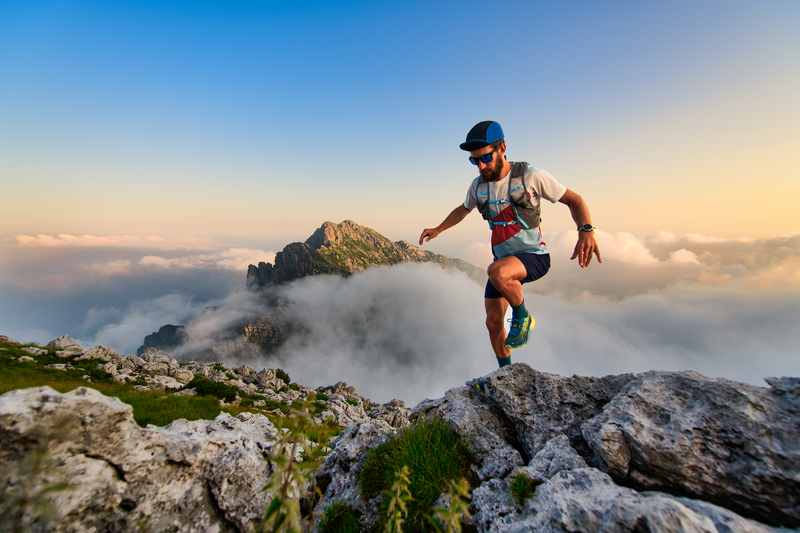
95% of researchers rate our articles as excellent or good
Learn more about the work of our research integrity team to safeguard the quality of each article we publish.
Find out more
ORIGINAL RESEARCH article
Front. Microbiol. , 02 April 2025
Sec. Aquatic Microbiology
Volume 16 - 2025 | https://doi.org/10.3389/fmicb.2025.1570274
Lionfishes (Pterois spp.), originally native to the Indo-Pacific and Red Sea, have become one of the most invasive marine species globally, including the recent establishment in the Mediterranean Sea. This study investigates the microbiota of lionfish to explore its potential role in their invasion success and establishment. Using high-throughput sequencing and microbiota analyses, we characterized the species-specific core microbiome and identified habitat-specific markers across different regions (Red Sea, Mediterranean Sea, Caribbean, and aquarium populations) and organs. Focusing on the Mediterranean invasion, we tracked lionfish distribution and population dynamics along the Israeli coastline from 2017 to 2023, monitoring size, seasonal trends, and depth preferences. Our findings reveal that lionfish initially established themselves in deeper waters before expanding to shallower habitats, with a gradual increase in population size and body length over time. From a microbial aspect, we compared the microbiota of lionfish organs and identified a similar pattern (Photobacterium), to Earlier Lessepsian migrants fish species. This study provides novel insights into the interactions between microbiota and host ecology, shedding light on the mechanisms that may support the successful invasion. This study contributes to the understanding of lionfish invasion dynamics in the Mediterranean. It highlights the microbiota as an integral component for studying the ecological and biological mechanisms underpinning invasive species’ success and establishment of lionfish.
Lionfish (LF) species (Pterois volitans and P. miles), are reef fish native to the Indo-Pacific and Red Sea, are considered among marine ecosystems’ most notorious invasive species (Côté and Smith, 2018). They have become significant and successful invaders with global distribution, including the northwestern Atlantic, the eastern coast of the United States, Bermuda, the Caribbean region and the Gulf of Mexico (Schofield, 2009; Côté and Smith, 2018), Brazil (Ferreira et al., 2015) and more recently, the Mediterranean Sea (Kletou et al., 2016). In the Mediterranean Sea, P. miles were first recorded near Israel in 1991 (Golani and Sonin, 1992), and the invasion notably accelerated from the mid-2010s and onwards. The Suez Canal has served as a key pathway for this introduction, also termed “Lessepsian migration” facilitating the species’ establishment in new habitats (Bariche et al., 2017; Stern et al., 2019). The unprecedented speed and scope of their spread highlight their adaptability and the significant impact they impose on invaded ecosystems.
Several biological and ecological factors contribute to the LF’s invasion success (Côté and Smith, 2018; Bottacini et al., 2024). Lionfish are generalist predators whose diet includes a wide variety of fish and invertebrates. A stomach content analysis has revealed that LF in the Mexican Caribbean preyed on nearly 50 different local fish species (Arredondo-Chavez et al., 2016), underscoring their lack of dietary selectivity and facilitating their adaptation to new environments. Their early maturation (within the first year) combined with a robust strategy (asynchronous egg release) and fast growth, contribute to the rapid increase in LF density and their successful establishment in invaded areas (Morris et al., 2011; Côté et al., 2013; Albins and Hixon, 2013). Additionally, the absence of natural predators in the invaded regions allows LF populations to grow unhindered, thus significantly disrupting the local marine ecosystems. This invasion leads to a decline in local species and changes biodiversity and community structure, affecting the reef ecosystems’ resilience (Albins and Hixon, 2008; Green et al., 2012; Côté and Smith, 2018).
Invasive species are often characterized by their remarkable adaptability, which enables them to thrive in novel environments with different abiotic and biotic conditions compared to their native habitats. The microbiota may influence various host functions, including immune responses, nutrient acquisition, and environmental resilience, providing an invasive species with significant advantages in unfamiliar territories (Aires et al., 2016; Ronanki, 2024; Coats and Rumpho, 2014). Another aspect concerns the symbionts of invasive species in the new environments. Numerous studies have shown that various stressors, such as temperature changes, pollution, and fluctuations in resource availability, can potentially impact the dynamics of bacterial invasion in aquatic ecosystems (Liu et al., 2012; Bagra et al., 2022). These stressors may not only affect the composition of bacteria and disrupt established symbiotic relationships but also create new niches that invading microorganisms can exploit (Amalfitano et al., 2015; Cavicchioli et al., 2019; Paerl et al., 2016).
Although research has explored the effects of environmental changes on microbiota, the specific role of microbiota in supporting the survival and establishment of invasive species remains inconclusive. The role of symbiotic bacteria in invasive species has been investigated mainly in terrestrial ecosystems (Lu et al., 2016; Bang et al., 2018). Research focused on insects for example, has demonstrated that altering the microbiota can influence various traits, including: suppressing native competitors (Vilcinskas et al., 2013), enhancing nutrient acquisition (Adams et al., 2011), promoting pest resilience (Brown et al., 2014) and increasing fecundity and survival rates (Himler et al., 2011). These traits contribute to establishing and spreading invasive species within new environments. However, only a few studies have demonstrated the contribution of the microbiota to invasion within marine ecosystems. Among them, a study on the invading alga Caulerpa racemosa showed that an increase in bacteria involved in sediment biogeochemical processes contributed to the invasion’s success by displacing native seagrasses (Aires et al., 2013). Another study demonstrated that symbiotic relationships between chemoautotrophic bacteria and marine invertebrates can influence metabolic capabilities and expand the adaptation to a variety of ecological niches for the host (Lee et al., 1999). Thus far, only a few studies have examined the LF microbiota aspect and have mainly focused on the Indo-Pacific (native) and western Atlantic (invaded) regions (Stevens and Olson, 2013; Stevens and Olson, 2015; Stevens et al., 2016). However, these were conducted about a decade ago, using methods that were less advanced and comprehensive than those available today, such as high-throughput amplicon sequencing and metagenomics.
Invasive species may serve as ideal models for investigating the host-microbe-environment interactions. By comparing the microbiota in native and invaded environments, we can investigate three aspects: (1) how the new environmental conditions shape and change the composition and diversity of the bacterial communities; (2) what is the stable core microbiota (that persists despite the changing conditions), and (3) to examine whether the microbiota impacts the success of invasion. This study compares the microbiome aspect of LF from different origins: The Mediterranean and the Caribbean (invaders), the Red Sea (native), and local aquarium. In addition, we chose to focus on the LF in the Mediterranean Sea, a relatively recent and successful invader, making it an ideal case study for examining its invasion, establishment, and interactions with microbial symbionts. To this end, fish surveys, which included fish counts and visually estimated lengths, were conducted over a five-year period. In parallel, for comparison, microbial profiling and trophic position (TP) of the two earlier Lessepsian invasive species, Sargocentron rubrum and Siganus rivulatus, were also described.
Between 2019 and 2022, a total of 90 fish specimens were sampled, comprising five individuals of S. rubrum, nine of S. rivulatus, and 79 of Pterois spp. (lionfish) (Supplementary Table S1). Lionfish were collected from the Gulf of Eilat in the Red Sea (RS), the Mediterranean coast of Israel (MS), along the Israeli coast, Sint Eustatius in the Caribbean Sea (CA) and from captive populations in the Israel Aquarium in Jerusalem (AQ_J).1 LF from the latter were originally captured off the coast of Kenya and kept in the aquarium since 2018. Fish specimens from the wild (from MS, RS and CA) were caught using spearguns and transported on ice to the laboratory. In contrast, captive fish specimens were collected with (hand) nets, sampled immediately, and returned to the aquarium. Size (total length) and sex (when applicable) indices were recorded for each individual. Sterile swabs were used to collect samples from four organs of each specimen: cloaca, mouth, gills, and skin. Surrounding water was also sampled, 1.5 liters filtered using a single-use “Nalgene RapidFlow Filters” 0.2 μm (Thermo Scientific, cat no. 566–0020, Israel). The swabs and filters were stored at −20°C for further analysis. In total, 378 (365 fish and 13 water) samples were collected and analyzed (Supplementary Table S2).
As part of the Morris Kahn Marine Research Station (MKMRS) monitoring program, fish surveys were conducted by SCUBA in central (Sdot-Yam) and northern (Achziv) Israel during the Spring (April to May) and Fall (October to November) at depths of 10, 25, and 45 meters. At each depth, an area of 250 square meters was surveyed. Surveys included fish counts and visually estimated lengths. The data on LF from these surveys were analyzed for the period of 2017–2023. The fish survey method and data are available at https://med-lter.haifa.ac.il/database-hub/.
DNA was extracted from all samples using the DNeasy PowerSoil Pro Kit (Qiagen)2 following the manufacturer’s instructions. Partial sequences of the 16S rRNA gene at the V4 hypervariable region were amplified using the primers 518F (ACCAGCAGCCGCGGTAATACG) and 806R (GGACTACNVGGGTWTCTAAT) (based on Apprill et al., 2015; Parada et al., 2016 with few modifications) that contained 5′ common sequence tags (CS1 and CS2) (Moonsamy et al., 2013). Amplicons were generated using a two-stage PCR amplification protocol described by Naqib et al. (2018). Cycling conditions for the first stage PCR were 94°C for 15 s, 50°C for 20s, and 72°C for 20s for 15 cycles, with an additional 15 cycles with 62°C for 15 s (annealing stage). Subsequent steps were carried out at the Genome Research Core (GRC) within the Research Resources Center (RRC) at the University of Illinois at Chicago (UIC). A second amplification was performed for each sample, with a separate primer pair with a unique 10-base barcode obtained from the Access Array Barcode Library for Illumina (Fluidigm, South San Francisco, CA; Item# 100-4876). Cycling conditions were 95°C for 5 min, followed by eight cycles of 95°C for 30 s, 60°C for 30 s and 72°C for 30 s. The amplified barcoded PCR products were pooled and purified using an AMPure XP cleanup protocol (0.6X, vol/vol; Agencourt, Beckmann-Coulter). With a 15% phiX spike-in, the pooled libraries were loaded onto an Illumina MiniSeq mid-output flow cell and sequence (2×153 bases paired-end reads). Barcode sequences were used for sequence read de-multiplexing of raw data, which was then recovered as FASTQ-formatted files. Raw sequence data is available in the NCBI SRA database under PRJNA1213691.
The Dada2 pipeline3 (dada2 package version 1.20.0) was used for sequence data processing. The processing was conducted for each set of samples and each run separately for the following steps: Sequences were filtered and trimmed for quality using the “filterAndTrim” command with the parameters maxN set to zero, maxEE set to 2, trimLeft set to 21 and 20 bases for the forward and reverse reads, respectively. The sequence error estimation model was calculated using the “learnErrors” option, and then, the dada2 algorithm for error correction was applied using default parameters. Forward and reverse reads were then merged with minimum overlap set at eight bp. Suspected chimera was detected and removed using the command “removeBimeraDenovo,” and a count table was produced. To obtain a taxonomic assignment for each ASV, ASV sequences were aligned to the ARB-Silva small subunit rRNA database (version Silva_nr_138.1) using the command “assignTaxonomy” with default parameters. However, minBoot set at 80% (Supplementary Table S2).
Microbiota analysis procedures were conducted in R (version 4.3.1). For analysis of microbiota composition, counts data had been normalized by the cumulative sums squares (CSS) method using the R package “metagenomSeq” (version 1.42.0). Normalized counts were then summed sample-wise, based on taxonomic assignment, up to the genus taxonomy level. Core microbiota composition was determined based on the prevalence of the different genera detected. A genus was considered a core member if its prevalence at each site tested was above 25%. To test the effect of the collection site (Sea) or organ on the composition of the microbiota, a permutational analysis of variance (PERMANOVA) test was performed using the “adonis2” function in the R package “vegan” (version 2.6.4). Non-metric multidimensional scaling (NMDS) ordination was calculated using the R package “vegan” based on Bray-Curtis dissimilarities. To extract microbiota markers, Linear Discriminant Analysis (LDA) Effect Size (LEfSe) was calculated using the function “run_lefse” from package “microbiomeMarker” (version 1.6.0). The association was significant for Bejnamini-Hochberg adjusted p value < 0.05 and LDA effect size of 2. For the calculation of the effect of sex, PERMANOVA was performed separately, using 112 samples corresponding to 34 fish specimens for which sex could be determined. Additionally, the contribution of sex to variance was tested by canonical correspondence analysis (CCA) in “vegan” considering the model microbiota~sex+site+organ. CCA model and items were considered significant at p < 0.05.
For alpha-diversity parameters calculations, the count data was subsampled to 4,000 reads per sample using the function “rrarefy” from the R package “vegan” package (version 2.6.4). Using the rarified data, Shannon H’s index of diversity, and the Simpson’s index for evenness and richness, calculated as observe number of genera were calculated with “vegan.” To test the effect of site, organ and their interaction on alpha diversity parameters, the aligned rank-transformed (ART) ANOVA test was applied, with the R package “ARTool” (version 0.11.1). Post-hoc pair-wise tests were also conducted with package “ARTool” using the “art.con” function and the Benjamini-Hochberg method for p value adjustment against false discovery.
Lionfish microbiota composition was compared among four sites (MS, RS, CA and AQ_J) and among the four organs: mouth, gills, cloaca, and skin (Total 307 samples; Supplementary Table S1). To define the LF core microbiota, we examined the prevalence of the different genera identified and considered those with prevalence >25% at all of the examined sites. Based on these criteria, 18 bacterial genera were recognized as the LF core microbiota (Figure 1A; Supplementary Table S2). The most prevalent genera were Photobacterium, Vibrio, Pseudomonas, and Shewanella (prevalence above 80% of the samples), all belonging to the class Gammaproteobacteria. While a robust core microbiota was thus suggested, an inspection of the dominant genera in each organ at each site (>4% mean relative abundance) indicated major differences in composition between sampling sites (Figure 1B). The contribution of the site to variation in microbiota composition, as assessed by PERMANOVA was highly significant (p < 0.001, R2 = 0.133). Pair-wise comparisons further indicated unique microbiota composition for each site (Supplementary Table S3). This was corroborated by NMDS ordination (Figure 2A). Linear decomposition model (LDA) effect size analysis was used to identify key genera as markers of each site (Supplementary Table S4, LDA). The most noted genera identified (LDA score > 3) were Psyhromonas (AQ_J), Pseudomonas (CA), Photobacterium and Vibrio (MS), Cetobacterium, including Clostridiaceae and Caedibacter (RS) (Figure 2B). The contribution of the organ to variation was examined for each site separately, using PERMANOVA. Organ effect was significant for LF sampled at site RS (p < 0.001, R2 = 0.087) and CA (p < 0.001, R2 = 0.066), as was indicated from inspection of composition (Figure 1B). Post-hoc pairwise tests for organs in RS concluded a significant difference between each pair, with the greatest effect between the gills and the cloaca microbiota (gills-cloaca: p < 0.001, R2 = 0.089; gills-mouth: p = 0.005, R2 = 0.041; gills-skin: p < 0.001, R2 = 0.072; mouth-cloaca: p < 0.001, R2 = 0.066; mouth-skin: p = 0.006, R2 = 0.046; skin-cloaca: p = 0.022, R2 = 0.039) while in site CA significant difference was observed only between skin and cloaca (p < 0.029, R2 = 0.039). The effects of site and organ were also reflected in microbiota diversity, examined at the genus level of taxonomy. ART-ANOVA test confirmed the effect of the site, without significant interaction for Shannon H′ and Simpson indices (Table 1). Richness, calculated as the observed number of genera, was significant for site and organ as well as their interaction Based on pairwise comparisons among sites the CA site had the lower diversity compared to all other sites, and MS diversity was lower than that of AQ_J. Pairwise comparison among organs calculated significantly lower diversity for the cloaca compared to the mouth and the skin (Supplementary Table S5). Another aspect examined for effect on microbiota composition was sex. The sex could be identified for a subset of 34 fish specimens (112 samples). PERMANOVA test indicated no significant effect for sex. However, canonical correspondence analysis (CCA) did significantly link sex to microbiota composition (χ2 = 0.31, p = 0.005) and explains ~1.1% of inertia (Figure 3).
Figure 1. Composition of lionfish microbiota. Lionfish microbiota was determined by amplicon sequencing of 16S rRNA gene fragments from total DNA extracted from 307 samples, representing the gills, cloaca, skin and mouth of specimens collected at the Mediterranean Sea (MS), Red Sea (RS), Caribbean Sea (CA) and the Jerusalem aquarium (AQ_J). (A) Core microbiota composition. Includes genera for which prevalence was >25% at each of the sampled sites. (B) Composition of dominant genera (relative abundance >5% at least at one site).
Figure 2. Variation in lionfish microbiota composition among sites. (A) Non-metric multidimensional scaling (NMDS) ordination, based on Bray-Curtis distances (StressK = 3 = 0.16). (B) Microbial markers for sites, based on linear decomposition model effect size (LEfSe) analysis. Presented are significant markers (Benjamini-Horchberg adjusted p value < 0.05) for which the effect size was >2.5.
Figure 3. Contribution of sex to variation in lionfish microbiota composition. Canonical correspondence analysis (CCA) analysis determined sex as a significant factor (p < 0.05) contributing 2.8% of variance in microbiota composition. Presented is CCA ordination plot.
Interestingly, when analyzing the bacterial overlap at the genus level across paired organs, it was found that over 67% of the bacteria identified in MS were shared between the two regions. Conversely, a notably higher percentage of bacteria was unique to RS, with 47–41%, compared to only 22–23% of unique bacteria in MS (Figure 4). Noting the major difference in the relative abundance of the Photobacterium in the MS vs. RS samples (Figures 1B, 2B), we extended our microbiota comparison to additional Lessepsian invasive fish species. We chose Sargocentron rubrum and Siganus rivulatus, known as Earlier Lessepsian migrants [S. rubrum reported by Haas and Steinitz, 1947 and S. rivulatus by Steinitz, 1927], and characterized their microbiota. Overall relative abundance of Photobacterium ranged differently levels among species (0.6–59.2% for S. rubrum; 0–24.2% for S. rivulatus). Nevertheless, regardless of the organ, Photobacterium was much more dominant in MS specimens compared to RS ones (Figure 5). Interestingly, the Photobacterium relative abundance was negligible (less than 1%) in the MS water samples (Supplementary Table S2).
Figure 4. Venn diagrams presenting shared and unique microbial genera among sites for different organs of Lionfish. Numbers in brackets represent the percentage from total number identified genera at the specific site and organ.
Figure 5. Dominance of Photobacterium across species, sites and organs. Relative abundances of Photobacterium are presented as pie charts.
Based on the fish surveys, we analyzed the LF data and compared the number of fish observation and their size by site, year, season, and depth (Figure 6). LF were first recorded in fish surveys in 2017 in the northern site (Achziv). The first sightings of LF in the Sdot-Yam area occurred only 2 years later. At both sites, initial observations of LF were recorded from the deepest transect (45 m), with sightings at shallower depths (25 m and 10 m) appearing only 2–3 years later. A consistent upward trend in LF population size was observed at Achziv. In contrast, no such trend was noted at Sdot-Yam (Figure 6A). Nevertheless, at both sites, the majority of observations were in the fall (Figure 6B). Fish surveys also assessed the approximate size of the fish (in terms of length) and indicated a substantial increase in size observation between 2017 and 2023 (Figure 7A). Length measurement of collected fish specimens from MS (2019–2021), RS (2020–2022) and CA (2021) confirmed that during the first years of invasion, LF at MS were smaller in size compared to RS or CA (Figure 7B). Considering that smaller sizes may be accounted for by the quality of nutrition, we examined the TP value. In LF, the value of TP was the lowest in the CA. However, no differences were observed between RS and MS (Figure 7C). Interestingly, the opposite trend was found for the two other Lassepsian invaders examined, S. rubrum and S. rivulatus, TP values were lower in MS compared to RS.
Figure 6. Lionfish establishment in the Mediterranean Sea (2017–2022). Numbers of observations of lionfish at different seafloor depths along the Achziv and Sdot-Yam transects (A) or at different seasons (B). Taken from MKMRS database (https://med-lter.haifa.ac.il/database-hub/).
Figure 7. Lionfish establishment at the Mediterranean Sea (2017–2023). (A) Size estimate distribution from visual fish surveys. (B) Size distribution of captured lionfish specimens at different sites. (C) Trophic position of captured lionfish specimens, calculated based on Trophic isotopic discrimination of amino acids (glutamic acid and phenylalanine) (Takizawa and Chikaraishi, 2024).
Lionfish are widely regarded as one of the most invasive and successfully established species in the marine environment. Originally native to the Indo-Pacific Ocean and the Red Sea, they have spread across the entire tropical western Atlantic, including the Brazilian coast and the Mediterranean Sea (Côté and Smith, 2018 Soares et al., 2022, 2023 Kletou et al., 2016). As previously described, various parameters such as the ability to survive, reproduction rate, the absence of predators, and its adaptation to a wide range of conditions, contributed to the success of its invasion and establishment (Bottacini et al., 2024). In this study, we focused on the microbiome aspect of LF, aiming to characterize the core microbiome shared by the species and to examine differences and variations across different habitats. We also followed the process of the Lessepsian migration and the establishment of LF populations in the Mediterranean Sea.
The microbiota of invasive species is crucial to their success and interactions in new ecosystems. Microbial diversity and functional stability can influence how likely an invasive species is to establish itself (Amalfitano et al., 2015 Huang et al., 2019). We characterized the core microbiome of LF based on samples collected from four sites, and various organs. The Gammaproteobacteria class exhibited the highest relative abundance within the core microbiome, with dominant genera such as Photobacterium, Vibrio, Pseudomonas, and Shewanella (Figures 1A, 2C). The information on the microbiota of LF is very limited; however, Stevens and Olson (2015) and Stevens et al. (2016) reported similar findings regarding the bacterial groups in LF from the Indo-Pacific and Western Atlantic. Notably, Pseudoalteromonas and Photobacterium, identified as key markers in the invaded regions (CA and MS, respectively, Figure 1B) are known to exhibit broad antibacterial activity. Compared to that of local fish, this robust antibacterial capability may provide LF with a competitive advantage, enhancing their resilience and success in new habitats (Stevens et al., 2016).
Various environmental parameters, such as temperature, pH, and salinity, have been shown to significantly influence the diversity and abundance of microbiota (Thompson et al., 2017; Coutinho et al., 2015; Hicks et al., 2018). In this study, we observed notable differences in microbiota composition across the analyzed sites, with each site characterized by unique bacterial markers. Identifying site-specific markers may facilitate tracing the origin of LF habitats, providing a valuable tool for monitoring their distribution. This knowledge is particularly relevant in cases of species invasion, where understanding habitat origins can inform management strategies and control measures. In the RS, which represents a native habitat for LF, we identified the highest number of significant microbial markers (with scores exceeding 2.5). This high number of markers may also reflect the influence of the organ factor, which contributed to the microbiota variation in this region, adaptation and fostering niche-specific microbiota associations within different organs (Kuang et al., 2020; Meron et al., 2020). In contrast, fish introduced to new habitats, such as in invasive contexts, may only develop distinct organ-specific microbiota at later stages of establishment. The unique and diverse bacterial communities in each organ of RS lionfish suggest the evolution of stable and well-adapted microbiota, shaped over generations by local environmental pressures. In the CA region, a partial trend was observed, potentially reflecting an intermediate stage in establishing lionfish populations. First documented in the Caribbean in the early 2000s, lionfish were officially recognized as a successful invasive species around 2004–2005 (Schofield, 2009). This indicates that LF in the CA region are beginning to adapt to the local environment. By contrast, the relatively recent invasion of LF in the MS region suggests that adaptation and microbiome stabilization are still in their early stages.
In the case of LF from AQ_J, although their origin traces back to the coast of Kenya (a native habitat for LF), the aquarium environment—characterized by artificial water in a closed system—differs significantly from natural conditions. This divergence is also reflected in the composition of the bacterial community. This may explain the observed similarity in microbiota composition across organs and the absence of organ-specific effects in the AQ_J site, a pattern similar to those observed in invaded regions. It is also noteworthy that lionfish invasions in the Atlantic Ocean and Caribbean Sea are largely attributed to releases from aquariums. Such conditions may have shaped the microbiota of released lionfish, resulting in differences from their wild counterparts. While data for direct comparisons are unavailable, this highlights the potential role of aquarium environments in influencing microbiota composition in ways that could facilitate invasion success. Moreover, the environment serves as a microbial reservoir to which organisms are continuously exposed, facilitating the acquisition and exchange of bacteria between the host and its surroundings. Stevens and Olson (2013) demonstrated that eggs extracted from the ovaries of pregnant LF were free of bacteria, indicating the absence of vertical transmission and highlighting the environment’s role as a source of bacterial acquisition for the microbiota composition. The Sex factor was also found to significantly influence the microbiota of LF as reported previously in other marine fish. Most studies have shown sex-based differences in the microbiota of the intestinal organs (Piazzon et al., 2019; Martyniuk et al., 2022; Li et al., 2022). However, microbiota differences have also been documented in other organs, such as the skin (Kramp et al., 2022; Casadei et al., 2023) and buccal cavity (Abdelhafiz et al., 2021). These findings, similarly observed in LF, highlight the role of physiological and hormonal differences between sexes in shaping microbial communities, potentially influencing immune responses and adaptation to environmental conditions (Chen et al., 2018).
Early stages of establishment of the lionfish (Pterois miles) in the Mediterranean was reported around 2012 from Lebanon (Bariche et al., 2013), although a single observation from Israel dates back to 1991 (Golani and Sonin, 1992). The second sighting off the Israeli coast occurred in 2013 within the northern Achziv marine reserve, and during 2015 the lionfish has progressed southward to central Israel (Stern et al., 2018). By 2024, P. miles had spread westward to Rhodes and Sicily, successfully establishing populations throughout the eastern Mediterranean (Bottacini et al., 2024). Genetic studies have confirmed that this invasion originated from the Red Sea via the Suez Canal (Bariche et al., 2017; Dimitriou et al., 2019). The Suez Canal serves as one of the world’s busiest marine trade routes and provides easy access for non-indigenous species to migrate from the RS to MS through ballast water or as fouling communities on ships (Williams et al., 1988; Galil et al., 2015; Lavoie et al., 1999; Costello et al., 2022). The establishment of lionfish in the Mediterranean follows a well-documented pattern of biological invasion, where populations stabilize in nearby regions before expanding to more distant areas. This pattern is also characteristic of other invasive species, such as Diadema setosum, which have similarly followed the Lessepsian migration route through the Suez Canal (Zirler et al., 2023). During the establishment process, invasive populations grow, adapt to local environmental conditions, and increase their potential for further expansion (Kolar and Lodge, 2001; Zirler et al., 2023).
We monitored the presence and distribution of LF (P. miles) along the Israeli coast beginning in 2017, focusing on two sites, two seasons and three depth zones. Over the 5 years, we observed, in Israeli coast, a spreading process, north to south and from deeper to shallower waters (Figures 6A,B), similar to the findings of Stern et al. (2018). The initial invasion of LF along the northern coast of Israel (Achziv), can be attributed by the cyclonic circulation pattern characterizing the Levant Basin in the eastern Mediterranean (Pinardi and Masetti, 2000); however, it is more likely explained by the continuous complex rocky habitats, which may serve as a suitable recruitment area and are characteristic of the initial habitats where LF populations first established in Cyprus, Turkey, and Greece (Stern et al., 2018). Notable seasonal differences in lionfish density and distribution were observed, with higher numbers recorded in the fall. A similar pattern has been reported in Israel, where the abundance of other invasive fish in rocky reefs tends to rise during this season (Lazarus et al., 2022). This seasonal trend may be linked to the LF’s physiological adaptations to warmer temperatures. For instance, in Cyprus, LF reproduction peaks during the summer and fall (Savva et al., 2020; Mouchlianitis et al., 2022). Additionally, lionfish exhibit enhanced feeding efficiency and increased food intake at elevated temperatures, along with a lower metabolic cost of digestion, allowing them to exploit prey resources more effectively while maintaining energy efficiency (Steell et al., 2019). Environmental factors such as temperature fluctuations, predation pressure, food availability, and reproductive cycles may drive shifts in habitat preferences, leading lionfish to adjust their depth range or seek more sheltered areas (Eddy et al., 2016). Seasonal health status may also play a role in fish distribution and behavior; for example, Minnow fish species exhibited significantly better health status (lower HAI score) and lower parasite abundance and diversity in autumn compared to spring (Almeida et al., 2024). The observed seasonal trends in lionfish density and distribution highlight the need for further research to better understand the factors influencing this seasonal pattern. Initial lionfish observations were recorded at a depth of approximately 45 meters before they gradually appeared in shallower waters (25 and 10 meters), consistent with findings reported by Stern et al. (2018). This distribution pattern may suggest that deeper habitats likely offered a more protected environment with reduced competition, abundant food resources, and lower predation pressure (Jones and Ricciardi, 2014; Chaikin et al., 2022). Airey et al. (2023) examined the post-settlement dispersal patterns of invasive lionfish, highlighting the connection between deep and shallow habitats. Their study found that approximately 34.5% of lionfish in shallow waters had migrated from deeper habitats, whereas only about 4% moved in the opposite direction, from shallow to deep waters. This pattern suggests that the primary direction of LF dispersal after settlement is from deeper regions to shallower habitats, emphasizing the role of deep-water areas as potential initial settlement zones before LF spreads into more coastal regions. In addition, their ability to exploit a range of depths likely contributes to their success as an invasive species (Kletou et al., 2016).
An increasing trend in the average body size of LF has been observed over the years in surveys conducted in the Mediterranean Sea. The average length (TL) of LF sampled in the RS region (where LF are native) and in CA (an earlier invasion area compared to MS) was approximately 30 cm. In contrast, the average length of LF sampled in the MS region was significantly smaller and only after 5 years (in 2022) did the LF population in this region reach this size. This observation may indicate the species’ establishment process and thriving in newly invaded regions. Furthermore, studies show that larger body sizes offer several advantages, including improved resources competition, enhanced survival in various environments, and increased reproductive rates. As a result, species with larger body sizes are generally more successful as invaders (Roy et al., 2002; Schröder et al., 2009).
Dietary flexibility plays a crucial role in the success of invasive species as it enables them to exploit new resources in diverse environments. Previously studies that compared Lessepsian carnivorous invaders and their counterparts in the RS showed significant differences in isotopic patterns. These differences were more pronounced in S. rubrum, which is an earlier invader, compared to Pterois miles (Tsadok et al., 2023). A similar trend was seen in the calculated TP, where significant differences appeared only in the earlier-established invaders (Figure 7C). In contrast, no difference was seen in TP of LF, which may suggest that the LF are still in the process of establishment and adaptation into the new environment. However, the TP of Caribbean LF was significantly lower from both MS and RS, which again emphasizes the environmental factor. In both S. rubrum (carnivores) and s. rivulatus, (herbivorous) the TP was higher in the RS than in the MS. This reflects how the Mediterranean environment influences food web dynamics and necessitates dietary adjustments by invasive fish during their acclimatization and suggests that Lessepsian species capable of dietary adaptation may be more likely to become successful colonizers (Golani, 1993). It is important to note that fish size was not considered in the TP analyses in this study and it may have affected our results, since fish diet, and particularly LF’s diet shifts as they grow (Dahl and Patterson, 2014). Continued study of the LF in the Mediterranean Sea will be able to track the shifts that appear over time in isotope patterns and TP.
Photobacterium is widely found in marine environments, where it plays a significant role in ecosystem dynamics, particularly through its interactions with marine fish. This genus, part of the Vibrionaceae family, is notable for its symbiotic and pathogenic interactions with various marine organisms (see review: Labella et al., 2017). Symbiotically, certain Photobacterium species, such as P. phosphoreum and P. leiognathi, produce bioluminescence in light organs of fish, contributing to communication, predation, camouflage, and predator protection. Additionally, these species contribute to nutrient cycling by releasing essential fatty acids, which provide nutritional resources within marine food webs and support broader ecological interactions (Moi et al., 2017; Urbanczyk et al., 2011; Zarubin et al., 2012). However, much of the literature on Photobacterium species focuses on their potential pathogenicity. This genus is frequently linked to diseases in various marine species, especially fish. For instance, P. damselae, particularly its subspecies P. damselae and P. piscicida, poses significant threats to marine aquaculture and wild fish populations. These bacteria are responsible for causing photobacteriosis, a disease characterized by skin lesions, septicemia, and high mortality rates among affected fish, such as groupers and sea bream (Rivas et al., 2013; Labella et al., 2017; Austin and Austin, 2016).
In our study, Photobacterium was found in all samples and constituted a dominant component of the LF microbiome core. However, compared to the RS region, the relative abundance of Photobacterium was significantly higher in the MS, where it exceeded 50% across all examined organs. This high abundance may explain the lower number of taxa (Genus level) observed in the MS compared to the RS region, in all examined organs (Figure 4). Interestingly, further comparative analysis of other Lessepsian invasive fish species, S. rubrum S. rivulatus, revealed a similar trend, with a significantly higher relative abundance of Photobacterium in the fish organs from the MS compared to the RS region. Invasive species may acquire beneficial microbes from co-occurring native species, which can accelerate their adaptation to local conditions and reduce invasion lag times (Martignoni and Kolodny, 2023). The Photobacterium has been also found to have high chitin-degrading capabilities (Itoi et al., 2006). The high presence of Photobacterium in lionfish in the MS may provide them with a significant nutritional advantage by aiding in the digestion of chitin, allowing them to exploit a wider range of prey (such as crustaceans), including chitinous invertebrates, thereby improving their survivability and contributing to their persistence in the ecosystems.
Environmental stress (as a new habitat and conditions) can influence the host’s susceptibility and affect its sensitivity to the presence of the pathogen and the outbreak of disease (Bernardo-Cravo et al., 2020). In our samples, the dominant Photobacterium sequences found in both the MS and RS samples were identified as Photobacterium damselae subsp. damselae, based on BLAST results from NCBI. Interestingly, although this species is known to be pathogenic, no external or internal signs of disease were observed in the analyzed fish. This aligns with similar observations in additional studies on wild Mediterranean fish and sharks, where Photobacterium species presence did not correlate with visible disease symptoms (Meron et al., 2020; Bregman et al., 2023). The high relative abundance of Photobacterium in Mediterranean lionfish (LF) raises further questions about its potential role in the fish’s resistance and adaptation. For instance, are lionfish resistant to these pathogens, or do these bacteria contribute to their acclimatization to new environmental conditions, thereby enhancing their success as invasive species? These questions underscore the need for further research to explore the dynamics and ecological mechanisms underlying the interactions between lionfish and Photobacterium.
This study focuses on the microbiota of the lionfish (LF), characterizing its species-specific core microbiome and identifying habitat-specific markers while discussing its potential role in invasion and establishment. The findings suggest that lionfish (LF) are still establishing themselves in the Mediterranean environment but are exhibiting a growing presence. The relatively recent invasion of LF from the Red Sea to the Mediterranean offers a unique opportunity to monitor microbial and ecological establishment processes, including population distribution, body size, and dietary patterns. This understanding can offer valuable insights into ecological impacts and support the development of effective management strategies for invasive species.
The datasets presented in this study can be found in online repositories. The names of the repository/repositories and accession number(s) can be found in the article/Supplementary material.
The animal study was approved by Nature and Parks Authority, Israel (n. 2021/42722). The study was conducted in accordance with the local legislation and institutional requirements.
DM: Conceptualization, Data curation, Formal analysis, Funding acquisition, Investigation, Writing – original draft, Writing – review & editing. ML: Formal analysis, Methodology, Software, Writing – review & editing. SR: Conceptualization, Data curation, Writing – review & editing. YK: Data curation, Writing – review & editing. EK: Data curation, Resources, Writing – review & editing. KK-W: Data curation, Resources, Writing – review & editing. TZ-K: Data curation, Formal analysis, Methodology, Writing – review & editing. ES: Data curation, Formal analysis, Methodology, Writing – review & editing. RT: Data curation, Methodology, Writing – review & editing. HN: Data curation, Methodology, Writing – review & editing. SE: Data curation, Methodology, Writing – review & editing. DT: Conceptualization, Data curation, Funding acquisition, Resources, Writing – review & editing.
The author(s) declare that financial support was received for the research and/or publication of this article. The research leading to these results received partial funding from the European Union’s Horizon 2020 research and innovation program under grant agreement no 10730, ASSEMBLE Plus project.
We would like to thank the staff at Caribbean Netherlands Science Institute (CNSI), The Gottesman Family Israel Aquarium, Jerusalem and Morris Kahn Marine Research Station (MKMRS) for their assistance and support.
The authors declare that the research was conducted in the absence of any commercial or financial relationships that could be construed as a potential conflict of interest.
The authors declare that no Gen AI was used in the creation of this manuscript.
All claims expressed in this article are solely those of the authors and do not necessarily represent those of their affiliated organizations, or those of the publisher, the editors and the reviewers. Any product that may be evaluated in this article, or claim that may be made by its manufacturer, is not guaranteed or endorsed by the publisher.
The Supplementary material for this article can be found online at: https://www.frontiersin.org/articles/10.3389/fmicb.2025.1570274/full#supplementary-material
Abdelhafiz, Y., Fernandes, J. M., Stefani, E., Albanese, D., Donati, C., Kiron, V., et al. (2021). Power play of commensal bacteria in the buccal cavity of female Nile tilapia. Front Microbiol. 12:773351.
Adams, A. S., Jordan, M. S., Adams, S. M., Suen, G., Goodwin, L. A., Davenport, K. W., et al. (2011). Cellulose-degrading bacteria associated with the invasive woodwasp Sirex noctilio. ISME J. 5, 1323–1331. doi: 10.1038/ismej.2011.14
Aires, T., Serrão, E. A., and Engelen, A. H. (2016). Host and environmental specificity in bacterial communities associated to two highly invasive marine species (genus Asparagopsis). Front. Microbiol. 7:559. doi: 10.3389/fmicb.2016.00559
Aires, T., Serrão, E. A., Kendrick, G., Duarte, C. M., and Arnaud-Haond, S. (2013). Invasion is a community affair: clandestine followers in the bacterial community associated to green algae, Caulerpa racemosa, track the invasion source. PLoS One. 8:e68429. doi: 10.1371/journal.pone.0068429
Airey, M. E., Fogg, A. Q., and Drew, J. A. (2023). Invasive lionfish dispersal between shallow-and deep-water habitats within coastal Floridian waters. Biol. Invasions. 25, 3983–3991. doi: 10.1007/s10530-023-03153-w
Albins, M. A., and Hixon, M. A. (2008). Invasive indo-Pacific lionfish Pterois volitans reduce recruitment of Atlantic coral-reef fishes. Mar. Ecol. Prog. Ser. 367, 233–238. doi: 10.3354/meps07620
Albins, M. A., and Hixon, M. A. (2013). Worst case scenario: potential long-term effects of invasive predatory lionfish (Pterois volitans) on Atlantic and Caribbean coral-reef communities. Environ. Biol. Fish 96, 1151–1157. doi: 10.1007/s10641-011-9795-1
Almeida, D., Alcaraz-Hernández, J. D., Cruz, A., Lantero, E., Fletcher, D. H., and García-Berthou, E. (2024). Seasonal effects on health status and parasitological traits of an invasive minnow in Iberian waters. Animals. 14:1502. doi: 10.3390/ani14101502
Amalfitano, S., Coci, M., Corno, G., and Luna, G. M. (2015). A microbial perspective on biological invasions in aquatic ecosystems. Hydrobiologia. 746, 13–22. doi: 10.1007/s10750-014-2002-6
Apprill, A., McNally, S., Parsons, R., and Weber, L. (2015). Minor revision to V4 region SSU rRNA 806R gene primer greatly increases detection of SAR11 bacterioplankton. Aquat. Microb. Ecol. 75, 129–137. doi: 10.3354/ame01753
Arredondo-Chavez, A. T., Sanchez-Jimenez, J. A., Avila-Morales, O. G., Torres-Chavez, P., Herrerias-Diego, Y., Medina-Nava, M., et al. (2016). Spatio-temporal variation in the diet composition of red lionfish, Pterois volitans (Actinopterygii: Scorpaeniformes: Scorpaenidae), in the Mexican Caribbean: insights into the ecological effect of the alien invasion. Acta Ichthyol. Piscat. 46, 182–200. doi: 10.3750/AIP2016.46.3.03
Austin, B., and Austin, D. A. (2016). Bacterial fish pathogens: Disease of farmed and wild fish. Springer, Cham, Switzerland: Springer.
Bagra, K., Bellanger, X., Merlin, C., Singh, G., Berendonk, T. U., and Klümper, U. (2022). Exposure to environmental stress decreases the resistance of river microbial communities towards invasion by antimicrobial resistant bacteria. BioRxiv. doi: 10.1101/2022.11.19.517188
Bang, C., Dagan, T., Deines, P., Dubilier, N., Duschl, W. J., Fraune, S., et al. (2018). Metaorganisms in extreme environments: do microbes play a role in organismal adaptation? Zoology. 127, 1–19. doi: 10.1016/j.zool.2018.02.004
Bariche, M., Kleitou, P., Kalogirou, S., and Bernardi, G. (2017). Genetics reveal the identity and origin of the lionfish invasion in the Mediterranean Sea. Sci. Rep. 7:6782. doi: 10.1038/s41598-017-07326-1
Bariche, M., Torres, M., and Azzurro, E. (2013). The presence of the invasive lionfish Pterois miles in the Mediterranean Sea. Mediterr. Mar. Sci. 14, 292–294. doi: 10.12681/mms.428
Bernardo-Cravo, A. P., Schmeller, D. S., Chatzinotas, A., Vredenburg, V. T., and Loyau, A. (2020). Environmental factors and host microbiomes shape host–pathogen dynamics. Trends Parasitol. 36, 616–633. doi: 10.1016/j.pt.2020.04.010
Bottacini, D., Pollux, B. J., Nijland, R., Jansen, P. A., Naguib, M., and Kotrschal, A. (2024). Lionfish (Pterois miles) in the Mediterranean Sea: a review of the available knowledge with an update on the invasion front. NeoBiota. 92, 233–257. doi: 10.3897/neobiota.92.110442
Bregman, G., Lalzar, M., Livne, L., Bigal, E., Zemah-Shamir, Z., Morick, D., et al. (2023). Preliminary study of shark microbiota at a unique mix-species shark aggregation site, in the Eastern Mediterranean Sea. Front. microbiol. 14:1027804.
Brown, A. M., Huynh, L. Y., Bolender, C. M., Nelson, K. G., and McCutcheon, J. P. (2014). Population genomics of a symbiont in the early stages of a pest invasion. Mol. Ecol. 23, 1516–1530. doi: 10.1111/mec.12366
Casadei, E., Mani, A., Cisco, M., Vågnes, Ø., Salinas, I., Patel, S., et al. (2023). Sex-dependent effects of mechanical delousing on the skin microbiome of broodstock Atlantic salmon (Salmo salar L.). Sci Rep 13:10824.
Cavicchioli, R., Ripple, W. J., Timmis, K. N., Azam, F., Bakken, L. R., Baylis, M., et al. (2019). Scientists’ warning to humanity: microorganisms and climate change. Nat. Rev. Microbiol. 17, 569–586. doi: 10.1038/s41579-019-0222-5
Chaikin, S., Dubiner, S., and Belmaker, J. (2022). Cold-water species deepen to escape warm water temperatures. Glob. Ecol. Biogeogr. 31, 75–88. doi: 10.1111/geb.13414
Chen, L., Hu, C., Lai, N. L. S., Zhang, W., Hua, J., Lam, P. K., et al. (2018). Acute exposure to PBDEs at an environmentally realistic concentration causes abrupt changes in the gut microbiota and host health of zebrafish. Environ. Pollut. 240, 17–26.
Coats, V. C., and Rumpho, M. E. (2014). The rhizosphere microbiota of plant invaders: an overview of recent advances in the microbiomics of invasive plants. Front. Microbiol. 5:368. doi: 10.3389/fmicb.2014.00368
Costello, K. E., Lynch, S. A., McAllen, R., O'Riordan, R. M., and Culloty, S. C. (2022). Assessing the potential for invasive species introductions and secondary spread using vessel movements in maritime ports. Mar. Pollut. Bull. 177:113496. doi: 10.1016/j.marpolbul.2022.113496
Côté, I. M., Green, S. J., and Hixon, M. A. (2013). Predatory fish invaders: insights from indo-Pacific lionfish in the western Atlantic and Caribbean. Biol. Conserv. 164, 50–61. doi: 10.1016/j.biocon.2013.04.014
Côté, I. M., and Smith, N. S. (2018). The lionfish Pterois sp. invasion: has the worst-case scenario come to pass? J. Fish Biol. 92, 660–689. doi: 10.1111/jfb.13544
Coutinho, F. H., Meirelles, P. M., Moreira, A. P. B., Paranhos, R. P., Dutilh, B. E., and Thompson, F. L. (2015). Niche distribution and influence of environmental parameters in marine microbial communities: a systematic review. PeerJ. 3:e1008. doi: 10.7717/peerj.1008
Dahl, K. A., and Patterson, W. F. III. (2014). Habitat-specific density and diet of rapidly expanding invasive red lionfish, Pterois volitans, populations in the northern Gulf of Mexico. PLoS One. 9:e105852. doi: 10.1371/journal.pone.0105852
Dimitriou, A. C., Chartosia, N., Hall-Spencer, J. M., Kleitou, P., Jimenez, C., Antoniou, C., et al. (2019). Genetic data suggest multiple introductions of the lionfish (Pterois miles) into the Mediterranean Sea. Diversity. 11:149. doi: 10.3390/d11090149
Eddy, C., Pitt, J., Morris, J. A. Jr., Smith, S., Goodbody-Gringley, G., and Bernal, D. (2016). Diet of invasive lionfish (Pterois volitans and P. miles) in Bermuda. Mar. Ecol. Prog. Ser. 558, 193–206. doi: 10.3354/meps11838
Ferreira, C. E., Luiz, O. J., Floeter, S. R., Lucena, M. B., Barbosa, M. C., Rocha, C. R., et al. (2015). First record of invasive lionfish (Pterois volitans) for the Brazilian coast. PLoS One. 10:e0123002. doi: 10.1371/journal.pone.0123002
Galil, B. S., Boero, F., Campbell, M. L., Carlton, J. T., Cook, E., Fraschetti, S., et al. (2015). ‘Double trouble’: the expansion of the Suez Canal and marine bioinvasions in the Mediterranean Sea. Biol. Invasions. 17, 973–976. doi: 10.1007/s10530-014-0778-y
Golani, D. (1993). Trophic adaptation of Red Sea fishes to the eastern Mediterranean environment - a review and new data. Isr. J. Zool. 39, 391–402.
Golani, D., and Sonin, O. (1992). New records of the Red Sea fishes, Pterois miles (Scorpaenidae) and Pteragogus pelycus (Labridae) from the eastern Mediterranean Sea. Japanese J. Ichthyol. 39, 167–169. doi: 10.1007/BF02906001
Green, S. J., Akins, J. L., Maljković, A., and Côté, I. M. (2012). Invasive lionfish drive Atlantic coral reef fish declines. PLoS One. 7:e32596. doi: 10.1371/journal.pone.0032596
Haas, G., and Steinitz, H. (1947). Erythrean fishes on the Mediterranean coast of Palestine. Nature. 160:28. doi: 10.1038/160028b0
Hicks, N., Liu, X., Gregory, R., Kenny, J., Lucaci, A., Lenzi, L., et al. (2018). Temperature driven changes in benthic bacterial diversity influences biogeochemical cycling in coastal sediments. Front. Microbiol. 9:1730. doi: 10.3389/fmicb.2018.01730
Himler, A. G., Adachi-Hagimori, T., Bergen, J. E., Kozuch, A., Kelly, S. E., Tabashnik, B. E., et al. (2011). Rapid spread of a bacterial symbiont in an invasive whitefly is driven by fitness benefits and female bias. Science 332, 254–256. doi: 10.1126/science.1199410
Huang, J., Zhang, Y., and Yan, S. (2019). Microbial plasticity and the invasion success of non-native species. Microbiome 13:34. doi: 10.1186/s40168-025-02030-z
Itoi, S., Okamura, T., Koyama, Y., and Sugita, H. (2006). Chitinolytic bacteria in the intestinal tract of Japanese coastal fishes. Can. J. Microbiol. 52, 1158–1163. doi: 10.1139/w06-082
Jones, L. A., and Ricciardi, A. (2014). The influence of pre-settlement and early post-settlement processes on the adult distribution and relative dominance of two invasive mussel species. Freshw. Biol. 59, 1086–1100. doi: 10.1111/fwb.12331
Kramp, R. D., Kohl, K. D., and Stephenson, J. F. (2022). Skin bacterial microbiome diversity predicts lower activity levels in female, but not male, guppies. Poecilia reticulata. Biol. Lett. 18:20220167.
Kletou, D., Hall-Spencer, J. M., and Kleitou, P. (2016). A lionfish (Pterois miles) invasion has begun in the Mediterranean Sea. Mar. Biodiver. Rec. 9, 1–7. doi: 10.1186/s41200-016-0065-y
Kolar, C. S., and Lodge, D. M. (2001). Progress in invasion biology: predicting invaders. Trends Ecol. Evol. 16, 199–204. doi: 10.1016/S0169-5347(01)02101-2
Kuang, T., He, A., Lin, Y., Huang, X., Liu, L., and Zhou, L. (2020). Comparative analysis of microbial communities associated with the gill, gut, and habitat of two filter-feeding fish. Aquacult. Rep. 18:100501. doi: 10.1016/j.aqrep.2020.100501
Labella, A. M., Arahal, D. R., Castro, D., Lemos, M. L., and Borrego, J. J. (2017). Revisiting the genus Photobacterium: taxonomy, ecology and pathogenesis. Int. Microbiol. 20, 1–10. doi: 10.2436/20.1501.01.280
Lavoie, D. M., Smith, L. D., and Ruiz, G. M. (1999). The potential for intracoastal transfer of non-indigenous species in the ballast water of ships. Estuar. Coast. Shelf Sci. 48, 551–564. doi: 10.1006/ecss.1999.0467
Lazarus, M., Frid-Landau, O., and Yahel, R. (2022). Marine BioBlitz- biological survey of the Israelis MPAs in the Mediterranean Sea-summary report for 2015, 2017, 2019, 2021 - chapter B- composition of fish community. Jerusalem: Israel Nature and Parks Authority (in Hebrew), 90.
Lee, R. W., Robinson, J. J., and Cavanaugh, C. M. (1999). Pathways of inorganic nitrogen assimilation in chemoautotrophic bacteria–marine invertebrate symbioses: expression of host and symbiont glutamine synthetase. J. Exp. Biol. 202, 289–300. doi: 10.1242/jeb.202.3.289
Li, H., Lu, L., Chen, R., Li, S., and Xu, D. (2022). Exploring sexual dimorphism in the intestinal microbiota of the yellow drum (Nibea albiflora, Sciaenidae). Front Microbiol. 12:808285.
Liu, M., Bjørnlund, L., Rønn, R., Christensen, S., and Ekelund, F. (2012). Disturbance promotes non-indigenous bacterial invasion in soil microcosms: analysis of the roles of resource availability and community structure. PLoS One 7:e45306. doi: 10.1371/journal.pone.0045306
Lu, M., Hulcr, J., and Sun, J. (2016). The role of symbiotic microbes in insect invasions. Annu. Rev. Ecol. Evol. Syst. 47, 487–505. doi: 10.1146/annurev-ecolsys-121415-032050
Martignoni, M. M., and Kolodny, O. (2023). Microbiome transfer from native to invasive species may increase invasion risk and shorten invasion lag. bioRxiv. doi: 10.1101/2023.08.28.555072
Martyniuk, C. J., Buerger, A. N., Vespalcova, H., Rudzanova, B., Sohag, S. R., Hanlon, A. T., et al. (2022). Sex-dependent host-microbiome dynamics in zebrafish: Implications for toxicology and gastrointestinal physiology. Comp. Biochem. Physiol. Part D Genomics Proteomics. 42:100993.
Meron, D., Davidovich, N., Ofek-Lalzar, M., Berzak, R., Scheinin, A., Regev, Y., et al. (2020). Specific pathogens and microbial abundance within liver and kidney tissues of wild marine fish from the eastern Mediterranean Sea. Microb. Biotechnol. 13, 770–780. doi: 10.1111/1751-7915.13537
Moi, I. M., Roslan, N. N., Leow, A. T. C., Ali, M. S. M., Rahman, R. N. Z. R. A., Rahimpour, A., et al. (2017). The biology and the importance of Photobacterium species. Appl. Microbiol. Biotechnol. 101, 4371–4385. doi: 10.1007/s00253-017-8300-y
Moonsamy, P. V., Williams, T., Bonella, P., Holcomb, C. L., Höglund, B. N., Hillman, G., et al. (2013). High throughput HLA genotyping using 454 sequencing and the Fluidigm access Array™ system for simplified amplicon library preparation. Tissue Antigens. 81, 141–149. doi: 10.1111/tan.12071
Morris, J. A. Jr, Thomas, A., Rhyne, A. L., Breen, N., Akins, L., and Nash, B. (2011). Nutritional properties of the invasive lionfish: A delicious and nutritious approach for controlling the invasion. AACL Bioflux. 4, 21–26.
Mouchlianitis, F. A., Kalaitzi, G., Kleitou, P., Savva, I., Kletou, D., and Ganias, K. (2022). Reproductive dynamics of the invasive lionfish (Pterois miles) in the eastern Mediterranean Sea. J. Fish Biol. 100, 574–581. doi: 10.1111/jfb.14971
Naqib, A., Poggi, S., Wang, W., Hyde, M., Kunstman, K., and Green, S. J. (2018). “Making and sequencing heavily multiplexed, high-throughput 16S ribosomal RNA gene amplicon libraries using a flexible, two-stage PCR protocol” in Gene expression analysis: methods and protocols. eds. N. Raghavachari and N. Garcia-Reyero (New York, NY: Springer New York), 149–169.
Paerl, H. W., Gardner, W. S., Havens, K. E., Joyner, A. R., McCarthy, M. J., Newell, S. E., et al. (2016). Mitigating cyanobacterial harmful algal blooms in aquatic ecosystems impacted by climate change and anthropogenic nutrients. Harmful Algae. 54, 213–222. doi: 10.1016/j.hal.2015.09.009
Parada, A. E., Needham, D. M., and Fuhrman, J. A. (2016). Every base matters: assessing small subunit rRNA primers for marine microbiomes with mock communities, time series and global field samples. Environ. Microbiol. 18, 1403–1414. doi: 10.1111/1462-2920.13023
Piazzon, M. C., Naya-Català, F., Simó-Mirabet, P., Picard-Sánchez, A., Roig, F. J., Calduch-Giner, J. A., et al. (2019). Sex, age, and bacteria: how the intestinal microbiota is modulated in a protandrous hermaphrodite fish. Front. microbiol. 10:2512.
Pinardi, N., and Masetti, E. (2000). Variability of the large scale general circulation of the Mediterranean Sea from observations and modelling: a review. Palaeogeography, Palaeoclimatology, Palaeoecology, 158, 153–173.
Rivas, A. J., Lemos, M. L., and Osorio, C. R. (2013). Photobacterium damselae subsp. damselae, a bacterium pathogenic for marine animals and humans. Front. Microbiol. 4:283. doi: 10.3389/fmicb.2013.00283
Ronanki, J. (2024). Metabolic adaptations in the gut microbiome of invasive marine fish species. NATURALISTA CAMPANO. 28, 3108–3118.
Roy, K., Jablonski, D., and Valentine, J. W. (2002). Body size and invasion success in marine bivalves. Ecol. Lett. 5, 163–167. doi: 10.1046/j.1461-0248.2002.00316.x
Savva, I., Chartosia, N., Antoniou, C., Kleitou, P., Georgiou, A., Stern, N., et al. (2020). They are here to stay: the biology and ecology of lionfish (Pterois miles) in the Mediterranean Sea. J. Fish Biol. 97, 148–162.
Schofield, P. J. (2009). Geographic extent and chronology of the invasion of non-native lionfish (Pterois volitans and P. miles) in the Western North Atlantic and Caribbean Sea. Aquat. Invasions. 4, 473–479. doi: 10.3391/ai.2009.4.3.5
Schröder, A., Nilsson, K. A., Persson, L., Van Kooten, T., and Reichstein, B. (2009). Invasion success depends on invader body size in a size-structured mixed predation–competition community. J. Anim. Ecol. 78, 1152–1162. doi: 10.1111/j.1365-2656.2009.01590.x
Soares, M. O., Feitosa, C. V., Garcia, T. M., Cottens, K. F., Vinicius, B., Paiva, S. V., et al. (2022). Lionfish on the loose: Pterois invade shallow habitats in the tropical southwestern Atlantic. Front. mar. sci. 9:956848.
Soares, M. O., Pereira, P. H., Feitosa, C. V., Maggioni, R., Rocha, R. S., Bezerra, L. E. A., et al. (2023). Lessons from the invasion front: Integration of research and management of the lionfish invasion in Brazil. J Environ Manage. 340:117954.
Steell, S. C., Van Leeuwen, T. E., Brownscombe, J. W., Cooke, S. J., and Eliason, E. J. (2019). An appetite for invasion: digestive physiology, thermal performance and food intake in lionfish (Pterois spp.). J. Exp. Biol. 222:jeb209437. doi: 10.1242/jeb.209437
Steinitz, W. (1927). Beiträge zur Kenntnis der Küstenfauna Palästinas, Erster Teil. Pubbl. Stn. Zool. Napoli. 8, 311–353.
Stern, N., Jimenez, C., Huseyinoglu, M. F., Andreou, V., Hadjioannou, L., Petrou, A., et al. (2019). Constructing the genetic population demography of the invasive lionfish Pterois miles in the Levant Basin, Eastern Mediterranean. Mitochondrial DNA Part A. 30, 249–255. doi: 10.1080/24701394.2018.1482284
Stern, N., Rothman, S. B., Hüseyinoglu, M. F., and Öztürk, B. (2018). “Iron lion Zion: the successful, albeit lingered, invasion of the lionfish in the Israeli Mediterranean Sea” in Lionfish invasion and its Management in the Mediterranean Sea. Turkish marine research foundation (TUDAV) publication Istanbul, Turkey. vol. 49, 51–56.
Stevens, J. L., Jackson, R. L., and Olson, J. B. (2016). Bacteria associated with lionfish (Pterois volitans/miles complex) exhibit antibacterial activity against known fish pathogens. Mar. Ecol. Prog. Ser. 558, 167–180. doi: 10.3354/meps11789
Stevens, J. L., and Olson, J. B. (2013). Invasive lionfish harbor a different external bacterial community than native Bahamian fishes. Coral Reefs. 32, 1113–1121. doi: 10.1007/s00338-013-1072-7
Stevens, J. L., and Olson, J. B. (2015). Bacterial communities associated with lionfish in their native and invaded ranges. Mar. Ecol. Prog. Ser. 531, 253–262. doi: 10.3354/meps11323
Takizawa, Y., and Chikaraishi, Y. (2024). Trophic isotopic discrimination of amino acids (glutamic acid and phenylalanine) in the leopard gecko Eublepharis macularius: a report based on a controlled feeding experiment. Res. Org. Geochem. 40, 1–7. doi: 10.20612/rog.40.1_1
Thompson, L. R., Williams, G. J., Haroon, M. F., Shibl, A., Larsen, P., Shorenstein, J., et al. (2017). Metagenomic covariation along densely sampled environmental gradients in the Red Sea. ISME J. 11, 138–151. doi: 10.1038/ismej.2016.99
Tsadok, R., Zemah-Shamir, Z., Shemesh, E., Martinez, S., Ramon, D., Kolski, I., et al. (2023). Dietary habits change of Lessepsian migrants’ fish from the Red Sea to the eastern Mediterranean Sea. Aquat. Invasions. 18, 521–531. doi: 10.3391/ai.2023.18.4.113532
Urbanczyk, H., Ast, J. C., and Dunlap, P. V. (2011). Phylogeny, genomics, and symbiosis of Photobacterium. FEMS Microbiol. Rev. 35, 324–342. doi: 10.1111/j.1574-6976.2010.00250.x
Vilcinskas, A., Stoecker, K., Schmidtberg, H., Röhrich, C. R., and Vogel, H. (2013). Invasive harlequin ladybird carries biological weapons against native competitors. Science. 340, 862–863. doi: 10.1126/science.1234032
Williams, R. J., Griffiths, F. B., Van der Wal, E. J., and Kelly, J. (1988). Cargo vessel ballast water as a vector for the transport of non-indigenous marine species. Estuar. Coast. Shelf Sci. 26, 409–420. doi: 10.1016/0272-7714(88)90021-2
Zarubin, M., Belkin, S., Ionescu, M., and Genin, A. (2012). Bacterial bioluminescence as a lure for marine zooplankton and fish. Proc. Natl. Acad. Sci. 109, 853–857. doi: 10.1073/pnas.1116683109
Zirler, R., Leck, L. A., Farkash, T. F., Holzknecht, M., Kroh, A., Gerovasileiou, V., et al. (2023). Gaining a (tube) foothold–trends and status following two decades of the long-spined echinoid Diadema setosum (Leske, 1778) invasion to the Mediterranean Sea. Front. Mar. Sci. 10:1152584. doi: 10.3389/fmars.2023.1152584
Keywords: microbiota, lionfish, bacterial profile, Mediterranean Sea, Red Sea, invasion, establishment, Photobacterium
Citation: Meron D, Lalzar M, Rothman SB-S, Kroin Y, Kaufman E, Kitson-Walters K, Zvi-Kedem T, Shemesh E, Tsadok R, Nativ H, Einbinder S and Tchernov D (2025) Microbiota dynamics in lionfish (Pterois): insights into invasion and establishment in the Mediterranean Sea. Front. Microbiol. 16:1570274. doi: 10.3389/fmicb.2025.1570274
Received: 03 February 2025; Accepted: 11 March 2025;
Published: 02 April 2025.
Edited by:
Jiajia Ni, Guangzhou National Laboratory, ChinaReviewed by:
Chun Wang, Beijing Technology and Business University, ChinaCopyright © 2025 Meron, Lalzar, Rothman, Kroin, Kaufman, Kitson-Walters, Zvi-Kedem, Shemesh, Tsadok, Nativ, Einbinder and Tchernov. This is an open-access article distributed under the terms of the Creative Commons Attribution License (CC BY). The use, distribution or reproduction in other forums is permitted, provided the original author(s) and the copyright owner(s) are credited and that the original publication in this journal is cited, in accordance with accepted academic practice. No use, distribution or reproduction is permitted which does not comply with these terms.
*Correspondence: Dalit Meron, ZG1lcm9uQHVuaXYuaGFpZmEuYWMuaWw=
Disclaimer: All claims expressed in this article are solely those of the authors and do not necessarily represent those of their affiliated organizations, or those of the publisher, the editors and the reviewers. Any product that may be evaluated in this article or claim that may be made by its manufacturer is not guaranteed or endorsed by the publisher.
Research integrity at Frontiers
Learn more about the work of our research integrity team to safeguard the quality of each article we publish.