- 1Counterpoint Biomedica LLC, Santa Monica, CA, United States
- 2Delta Next-Gene, LLC, Santa Monica, CA, United States
The ‘Clovis Point’—an enabling prehistoric gain-of-function in stone-age tool technologies which empowered the Paleoindian-Americans to hunt, to strike-deep, and to kill designated target megafauna more efficiently—was created biochemically by molecular-genetic bio-engineering. This Biomedical “Clovis Point” was crafted by adapting a broad-spectrum Pan-Collagen Binding Domain (Pan-Coll/CBD) found within the immature pre-pro-peptide segment of Von Willebrand Factor into a constructive series of advanced medical applications. Developed experimentally, preclinically, and clinically into a cutting-edge Biotechnology Platform, the Clovis Point is suitable for 1) solid-state binding of growth factors on collagenous scaffolds for improved orthopedic wound healing, 2) promoting regeneration of injured/diseased tissues; and 3) autologous stem cell capture, expansion, and gene-based therapies. Subsequent adaptations of the high-affinity Pan-Coll/CBD (exposed-collagen-seeking/surveillance function) for intravenous administration in humans, enabled the physiological delivery, aka Pathotropic Targeting to diseased tissues via the modified envelopes of gene vectors; enabling 4) precision tumor-targeting for cancer gene therapy and 5) adoptive/localized immunotherapies, demonstrating improved long-term survival value—thus pioneering a proximal and accessible cell cycle control point for cancer management—empowering modern medical oncologists to address persistent problems of chemotherapy resistance, recurrence, and occult progression of metastatic disease. Recent engineering adaptations have advanced the clinical utility to include the targeted delivery of small molecule APIs: including taxanes, mAbs, and RNA-based therapeutics.
1 Introduction
With the advantages of retrospection, we are privileged to reflect on significant clinical progress and to honor our many colleagues and collaborators who participated in this enduring epic of gene discovery and biochemical pathway characterization which connects the conceptual principles of stem cell biology, signal transduction, protein phosphorylation, wound-healing, inflammation, and co-carcinogenesis to the driving oncogene addictions and the key rate-limiting growth factors and tumor suppressors governing mammalian cell cycle control. Herein, in the context of this review, we present the allegory of the ‘Clovis Point’—a prehistoric gain-of-function in stone-age tool making technologies which empowered the Paleoindian-Americans to hunt, to strike-deep, and to kill designated target megafauna more efficiently—as an instructive allegory and an enabling biotechnology platform. Another useful perspective and unifying concept is a selective focus on the ‘Proline-Directed Protein Kinases’ (Liu and Kipreos, 2000; Kannan and Neuwald, 2004) —40 serine/threonine kinases demonstrating a preference for proline at the P +1 position (Vulliet et al., 1989; Hall et al., 1990; Zhu et al., 2005) — that is, a selective, sharp focus on the family of mitogen-activated protein kinases (MAPKs) which mediate receptor-signaling events (Willams et al., 1993; Gordon et al., 1996; Hall et al., 1996); and the cyclin-dependent protein kinases (CDKs), targeted (as heterodimers) by the cognate “cyclin partner” to specific substrate proteins (Hall et al., 1991; Elledge et al., 1992; Peeper et al., 1993), and which mediate a myriad of intricate protein-protein interactions (PPIs) in biochemical pathways that regulate stem cell growth, proliferative competence, the cell division cycle, sustained survival, and differentiation processes under physiological conditions. The characterization of mitogenic signal transduction, from growth factor receptors to the immediate early events of gene expression, provides mechanistic understanding and new molecular tools for both regenerative and interventional medicine.
2 Solid-state binding of bioactive growth (and survival) factors on collagenous scaffolds
To facilitate orthopedic wound healing and to promote the regeneration of injured tissues, a Biomedical “Clovis Point” was crafted by adapting a broad-spectrum Pan-Collagen-type Binding Domain (Pan-Coll/CBD) found within the immature pre-pro-peptide segment of Von Willebrand Factor into a constructive series for advanced medical applications (Figure 1). The conceptual bioengineering, expression, purification, and refolding/renaturation of the first collagen-binding TGF-β (and related) fusion proteins from microorganisms, enabled high-yield, cost-effective bioproduction of recombinant human TGF-β/BMP fusion proteins for preclinical studies: wherein enhanced osteogenesis was observed, as was the migration, growth, and differentiation of bone marrow mesenchymal cells (Tuan et al., 1996; Han et al., 1997; Andrades et al., 1999; Han et al., 2002). Preclinical studies included a collagen-targeted fibroblast growth factor (CBD-bFGF) which was shown to be effective in a diabetic wound healing model (Andrades et al., 2000; Andrades et al., 2001); and epidermal growth factor (CBD-EGF) which was shown to be effective in an animal model of experimental colitis (Hall et al., 2000a). The histological observations of exceedingly primitive stem cells migrating into growth-factor functionalized (yet acellular) collagen fiber scaffolds (Andrades et al., 1996), prompted a series of experiments on human bone marrow, where the clinical utility of CBD-TGF-β is acting as a “survival factor,” was used for the selective capture and characterization of a pre-hematopoietic, premesenchymal stem cell (Hall et al., 2001), presented clinically as an autologous stem cell platform for retrovirus-mediated gene therapy, exemplified by cell-based delivery and engraftment of the missing coagulation factor(s) of hemophilia (Gordon et al., 1997).
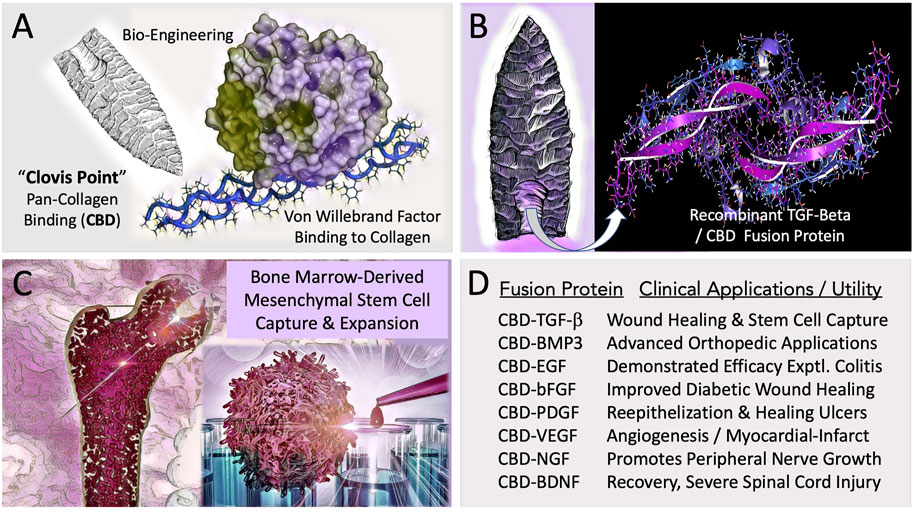
FIGURE 1. Engineering and Potential Clinical Utility of Collagen Binding (CBD) Growth Factors. (A) Concept of “hafting” a Pan-Collagen Binding Domain (CBD)—a molecular “Clovis Point”—by adaptive bio-engineering of vWF. (B) Chimeric growth factor protein expression in microorganisms, followed by purification and renaturation of the recombinant fusion polypeptides, (e.g., CBD-BMP3) provided the abundant bioactive materials needed for preclinical studies and translational research. (C) Graphic figure depicts the capture, expansion, conditioning, and differentiation of bone-marrow derived pre-hematopoietic, pre-mesenchymal stem cells for cell- and gene-based therapies. (D) Examples of collagen-targeted growth factors, highlighting advances in the potential utility of the biotechnology platform in the form of preclinical demonstrations.
We celebrate the advancement of the conceptual platform in its ongoing translation to the clinic, along with the innovative variety of chimeric fusion proteins, collagen binding domains, and related binding motifs, as well as the increasing arrays of functionalized matrices, bandages, sponges, hydrogels, and membranes that have since been developed and proven in principle (Addi et al., 2017). Most notably, in cardiovascular disease: where a myocardial patch made of collagen membranes loaded with collagen-binding human vascular endothelial growth factor (CBD-VEGF) was shown to accelerate healing of the injured rabbit heart (Gao et al., 2011); and new approaches now include the selection and preconditioning of autologous mesenchymal stem cells with defined growth factors to improve the homing ability, engraftment, and survival of both the implanted cells and the ischemic cardiac tissue (Matta et al., 2022). Encouraging progress has been made in the translation to neurology and spinal cord injury: where collagen-based scaffolds and mesenchymal stem cells are employed with success in the experimental treatment of spinal cord injuries in humans and in animal models (Zachariou et al., 2022). Indeed, in traumatic models involving complete spinal cord transection the application of a linear collagen scaffold loaded with a collagen-binding fusion construct of a brain-derived neurotropic factor (CBD-BDNF) i) enhances functional recovery of a severed spinal cord by facilitating peripheral nerve infiltration and ingrowth (Han et al., 2014) and ii) enables neural stem cell capture and growth-factor conditioning for severe spinal cord injury repair (Li et al., 2016).
3 Going mobile: The pathotropic targeting of gene therapy vectors to diseased tissues
At the turn of the century, the promise of tumor-targeted gene therapies was generally considered a highly desirable yet elusive clinical goal—(Researchers Get a Dose of Reality as Logistics Stymie Gene Therapy (Langreth and Moore, WSJ, 27 Oct 1999). Indeed, the large number and diversity of collagen proteins, which includes some 28 family members (Ricard-Blum, 2011), make physiological targeting of a specific type of exposed collagen protein problematic. This problem is made even more complex with the realization that both cardiovascular injury and the stroma of invasive metastatic cancers are associated with high expression of extracellular signaling molecules, including collagen-triple-helix- containing-protein 1 (Mei et al., 2020): which activates an array of extracellular matrix metalloproteases that degrade and thereby alter the collagenous proteins (a proteolytic anaplasia), modifying the extracellular matrix of the vascular lesion, and creating what amounts to a collagenous anaplastic “jailbreak” of sorts for transformed cancer stem cells undergoing epithelial to mesenchymal transition (EMT). Fortunately, a unique and clinically useful feature of the high-affinity Pan-Collagen-type CBD-Growth Factor constructs and chimeric fusion proteins we originally developed for collagenous bandages, stem cell capture, and wound healing applications, was a demonstrable and advantageous pan-collagenous protein binding property (including denatured gelatin), which was adaptively engineered by experimentation into a high-affinity lesion-targeting property or gain-of function transposed into the protein envelopes of various experimental gene therapy vectors (Hall et al., 2000b; Gordon et al., 2002)— demonstrating the remarkable lesion-targeting gain-of-function in preclinical studies of vascular restenosis following balloon injury (Xu et al., 2001), and in animal models of metastatic cancer (Gordon et al., 2000).
4 A proper genetic payload: Proto-oncogene discovery and biochemical pathway characterization
A simplified schematic view of biological signal transduction: from growth factor receptors, through receptor mediated tyrosine phosphorylation(s) and characteristic phospholipid effects, is shown as Figure 2, identifying the levels of organization and executive protein kinases involved. Our collaborative contributions to the field of biocybernetics, specifically proline-directed protein phosphorylation, include the identification and characterization of the Hera kinase, as a consort of the human EGF-receptor (Williams et al., 1993). This human-EGF-Receptor-associated protein kinase, designated p38α MAPK14, plays a major role regulating EGF-receptor function(s), stress responses, tumor dormancy, chemo-resistance, and cancer stem cell (CSC) survival (Martínez-Limón et al., 2020; Kudaravalli et al., 2022). Biochemical characterization and cloning of the human cyclin-dependent kinase activating kinase CAK1 (Yee et al., 1995; Yee et al., 1996), and its obligatory assembly factor, ménage à trois, MAT1 (Wang et al., 2000), provided additional mechanistic links to DNA replication and transcription, as well as the executive enzymology functioning upstream of the Cyclin/Cdk complexes governing cell cycle control.
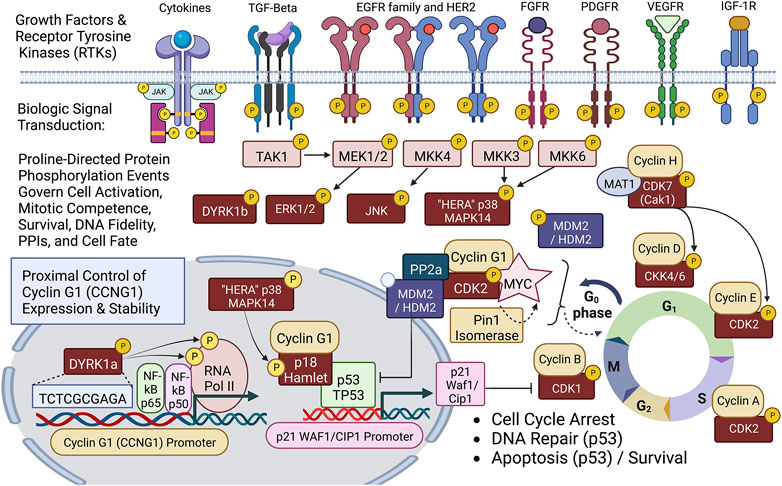
FIGURE 2. Diagram of site-specific proline-directed protein phosphorylation events governing stem cell activation: Identifying the proximal bio-cybernetics, driving oncogenes, and executive enzymology at the foundations of stem cell activation, cellular transformation, stem cell survival, sustained proliferation, and cell cycle control. The diagram highlights the executive regulatory flow of site-specific protein phosphorylation events linking the activation of major growth factor receptors to a series of mitogenic proline-directed signal transductions cascading through the “Hera kinase” (p38, human EGF-receptor- associated Mapk14) and DYRK1a, directing immediate early events leading to the activation of the Cyclin G1 (CCNG1) promoter and expression of the Pivotal Cyclin G1/Cdk2/Myc/Pin1/Mdm2/HDM2/p53 Axis of cell cycle control. Note: Cyclin G1 interacts physically with p18 Hamlet, a transcriptional activator and target substrate of p38 Hera/Mapk14, which mediates p53-dependent transcriptional responses to DNA damage and genotoxic stress in proliferative cells. Moreover, p38 Mapk14 and p18 Hamlet are implicated in chromatin-remodeling and transcriptional activation complexes associated with muscle differentiation. See text for additional details.
The molecular cloning of the first human cyclin G-type gene (Wu et al., 1994), followed by studies of its overexpression and survival function in osteosarcoma cells—which lack a functional/wild type p53 (TP53) tumor suppressor (Wu et al., 1995)—provided a conceptual missing link in the proximal/initiating/transcriptional pathways driving the immediate early molecular genetics of neoplastic transformation and oncogenesis. While Cyclin G1 is a transcriptional target of p53 (TP53), the major tumor suppressor, the CCNG1 gene is overexpressed in many cancer cells in the absence of a functional p53, which led to the hypothesis and eventually the experimental confirmation that Cyclin G1 (CCNG1) represents a valid, proximal, and transforming proto-oncogene in its own right. Thus, the Cyclin G1 oncoprotein represents a singular strategic target and a proximal point of therapeutic intervention in the clinical management of human cancers. While antisense constructs of Cyclin G1, antisense Cyclin D1, and enforced expression of p21, were each found to be effective as transgenes (payloads) in blocking cancer cell survival in vitro, Cyclin G1 was selected for clinical development in a series of truncation experiments, using both point mutations and deletion mutants, to discern a potent dominant-negative “blocking” construct of the Cyclin G1 pathway, which was determined to be essential for the survival and proliferation of cancer cells derived from each of the three germ layers. Thus, a retroviral expression vector bearing an inhibitory construct of the Cyclin G1 (CCNG1) gene was developed (DeltaRex-G) and deployed for clinical gene therapy trials, where broad-spectrum bioactivity was observed (Gordon and Hall, 2010).
5 The pathotropic targeting platform enables gene therapy/immunotherapy
In terms of pathotropic tumor targeting, the pan-collagen binding Clovis point represents a powerful and enabling biotechnology for therapeutic gene delivery by its active and selective partitioning to injured and/or diseased tissues, including advanced metastatic cancers via exposed collagen. In terms of validating the strategic therapeutic ‘payload,’ it was, indeed, the demonstrated anti-cancer activities of DeltaRex-G (i.e., dnG1 enforced expression) observed in vivo, in advanced cases of chemo-resistant metastatic cancers, wherein the first blessings of remission were observed, following repeated DeltaRex-G infusions, and the first demonstrations of improved long-term survival were achieved. Moreover, the versatility of the gene delivery platform (simple intravenous infusions) enabled the first clinical demonstrations of localized (tumor-targeted), i.e., personalized cancer vaccinations in situ—using granulocyte macrophage colony stimulating factor (GMCSF) as a first immune-stimulating payload (pulsed, following DeltaRex-G tumor debulking)—where again, significant improvements in the survival of end-stage patients were demonstrated (Gordon et al., 2008; Ignacio et al., 2010). Detailed histological examinations of immune cell trafficking within the tumor microenvironment of DeltaRex-G-treated tumors revealed the selective killing (apoptosis) of cancer cells, tumor-associated stromal elements, and the proliferative cells of tumor neo-vasculature, while preserving of a patient’s innate tumor surveillance function (Stendhal-Dy et al., 2018), which appears favorable for synergies and combinatorial approaches with available immunotherapy agents in the clinical management of metastatic disease.
6 A Proximal Oncology: Pioneering a proximal and accessible locus of cell cycle control
Discerning the upstream signaling pathways that link cell growth and DNA replication to cell cycle progression can be a daunting endeavor in a landscape where the vast numbers of cell cycle checkpoint regulators and their myriad of protein-protein interactions (PPIs) reach a zillion (Yasutis and Kozminski, 2013). It is in this regard, that DeltaRex-G stands alone in defining a single proximal (gene locus) and a strategic point of cell cycle control (dnG1 blockade) suitable for broad-spectrum cancer gene therapy. Thus, the Pivotal Cyclin G1 axis was conferred as a uniquely useful and accessible point of clinical cell cycle control, with a review of molecular mechanisms for oncologists (Al-Shihabi et al., 2018; Gordon et al., 2018)—demonstrating long-term survival benefits of a Cyclin G1 pathway blocker in a broad spectrum of advanced cancers, when provided as monotherapy or combined with adjunctive immunotherapy; empowering modern medical oncologists to address persistent problems of chemotherapy resistance, recurrence, and occult progression of metastatic disease. An updated diagram of the key oncogenic drivers of the Pivotal Cyclin G1 Axis is shown in Figure 3, presenting the Cyclin G1/Cdk/Myc/Pin1/Mdm2 as a fundamental set of interacting proto-oncogenes—which acting together create a stabilizing/reinforcing molecular-genetic flywheel, exhibiting momentum, in terms of oncoprotein stability—thus, maintaining stem cell competence and stem cell survival programs: from the beginnings of stem cell activation from quiescence (Go/G1), through the phases of the cell division cycle, and further, with mechanistic links extending from the checkpoint guardians of DNA fidelity to cellular differentiation and fate. In normal development, p53 tumor suppressor and Cyclin G1cooperate in mediating genome stability in somatic cells, both being required for DNA repair, with Cyclin G1 playing a positive role in the coordination of cell growth and cell proliferation (Faradji et al., 2011; Bayer et al., 2017). Follow-up studies of archived tumor samples in a long-term survivor (>12 years) of metastatic pancreatic cancer following repeated infusions of DeltaRex-G, revealed a p53 (TP53) mutation (G199V) associated with an increased anti-apoptotic survival function, affirming the therapeutic efficacy of DeltaRex-G in the background of p53 suppressor mutations (Morse et al., 2021). Indeed, recent studies have confirmed that the Cyclin G1 (CCNG1 proto-oncogene) is upregulated by mutations in tumor protein p53 (TP53), which is associated with both tumorigenesis and tumor progression (Xu et al., 2019).
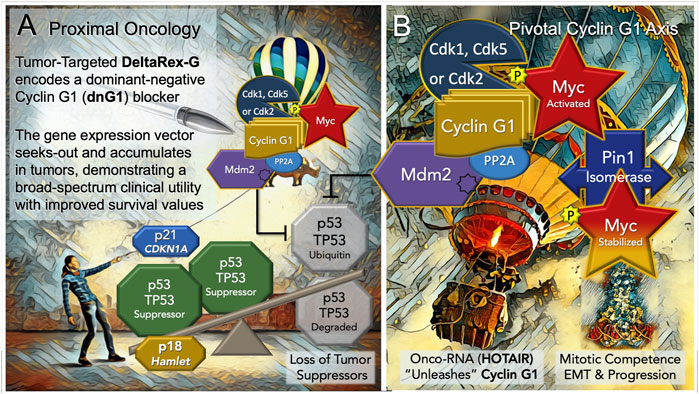
FIGURE 3. Blocking the “Cyclin G1 Axis” of Interacting Oncogenes and Tumor Suppressors. (A) DeltaRex-G, encoding a cytocidal ‘dominant negative’, i.e. a truncated construct (dnG1) of the executive cyclin G1 oncogene (CCNG1), is shown as a molecular-genetic “silver bullet,” blocking the Pivotal Cyclin G1 Axis of associated oncogenes, which is activated by signal transduction and/or loss of natural tumor suppressors (p53 (TP53); p21, CDKN1A; and p18, Hamlet). (B) The Cyclin G1 Axis of interacting oncoproteins include Cyclin G1, Myc, Cdk2, Cdk5 or Cdk1, Mdm2, and the proline-directed/phosphorylation-dependent Pin1 isomerase, which stabilizes the Cyclin G1/Cdk-activated Myc oncoprotein by phosphorylation (at serine 62) and maintains its transcriptional bioactivity. Note: loss of the natural tumor suppressors—by 1) mutations in p53, 2) loss of regulatory miRNAs, 3) by viral subversion (e.g., miR-122), or 4) by a growing number of oncogenic-RNAs (e.g., HOTAIR)— “unleashes” the expression and functions of the Cyclin G1 Axis which lead to stem cell activation, tumorigenesis, error-prone DNA replication, increasing oncogene addictions, EMT/metastasis, and progression of cancers.
The Cyclin G1 proto-oncogene is known to be activated and up-regulated by mutation and viral subversion, as well as a growing number of long-noncoding oncogenic RNAs, and mitogenic signal transduction. Notably, the hepatitis B virus HBx protein deregulates cell cycle checkpoint controls and enhances hepatoblastoma cell proliferation, in part, by suppressing miR-122, a predominant negative regulator of Cyclin G1 (CCNG1) expression, which is lost in the process of neoplastic transformation and oncogenesis (Bandopadhyay et al., 2016). Recent, studies of experimental Sendai virus infection in murine embryo fibroblasts revealed new molecular mechanisms by which NF-kB signaling components (p50 and p65 subunits) are recruited to the cell nucleus (see Figure 2) to directly activate and up-regulate both Cyclin G1 (CCNG1) and p21 (CDKN1) at the level of gene expression, enhancing the transcription of both genes upon virus infection (Burns and Kerppola, 2022). A sharp focus at the level of the human Cyclin G1/CCNG1 promoter reveals a DNA regulatory cis-element (tandem TCTCGCGAGA motif) that is, limited to approximately 5% of human genes, particularly those involved in enhanced protein synthesis and transition phases of the cell division cycle (Wyrwicz et al., 2007). Remarkably, the Dyrk1a protein kinase was revealed as a gene-specific RNA Polymerase II kinase (Di Vona et al., 2015), which selectively recognizes the aforementioned palindromic DNA motif, which is also clearly present at the level of the human CCNG1 promoter.
The CCNG1 gene itself is subject to dysregulation by the action of oncogenic long-noncoding RNAs (Cheng et al., 2018; Li et al., 2019; Abula et al., 2022); and by direct/activating mutations. A close examination of the pathogenesis of Burkitt’s lymphoma in a screening for IG-translocations to non-MYC partners that could cooperate with MYC in pathogenesis, identified a telltale IGK-GGNG1 translocation: a chromosomal breakpoint at the CCNG1 promoter that had apparently occurred earlier (at pre-B or immature B Cell stage) in the evolution of the tumor, which suggested that IG-MYC translocations may not always be the initial genetic event in Burkitt’s lymphoma (López et al., 2019). Focused studies of IGH rearrangements in myeloid neoplasms identified an IGH-CCNG1 breakpoint translocation at the cyclin G1 (CCNG1) promoter, which demonstrated overexpression of CCNG1 in the patient’s bone marrow (Xiao et al., 2020). The patient was diagnosed with chemotherapy-related acute myeloid leukemia and succumbed to the disease 2 months later. The authors of the study identified Delta-Rex-G as a potential intervention, noting its impressive results regarding long-term cancer-free survival in clinical trials, and suggesting clinical evaluation of the anti-CCNG1 strategy as a treatment in such cases with very poor prognosis.
One can now extend the epic of gene discovery from Hera to Hamlet: that is, from the p38 (MAPK14), kinase that is, at times, physically associated with the EGF-receptor to p18 Hamlet, a highly conserved protein ‘sensor,’ partner of both p53 and Cyclin G1, and critical governor of cell fate. The “Hamlet” gene, originally cloned and characterized by Xu et al. as a novel Cyclin-G1- binding protein: exhibits both a nuclear targeting motif and a zinc-finger motif commonly found in transcription factors (Xu et al., 2000). It turns out that p18 FX3/Hamlet (originally FX3, aka ZNHIT1, aka Hamlet) is directly phosphorylated and regulated by the p38 stress-related proline-directed Hera kinase/MAPK14 (Cuadrado et al., 2007). Indeed, p18 Hamlet interacts with p53, as well as Cyclin G1, and activates p53-dependent gene promoters. Normally tightly regulated by Cyclin G1, which induces its degradation, p18 Hamlet accumulates in response to genotoxic stresses such as UV or cisplatin treatment, and thereby acts as a sensor which mediates p53-dependent responses to different genotoxic stresses (Lafarga et al., 2007)—balancing the molecular mechanisms between cell cycle arrest (and DNA 216 repair) by the p53-dependent up-regulation of p21Cip1 (CDKN1), or the equally dramatic alternative: death by apoptosis, via p53-dependent induction of proapoptotic promoters. In terms of the Proximal Oncology presented herein (Figure 3), p18 Hamlet physically links p38 Hera/MAPK14 to the complex regulation and functions of the p53 tumor suppressor (Vousden and Prives, 2009), thereby defining a prospective tumor suppressor that is, essentially lost, as is p53, with oncogenic activation and overexpression of the Pivotal Cyclin G1 (CCNG1) Axis.
Of considerable relevance and conceptual support for this highly simplified model of the Cyclin G1 Axis are comparative screens identifying genetic variants in cell cycle and checkpoint control pathways that confer particular susceptibility to aggressive cancers: Notably, CCNG1, CDK2, CDK5, and MDM2 were identified among the Top-7 genes with variants associated with aggressive prostate cancer (Kibel et al., 2016). Likewise, comparatively high expression of the mitogen-activated protein kinase kinase 3, MKK3 (see Figure 2), which activates Myc transcription, is associated with an increased incidence of triple-negative breast cancer and a worse clinical outcome (Yang et al., 2020). Armed with the enabling potentiality of tumor-targeted gene delivery, with a broad spectrum bioactive, in the embodiment of DeltaRex-G, the challenge remains to match the precision medicine with the patient’s tumor burden in a timely manner (Ravicz et al., 2021), with thoughts of cancer control and improved long-term outcome in mind. In closing, we reflect upon the development of new Clovis Point platforms for the delivery of future medicines, including the tumor-targeted delivery small molecules (e.g., paclitaxel), monoclonal antibodies, and future RNA-based therapeutics. Taken together, the concepts of collagen binding growth factors, autologous stem cell capture, and pathotropic targeting have provided new insights and molecular-genetic tools for wound healing, tissue regeneration, and cancer control.
Author contributions
FH and EG both contributed to the literature review and manuscript drafting. The final manuscript was approved by both authors.
Acknowledgments
The authors are grateful to the molecular biologists, scientists, physicians and patients who participated in drug discovery, translational research, and DeltaRex-G clinical trials. Note the stick and ribbon diagram of a TGF/BMP renatured growth factor, and the attendant artwork presented in Figure 1 were provided by Shutterstock; Figure 2 was composed with BioRender.com software.
Conflict of interest
FH and EG are founders and executive officers of Counterpoint Biomedica LLC and Delta Next-Gene, LLC and are co-inventors of the targeted gene delivery system represented by the pan-collagenous CLOVIS POINT and DeltaRex-G.
Publisher’s note
All claims expressed in this article are solely those of the authors and do not necessarily represent those of their affiliated organizations, or those of the publisher, the editors and the reviewers. Any product that may be evaluated in this article, or claim that may be made by its manufacturer, is not guaranteed or endorsed by the publisher.
References
Abula, A., Saimaiti, G., Maimaiti, X., Wuqikun, W., Abulaiti, A., Ren, P., et al. (2022). The stimulative function of long noncoding RNA CDKN2B-AS1 in osteosarcoma by targeting the microRNA-122/CCNG1 axis. J. Recept. Sig. Transduct. Res. 42, 71–79. doi:10.1080/10799893.2020.1850784
Addi, C., Murschel, F., and Gregory De Crescenzo, G. (2017). Design and use of chimeric proteins containing a collagen-binding domain for wound healing and bone regeneration, (Review Article). Tissue Eng. 23, 163–182. doi:10.1089/ten.TEB.2016.0280
Al-Shihabi, A., Chawla, S. P., Hall, F. L., and Gordon, E. M. (2018). Exploiting oncogenic drivers along the CCNG1 pathway for cancer therapy and gene therapy. Mol. Ther. Oncolytics 11, 122–126. doi:10.1016/j.omto.2018.11.002
Andrades, J. A., Wu, L. Y., Hall, F. L., Nimni, M. E., and Becerra, J. (2000). Engineering, expression, and renaturation of a collagen-targeted human bFGF fusion protein. Growth factors. 18, 261–275. doi:10.3109/08977190109029115
Andrades, J. A., Han, B., Becerra, J., Sorgente, N., Hall, F. L., and Nimni, M. E. (1999). A recombinant human TGF-beta1 fusion protein with collagen-binding domain promotes migration, growth, and differentiation of bone marrow mesenchymal cells. Exp. Cell. Res. 250, 485–498. doi:10.1006/excr.1999.4528
Andrades, J. A., Nimni, M. E., Han, B., Ertl, D. C., Hall, F. L., and Becerra, J. (1996). Type I collagen combined with a recombinant TGF-beta serves as a scaffold for mesenchymal stem cells. Int.l J. Dev. Biol. 40, S107–S108.
Andrades, J. A., Santamaria, J. A., Wu, L. T., Hall, F. L., Nimni, M. E., and Becerra, J. (2001). Production of a recombinant human basic fibroblast growth factor with a collagen binding domain. Protoplasma 218, 95–103. doi:10.1007/BF01288365
Bandopadhyay, M., Sarkar, N., Datta, S., Das, D., Pal, A., Panigrahi, R., et al. (2016). Hepatitis B virus X protein mediated suppression of miRNA-122 expression enhances hepatoblastoma cell proliferation through cyclin G1-p53 axis. Infect. Agents Cancer 11, 40. doi:10.1186/s13027-016-0085-6
Bayer, F. E., Zimmermann, M., Fischer, P., Gromoll, C., Preiss, A., and Nagel, A. C. (2017). p53 and cyclin G cooperate in mediating genome stability in somatic cells of Drosophila. Sci. Rep 7, 17890. doi:10.1038/s41598-017-17973-z
Burns, V. E., and Kerppola, T. K . (2022). Keap1 moderates the transcription of virus induced genes through G9a-GLP and NFκB p50 recruitment. Immunology 167, 105–121. doi:10.1111/imm.13527
Cheng, D., Deng, J., Zhang, B., He, X., Meng, Z., Li, G., et al. (2018). LncRNA HOTAIR epigenetically suppresses miR-122 expression in hepatocellular carcinoma via DNA methylation. EBioMedicine 36, 159–170. doi:10.1016/j.ebiom.2018.08.055
Cuadrado, A., Lafarga, V., Cheung, P., Dolado, I., Llanos, S., Cohen, P., et al. (2007). A new p38 MAP kinase-regulated transcriptional coactivator that stimulates p53-dependent apoptosis. EMBO J. 26, 2115–2126. doi:10.1038/sj.emboj.7601657
Di Vona, C., Bezdan, D., Islam, A., Salichs, E., Lopez-Bigas, N., Ossowski, S., et al. (2015). Chromatin-wide profiling of DYRK1A reveals a role as a gene-specific RNA polymerase II CTD kinase. Mol. Cell. 57, 506–520. doi:10.1016/j.molcel.2014.12.026
Elledge, S. J., Richman, R., Hall, F. L., Williams, R. T., Lodgson, N., and Harper, J. W. (1992). CDK2 encodes a 33-kDa cyclin A-associated protein kinase and is expressed before CDC2 in the cell cycle. Proc. Nati. Acad. Sci. U. S. A. 89, 2907–2911. doi:10.1073/pnas.89.7.2907
Faradji, F., Bloyer, S., Dardalhon-Cuménal, D., Randsholt, N. B., and Peronnet, F. (2011). Drosophila melanogaster Cyclin G coordinates cell growth and cell proliferation. Cell. Cycle 10, 805–818. doi:10.4161/cc.10.5.14959
Gao, J., Liu, J., Gao, Y., Wang, C., Zhao, Y., Chen, B., et al. (2011). A myocardial patch made of collagen membranes loaded with collagen-binding human vascular endothelial growth factor accelerates healing of the injured rabbit heart. Tissue Eng. Part A. 17, 2739–2747. Epub 2011 Aug 2. PMID: 21682575. doi:10.1089/ten.TEA.2011.0105
Gordon, E. M., Hall, F. L., Beart, R. W., and Anderson, W. F. (2002). “Chapter 14: Genetic engineering of targeted retroviral vectors,” in Vector targeting for therapeutic gene delivery (New York, United States: Wiley). First published: 09 August 2002. doi:10.1002/0471234303.ch14
Gordon, E. M., and Hall, F. L. (2010). Rexin-G, a targeted genetic medicine for cancer. Expert Opin. Biol. Ther. 10, 819–832. doi:10.1517/14712598.2010.481666
Gordon, E. M., Levy, J. P., Reed, R. A., Petchpud, W. N., Liu, L., Wendler, C. B., et al. (2008). Targeting metastatic cancer from the inside: A new generation of targeted gene delivery vectors enables personalized cancer vaccination in situ. Intl. J. Oncol. 33, 665–675. doi:10.3892/ijo_00000052
Gordon, E. M., Liu, P. X., Chen, Z. H., Liu, L., Whitley, M. D., Gee, C., et al. (2000). Inhibition of metastatic tumor growth in nude mice by portal vein infusions of matrix-targeted retroviral vectors bearing a cytocidal cyclin G1 construct. Cancer Res. 60, 3343–3347.
Gordon, E. M., Natarajan, V., Salazar, R., Tang, H., Schmidler-Sapiro, K., Buckley, S., et al. (1996). Factor XII-induced mitogenesis is mediated via a distinct signal transduction pathway that activates a mitogen-activated protein kinase. Proc. Natl. Acad. Sci. U. S. A. 93, 2174–2179. doi:10.1073/pnas.93.5.2174
Gordon, E. M., Ravicz, J. R., Liu, S., Chawla, S. P., and Hall, F. L. (2018). Cell cycle checkpoint control: The cyclin G1/Mdm2/p53 axis emerges as a strategic target for broad-spectrum cancer gene therapy - a review of molecular mechanisms for oncologists. Mol. Clin. Oncol. 9, 115–134. doi:10.3892/mco.2018.1657
Gordon, E. M., Skotzko, M., Kundu, R. K., Han, B., Andrades, J., Nimni, M., et al. (1997). Capture and expansion of bone marrow-derived mesenchymal progenitor cells with a transforming growth factor-beta1-von Willebrand's factor fusion protein for retrovirus-mediated delivery of coagulation factor IX. Hum. Gene Ther. 20, 1385–1394. doi:10.1089/hum.1997.8.11-1385
Hall, F. L., Benya, P. D., Padilla, S. R., Denise Carbonaro-Hall, D., Williams, R., Buckley, S., et al. (1996). Transforming growth factor-beta type-II receptor signalling: Intrinsic/associated casein kinase activity, receptor interactions and functional effects of blocking antibodies. Biochem. J. 316, 303–310. doi:10.1042/bj3160303
Hall, F. L., Braun, R. K., Mihara, K., Fung, Y. T., Berndt, N., Carbonaro-Hall, D. A., et al. (1991). Characterization of the cytoplasmic proline-directed protein kinase in proliferative cells and tissues as a heterodimer comprised of p34 cdc2 and p58 cyclin A. J. Biol. Chem. 266, 17430–17440. doi:10.1016/s0021-9258(19)47391-2
Hall, F. L., Han, B., Kundu, R. K., Yee, A., Nimni, M. E., and Gordon, E. M. (2001). Phenotypic differentiation of TGF-beta1-responsive pluripotent pre-mesenchymal pre-hematopoietic progenitor (P4 stem) cells from murine bone marrow. J. Hematother. Stem Cell. Res. 10, 261–271. doi:10.1089/15258160151134962
Hall, F. L., Kaiser, A., Liu, L., Chen, Z. H., Hu, J., Nimni, M. E., et al. (2000a). Design, expression, and renaturation of a lesion-targeted recombinant epidermal growth factor-von Willebrand factor fusion protein: Efficacy in an animal model of experimental colitis. Intl. J. Mol. Med. 6, 635–643. doi:10.3892/ijmm.6.6.635
Hall, F. L., Liu, L., Zhu, N. L., Stapfer, M., Anderson, W. F., Beart, R. W., et al. (2000b). Molecular engineering of matrix-targeted retroviral vectors incorporating a surveillance function inherent in von Willebrand factor. Hum. Gene Ther. 11, 983–993. doi:10.1089/10430340050015293
Hall, F. L., Mitchell, J. P., and Vulliet, P. R. (1990). Phosphorylation of synapsin I at a novel site by proline-directed protein kinase. J. Biol. Chem. 265, 6944–6948. doi:10.1016/s0021-9258(19)39241-5
Han, B., Hall, F. L., and Nimni, M. E. (1997). Refolding of a recombinant collagen-targeted TGF-beta2 fusion protein expressed in Escherichia coli. Protein Express. Purif. 11, 169–178. doi:10.1006/prep.1997.0784
Han, B., Perelman, N., Tang, B., Hall, F., Shors, E. C., and Nimni, M. E. (2002). Collagen-targeted BMP3 fusion proteins arrayed on collagen matrices or porous ceramics impregnated with Type I collagen enhance osteogenesis in a rat cranial defect model. J. Ortho. Res. 20, 747–755. doi:10.1016/S0736-0266(01)00157-7
Han, S., Wang, B., Jin, W., Xiao, Z., Chen, B., Xiao, H., et al. (2014). The collagen scaffold with collagen binding BDNF enhances functional recovery by facilitating peripheral nerve infiltrating and ingrowth in canine complete spinal cord transection. Spinal Cord. 52, 867–873. doi:10.1038/sc.2014.173
Ignacio, J. G., Chawla, S. P., Manalo, R. E., Baniqued, L., San Juan, F. S., Madamba, A., et al. (2010). A phase I/II study of intravenous Rexin-G and Reximmune-C for cancer immunotherapy: The GeneVieve protocol. J. Clin. Oncol. 28, 2546. doi:10.1200/jco.2010.28.15_suppl.2546
Kannan, N., and Neuwald, A. F. (2004). Evolutionary constraints associated with functional specificity of the CMGC protein kinases MAPK, CDK, GSK, SRPK, DYRK, and CK2alpha. Protein Sci. 13, 2059–2077. doi:10.1110/ps.04637904
Kibel, A. S., Ahn, J., Isikbay, M., Klim, A., Wu, W. S., Hayes, R. B., et al. (2016). Genetic variants in cell cycle control pathway confer susceptibility to aggressive prostate carcinoma. Prostate 76, 479–490. doi:10.1002/pros.23139
Kudaravalli, S., den Hollander, P., and Mani, S. A. (2022). Role of p38 MAP kinase in cancer stem cells and metastasis. Oncogene 41, 3177–3185. doi:10.1038/s41388-022-02329-3
Lafarga, V., Cuadrado, A., and Nebreda, A. R. (2007). p18(Hamlet) mediates different p53-dependent responses to DNA-damage inducing agents. Cell. Cycle 6, 2319–2322. doi:10.4161/cc.6.19.4741
Li, C., Hu, G., Wei, B., Wang, L., and Liu, N. (2019). lncRNA LINC01494 promotes proliferation, migration and invasion in glioma through miR-122-5p/CCNG1 Axis. OncoTargets Ther 12, 7655–7662. doi:10.2147/OTT.S213345
Li, X., Liu, S., Zhao, Y., Li, J., Ding, W., Han, S., et al. (2016). Training neural stem cells on functional collagen scaffolds for severe spinal cord injury repair. Adv. Funct. Mater. 26, 5835–5847. doi:10.1002/adfm.201601521
Liu, J., and Kipreos, E. T. (2000). Evolution of cyclin-dependent kinases (CDKs) and CDK- activating kinases (CAKs): Differential conservation of CAKs in yeast and metazoa. Mol. Biol. Evol. 17, 1061–1074. doi:10.1093/oxfordjournals.molbev.a026387
López, C., Kleinheinz, K., Aukema, S. M., Rohde, M., Bernhart, S. H., Hübschmann, D., et al. (2019). Genomic and transcriptomic changes complement each other in the pathogenesis of sporadic Burkitt lymphoma. Nat. Commun. 10, 1459. doi:10.1038/s41467-019-08578-3
Martínez-Limón, A., Joaquin, M., Caballero, M., Posas, F., and de Nada, E. (2020). The p38 pathway: From biology to cancer therapy. Intl. J. Mol. Sci. 21, 1913. doi:10.3390/ijms21061913
Matta, A., Nader, V., Lebrin, N., Gross, F., Prats, A. C., Cussac, D., et al. (2022). Pre-conditioning methods and novel approaches with mesenchymal stem cells therapy in cardiovascular disease. Cells 11, 1620. doi:10.3390/cells11101620
Mei, D., Zhu, Y., Zhang, L., and Wei, W. (2020). The role of CTHRC1 in regulation of multiple signaling and tumor progression and metastasis. Mediat. Inflamm. 12, 9578701. doi:10.1155/2020/9578701
Morse, M. A., Chawla, S. P., Wong, T. Z., Bruckner, H. W., Hall, F. L., and Gordon, E. M. (2021). Tumor protein p53 mutation in archived tumor samples from a 12-year survivor of stage 4 pancreatic ductal adenocarcinoma may predict long-term survival with DeltaRex-G: A case report and literature review. Mol. Clin. Oncol. 15, 186. doi:10.3892/mco.2021.2348
Peeper, D. S., Parker, L. L., Ewen, M. E., Toebes, M., Hall, F. L., Xu, Z., et al. (1993). A- and B- type cyclins differentially modulate substrate specificity of cyclin-cdk complexes. EMBO J. 12, 1947–1954. doi:10.1002/j.1460-2075.1993.tb05844.x
Ravicz, J., Szeto, C., Reddy, S., Chawla, S. P., Morse, M. A., Gordon, E. M., et al. (2021). CCNG1 oncogene: A novel biomarker for cancer therapy/gene therapy. J. Cancer Res.Cell. Ther. 5, 01–09. doi:10.31579/2640-1053/090
Ricard-Blum, S. (2011). The collagen family. Cold Spring Harb. Perspect. Biol. 3, a004978. doi:10.1101/cshperspect.a004978
Stendhal-Dy, P., Chawla, S. P., Hall, F. L., and Gordon, E. M. (2018). Immune cell trafficking in the tumor microenvironment of human cyclin G1 (CCNG1) inhibitor-treated tumors. (Review. ). Brit. J. Cancer Res. 1, 202–207. doi:10.31488/bjcr.117
Tuan, T. L., Cheung, D. T., Wu, L. T., Yee, A., Gabriel, S., Han, B., et al. (1996). Engineering, expression and renaturation of targeted TGF-Beta fusion proteins. Conn. Tiss. Res. 34, 1–9. doi:10.3109/03008209609028888
Vousden, K. H., and Prives, C. (2009). Blinded by the light: The growing complexity of p53. Cell. 137, 413–431. doi:10.1016/j.cell.2009.04.037
Vulliet, P. R., Hall, .F. L., Mitchell, J. P., and Hardie, D. G. (1989). Identification of a novel proline-directed serine/threonine protein kinase in rat pheochromocytoma. J. Biol. Chem. 264, 16292–16298. doi:10.1016/s0021-9258(18)71620-7
Wang, Y., Xu, F., and Hall, F. L. (2000). The MAT1 cyclin-dependent kinase-activating kinase (CAK) assembly/targeting factor interacts physically with the MCM7 DNA licensing factor. FEBS Lett. 484, 17–21. doi:10.1016/s0014-5793(00)02117-7
Williams, R., Sanghera, J., Wu, F., Carbonaro-Hall, D., Campbell, D. L., Warburton, D., et al. (1993). Identification of a human epidermal growth factor receptor-associated protein kinase as a new member of the mitogen-activated protein kinase/extracellular signal-regulated protein kinase family. J. Biol. Chem. 268, 18213–18217. doi:10.1016/s0021-9258(17)46832-3
Wu, L., Yee, A., Carbonaro-Hall, D., Tolo, V., and Hall, F. (1994). Molecular-cloning of the human CYCG1 gene encoding a G-type cyclin - overexpression in human osteosarcoma cells. Oncol. Rep. 1, 705–711. doi:10.3892/or.1.4.705
Wu, L., Yee, A., Liu, L., Carbonaro-Hall, D., Venkatesan, N., Tolo, V., et al. (1995). Early G1 induction of p21/waf1/cip1 in synchronized osteosarcoma cells is independent of p53. Oncol. Rep. 2, 227–231. doi:10.3892/or.2.2.227
Wyrwicz, L. S., Gaj, P., Hoffmann, M., Rychlewski, L., and Ostrowski, J. (2007). A common cis-element in promoters of protein synthesis and cell cycle genes. Acta Biochim. Pol. 54, 89–98. doi:10.18388/abp.2007_3273
Xiao, A. W., Jia, Y., Baughn, L. B., Pearce, K. E., Pitel, B. A., Aster, J. C., et al. (2020). IGH rearrangement in myeloid neoplasms. Haematologica 105, e315–e317. doi:10.3324/haematol.2020.246744
Xu, F., Prescott, M., Liu, P. X., Chen, X. H., Liau, G., Gordon, E. M., et al. (2001). Long term inhibition of neointima formation in balloon-injured rat arteries by intraluminal instillation of a matrix-targeted retroviral vector bearing a cytocidal mutant cyclin G1 construct. Intl. J. Mol. Med. 8, 19–30. doi:10.3892/ijmm.8.1.19
Xu, F., Wang, Y., and Hall, F. L. (2000). Molecular cloning and characterization of FX3, a novel zinc-finger protein. Oncol. Rep. 7, 995–1001. doi:10.3892/or.7.5.995
Xu, Y., Zhang, Q., Miao, C., Dongol, S., Li, Y., Jin, C., et al. (2019). CCNG1 (Cyclin G1) regulation by mutant-P53 via induction of Notch3 expression promotes high-grade serous ovarian cancer (HGSOC) tumorigenesis and progression. Cancer Med. 8, 351–362. doi:10.1002/cam4.1812
Yang, X., Amgad, M., Cooper, L., Du, Y., Fu, H., and Ivanov, A. A. (2020). High expression of MKK3 is associated with worse clinical outcomes in African American breast cancer patients. J. Transl. Med. 18, 334. doi:10.1186/s12967-020-02502-w
Yasutis, K. M., and Kozminski, K. G. (2013). Cell cycle checkpoint regulators reach a zillion. Cell. Cycle 12, 1501–1509. doi:10.4161/cc.24637
Yee, A., Nichols, M. A., Hall, F. L., Kobayashi, R., and Xiong, Y. (1995). Molecular cloning of CDK7-associated human MAT1, a cyclin-dependent kinase-activating kinase (CAK) assembly factor. Cancer Res. 15, 6058–6062.
Yee, A., Wu, L., Kobayashi, R., Xiong, Y., and Hall, F. L. (1996). Biochemical characterization of the human cyclin-dependent protein kinase activating kinase: Identification of p35 as a novel regulatory subunit. J. Biol. Chem. 271, 471–477. doi:10.1074/jbc.271.1.471
Zachariou, D., Evangelopoulos, D. S., Rozis, M., Papagrigorakis, E., Galanis, A., Vavourakis, M., et al. (2022). Application of collagen-based scaffolds for the treatment of spinal cord injuries in animal models: A literature update. Cureus 14, e25997. doi:10.7759/cureus.25997
Zhu, G., Fuji, K., Belkina, N., Liu, Y., James, M., Herrero, J., et al. (2005). Exceptional disfavor for proline at the P + 1 position among AGC and CAMK kinases establishes reciprocal specificity between them and the proline-directed kinases. J. Biol. Chem. 280, 10743–10748. doi:10.1074/jbc.M413159200
Keywords: pan-collagen-binding domain, wound healing, stem cell capture, cancer gene therapy, immunotherapy, deltarex-G, CCNG1
Citation: Gordon EM and Hall FL (2023) The advent of a pan-collagenous CLOVIS POINT for pathotropic targeting and cancer gene therapy, a retrospective. Front. Mol. Med. 3:1125928. doi: 10.3389/fmmed.2023.1125928
Received: 16 December 2022; Accepted: 13 February 2023;
Published: 28 February 2023.
Edited by:
Shanchun Guo, Xavier University of Louisiana, United StatesReviewed by:
Hua Yang, University of South Florida, United StatesJinzhong Chen, Fudan University, China
Copyright © 2023 Gordon and Hall. This is an open-access article distributed under the terms of the Creative Commons Attribution License (CC BY). The use, distribution or reproduction in other forums is permitted, provided the original author(s) and the copyright owner(s) are credited and that the original publication in this journal is cited, in accordance with accepted academic practice. No use, distribution or reproduction is permitted which does not comply with these terms.
*Correspondence: Erlinda M. Gordon, ZXJsaW5kYS5nb3Jkb25AZ21haWwuY29t