- 1Geomicrobiology, Marine Geosystems, GEOMAR Helmholtz Centre for Ocean Research Kiel, Kiel, Germany
- 2Benthic Biogeochemistry, Marine Geosystems, GEOMAR Helmholtz Centre for Ocean Research Kiel, Kiel, Germany
The majority of the organic matter (OM) degradation on the seafloor occurs in coastal regions. Since oxygen (O2) becomes quickly depleted in the top sediments, most of the OM decomposition is driven by microbial sulfate reduction (SR) and fermentation, the latter generating molecular hydrogen (H2). If the H2 is not consumed by hydrogenotrophic microorganisms and accumulates in the sedimentary porewaters, OM degradation is hindered. Despite the importance of H2 scavenging microorganisms for OM mineralization, the knowledge on H2 oxidizers and their constraints in coastal marine sediments is still quite limited. Here we investigated the role of H2 oxidizers in top (2 to 5 cm, suboxic-sulfidic) and bottom (18 to 22 cm, sulfidic) coastal sediments from a location exposed to seasonal hypoxia in the SW Baltic Sea. We used sediments from April, May and August, representative of different seasons. We spiked respective sediment slurries with H2 and incubated them for up to 4 weeks under O2-free conditions. H2 consumption potential, methane production and shifts in bacterial and archaeal 16S rRNA gene amplicons (generated from RNA) were assessed over time. The seasonal variations in sedimentary community compositions and pore water geochemistry already gave distinct starting conditions for the H2 enrichments. Sediments exposed to near anoxic bottom water conditions favored a microbial starter community exhibiting the highest H2 oxidation potential. Most of the observed H2 oxidation potential appeared associated with hydrogenotrophic sulfate reducers. The putative involvement of massively enriched ANME in H2 cycling in May 18 to 22 cm sediment horizons is conspicuous. While the differences in the observed H2 oxidation potentials in the studied sediment slurries are likely related to the (season-depending) overall redox state of the sediments and interstitial waters, the influence of microbial interconnections could not be fully resolved and evaluated, demonstrating the need for further consumption- and community-based studies.
Introduction
With 70% of our planet’s surface being covered by marine sediments, they represent the largest reservoir of organic carbon on Earth and play a pivotal role for global carbon cycling (Middelburg et al., 1993). Although coastal sediments cover a relatively small area of the seafloor (9%), they account for most of the organic carbon decomposition (87%) (Middelburg et al., 1993). Most of the organic matter (OM) degradation in organic-rich marine sediments is driven by fermentation and microbial sulfate reduction (SR), as oxygen (O2) becomes quickly depleted in the top sediments (Jørgensen, 1982).
Fermentation of OM is the central pathway of generating molecular hydrogen (H2) in sediments (Laanbroek and Veldkamp, 1982; Dolfing, 1988). Accumulation of H2 already in the nM-range renders fermentation thermodynamically unfeasible; thus, fermentative OM degradation becomes limited or even stops if H2 is not scavenged rapidly by hydrogenotrophic microorganisms (Wolin, 1976; Hallenbeck, 2009). The control of in situ H2 concentrations occurs through syntrophic interactions between hydrogenogenic and hydrogenotrophic microbial processes, which generally rely on short-range interactions between individual microbial organisms in close spatial proximity to each other. In accordance with the thermodynamic constraints of syntrophic metabolisms and interactions, H2 turnover is fast and its residence time is very short. Consequently, measured H2 concentrations in sediment porewaters are commonly quite low (typically <60 nM) (Novelli et al., 1987; Novelli et al., 1988; Hoehler et al., 1994, 1998; Lin et al., 2012). Due to the broad spectrum of hydrogenotrophic microorganisms in O2-free sediments, including nitrate-, manganese-, sulfate-, and iron-reducers as well as methanogens, a huge variety and variability of syntrophic interactions exists (Lovley and Goodwin, 1988; Hoehler et al., 1998; Vandieken et al., 2014).
Consequently, phylogenetically and functionally diverse microbes are capable of utilizing H2 as energy source (Greening et al., 2016; Adam and Perner, 2018a; Lappan et al., 2023) and an even larger enzymatic repertoire is likely masked among the uncultured microbial majority (Adam and Perner, 2018b). In anoxic sediments, common H2 utilizers include sulfate reducing Bacteria (SRB), producing toxic hydrogen sulfide, hydrogenotrophic methanogens, generating the greenhouse gas methane, and homoacetogens producing acetate (Lovley et al., 1982; Hoehler et al., 1999; Ragsdale and Pierce, 2008). Given that SR is thermodynamically more favorable than both hydrogenotrophic methanogenesis and homoacetogenesis, SRB have been documented to outcompete hydrogenotrophic methanogens and, respectively, homoacetogens when H2 levels fall below a particular threshold concentration (Oremland and Polcin, 1982; Lin et al., 2012). In contrast, at high H2 concentrations, as it is often imposed in physiological experiments, simultaneous occurrence of several H2-scavenging processes is possible (Holmer and Kristensen, 1994; Lay et al., 1998; Timmers et al., 2015). Conclusively, the competition for H2 determines the flow of carbon and electrons and the degree at which hydrogen sulfide, methane and/or acetate is generated, impacting the sediment ecosystem and beyond. Despite the profound role that H2 has for OM break down and the type of generated products, the knowledge on the ecophysiology of H2 converting microbes in these sediments is still quite limited.
So far only a limited number of studies have attempted to disentangle the H2 oxidation potential, associated microbial communities and dynamic response to changing environmental conditions in marine anoxic sediments (Hamann et al., 2016; Dyksma et al., 2018). This is true in particular for coastal sediments, which are usually exposed to seasonal variations with respect to bottom water temperature and chemistry. Given environmental dynamics, steady-state conditions - assumed to underly syntrophic interactions in anoxic sediments can most likely not be established, thus a stable H2-metabolic community is not to be expected. The Boknis Eck long-term study site, in the SW Baltic Sea is an ideal natural laboratory to test seasonal dynamics in coastal marine sediments. It is located at 28 m water depth, sediments are rich in OM (~5% wt. %) (Dale et al., 2013; Wallmann et al., 2022) and are exposed to late summer seasonal hypoxia (O2 < 63 μM) /anoxia (no O2) (Dale et al., 2013; Perner et al., 2022). Peak sulfate reduction rates (SRR) in summer are considerably higher and at the surface as opposed to SRR in winter and early spring where they are 10-fold lower and maxima are reached below the first centimeters (Dale et al., 2013; Perner et al., 2022). Experiments targeting methanogenic processes in these sediments have suggested that SRB and methanogens are both active in the sulfate reducing zone, but that methanogens are using H2 alternative substrates such as methylated compounds or methanol (Maltby et al., 2018). Anaerobic oxidation of methane (AOM) and anaerobic methane oxidizing Archaea (ANME) have been documented down to depths of 25 cm at Boknis Eck (Treude et al., 2005; Perner et al., 2022).
In this study, we aimed to elucidate the H2-oxidizing potential and microbial growth in the presence of sub-millimolar H2 concentrations. For that, we designed an experimental setup where we offered H2 in excess (2% in headspace or ~ 1.2 μM in solution) to marine sediments and kept them under anoxic conditions. Coastal sediments at Boknis Eck (SW Baltic Sea) exposed to seasonal hypoxia were used for incubation experiments. Sediments were collected in April where bottom waters were fully oxic, in May where bottom waters started to become O2 depleted and in August, where bottom waters were near anoxia. H2 consumption rates and microbial shifts in the sediment slurries were monitored under anoxic conditions for up to 4 weeks to address the full H2 oxidizing potential when H2 is available in excess relative to in situ conditions.
Materials and methods
Sample collection
Sediment samples were collected in April, May and August 2022 in close proximity to the time series station Boknis Eck in the Eckernförde Bay (South-West Baltic Sea, Germany; see Figure 1a) (54°31.76’ N, 10°02.50′ E). Sediment cores (Figure 1b) were taken using a Minicorer (MIC) device, transported to land within 3 h of sample retrieval and kept at 4°C until the subsampling for setting up hydrogen incubation experiments was performed (max. 24 h after core retrieval). Pore water extractions were performed from neighboring sediment cores at the respective sampling days (profiles of selected biogeochemical parameters can be found in Figure 1c).
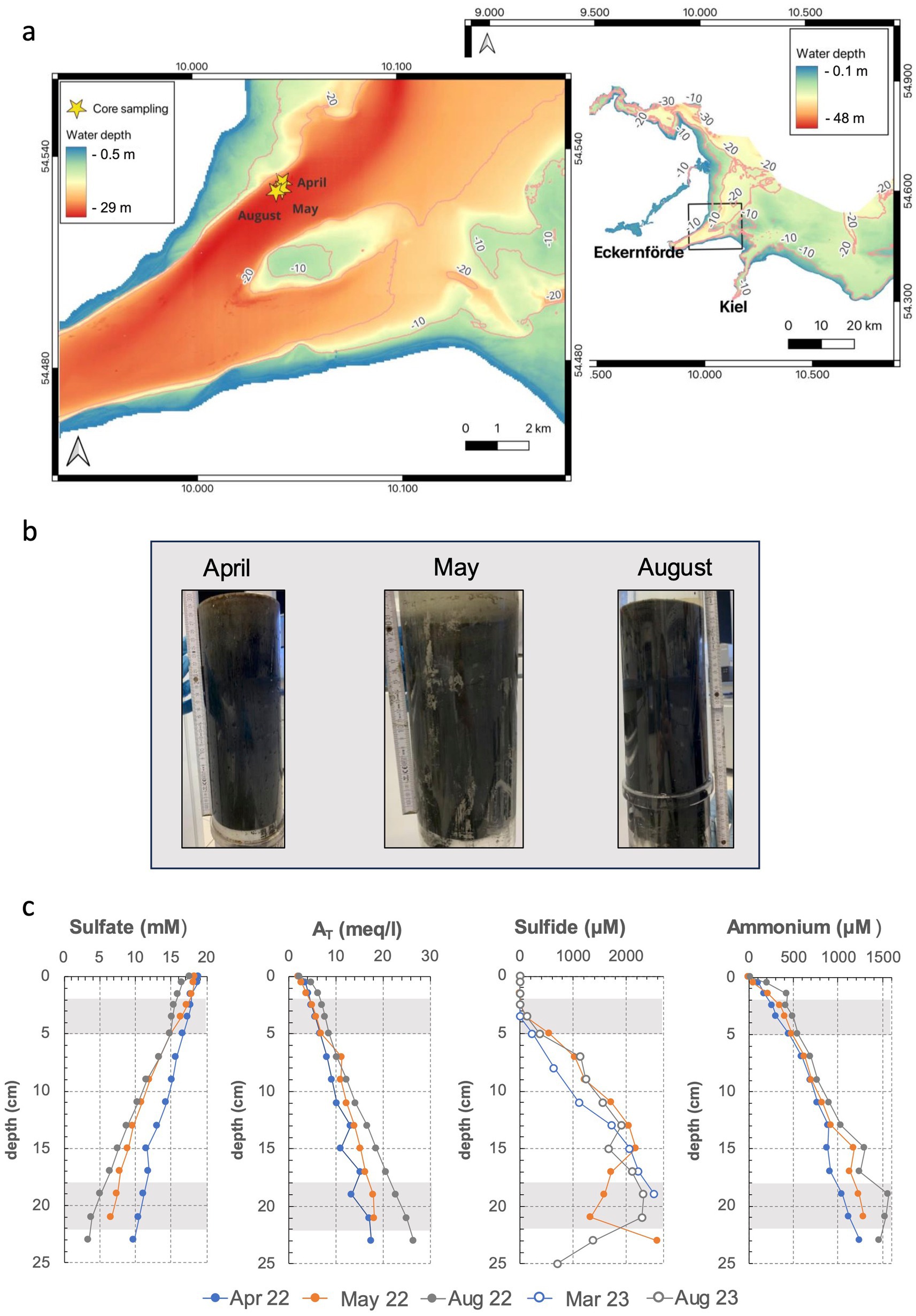
Figure 1. Map of the sampling area in the South-Western Baltic Sea (a), sampled sediment cores (b) and porewater profiles of sulfate, total alkalinity (AT), sulfide and ammonium (c). Geochemical data is derived from (Dale et al., 2024), except for May 2022 and August 2023 (M. Perner, unpublished data). For April and August 2022, no sulfide data are available.
Hydrogen incubation experiments
For hydrogen consumption measurements sediment cores were subsampled in 2–5 cm and 18–22 cm horizons and mixed to obtain a homogenous dispersion of the sediment communities in each subsample. For later analyses of the microbial communities, aliquots of the subsamples were immediately frozen at −70°C. Under anaerobic conditions (5:95 H2:N2 atmosphere) aliquots of 1 mL of the subsampled sediments were distributed into 60 mL serum bottles filled with 10 mL of a modified, organic-free artificial seawater (MJ) medium (Sako et al., 1996; Hansen and Perner, 2015). Negative controls were set up by adding formaldehyde to a final concentration of 4%. Each sample and control slurry was set up in triplicates and purged with a gas mixture (2:20:78 H2:CO2:He) to obtain a H2 concentration of 2% in the head space. H2 consumption was monitored by weekly measurements of the H2 concentrations in the head space as previously described (Hansen and Perner, 2015; Adam et al., 2021), over a time period of up to 26 days. All measurements were performed using a TRACE GC Ultra gas chromatograph (ThermoFisherScientific, Waltham, MA, United States), equipped with a ShinCarbon ST 100/120 column (Restek Corporation, Bellefonte, PA, United States) and a Pulsed Discharge Detector (Vici Valco Instruments, Houston, TX, United States) with a detection limit of 10 ppb. Head space samples of the sediment incubations were diluted 1:124 for measuring in the calibrated range of up to 191 ppm H2 (RMSE 1.46 ppm). The methane concentration in the head space was calculated from the same chromatograms used for H2 measurements but using a separate calibration (RMSE 20.11 ppm in the range of 597–1792 ppm) and manual integration of the methane peak. During the incubation period, all samples and controls were kept at the respective in situ bottom water temperature measured at the date of the sediment core retrieval: 4°C for April, 5°C for May and 10.5°C for August incubations.
At the beginning and termination of the incubation experiments 1 mL subsamples of each sediment slurry (incl. controls) were taken and fixed with formaldehyde (4% end concentration) at 4°C overnight. After harvesting (5 min, 5,000 g, 4°C) and two wash steps with 1 mL of 1x Phosphate Buffered Saline solution (PBS), the fixed cells were stored in 1 mL of PBS:Ethanol (1:1) at −20°C. Subsequent DAPI-based cell enumerations were performed as described in (Perner et al., 2022) and used to calculate hydrogen consumption rates as previously described (Hansen and Perner, 2015). For taxonomic analyses of the respective microbial communities at the end of the incubations, sediment slurries (excl. controls) were harvested at 20,000 g (4°C) for 30 min, washed with 10 mL of 1xPBS and frozen at −70°C.
Nucleotide extractions, 16S rRNA and mcr gene analyses
RNA and DNA extractions of the original sediment subsamples (t0) and the harvested slurries were performed using the NucleoBond RNA Soil Mini Kit with the additional DNA set according to the manufacturer’s instructions (Macherey-Nagel, Düren, Germany). Residual DNA was removed from the extracted RNA by means of the RapidOut DNA Removal Kit (ThermoFisher Scientific) and cDNA synthesized with the SuperScript™ VILO™ cDNA synthesis kit (ThermoFisher Scientific) following the manufacturer’s protocols. Purification of the cDNA was performed using the clean and concentrator-5 cleanup kit (Zymo Research, Irvine, CA, United States) according to the cDNA protocol. The V3-V4 regions of bacterial 16S rRNA genes and V4-V5 regions of archaeal 16S rRNA genes were amplified from the purified cDNA and prepared for Illumina MiSeq sequencing using the Bact_341F/805R, Arch_519F/915R and Arch 524F/958R primer pairs as previously described (Adam et al., 2019). Equimolar pools of purified amplicons were sequenced in a 2×300 paired-end sequencing run on Illumina’s Mi-Seq platform.
Demultiplexed raw sequences were processed in the Qiime2 environment (Bolyen et al., 2019) following a previously reported workflow (Adam-Beyer et al., 2023). Prior to merging with the dada2-plugin (Callahan et al., 2016), primer sequences were removed and the individual raw sequences were trimmed to 260 nucleotides, each. A pretrained classifier based on SILVA database release 138 (Quast et al., 2013) and the feature-classifier plugin (Bokulich et al., 2018) were used for taxonomic assignments. After removal of contaminating sequences (i.e., eukaryotic and chloroplast sequences as well as bacterial sequences in the archaeal data set and vice versa), phylogenetic trees were calculated using the “align-to-tree-mafft-fasttree” pipeline (Price et al., 2010). Relative abundance calculations, generation of tax plots and differential abundance analyses were performed using the microeco package (Liu et al., 2021) in R (version 4.3.1; https://www.r-project.org/). For differential abundance calculations, the random forest and differential test approach was applied after a rarefication to 9,500 sequences/sample for bacterial and 7,000 sequences/sample for archaeal analyses.
Due to the conspicuously high enrichments of ANME 16S tags present only in the 18 to 22 cm sediment incubations from May and their ambiguous involvement in methane cycling in the corresponding samples, genes encoding for the methyl coenzyme M reductases (Mcr, key enzyme for methanogenesis and methanotrophy) of methanogens, ANME-1 and ANME-2 were PCR-amplified from DNA extractions of the May 18–22 cm samples. Primer pairs used for the amplification were mcrI (Springer et al., 1995) for mcr genes of hydrogenotrophic methanogens, ANME-1-mcrI for ANME-1 related mcr genes and mcrANME-2 for ANME-2 related mcr genes (Lever and Teske, 2015). Amplification was performed using the Phusion High-Fidelity PCR-Kit (Thermo Scientific) with the following conditions: initial denaturation for 30 s (98°C), followed by 31 cycles of denaturation (10 s at 98°C), annealing for 15 s (46°C for mcrI, 57°C for ANME-1-mcrI and mcr-ANME-2) and extension (15 s at 72°C). Final extension was carried out for (5 min at 72°C). PCR products were checked for the correct length via agarose gel electrophoresis: 470 bp for mcrI, 480 bp for ANME-1-mcrI and 155 bp for mcr-ANME-2. After purification with the clean and concentrator-5 purification kit (Zymo Research), PCR products were cloned into E. coli DH5 α cells using the pGEM-T vector system (Promega, Madison, WI, United States) according to the manufacturer’s instructions. Colony-PCRs of 20 white colonies each were performed for ANME-1-mcrI and mcr-ANME-2 clones using common M13 primers and the Phusion High-Fidelity PCR Kit as detailed above, with the exception of an annealing temperature of 58°C. Purified PCR products were sent for Sanger sequencing with the M13 forward primer at Eurofins Genomics (Cologne, Germany). Vector contaminations were removed and the mcr-sequences were aligned with reference mcr sequences using Bioedit (Hall, 1999). A phylogenetic tree was constructed with the MEGA X software using the maximum-likelihood method with a Tamura-Nei model and bootstrapping with 1,000 replicates (Kumar et al., 2018).
CARD-FISH and cell counts
For all sediment slurries cell numbers were determined by DAPI staining as described above. In order to monitor if the increase in ANME-related 16S rRNA gene tags coincides with an overall increase of archaeal cell abundances, for the 18 to 22 cm sediments from May CARD-FISH (catalyzed reporter deposition-fluorescence in situ hybridization) was additionally performed using the EUB I and EUB II as well as the Arch915 probes for Bacteria (EUB I and II) and Archaea (Arch915), respectively (Pernthaler et al., 2002). Preparation of filters, permeabilization and hybridization were performed as previously described for sediment samples from Boknis Eck (Perner et al., 2022). Relative abundances of bacterial and archaeal cells were determined by comparing enumerations of hybridized cells against DAPI-stained cells.
Data availability
Raw reads of 16S rRNA gene amplicons and mcr genes were deposited at the National Center for Biotechnology Information (NCBI) and can be accessed under BioProject PRJNA1213115.
Results
In order to assess the H2 consumption potential and shifts in H2-metabolizing organisms in seasonally hypoxic sediments from the SW Baltic Sea, we monitored microbial H2 utilization and methane cycling in H2-spiked sediment slurries. Samples were taken from 2 sediment horizons (near top and bottom of MIC cores, i.e., 2–5 cm and 18–22 cm, respectively) of three different months (April, May and August), reflecting the seasonality. Additionally, cell numbers were determined and RNA extracted to generate 16S rRNA genes aiming to link H2 consumption with community shifts in the incubation experiments. For the May 18 to 22 cm sediment slurries, the presence and diversity of mcr genes (encoding for methyl coenzyme M reductase) were additionally determined based on DNA, as well as quantitative shifts of Bacteria relative to Archaea documented via CARD-FISH. These analyses were limited to the May bottom slurries, as only these exhibited a massive enrichment in ANME-related 16S rRNA gene tags.
Hydrogen consumption rates and monitored methane concentrations in sediment slurries
Hydrogen consumption
In the head space of the sediment slurries using top sediments (2 to 5 cm) from May and August and bottom sediments (18 to 22 cm) from August, on average nearly all of the offered H2 (i.e., 35–40 μmol equivalent to ~2% in headspace) was consumed within 3 weeks (Figure 2, Supplementary Figure S1). In contrast, even after approx. Four weeks of incubation, more than half of the initially added H2 was still present in both tested April sediment horizons and in the bottom May sediment slurries (exception was sediment slurry II of bottom sediments from May, where all H2 was used) (Supplementary Figure S1). In the April sediment slurries H2 consumption dropped at a relatively constant rate over time. The May and August incubations appeared to experience a H2 consumption boost after about one to 2 weeks (Figure 2), suggesting that microbial communities had to adapt, microbial growth had to overcome a lag phase or specific metabolic byproducts had to be produced before elevated H2 consumption could kick in. In April and May slurries, more H2 was consumed in the top than in the bottom sediments (Supplementary Figure S1). The April slurries exhibited the by far lowest consumption rates (7.3 ± 3.4 and 22.1 ± 2.7 nmol H2 mL−1 h−1 sediment −1 or 0.002 ± 0.0009 and 0.003 ± 0.0004 fmol H2 cell−1 h−1, respectively, the latter assuming that all cells are using H2). The highest rates measured in bottom August sediments were up to 10 times higher than the lowest rates for April sediments (up to 74.8 ± 5.5 nmol H2h−1mL sediment −1 or 0.018 ± 0.001 fmol H2 cell−1 h−1) (Figures 3a,b). When considering H2 consumption per cell, rates in May and August top and May bottom sediments were comparable, whereas those from August bottom sediments were 1.4 times higher (Figure 3b). Overall, the H2 consumption rates per volume and hour were in the upper range of and/or up to 2.7-fold higher relative to those determined for hydrothermal fluid samples in previous H2 supplemented fluid incubations (Perner et al., 2011; Perner et al., 2013). In stark contrast, the per cell estimates of H2 consumption was up to 17,000-fold lower than rates observed in previous H2 incubation experiments of hydrothermal fluids (Perner et al., 2013). Consequently, either only a small proportion of the microbial community is in fact consuming H2 or many cells may have the H2 consumption ability but are doing this at extremely slow rates.
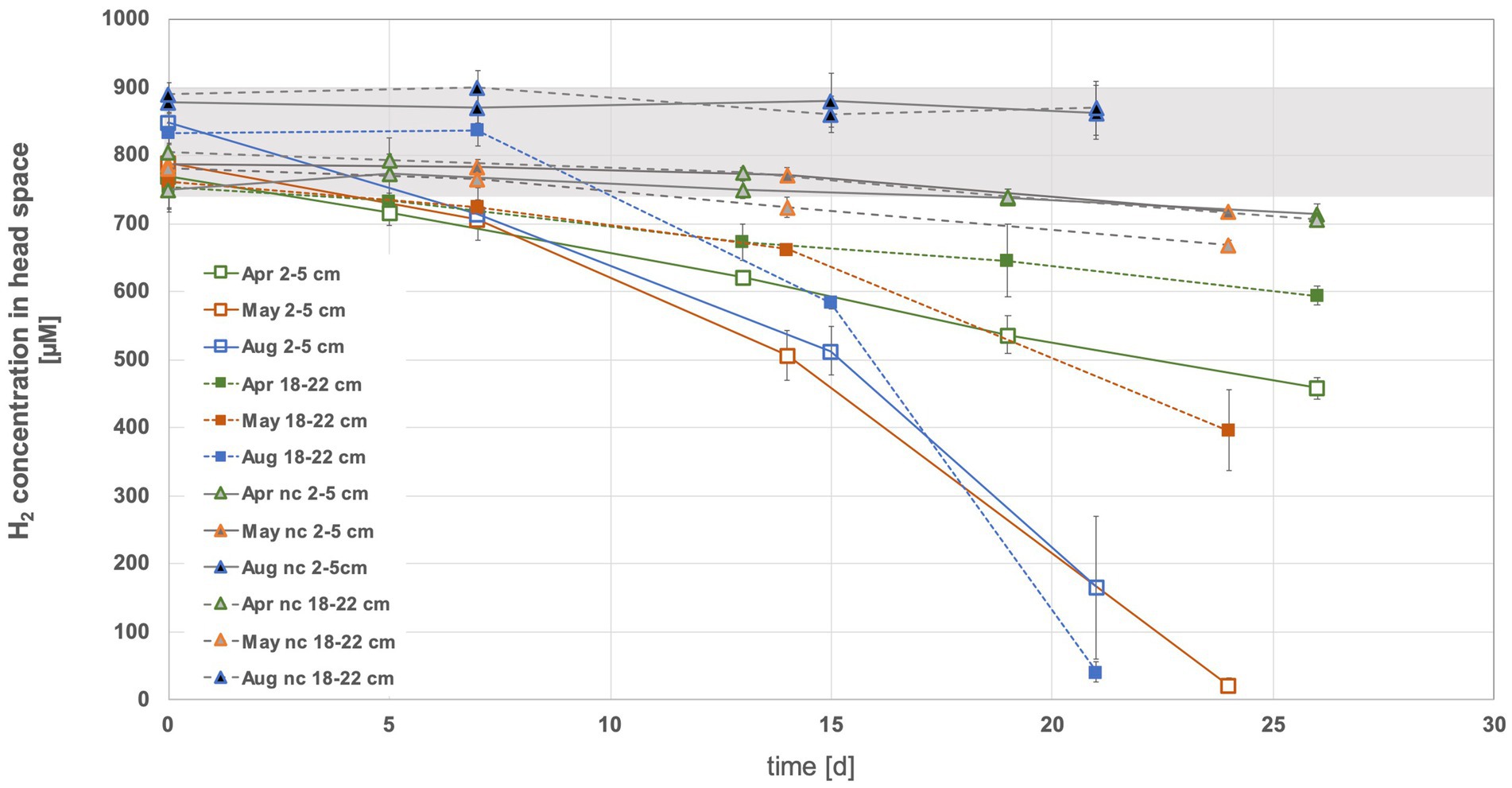
Figure 2. Hydrogen consumption in the headspace of sediments slurries with top (2 to 5 cm) and bottom (18 to 22 cm) sediments from April, May and August 2022. Sample names where nc precedes the month denote the negative controls. These were sediments treated with formaldehyde and where it was assumed that microbial activity ceased. The grey area denotes the range of hydrogen at the start of the experiment.
Monitored methane contents
Methane increase in head spaces was visible, albeit generally low and highest methane accumulation of up to 0.3 μmol (in May bottom incubations) was still a factor of 100 lower compared to total H2 consumption. However, especially during the second week of incubation the increase of methane concentrations indicated active methanogenesis in some of the incubated sediment slurries. The 2–5 cm incubations in April for example, showed an up to 3-fold increase in methane content (57% more than in the respective negative control, see Figure 4). The produced methane in April top sediment incubations appeared to be consumed during the third week, leveling off at the initial methane content. In case of the May incubations a slight initial methane consumption was observed for both of the incubated sediment depths, followed by an apparent methane production phase. This lasted until the end of the experiment for the 2 to 5 cm incubations, while it was followed by a decrease of the methane content in the 18 to 22 cm incubation during week three. Overall, the methane contents in the May incubations were higher (up to 50% during the peaks in the second week) than those observed during the other months (Figure 4). Interestingly, the 18–22 cm incubations of August sediments were the only ones characterized by a constant increase in methane content. The top sediment incubations from August were characterized by starting methane contents and an initial decrease similar to the bottom incubations from May. The methane consumption phase however, only started in incubation week three. The final methane contents were nearly the same for both sediment layers of the August incubations (Figure 4). The observed methane accumulation and consumption indicate the fine tuning among the communities for cycling biologically generated methane or specific shifts in methane-metabolizing communities at increased H2 concentrations.
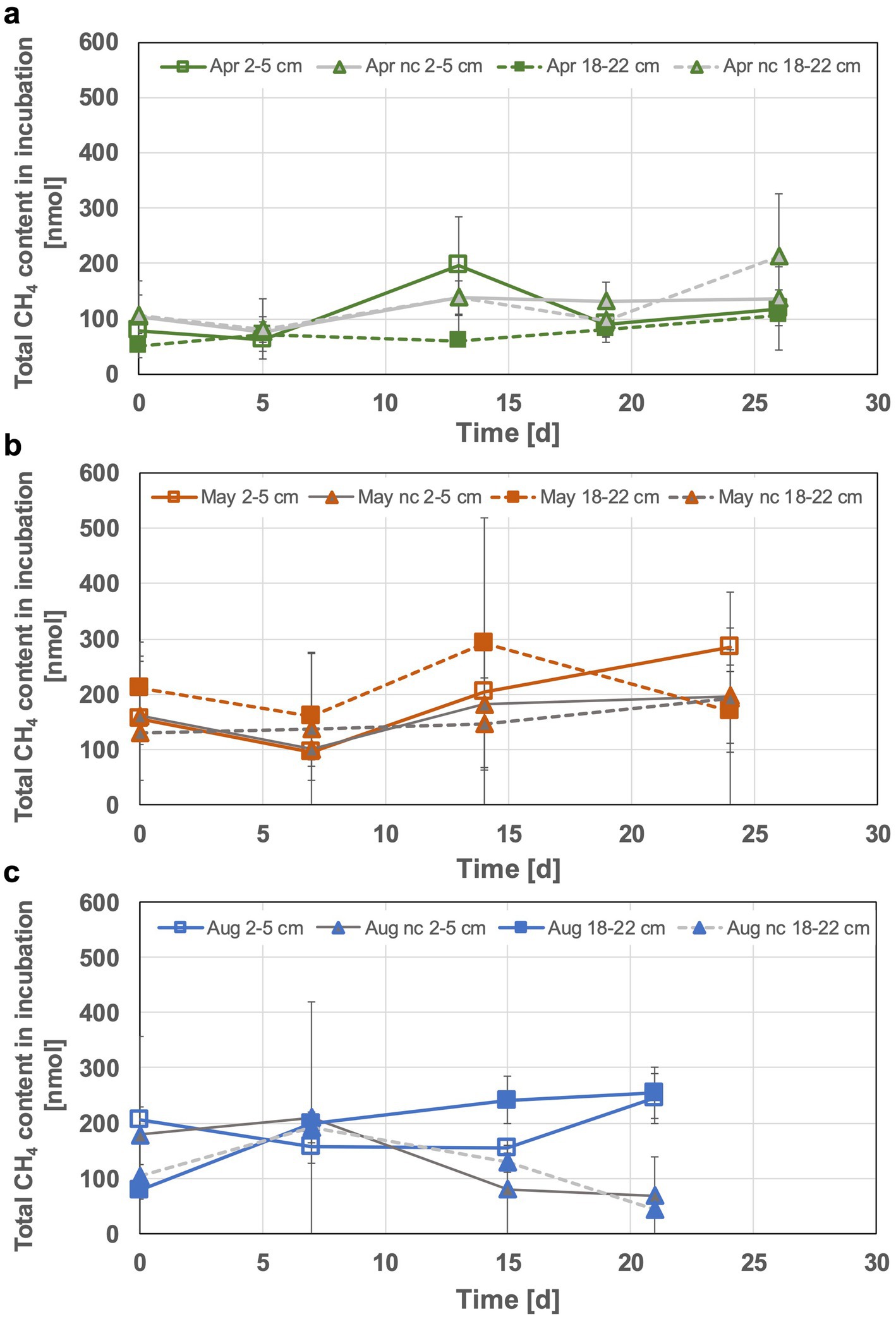
Figure 4. Monitored total methane content in the sediment incubations of April (a), May (b) and August (c). The 2–5 cm incubations are displayed with solid lines, 18–22 cm incubations with dashed lines and nc denotes formaldehyde treated incubations (negative controls).
Microbial community shifts in sediment slurries
Community compositions at the start of the experiments
The most abundant 30 taxa made up 62 to 69% of the bacterial 16S rRNA gene tags of the top (2 to 5 cm) in April, May and August consisting of SRB, namely Desulfosarcinaceae (mainly Sva0081) and Desulfatiglandaceae, sulfur oxidizing Bacteria (SOB) affiliated with Ectothiorhodospiraceae, Chromatiaceae and uncultured Thiotrichaceae, Sulfurimonadaceae, B2M28, and organotrophic Pirellulaceae (Figures 5a,b). The high proportions of less abundant taxa (>30%) further suggest a high phylogenetic diversity in the sampled top sediments. In the August top sediments organotrophic Pirellulaceae and among the SRB Desulfocapsaceae were at least twice as abundant, and Ectothiorhodospiraceae and Desulfatiglandaceae were roughly half as abundant as in the April and May starter sediments (Figure 5). The initial microbial communities of the slurries reflected the conditions in the natural sediment horizons observed in former years (Perner et al., 2022).
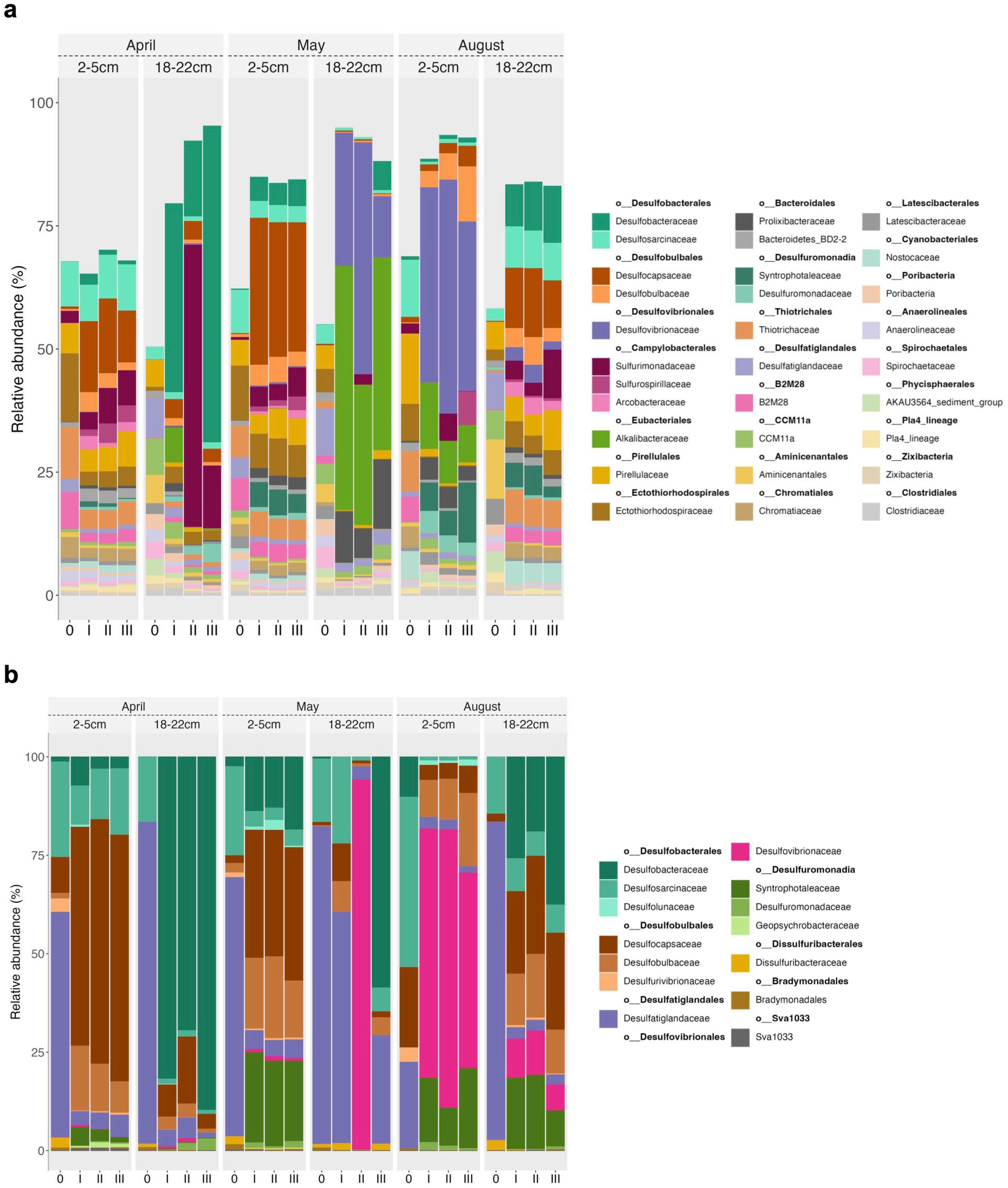
Figure 5. Taxonomy plots based on RNA of 16S amplicons on a family level for the 30 most abundant bacterial taxa (a) and putative Sulfate Reducing Bacteria (SRB) (b). The numbers at the bottom of the graph denote the respective communities at the start of the experiments (0) and at the end of the experiment in triplicates (I, II, III). The SRB subplot (b) is scaled to 100%.
The bottom sediments (18 to 22 cm) were characterized by a higher proportion of less abundant taxa (42 to 50%). Among the most abundant 30 taxa were mainly Desulfatiglandaceae and less Desulfosarcinaceae, few SOB mainly of Ectothiorhodospiraceae (< 1%), and organotrophic Pirellulaceae, CCM11a, Aminicenentales, and Latescibacteraceae. Again, this largely compares to what has been described for Boknis Eck sediment cores before (Perner et al., 2022). As already noted during previous sampling campaigns at Boknis Eck, the archaeal community was similar in all cores and remained remarkably constant during the seasons and the two depth horizons (Perner et al., 2022). Archaea were mostly (78–89% of archaeal 16S tags) dominated by members of the Bathyarchaeia, Lokiarchaeia, Marine Benthic Group D, Woesearchaeales and Deep Sea Euryarchaeotic Group (DSEG) (Figure 6a). Albeit in predominantly small proportions (<10%), ANME and traditional methanogens (e.g., unclassified Methanofastidiosales and Methanosarcinaceae) were present in all starter communities except for May and August top sediments (lacking ANME) (Figure 6).
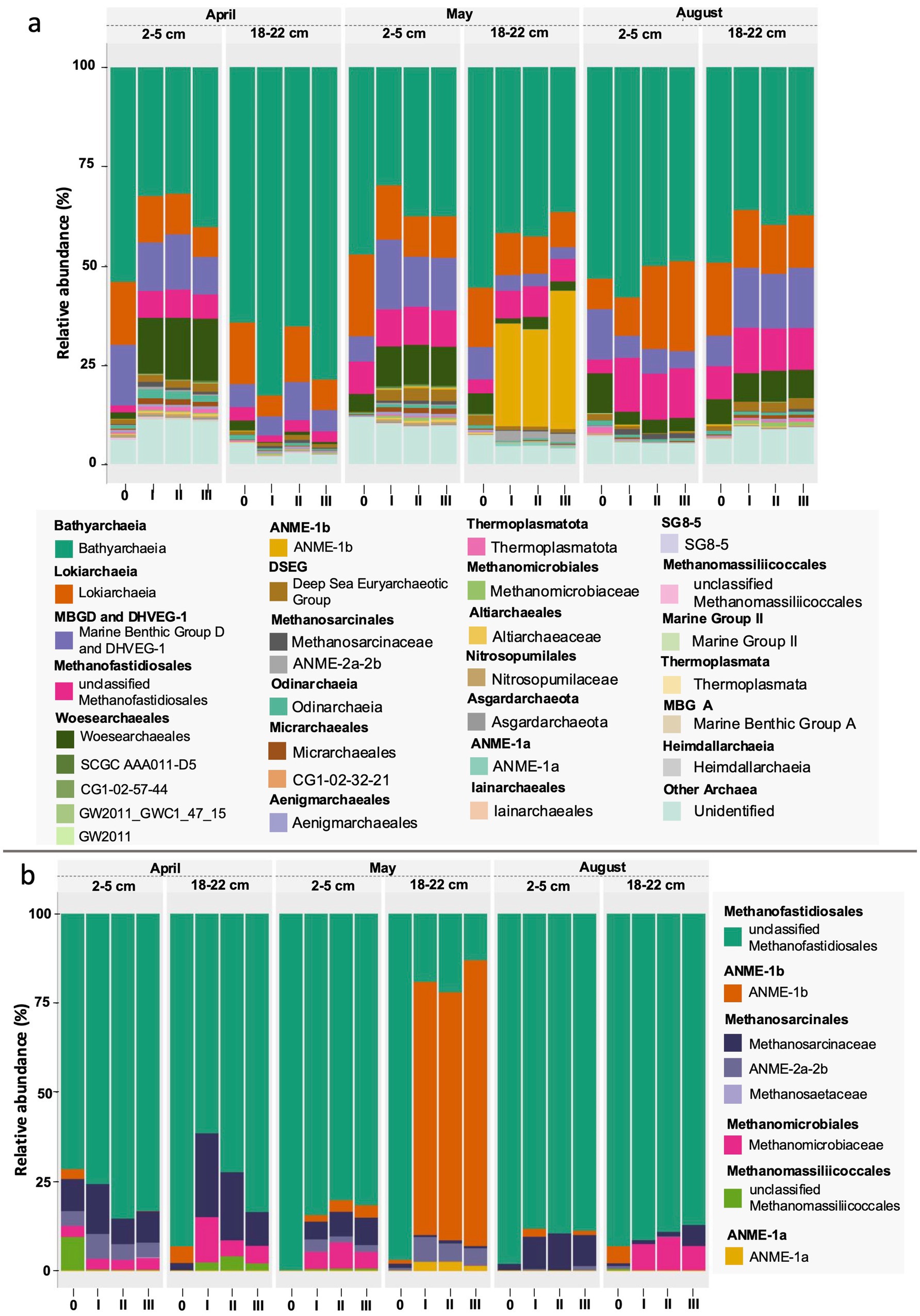
Figure 6. Taxonomy plots based on RNA of 16S amplicons on a family level for the 30 most abundant archaeal taxa (a) and traditional methanogens and anaerobic methane oxidizers (ANME) (b). The numbers at the bottom of the graph denote the respective communities at the start of the experiments (0) and at the end of the experiment in triplicates (I, II, III). The methanogens/ANME subplot (b) is scaled to 100%.
Community shifts after 3 weeks of incubations
During the course of the experiment the microbial communities changed considerably in the sediment incubations (Figures 5, 6, Supplementary Figure S2). Depending on the sediment slurry, the bacterial communities exhibited strong enrichments mostly pronounced in potential SRB, some Gammaproteobacteria typically characterized as SOB, and Alkalibacter (Firmicutes) (Figure 5, Supplementary Figure S2). Interestingly, among the Archaea, the most pronounced shift was an increase in certain groups of ANME in the bottom sediments of May slurries (Figure 6).
Top sediments. During the experiments the top sediment slurries of April and May became enriched in members of the Desulfobacterium catecholicum group and Desulfotalea genus (Desulfocapsaceae) (particularly in the May sediments), and in Sulfurimonadaceae but depleted in Thiogranum spp. (Ectothiorhodospiraceae) (less so in May), Desulfatiglandaceae, and B2M28 (uncultured Gammaproteobacteria). Furthermore, Syntrophotaleaceae notably increased (up to 5%) during May top sediment incubations. Desulfosarcinaceae were unchanged for April but depleted in May. August top sediments became enriched in Desulfovibrionaceae (from <0.1% to up to 47%), Desulfobulbaceae (mainly of the Desulfobulbus genus), Alkalibacteraceae and Syntrophotaleaceae during the course of the experiment (Figure 5). In all sediment incubations the archaeal communities were generally dominated by Bathyarchaeia, regardless of the month or depth horizon (Figure 6). In April and May top sediment incubations Woesearchaeales became significantly enriched (10-fold and 2-fold, respectively) but their abundance decreased approximately 3-fold in August top sediments relative to the starter top sediment community (Figure 6a). Relative to the beginning of the sedimentary archaeal communities, abundances of members of the Marine Benthic Group D were slightly reduced in April, more than doubled in May, and were roughly halved in August top sediments over time. Lokiarchaeotal 16S rRNA gene amplicons were predominantly inconsistent in trends for the different slurries, while the abundances of unclassified methanogenic Methanofastidiosales increased during all incubations of the 2 to 5 cm sediment horizons (Figures 6a,b). This effect was differently pronounced for the distinct top sediment incubations: while the increase was approximately 1.5-fold in May, in August incubations it was roughly 3.6-fold and peaked with approximately 4-fold higher abundances of Methanofastidiosales at the end of the April incubations.
On a level of significance, taxa that were important for differentiating April, May and August incubations at the end of the experiment from each other were for April and May as opposed to August Desulfobacterium, Thiogranum, and B2M28, for May as opposed to the other experiments Desulfotalea, and for August Desulfopila (Desulfocapsaceae) (Figures 5, 7a, Supplementary Figure S2). The most important archaeal taxa distinguishing the April and May communities from the August top sediment slurries were Woesearchaeales and DSEG, while for May slurries with 2 to 5 cm sediments the most important taxon differentiating them from April and August incubations was the methanogen Methanogenium (Methanomicrobiaceae) (Figures 6, 7b).
Bottom sediments. April experiments exhibited enrichments, which primarily included differently strong shifts towards Desulfobacteraceae in all replicates and Sulfurimonadaceae in two out of the three replicates. For May experiments the trend between the three replicates was more consistent towards clade WCHB1-32 (Prolixibacteraceae, 14–17%), Alkalibacteraceae and Desulfovibrionaceae with variations in the abundance of the respective 16S rRNA gene amplicons of the latter two families between the three replicates. Replicate I was enriched towards 50% of Alkalibacter and 25% of Halodesulfovibrio, replicate II towards 29% (Alkalibacter) and 47% (Desulfovibrio 38% and Halodesulfovibrio 9%) and replicate III towards 40% (Alkalibacter) and 12% (Halodesulfovibrio), respectively (Figure 5, Supplementary Figure S2). Shifts in the August experiments were most consistent, where all replicates showed very consistent trends. In August bottom sediments no clearly dominating lineages were observed but rather numerous taxa became enriched such as SRB of Desulfobacteraceae, Desulfosarcinaceae and Desulfocapsaceae, organotrophic Syntrophotaleaceae and putative SOB of the Thiotrichaceae and Sulfurimonadaceae families (Figure 5). Overall, only minor changes were observed over time in the abundances of the different taxa forming the archaeal communities of the April and August bottom sediment incubations. In the May bottom sediment slurries however, a massive enrichment of 16S rRNA gene tags allocated to ANME-1b was striking - from <0.1% of all archaeal 16S amplicons at the start of the experiment to 24–35% at the end of the slurry experiments (Figure 6a). Simultaneously, quantitative CARD-FISH analyses revealed an increase of archaeal probe signals from <4% at the start of the experiments to up to 25% of all DAPI-stained cells at the end of the experiments (Figure 8). In detail, at the end of the enrichment experiment, May slurries with bottom sediments were mostly dominated by ANME-1a, ANME1-b, ANME-2a-2b (summing up to 27–38%), accompanied by a smaller proportion (6–8%) of 16S rRNA gene amplicons related to known traditional methanogens. The majority of the latter group was associated with unclassified members of the order Methanofastidiosales (6–8%). Other known methanogenic genera like Methanosarcina were identified at very low abundances (<0.2%, each) in the bottom sediment incubations in May (Figure 6). In all other bottom sediment slurries ANME remained below 0.03% of archaeal 16S rRNA amplicons, while abundances of methanogens (again predominantly Methanofastidiosales) in August bottom sediment incubations exceeded those of May with 12 to 13% in total (Figure 6a). A closer look at only the “traditional” methanogens and ANME distribution of 16S rRNA gene amplicons demonstrated that the abundance of ANME-1b decreased almost completely in April bottom sediments during incubations (Figure 6b). The fact that the April bottom sediment incubations were the only ones exhibiting no noticeable increase in 16S rRNA gene amplicons of methanogens, could indicate that methanogenesis was negatively affected by increased H2 concentrations and/or the overall redox state in the incubations. Despite the high proportions and enrichment of methanogens in the August bottom sediment slurries (Figure 6a), the abundance of ANME ceased completely in two of the three replicates (Figures 6a,b), indicating that the presence of substrates was not decisive of AOM activity and growth of ANME in our sediment incubations.
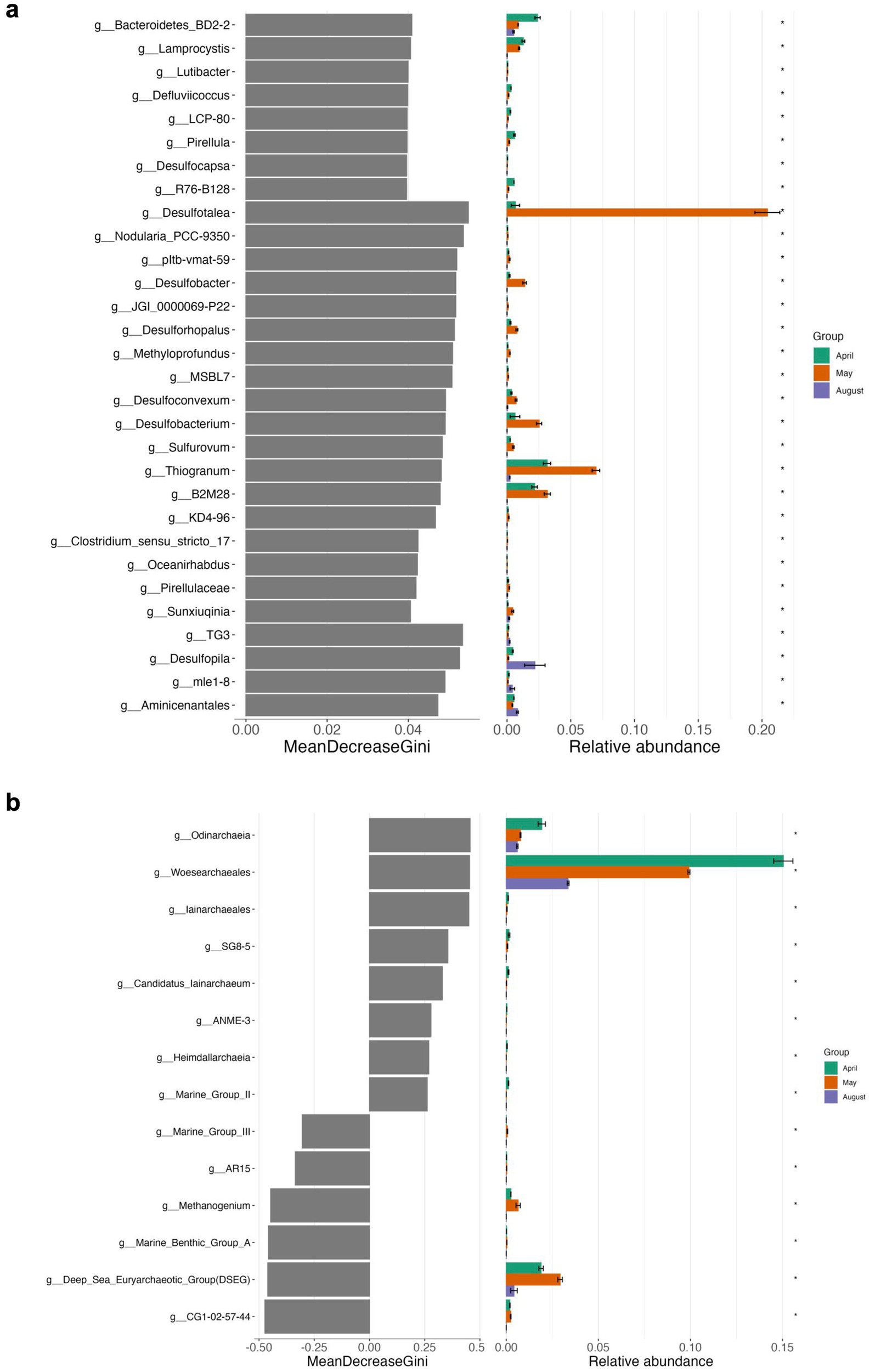
Figure 7. Differential abundance analyses using random forest testing on genus level for Bacteria (a) and Archaea (b) of top sediment incubations. The MeanDecreaseGini score represents a measure for the importance of a genus in the random forest model.
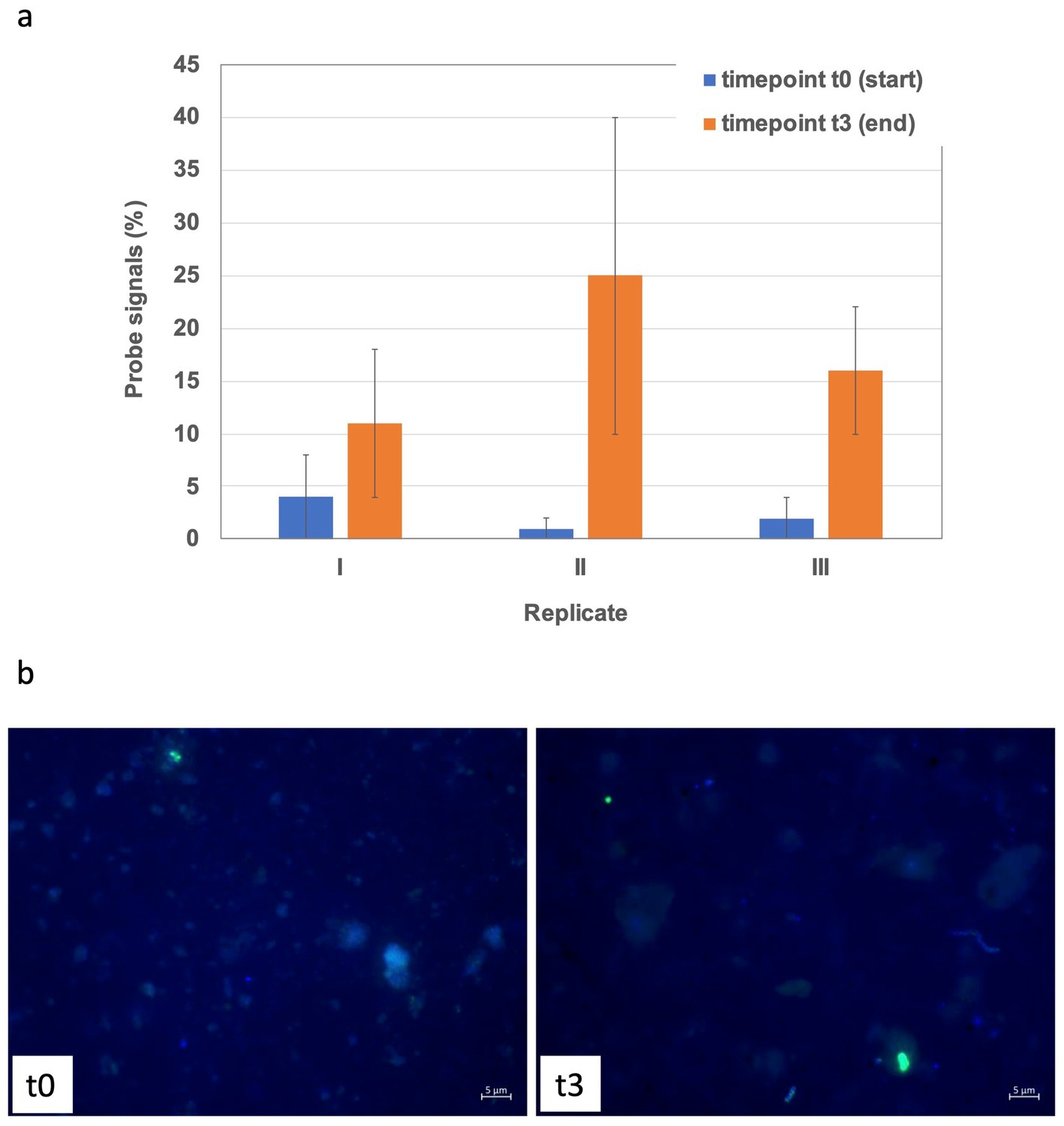
Figure 8. CARD-FISH based quantification of Archaea (a) in the May 18 to 22 cm slurry and exemplary microscopic CARD-FISH images (b) at the start and end of the experiments. Fluorescence signals are shown as overlays, where those of the archaeal probe ARCH915 are displayed in green, while DAPI signals representing all cells are shown in blue color.
In summary, bottom sediments were mostly affected by bacterial rather than archaeal community shifts (exception May) where the most important groups differentiating the slurry experiments from each other at the end of the experiments were Desulfobacterium (Desulfobacteraceae) (April), WCHB1-32 (Prolixibacteraceae, Bacteroidales) Desulfovibronaceae and Alkalibacter (Alkalibacteraceae, Firmicutes) (May) and phylogenetically diverse SRB such as Sva0081 (Desulfosarcinaceae), Desulfobacterium catecholicum (Desulfocapsaceae), Desulfobulbus (Desulfoblbaceae) and Syntrophotalea (Syntrophotaleaceae) as well as potential SOB such as Thiogranum (Ectothiorhodospiraceae), Thiotrichaceae, B2M28 and Lamprocystis (Chromatiaceae) (August) (Figures 5, 9a, Supplementary Figure S2). In contrast, among the Archaea, on a level of significance only August slurries demonstrated enrichments in Marine Benthic Group D, DSEG, Woesearchaeales and some Methanogenium and May bottom sediments enrichments in ANME-1b during incubations (for details see also above, Figures 6, 9b).
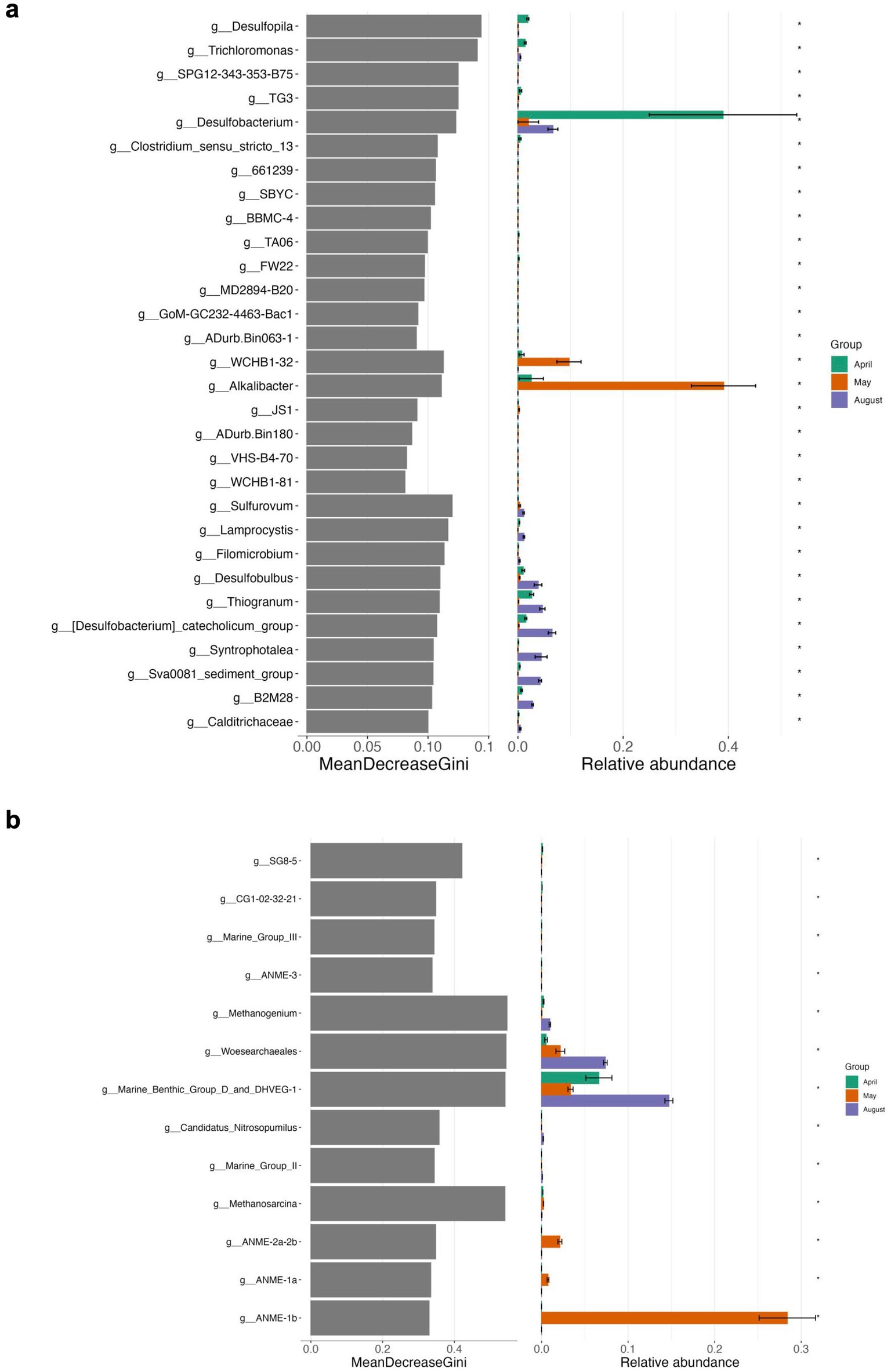
Figure 9. Differential abundance analyses using random forest testing on genus level for Bacteria (a) and Archaea (b) of bottom sediment incubations. The MeanDecreaseGini score represents a measure for the importance of a genus in the random forest model.
To better understand and characterize the conspicuous enrichment of ANME genes encoding Mcr (methyl coenzyme M reductase) were amplified from May slurries with 18 to 22 cm sediments. Generic primers used for detecting mcr genes from classical euryarchaeotal methanogens did not yield any products, neither at the start nor at the end of the experiments, albeit the positive control Methanosarcina mazei gave signals (data not shown). ANME-1 and ANME-2 specific mcr primers gave significant PCR products at the end of the experiments but gave no products at the start of the experiment, further confirming the enrichment of ANME during incubation at increased H2 concentrations. Phylogenetic analyses of sequenced mcr-gene clones documented three distinct clone clusters (all generated with ANME-1 specific primers) placed within the ANME-1 mcr gene cluster (Figure 10). Clones generated with ANME-2 specific primers were less diverse: only one of them clustered together with other ANME-2 mcr genes, while the rest (18 clones) represented one large cluster exhibiting highest similarity with the mcr genes of the methanogenic Methanosaeta harundinacea and Methanomassillicoccus luminyensis species (Figure 10).
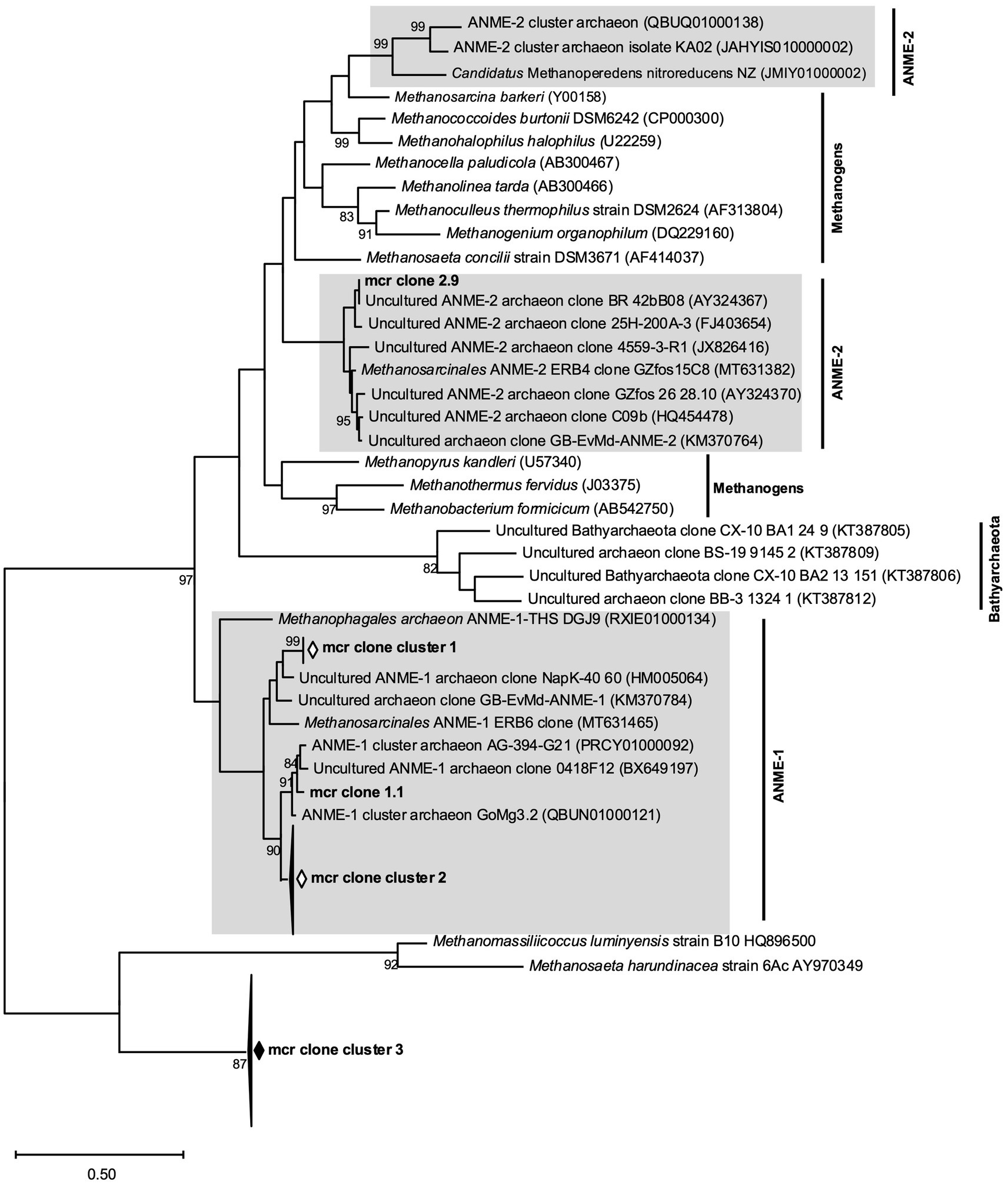
Figure 10. Phylogenetic tree of mcr genes from May bottom sediment incubations generated using ANME specific primer pairs (mcr clones) as well as reference mcr genes from methanogens, ANME-1, ANME-2 and Bathyarchaeota. The scale bar denotes the number of substitutions per nucleotide position and bootstrap values are only indicated if greater than 80%.
Discussion
Linking hydrogen consumption rates with shifts in the microbial community compositions
Our incubation experiments revealed the capability of microbial organisms and communities to adapt to increased H2 concentrations. The shifts in microbial diversity were largely consistent in triplicate experiments. However, we observed large phylogenetic differences in the initial and incubated samples depending on the time of sediment sampling (April, May or August), indicating a marked seasonality in line with previous observations (Perner et al., 2022). We predominantly observed a season-dependent enrichment of organisms, which became dominant during incubations, although they were below detection limit at the start of the experiment. Furthermore, during most slurry incubation experiments microbial diversity - as shown by Shannon diversity indices - decreased, with the most pronounced effects notable in the May bottom sediments (Supplementary Figure S4). This observation may not only result from the elevated H2 concentrations but also from the “bottle effect,” frequently observed in lab-based incubation experiments that are cut off from the in situ supply of OM, nutrients, electron donors and acceptors and leading to significant shifts of microbial communities (e.g., Baltar et al., 2012; Ionescu et al., 2015). Furthermore, the artificial seawater medium used for setting up the sediment slurries differs from the geochemical composition of the respective pore waters of the incubated sediment layers (e.g., in hydrogen sulfide concentrations), most likely also influencing microbial activity and communities. Overall, this indicates that incubation at the offered H2 concentrations and medium conditions does not reflect in situ H2 availability and consumption, but rather provides insights into the H2 oxidizing potential of the respective microbial communities. It should be noted, though, that these experiments currently represent the closest quantitative approximation to in situ H2 consumption rates, as in situ measurements are hampered by the commonly low H2 concentrations and continuous microbial H2 production in anoxic sediments.
Enrichment of various SRB was obvious in most incubations
While the incubations were carried out with sediment samples obtained under different in situ redox conditions due to seasonally occurring bottom water hypoxia and shifts in biogeochemical gradients in near-surface sediments, redox conditions in the incubations were essentially targeted to enable microbial SR (initial SO42− concentration ~ 15 mM). Desulfatiglandaceae were most abundant among putative sulfate-reducing microorganisms (SRM) in situ and present in all initial slurries and at both sampling depths, but their relative abundance decreased strongly during incubations at increased H2 concentrations. Desulfatiglandaceae are considered as highly versatile microorganisms which can metabolize a large variety of organic substrates and also might be able to adapt to non-SR metabolisms (acetogenesis, fermentation) in the absence of sulfate (Jochum et al., 2018). At the end of the H2 amended incubations however, other putative SRMs were enriched with strong dependence on seasonality. Members of the respective genera, such as Desulfobacterium, Desulfotalea and Desulfovibrio are commonly described as metabolically versatile SRB (including autotrophic lifestyles) with the ability to use H2 as an energy source (Brysch et al., 1987; Knoblauch et al., 1999; Baffert et al., 2019). The enrichment of initially low abundant SRM and the (compared to initial sediment communities) decreased abundances of Desulfatiglandaceae in the H2-spiked incubations may indicate that H2 is not a major electron donor for SRM in Boknis Eck sediments in the sampled seasons.
The response to H2 addition is season-dependent
The top sediments of April and May exhibited a similar shift in microbial community composition among the classifiable bacterial families (Figure 5). Yet, May slurries with top sediments consumed around twice as much H2 per cell (Figure 3b) during the same incubation period than the April slurries kept under the same conditions. This may be explained by a microbial community adapted to a higher H2-availability in the sediments. Phytoplankton blooms are known to occur in late winter/early spring at Boknis Eck (Smetacek et al., 1984; Bange et al., 2011) and synthesizing fresh OM, which essentially rain down on the seafloor. Input of labile OM and rising temperatures from spring onwards stimulate increased OM degradation, thereby producing H2 and consuming primarily O2, consequently, giving rise to microbes catalyzing hydrogenotrophic processes. For example, H2 consumption in the May top sediment slurries appeared to be related to members of the Desulfotalea genus (Desulfocapsaceae), which are known H2 oxidizers (Knoblauch et al., 1999) and made up 20% of the bacterial 16S rRNA gene tags in May but were absent in two of the three replicates in April top sediment incubations (Supplementary Figure S2). Within the Desulfocapsaceae family members of the facultatively H2 oxidizing [Desulfobacterium] catecholicum group (type strain reclassified as Desulfocastanea within the same family) (Galushko and Kuever, 2019) showed the highest enrichment (up to 12% on the genus level) in April top sediment incubations. Thus, H2 consumption in the incubation experiments was essentially coupled to SR, which is in accordance with sulfate availability and the relative dominance of microbial SR in top sediments compared to other anaerobic processes. However, albeit only in small relative abundance, the May top sediments became also enriched in methanogenic Methanogenium and DSEG as important taxa for differentiating the slurry community from the other top sediment incubations (Figures 6, 7b). Consequently, besides SRM, also other organisms are directly or indirectly affected by increased H2 supply and likely consume H2, metabolic intermediates or end-products (e.g., acetate or methane). Additionally, the top April and May sediment incubations were characterized by approximately 30 and 15%, respectively, of low-abundant and/or unclassified Bacteria (Figure 5b). This uncalled-for group likely masks H2 utilizing ability which remains, with our current tools, undetectable. As expected in the light of substrate competition with SRM, in all sediment incubations hydrogenotrophic methanogens were (at most) only present in small numbers. Instead, uncultured members of the methylotrophic Methanofastidiosales (Nobu et al., 2016) dominated within the methanogenic communities (Figure 6b). Despite differing abundances of methanogens already at the start of the experiments, April and May top sediment slurries demonstrated comparable methane contents in the first 2 weeks of incubation (Figure 4), which may be related to the higher cell counts in the April top sediments. The ongoing increase of methane concentrations during the second half of the May top sediment incubations may be explained by the initial starting conditions providing labile OM input, lower O2 concentrations in the bottom waters and higher temperatures compared to the April incubations (see above).
In total, the microbes in the May bottom sediment slurries consumed 50% more H2 than those from April sediments, but compared to May top sediments H2 consumption was roughly halved (Figure 2, Supplementary Figure S1). Per volume H2 consumption in April bottom sediments was less than 50% of that from April top sediment incubations (Figure 3a). However, when considering the per cell rate, the difference in H2 consumption between the top and bottom sediment incubations of both April and May were less pronounced (Figure 3b). The lower H2 consumption rates in April bottom slurries coincide with enrichments of putatively autotrophic Sulfurimonas (55% in replicate II and 15% in replicate III) and Desulfobacterium spp. (40% I replicate I, 15% in replicate II and 65% in replicate III) (Supplementary Figure S3a). Sulfurimonas spp. are hallmarked by versatile metabolisms and are capable of oxidizing H2, hydrogen sulfide, elemental sulfur, thiosulfate, sulfite coupled to reduction of nitrate, nitrite, manganese oxide or O2 and can grow autotrophically or with acetate or various organic compounds (Han and Perner, 2014, 2015; Han and Perner, 2016; Henkel et al., 2021). Physiological experiments demonstrate that Sulfurimonas consumes H2, but also that significant growth requires a sulfur source. If offered, it prefers reduced sulfur species as energy source, coupling their oxidation to nitrate reduction (Han and Perner, 2014). Desulfobacterium spp. can couple sulfate and thiosulfate respiration with lactate, H2 oxidation with SR or perform pyruvate fermentation (Neretin et al., 2003). Members of this group have also been associated with dimethylsulfoxide (DMSO) reduction (Jonkers et al., 1996). Although our experiments do not resolve if and which electron acceptors other than sulfate became available in the course of the incubations, the dominance of Sulfurimonas and Desulfobacterium spp. in bottom April slurries suggests active sulfur cycling between the taxa when starting sediments are exposed to anoxic conditions.
Increased per cell H2 consumption rates of May bottom sediments align with shifts towards SRB of the Desulfovibrionaceae (Desulfovibrio and Halodesulfovibrio), Prolixibacteraceae (WCHB1-32) and Alkalibacteraceae (Alkalibacter)—at the start they made up less than 1% and at the end between 12 and 47%, ca 6 to 14% and 28 to 50%, respectively, of all bacterial 16S rRNA gene amplicons (Figure 5a). This is a notable difference between the communities from May bottom sediments and the April slurries and as such to all other slurries. Alkalibacter spp. are not known to utilize H2 or sulfate but instead ferment distinct organic compounds (e.g., mono- and disaccharides released in the course of polymer degradation), producing formate, acetate, ethanol, hydrogen and CO2 and favoring alkaline environments (Garnova et al., 2004; Namirimu et al., 2022). In contrast, Desulfovibrio can couple oxidation of lactate, formate and H2 to SR (Postgate and Campbell, 1966). Some strains can grow autotrophically (Sánchez-Andrea et al., 2020) or appear to be involved in DMSO reduction (Jonkers et al., 1996). Some strains are also involved in iron reduction (Gonzalez et al., 2024) which could explain why sulfide concentrations experience a low in the starter sediments between 18 and 22 cm (cf. Figure 1c). Strains have also been documented to be involved in replacing anhydrite (Ca[SO4]) with authigenic carbonates (Zueblin, 1988). When either OM or methane (in conjunction with ANME) is available, bicarbonate is produced, increasing alkalinity and causing an oversaturation of calcium- and carbonate-ions; thus, forming secondary carbonate precipitates (Rouwendaal et al., 2023). If this were the case then the enrichment of Alkalinibacteraceae could be explained.
Transient amounts of methane, although low compared to H2 supply and consumption were slightly higher compared to other experiments. In May bottom sediments, methane reached a maximum after 1 week of incubations and was then consumed rapidly but started accumulating again slowly towards the end of the experiment. Although no methanogenic mcr genes were identified in the respective sediment slurries, uncultured members of the methylotrophic Methanofastidiosales (Nobu et al., 2016) made up ~6–8% of the archaeal community, similar to what was found in most other slurries (Figure 6a). It remains unclear though, why in this specific slurry methane production was slightly higher than in the other respective slurries. A possible explanation may be the presence of alternative “non-traditional” methanogens: In the last years evidence has grown that methane metabolisms might be more widespread among Archaea than previously assumed. For example, homologues of genes encoding enzymes involved in hydrogenotrophic and methylotrophic methanogenesis have been detected in Bathyarchaeota and Verstraetarchaeota (Evans et al., 2015; Vanwonterghem et al., 2016; Zhou et al., 2018). Previous experiments conducted with Boknis Eck sediments recognized that under the tested conditions methylated substrates or methanol rather than H2 served as base for methanogenesis, yet phylogenetic profiling was not conducted and thus the archaeal lineages responsible for the methanogenic activity were not identified (Maltby et al., 2018). Bathyarchaeota were present in all our incubated sediment slurries, but no specific enrichment could be noted for the May 18 to 22 cm sediments (Figure 6a). However, different bathyarchaeotal populations may be involved in producing methane during specific slurry conditions. Then again, this could be visualized by transcriptomic data, which are lacking for the sediment slurries set up in this study. On the other hand, previous work has proposed that ANME may be capable of methanogenesis under specific environmental conditions (Biddle et al., 2012; Kevorkian et al., 2021), as they possess the enzymatic repertoire for hydrogenotrophic methanogenesis but reverse it to perform AOM (Timmers et al., 2017). Since this reverse methanogenesis needs to be highly exergonic and may rely on inter-species H2 transfer, (just like fermentation) it is not feasible under high H2 concentrations (Hoehler et al., 1994; Coon et al., 2023), which may be the cause for the decrease of ANME-abundances in August bottom sediments. Furthermore, analyses of various metagenome-derived genomes revealed the presence of [NiFe]-hydrogenases in ANME-1a, ANME-1b and ANME-1c clades, but it still remains unclear, whether these typical H2 uptake enzymes are metabolically involved or just a hereditary remnant in ANME-1 (Benito Merino et al., 2022; Laso-Pérez et al., 2023).
Given the massive enrichment of ANME in the May bottom incubations within only 3 weeks and the elevated methane concentrations over a large part of the incubation period, it is tempting to speculate that at least parts of the ANME populations may be indeed operating hydrogenotrophic methanogenesis and that methanogenic microorganisms can effectively compete for hydrogen under sulfate reducing conditions. However, this would be highly uncommon and conspicuous, since methanogenesis is thermodynamically unfavorable relative to sulfate reduction and, thus, usually limited to turnover of non-competitive methanogenic substrates (e.g., methylamines) in natural habitats (e.g., Oremland and Polcin, 1982). Further, we note, that methane concentrations remained low compared to consumed H2 throughout the experiments with only small changes compared to control incubations. Thus, if enrichment of ANME is related to hydrogenotrophic methanogenesis, it needs to be balanced by methane consumption through AOM. This would involve some very efficient niche partitioning of forward and backward catalyzing organisms and requires further investigation. Still, while cryptic methane cycling under sulfate-reducing conditions was reported in the past (Krause et al., 2023), it is, again, usually related to methanogenesis from non-competitive substrates and only of minor importance for net substrate turnover at non-limiting sulfate concentrations (Kevorkian et al., 2022). Methane production from non-competitive substrates such as methanol has been observed in ANME-1 dominated microbial mats (Bertram et al., 2013) and may also be a putative metabolic pathway for ANME in our sediment incubations. However, given the presence of methylotrophic methanogens in all sediment slurries, methylotrophic methanogenesis of ANME – with or without cryptic methane cycling – may not explain why the ANME enrichment is limited to incubations with May bottom sediments. Taking into account that in the past, methanogenic growth of ANME was mainly inferred from indirect evidence such as mcrA gene copy numbers in methanogenic sediments below the sulfate–methane transition zone (SMTZ) (e.g., Lever et al., 2023; Deng et al., 2025), and that there is no direct evidence of hydrogen-based methanogenic growth of ANME-1 from incubation studies (e.g., Benito Merino et al., 2022), it needs to be considered that apparent hydrogenotrophic growth of ANME is potentially unrelated to methanogenesis, but instead either to the turnover of metabolic intermediates or products from dissimilatory sulfate reduction (e.g., S(0), Fang et al., 2020; Wang et al., 2023) or reactive compounds being present and characteristic in May sediments due to the beginning decrease of oxygen concentrations in overlying bottom waters. Alongside the dramatic increase in ANME, members of Desulfovibrionaceae and Alkalibacter became considerably enriched in the course of the May bottom incubations. Previous experiments have suggested that sulfate reducing H2 oxidizers and hydrogenotrophic methanogens induce an increase in pH related to intense proton- and bicarbonate utilization (Dopffel et al., 2023). Hence, we posit that here Desulfovibrio potentially interacting with a methanogen from the Methanofastidiosales or Bathyarchaeota populations produce alkalinity that favors Alkalibacter. Interestingly, despite similar shifts in Desulfovibrionaceae abundances in August top sediment incubations, May bottom slurries are the only slurries where the explicitly high enrichment of Alkalibacteraceae is featured, although alkalinity production through hydrogenotrophic SR and methanogenesis is expected to be largely similar in other incubations with intense H2 consumption.
Overall, the distinct enrichment of both ANME and Alkalibacter in May bottom sediments are considered as indicative of specific properties of starter sediments. These specific properties of May bottom sediments may be related to the shift from oxic to anoxic conditions in the overlying bottom waters compared to April experiments. This change could induce community shifts and the availability of additional electron acceptors. It could be further related to abiotically or metabolically produced compounds or viruses modulating microbial activity, as it has been documented that certain viruses appear specific to, e.g., ANME (Laso-Pérez et al., 2023).
The August sediment slurries consumed the most H2 during incubations (Figures 2, 3). The August top sediment incubations were characterized by a quite different bacterial composition relative to the slurries from April and May. Apart from the enrichment in Desulfovibrionaceae and Alkalibacteraceae, a shifting towards Desulfopila and non-SR organotrophs was observed. Desulfopila spp. use a broad range of organic electron donors for SR, are involved in iron cycling and can also grow with H2 chemolithoautotrophically (Gittel et al., 2010; Beese-Vasbender et al., 2015).
The August bottom sediments with the overall highest H2 consumption per cell were the most phylogenetically diverse assemblage containing SRB and SOB as well as shifts towards Methanogenium and MBG-D. It may be this higher phylogenetic diversity that facilitates the interactions and thus metabolic exchange furthering elevated H2 consumption. Moreso, several putative SOB encode hydrogenase genes on their genome (e.g., Anantharaman et al., 2013; Tengölics et al., 2014), which may partake in consuming the H2. The reason for this distinct development of the community in August sediments may be related to more established reduced conditions in sediments as opposed to April and May sediments. Possible reasons could include the availability of compounds released from mineral grains, such as phosphate and metals like iron or manganese.
Conclusion
Although all of the incubated sediments originate from the same location, the seasonal variations in sedimentary community composition and pore water geochemistry set distinct starting conditions for the H2 enrichments. Sediments exposed to hypoxic bottom water conditions favor a microbial starter community with the highest H2 oxidation potential. This may be related to the overall redox state of the sediment and interstitial waters and more specifically to interactions with dissolved and solid sulfur and iron species. The incubation experiments indicate the breadth of biotic and abiotic interactions that are taking place under anoxic conditions, where minerals and likely OM content and quality affect metabolisms, microbial activity and thus community compositions. Even though most of the observed H2 oxidation potential can be related to hydrogenotrophic SRB, the putative involvement of massively enriched ANME in the H2 cycling of May bottom sediment incubations is conspicuous. The tools and methods applied in this study could not complete the picture of the complex microbial and especially metabolic networks involved in our observations. Detailed metagenomic and/or metatranscriptomic analyses could contribute indications of putatively active metabolic processes but also cannot provide a clear proof of the involvement. Thus, further ecophysiological experiments (extending the here described experiments with, e.g., quantification of hydrogen sulfide production and stable carbon isotope analyses of methylated substrates and methane) are needed to obtain a comprehensive picture of the microbial processes involved in hydrogen and methane cycling taking place in sediments underlying seasonally hypoxic bottom waters.
Data availability statement
The datasets presented in this study can be found in online repositories. The names of the repository/repositories and accession number(s) can be found at: https://www.ncbi.nlm.nih.gov/, PRJNA1213115.
Author contributions
NA-B: Data curation, Formal analysis, Investigation, Methodology, Software, Validation, Visualization, Writing – original draft, Writing – review & editing. CD: Formal analysis, Writing – review & editing. MS: Formal analysis, Validation, Writing – review & editing. MP: Conceptualization, Formal analysis, Funding acquisition, Investigation, Supervision, Visualization, Writing – original draft, Writing – review & editing.
Funding
The author(s) declare that financial support was received for the research and/or publication of this article. The presented work and its publication were funded in the framework of the “program-oriented funding” (PoF IV, Topic 6) of the Helmholtz Association.
Acknowledgments
We thank the crew of the FK Littorina for helping with sediment sampling. We thank Vincent Richter and Merle Kühl for performing CARD-FISH with the bottom May sediments slurries and Gabi Schüßler for cell counts.
Conflict of interest
The authors declare that the research was conducted in the absence of any commercial or financial relationships that could be construed as a potential conflict of interest.
The author(s) declared that they were an editorial board member of Frontiers, at the time of submission. This had no impact on the peer review process and the final decision.
Generative AI statement
The authors declare that no Gen AI was used in the creation of this manuscript.
Publisher’s note
All claims expressed in this article are solely those of the authors and do not necessarily represent those of their affiliated organizations, or those of the publisher, the editors and the reviewers. Any product that may be evaluated in this article, or claim that may be made by its manufacturer, is not guaranteed or endorsed by the publisher.
Supplementary material
The Supplementary material for this article can be found online at: https://www.frontiersin.org/articles/10.3389/fmicb.2025.1565157/full#supplementary-material
References
Adam, N., Han, Y., Laufer-Meiser, K., Bährle, R., Schwarz-Schampera, U., Schippers, A., et al. (2021). Deltaproteobacterium strain KaireiS1, a mesophilic, hydrogen-oxidizing and sulfate-reducing bacterium from an inactive Deep-Sea hydrothermal chimney. Front. Microbiol. 12:686276. doi: 10.3389/fmicb.2021.686276
Adam, N., Kriete, C., Garbe-Schönberg, D., Gonnella, G., Krause, S., Schippers, A., et al. (2019). Microbial community compositions and geochemistry of sediments with increasing distance to the hydrothermal vent outlet in the Kairei field. Geomicrobiol J. 37, 242–254. doi: 10.1080/01490451.2019.1694107
Adam, N., and Perner, M. (2018a). Microbially mediated hydrogen cycling in Deep-Sea hydrothermal vents. Front. Microbiol. 9:2873. doi: 10.3389/fmicb.2018.02873
Adam, N., and Perner, M. (2018b). Novel hydrogenases from deep-sea hydrothermal vent metagenomes identified by a recently developed activity-based screen. ISME J. 12, 1225–1236. doi: 10.1038/s41396-017-0040-6
Adam-Beyer, N., Laufer-Meiser, K., Fuchs, S., Schippers, A., Indenbirken, D., Garbe-Schönberg, D., et al. (2023). Microbial ecosystem assessment and hydrogen oxidation potential of newly discovered vent systems from the central and south-east Indian ridge. Front. Microbiol. 14:1173613. doi: 10.3389/fmicb.2023.1173613
Anantharaman, K., Breier, J. A., Sheik, C. S., and Dick, G. J. (2013). Evidence for hydrogen oxidation and metabolic plasticity in widespread deep-sea sulfur-oxidizing bacteria. Proc. Natl. Acad. Sci. USA 110, 330–335. doi: 10.1073/pnas.1215340110
Baffert, C., Kpebe, A., Avilan, L., and Brugna, M. (2019). Hydrogenases and H2 metabolism in sulfate-reducing bacteria of the Desulfovibrio genus. Adv. Microb. Physiol. 74, 143–189. doi: 10.1016/bs.ampbs.2019.03.001
Baltar, F., Lindh, M. V., Parparov, A., Berman, T., and Pinhassi, J. (2012). Prokaryotic community structure and respiration during long-term incubations. MicrobiologyOpen 1, 214–224. doi: 10.1002/mbo3.25
Bange, H. W., Hansen, H. P., Malien, F., Laß, K., Dale, A. W., Karstensen, J., et al. (2011). Boknis Eck time Series Station (SW Baltic Sea): measurements from 1957 to 2010. LOICZ Inprint 2011, 16–22.
Beese-Vasbender, P. F., Nayak, S., Erbe, A., Stratmann, M., and Mayrhofer, K. J. J. (2015). Electrochemical characterization of direct electron uptake in electrical microbially influenced corrosion of iron by the lithoautotrophic SRB Desulfopila corrodens strain IS4. Electrochim. Acta 167, 321–329. doi: 10.1016/j.electacta.2015.03.184
Benito Merino, D., Zehnle, H., Teske, A., and Wegener, G. (2022). Deep-branching ANME-1c archaea grow at the upper temperature limit of anaerobic oxidation of methane. Front. Microbiol. 13:988871. doi: 10.3389/fmicb.2022.988871
Bertram, S., Blumenberg, M., Michaelis, W., Siegert, M., Kruger, M., and Seifert, R. (2013). Methanogenic capabilities of ANME-archaea deduced from (13) C-labelling approaches. Environ. Microbiol. 15, 2384–2393. doi: 10.1111/1462-2920.12112
Biddle, J. F., Cardman, Z., Mendlovitz, H., Albert, D. B., Lloyd, K. G., Boetius, A., et al. (2012). Anaerobic oxidation of methane at different temperature regimes in Guaymas Basin hydrothermal sediments. ISME J. 6, 1018–1031. doi: 10.1038/ismej.2011.164
Bokulich, N. A., Kaehler, B. D., Rideout, J. R., Dillon, M., Bolyen, E., Knight, R., et al. (2018). Optimizing taxonomic classification of marker-gene amplicon sequences with QIIME 2's q2-feature-classifier plugin. Microbiome 6:90. doi: 10.1186/s40168-018-0470-z
Bolyen, E., Rideout, J. R., Dillon, M. R., Bokulich, N. A., Abnet, C. C., Al-Ghalith, G. A., et al. (2019). Reproducible, interactive, scalable and extensible microbiome data science using QIIME 2. Nat. Biotechnol. 37, 852–857. doi: 10.1038/s41587-019-0209-9
Brysch, K., Schneider, C., Fuchs, G., and Widdel, F. (1987). Lithoautotrophic growth of sulfate-reducing Bacteria, and description of Desulfobacterium-Autotrophicum gen-Nov Sp-Nov. Arch. Microbiol. 148, 264–274. doi: 10.1007/Bf00456703
Callahan, B. J., McMurdie, P. J., Rosen, M. J., Han, A. W., Johnson, A. J., and Holmes, S. P. (2016). DADA2: high-resolution sample inference from Illumina amplicon data. Nat. Methods 13, 581–583. doi: 10.1038/nmeth.3869
Coon, G. R., Duesing, P. D., Paul, R., Baily, J. A., and Lloyd, K. G. (2023). Biological methane production and accumulation under sulfate-rich conditions at Cape Lookout bight NC. Front. Microbiol. 14:1268361. doi: 10.3389/fmicb.2023.1268361
Dale, A. W., Bertics, V. J., Treude, T., Sommer, S., and Wallmann, K. (2013). Modeling benthic-pelagic nutrient exchange processes and porewater distributions in a seasonally hypoxic sediment: evidence for massive phosphate release by Beggiatoa? Biogeosciences 10, 629–651. doi: 10.5194/bg-10-629-2013
Dale, A. W., Geilert, S., Diercks, I., Fuhr, M., Perner, M., Scholz, F., et al. (2024). Seafloor alkalinity enhancement as a carbon dioxide removal strategy in the Baltic Sea. Commun. Earth Environ. 5:452. doi: 10.1038/s43247-024-01569-3
Deng, L., Bolsterli, D., Glombitza, C., Jorgensen, B. B., Roy, H., and Lever, M. A. (2025). Drivers of methane-cycling archaeal abundances, community structure, and catabolic pathways in continental margin sediments. Front. Microbiol. 16:1550762. doi: 10.3389/fmicb.2025.1550762
Dolfing, J. (1988). “Acetogenesis” in Biology of anaerobic microorganisms. ed. A. Zehnder (New York: Wiley-Interscience), 417–468.
Dopffel, N., Mayers, K., Kedir, A., Alagic, E., An-Stepec, B. A., Djurhuus, K., et al. (2023). Microbial hydrogen consumption leads to a significant pH increase under high-saline-conditions: implications for hydrogen storage in salt caverns. Sci. Rep. 13:10564. doi: 10.1038/s41598-023-37630-y
Dyksma, S., Pjevac, P., Ovanesov, K., and Mussmann, M. (2018). Evidence for H2 consumption by uncultured Desulfobacterales in coastal sediments. Environ. Microbiol. 20, 450–461. doi: 10.1111/1462-2920.13880
Evans, P. N., Parks, D. H., Chadwick, G. L., Robbins, S. J., Orphan, V. J., Golding, S. D., et al. (2015). Methane metabolism in the archaeal phylum Bathyarchaeota revealed by genome-centric metagenomics. Science 350, 434–438. doi: 10.1126/science.aac7745
Fang, W., Gu, M., Liang, D., Chen, G. H., and Wang, S. (2020). Generation of zero valent sulfur from dissimilatory sulfate reduction under methanogenic conditions. J. Hazard. Mater. 383:121197. doi: 10.1016/j.jhazmat.2019.121197
Galushko, A., and Kuever, J. (2019). “Desulfocastanea gen. nov” in Bergey's manual of systematics of Archaea and Bacteria, eds. M. E. Trujillo, S. Dedysh, P. DeVos, B. Hedlund, P. Kämpfer, F. A. Rainey et al. New York: John Wiley and Sons Inc., 1–4.
Garnova, E. S., Zhilina, T. N., Tourova, T. P., Kostrikina, N. A., and Zavarzin, G. A. (2004). Anaerobic, alkaliphilic, saccharolytic bacterium Alkalibacter saccharofermentans gen. Nov., sp. nov. from a soda lake in the Transbaikal region of Russia. Extremophiles 8, 309–316. doi: 10.1007/s00792-004-0390-7
Gittel, A., Seidel, M., Kuever, J., Galushko, A. S., Cypionka, H., and Könneke, M. (2010). Desulfopila inferna sp. nov., a sulfate-reducing bacterium isolated from the subsurface of a tidal sand-flat. Int. J. Syst. Evol. Microbiol. 60, 1626–1630. doi: 10.1099/ijs.0.015644-0
Gonzalez, V., Abarca-Hurtado, J., Arancibia, A., Claverias, F., Guevara, M. R., and Orellana, R. (2024). Novel insights on extracellular Electron transfer networks in the Desulfovibrionaceae family: unveiling the potential significance of horizontal gene transfer. Microorganisms 12:796. doi: 10.3390/microorganisms12091796
Greening, C., Biswas, A., Carere, C. R., Jackson, C. J., Taylor, M. C., Stott, M. B., et al. (2016). Genomic and metagenomic surveys of hydrogenase distribution indicate H2 is a widely utilised energy source for microbial growth and survival. ISME J. 10, 761–777. doi: 10.1038/ismej.2015.153
Hall, T. A. (1999). BioEdit: a user-friendly biological sequence alignment editor and analysis program for windows 95/98/NT. Nucleic Acids Symp. Ser. 41, 95–98.
Hallenbeck, P. C. (2009). Fermentative hydrogen production: principles, progress, and prognosis. Int. J. Hydrog. Energy 34, 7379–7389. doi: 10.1016/j.ijhydene.2008.12.080
Hamann, E., Gruber-Vodicka, H., Kleiner, M., Tegetmeyer, H. E., Riedel, D., Littmann, S., et al. (2016). Environmental Breviatea harbour mutualistic Arcobacter epibionts. Nature 534, 254–258. doi: 10.1038/nature18297
Han, Y., and Perner, M. (2014). The role of hydrogen for Sulfurimonas denitrificans' metabolism. PLoS One 9:e106218. doi: 10.1371/journal.pone.0106218
Han, Y., and Perner, M. (2015). The globally widespread genus Sulfurimonas: versatile energy metabolisms and adaptations to redox clines. Front. Microbiol. 6:989. doi: 10.3389/fmicb.2015.00989
Han, Y., and Perner, M. (2016). Sulfide consumption in Sulfurimonas denitrificans and heterologous expression of its three sulfide-Quinone reductase homologs. J. Bacteriol. 198, 1260–1267. doi: 10.1128/JB.01021-15
Hansen, M., and Perner, M. (2015). A novel hydrogen oxidizer amidst the sulfur-oxidizing Thiomicrospira lineage. ISME J. 9, 696–707. doi: 10.1038/ismej.2014.173
Henkel, J. V., Vogts, A., Werner, J., Neu, T. R., Sproer, C., Bunk, B., et al. (2021). Candidatus Sulfurimonas marisnigri sp. nov. and Candidatus Sulfurimonas baltica sp. nov., thiotrophic manganese oxide reducing chemolithoautotrophs of the class Campylobacteria isolated from the pelagic redoxclines of the Black Sea and the Baltic Sea. Syst. Appl. Microbiol. 44:126155. doi: 10.1016/j.syapm.2020.126155
Hoehler, T. M., Albert, D. B., Alperin, M. J., and Martens, C. S. (1999). Acetogenesis from CO2 in an anoxic marine sediment. Limnol. Oceanogr. 44, 662–667. doi: 10.4319/lo.1999.44.3.0662
Hoehler, T. M., Alperin, M. J., Albert, D. B., and Martens, C. S. (1994). Field and laboratory studies of methane oxidation in an anoxic marine sediment - evidence for a methanogen-sulfate reducer consortium. Glob. Biogeochem. Cycles 8, 451–463. doi: 10.1029/94gb01800
Hoehler, T. M., Alperin, M. J., Albert, D. B., and Martens, C. S. (1998). Thermodynamic control on hydrogen concentrations in anoxic sediments. Geochim. Cosmochim. Acta 62, 1745–1756. doi: 10.1016/S0016-7037(98)00106-9
Holmer, M., and Kristensen, E. (1994). Coexistence of sulfate reduction and methane production in an organic-rich sediment. Mar. Ecol. Prog. Ser. 107, 177–184. doi: 10.3354/meps107177
Ionescu, D., Bizic-Ionescu, M., Khalili, A., Malekmohammadi, R., Morad, M. R., de Beer, D., et al. (2015). A new tool for long-term studies of POM-bacteria interactions: overcoming the century-old bottle effect. Sci. Rep. 5:14706. doi: 10.1038/srep14706
Jochum, L. M., Schreiber, L., Marshall, I. P. G., Jorgensen, B. B., Schramm, A., and Kjeldsen, K. U. (2018). Single-cell genomics reveals a diverse metabolic potential of uncultivated Desulfatiglans-related Deltaproteobacteria widely distributed in marine sediment. Front. Microbiol. 9:2038. doi: 10.3389/fmicb.2018.02038
Jonkers, H. M., van der Maarel, M. J. E. C., van Gemerden, H., and Hansen, T. A. (1996). Dimethylsulfoxide reduction by marine sulfate-reducing bacteria. FEMS Microbiol. Lett. 136, 283–287. doi: 10.1111/j.1574-6968.1996.tb08062.x
Jørgensen, B. B. (1982). Mineralization of organic matter in the sea bed—the role of sulphate reduction. Nature 296, 643–645. doi: 10.1038/296643a0
Kevorkian, R. T., Callahan, S., Winstead, R., and Lloyd, K. G. (2021). ANME-1 archaea may drive methane accumulation and removal in estuarine sediments. Environ. Microbiol. Rep. 13, 185–194. doi: 10.1111/1758-2229.12926
Kevorkian, R. T., Sipes, K., Winstead, R., Paul, R., and Lloyd, K. G. (2022). Cryptic methane-cycling by methanogens during multi-year incubation of estuarine sediment. Front. Microbiol. 13:847563. doi: 10.3389/fmicb.2022.847563
Knoblauch, C., Sahm, K., and Jorgensen, B. B. (1999). Psychrophilic sulfate-reducing bacteria isolated from permanently cold arctic marine sediments: description of Desulfofrigus oceanense gen. Nov., sp. nov., Desulfofrigus fragile sp. nov., Desulfofaba gelida gen. Nov., sp. nov., Desulfotalea psychrophila gen. Nov., sp. nov. and Desulfotalea arctica sp. nov. Int. J. Syst. Evol. Microbiol. 49 Pt 4, 1631–1643. doi: 10.1099/00207713-49-4-1631
Krause, S. J. E., Liu, J. R., Yousavich, D. J., Robinson, D., Hoyt, D. W., Qin, Q. H., et al. (2023). Evidence of cryptic methane cycling and non-methanogenic methylamine consumption in the sulfate-reducing zone of sediment in the Santa Barbara Basin. California. Biogeosci. 20, 4377–4390. doi: 10.5194/bg-20-4377-2023
Kumar, S., Stecher, G., Li, M., Knyaz, C., and Tamura, K. (2018). MEGA X: molecular evolutionary genetics analysis across computing platforms. Mol. Biol. Evol. 35, 1547–1549. doi: 10.1093/molbev/msy096
Laanbroek, H. J., and Veldkamp, H. (1982). Microbial interactions in sediment communities. Philos. Trans. R. Soc. London Series B Biol. Sci. 297, 533–550. doi: 10.1098/rstb.1982.0059
Lappan, R., Shelley, G., Islam, Z. F., Leung, P. M., Lockwood, S., Nauer, P. A., et al. (2023). Molecular hydrogen in seawater supports growth of diverse marine bacteria. Nat. Microbiol. 8, 581–595. doi: 10.1038/s41564-023-01322-0
Laso-Pérez, R., Wu, F., Crémière, A., Speth, D. R., Magyar, J. S., Zhao, K., et al. (2023). Evolutionary diversification of methanotrophic ANME-1 archaea and their expansive virome. Nat. Microbiol. 8, 231–245. doi: 10.1038/s41564-022-01297-4
Lay, J.-J., Li, Y.-Y., and Noike, T. (1998). Interaction between homoacetogens and methanogens in lake sediments. J. Ferment. Bioeng. 86, 467–471. doi: 10.1016/s0922-338x(98)80153-0
Lever, M. A., Alperin, M. J., Hinrichs, K. U., and Teske, A. (2023). Zonation of the active methane-cycling community in deep subsurface sediments of the Peru trench. Front. Microbiol. 14:1192029. doi: 10.3389/fmicb.2023.1192029
Lever, M. A., and Teske, A. P. (2015). Diversity of methane-cycling archaea in hydrothermal sediment investigated by general and group-specific PCR primers. Appl. Environ. Microbiol. 81, 1426–1441. doi: 10.1128/AEM.03588-14
Lin, Y. S., Heuer, V. B., Goldhammer, T., Kellermann, M. Y., Zabel, M., and Hinrichs, K. U. (2012). Towards constraining H2 concentration in subseafloor sediment: a proposal for combined analysis by two distinct approaches. Geochim. Cosmochim. Acta 77, 186–201. doi: 10.1016/j.gca.2011.11.008
Liu, C., Cui, Y., Li, X., and Yao, M. (2021). Microeco: an R package for data mining in microbial community ecology. FEMS Microbiol. Ecol. 97:255. doi: 10.1093/femsec/fiaa255
Lovley, D. R., Dwyer, D. F., and Klug, M. J. (1982). Kinetic analysis of competition between sulfate reducers and methanogens for hydrogen in sediments. Appl. Environ. Microbiol. 43, 1373–1379. doi: 10.1128/aem.43.6.1373-1379.1982
Lovley, D. R., and Goodwin, S. (1988). Hydrogen concentrations as an Indicator of the predominant terminal Electron-accepting reactions in aquatic sediments. Geochim. Cosmochim. Acta 52, 2993–3003. doi: 10.1016/0016-7037(88)90163-9
Maltby, J., Steinle, L., Loscher, C. R., Bange, H. W., Fischer, M. A., Schmidt, M., et al. (2018). Microbial methanogenesis in the sulfate-reducing zone of sediments in the Eckernforde Bay SW Baltic Sea. Biogeosciences 15, 137–157. doi: 10.5194/bg-15-137-2018
Middelburg, J. J., Vlug, T., and Vandernat, F. J. W. A. (1993). Organic-matter mineralization in marine systems. Glob. Planet. Chang. 8, 47–58. doi: 10.1016/0921-8181(93)90062-S
Namirimu, T., Yu, J., Yang, J.-A., Yang, S.-H., Kim, Y. J., and Kwon, K. K. (2022). Alkalibacter rhizosphaerae sp. nov., a CO-utilizing bacterium isolated from tidal flat sediment, and emended description of the genus Alkalibacter. Int. J. Syst. Evol. Microbiol. 72:5495. doi: 10.1099/ijsem.0.005495
Neretin, L. N., Schippers, A., Pernthaler, A., Hamann, K., Amann, R., and Jørgensen, B. B. (2003). Quantification of dissimilatory (bi)sulphite reductase gene expression in Desulfobacterium autotrophicum using real-time RT-PCR. Environ. Microbiol. 5, 660–671. doi: 10.1046/j.1462-2920.2003.00452.x
Nobu, M. K., Narihiro, T., Kuroda, K., Mei, R., and Liu, W. T. (2016). Chasing the elusive Euryarchaeota class WSA2: genomes reveal a uniquely fastidious methyl-reducing methanogen. ISME J. 10, 2478–2487. doi: 10.1038/ismej.2016.33
Novelli, P. C., Michelson, A. R., Scranton, M. I., Banta, G. T., Hobbie, J. E., and Howarth, R. W. (1988). Hydrogen and acetate cycling in two sulfate-reducing sediments - Buzzards Bay and town cove mass. Geochim. Cosmochim. Acta 52, 2477–2486. doi: 10.1016/0016-7037(88)90306-7
Novelli, P., Scranton, M. I., and Michener, R. H. (1987). Hydrogen distributions in marine sediments. Limnol. Oceanogr. 32, 565–576.
Oremland, R. S., and Polcin, S. (1982). Methanogenesis and sulfate reduction: competitive and noncompetitive substrates in estuarine sediments. Appl. Environ. Microbiol. 44, 1270–1276. doi: 10.1128/aem.44.6.1270-1276.1982
Perner, M., Hansen, M., Seifert, R., Strauss, H., Koschinsky, A., and Petersen, S. (2013). Linking geology, fluid chemistry, and microbial activity of basalt- and ultramafic-hosted deep-sea hydrothermal vent environments. Geobiology 11, 340–355. doi: 10.1111/gbi.12039
Perner, M., Hentscher, M., Rychlik, N., Seifert, R., Strauss, H., and Bach, W. (2011). Driving forces behind the biotope structures in two low-temperature hydrothermal venting sites on the southern mid-Atlantic ridge. Environ. Microbiol. Rep. 3, 727–737. doi: 10.1111/j.1758-2229.2011.00291.x
Perner, M., Wallmann, K., Adam-Beyer, N., Hepach, H., Laufer-Meiser, K., Böhnke, S., et al. (2022). Environmental changes affect the microbial release of hydrogen sulfide and methane from sediments at Boknis Eck (SW Baltic Sea). Front. Microbiol. 13:1096062. doi: 10.3389/fmicb.2022.1096062
Pernthaler, A., Pernthaler, J., and Amann, R. (2002). Fluorescence in situ hybridization and catalyzed reporter deposition for the identification of marine bacteria. Appl. Environ. Microbiol. 68, 3094–3101. doi: 10.1128/AEM.68.6.3094-3101.2002
Postgate, J. R., and Campbell, L. L. (1966). Classification of Desulfovibrio species, the nonsporulating sulfate-reducing bacteria. Bacteriol. Rev. 30, 732–738. doi: 10.1128/br.30.4.732-738.1966
Price, M. N., Dehal, P. S., and Arkin, A. P. (2010). FastTree 2--approximately maximum-likelihood trees for large alignments. PLoS One 5:e9490. doi: 10.1371/journal.pone.0009490
Quast, C., Pruesse, E., Yilmaz, P., Gerken, J., Schweer, T., Yarza, P., et al. (2013). The SILVA ribosomal RNA gene database project: improved data processing and web-based tools. Nucleic Acids Res. 41, D590–D596. doi: 10.1093/nar/gks1219
Ragsdale, S. W., and Pierce, E. (2008). Acetogenesis and the wood-Ljungdahl pathway of CO(2) fixation. Biochim. Biophys. Acta 1784, 1873–1898. doi: 10.1016/j.bbapap.2008.08.012
Rouwendaal, S. E., Birgel, D., Grossi, V., Aloisi, G., Guibourdenche, L., Labrado, A. L., et al. (2023). Two modes of gypsum replacement by carbonate and native sulfur in the Lorca Basin SE Spain. Front. Earth Sci. 11:1153415. doi: 10.3389/feart.2023.1153415
Sako, Y., Takai, K., Ishida, Y., Uchida, A., and Katayama, Y. (1996). Rhodothermus obamensis sp. nov., a modern lineage of extremely thermophilic marine bacteria. Int. J. Syst. Bacteriol. 46, 1099–1104. doi: 10.1099/00207713-46-4-1099
Sánchez-Andrea, I., Guedes, I. A., Hornung, B., Boeren, S., Lawson, C. E., Sousa, D. Z., et al. (2020). The reductive glycine pathway allows autotrophic growth of Desulfovibrio desulfuricans. Nat. Commun. 11:5090. doi: 10.1038/s41467-020-18906-7
Smetacek, V., von Bodungen, B., Knoppers, B., Peinert, R., Pollehne, F., Stegmann, P., et al. (1984). Seasonal stages characterizing the annual cycle of an inshore pelagic system. Rapp. Proces Verbaux Reun. Cons. Int. 183, 126–135.
Springer, E., Sachs, M. S., Woese, C. R., and Boone, D. R. (1995). Partial gene sequences for the a subunit of methyl-coenzyme M reductase (mcrI) as a phylogenetic tool for the family Methanosarcinaceae. Int. J. Syst. Evol. Microbiol. 45, 554–559. doi: 10.1099/00207713-45-3-554
Tengölics, R., Meszaros, L., Gyori, E., Doffkay, Z., Kovacs, K. L., and Rakhely, G. (2014). Connection between the membrane electron transport system and Hyn hydrogenase in the purple sulfur bacterium, Thiocapsa roseopersicina BBS. Biochim. Biophys. Acta 1837, 1691–1698. doi: 10.1016/j.bbabio.2014.07.021
Timmers, P. H., Gieteling, J., Widjaja-Greefkes, H. C., Plugge, C. M., Stams, A. J., Lens, P. N., et al. (2015). Growth of anaerobic methane-oxidizing archaea and sulfate-reducing bacteria in a high-pressure membrane capsule bioreactor. Appl. Environ. Microbiol. 81, 1286–1296. doi: 10.1128/AEM.03255-14
Timmers, P. H., Welte, C. U., Koehorst, J. J., Plugge, C. M., Jetten, M. S., and Stams, A. J. (2017). Reverse Methanogenesis and respiration in Methanotrophic Archaea. Archaea 2017, 1654237–1654222. doi: 10.1155/2017/1654237
Treude, T., Krüger, M., Boetius, A., and Jørgensen, B. B. (2005). Environmental control on anaerobic oxidation of methane in the gassy sediments of Eckernförde Bay (German Baltic). Limnol. Oceanogr. 50, 1771–1786. doi: 10.4319/lo.2005.50.6.1771
Vandieken, V., Finke, N., and Thamdrup, B. (2014). Hydrogen, acetate, and lactate as electron donors for microbial manganese reduction in a manganese-rich coastal marine sediment. FEMS Microbiol. Ecol. 87, 733–745. doi: 10.1111/1574-6941.12259
Vanwonterghem, I., Evans, P. N., Parks, D. H., Jensen, P. D., Woodcroft, B. J., Hugenholtz, P., et al. (2016). Methylotrophic methanogenesis discovered in the archaeal phylum Verstraetearchaeota. Nat. Microbiol. 1:16170. doi: 10.1038/nmicrobiol.2016.170
Wallmann, K., Diesing, M., Scholz, F., Rehder, G., Dale, A. W., Fuhr, M., et al. (2022). Erosion of carbonate-bearing sedimentary rocks may close the alkalinity budget of the Baltic Sea and support atmospheric CO2 uptake in coastal seas. Front. Mar. Sci. 9:968069. doi: 10.3389/fmars.2022.968069
Wang, S., Lu, Q., Liang, Z., Yu, X., Lin, M., Mai, B., et al. (2023). Generation of zero-valent sulfur from dissimilatory sulfate reduction in sulfate-reducing microorganisms. Proc. Natl. Acad. Sci. 120:e2220725120. doi: 10.1073/pnas.2220725120
Wolin, M. J. (1976). “Interactions between H2-producing and methane-producing species” in Microbial formation and utilization of gases. eds. H. G. Schlegel, G. Gottschalk, and N. Pfennig (Göttingen: Goltze), 141–150.
Zhou, Z., Pan, J., Wang, F., Gu, J.-D., and Li, M. (2018). Bathyarchaeota: globally distributed metabolic generalists in anoxic environments. FEMS Microbiol. Rev. 42, 639–655. doi: 10.1093/femsre/fuy023
Keywords: hydrogen oxidation, hydrogen consumption, marine sediments, methane metabolism, methanogenesis, AOM, ANME, SRB
Citation: Adam-Beyer N, Deusner C, Schmidt M and Perner M (2025) Microbial hydrogen oxidation potential in seasonally hypoxic Baltic Sea sediments. Front. Microbiol. 16:1565157. doi: 10.3389/fmicb.2025.1565157
Edited by:
Danny Ionescu, Technical University of Berlin, GermanyReviewed by:
Julia Kurth, University of Marburg, GermanyMarcus Elvert, University of Bremen, Germany
Sai Xu, Nanjing University of Science and Technology, China
Copyright © 2025 Adam-Beyer, Deusner, Schmidt and Perner. This is an open-access article distributed under the terms of the Creative Commons Attribution License (CC BY). The use, distribution or reproduction in other forums is permitted, provided the original author(s) and the copyright owner(s) are credited and that the original publication in this journal is cited, in accordance with accepted academic practice. No use, distribution or reproduction is permitted which does not comply with these terms.
*Correspondence: Mirjam Perner, bXBlcm5lckBnZW9tYXIuZGU=