted metal-organic framework. Angew. Chem. Int. Ed. 55 (46), 14310–14314. doi:10.1002/anie.201608597
Zhang, X.-D., Hou, S.-Z., Wu, J.-X., and Gu, Z.-Y. (2020). Two-dimensional metal–organic framework nanosheets with cobalt-porphyrins for high-performance CO2 electroreduction. Chem. Eur J. 26 (7), 1604–1611. doi:10.1002/chem.201904072
Zhang, X., Wu, Z., Zhang, X., Li, L., Li, Y., Xu, H., et al. (2017). Highly selective and active CO2 reduction electrocatalysts based on cobalt phthalocyanine/carbon nanotube hybrid structures. Nat. Commun. 8, 14675. doi:10.1038/ncomms14675
Keywords: cobalt porphyrin, Carbon fixation, CO2 reduction, catalysis, protein design, proton reduction
Citation: Alcala-Torano R, Halloran N, Gwerder N, Sommer DJ and Ghirlanda G (2021) Light-Driven CO2 Reduction by Co-Cytochrome b562. Front. Mol. Biosci. 8:609654. doi: 10.3389/fmolb.2021.609654
Received: 23 September 2020; Accepted: 11 January 2021;
Published: 15 April 2021.
Edited by:
Nikolaos E. Labrou, Agricultural University of Athens, GreeceReviewed by:
Kamlendra Singh, Karolinska Institute, SwedenChristopher Berndsen, James Madison University, United States
Copyright © 2021 Alcala-Torano, Halloran, Gwerder, Sommer and Ghirlanda. This is an open-access article distributed under the terms of the Creative Commons Attribution License (CC BY). The use, distribution or reproduction in other forums is permitted, provided the original author(s) and the copyright owner(s) are credited and that the original publication in this journal is cited, in accordance with accepted academic practice. No use, distribution or reproduction is permitted which does not comply with these terms.
*Correspondence: Giovanna Ghirlanda, Z2doaXJsYW5kYUBhc3UuZWR1