- 1Division of Respiratory Disease, Department of Internal Medicine, The Jikei University School of Medicine, Tokyo, Japan
- 2Department of Translational Research for Exosomes, The Jikei University School of Medicine, Tokyo, Japan
Respiratory diseases and their comorbidities, such as cardiovascular disease and muscle atrophy, have been increasing in the world. Extracellular vesicles (EVs), which include exosomes and microvesicles, are released from almost all cell types and play crucial roles in intercellular communication, both in the regulation of homeostasis and the pathogenesis of various diseases. Exosomes are of endosomal origin and range in size from 50 to 150 nm in diameter, while microvesicles are generated by the direct outward budding of the plasma membrane in size ranges of 100–2,000 nm in diameter. EVs can contain various proteins, metabolites, and nucleic acids, such as mRNA, non-coding RNA species, and DNA fragments. In addition, these nucleic acids in EVs can be functional in recipient cells through EV cargo. The endothelium is a distributed organ of considerable biological importance, and disrupted endothelial function is involved in the pathogenesis of respiratory diseases such as chronic obstructive pulmonary disease, pulmonary hypertension, and acute respiratory distress syndrome. Endothelial cell-derived EVs (EC-EVs) play crucial roles in both physiological and pathological conditions by traveling to distant sites through systemic circulation. This review summarizes the pathological roles of vascular microRNAs contained in EC-EVs in respiratory diseases, mainly focusing on chronic obstructive pulmonary disease, pulmonary hypertension, and acute respiratory distress syndrome. Furthermore, this review discusses the potential clinical usefulness of EC-EVs as therapeutic agents in respiratory diseases.
Introduction
Respiratory diseases are leading causes of death and disability in the world (Soriano et al., 2020). Noxious agents such as cigarette smoke and air pollution are the causes and aggravating factors of a variety of respiratory diseases (Renzoni et al., 2014; Vij et al., 2018). They impose an immense worldwide health burden, with hundreds of millions of people suffering from respiratory diseases each year (Soriano et al., 2020). Therefore, there is an urgent need to elucidate the pathogenesis of respiratory diseases and to develop novel therapies to treat them. To date, a number of studies have demonstrated the involvement of the vascular endothelium in the pathogenesis of respiratory diseases (Dai et al., 2018; Huertas et al., 2018; Leligdowicz et al., 2018). Pulmonary vascular endothelium is strategically located to separate blood from air and to filter the blood before it enters systemic circulation. Then, endothelial cells (ECs) play essential roles in maintaining lung homeostasis by optimizing gas exchange, reducing vascular tone, coordinating of blood flow control, and modulating hypoxic vasoconstriction (Cahill and Redmond, 2016; Hsia et al., 2016). Moreover, extensive researches have confirmed that vascular endothelium is a highly specialized metabolically active organ possessing numerous physiological, immunological, and synthetic functions (Colbert and Schmidt, 2016; Wong et al., 2017). Among the various important functions of vascular endothelium is the secretion of extracellular vesicles (EVs), which are key players in intercellular communication (Fujita et al., 2016). EVs, which include exosomes and microvesicles, are released from almost all cell types. EVs contain various compositions of nucleic acids, namely mRNAs, microRNAs (miRNAs), and DNA fragments, in addition to proteins and lipids (Kubo, 2018). The most common proteins that compose EVs are tetraspanins (CD9, CD63, CD81, and CD82), which are a protein superfamily that interacts with many protein partners including major histocompatibility complex (MHC) molecules and integrins. Tetraspanins are involved in the organization of functional molecular complexes called tetraspanin-enriched microdomains (Willms et al., 2018). EVs also contain heat shock proteins (Hsp70 and Hsp90) that associate with antigen presentation (Zhang et al., 2019b). Membrane-associated proteins (annexins, GTPases, and flotillin), multivesicular bodies (MVBs) synthesis proteins (ALG-2-interacting protein X (Alix), tumor susceptibility gene 101 (Tsg101)), and major MHC classes I and II are also present in EVs from most cell types (Hessvik and Llorente, 2018). Furthermore, the lipid composition of EVs reveals sphingomyelin, cholesterol, ceramide, and glycophospholipids (Skotland et al., 2019). EV compositions have the ability to play important roles in homeostasis and pathological processes, such as angiogenesis, inflammation, and senescence (Fujita et al., 2015b; Andrews and Rizzo, 2016; Takasugi, 2018). Notably, EVs derived from ECs (EC-EVs) have attracted significant attention as biological mediators and potential therapeutic targets in respiratory diseases (Kadota et al., 2016; Lanyu and Feilong, 2019). However, the precise relevance is incompletely understood. In this review, we summarize the pathological roles of vascular miRNAs contained in EC-EVs in respiratory diseases, mainly focusing on chronic obstructive pulmonary disease (COPD), pulmonary hypertention (PH), and acute respiratory distress syndrome (ARDS). Moreover, we also discuss the potential clinical usefulness of EVs as therapeutic agents in respiratory diseases.
Extracellular Vesicles
In recent decades, EVs have emerged as essential players of intercellular communication. Membrane-bound vesicles outside cells were first identified in mice 50 years ago (Anderson, 1969). Pan B. T. and Johnstone R. M. reported that small vesicles are released from sheep reticulocytes in 1983 and they proposed to define such small vesicles as exosomes in 1987 (Pan and Johnstone, 1983; Johnstone et al., 1987). In 2007 and 2010, exosomes were reported to contain both mRNAs and miRNAs, which can be delivered to other cells and can function in their new location (Valadi et al., 2007; Kosaka et al., 2010). Following these studies, numerous additional EV studies have been conducted in various fields, including lung disease pathogenesis (Fujita et al., 2015a; Genschmer et al., 2019; Kadota et al., 2020). EVs are released into the extracellular environment by almost all cell types, including epithelial cells, fibroblasts, ECs, cancer cells, stem cells, and immune cells (Whiteside, 2018; Li et al., 2019; Mohan et al., 2020). Moreover, EVs have been isolated from diverse bodily fluids, including blood, urine (Street et al., 2017), saliva (Machida et al., 2015), breast milk (Qin et al., 2016), bronchoalveolar lavage fluid (Admyre et al., 2003), semen (Madison et al., 2015), amniotic fluid (Dixon et al., 2018), ascites (Yun et al., 2019), and sputum (Njock et al., 2019). Therefore, EVs reflect various pathological conditions, and have attracted attention as a biomarker for lung diseases (Kadota et al., 2017).
The two major categories of EVs include exosomes and microvesicles (also referred to as ectosomes and microparticles), which are classified on the basis of their biogenesis and secretion mechanisms (Raposo and Stoorvogel, 2013). Exosomes are of endosomal origin and have a size range of 50-150 nm in diameter. Exosomes are generated through a process that involves double invagination of the plasma membrane and the formation of intracellular MVBs containing intraluminal vesicles, which are released into the extracellular space by the fusion of the peripheral membrane of the MVBs with the plasma membrane (Kalluri and LeBleu, 2020). Exosomes can deliver their components to target cells through clathrin- or caveolin-dependent endocytosis, phagocytosis, micropinocytosis, lipid raft-mediated internalization, and membrane fusion (Mulcahy et al., 2014). This results in the delivery of proteins and RNAs into the membrane or cytosol of the recipient cell, and the transfer of this material can regulate gene expression and fate decisions of target cells (Lakkaraju and Rodriguez-Boulan, 2008). By contrast, microvesicles are vesicles generated by the direct outward budding of the plasma membrane with a size range of 100-2000 nm in diameter. Microvesicles are rich in phosphatidylserine, and their basic cargo includes membrane components similar to those of the parent cell membrane (Hargett and Bauer, 2013). Although the origins of exosomes and microvesicles have been defined, current technologies cannot reliably separate EVs (Merchant et al., 2017). The International Society for Extracellular Vesicles (ISEV) advocates “extracellular vesicle” (EV) as the generic term for particles released from the cell that are all lipid bilayer-enclosed extracellular structures (Thery et al., 2018). In this review, we use the term EVs.
The significance of EVs lies in their capacity to deliver their cargoes to various sites in the body and influence a wide variety of biological processes of the recipient cells (Fujita et al., 2016). Additionally, the compositions and functions of EVs are modulated in response to environmental stressors. Specifically, in the lung, it has been reported that hypoxia, infection, and cigarette smoke increase the numbers of EVs and alter their compositions (de Jong et al., 2012; Hargett and Bauer, 2013; Fujita et al., 2015a). Therefore, EVs are important paracrine mediators not only in homeostasis but also in disease pathogenesis. These properties suggest that EVs can be useful as biomarkers for the diagnosis, prognosis, and therapeutic response of various lung diseases.
Non-coding RNAs in EVs
A non-coding RNA (ncRNA) is a generic term for RNA that does not code for proteins (Fatima and Nawaz, 2017). NcRNAs can influence numerous molecular targets to drive specific cell biological responses and fates (Cech and Steitz, 2014; Anastasiadou et al., 2018b). In 1993, lin4, a first ncRNA that negatively regulates the level of LIN-14 protein, was discovered in a nematode (Lee et al., 1993). In 1998, RNA interference (RNAi), a sequence-specific suppression phenomenon induced by double-stranded RNA, was discovered (Hannon, 2002). In 2000, it was revealed that another ncRNA, let-7, is widely conserved in many species, including humans, which dramatically improves the recognition of short ncRNAs (Reinhart et al., 2000). To date, the development of next-generation sequencing and RNA sequencing has led to the discovery of a multitude of ncRNA species, such as small interfering RNAs (siRNAs), P-element-induced wimpy testes (piwi)-interacting RNAs (piRNAs), enhancer RNAs (eRNAs), miRNAs, and the long-noncoding RNAs (lncRNAs) (Cech and Steitz, 2014; Espinosa, 2016; Anastasiadou et al., 2018b). They have crucial roles in the regulation of the genome, from transcription into mRNA, to mRNA processing, to translation into proteins (Vencken et al., 2015). As a result, ncRNAs are involved in various processes, including cell proliferation, tissue differentiation, metabolic regulation, the cell cycle, apoptosis, and metastasis (Guo et al., 2014). Thus, dysregulation of ncRNAs may lead to dysfunctions and diseases (Anastasiadou et al., 2018a). It has been reported that EVs contain various types of functional ncRNAs (Xie et al., 2019). Here, we mainly focus on EV miRNAs that have been widely explored and have potential for use as biological mediators and therapeutic targets for various diseases.
MiRNAs are endogenously expressed, single-stranded RNAs that are approximately 18-25 nucleotides in length (Mohr and Mott, 2015). As mentioned above, miRNAs are important regulators in several biological processes. Indeed, the development and progression of various diseases have been associated with miRNA expressions (Rupani et al., 2013). The biogenesis of miRNAs takes place through a multi-step process, and miRNAs regulate gene expression via binding to the 3'-untranslated region of mRNAs to induce degradation and translational repression, thereby suppressing the expression of target genes (Bartel, 2018; Becker et al., 2019). One miRNA may target many mRNAs, and conversely, one mRNA may be regulated by several miRNAs (Matsuyama and Suzuki, 2019).
It has been reported that miRNAs can stably exist in bodily fluids (Salido-Guadarrama et al., 2014). Extracellular miRNAs are protected from decomposition and stabilized by being packed into EVs, loaded into high density lipoproteins, and bound to argonaute 2 protein, which is the key effector protein of miRNA-mediated silencing (Ha and Kim, 2014; Tabet et al., 2014). EV miRNA and mRNA profiles differ from those of the parental cells, suggesting that cells have an active selection mechanism for EV cargo (Zhang et al., 2017). The miRNAs are not randomly incorporated into EVs, because the proportion of miRNAs is higher in EVs than in parental cells, and EV miRNA expression levels are altered under different physiological conditions (Goldie et al., 2014). These results indicate that parental cells have a sorting mechanism that guides specific intracellular miRNAs to enter EVs (Zhang et al., 2015). Sorted miRNAs are delivered by EVs to recipient cells, regulating biological processes such as cell differentiation, proliferation, apoptosis, and metabolism (Kim et al., 2017). Therefore, EV miRNAs are associated with inflammatory conditions, metabolic disorders, malignancies, and autoimmune disorders (Adyshev et al., 2014). Although EVs have various functional compositions except miRNAs, EV miRNA cargo has the potential to regulate gene expression both locally and remotely, and are therefore drawing attention in various fields (Chen et al., 2017; O'Brien et al., 2018; Cavallari et al., 2019).
Endothelial Cell-Derived EVs in Endothelial Physiology
The vascular endothelium plays a wide variety of critical roles in the maintenance of vascular homeostasis (Pi et al., 2018). Vessels deliver metabolites and oxygen to tissues and export waste products to sustain the well-being of an organism (Helms et al., 2018). The endothelium is the monolayer of ECs lining the lumen of blood or lymph vessels, which are able to differentiate into arterial, venous, and lymphatic cells during development and act as a barrier between the blood or lymph and tissues (Hulshoff et al., 2019). The endothelium is also a distributed organ of considerable biological potential that not only serves as a barrier, but also perform other distinctive biologic functions (Rohlenova et al., 2018). In addition, the ECs interact with circulating cells and cells present in the vascular wall. Interestingly, ECs display functional heterogeneity in different tissues and organs, which suggests that ECs alter their function for adapting microenvironment (Kruger-Genge et al., 2019). In the lungs, ECs have a variety of physiological functions, including optimizing gas exchange, the reduction of vascular tone, the coordination of blood flow, and the modulation of hypoxic vasoconstriction (Suresh and Shimoda, 2016). ECs can shed EVs directly into the bloodstream and deliver them to distant sites (Hromada et al., 2017). Thus, the functions of ECs are partially performed through delivering EVs.
Several unique proteome and nucleic acid cargoes have been found in EC-EVs (Letsiou and Bauer, 2018). EC-EVs are defined according to the expression of endothelial membrane-specific antigens. CD62E (E-selectin) and CD144 (vascular endothelial (VE)-cadherin) are specific markers for EC-EVs (Dignat-George and Boulanger, 2011). Angiotensin converting enzyme (ACE) can also serve to identify specific EC-EVs subpopulations, as it is abundantly expressed in pulmonary capillary endothelium (Takei et al., 2019b). There are other common EC-EV markers, such as CD54 (ICAM-1: intercellular adhesion molecule 1), CD62P (P-selectin), CD31 (PECAM: platelet-endothelium cell adhesion molecule), CD105 (endoglin, a growth-related protein), and CD146 (MCAM: melanoma cell adhesion molecule) (Choi et al., 2015). Since these are also expressed in platelet-derived microparticles, EC-EV specificity is guaranteed by the absence of the platelet-specific glycoprotein Ib marker CD42b (Chironi et al., 2009).
Regarding the nucleic acid cargo of EC-EVs, an analysis of miRNAs in both EC-EVs and parental cells has demonstrated that different miRNAs are distributed differentially between cells and EVs (van Balkom et al., 2015). In this study, the 10 most abundant miRNAs in EVs are miR-10b, let-7i, miR-126, miR-100, miR-27b, miR-30a, miR-25, miR-221, miR-191, and miR-411. Among these, angiogenesis-related miRNAs, such as miR-126, miR-25, and miR-221, are higher in abundance in EVs than in cells. Data indicate that these miRNAs may be sorted within EVs on purpose. Mechanistically, miR-126-3p regulates angiogenesis via modulating angiogenic growth factors, such as vascular endothelial growth factor (VEGF) and fibroblast growth factor (FGF)-2, and promotes blood vessel formation by repressing the expression of sprouty-related protein-1 (Spred-1), an intracellular inhibitor of angiogenic signaling (Wang et al., 2008; Todorova et al., 2017). Furthermore, miR-25 and miR-221 affect EC proliferation (Nicoli et al., 2012; Mujahid et al., 2013; Zhang et al., 2019a). These miRNA cargos permit EC-EVs to play pivotal roles in regulating angiogenesis, possibly resulting in homeostatic control during endothelial physiology (Figure 1).
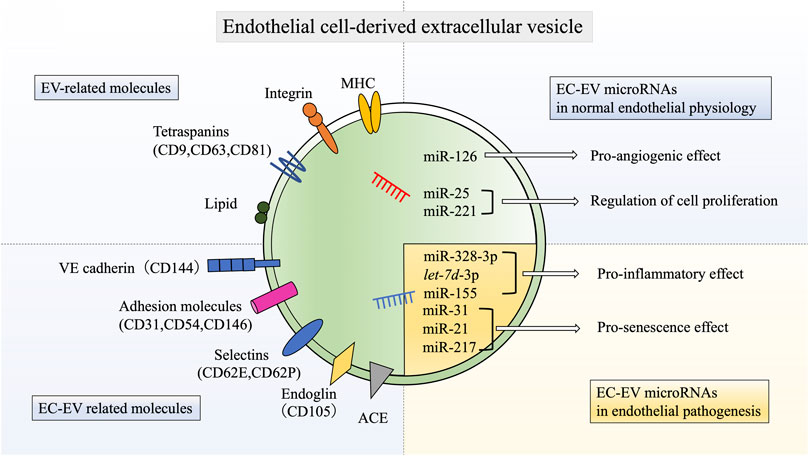
FIGURE 1. Schematic molecular components of endothelial cell-derived extracellular vesicles. EC-EVs have various EV-related molecules, such as tetraspanins, MHC, integrins, and lipids. EC-EVs also have unique endothelial markers, including VE-cadherin, E-selectin, P-selectin, PECAM, ICAM-1, MCAM, endoglin, and ACE. In addition, EC-EVs also contain various miRNAs that have biological functions in both normal endothelial physiology and pathogenesis. EC: endothelial cell, EV: extracellular vesicle, miR: microRNA, CD144: vascular endothelial (VE)-cadherin, CD31: platelet-endothelium cell adhesion molecule (PECAM), CD54: intercellular adhesion molecule 1(ICAM-1), CD146: melanoma cell adhesion molecule (MCAM), CD62E: E-selectin, CD62P: P-selectin, ACE: angiotensin converting enzyme, MHC: major histocompatibility complex.
Endothelial Cell-Derived EVs in Endothelial Pathogenesis
Endothelial dysfunction is a key initiating event in several pathological conditions. Vascular disorders increase permeability that can lead to vascular leakage and edema formation, perturb the balance between vasoconstriction and vasodilation, initiate the expression of adhesion molecules for inflammatory cell recruitment, and activate pro-inflammatory and pro-coagulant molecules (Rajendran et al., 2013; Fernandez-Hernando and Suarez, 2018; Huertas et al., 2018). Pulmonary ECs are exposed to various stresses, such as physiologic mechanical stress, endotoxins, and cigarette smoke. These stress stimuli impair endothelial functions and induce EV secretion (Heiss et al., 2008; Gordon et al., 2011; Liu et al., 2014; Letsiou et al., 2015). Furthermore, it has been reported that elevated levels of CD42b-/CD31+ endothelial microparticles are associated with vascular disease and endothelial dysfunction in patients with coronary artery disease, renal failure, and metabolic disorders (Amabile et al., 2005; Chironi et al., 2009; Wu et al., 2020). These evidences indicate that EC-EVs are also involved in endothelial dysfunction and various types of disease pathogenesis. Therefore, we will describe the pathological roles of EC-EVs in terms of endothelial dysfunction, especially inflammation, and senescence (Table 1).
Inflammation
The number of EVs is elevated in acute and chronic inflammatory diseases, such as sepsis, atherosclerosis, and diabetes mellitus (Helmke and von Vietinghoff, 2016; Freeman et al., 2018; Murao et al., 2020). In addition, emerging evidence implicates EVs as a causal and contributing factor in altering vascular cell phenotypes by mediating proinflammatory signaling pathways (Andrews and Rizzo, 2016). In human aortic ECs, EVs secreted by tumor necrosis factor-α (TNF-α) stimulation can act as paracrine mediators, inducing an inflammatory response (Curtis et al., 2009). Similarly, TNF-α-derived EVs activate the nuclear factor kappa-light-chain-enhancer of activated B cells (NF-κβ) pathway in human pulmonary microvascular ECs, thereby facilitating the inflammatory response (Liu et al., 2017). When exposed to inflammatory stimuli, ECs can sort many inflammation-related miRNAs, especially miR-328-3p and let-7d-3p, into EC-EVs, resulting in pathological neovascularization and vascular leakage (Yamamoto et al., 2015). Moreover, the atherosclerosis inducer, oxidized low-density lipoprotein (ox-LDL), is able to upregulate miR-155 expression in ECs. This miRNA is enriched in ox-LDL-induced EC-EVs, and miR-155-containing EVs regulate inflammation in association with atherosclerosis by monocyte activation (He et al., 2018). Thus, EC-EVs have the potential to induce inflammation through the composition of their cargoes.
Senescence
Senescence is a cellular response that induces irreversible growth arrest accompanied by distinct phenotypic alterations, such as chromatin rearrangement, metabolic reprogramming, autophagy modulation, and the implementation of a complex proinflammatory secretome, collectively referred as the senescence-associated secretory phenotype (SASP) (Campisi and d'Adda di Fagagna, 2007). Senescence has important roles in normal development, in maintaining tissue homeostasis, and in limiting tumor progression. However, senescence has also been implicated as a major cause of age-related diseases (van Deursen, 2014). Senescent ECs have been implicated in the pathogenesis of endothelial dysfunction. Several lines of evidence suggest that senescent ECs are involved in responses to different types of damage, such as telomere erosion, ROS-mediated DNA damage, vascular inflammation, mitochondrial dysfunction, and the renin-angiotensin-aldosterone system, which are characteristic of dysregulation of vascular tone, increases in endothelium permeability, and impairment of angiogenesis and mitochondrial biogenesis, leading to endothelial dysfunction.
Importantly, accumulating evidence suggests that EVs derived from senescent cells can regulate the phenotype of recipient cells similar to SASP (Kadota et al., 2018). Our recent study showed that human primary lung fibroblasts from patients with idiopathic pulmonary fibrosis (IPF) have a senescent phenotype and secrete increased levels of EVs containing miR-23b-3p and miR-494-3p, which has been shown to induce mitochondrial damage, increase epithelial-cell DNA damage responses, and further lead to senescence (Kadota et al., 2020). This indicates that senescent fibroblasts control their surroundings by reinforcing senescence in a paracrine manner through EV cargoes like SASP. EC-EVs have been found to be an important mediator associated with cell senescence in the microenvironment (Takasugi, 2018). It has been reported that EVs derived from senescent ECs induce premature senescence in normal ECs in a ROS-dependent manner (Burger et al., 2012). Indeed, senescent EC-derived EVs have been reported to contain miR-31, miR-21, and miR-217, which act as pro-senescence effectors to ECs (Weilner et al., 2016; Alique et al., 2017; Lee et al., 2019; Mensa et al., 2020). In the endothelium, we can speculate that EVs derived from senescent ECs may transfer pro-senescence signals, resulting in disease progression and causing comorbidities through spreading cellular senescence.
There are many additional reports describing various functions of EC-EVs in association with homeostasis and pathogenesis, and EV miRNAs seem to play a major role in these functions. Next, we will review the functions of EC-EVs and their miRNAs in respiratory disease pathogenesis (Figure 2).
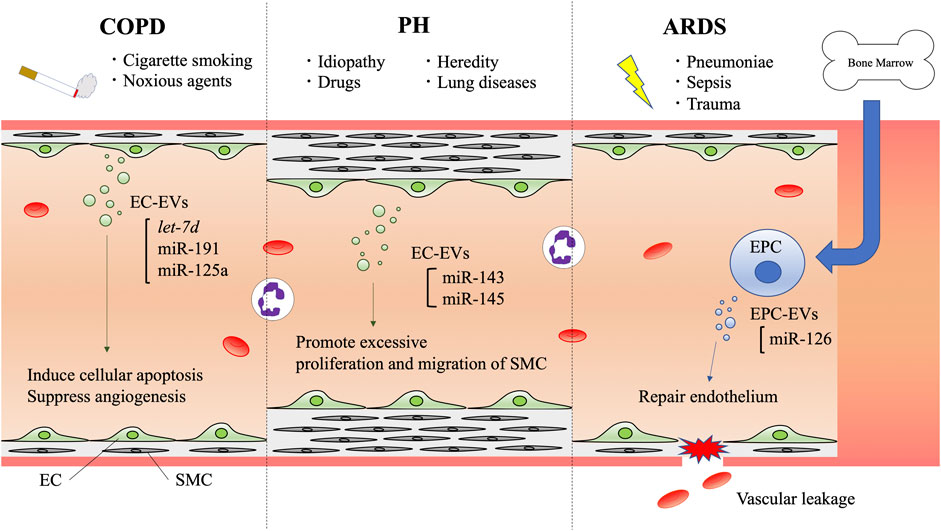
FIGURE 2. Schematic diagram of endothelial cell-derived extracellular vesicle communication in respiratory diseases. EVs are secreted to the bloodstream by vascular endothelial cells or endothelial progenitor cells. In COPD, cigarette smoke and noxious agents induce EV secretion, and their cargoes are involved in inducing cellular apoptosis and suppressing angiogenesis. In PH, several factors such as heredity, drugs, and other lung diseases are known to cause PH. Histological features have revealed EC proliferation and SMCs with vascular remodeling. ECs and SMCs crosstalk with each other via EVs. EC-EV miR-143 and miR-145 have the potential to promote excessive proliferation and migration of SMCs. In ARDS, pneumoniae, sepsis, and trauma can induce vascular leakage. EPCs derived from bone marrow secrete EVs that contain miR-126, which can help to repair endothelium. COPD: chronic obstructive pulmonary disease, PH: pulmonary hypertension, ARDS: acute respiratory distress syndrome, EC: endothelial cell, EPC: endothelial progenitor cell, SMC: smooth muscle cell, EV: extracellular vesicle, miR: microRNA.
Endothelial Cell-Derived EVs in Chronic Obstructive Pulmonary Disease
COPD is a common disease and a leading cause of morbidity and mortality worldwide. COPD is characterized by chronic inflammation and airflow limitation that is not fully reversible. The common symptoms are dyspnea, cough, and sputum production (Vogelmeier et al., 2017). COPD is usually caused by inhaled cigarette smoke and other noxious particles or gases. In addition, host factors are also relevant, for instance genetic abnormalities, those born with low lung function, and abnormal lung development (Stocks and Sonnappa, 2013). Most patients with COPD in the general population are probably affected by both multiple genetic factors and environmental factors. However, approximately 1–5% of COPD cases have α-1-antitrypsin deficiency which causes emphysema in nonsmokers (Takei et al., 2019a). Although the pathogenesis of COPD is very complicated, including chronic inflammation, proteinase/anti-proteinase imbalance, oxidative stress, and alveolar cell apoptosis, there are several reports showing that the vascular endothelium is deeply involved in the pathogenesis of COPD (Fischer et al., 2015; Schuliga, 2015; Lomas, 2016). For instance, cigarette smoking initiates pulmonary vascular malfunction through direct injury to ECs (Polverino et al., 2017), resulting in a persistent inflammatory response and pulmonary emphysema in lung tissues (Chen et al., 2012). Indeed, the presence of endothelial dysfunction has been confirmed in smokers and in patients with COPD (Piccari et al., 2020).
EC-EVs are associated with pulmonary and systemic vascular damage or other biological activities relevant to COPD pathogenesis. Endothelial damage has been reported to occur during acute exacerbations of COPD, and EVs can affect this process (Takahashi et al., 2012). COPD exacerbations are crucial events in the course of the disease that accelerate the progressive decline in lung function and increase the risk of death (Beasley et al., 2012; Song et al., 2014). The clusters of differentiation CD144+, CD31+, and CD62E+ EV reflect endothelial damage during COPD exacerbations, indicating that EV levels in patients with COPD exacerbation are significantly higher than in stable patients (Takahashi et al., 2012). Although further research on the role of these EVs is needed to elucidate their pathophysiology, it is likely that EC-EVs are involved in the mechanisms of COPD development.
There are various reports on the association between smoking and EVs in the pathogenesis of COPD (Hobbs and Hersh, 2014; Kara et al., 2016; Strulovici-Barel et al., 2016). Cigarette smoke extract (CSE) has been confirmed to reduce the level of α1-antitrypsin secretion by ECs, suggesting that CSE may also inhibit α1-antitrypsin transport via EC-EVs (Lockett et al., 2014). Our group showed that EVs from CSE-induced bronchial epithelial cells can cause airway fibrosis in COPD pathogenesis through autophagy suppression by EV miR-210 (Fujita et al., 2015a). It is not surprising that EC-EVs function and containing miRNAs can be altered in response to cigarette smoke exposure. Indeed, circulating EC-EVs released during cigarette smoke exposure are significantly enriched in vascular disease-related miRNAs, such as let-7d, miR-191, and miR-125a. EC-EVs containing these miRNAs may play key roles in inflammation and vascular remodeling changes in response to cigarette smoke (Serban et al., 2016). Let-7d induces cellular apoptosis and suppresses the proliferation, migration, and tubulogenesis of ECs (Ji et al., 2019). MiR-125a and miR-191 also promote cell apoptosis and suppress angiogenesis (Svensson et al., 2014; Gu et al., 2017; Du et al., 2019). These evidences suggest that EC-EVs and their miRNAs are involved in COPD pathogenesis and disease progression.
EVs released from the vascular endothelium circulate throughout the body and influences various distant sites. Therefore, EC-EVs not only participate in COPD pathogenesis, but are expected to be involved in the development of its various comorbidities. Systemic manifestations and comorbidities of COPD include skeletal muscle wasting, cardiovascular disease, osteoporosis, anemia, metabolic syndrome, and depression (Breyer et al., 2014; Caram et al., 2016; Inoue et al., 2016; Martinez Rivera et al., 2016; To et al., 2017; Xiong et al., 2018). As an example, it has been reported that EVs may play a role in linking COPD to cardiovascular comorbidities. Increased EC-EV levels in patients with COPD are related to prevalence of cardiovascular disease, even when compensating for confounding factors (Garcia-Lucio et al., 2018). Although further studies will be needed to elucidate the pathogenic EC-EV functions in linking COPD to the comorbidities, circulating EC-EVs in patients with COPD might exacerbate systemic endothelial injury, potentially leading to the development of cardiovascular disease. As another example, several miRNAs, such as miR-21, miR-134a, miR-133, and miR-206, have been proposed as potential biomarkers of sarcopenia in patients with COPD, and the levels of miR-21 and miR-206 in particular are strongly correlated with hand-grip strength (Qaisar et al., 2020). This suggests that EC-EVs may be involved in COPD-related sarcopenia pathogenesis, since miR-21 is enriched in EVs derived from senescent ECs as mentioned previously. Future research will elucidate the pathogenesis of COPD and the mechanism of its complications through EC-EV cargo. It is expected that the EC-EVs and their miRNAs will be used as biomarkers and targets of treatment.
Endothelial Cell-Derived EVs in Pulmonary Hypertension
PH is a severe disease characterized by elevated pulmonary arterial pressure and pulmonary vascular resistance, resulting in right ventricular hypertrophy, progressive fibrosis, right ventricular failure, and low cardiac output, leading to increase morbidity and mortality (Wei et al., 2013). Clinically, PH is divided into five groups, which are pulmonary arterial hypertension (PAH), pulmonary hypertension due to left heart disease, pulmonary hypertension due to lung diseases, chronic thromboembolic pulmonary hypertension, and pulmonary hypertension due to other disorders (Simonneau et al., 2019). Although several drugs are available for the treatment of PH, there is no cure for this disease, and current therapies are limited in reversing vascular remodeling. Thus, the prognosis remains poor, particularly for patients with severe PH (Hoeper et al., 2017). The histological features reveal proliferation of ECs and smooth muscle cells (SMCs) with irreversible vascular remodeling. EC dysfunction is considered to be a critical initiating factor in the development and progression of PAH. Furthermore, it is known that hypoxia and inflammation contribute to the development of endothelial damage (Rabinovitch et al., 2014). Then, sustained vasoconstriction and vascular remodeling result in increased pulmonary arterial pressure and pulmonary vascular resistance (Ranchoux et al., 2018).
There is accumulating evidence for a critical role of EC-EVs and their miRNAs in the pathogenesis of PH (Wei et al., 2013; de la Cuesta et al., 2019; Khandagale et al., 2020). Circulation levels of EC-EVs are increased in patients with PH, and levels correlate with pulmonary arterial pressure, pulmonary vascular resistance, thrombotic tendency, and mortality (Bakouboula et al., 2008; Aliotta et al., 2013). CD31+ and CD144+ EVs correlate with mean pulmonary arterial pressure and brain natriuretic peptide (BNP), suggesting that these EC-EVs are likely indicators of the hemodynamic severity of PH. CD62E+ EVs do not correlate with hemodynamic severity, but they do with C-reactive protein. This suggests that subpopulations of EC-EVs may reflect the diversity of injuries that occur in PH (Amabile et al., 2008).
Additionally, several miRNAs are involved in the pathogenesis of PH. MiR-143 and miR-145 are enriched in EVs secreted by Kruppel-like factor 2-transduced ECs, and are transferred from ECs to SMCs (Hergenreider et al., 2012; Doddaballapur et al., 2015). These miRNAs, which are increased in patients with PH and in a hypoxic mouse model of PH, promote excessive proliferation and migration of pulmonary artery SMCs, leading to PH development (Caruso et al., 2012; Mohsenin, 2016). Inhibition of miR-143 and miR-145 protects against the development of PH, suggesting that there is an important role for miR-143 and miR-145 in PH pathogenesis (Deng et al., 2015). As shown in these reports, EC-EVs and their miRNAs are involved in the pathology of PH.
Endothelial Cell-Derived EVs in Acute Respiratory Distress Syndrome
ARDS is a devastating and rapidly progressive respiratory disorder that is characterized by increased release of inflammatory mediators and disruption of the integrity of alveolar and vascular endothelial barriers (Thompson et al., 2017). The pathogenesis of ARDS is the destruction of the epithelial and endothelial layers caused by a variety of pulmonary or systemic inflammatory stimuli, including bacteria, viruses, thrombin, mechanical stretching, cytokines, and chemokines (Williams et al., 2017; Batah and Fabro, 2020; Xie et al., 2020). Cytokines and inflammatory mediators play essential roles in initiating and mediating ARDS, with inflammatory responses including endothelial adhesion molecule expression, the release of pro-inflammatory mediators, EC cytoskeleton rearrangement, and apoptosis (Maniatis et al., 2008; Wang et al., 2019). These stimuli contribute to lung injury by promoting EC barrier disruption and excessive translocation of inflammatory immune cells into the lungs (Curtis et al., 2013). In addition, ECs are activated by inflammatory stimuli, such as interleukin (IL)-1, IL-6, TNF-α, and oxidative stress in ARDS. Activated ECs promote microvascular injury by releasing complement and proinflammatory cytokines. Then, this injury disrupts EC tight junctions and adherens junctions, resulting in increased alveolar capillary permeability (Lu et al., 2012; Guo et al., 2016). Therefore, endothelial dysfunction leads to increasing vascular permeability and subsequent pulmonary edema and respiratory failure (Bellani et al., 2016; Fan et al., 2018).
Recent studies have demonstrated that EC-EVs are involved in EC dysfunction and the pathogenesis of ARDS (Li et al., 2015a; Shaver et al., 2017). It has been reported that EC-EVs induce endothelial dysfunction by causing the impairment of endothelium vasodilation. In an animal acute lung injury (ALI) model, plasminogen activator inhibitor-1 (PAI-1)-induced EC-EVs lead to the compromise of the alveolar-capillary barrier, inducing pulmonary edema and stimulating interstitial neutrophil accumulation (Densmore et al., 2006). In addition, when EC-EVs are injected intravenously into mice, it triggers pulmonary and systemic release of IL-1β and TNF-α and enhances lung injury in a lipopolysaccharide (LPS)-induced ALI (Buesing et al., 2011). Other studies have shown that LPS increases the number of circulating EC-EVs that express CD144, CD62E, CD54, or CD31 compared to healthy controls in a murine model (Li et al., 2015b; Yu et al., 2017). Indeed, EC-EVs are elevated in the plasma of patients with ARDS compared to healthy controls and in patients with cardiogenic pulmonary edema. These facts suggest that EC-EVs can contribute to the development of ARDS (Chen et al., 2017).
Patients with ARDS may develop acute renal failure, heart failure, thrombosis, and eventually multiple organ failure (Evans and Zhao, 2017; Araujo et al., 2019; Fowler et al., 2019). Intriguingly, Coronavirus Disease 2019 (COVID-19) infection, which can lead to ARDS and thrombosis, may be related to EC-EVs (Mehta et al., 2020; Zaid et al., 2020). It has been suggested that COVID-19 virus infects ECs by binding the ACE-2 receptor and using it for internalization, then the virus directly attacks microvascular ECs (Zhang et al., 2020). In addition, there is evidence that EC-EVs transfer ACE-2 to recipient cells (Wang et al., 2020). These facts suggest that EC-EVs may contribute to spread of this virus and support internalization (Hassanpour et al., 2020). Thus, understanding the function of EC-EVs in COVID-19-related ARDS is conducive to early identification and proper treatment of ARDS.
Endothelial Progenitor Cell-Derived EV Therapy
Currently, endothelial progenitor cell (EPC)-derived EVs (EPC-EVs) are used as therapeutics for various experimental diseases, including ARDS. Asahara et al. reported that EPCs can be isolated from human peripheral blood and bone marrow by utilizing markers for hematopoietic stem cells. It has thus been confirmed that these cells can differentiate into ECs (Asahara et al., 1997; Peters, 2018). Circulating EPCs derived from bone marrow migrate toward vascular lesions and contribute to vasculogenesis (Asahara et al., 1999). EPCs probably participate in maintaining vascular homeostasis and facilitate vascular repair (Kalka et al., 2000). Moreover, EPCs have been shown to exert their beneficial effects through paracrine mechanisms, including through the transmission of mediators via EVs. EPC-EVs could be incorporated into ECs to enhance their proliferation, migration, and angiogenic tubule formation (Zhang et al., 2016). Moreover, these EVs have beneficial effects on various preclinical models, such as sepsis, acute kidney injury, and ischemia (Cantaluppi et al., 2012; Ranghino et al., 2012; Zhou et al., 2018).
To date, there are no approved pharmacological treatments for ARDS. Therefore, EVs and their miRNAs have attracted attention as a pathological mediator and treatment method for ARDS in recent years (Fujita et al., 2018). In preclinical studies, intratracheal administration of EPC-EVs has also been shown to reduce lung injury through decreased local inflammatory cytokines, lung permeability, and neutrophil migration (Zhou et al., 2019). Previous evidences have indicated that miR-126, which is an endothelial-specific miRNA and is highly expressed in ECs, is involved in the regulation of vascular integrity and angiogenesis (Guo et al., 2016; Wu et al., 2016). Remarkably, miR-126-3p and -5p are abundant in EPC-EVs and have the ability to reduce the expression of genes associated with the development of ARDS (Zhou et al., 2019). In fact, recent studies have shown that miR-126-3p maintains the integrity of the endothelial barrier by targeting phosphoinositide-3-kinase regulatory subunit 2 (PIK3R2) and Spred-1, leading to the stabilization of VE-cadherin. Additionally, miR-126-5p inhibits inflammatory alarmin high mobility group box-1 (HMGB1) and the permeability factor VEGFα, attenuating endothelium barrier disruption and decreasing vascular permeability (Xi et al., 2017; Zhou et al., 2019). Conversely, suppression of miR-126 has been shown to increase vascular permeability (Pepini et al., 2010; Fernandez-Hernando and Suarez, 2018). These studies indicate that EPC-EVs may have novel therapeutic potential for ARDS through unique miRNA-related mechanisms.
Conclusion
The vascular endothelium is a distributed organ of considerable biological importance and has been shown to contribute to vascular homeostasis and disease. As shown in this review, EC-EVs are associated with vascular homeostasis and pathological conditions such as inflammation and senescence, and also play an important role in the pathogenesis of respiratory diseases such as COPD, PH, and ARDS. In normal endothelial physiology, EC-EVs function by maintaining homeostasis, and it has been found that the EVs are mainly involved in angiogenesis. Angiogenesis is facilitated by the miRNAs contained in EC-EVs, which regulate angiogenic signals and vascular EC proliferation, resulting in homeostatic control of vasculature physiology. In endothelial pathogenesis, EC-EVs alter their secretion and components in response to various stimuli, and they further regulate inflammation and senescence phenotypes, resulting in the progression of respiratory diseases and their comorbidities. In terms of EV therapeutics, EPC-EVs have the potential to reduce lung damage through local reduction of inflammatory cytokines, lung permeability, and neutrophil migration in ARDS pathogenesis.
EC-EVs are useful for mechanistic research and biomarker discovery, because blood vessels are located in places that are easier to approach than other organs. It is now thought that EC-EVs are involved in not only respiratory disease progression, but also in the development of systemic comorbidities through circulating cargoes. Thus, EVs and their miRNAs may be clinically useful, but the mechanism of their effects has not yet been fully elucidated. Understanding the pathological effects of EC-EVs and their cargo may bring mechanistic insights to the development of lung disease comorbidities, such as cardiovascular disease and muscle atrophy. Furthermore, the identification of pathological EC-EVs will lead to the discovery of novel progressive or predictive biomarkers for lung diseases and their systemic complications. Since the vascular endothelium is present in almost all organs, it has been researched in various fields and has the potential to be applied in different fields that support each other. In conclusion, we speculate that research on vascular endothelium and EC-EVs of lung diseases and their comorbidities will be useful not only for understanding pathophysiology, but also for developing novel treatments for various diseases (Figure 3).
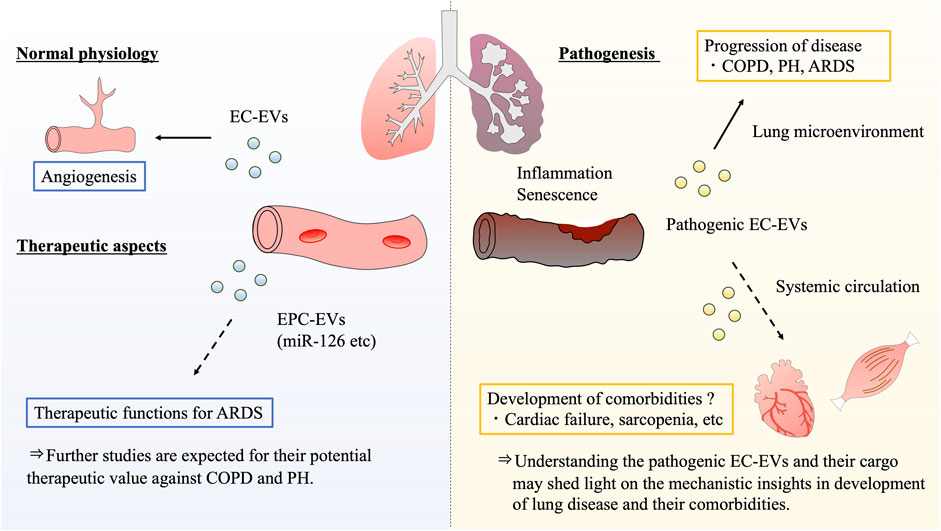
FIGURE 3. Summary and future perspectives of endothelial cell-derived extracellular vesicle potential. Based on the previous evidences, it is clear that EC-EVs have the functions of regulating angiogenesis in normal endothelial physiology. With regard to therapeutic aspect, EPC-EVs may have the potential to be developed as novel treatments for various respiratory diseases. Recent studies indicate that EPC-EVs have therapeutic functions for ARDS through unique miRNA-related action mechanisms. On the other hand, pathogenic EC-EVs further promote inflammation and senescence phenotypes in the endothelial microenvironment, resulting in the progression of respiratory diseases such as COPD, PH, and ARDS. Notably, EC-EVs circulate throughout the body, the EVs exert influences over various sites. As future perspectives, pathogenic EC-EVs can form the respiratory disease pathogenesis, and are expected to be involved in the development of their comorbidities. COPD: chronic obstructive pulmonary disease, PH: pulmonary hypertension, ARDS: acute respiratory distress syndrome, EC: endothelial cell, EPC: endothelial progenitor cell, EV: extracellular vesicle.
Author Contributions
SF and YF are responsible to the concept of the review. SF, YF, TK, JA, and KK wrote the manuscript. The manuscript was finalized by YF with the assistance of all authors.
Funding
The authors gratefully acknowledge finding by KAKENHI (19K17649) and GSK Japan Research Grant 2020.
Conflict of Interest
The authors declare that the research was conducted in the absence of any commercial or financial relationships that could be construed as a potential conflict of interest.
References
Admyre, C., Grunewald, J., Thyberg, J., Gripenbäck, S., Tornling, G., Eklund, A., et al. (2003). Exosomes with major histocompatibility complex class II and co-stimulatory molecules are present in human BAL fluid. Eur. Respir. J. 22, 578–583. doi:10.1183/09031936.03.00041703
Adyshev, D. M., Elangovan, V. R., Moldobaeva, N., Mapes, B., Sun, X., and Garcia, J. G. (2014). Mechanical stress induces pre-B-cell colony-enhancing factor/NAMPT expression via epigenetic regulation by miR-374a and miR-568 in human lung endothelium. Am. J. Respir. Cell Mol. Biol. 50, 409–418. doi:10.1165/rcmb.2013-0292OC
Aliotta, J. M., Pereira, M., Amaral, A., Sorokina, A., Igbinoba, Z., Hasslinger, A., et al. (2013). Induction of pulmonary hypertensive changes by extracellular vesicles from monocrotaline-treated mice. Cardiovasc. Res. 100, 354–362. doi:10.1093/cvr/cvt184
Alique, M., Ruíz-Torres, M. P., Bodega, G., Noci, M. V., Troyano, N., Bohórquez, L., et al. (2017). Microvesicles from the plasma of elderly subjects and from senescent endothelial cells promote vascular calcification. Aging (Albany NY) 9, 778–789. doi:10.18632/aging.101191
Amabile, N., Guérin, A. P., Leroyer, A., Mallat, Z., Nguyen, C., Boddaert, J., et al. (2005). Circulating endothelial microparticles are associated with vascular dysfunction in patients with end-stage renal failure. J. Am. Soc. Nephrol. 16, 3381–3388. doi:10.1681/ASN.2005050535
Amabile, N., Heiss, C., Real, W. M., Minasi, P., Mcglothlin, D., Rame, E. J., et al. (2008). Circulating endothelial microparticle levels predict hemodynamic severity of pulmonary hypertension. Am. J. Respir. Crit. Care Med. 177, 1268–1275. doi:10.1164/rccm.200710-1458OC
Anastasiadou, E., Faggioni, A., Trivedi, P., and Slack, F. J. (2018a). The nefarious nexus of noncoding RNAs in cancer. Int. J. Mol. Sci. 19, 2072. doi:10.3390/ijms19072072
Anastasiadou, E., Jacob, L. S., and Slack, F. J. (2018b). Non-coding RNA networks in cancer. Nat. Rev. Cancer 18, 5–18. doi:10.1038/nrc.2017.99
Anderson, H. C. (1969). Vesicles associated with calcification in the matrix of epiphyseal cartilage. J. Cell Biol. 41, 59–72. doi:10.1083/jcb.41.1.59
Andrews, A. M., and Rizzo, V. (2016). Microparticle-induced activation of the vascular endothelium requires caveolin-1/caveolae. PLoS One 11, e0149272. doi:10.1371/journal.pone.0149272
Araújo, C. B., De Oliveira Neves, F. M., De Freitas, D. F., Arruda, B. F. T., de Macêdo Filho, L. J. M., Salles, V. B., et al. (2019). Angiopoietin-2 as a predictor of acute kidney injury in critically ill patients and association with ARDS. Respirology 24, 345–351. doi:10.1111/resp.13464
Asahara, T., Masuda, H., Takahashi, T., Kalka, C., Pastore, C., Silver, M., et al. (1999). Bone marrow origin of endothelial progenitor cells responsible for postnatal vasculogenesis in physiological and pathological neovascularization. Circ. Res. 85, 221–8. doi:10.1161/01.res.85.3.221
Asahara, T., Murohara, T., Sullivan, A., Silver, M., Van Der Zee, R., Li, T., et al. (1997). Isolation of putative progenitor endothelial cells for angiogenesis. Science 275, 964–967. doi:10.1126/science.275.5302.964
Bakouboula, B., Morel, O., Faure, A., Zobairi, F., Jesel, L., Trinh, A., et al. (2008). Procoagulant membrane microparticles correlate with the severity of pulmonary arterial hypertension. Am. J. Respir. Crit. Care Med. 177, 536–543. doi:10.1164/rccm.200706-840OC
Batah, S. S., and Fabro, A. T. (2020). Pulmonary pathology of ARDS in COVID-19: a pathological review for clinicians. Respir. Med. 176, 106239. doi:10.1016/j.rmed.2020.106239
Beasley, V., Joshi, P. V., Singanayagam, A., Molyneaux, P. L., Johnston, S. L., and Mallia, P. (2012). Lung microbiology and exacerbations in COPD. Int. J. Chron. Obstruct. Pulmon. Dis. 7, 555–569. doi:10.2147/COPD.S28286
Becker, W. R., Ober-Reynolds, B., Jouravleva, K., Jolly, S. M., Zamore, P. D., and Greenleaf, W. J. (2019). High-throughput analysis reveals rules for target RNA binding and cleavage by AGO2. Mol. Cell 75, 741–e11. doi:10.1016/j.molcel.2019.06.012
Bellani, G., Laffey, J. G., Pham, T., Fan, E., Brochard, L., Esteban, A., et al. (2016). Epidemiology, patterns of care, and mortality for patients with acute respiratory distress syndrome in intensive care units in 50 countries. JAMA 315, 788–800. doi:10.1001/jama.2016.0291
Breyer, M. K., Spruit, M. A., Hanson, C. K., Franssen, F. M., Vanfleteren, L. E., Groenen, M. T., et al. (2014). Prevalence of metabolic syndrome in COPD patients and its consequences. PLoS One 9, e98013. doi:10.1371/journal.pone.0098013
Buesing, K. L., Densmore, J. C., Kaul, S., Pritchard, K. A., Jarzembowski, J. A., Gourlay, D. M., et al. (2011). Endothelial microparticles induce inflammation in acute lung injury. J. Surg. Res. 166, 32–39. doi:10.1016/j.jss.2010.05.036
Burger, D., Kwart, D. G., Montezano, A. C., Read, N. C., Kennedy, C. R., Thompson, C. S., et al. (2012). Microparticles induce cell cycle arrest through redox-sensitive processes in endothelial cells: implications in vascular senescence. J. Am. Heart. Assoc. 1, e001842. doi:10.1161/JAHA.112.001842
Cahill, P. A., and Redmond, E. M. (2016). Vascular endothelium-Gatekeeper of vessel health. Atherosclerosis 248, 97–109. doi:10.1016/j.atherosclerosis.2016.03.007
Campisi, J., and D’adda Di Fagagna, F. (2007). Cellular senescence: when bad things happen to good cells. Nat. Rev. Mol. Cell Biol. 8, 729–740. doi:10.1038/nrm2233
Cantaluppi, V., Gatti, S., Medica, D., Figliolini, F., Bruno, S., Deregibus, M. C., et al. (2012). Microvesicles derived from endothelial progenitor cells protect the kidney from ischemia-reperfusion injury by microRNA-dependent reprogramming of resident renal cells. Kidney Int. 82, 412–427. doi:10.1038/ki.2012.105
Caram, L. M., Ferrari, R., Naves, C. R., Coelho, L. S., Vale, S. A., Tanni, S. E., et al. (2016). Risk factors for cardiovascular disease in patients with COPD: mild-to-moderate COPD versus severe-to-very severe COPD. J. Bras. Pneumol. 42, 179–184. doi:10.1590/S1806-37562015000000121
Caruso, P., Dempsie, Y., Stevens, H. C., Mcdonald, R. A., Long, L., Lu, R., et al. (2012). A role for miR-145 in pulmonary arterial hypertension: evidence from mouse models and patient samples. Circ. Res. 111, 290–300. doi:10.1161/CIRCRESAHA.112.267591
Cavallari, C., Dellepiane, S., Fonsato, V., Medica, D., Marengo, M., Migliori, M., et al. (2019). Online hemodiafiltration inhibits inflammation-related endothelial dysfunction and vascular calcification of uremic patients modulating miR-223 expression in plasma extracellular vesicles. J. Immunol. 202, 2372–2383. doi:10.4049/jimmunol.1800747
Cech, T. R., and Steitz, J. A. (2014). The noncoding RNA revolution-trashing old rules to forge new ones. Cell 157, 77–94. doi:10.1016/j.cell.2014.03.008
Chen, J., Hu, C., and Pan, P. (2017). Extracellular vesicle microRNA transfer in lung diseases. Front. Physiol. 8, 1028. doi:10.3389/fphys.2017.01028
Chen, Y., Luo, H., Kang, N., Guan, C., Long, Y., Cao, J., et al. (2012). Beraprost sodium attenuates cigarette smoke extract-induced apoptosis in vascular endothelial cells. Mol. Biol. Rep. 39, 10447–10457. doi:10.1007/s11033-012-1924-1
Chironi, G. N., Boulanger, C. M., Simon, A., Dignat-George, F., Freyssinet, J. M., and Tedgui, A. (2009). Endothelial microparticles in diseases. Cell Tissue Res 335, 143–151. doi:10.1007/s00441-008-0710-9
Choi, D. S., Kim, D. K., Kim, Y. K., and Gho, Y. S. (2015). Proteomics of extracellular vesicles: exosomes and ectosomes. Mass Spectrom. Rev. 34, 474–490. doi:10.1002/mas.21420
Colbert, J. F., and Schmidt, E. P. (2016). Endothelial and microcirculatory function and dysfunction in sepsis. Clin. Chest Med. 37, 263–275. doi:10.1016/j.ccm.2016.01.009
Curtis, A. M., Edelberg, J., Jonas, R., Rogers, W. T., Moore, J. S., Syed, W., et al. (2013). Endothelial microparticles: sophisticated vesicles modulating vascular function. Vasc. Med. 18, 204–214. doi:10.1177/1358863X13499773
Curtis, A. M., Wilkinson, P. F., Gui, M., Gales, T. L., Hu, E., and Edelberg, J. M. (2009). p38 mitogen-activated protein kinase targets the production of proinflammatory endothelial microparticles. J. Thromb. Haemost. 7, 701–709. doi:10.1111/j.1538-7836.2009.03304.x
Dai, Z., Zhu, M. M., Peng, Y., Jin, H., Machireddy, N., Qian, Z., et al. (2018). Endothelial and Smooth Muscle Cell Interaction via FoxM1 Signaling Mediates Vascular Remodeling and Pulmonary Hypertension. Am. J. Respir. Crit. Care Med. 198, 788–802. doi:10.1164/rccm.201709-1835OC
De Jong, O. G., Verhaar, M. C., Chen, Y., Vader, P., Gremmels, H., Posthuma, G., et al. (2012). Cellular stress conditions are reflected in the protein and RNA content of endothelial cell-derived exosomes. J. Extracell Vesicles 1, 18396. doi:10.3402/jev.v1i0.18396
De La Cuesta, F., Passalacqua, I., Rodor, J., Bhushan, R., Denby, L., and Baker, A. H. (2019). Extracellular vesicle cross-talk between pulmonary artery smooth muscle cells and endothelium during excessive TGF-β signalling: implications for PAH vascular remodelling. Cell Commun. Signal 17, 143. doi:10.1186/s12964-019-0449-9
Deng, L., Blanco, F. J., Stevens, H., Lu, R., Caudrillier, A., Mcbride, M., et al. (2015). MicroRNA-143 activation regulates smooth muscle and endothelial cell crosstalk in pulmonary arterial hypertension. Circ. Res. 117, 870–883. doi:10.1161/CIRCRESAHA.115.306806
Densmore, J. C., Signorino, P. R., Ou, J., Hatoum, O. A., Rowe, J. J., Shi, Y., et al. (2006). Endothelium-derived microparticles induce endothelial dysfunction and acute lung injury. Shock 26, 464–471. doi:10.1097/01.shk.0000228791.10550.36
Dignat-George, F., and Boulanger, C. M. (2011). The many faces of endothelial microparticles. Arterioscler Thromb. Vasc. Biol. 31, 27–33. doi:10.1161/ATVBAHA.110.218123
Dixon, C. L., Sheller-Miller, S., Saade, G. R., Fortunato, S. J., Lai, A., Palma, C., et al. (2018). Amniotic fluid exosome proteomic profile exhibits unique pathways of term and preterm labor. Endocrinology 159, 2229–2240. doi:10.1210/en.2018-00073
Doddaballapur, A., Michalik, K. M., Manavski, Y., Lucas, T., Houtkooper, R. H., You, X., et al. (2015). Laminar shear stress inhibits endothelial cell metabolism via KLF2-mediated repression of PFKFB3. Arterioscler Thromb. Vasc. Biol. 35, 137–145. doi:10.1161/ATVBAHA.114.304277
Du, K., Zhao, C., Wang, L., Wang, Y., Zhang, K. Z., Shen, X. Y., et al. (2019). MiR-191 inhibit angiogenesis after acute ischemic stroke targeting VEZF1. Aging (Albany NY) 11, 2762–2786. doi:10.18632/aging.101948
Espinosa, J. M. (2016). Revisiting lncRNAs: how do you know yours is not an eRNA? Mol. Cell 62, 1–2. doi:10.1016/j.molcel.2016.03.022
Evans, C. E., and Zhao, Y. Y. (2017). Impact of thrombosis on pulmonary endothelial injury and repair following sepsis. Am. J. Physiol. Lung Cell. Mol. Physiol. 312, L441–L451. doi:10.1152/ajplung.00441.2016
Fan, E., Brodie, D., and Slutsky, A. S. (2018). Acute respiratory distress syndrome: advances in diagnosis and treatment. JAMA 319, 698–710. doi:10.1001/jama.2017.21907
Fatima, F., and Nawaz, M. (2017). Vesiculated long non-coding RNAs: offshore packages deciphering trans-regulation between cells, cancer progression and resistance to therapies. Noncoding RNA 3, 10. doi:10.3390/ncrna3010010
Fernández-Hernando, C., and Suárez, Y. (2018). MicroRNAs in endothelial cell homeostasis and vascular disease. Curr. Opin. Hematol. 25, 227–236. doi:10.1097/MOH.0000000000000424
Fischer, B. M., Voynow, J. A., and Ghio, A. J. (2015). COPD: balancing oxidants and antioxidants. Int. J. Chron. Obstruct. Pulmon. Dis. 10, 261–76. doi:10.2147/COPD.S42414
Fowler, A. A., Truwit, J. D., Hite, R. D., Morris, P. E., Dewilde, C., Priday, A., et al. (2019). Effect of vitamin C infusion on organ failure and biomarkers of inflammation and vascular injury in patients with sepsis and severe acute respiratory failure: the CITRIS-ALI randomized clinical trial. JAMA 322, 1261–1270. doi:10.1001/jama.2019.11825
Freeman, D. W., Noren Hooten, N., Eitan, E., Green, J., Mode, N. A., Bodogai, M., et al. (2018). Altered extracellular vesicle concentration, cargo, and function in diabetes. Diabetes 67, 2377–2388. doi:10.2337/db17-1308
Fujita, Y., Araya, J., Ito, S., Kobayashi, K., Kosaka, N., Yoshioka, Y., et al. (2015a). Suppression of autophagy by extracellular vesicles promotes myofibroblast differentiation in COPD pathogenesis. J. Extracell Vesicles 4, 28388. doi:10.3402/jev.v4.28388
Fujita, Y., Kadota, T., Araya, J., Ochiya, T., and Kuwano, K. (2018). Clinical application of mesenchymal stem cell-derived extracellular vesicle-based therapeutics for inflammatory lung diseases. J. Clin. Med. 7, 355. doi:10.3390/jcm7100355
Fujita, Y., Kosaka, N., Araya, J., Kuwano, K., and Ochiya, T. (2015b). Extracellular vesicles in lung microenvironment and pathogenesis. Trends Mol. Med. 21, 533–542. doi:10.1016/j.molmed.2015.07.004
Fujita, Y., Yoshioka, Y., and Ochiya, T. (2016). Extracellular vesicle transfer of cancer pathogenic components. Cancer Sci. 107, 385–390. doi:10.1111/cas.12896
García-Lucio, J., Peinado, V. I., De Jover, L., Del Pozo, R., Blanco, I., Bonjoch, C., et al. (2018). Imbalance between endothelial damage and repair capacity in chronic obstructive pulmonary disease. PLoS One 13, e0195724, doi:10.1371/journal.pone.0195724
Genschmer, K. R., Russell, D. W., Lal, C., Szul, T., Bratcher, P. E., Noerager, B. D., et al. (2019). Activated PMN exosomes: pathogenic entities causing matrix destruction and disease in the lung. Cell 176, 113–e15. doi:10.1016/j.cell.2018.12.002
Goldie, B. J., Dun, M. D., Lin, M., Smith, N. D., Verrills, N. M., Dayas, C. V., et al. (2014). Activity-associated miRNA are packaged in Map1b-enriched exosomes released from depolarized neurons. Nucleic Acids Res. 42, 9195–208. doi:10.1093/nar/gku594
Gordon, C., Gudi, K., Krause, A., Sackrowitz, R., Harvey, B. G., Strulovici-Barel, Y., et al. (2011). Circulating endothelial microparticles as a measure of early lung destruction in cigarette smokers. Am. J. Respir. Crit. Care Med. 184, 224–232. doi:10.1164/rccm.201012-2061OC
Gu, Y., Ampofo, E., Menger, M. D., and Laschke, M. W. (2017). miR-191 suppresses angiogenesis by activation of NF-κB signaling. FASEB J 31, 3321–3333. doi:10.1096/fj.201601263R
Guo, C., Goodwin, A., Buie, J. N., Cook, J., Halushka, P., Argraves, K., et al. (2016). A stromal cell-derived factor 1 alpha analogue improves endothelial cell function in lipopolysaccharide-induced acute respiratory distress syndrome. Mol. Med. 22, 115–123. doi:10.2119/molmed.2015.00240
Guo, L., Zhao, Y., Yang, S., Zhang, H., and Chen, F. (2014). An integrated analysis of miRNA, lncRNA, and mRNA expression profiles. Biomed. Res. Int. 2014, 345605. doi:10.1155/2014/345605
Ha, M., and Kim, V. N. (2014). Regulation of microRNA biogenesis. Nat. Rev. Mol. Cell Biol. 15, 509–24. doi:10.1038/nrm3838
Hargett, L. A., and Bauer, N. N. (2013). On the origin of microparticles: from “platelet dust” to mediators of intercellular communication. Pulm Circ. 3, 329–40. doi:10.4103/2045-8932.114760
Hassanpour, M., Rezaie, J., Nouri, M., and Panahi, Y. (2020). The role of extracellular vesicles in COVID-19 virus infection. Infect. Genet. Evol. 85, 104422. doi:10.1016/j.meegid.2020.104422
He, S., Wu, C., Xiao, J., Li, D., Sun, Z., and Li, M. (2018). Endothelial extracellular vesicles modulate the macrophage phenotype: Potential implications in atherosclerosis. Scand. J. Immunol. 87, e12648. doi:10.1111/sji.12648
Heiss, C., Amabile, N., Lee, A. C., Real, W. M., Schick, S. F., Lao, D., et al. (2008). Brief secondhand smoke exposure depresses endothelial progenitor cells activity and endothelial function: sustained vascular injury and blunted nitric oxide production. J. Am. Coll. Cardiol. 51, 1760–1771. doi:10.1016/j.jacc.2008.01.040
Helmke, A., and Von Vietinghoff, S. (2016). Extracellular vesicles as mediators of vascular inflammation in kidney disease. World J. Nephrol. 5, 125–38. doi:10.5527/wjn.v5.i2.125
Helms, C. C., Gladwin, M. T., and Kim-Shapiro, D. B (2018). Erythrocytes and vascular function: oxygen and nitric oxide. Front. Physiol. 9, 125. doi:10.3389/fphys.2018.00125
Hergenreider, E., Heydt, S., Tréguer, K., Boettger, T., Horrevoets, A. J., Zeiher, A. M., et al. (2012). Atheroprotective communication between endothelial cells and smooth muscle cells through miRNAs. Nat. Cell Biol. 14, 249–256. doi:10.1038/ncb2441
Hessvik, N. P., and Llorente, A. (2018). Current knowledge on exosome biogenesis and release. Cell Mol. Life Sci. 75, 193–208. doi:10.1007/s00018-017-2595-9
Hobbs, B. D., and Hersh, C. P. (2014). Integrative genomics of chronic obstructive pulmonary disease. Biochem. Biophys. Res. Commun. 452, 276–286. doi:10.1016/j.bbrc.2014.07.086
Hoeper, M. M., Ghofrani, H. A., Grünhig, E., Klose, H., Olschewski, H., and Rosenkranz, S. (2017). Pulmonary ypertension. Dtsch Arztebl Int. 114, 73–84. doi:10.3238/arztebl.2017.0073
Hromada, C., Mühleder, S., Grillari, J., Redl, H., and Holnthoner, W. (2017). Endothelial extracellular vesicles-promises and challenges. Front. Physiol. 8, 275. doi:10.3389/fphys.2017.00275
Hsia, C. C., Hyde, D. M., and Weibel, E. R. (2016). Lung structure and the intrinsic challenges of gas exchange. Compr. Physiol. 6, 827–95. doi:10.1002/cphy.c150028
Huertas, A., Guignabert, C., Barberà, J. A., Bärtsch, P., Bhattacharya, J., Bhattacharya, S., et al. (2018). Pulmonary vascular endothelium: the orchestra conductor in respiratory diseases: Highlights from basic research to therapy. Eur. Respir. J. 51, 1700745. doi:10.1183/13993003.00745-2017
Hulshoff, M. S., Del Monte-Nieto, G., Kovacic, J., and Krenning, G. (2019). Non-coding RNA in endothelial-to-mesenchymal transition. Cardiovasc. Res. 115, 1716–1731. doi:10.1093/cvr/cvz211
Inoue, D., Watanabe, R., and Okazaki, R. (2016). COPD and osteoporosis: links, risks, and treatment challenges. Int. J. Chron. Obstruct. Pulmon. Dis. 11, 637–648. doi:10.2147/COPD.S79638
Ji, X., Hua, H., Shen, Y., Bu, S., and Yi, S. (2019). Let-7d modulates the proliferation, migration, tubulogenesis of endothelial cells. Mol. Cell Biochem. 462, 75–83. doi:10.1007/s11010-019-03611-x
Johnstone, R. M., Adam, M., Hammond, J. R., Orr, L., and Turbide, C. (1987). Vesicle formation during reticulocyte maturation. Association of plasma membrane activities with released vesicles (exosomes). J. Biol. Chem. 262, 9412–9420. doi:10.1016/s0021-9258(18)48095-7
Kadota, T., Fujita, Y., Yoshioka, Y., Araya, J., Kuwano, K., and Ochiya, T. (2016). Extracellular Vesicles in Chronic Obstructive Pulmonary Disease. Int. J. Mol. Sci. 17, 1801. doi:10.3390/ijms17111801
Kadota, T., Fujita, Y., Yoshioka, Y., Araya, J., Kuwano, K., and Ochiya, T. (2018). Emerging role of extracellular vesicles as a senescence-associated secretory phenotype: Insights into the pathophysiology of lung diseases. Mol. Aspects Med. 60, 92–103. doi:10.1016/j.mam.2017.11.005
Kadota, T., Yoshioka, Y., Fujita, Y., Araya, J., Minagawa, S., Hara, H., et al. (2020). Extracellular vesicles from fibroblasts induce epithelial-cell senescence in pulmonary fibrosis. Am. J. Respir. Cell Mol. Biol. 63, 623–636. doi:10.1165/rcmb.2020-0002OC
Kadota, T., Yoshioka, Y., Fujita, Y., Kuwano, K., and Ochiya, T. (2017). Extracellular vesicles in lung cancer-From bench to bedside. Semin. Cell Dev. Biol. 67, 39–47. doi:10.1016/j.semcdb.2017.03.001
Kalka, C., Masuda, H., Takahashi, T., Kalka-Moll, W. M., Silver, M., Kearney, M., et al. (2000). Transplantation of ex vivo expanded endothelial progenitor cells for therapeutic neovascularization. Proc. Natl. Acad. Sci. USA 97, 3422–3427. doi:10.1073/pnas.070046397
Kalluri, R., and Lebleu, V. S. (2020). The biology, function, and biomedical applications of exosomes. Science 367, eaau6977. doi:10.1126/science.aau6977
Kara, M., Kirkil, G., and Kalemci, S. (2016). Differential expression of microRNAs in chronic obstructive pulmonary disease. Adv. Clin. Exp. Med. 25, 21–26. doi:10.17219/acem/28343
Khandagale, A., Åberg, M., Wikström, G., Bergström Lind, S., Shevchenko, G., Björklund, E., et al. (2020). Role of extracellular vesicles in pulmonary arterial hypertension: modulation of pulmonary endothelial function and angiogenesis. Arterioscler. Thromb. Vasc. Biol. 40, 2293–2309. doi:10.1161/ATVBAHA.120.314152
Kim, K. M., Abdelmohsen, K., Mustapic, M., Kapogiannis, D., and Gorospe, M. (2017). RNA in extracellular vesicles, Wiley Interdiscip. Rev. RNA 8, 1413. doi:10.1002/wrna.1413
Kosaka, N., Iguchi, H., Yoshioka, Y., Takeshita, F., Matsuki, Y., and Ochiya, T. (2010). Secretory mechanisms and intercellular transfer of microRNAs in living cells. J. Biol. Chem. 285, 17442–17452. doi:10.1074/jbc.M110.107821
Krüger-Genge, A., Blocki, A., Franke, R. P., and Jung, F. (2019). Vascular Endothelial Cell Biology: An Update. Int. J. Mol. Sci. 20, 4411. doi:10.3390/ijms20184411
Kubo, H (2018). Extracellular vesicles in lung disease. Chest 153, 210–216. doi:10.1016/j.chest.2017.06.026
Lakkaraju, A., and Rodriguez-Boulan, E. (2008). Itinerant exosomes: emerging roles in cell and tissue polarity. Trends Cell. Biol. 18, 199–209. doi:10.1016/j.tcb.2008.03.002
Lanyu, Z., and Feilong, H. (2019). Emerging role of extracellular vesicles in lung injury and inflammation. Biomed. Pharmacother. 113, 108748. doi:10.1016/j.biopha.2019.108748
Lee, R. C., Feinbaum, R. L., and Ambros, V. (1993). The C. elegans heterochronic gene lin-4 encodes small RNAs with antisense complementarity to lin-14. Cell 75, 843–854. doi:10.1016/0092-8674(93)90529-y
Lee, S. H., Lee, J. H., Lee, H. Y., and Min, K. J. (2019). Sirtuin signaling in cellular senescence and aging. BMB Rep. 52, 24–34. doi:10.5483/bmbrep.2019.52.1.290
Leligdowicz, A., Chun, L. F., Jauregui, A., Vessel, K., Liu, K. D., Calfee, C. S., et al. (2018). Human pulmonary endothelial cell permeability after exposure to LPS-stimulated leukocyte supernatants derived from patients with early sepsis. Am. J. Physiol. Lung Cell Mol. Physiol. 315, L638–l644. doi:10.1152/ajplung.00286.2018
Letsiou, E., and Bauer, N. (2018). Endothelial Extracellular Vesicles in Pulmonary Function and Disease. Curr. Top Membr 82, 197–256. doi:10.1016/bs.ctm.2018.09.002
Letsiou, E., Sammani, S., Zhang, W., Zhou, T., Quijada, H., Moreno-Vinasco, L., et al. (2015). Pathologic mechanical stress and endotoxin exposure increases lung endothelial microparticle shedding. Am. J. Respir. Cell Mol. Biol. 52, 193–204. doi:10.1165/rcmb.2013-0347OC
Li, H., Meng, X., Gao, Y., and Cai, S. (2015a). Isolation and phenotypic characteristics of microparticles in acute respiratory distress syndrome. Int. J. Clin. Exp. Pathol. 8, 1640–1648.
Li, H., Meng, X., Liang, X., Gao, Y., and Cai, S. (2015b). Administration of microparticles from blood of the lipopolysaccharide-treated rats serves to induce pathologic changes of acute respiratory distress syndrome. Exp. Biol. Med. (Maywood) 240, 1735–1741. doi:10.1177/1535370215591830
Li, K., Chen, Y., Li, A., Tan, C., and Liu, X. (2019). Exosomes play roles in sequential processes of tumor metastasis. Int. J. Cancer 144, 1486–1495. doi:10.1002/ijc.31774
Liu, H., Ding, L., Zhang, Y., and Ni, S. (2014). Circulating endothelial microparticles involved in lung function decline in a rat exposed in cigarette smoke maybe from apoptotic pulmonary capillary endothelial cells. J. Thorac. Dis. 6, 649–655. doi:10.3978/j.issn.2072-1439.2014.06.26
Liu, Y., Zhang, R., Qu, H., Wu, J., Li, L., and Tang, Y. (2017). Endothelial microparticles activate endothelial cells to facilitate the inflammatory response. Mol. Med. Rep. 15, 1291–1296. doi:10.3892/mmr.2017.6113
Lockett, A. D., Brown, M. B., Santos-Falcon, N., Rush, N. I., Oueini, H., Oberle, A. J., et al. (2014). Active trafficking of alpha 1 antitrypsin across the lung endothelium. PLoS One 9, e93979. doi:10.1371/journal.pone.0093979
Lomas, D. A. (2016). Does protease-antiprotease imbalance explain chronic obstructive pulmonary disease?. Ann. Am. Thorac. Soc. 13 (Suppl 2), S130–S137. doi:10.1513/AnnalsATS.201504-196KV
Lu, Q., Newton, J., Hsiao, V., Shamirian, P., Blackburn, M. R., and Pedroza, M. (2012). Sustained adenosine exposure causes lung endothelial barrier dysfunction via nucleoside transporter-mediated signaling. Am. J. Respir. Cell Mol. Biol. 47, 604–613. doi:10.1165/rcmb.2012-0012OC
Machida, T., Tomofuji, T., Ekuni, D., Maruyama, T., Yoneda, T., Kawabata, Y., et al. (2015). MicroRNAs in Salivary Exosome as Potential Biomarkers of Aging. Int. J. Mol. Sci. 16, 21294–21309. doi:10.3390/ijms160921294
Madison, M. N., Jones, P. H., and Okeoma, C. M. (2015). Exosomes in human semen restrict HIV-1 transmission by vaginal cells and block intravaginal replication of LP-BM5 murine AIDS virus complex. Virology 482, 189–201. doi:10.1016/j.virol.2015.03.040
Maniatis, N. A., Kotanidou, A., Catravas, J. D., and Orfanos, S. E. (2008). Endothelial pathomechanisms in acute lung injury. Vascul. Pharmacol. 49, 119–133. doi:10.1016/j.vph.2008.06.009
Martinez Rivera, C., Costan Galicia, J., Alcázar Navarrete, B., Garcia-Polo, C., Ruiz Iturriaga, L. A., Herrejón, A., et al. (2016). Factors associated with depression in COPD: a multicenter study. Lung 194, 335–143. doi:10.1007/s00408-016-9862-7
Matsuyama, H., and Suzuki, H. I. (2019). Systems and synthetic microRNA biology: from biogenesis to disease pathogenesis. Int. J. Mol. Sci. 21, 132. doi:10.3390/ijms21010132
Mehta, P., Mcauley, D. F., Brown, M., Sanchez, E., Tattersall, R. S., and Manson, J. J. (2020). COVID-19: consider cytokine storm syndromes and immunosuppression. The Lancet 395, 1033–1034. doi:10.1016/s0140-6736(20)30628-0
Mensà, E., Guescini, M., Giuliani, A., Bacalini, M. G., Ramini, D., Corleone, G., et al. (2020). Small extracellular vesicles deliver miR-21 and miR-217 as pro-senescence effectors to endothelial cells. J. Extracell Vesicles 9, 1725285. doi:10.1080/20013078.2020.1725285
Merchant, M. L., Rood, I. M., Deegens, J. K. J., and Klein, J. B. (2017). Isolation and characterization of urinary extracellular vesicles: implications for biomarker discovery. Nat. Rev. Nephrol. 13, 731–749. doi:10.1038/nrneph.2017.148
Mohan, A., Agarwal, S., Clauss, M., Britt, N. S., and Dhillon, N. K. (2020). Extracellular vesicles: novel communicators in lung diseases. Respir. Res. 21, 175. doi:10.1186/s12931-020-01423-y
Mohr, A. M., and Mott, J. L. (2015). Overview of microRNA biology. Semin. Liver Dis. 35, 3–11. doi:10.1055/s-0034-1397344
Mohsenin, V. (2016). The emerging role of microRNAs in hypoxia-induced pulmonary hypertension. Sleep Breath 20, 1059–67. doi:10.1007/s11325-016-1351-y
Mujahid, S., Nielsen, H. C., and Volpe, M. V. (2013). MiR-221 and miR-130a regulate lung airway and vascular development. PLoS One 8, e55911. doi:10.1371/journal.pone.0055911
Mulcahy, L. A., Pink, R. C., and Carter, D. R. (2014). Routes and mechanisms of extracellular vesicle uptake. J. Extracell Vesicles 3, 24641. doi:10.3402/jev.v3.24641
Murao, A., Brenner, M., Aziz, M., and Wang, P. (2020). Exosomes in Sepsis. Front. Immunol. 11, 2140. doi:10.3389/fimmu.2020.02140
Nicoli, S., Knyphausen, C. P., Zhu, L. J., Lakshmanan, A., and Lawson, N. D. (2012). miR-221 is required for endothelial tip cell behaviors during vascular development. Dev. Cell 22, 418–429. doi:10.1016/j.devcel.2012.01.008
Njock, M. S., Guiot, J., Henket, M. A., Nivelles, O., Thiry, M., Dequiedt, F., et al. (2019). Sputum exosomes: promising biomarkers for idiopathic pulmonary fibrosis. Thorax 74, 309–312. doi:10.1136/thoraxjnl-2018-211897
O’brien, K. P., Khan, S., Gilligan, K. E., Zafar, H., Lalor, P., Glynn, C., et al. (2018). Employing mesenchymal stem cells to support tumor-targeted delivery of extracellular vesicle (EV)-encapsulated microRNA-379. Oncogene 37, 2137–2149. doi:10.1038/s41388-017-0116-9
Pan, B. T., and Johnstone, R. M. (1983). Fate of the transferrin receptor during maturation of sheep reticulocytes in vitro: selective externalization of the receptor. Cell 33, 967–978. doi:10.1016/0092-8674(83)90040-5
Pepini, T., Gorbunova, E. E., Gavrilovskaya, I. N., Mackow, J. E., and Mackow, E. R. (2010). Andes virus regulation of cellular microRNAs contributes to hantavirus-induced endothelial cell permeability. J. Virol. 84, 11929–11936. doi:10.1128/JVI.01658-10
Peters, E. B. (2018). Endothelial Progenitor Cells for the Vascularization of Engineered Tissues. Tissue Eng. Part B Rev. 24, 1–24. doi:10.1089/ten.TEB.2017.0127
Pi, X., Xie, L., and Patterson, C. (2018). Emerging roles of vascular endothelium in metabolic homeostasis. Circ. Res. 123, 477–494. doi:10.1161/CIRCRESAHA.118.313237
Piccari, L., Del Pozo, R., Blanco, I., García-Lucio, J., Torralba, Y., Tura-Ceide, O., et al. (2020). Association between systemic and pulmonary vascular dysfunction in COPD. Int. J. Chron. Obstruct. Pulmon. Dis. 15, 2037–2047. doi:10.2147/COPD.S257679
Polverino, F., Laucho-Contreras, M. E., Petersen, H., Bijol, V., Sholl, L. M., Choi, M. E., et al. (2017). A pilot study linking endothelial injury in lungs and kidneys in chronic obstructive pulmonary disease. Am. J. Respir. Crit. Care Med. 195, 1464–1476. doi:10.1164/rccm.201609-1765OC
Qaisar, R., Karim, A., Muhammad, T., and Shah, I. (2020). Circulating biomarkers of accelerated sarcopenia in respiratory diseases. Biology (Basel) 9, 322. doi:10.3390/biology9100322
Qin, W., Tsukasaki, Y., Dasgupta, S., Mukhopadhyay, N., Ikebe, M., and Sauter, E. R. (2016). Exosomes in human breast milk promote EMT. Clin. Cancer Res. 22, 4517–4524. doi:10.1158/1078-0432.CCR-16-0135
Rabinovitch, M., Guignabert, C., Humbert, M., and Nicolls, M. R. (2014). Inflammation and immunity in the pathogenesis of pulmonary arterial hypertension. Circ. Res. 115, 165–175. doi:10.1161/CIRCRESAHA.113.301141
Rajendran, P., Rengarajan, T., Thangavel, J., Nishigaki, Y., Sakthisekaran, D., Sethi, G., et al. (2013). The vascular endothelium and human diseases. Int. J. Biol. Sci. 9, 1057–1069. doi:10.7150/ijbs.7502
Ranchoux, B., Harvey, L. D., Ayon, R. J., Babicheva, A., Bonnet, S., Chan, S. Y., et al. (2018). Endothelial dysfunction in pulmonary arterial hypertension: an evolving landscape (2017 Grover Conference Series). Pulm. Circ. 8, 2045893217752912. doi:10.1177/2045893217752912
Ranghino, A., Cantaluppi, V., Grange, C., Vitillo, L., Fop, F., Biancone, L., et al. (2012). Endothelial progenitor cell-derived microvesicles improve neovascularization in a murine model of hindlimb ischemia. Int. J. Immunopathol. Pharmacol. 25, 75–85. doi:10.1177/039463201202500110
Raposo, G., and Stoorvogel, W. (2013). Extracellular vesicles: exosomes, microvesicles, and friends. J. Cell Biol. 200, 373–383. doi:10.1083/jcb.201211138
Reinhart, B. J., Slack, F. J., Basson, M., Pasquinelli, A. E., Bettinger, J. C., Rougvie, A. E., et al. (2000). The 21-nucleotide let-7 RNA regulates developmental timing in Caenorhabditis elegans. Nature 403, 901–906. doi:10.1038/35002607
Renzoni, E., Srihari, V., and Sestini, P. (2014). Pathogenesis of idiopathic pulmonary fibrosis: review of recent findings. F1000Prime Rep. 6, 69. doi:10.12703/P6-69
Rohlenova, K., Veys, K., Miranda-Santos, I., De Bock, K., and Carmeliet, P. (2018). Endothelial Cell Metabolism in Health and Disease. Trends Cell Biol. 28, 224–236. doi:10.1016/j.tcb.2017.10.010
Rupani, H., Sanchez-Elsner, T., and Howarth, P. (2013). MicroRNAs and respiratory diseases. Eur. Respir. J. 41, 695–705. doi:10.1183/09031936.00212011
Salido-Guadarrama, I., Romero-Cordoba, S., Peralta-Zaragoza, O., Hidalgo-Miranda, A., and Rodríguez-Dorantes, M. (2014). MicroRNAs transported by exosomes in body fluids as mediators of intercellular communication in cancer. Oncol. Targets Ther. 7, 1327–1338. doi:10.2147/OTT.S61562
Schuliga, M. (2015). NF-kappaB signaling in chronic inflammatory airway disease. Biomolecules 5, 1266–1283. doi:10.3390/biom5031266
Serban, K. A., Rezania, S., Petrusca, D. N., Poirier, C., Cao, D., Justice, M. J., et al. (2016). Structural and functional characterization of endothelial microparticles released by cigarette smoke. Sci. Rep. 6, 31596. doi:10.1038/srep31596
Shaver, C. M., Woods, J., Clune, J. K., Grove, B. S., Wickersham, N. E., Mcneil, J. B., et al. (2017). Circulating microparticle levels are reduced in patients with ARDS. Crit. Care 21, 120. doi:10.1186/s13054-017-1700-7
Simonneau, G., Montani, D., Celermajer, D. S., Denton, C. P., Gatzoulis, M. A., Krowka, M., et al. (2019). Haemodynamic definitions and updated clinical classification of pulmonary hypertension. Eur. Respir. J. 53, 1801913. doi:10.1183/13993003.01913-2018
Skotland, T., Hessvik, N. P., Sandvig, K., and Llorente, A. (2019). Exosomal lipid composition and the role of ether lipids and phosphoinositides in exosome biology. J. Lipid Res. 60, 9–18. doi:10.1194/jlr.R084343
Song, Q., Christiani, D. C., Xiaorong, W., and Ren, J. (2014). The global contribution of outdoor air pollution to the incidence, prevalence, mortality and hospital admission for chronic obstructive pulmonary disease: a systematic review and meta-analysis. Int. J. Environ. Res. Public Health 11, 11822–11832. doi:10.3390/ijerph111111822
Soriano, J. B., Kendrick, P. J., Paulson, K. R., Gupta, V., Abrams, E. M., Adedoyin, R. A., et al. (2020). Prevalence and attributable health burden of chronic respiratory diseases, 1990-2017: a systematic analysis for the Global Burden of Disease Study 2017. The Lancet Resp. Med. 8, 585–596. doi:10.1016/s2213-2600(20)30105-3
Stocks, J., and Sonnappa, S. (2013). Early life influences on the development of chronic obstructive pulmonary disease. Ther. Adv. Respir. Dis. 7, 161–173. doi:10.1177/1753465813479428
Street, J. M., Koritzinsky, E. H., Glispie, D. M., and Yuen, P. S. T. (2017). Urine Exosome Isolation and Characterization. Methods Mol. Biol. 1641, 413–423. doi:10.1007/978-1-4939-7172-5_23
Strulovici-Barel, Y., Staudt, M. R., Krause, A., Gordon, C., Tilley, A. E., Harvey, B. G., et al. (2016). Persistence of circulating endothelial microparticles in COPD despite smoking cessation. Thorax 71, 1137–1144. doi:10.1136/thoraxjnl-2015-208274
Suresh, K., and Shimoda, L. A. (2016). Lung Circulation. Compr. Physiol. 6, 897–943. doi:10.1002/cphy.c140049
Svensson, D., Gidlöf, O., Turczyńska, K. M., Erlinge, D., Albinsson, S., and Nilsson, B. O. (2014). Inhibition of microRNA-125a promotes human endothelial cell proliferation and viability through an antiapoptotic mechanism. J. Vasc. Res. 51, 239–245. doi:10.1159/000365551
Tabet, F., Vickers, K. C., Cuesta Torres, L. F., Wiese, C. B., Shoucri, B. M., Lambert, G., et al. (2014). HDL-transferred microRNA-223 regulates ICAM-1 expression in endothelial cells. Nat. Commun. 5, 3292. doi:10.1038/ncomms4292
Takahashi, T., Kobayashi, S., Fujino, N., Suzuki, T., Ota, C., He, M., et al. (2012). Increased circulating endothelial microparticles in COPD patients: a potential biomarker for COPD exacerbation susceptibility. Thorax 67, 1067–1074. doi:10.1136/thoraxjnl-2011-201395
Takasugi, M. (2018). Emerging roles of extracellular vesicles in cellular senescence and aging. Aging Cell 17, e12734. doi:10.1111/acel.12734
Takei, N., Suzuki, M., Makita, H., Konno, S., Shimizu, K., Kimura, H., et al. (2019a). Serum alpha-1 antitrypsin levels and the clinical course of chronic obstructive pulmonary disease. Int. J. Chron. Obstruct. Pulmon. Dis. 14, 2885–2893. doi:10.2147/COPD.S225365
Takei, Y., Yamada, M., Saito, K., Kameyama, Y., Sugiura, H., Makiguchi, T., et al. (2019b). Increase in circulating ACE-positive endothelial microparticles during acute lung injury. Eur. Respir. J. 54, 1801188. doi:10.1183/13993003.01188-2018
Théry, C., Witwer, K. W., Aikawa, E., Alcaraz, M. J., Anderson, J. D., Andriantsitohaina, R., et al. (2018). Minimal information for studies of extracellular vesicles 2018 (MISEV2018): a position statement of the International Society for Extracellular Vesicles and update of the MISEV2014 guidelines. J. Extracell Vesicles 7, 1535750. doi:10.1080/20013078.2018.1535750
Thompson, B. T., Chambers, R. C., and Liu, K. D. (2017). Acute Respiratory Distress Syndrome. N. Engl. J. Med. 377, 562–572. doi:10.1056/NEJMra1608077
To, M., Swallow, E. B., Akashi, K., Haruki, K., Natanek, S. A., Polkey, M. I., et al. (2017). Reduced HDAC2 in skeletal muscle of COPD patients. Respir. Res. 18, 99. doi:10.1186/s12931-017-0588-8
Todorova, D., Simoncini, S., Lacroix, R., Sabatier, F., and Dignat-George, F. (2017). Extracellular Vesicles in Angiogenesis. Circ. Res. 120, 1658–1673. doi:10.1161/CIRCRESAHA.117.309681
Valadi, H., Ekström, K., Bossios, A., Sjöstrand, M., Lee, J. J., and Lötvall, J. O. (2007). Exosome-mediated transfer of mRNAs and microRNAs is a novel mechanism of genetic exchange between cells. Nat. Cell Biol. 9, 654–659. doi:10.1038/ncb1596
Van Balkom, B. W., Eisele, A. S., Pegtel, D. M., Bervoets, S., and Verhaar, M. C. (2015). Quantitative and qualitative analysis of small RNAs in human endothelial cells and exosomes provides insights into localized RNA processing, degradation and sorting. J. Extracell Vesicles 4, 26760. doi:10.3402/jev.v4.26760
Van Deursen, J. M. (2014). The role of senescent cells in ageing. Nature 509, 439–446. doi:10.1038/nature13193
Vencken, S. F., Greene, C. M., and Mckiernan, P. J. (2015). Non-coding RNA as lung disease biomarkers. Thorax 70, 501–503. doi:10.1136/thoraxjnl-2014-206193
Vij, N., Chandramani-Shivalingappa, P., Van Westphal, C., Hole, R., and Bodas, M. (2018). Cigarette smoke-induced autophagy impairment accelerates lung aging, COPD-emphysema exacerbations and pathogenesis. Am. J. Physiol. Cell Physiol. 314, C73–C87. doi:10.1152/ajpcell.00110.2016
Vogelmeier, C. F., Criner, G. J., Martinez, F. J., Anzueto, A., Barnes, P. J., Bourbeau, J., et al. (2017). Global strategy for the diagnosis, management, and prevention of chronic obstructive lung disease 2017 report: GOLD executive summary. Eur. Respir. J. 49, 557–582. doi:10.1183/13993003.00214-2017
Wang, J., Chen, S., and Bihl, J. (2020). Exosome-mediated transfer of ACE2 (angiotensin-converting enzyme 2) from endothelial progenitor cells promotes survival and function of endothelial cell. Oxid. Med. Cell Longev. 2020, 4213541. doi:10.1155/2020/4213541
Wang, S., Aurora, A. B., Johnson, B. A., Qi, X., Mcanally, J., Hill, J. A., et al. (2008). The endothelial-specific microRNA miR-126 governs vascular integrity and angiogenesis. Dev. Cell 15, 261–271. doi:10.1016/j.devcel.2008.07.002
Wang, S., Cao, F., Gu, X., Chen, J., Xu, R, Huang, Y., et al. (2019). LncRNA XIST, as a ceRNA of miR-204, aggravates lipopolysaccharide-induced acute respiratory distress syndrome in mice by upregulating IRF2. Int. J. Clin. Exp. Pathol. 12, 2425–2434.
Wei, C., Henderson, H., Spradley, C., Li, L., Kim, I. K., Kumar, S., et al. (2013). Circulating miRNAs as potential marker for pulmonary hypertension. PLoS One 8, e64396. doi:10.1371/journal.pone.0064396
Weilner, S., Schraml, E., Wieser, M., Messner, P., Schneider, K., Wassermann, K., et al. (2016). Secreted microvesicular miR-31 inhibits osteogenic differentiation of mesenchymal stem cells. Aging Cell 15, 744–754. doi:10.1111/acel.12484
Whiteside, T. L. (2018). Exosome and mesenchymal stem cell cross-talk in the tumor microenvironment. Semin. Immunol. 35, 69–79. doi:01.1016/j.smim.2017.12.003
Williams, A. E., José, R. J., Mercer, P. F., Brealey, D., Parekh, D., Thickett, D. R., et al. (2017). Evidence for chemokine synergy during neutrophil migration in ARDS. Thorax 72, 66–73. doi:10.1136/thoraxjnl-2016-208597
Willms, E., Cabañas, C., Mäger, I., Wood, M. J. A., and Vader, P. (2018). Extracellular vesicle heterogeneity: subpopulations, isolation techniques, and diverse functions in cancer progression. Front. Immunol. 9, 738. doi:10.3389/fimmu.2018.00738
Wong, B. W., Marsch, E., Treps, L., Baes, M., and Carmeliet, P. (2017). Endothelial cell metabolism in health and disease: impact of hypoxia. EMBO J. 36, 2187–2203. doi:10.15252/embj.201696150
Wu, S. F., Noren Hooten, N., Freeman, D. W., Mode, N. A., Zonderman, A. B., and Evans, M. K. (2020). Extracellular vesicles in diabetes mellitus induce alterations in endothelial cell morphology and migration. J. Transl. Med. 18, 230. doi:10.1186/s12967-020-02398-6
Wu, X. J., Zhao, Z. F., Kang, X. J., Wang, H. J., Zhao, J., and Pu, X. M. (2016). MicroRNA-126-3p suppresses cell proliferation by targeting PIK3R2 in Kaposi’s sarcoma cells. Oncotarget 7, 36614–36621. doi:10.18632/oncotarget.9311
Xi, T., Jin, F., Zhu, Y., Wang, J., Tang, L., Wang, Y., et al. (2017). MicroRNA-126-3p attenuates blood-brain barrier disruption, cerebral edema and neuronal injury following intracerebral hemorrhage by regulating PIK3R2 and Akt. Biochem. Biophys. Res. Commun. 494, 144–151. doi:10.1016/j.bbrc.2017.10.064
Xie, Y., Dang, W., Zhang, S., Yue, W., Yang, L., Zhai, X., et al. (2019). The role of exosomal noncoding RNAs in cancer. Mol. Cancer 18, 37. doi:10.1186/s12943-019-0984-4
Xie, Y., Qian, Y., Wang, Y., Liu, K., and Li, X. (2020). Mechanical stretch and LPS affect the proliferation, extracellular matrix remodeling and viscoelasticity of lung fibroblasts. Exp. Ther. Med. 20, 5. doi:10.3892/etm.2020.9133
Xiong, W., Xu, M., Pudasaini, B., Guo, X., and Liu, J. (2018). The influence of anemia on one-year exacerbation rate of patients with COPD-PH. BMC Pulm. Med. 18, 143. doi:10.1186/s12890-018-0693-6
Yamamoto, S., Niida, S., Azuma, E., Yanagibashi, T., Muramatsu, M., Huang, T. T., et al. (2015). Inflammation-induced endothelial cell-derived extracellular vesicles modulate the cellular status of pericytes. Sci. Rep. 5, 8505. doi:10.1038/srep08505
Yu, Y., Jing, L., Zhang, X., and Gao, C. (2017). Simvastatin Attenuates Acute Lung Injury via Regulating CDC42-PAK4 and Endothelial Microparticles. Shock 47, 378–384. doi:10.1097/SHK.0000000000000723
Yun, J., Han, S.-B., Kim, H. J., Go, S.-i., Lee, W. S., Bae, W. K., et al. (2019). Exosomal miR-181b-5p Downregulation in Ascites Serves as a Potential Diagnostic Biomarker for Gastric Cancer-associated Malignant Ascites. J. Gastric Cancer 19, 301–314. doi:10.5230/jgc.2019.19.e27
Zaid, Y., Puhm, F., Allaeys, I., Naya, A., Oudghiri, M., Khalki, L., et al. (2020). Platelets Can Associate with SARS-Cov-2 RNA and Are Hyperactivated in COVID-19. Circ. Res. 127, 1404–1418. doi:10.1161/CIRCRESAHA.120.317703
Zhang, D., Lee, H., Zhu, Z., Minhas, J. K., and Jin, Y. (2017). Enrichment of selective miRNAs in exosomes and delivery of exosomal miRNAs in vitro and in vivo. Am. J. Physiol. Lung Cell Mol. Physiol. 312, L110–l121. doi:10.1152/ajplung.00423.2016
Zhang, H., Penninger, J. M., Li, Y., Zhong, N., and Slutsky, A. S. (2020). Angiotensin-converting enzyme 2 (ACE2) as a SARS-CoV-2 receptor: molecular mechanisms and potential therapeutic target. Intens. Care Med. 46, 586–590. doi:10.1007/s00134-020-05985-9
Zhang, J., Chen, C., Hu, B., Niu, X., Liu, X., Zhang, G., et al. (2016). Exosomes derived from human endothelial progenitor cells accelerate cutaneous wound healing by promoting angiogenesis through Erk1/2 signaling. Int. J. Biol. Sci. 12, 1472–1487. doi:10.7150/ijbs.15514
Zhang, J., Li, S., Li, L., Li, M., Guo, C., Yao, J., et al. (2015). Exosome and exosomal microRNA: trafficking, sorting, and function. Genom. Proteom. Bioinform. 13, 17–24. doi:10.1016/j.gpb.2015.02.001
Zhang, Q., Liu, C., Li, Q., Li, J., Wu, Y., and Liu, J. (2019a). MicroRNA-25-5p counteracts oxidized LDL-induced pathological changes by targeting neuronal growth regulator 1 (NEGR1) in human brain micro-vessel endothelial cells. Biochimie. 165, 141–149. doi:10.1016/j.biochi.2019.07.020
Zhang, Y., Liu, Y., Liu, H., and Tang, W. H. (2019b). Exosomes: biogenesis, biologic function and clinical potential. Cell Biosci. 9, 19. doi:10.1186/s13578-019-0282-2
Zhou, Y., Li, P., Goodwin, A. J., Cook, J. A., Halushka, P. V., Chang, E., et al. (2018). Exosomes from endothelial progenitor cells improve the outcome of a murine model of sepsis. Mol. Ther. 26, 1375–1384. doi:10.1016/j.ymthe.2018.02.020
Keywords: vascular endothelial cell, extracellular vesicle, exosome, microvesicle, microRNA, respiratory disease
Citation: Fujimoto S, Fujita Y, Kadota T, Araya J and Kuwano K (2021) Intercellular Communication by Vascular Endothelial Cell-Derived Extracellular Vesicles and Their MicroRNAs in Respiratory Diseases. Front. Mol. Biosci. 7:619697. doi: 10.3389/fmolb.2020.619697
Received: 20 October 2020; Accepted: 30 December 2020;
Published: 29 January 2021.
Edited by:
David De Gonzalo-Calvo, Lleida Institute for Biomedical Research (IRBLleida), SpainReviewed by:
Mehdi Kabani, Centre National de la Recherche Scientifique (CNRS), FranceMuhammad Nawaz, University of Gothenburg, Sweden
Copyright © 2021 Fujimoto, Fujita, Kadota, Araya and Kuwano. This is an open-access article distributed under the terms of the Creative Commons Attribution License (CC BY). The use, distribution or reproduction in other forums is permitted, provided the original author(s) and the copyright owner(s) are credited and that the original publication in this journal is cited, in accordance with accepted academic practice. No use, distribution or reproduction is permitted which does not comply with these terms.
*Correspondence: Yu Fujita, eXV1Z290QGppa2VpLmFjLmpw