- 1Department of Biotechnology and Bioindustry Sciences, National Cheng Kung University, Tainan, Taiwan
- 2Institute of Biomedical Sciences, National Sun Yat-sen University, Kaohsiung, Taiwan
The evolutionarily conserved Hippo kinase signaling cascade governs cell proliferation, tissue differentiation and organ size, and can promote tumor growth and cancer metastasis when dysregulated. Unlike conventional signaling pathways driven by ligand-receptor binding to initiate downstream cascades, core Hippo kinases are activated not only by biochemical cues but also by mechanical ones generated from altered cell shape, cell polarity, cell-cell junctions or cell-extracellular matrix adhesion. In this review, we focus on recent advances showing how mechanical force acts through the actin cytoskeleton to regulate the Hippo pathway during cell movement and cancer invasion. We also discuss how this force affects YAP-dependent tissue growth and cell proliferation, and how disruption of that homeostatic relationship contributes to cancer metastasis.
Introduction
Hippo (Hpo) signaling was initially identified through a genetic screen for cell size control in Drosophila melanogaster, revealing a conserved pathway that regulates organ size, cell fate, tissue homeostasis and tumor progression. The Hpo network is primarily a core kinase cascade, involving Hpo/mammalian Ste20-like kinases 1/2 (MST1/2), Salvador (Sav)/SAV1, Mats/MOB kinase activator 1A/B (MOB1A/B), and Warts (Wts)/large tumor suppressor kinase 1/2 (LATS1/2). This latter phosphorylates the transcription factor Yorki (Yki)/Yes-associated protein (YAP)/transcriptional coactivator with PDZ-binding motif (TAZ) to prevent its nuclear translocation, thereby blocking target gene activation (Ma et al., 2019) (Figure 1). Over the past decade, extensive research has established links between the Hpo pathway and organ size restriction, mainly based on the key roles of Hpo protein in controlling genes responsible for cell proliferation and anti-apoptosis. Therefore, how cells sense confluence to arrest the cell cycle and how mechanical force impacts on cell-cell contacts through junctional proteins is a critical facet of tissue architecture regulation during animal development. Disruption of epithelial homeostasis induced by overexpression of Yki or mutation of its upstream kinases allows tumor cells to freely proliferate and metastasize. Hpo signaling is unlike conventional ligand-receptor transduction pathways in that it integrates diverse upstream stimuli such as soluble factors, cell-cell adhesion, mechanical tension driven by the actin cytoskeleton, and force conveyed from the extra-cellular matrix (ECM). In this review, we summarize how the Hpo network functions and is regulated, the influence of its upstream inputs on physiology, and the contribution of its dysregulation to cancer metastasis. Since Hpo is an evolutionarily conserved pathway, components we describe herein include both Drosophila molecules and the corresponding vertebrate homologs.
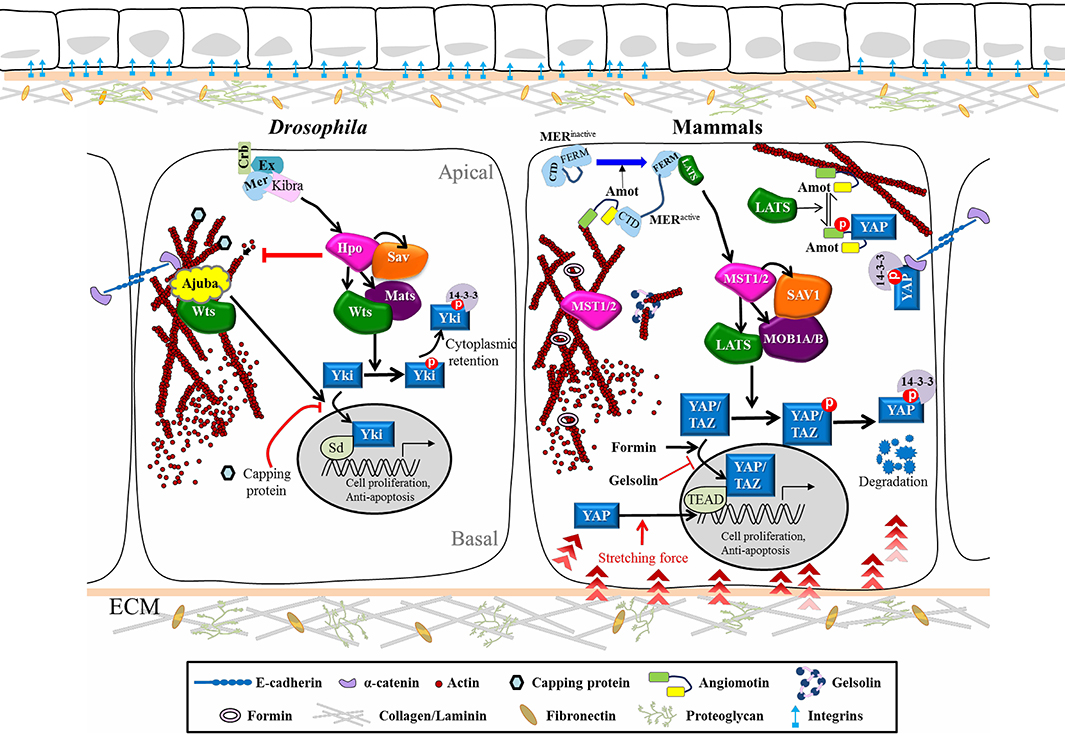
Figure 1. Cytoskeletal Regulation of the Hippo pathway in Drosophila and Mammals. Schematic diagram shows the core kinase cascade of the Hippo pathway and its interplay with upstream cytoskeletal regulators in Drosophila and mammals. Corresponding colors and shapes are used to indicate homologous components in both systems. When Hippo signaling is off, the nuclear effector Yki/YAP/TAZ can bind to the transcription factor Sd/TEAD to turn on target genes. Upon phosphorylation of Hpo/MST1/2 by upstream stimuli, Wts/LATS1/2 is activated and phosphorylates Yki/YAP/TAZ, which makes the later ones retain in the cytosol or further undergo degradation. The cytoskeletal regulators can also control the nuclear translocation of Yki/YAP/TAZ without their phosphorylation by Wts/LATS1/2. See text for further details. Hpo, Hippo; Sav, Salvador; Wts, Warts; Mats, Mob as a tumor suppressor; Yki, Yorkie; Ex, Expanded; Mer, Merlin; Crb, Crumbs; Sd, Scalloped.
Components and Regulation of Hippo Signaling
The first mutant component of the Hpo pathway, wts, was retrieved from a forward genetic screen seeking tumor suppressors, with hpo, sav and mats being later identified based on the same phenotype of tissue overgrowth in mosaic mutant clones (Justice et al., 1995; Xu et al., 1995; Kango-Singh et al., 2002; Tapon et al., 2002; Harvey et al., 2003; Jia et al., 2003; Pantalacci et al., 2003; Lai et al., 2005; Zheng and Pan, 2019). Two of these components, hpo and wts, encode a serine/threonine kinase that belongs to the Ste-20 kinases and Nuclear Dbf2-related (NDR) family of proteins, respectively (Snigdha et al., 2019). Further studies have shown that the Hpo and Wts proteins both bind to the WW domain adaptor protein, Sav. Hpo phosphorylates Sav, which in turn phosphorylates Wts to activate the downstream Mats-Wts complex. The activated Wts kinase promotes Yki phosphorylation at Ser168, which induces association of Yki with 14-3-3 proteins, thereby reducing Yki nuclear localization (Oh and Irvine, 2008; Ren et al., 2010) (Figure 1). Very similar to the mechanism of Yki regulation in Drosophila, mammalian YAP is inactivated by LATS1/2 phosphorylation, resulting in 14-3-3 binding, retention in the cytosol, and subsequent protein degradation (Ma et al., 2019). Upon reduced Hpo signaling activity, the transcriptional coactivator Yki/YAP enters the nucleus where it works with DNA-binding transcription factors to activate genes involved in the cell cycle, cell proliferation and suppression of apoptosis (McClatchey and Yap, 2012). Recent live multiphoton microscopy analysis has further revealed that knock-in tagged Yki-Venus shuttles rapidly between the cytosol and the nucleus during interphase and then is enriched on mitotic chromatin, suggesting a role for Yki in regulating mitosis (Manning et al., 2018).
Cell-Cell Contact and the Hippo Pathway
There is clear evidence illustrating that the cell cycle is arrested in G1 when cell growth reaches full confluence, which is defined as contact inhibition (Eagle and Levine, 1967). Hpo signaling has been implicated in contact inhibition because of YAP exclusion from the nucleus when cultured cells contact each other through adhesion molecules (Zhao et al., 2007). Expression of E-Cadherin in the breast cancer cell line MDA-MB-231 prevents nuclear localization of YAP. Antibody treatment against the extra-cellular domain of E-Cadherin inhibits proliferation of another breast cancer cell line (MCF-7) by redistributing YAP from the nucleus to the cytoplasm (Kim et al., 2011). α-Catenin that is attached to the cytoplasmic domain of E-Cadherin is the density sensor of skin cells, and it has been demonstrated that YAP localization and phosphorylation in keratinocytes is regulated by its interaction with α-Catenin. α-Catenin binds to phosphorylated YAP1 in the presence of 14-3-3, which prevents YAP1 activation and nuclear translocation. Overexpression of α-Catenin in low-density culture brings YAP to the plasma membrane, an outcome not observed upon overexpression of other components of adherens junctions such as E-Cadherin (Schlegelmilch et al., 2011). These findings suggest that α-Catenin is the mediator that transduces mechanical cues from E-Cadherin in fully confluent cultures, keeping YAP/α-Catenin/14-3-3 close to the plasma membrane and thereby preventing YAP activity in the nucleus.
Ajuba is a LIM domain scaffold protein that is known to be recruited to adherens junctions through its association with α-Catenin (Marie et al., 2003). Increasing tension mediated by cytoskeleton in Drosophila wing discs recruits Warts to adherens junctions by Ajuba in a tension-dependent manner, which can suppress Warts activity and hence lead to activation of Yki downstream genes (Rauskolb et al., 2014; Alegot et al., 2019). This scenario supports the idea that mechanical force may stimulate cell proliferation in cell cultures (Boggiano and Fehon, 2012; McClatchey and Yap, 2012).
Mechanical Force Regulates Hippo Signaling
During tissue morphogenesis or organ development, cells constantly respond to mechanical stress from neighboring cells and the ECM, or to shear force when they migrate. Tension from different tissue geometries and degrees of matrix stiffness is transmitted through membrane receptors, the actin cytoskeleton and the nuclear membrane to affect gene expression within nuclei, which not only shapes tissue morphology but also determines cell cycle entry and cell fate specification. Recent research has unraveled the localizations of Hpo pathway components at cellular junctions, helping to more clearly depict how cellular morphology, the outside environment and F-actin architecture act together to control Hpo signaling activity (Figure 1). One cell culture study showed that mammalian MST1/2 is colocalized with filamentous actin and that disruption of actin stress fibers leads to MST1/2 activation (Densham et al., 2009). Studies in Drosophila have revealed that mutation in Capping proteins, a negative regulator of actin polymerization, causes F-actin accumulation, leading to upregulation of Yki target genes and tissue outgrowth in imaginal discs (Fernandez et al., 2011; Sansores-Garcia et al., 2011). Moreover, stress fibers or cell morphology itself can also promote YAP activity in mammalian cells in a LATS-dependent manner (Wada et al., 2011). Diaphanous, the mammalian Formin protein, facilitates actin filament assembly and also promotes YAP nuclear translocation, whereas the actin-severing components Gelsolin and Cofilin act as essential gate-keepers to antagonize the function of Yki/YAP in cell growth (Aragona et al., 2013; Gaspar and Tapon, 2014). According to these studies, actin polymerization positively regulates Yki/YAP activity. The upstream regulators relaying signals from membrane receptors to the cytoskeleton network were first identified in Drosophila, including Merlin (Mer) and Expanded (Ex) that coordinate cell proliferation. ex and mer double mutant cells exhibit tissue outgrowth and excessive BrdU staining, a phenotype similar to that caused by suppression of Hippo core kinase activity. Moreover, co-expression of Ex and Mer results in increased Warts phosphorylation, so Mer and Ex head the Hpo pathway (Hamaratoglu et al., 2006). Subsequently, hpo, sav, mats, wts or ex mutant clones were shown to display an F-actin accumulation phenotype, indicating that the Hpo pathway negatively controls actin filament assembly (Fernandez et al., 2011). However, overexpression of Moesin—an ERM (ezrin, radixin, moesin) protein that is localized at the apical domain of epithelia and promotes actin assembly—does not induce tissue outgrowth (Speck et al., 2003; Boggiano and Fehon, 2012). These observations suggest complex regulation of F-actin- and Hippo-dependent cell size control. Several lines of evidence indicate that the impact of cell morphology and mechanical stress on the Hpo pathway can also be eliminated by simply blocking Rho activity, which is required to build up the contractile network as cells react to a stiff matrix (Zhao et al., 2012; Aragona et al., 2013; Sun and Irvine, 2016). Interestingly, this regulation has been reported as being either LATS kinase-independent (Dupont et al., 2011) or -dependent (Wada et al., 2011; Zhao et al., 2012). Identification of Angiomotin (Amot) has clarified the relationship between F-actin and the Hpo pathway. Amot contains a conserved binding domain that can interact with F-actin or YAP. LATS-mediated phosphorylation of Amot prevents it from binding F-actin but promotes its association with YAP, leading to YAP retention in the cytosol (Mana-Capelli et al., 2014). This scenario potentially explains how the actin cytoskeleton is involved in LATS-independent regulation of YAP. In spatial control around the plasma membrane, Amot and the actin complex are regulated by Mer, a member of the ERM family of proteins that links the plasma membrane and actin filaments. ERM protein activity, including that of Mer, is negatively regulated by auto-inhibition via intramolecular binding between the N and C termini. Structure and function analyses have shown that interaction of Amot with Mer relieves such auto-inhibition and promotes Amot interaction with LATS through its C-terminal FERM domain (Li et al., 2015). Direct recruitment by Mer of LATS to the membrane further stimulates MST1/2-mediated phosphorylation of the LATS complex. Robust Mer-Wts/LATS binding by treatment with latrunculin B, an actin polymerization inhibitor, has been observed (Yin et al., 2013; Sun and Irvine, 2016), indicating that F-actin can potentiate this interaction. In addition, transmission of mechanical signals from the cytoskeleton to the nuclear membrane may also regulate Yki/YAP activity. Actomyosin filaments form a physical link with the nuclear envelope through the LINC (linker of nucleoskeleton and cytoskeleton) complex consisting of Lamin, Sun and Nesprin. Nesprin-1 and−2 are expressed ubiquitously in human. Both proteins encode an actin binding domain (ABD) at the N-terminus, a rod domain with several spectrin repeats that provide an interaction hub for Emerin and Lamin, and a C-terminal KASH domain inserted into the outer nuclear membrane (Janin and Gache, 2018). Nesprin-1 has recently been reported as playing a role in promoting nuclear localization of YAP in response to mechanical stretching to induce nuclear deformation in cultured cells (Driscoll et al., 2015). Furthermore, ECM-nuclear coupled stretching that flattens nuclei is sufficient to trigger nuclear import of YAP by diminishing the gating function of Nuclear Pore Complex (NPC) for selective transport (Elosegui-Artola et al., 2017). This latter study opens up a new avenue for investigating how mechanotransduction manipulates YAP nuclear transport, which may represent the mechanism by which Yki/YAP responds to mechanical cues when cells migrate through a crowded space in a short period of time.
The Hippo Pathway in Cell Migration and Cancer Metastasis
For tumor cells to successfully metastasize, the epithelial to mesenchymal transition (EMT), invasion, intravasation and immune system-mediated evasion/extravasation must take place (Warren et al., 2018; Zanconato et al., 2019). Apart from its canonical role in tumor size control, Hpo signaling has been reported to contribute to cancer dissemination. Junction dissociation, apical-basal polarity loss and cytoskeleton network rearrangements are hallmarks of EMT. Several studies have demonstrated that, together with EMT-associated gene mutation, activation of YAP/TAZ or dysregulation of MST1/2 or LATS1/2 leads to cancer metastasis. Consistent with this scenario, knockdown of YAP/TAZ reverses the mesenchymal morphology of cancer cells (Lamar et al., 2012; Janse van Rensburg and Yang, 2016). In a screen for functional substitutes of KRAS, YAP was shown to promote survival of RAS-dependent colon cancer cell lines. Interestingly, YAP transcriptional profiling revealed that activation of the EMT-inducing transcription factor Fos promotes expression of mesenchymal genes and downregulates junctional proteins like E-Cadherin and Occludin (Shao et al., 2014). Moreover, expression of canonical EMT-inducing transcription factors such as Snail1/2, Slug, ZEB1 (Zinc Finger E-box-binding Homeobox 1) and Twist is driven by YAP in a variety of cancers (Warren et al., 2018). Taken together, these studies indicate that the Hpo pathway is involved in activation of the EMT program.
Apart from being activated by F-actin-mediated mechanical tension, YAP and Yki also regulate the actin dynamics that allow cancer cells to change shape and invade surrounding tissues (Lamar et al., 2012; Warren et al., 2018). In a model of circulating cancer cells, YAP has been shown to transcriptionally activate ARHGAP29, an inhibitor of RhoA, thereby reducing the rigidity of the cytoskeleton network by escalating G- and F-actin turnover (Qiao et al., 2017). This model has been well acknowledged for investigating the mechanism of distal metastasis in cancers (Nagrath et al., 2007; Massague and Obenauf, 2016), and it provides a clear insight into how YAP promotes cancer cell motility by affecting actin dynamics. Another study using a soft-polymer microfluidics system to mimic frictional force in blood vessels shows that YAP is activated by fluid shear stress and that it is required for fluid flow-induced cell motility (Lee et al., 2017). As a mechanosensor, YAP receives signals from surrounding tissues and counteracts this stress by activating ARHGAP29 to control actin dynamics, which is critical for cells to retain flexibility or to extend protrusions for metastasis. During Drosophila border cell migration, Hpo core kinases have been shown to polarize the distribution of F-actin, allowing cells to extend forward protrusions. At cell-cell contacts that do not extend cellular protrusions, Wts is required to phosphorylate Ena (Enabled)/VASP (vasodilator-stimulated phosphoprotein), inhibiting the function of the latter in promoting actin filament assembly (Lucas et al., 2013) (Figure 2A). This genetic analysis suggests that inactivation of Hpo signaling at the outer rim of the migrating cell cluster promotes protrusion formation. However, how Hpo signaling is downregulated remains unclear. An independent study that screened for Rap1 (Ras-related protein 1)-interacting proteins in this collective cell migration system identified Hpo as binding to active-form Rap1 (Chang et al., 2018). That interaction greatly impedes Hpo auto-phosphorylation, a critical step required for activation of Hpo signaling, which induces persistent protrusions at the leading edge of migrating cohorts (Figure 2A). Rap1, a Ras family small GTPase, has been demonstrated to regulate adherens junction formation and integrin signaling and to promote tumorigenesis in various cancers under Ras hyperactivity. Interestingly, Rap2, a close homolog of Rap1, is antagonized by Rap1 in endothelial cells (Pannekoek et al., 2013), and it serves as a mechanosensor to relay stress from ECM, as well as activating Lats1/2 in response to low stiffness (Meng et al., 2018) (Figure 2B). However, there is only one Rap1 gene in the Drosophila melanogaster genome, and studies of border cell migration in that system have exposed its role in suppressing Hpo signaling activity (Chang et al., 2018). Thus, Rap1 function might be context-dependent, so it is of great interest to establish if the underlying molecular mechanism is conserved in other Drosophila organs/tissues.
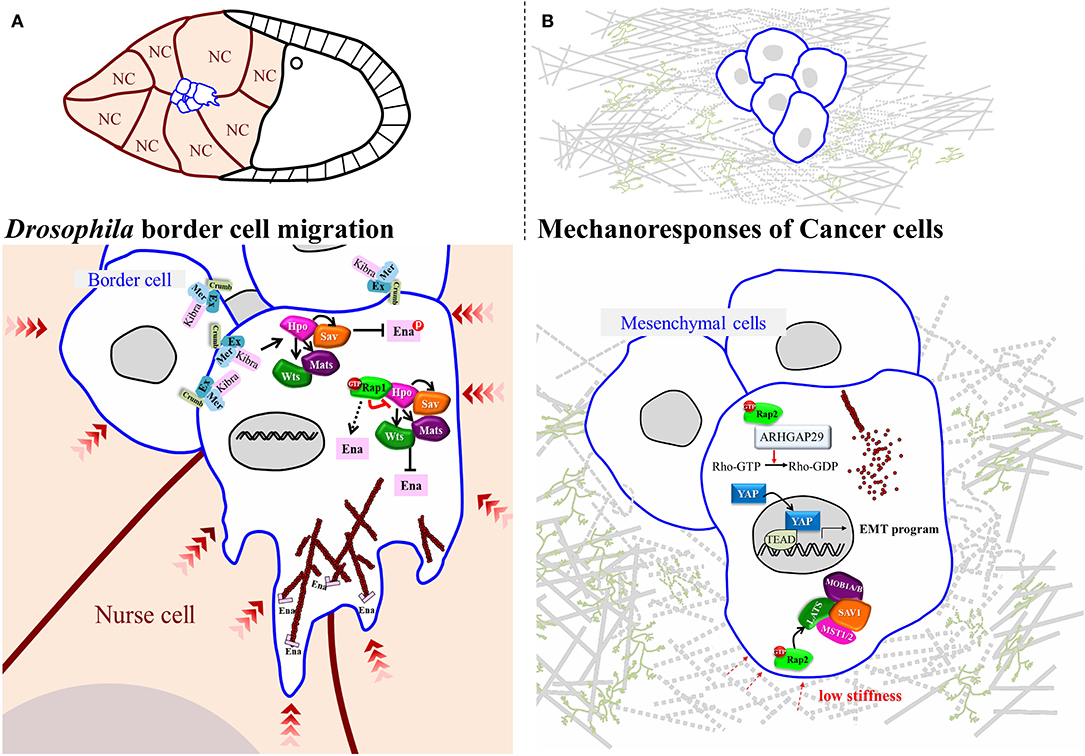
Figure 2. Regulatory Mechanisms for Small GTPase-Hippo-mediated Mechanoresponses in Drosophila and Mammals. (A) In Drosophila oogenesis, a small group of cells, named border cells, delaminate from the follicular epithelium and migrate through the germline cluster, nurse cells. During migration, the Hippo signaling is activated inside the migrating cluster where border cells form contact with each other. On the contrast, in the leading edge Rap1 mechanically suppresses Hippo pathway to promote actin-dependent protrusion. (B) In mammalian cells, at low stiffness Rap2 binds to and activates ARHGAP29 to turn on Hippo signaling, leading to actin depolymerization.
Concluding Remarks
From a simple screen for cell size control in the fly, the Hpo signaling pathway has now expanded into a complex and highly conserved kinase network, comprising dozens of components and integrating diverse upstream signals that are required for many developmental, homeostatic and pathological processes. Our review has focused on summarizing how upstream signals, such as cellular junctions, cytoskeleton proteins and mechanical forces, link Hpo core kinase activity and YAP/Yki nuclear transport. The most challenging task is to unravel how all contributory mechanical and chemical cues act together to regulate the Hpo pathway in vivo. Hpo pathway activity is switched on and off by dynamic phosphorylation and dephosphorylation, so phosphatases are likely to play a vital role. Another unresolved issue is how mechanical stress is detected in vivo to influence Hpo signaling. Although analysis of knock-in tagged Yki-Venus illustrates the effect of tissue folding on Yki nuclear-cytoplasmic transport during morphogenesis (Fletcher et al., 2018; Manning et al., 2018), it remains difficult to identify direct spatial control on the Hpo pathway via mechanical force. The newly invented optogenetic tool, GFP-LARIAT (GFP-light-activated reversible inhibition by assembled trap) inhibits the function of GFP-tagged proteins by sequestering target proteins into a blue light-mediated multimeric module (Lee et al., 2014). This approach has been applied to spatiotemporally inhibit cytoskeleton proteins, myosin, and E-cadherin, among others (Qin et al., 2017). It would be worth applying this approach to disrupt the actin cytoskeleton network in a small region of the plasma membrane to investigate the impact of mechanical force on Hpo signaling, as it would allow visualization of the Hpo reporter in real time.
Author Contributions
Y-CC and AJ co-wrote the manuscript. J-WW and C-WW accomplished all art work, critical reading, and comment on this manuscript.
Funding
This work was supported by grants to AJ by the Ministry of Science and Technology, Taiwan, ROC (MOST 107-2311-B-006−002 -MY3) and NHRI-108A1-MRCO-0319192.
Conflict of Interest
The authors declare that the research was conducted in the absence of any commercial or financial relationships that could be construed as a potential conflict of interest.
References
Alegot, H., Markosian, C., Rauskolb, C., Yang, J., Kirichenko, E., Wang, Y. C., et al. (2019). Recruitment of Jub by alpha-catenin promotes Yki activity and Drosophila wing growth. J. Cell. Sci. 132:jcs222018. doi: 10.1242/jcs.222018
Aragona, M., Panciera, T., Manfrin, A., Giulitti, S., Michielin, F., Elvassore, N., et al. (2013). A mechanical checkpoint controls multicellular growth through YAP/TAZ regulation by actin-processing factors. Cell 154, 1047–1059. doi: 10.1016/j.cell.2013.07.042
Boggiano, J. C., and Fehon, R. G. (2012). Growth control by committee: intercellular junctions, cell polarity, and the cytoskeleton regulate Hippo signaling. Dev. Cell 22, 695–702. doi: 10.1016/j.devcel.2012.03.013
Chang, Y. C., Wu, J. W., Hsieh, Y. C., Huang, T. H., Liao, Z. M., Huang, Y. S., et al. (2018). Rap1 negatively regulates the Hippo pathway to polarize directional protrusions in collective cell migration. Cell Rep. 22, 2160–2175. doi: 10.1016/j.celrep.2018.01.080
Densham, R. M., O'Neill, E., Munro, J., Konig, I., Anderson, K., Kolch, W., et al. (2009). MST kinases monitor actin cytoskeletal integrity and signal via c-Jun N-terminal kinase stress-activated kinase to regulate p21Waf1/Cip1 stability. Mol. Cell. Biol. 29, 6380–6390. doi: 10.1128/MCB.00116-09
Driscoll, T. P., Cosgrove, B. D., Heo, S. J., Shurden, Z. E., and Mauck, R. L. (2015). Cytoskeletal to nuclear strain transfer regulates YAP signaling in mesenchymal stem cells. Biophys. J. 108, 2783–2793. doi: 10.1016/j.bpj.2015.05.010
Dupont, S., Morsut, L., Aragona, M., Enzo, E., Giulitti, S., Cordenonsi, M., et al. (2011). Role of YAP/TAZ in mechanotransduction. Nature 474, 179–183. doi: 10.1038/nature10137
Eagle, H., and Levine, E. M. (1967). Growth regulatory effects of cellular interaction. Nature 213, 1102–1106. doi: 10.1038/2131102a0
Elosegui-Artola, A., Andreu, I., Beedle, A. E. M., Lezamiz, A., Uroz, M., Kosmalska, A. J., et al. (2017). Force triggers YAP nuclear entry by regulating transport across nuclear pores. Cell 171, 1397–1410.e1314. doi: 10.1016/j.cell.2017.10.008
Fernandez, B. G., Gaspar, P., Bras-Pereira, C., Jezowska, B., Rebelo, S. R., and Janody, F. (2011). Actin-capping protein and the Hippo pathway regulate F-actin and tissue growth in Drosophila. Development 138, 2337–2346. doi: 10.1242/dev.063545
Fletcher, G. C., Diaz-de-la-Loza, M. D., Borreguero-Munoz, N., Holder, M., Aguilar-Aragon, M., and Thompson, B. J. (2018). Mechanical strain regulates the Hippo pathway in Drosophila. Development 145:dev159467. doi: 10.1242/dev.159467
Gaspar, P., and Tapon, N. (2014). Sensing the local environment: actin architecture and Hippo signalling. Curr. Opin. Cell. Biol. 31, 74–83. doi: 10.1016/j.ceb.2014.09.003
Hamaratoglu, F., Willecke, M., Kango-Singh, M., Nolo, R., Hyun, E., Tao, C., et al. (2006). The tumour-suppressor genes NF2/Merlin and expanded act through Hippo signalling to regulate cell proliferation and apoptosis. Nat. Cell Biol. 8, 27–36. doi: 10.1038/ncb1339
Harvey, K. F., Pfleger, C. M., and Hariharan, I. K. (2003). The Drosophila Mst ortholog, hippo, restricts growth and cell proliferation and promotes apoptosis. Cell 114, 457–467. doi: 10.1016/S0092-8674(03)00557-9
Janin, A., and Gache, V. (2018). Nesprins and lamins in health and diseases of cardiac and skeletal muscles. Front. Physiol. 9:1277. doi: 10.3389/fphys.2018.01277
Janse van Rensburg, H. J., and Yang, X. (2016). The roles of the Hippo pathway in cancer metastasis. Cell Signal 28, 1761–1772. doi: 10.1016/j.cellsig.2016.08.004
Jia, J., Zhang, W., Wang, B., Trinko, R., and Jiang, J. (2003). The Drosophila Ste20 family kinase dMST functions as a tumor suppressor by restricting cell proliferation and promoting apoptosis. Genes Dev. 17, 2514–2519. doi: 10.1101/gad.1134003
Justice, R. W., Zilian, O., Woods, D. F., Noll, M., and Bryant, P. J. (1995). The Drosophila tumor suppressor gene warts encodes a homolog of human myotonic dystrophy kinase and is required for the control of cell shape and proliferation. Genes Dev. 9, 534–546. doi: 10.1101/gad.9.5.534
Kango-Singh, M., Nolo, R., Tao, C., Verstreken, P., Hiesinger, P. R., Bellen, H. J., et al. (2002). Shar-pei mediates cell proliferation arrest during imaginal disc growth in Drosophila. Development 129, 5719–5730. doi: 10.1242/dev.00168
Kim, N. G., Koh, E., Chen, X., and Gumbiner, B. M. (2011). E-cadherin mediates contact inhibition of proliferation through Hippo signaling-pathway components. Proc. Natl. Acad. Sci. U.S.A. 108, 11930–11935. doi: 10.1073/pnas.1103345108
Lai, Z. C., Wei, X., Shimizu, T., Ramos, E., Rohrbaugh, M., Nikolaidis, N., et al. (2005). Control of cell proliferation and apoptosis by mob as tumor suppressor, mats. Cell 120, 675–685. doi: 10.1016/j.cell.2004.12.036
Lamar, J. M., Stern, P., Liu, H., Schindler, J. W., Jiang, Z. G., and Hynes, R. O. (2012). The Hippo pathway target, YAP, promotes metastasis through its TEAD-interaction domain. Proc. Natl. Acad. Sci. U.S.A. 109, E2441–E2450. doi: 10.1073/pnas.1212021109
Lee, H. J., Diaz, M. F., Price, K. M., Ozuna, J. A., Zhang, S., Sevick-Muraca, E. M., et al. (2017). Fluid shear stress activates YAP1 to promote cancer cell motility. Nat. Commun. 8:14122. doi: 10.1038/ncomms14122
Lee, S., Park, H., Kyung, T., Kim, N. Y., Kim, S., Kim, J., et al. (2014). Reversible protein inactivation by optogenetic trapping in cells. Nat Methods 11, 633–636. doi: 10.1038/nmeth.2940
Li, Y., Zhou, H., Li, F., Chan, S. W., Lin, Z., Wei, Z., et al. (2015). Angiomotin binding-induced activation of Merlin/NF2 in the Hippo pathway. Cell Res. 25, 801–817. doi: 10.1038/cr.2015.69
Lucas, E. P., Khanal, I., Gaspar, P., Fletcher, G. C., Polesello, C., Tapon, N., et al. (2013). The Hippo pathway polarizes the actin cytoskeleton during collective migration of Drosophila border cells. J. Cell. Biol. 201, 875–885. doi: 10.1083/jcb.201210073
Ma, S., Meng, Z., Chen, R., and Guan, K. L. (2019). The Hippo pathway: biology and pathophysiology. Annu. Rev. Biochem. 88, 577–604. doi: 10.1146/annurev-biochem-013118-111829
Mana-Capelli, S., Paramasivam, M., Dutta, S., and McCollum, D. (2014). Angiomotins link F-actin architecture to Hippo pathway signaling. Mol. Biol. Cell. 25, 1676–1685. doi: 10.1091/mbc.e13-11-0701
Manning, S. A., Dent, L. G., Kondo, S., Zhao, Z. W., Plachta, N., and Harvey, K. F. (2018). Dynamic fluctuations in subcellular localization of the Hippo pathway Effector Yorkie in vivo. Curr. Biol. 28, 1651–1660 e1654. doi: 10.1016/j.cub.2018.04.018
Marie, H., Pratt, S. J., Betson, M., Epple, H., Kittler, J. T., Meek, L., et al. (2003). The LIM protein Ajuba is recruited to cadherin-dependent cell junctions through an association with alpha-catenin. J. Biol. Chem. 278, 1220–1228. doi: 10.1074/jbc.M205391200
Massague, J., and Obenauf, A. C. (2016). Metastatic colonization by circulating tumour cells. Nature 529, 298–306. doi: 10.1038/nature17038
McClatchey, A. I., and Yap, A. S. (2012). Contact inhibition (of proliferation) redux. Curr. Opin. Cell. Biol. 24, 685–694. doi: 10.1016/j.ceb.2012.06.009
Meng, Z., Qiu, Y., Lin, K. C., Kumar, A., Placone, J. K., Fang, C., et al. (2018). RAP2 mediates mechanoresponses of the Hippo pathway. Nature 560, 655–660. doi: 10.1038/s41586-018-0444-0
Nagrath, S., Sequist, L. V., Maheswaran, S., Bell, D. W., Irimia, D., Ulkus, L., et al. (2007). Isolation of rare circulating tumour cells in cancer patients by microchip technology. Nature 450, 1235–1239. doi: 10.1038/nature06385
Oh, H., and Irvine, K. D. (2008). In vivo regulation of Yorkie phosphorylation and localization. Development 135, 1081–1088. doi: 10.1242/dev.015255
Pannekoek, W. J., Linnemann, J. R., Brouwer, P. M., Bos, J. L., and Rehmann, H. (2013). Rap1 and Rap2 antagonistically control endothelial barrier resistance. PLoS ONE 8:e57903. doi: 10.1371/journal.pone.0057903
Pantalacci, S., Tapon, N., and Leopold, P. (2003). The Salvador partner Hippo promotes apoptosis and cell-cycle exit in Drosophila. Nat. Cell Biol. 5, 921–927. doi: 10.1038/ncb1051
Qiao, Y., Chen, J., Lim, Y. B., Finch-Edmondson, M. L., Seshachalam, V. P., Qin, L., et al. (2017). YAP regulates actin dynamics through ARHGAP29 and promotes metastasis. Cell Rep. 19, 1495–1502. doi: 10.1016/j.celrep.2017.04.075
Qin, X., Park, B. O., Liu, J., Chen, B., Choesmel-Cadamuro, V., Belguise, K., et al. (2017). Cell-matrix adhesion and cell-cell adhesion differentially control basal myosin oscillation and Drosophila egg chamber elongation. Nat. Commun. 8:14708. doi: 10.1038/ncomms14708
Rauskolb, C., Sun, S., Sun, G., Pan, Y., and Irvine, K. D. (2014). Cytoskeletal tension inhibits Hippo signaling through an Ajuba-Warts complex. Cell 158, 143–156. doi: 10.1016/j.cell.2014.05.035
Ren, F., Zhang, L., and Jiang, J. (2010). Hippo signaling regulates Yorkie nuclear localization and activity through 14–3-3 dependent and independent mechanisms. Dev. Biol. 337, 303–312. doi: 10.1016/j.ydbio.2009.10.046
Sansores-Garcia, L., Bossuyt, W., Wada, K., Yonemura, S., Tao, C., Sasaki, H., et al. (2011). Modulating F-actin organization induces organ growth by affecting the Hippo pathway. EMBO J. 30, 2325–2335. doi: 10.1038/emboj.2011.157
Schlegelmilch, K., Mohseni, M., Kirak, O., Pruszak, J., Rodriguez, J. R., Zhou, D., et al. (2011). Yap1 acts downstream of alpha-catenin to control epidermal proliferation. Cell 144, 782–795. doi: 10.1016/j.cell.2011.02.031
Shao, D. D., Xue, W., Krall, E. B., Bhutkar, A., Piccioni, F., Wang, X., et al. (2014). KRAS and YAP1 converge to regulate EMT and tumor survival. Cell 158, 171–184. doi: 10.1016/j.cell.2014.06.004
Snigdha, K., Gangwani, K. S., Lapalikar, G. V., Singh, A., and Kango-Singh, M. (2019). Hippo signaling in cancer: lessons from Drosophila models. Front. Cell Dev. Biol. 7:85. doi: 10.3389/fcell.2019.00085
Speck, O., Hughes, S. C., Noren, N. K., Kulikauskas, R. M., and Fehon, R. G. (2003). Moesin functions antagonistically to the Rho pathway to maintain epithelial integrity. Nature 421, 83–87. doi: 10.1038/nature01295
Sun, S., and Irvine, K. D. (2016). Cellular organization and cytoskeletal regulation of the Hippo signaling network. Trends Cell Biol. 26, 694–704. doi: 10.1016/j.tcb.2016.05.003
Tapon, N., Harvey, K. F., Bell, D. W., Wahrer, D. C., Schiripo, T. A., Haber, D., et al. (2002). Salvador promotes both cell cycle exit and apoptosis in Drosophila and is mutated in human cancer cell lines. Cell 110, 467–478. doi: 10.1016/S0092-8674(02)00824-3
Wada, K., Itoga, K., Okano, T., Yonemura, S., and Sasaki, H. (2011). Hippo pathway regulation by cell morphology and stress fibers. Development 138, 3907–3914. doi: 10.1242/dev.070987
Warren, J. S. A., Xiao, Y., and Lamar, J. M. (2018). YAP/TAZ activation as a target for treating metastatic cancer. Cancers 10:115. doi: 10.3390/cancers10040115
Xu, T., Wang, W., Zhang, S., Stewart, R. A., and Yu, W. (1995). Identifying tumor suppressors in genetic mosaics: the Drosophila lats gene encodes a putative protein kinase. Development 121, 1053–1063.
Yin, F., Yu, J., Zheng, Y., Chen, Q., Zhang, N., and Pan, D. (2013). Spatial organization of Hippo signaling at the plasma membrane mediated by the tumor suppressor Merlin/NF2. Cell 154, 1342–1355. doi: 10.1016/j.cell.2013.08.025
Zanconato, F., Cordenonsi, M., and Piccolo, S. (2019). YAP and TAZ: a signalling hub of the tumour microenvironment. Nat. Rev. Cancer 19, 454–464. doi: 10.1038/s41568-019-0168-y
Zhao, B., Li, L., Wang, L., Wang, C. Y., Yu, J., and Guan, K. L. (2012). Cell detachment activates the Hippo pathway via cytoskeleton reorganization to induce anoikis. Genes Dev. 26, 54–68. doi: 10.1101/gad.173435.111
Zhao, B., Wei, X., Li, W., Udan, R. S., Yang, Q., Kim, J., et al. (2007). Inactivation of YAP oncoprotein by the Hippo pathway is involved in cell contact inhibition and tissue growth control. Genes Dev. 21, 2747–2761. doi: 10.1101/gad.1602907
Keywords: cell migration, Drosophila, mechanical force, metastasis, cell size control
Citation: Chang Y-C, Wu J-W, Wang C-W and Jang AC-C (2020) Hippo Signaling-Mediated Mechanotransduction in Cell Movement and Cancer Metastasis. Front. Mol. Biosci. 6:157. doi: 10.3389/fmolb.2019.00157
Received: 07 October 2019; Accepted: 17 December 2019;
Published: 31 January 2020.
Edited by:
Madhuri Kango-Singh, University of Dayton, United StatesReviewed by:
Giulia Ricci, University of Campania Luigi Vanvitelli, ItalyKaushlendra Tripathi, University of Alabama at Birmingham, United States
Copyright © 2020 Chang, Wu, Wang and Jang. This is an open-access article distributed under the terms of the Creative Commons Attribution License (CC BY). The use, distribution or reproduction in other forums is permitted, provided the original author(s) and the copyright owner(s) are credited and that the original publication in this journal is cited, in accordance with accepted academic practice. No use, distribution or reproduction is permitted which does not comply with these terms.
*Correspondence: Yu-Chiuan Chang, eWNjJiN4MDAwNDA7bWFpbC5uc3lzdS5lZHUudHc=; Anna C.-C. Jang, Y2NqYW5nJiN4MDAwNDA7bWFpbC5uY2t1LmVkdS50dw==