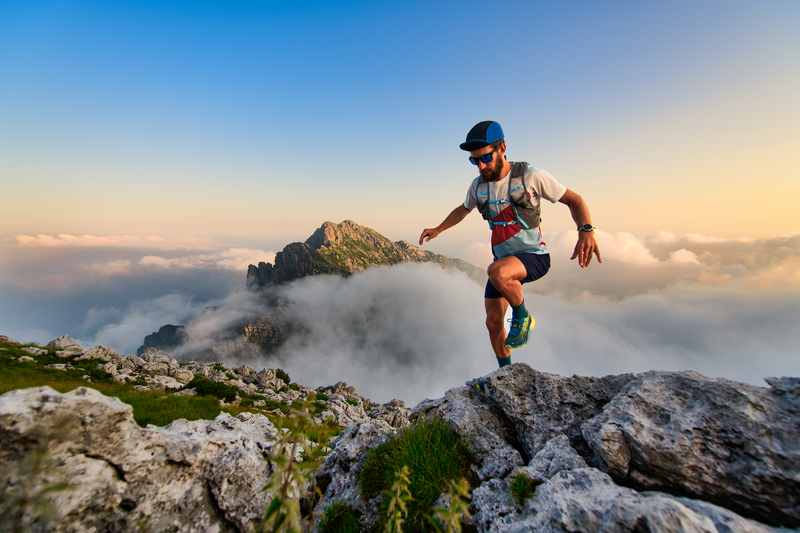
95% of researchers rate our articles as excellent or good
Learn more about the work of our research integrity team to safeguard the quality of each article we publish.
Find out more
ORIGINAL RESEARCH article
Front. Microbiol. , 24 March 2025
Sec. Phage Biology
Volume 16 - 2025 | https://doi.org/10.3389/fmicb.2025.1550534
This article is part of the Research Topic Innovations in Phage Biocontrol: Advancing Technology and Applications View all articles
Introduction: Antimicrobial resistance (AMR) is a major public health threat, driving the need for alternative treatments such as phage therapy. However, bacterial defense mechanisms, often regulated by the quorum sensing (QS) network and encoded in genomic islands (GIs), can generate phage-resistant mutants. Understanding these resistance mechanisms is essential for optimizing phage therapy.
Methods: This study analyzed 48 Klebsiella pneumoniae strains to identify pathogenicity islands (PAIs) containing anti-phage defense (APD) proteins. We constructed a knockout strain lacking the cyclase gene from the type II CBASS defense systems present in PAIs to investigate QS regulation and its role in cell viability. The LAMP-CRISPR-Cas13a technique was used to confirm gene knockout and to detect the main cyclase in type I CBASS systems, i.e., APECO1.
Results: A total of 309 pathogenicity islands (PAIs), containing 22.1% of anti-phage defense (APD) proteins, were identified. Type I and II CBASS APD systems were also detected in the genome of the 48, K. pneumoniae strains, and only two type II CBASS systems were located in PAIs. Alluding to these defense mechanisms, the QS revealed to be involved in the regulation of the type II CBASS systems contained in PAIs. Finally, the LAMP-CRISPR-Cas13a technology successfully detected the main cyclases habored in type I and II CBASS systems, respectively.
Discussion: The study findings highlight the regulatory role of the QS network in APD systems. Notably, this is the first study to develop an innovative biotechnological application for the LAMP-CRISPR-Cas13a rapid-technique (<2 h), thereby helping to optimize phage therapy by detecting bacterial resistance mechanisms and predicting the potential inefficacy of therapeutic phages and thus improving patient prognosis.
Antimicrobial resistance (AMR) is one of the major current global threats to public health, and it has been predicted that as many as 10 million people could die annually as a result of AMR by 2050 (Murray et al., 2022; Jonas et al., 2017; Tang et al., 2023). Worryingly, the 2022 Global Antimicrobial Resistance and Use Surveillance System (GLASS) report raised concerns about extremely high resistance rates among prevalent bacterial pathogens (Ajulo and Awosile, 2024). In this context, carbapenem-resistant Klebsiella pneumoniae is highlighted as a key pathogen against which common antibiotics are no longer effective. This critical situation has led to the increased administration of last-resort drugs, with resistance to these drugs increasing rapidly (Reyes et al., 2019). K. pneumoniae is included in the Bacterial Priority Pathogens List (BPPL) compiled by the World Health Organization (WHO), implying that measures for prevention and appropriate treatment of infections are crucial (WHO, 2024).
Regarding the scarcity of effective treatments for AMR infections, due to the emergence of resistance to all antibiotics used in clinical settings, therapeutic innovations such as phage therapy are becoming more important (Bleriot et al., 2023a). Phage therapy, the use of bacteriophages (phages, i.e., viruses that infect bacteria) to treat bacterial infections, has several advantages over other alternative treatments (Lin et al., 2017; Pacios et al., 2020; Singh et al., 2023). However, contact between bacteria and phage leads to the so-called phage-host arms race, and one of the greatest disadvantages of phage therapy is the frequent and rapid generation of phage-resistant bacterial mutants during treatment (Georjon and Bernheim, 2023; Wang and Zhang, 2023). Anti-phage defense (APD) mechanisms in bacteria are mainly based on (i) adsorption resistance, (ii) blockage of the phage infection once adsorbed, (iii) elimination of the viral genetic material, i.e., restriction-modification (RM) and clustered regularly interspaced short palindromic repeat (CRISPR)-associated protein (CRISPR-Cas) systems, and (iv) other mechanisms, including toxin–antitoxin (TA) systems and abortive infection (Abi) systems such as cyclic oligonucleotide-based anti-phage signaling systems (CBASS) (Wang and Zhang, 2023; Fillol-Salom et al., 2022).
APD systems are commonly contained in clusters of genes within a bacterial genome, known as genomic islands (GIs). These genes appear to have been acquired through horizontal gene transfer (HGT), which rapidly spreads genes that improve bacterial fitness and adaptation (Audrey et al., 2023; Dobrindt et al., 2004; Ramsay et al., 2023). The genes harbored in GIs are classified as pathogenicity islands (PAIs), metabolic islands (MIs), symbiotic islands (SIs), and resistance islands (RIs) (Ramsay et al., 2023; Leamon and Rothberg, 2009). Although APD systems are frequently clustered together in bacterial genomes in defense islands (DIs), recent findings have revealed the essential role of PAIs in holding and spreading bacterial defense systems. This highlights the importance of studying APD proteins to improve our understanding of bacterial immunity and to develop therapeutic strategies (Fillol-Salom et al., 2022; Doron et al., 2018; Oliveira et al., 2016). Some APD systems harbored by PAIs, such as CBASS and RM systems, protect bacteria from phage infections and also seem to influence bacterial competition and HGT, ultimately shaping microbial communities (Doron et al., 2018; Oliveira et al., 2016).
Furthermore, phages themselves can mobilize PAIs that contain immune systems, as shown in Staphylococcus aureus, in which phage-inducible chromosomal islands (PICIs) have been shown to facilitate the spread of APD genes (Humphrey et al., 2021). This suggests that PAIs act as key genetic reservoirs for bacterial defense, reinforcing the importance of studying their role in the evolution and adaptation of pathogens, as well as their impact on the efficacy of phage therapy (Fillol-Salom et al., 2022; Humphrey et al., 2021).
Among the APD systems, the CBASS systems are a family of defense systems composed of an oligonucleotide cyclase, which generates signaling cyclic oligonucleotides in response to phage infection. These cyclic oligonucleotides activate an effector that promotes cell death before phage replication is completed, therefore preventing the spread of phages to nearby cells (Millman et al., 2020). Diverse CBASS defense systems have been identified and are classified according to the composition of the CBASS operon, the activity of the effector protein, and the signaling molecule produced by the oligonucleotide cyclase. Type I and II systems are the most common. The type I CBASS system consists of an oligonucleotide cyclase and an effector protein, the so-called minimal configuration, and the effector activity is mainly based on the formation of pores in the membrane. In addition to the cyclase–effector framework, the type II CBASS system has two ancillary genes (cap2 and cap3) that are involved in expanding the range of phages against which it acts, and the effector protein is usually a phospholipase (Millman et al., 2020).
Importantly, many bacteria control the expression of several of these defense mechanisms, such as CBASS systems, through the quorum sensing (QS) network, which is defined as a cellular communication process mediated by signaling molecules called autoinducers (AIs) (Barrio-Pujante et al., 2024; Díaz, 2017). The QS of K. pneumoniae mainly relies on the use of autoinducer-2 (AI-2). AI-2 consists of a group of 4,5-dihydroxy-2,3-pentanedione (DPD) derivatives that can rapidly convert to one another, are involved in interspecies communication, and are capable of detecting other AIs in the medium such as AI-1 (exogenous N-Acyl homoserine lactones or AHLs) (Díaz, 2017; Pacheco et al., 2021). In a previous study, the inhibition of QS led to a reduction in the expression of CBASS proteins, which meant an improvement in phage infection, bringing to light the role that QS plays in controlling bacterial APD (Barrio-Pujante et al., 2024). Under this premise, the strategy of counteracting CBASS systems may be a useful way of enhancing the therapeutic outcomes of phages in clinical settings.
Detection of the anti-phage systems harbored in a targeted clinical strain can be used to overcome bacterial resistance to phage, hence enabling the application of personalized phage therapy treatments that improve patient prognosis. In this context, CRISPR-Cas systems are potentially promising tools, as they have yielded successful results regarding the development of detection protocols with high levels of sensitivity and specificity (Kellner et al., 2019; Chen et al., 2018; Zahra et al., 2023). These diagnostic techniques have previously been used to detect several microorganisms and also resistance genes (Ortiz-Cartagena et al., 2022, 2023; Curti et al., 2020; Wu et al., 2021).
In this study, we analyzed the APD genes contained in the PAIs detected in the genome of 48 K. pneumoniae isolates. We also conducted an epidemiological study of the CBASS systems located in these strains, focusing on the QS-based regulation of type II CBASS APD system in a carbapenemase-producing K. pneumoniae clinical strain. Finally, we broadened the range of biotechnological applications of CRISPR-Cas technology, by applying our previously developed diagnostic protocol based on the CRISPR-Cas13a system to detect anti-phage resistance genes in bacteria (Ortiz-Cartagena et al., 2022, 2023).
Genomes in contigs of 48 K. pneumoniae clinical isolates (Table 1), previously sequenced by Illumina MiSeq (BioProject codes listed in Table 1; Bleriot et al., 2023b; Grundmann et al., 2017), were studied. Contigs were assembled through an aleatory number of “Ns” to compress the genomes into single sequences with the Linux OS. In order to obtain files in GenBank format, the genomes were then reannotated using the PROKKA software tool (Seemann, 2014), also with the Linux OS.
In order to predict the GIs harbored in the genomes from 48 K. pneumoniae clinical isolates, the genomes were analyzed using Genomic Island Prediction (GIPSy) software (Soares et al., 2016) in Windows and applying standard values in steps 2–7, following the GIPSy manual (Version 1.1.2). The GIs were classified into PAIs, MIs, SIs, and RIs by using GIPSY software, with the genomes in GenBank format files as input and the genome of K. pneumoniae strain MGH78578 (GenBank: NC_009648) as the reference genome. The sequences of the predicted PAIs were extracted from their respective genomes; the “Ns” were removed, and the sequences were annotated using the PROKKA software tool, in all cases with the Linux OS. The fasta files of these protein sequences were analyzed, using the HHMER (Finn et al., 2011) and HHPred (Söding et al., 2005) servers, along with the NCBI conserved domains database (Wang et al., 2023), to identify and thus manually classify the proteins contained in them, as follows: (i) metabolic proteins; (ii) defense, resistance, and virulence proteins (DRV); (iii) DNA metabolism proteins; (iv) MGE; and (v) unknown proteins. APD genes were also detected by PADLOC, a web server for the identification of antiviral defense systems in microbial genomes, using GenBank format files as input; the defense sequences were then analyzed using HHMER and HHPred servers, as previously described (Payne et al., 2022). For the servers and database used in this bioinformatical analysis, standard values and default parameters were applied.
The K. pneumoniae strain 10ST340-VIM1 was selected from the bacterial strain collection (Table 1), to construct a knockout of the cyclase gene (10ST340-VIM1Δcyclase) present in a type II CBASS system, by a protocol using the Escherichia coli λ-Red system (Huang et al., 2014). This protocol is based on homologous recombination mediated by the λ-Red system of the λ phage that is used to eliminate specific genes in E. coli strains. In order to adapt it to K. pneumoniae, it was applied with some modifications. The homologous regions, required for the recombination step in the target genome, were of length 300 bp, and therefore, the length of the knockout cassette ends was increased to 300 bp. Plasmids and primers used for this goal are listed in Table 2.
Bacterial cultures were grown in Luria-Bertani (LB) broth (10 g/L tryptone, 5 g/L yeast extract, and 10 g/L NaCl) or on LB agar (LB broth supplemented with 20 g/L of agar). Hygromycin and apramycin were used to select transformant strains at concentrations of 100 and 50 μg/mL, respectively.
The cyclase gene was deleted from the K. pneumoniae 10ST340-VIM1 strain by the above-mentioned method (Huang et al., 2014). Briefly, the knockout cassette containing the apramycin resistance gene was electroporated into the K. pneumoniae 10ST340-VIM1 strain already transformed with plasmid pACBSR-Hyg, which expresses the λ-Red system and a hygromycin selection marker. Bacteria were incubated overnight on LB agar supplemented with apramycin, and correct insertion of the knockout cassette was confirmed by PCR (F_300up and B_Conf_Int). The K. pneumoniae 10ST340-VIM1 derivative colonies were grown on LB agar with apramycin (50 μg/mL), at 37°C for 3 days, before being incubated on LB agar with apramycin (150 μg/mL) and LB agar with hygromycin (100 μg/mL), overnight at 37°C, to test colonies for loss of the helper plasmid pACBSR-Hyg. For removal of resistance markers, positive colonies were electroporated with pFLP-Hyg and grown overnight at 30°C. The mutant colonies were then incubated at 43°C to remove the apramycin resistance from the inserted knockout cassette. Apramycin-susceptible mutant colonies were selected to confirm deletion of the cyclase gene by PCRs (F_300up/B_300down and F_Conf_Int/B_Conf_Int) and Sanger sequencing (Macrogen®).
Expression of effector I was quantified in the presence of 20 μM of AHL C6-HSL in both the 10ST340-VIM1 and 10ST340-VIM1Δcyclase K. pneumoniae strains. For this purpose, 40 μL of an overnight culture of the corresponding K. pneumoniae strain was inoculated in 4 mL flasks of LB-broth containing 20 μM C6-HSL (two biological samples of each strain). The flasks were then incubated at 37°C, with shaking at 180 rpm, until the optical density measured at a wavelength of 600 nm (OD600) reached 0.3.
Duplicate aliquots of 1 mL were removed from each flask for subsequent RNA extraction with the High Pure RNA Isolation Kit (Roche). Extraction was conducted following the manufacturer's instructions. The RNA extracted from each sample was measured with a Nanodrop spectrophotometer (NanoDrop Technologies) and adjusted to 50 ng/μL with nuclease-free water for use in qRT-PCR (LightCycler® 480). Specific primers and the corresponding Universal ProbeLibrary (UPL) probe for both the effector I and recA (Gomes et al., 2018) genes were designed using ProbeFinder software (Roche) and are listed in Table 3. This assay was conducted with the LightCycler® 480 Control Kit (Roche). Student's t-test (GraphPad Prism 9.0.0) was used to determine any statistically significant differences (p-value < 0.05) in gene expression.
Cell proliferation and cell viability levels in both 10ST340-VIM1 and 10ST340-VIM1Δcyclase K. pneumoniae strains cultured in the presence of 20 μM of C6-HSL (to overexpress the CBASS) were determined by an assay based on the WST-1 reagent, a red tetrazolium salt that turns yellow when cleaved to formazan by mitochondrial dehydrogenases (WST-1 Assay Protocol for Cell Viability, MERCK®). Briefly, overnight cultures of the corresponding K. pneumoniae strains were diluted 1:100 in LB broth (two biological samples of each strain) and the flasks were incubated at 37°C, with shaking at 180 rpm, until the OD600 reached 0.2. The cultures were then diluted to OD600 0.02 and added to the wells of 96-well plates and made up to a final volume of 100 μL supplemented with 20 μM of C6-HSL. The plates were incubated at 37°C, with shaking at 180 rpm for 24 h, and the OD600 was again read in a microplate reader (NanoQuant). Dilutions were made in new 96-well plates to adjust the OD600 to 0.02, and 10 μL of WST-1 reagent was then added to the plates, which were incubated at 37°C in the absence of light for 1.5 h. Cell proliferation and viability were quantified at OD480. Student's t-test (GraphPad Prism 9.0.0) was used to determine any statistically significant differences (p-value < 0.05) in proliferation levels. In this assay, negative controls consisted of LB without strains nor C6-HSL supplementation.
The gene sequences for cyclase APECO1 and type II CBASS system cyclase of each K. pneumoniae strain were assembled using Prokka software (Seemann, 2014). The target sequences were analyzed in silico by aligning each of the sequences of both kinds of cyclases using MEGA11 software (Tamura et al., 2021). For both types of detection, crRNA molecules were designed to target conserved regions, preferably targeting a C/G nucleotide for crRNA-Cas13a complex stability (Table 4). Thus, three pairs of LAMP primers were designed using PrimerExplorer V5 software (F3/B3, FIP/BIP, Floop/Bloop) in order to amplify the regions containing the targeted sequences (Table 4). The FIP LAMP primers included the T7 polymerase promoter in their sequences for the subsequent transcription step. The RNA reporter molecules, designed for signal amplification, harbor a single isomer derivative of fluorescein modification (FAM) at the 5′ extreme and a biotin molecule at the 3′ extreme.
The LAMP-CRISPR-Cas13a detection protocol, previously described by our research group (Ortiz-Cartagena et al., 2022, 2023), was used to detect the strains harboring type I and type II CBASS systems from among the 48 K. pneumoniae isolates. In order to identify type I CBASS systems, the LAMP-CRISPR-Cas13a technique was targeted to the cyclase APECO1 gene, located in several type I CBASS systems; the presence of type II CBASS systems was determined by identifying the cyclase gene contained in both type II CBASS systems detected in the PAIs. For this purpose, genomic DNA was extracted from the respective strains by the proteinase K-heat inactivation protocol (PK-HID) (Genoud et al., 2021). Briefly, a LAMP DNA amplification reaction [WarmStart® LAMP Kit (DNA and RNA), NEB, Ipswich, MA, USA] was performed for cyclase gene amplification, containing 5 μL of extraction, 12.5 μL of WarmStart LAMP 2x Master Mix, and 2.5 μL of Primer Mix 10x (FIP/BIP 16 μM, F3/B3 2 μM, LOOPF/LOOPB 4 μM, stock 10X) adjusted to a final volume of 25 μL with RNase-free water and incubated at 65°C for 1 h. Following, a Leptotrichia wadei, LwaCas13a, endonuclease [GenCRISPR™ Cas13a (C2c2) Nuclease, GenScript] detection was assessed, using 2 μL of cleavage buffer 10X [GenCRISPR™ Cas13a (C2c2) Nuclease, GenScript], 0.5 μL of dNTPs (HiScribe™ T7 Quick High Yield RNA Synthesis Kit, NEB), 0.5 μL of T7 polymerase (HiScribe™ T7 Quick High Yield RNA Synthesis Kit, NEB), 20 U of RNase murine inhibitor (NEB), 25 nM of LwaCas13a endonuclease, 50 nM of crRNA (IDT), 1,000 nM of reporter (GenScript), and 5 μL of LAMP amplicon, adjusted to a final volume of 20 μL with RNase-free water and incubated at 37°C for 30 min (Table 4). Negative controls for both amplification and detection reactions were carried out using RNase-free water instead of sample. Finally, the test results were revealed using HybriDetect lateral flow test strips (Milenia Biotec, Giessen, Germany), mixing 20 μL of collateral cleavage activity-based detection product with 80 μL of assay buffer supplemented with 5% polyethylene glycol in a 96-well plate where strip tests were placed. The test results are visible to the naked eye after 2–3 min.
Analysis of the genomes of 48 clinical isolates of K. pneumoniae led to the identification of a total of 309 regions with a high probability of being PAIs. Among these, 17 PAIs were detected in more than one K. pneumoniae strain, and the sequences were analyzed bioinformatically to determine the function of the proteins harbored in them, as greater spread could be related to important roles in bacterial fitness and adaptation (Figure 1A). The results showed that 31% of the proteins were related to MGE, 26% to DRV, 13% to DNA metabolism, 11% to metabolism, and 19% to unknown function. Furthermore, 24.4% of these proteins were involved in APD, and 46% of these corresponded to the DRV functional group, but also 25% to MGE, 12% to DNA metabolism, 9% to metabolism, and 8% were of unknown function. In particular, 90.5% of the APD proteins in MGE were proteins of phage origin. Finally, the organization of the APD proteins in the different APD systems revealed that most of these proteins are involved in surface modification (28.6%), TA (22.9%), and RM (21.4%) systems. We also identified several complete defense systems: 12 surface modifications, 2 TA, 2 RM, 2 CBASS, 1 Gabija, 1 dynamin, 1 Thoeris, and 3 putative systems (Figure 1B).
Figure 1. Results of the bioinformatic analysis of proteins contained in 17 K. pneumoniae PAIs. (A) Classification of proteins in functional groups, showing a number of proteins (left) and percentages of proteins (right) in each functional group of PAIs; the number of K. pneumoniae strains containing each PAI is also shown (right). (B) Global percentages of functional groups of proteins in PAIs (left); ADP proteins classified in the different functional groups (right); and a table containing the APD systems identified in these PAIs, their prevalence, and the number of the completed systems found (below).
The complete genome of the K. pneumoniae strains with CBASS systems harbored in PAIs, 10ST340-VIM1 and K3325S were exhaustively analyzed by PADLOC, along with the genomes from the other 46 K. pneumoniae strains, to identify CBASS systems not contained in PAIs. The genomic structure of the CBASS systems in both 10ST340-VIM1 and K3325S strains was similar (Table 5), and only these two CBASS systems (11%) were located in PAIs (Table 6). Regarding the classification of the CBASS systems detected, 63% belonged to type I and 37% to type II. None of the type I CBASS systems were contained in PAIs, and two of the three type II CBASS systems were located in PAIs of the 10ST340-VIM1 and K3325S strains. Analysis of the components of these CBASS systems revealed that the cyclase APECO1 gene was present in 13 of the 15 (87%) type I CBASS systems detected but not in any of the type II CBASS systems. Regarding the effector protein, the pore-forming function effector appeared in all type I and in one of three type II (33%) CBASS systems; however, phospholipase and nuclease effectors were only located in type II CBASS systems (Table 6). Finally, the cyclic-nucleotide signal was GMPAMPc in all of the CBASS systems analyzed (Table 6).
In order to study the regulatory role of QS in the expression of the type II CBASS system, we constructed a 10ST340-VIM1Δcyclase K. pneumoniae strain, and deletion of the gene was consecutively confirmed by the LAMP-CRISPR-Cas13a technology (Figure 2). A quantitative study of the type II CBASS system expression under QS activation conditions was conducted by measuring effector I gene expression levels in the presence of the AHL C6-HSL, in both 10ST340-VIM1 and 10ST340-VIM1Δcyclase K. pneumoniae strains. The results revealed overexpression of the APD system in the 10ST340-VIM1 K. pneumoniae strain (1.23) (Table 7). By contrast, the level of expression of the type II CBASS system in the 10ST340-VIM1Δcyclase K. pneumoniae strain (0.92) was similar to that of the recA housekeeping gene (Table 7).
Figure 2. Results of the cyclase gene knockout construction by λ-Red protocol. Confirmation of the deletion of the cyclase gene in two mutants by LAMP-CRISPR-Cas13a protocol.
The role of CBASS in bacterial viability was studied using an assay based on the WST-1 reagent. With this aim, cellular respiration levels in the 10ST340-VIM1 and 10ST340-VIM1Δcyclase K. pneumoniae strains were compared, using the AHL C6-HSL to induce the CBASS system. There was no significant difference in the viability of the 10ST340-VIM1Δcyclase K. pneumoniae strain with and without the autoinducer C6-HSL (Figure 3B), in contrast to the 10ST340-VIM1 K. pneumoniae strain, which showed a significant reduction in cell respiration when treated with AHL C6-HSL (Figure 3A). By contrast, the viability of the 10ST340-VIM1 K. pneumoniae strain was significantly higher than that of the 10ST340-VIM1Δcyclase K. pneumoniae strain (Figure 3C). Finally, the viability of both strains was very similar in the presence of AHL C6-HSL, except in one of the 10ST340-VIM1 K. pneumoniae strains that grew more than the other 10ST340-VIM1 and 10ST340-VIM1Δcyclase K. pneumoniae strains (Figure 3D).
Figure 3. Cell viability in the presence and absence of AHL C6-HSL in both 10ST340-VIM1 and 10ST340-VIM1Δcyclase K. pneumoniae strains. (A) Comparison of cell viability in two 10ST340-VIM1 biological replicates. (B) Comparison of cell viability in two 10ST340-VIM1Δcyclase biological replicates. (C) Comparison of cell viability in the 10ST340-VIM1 and 10ST340-VIM1Δcyclase strains in the absence of AHL C6-HSL. (D) Comparison of cell viability in the 10ST340-VIM1 and 10ST340-VIM1Δcyclase strains in the presence of AHL C6-HSL. WT: 10ST340-VIM1 K. pneumoniae strain; KO: 10ST340-VIM1Δcyclase K. pneumoniae strain; HEX: AHL C6-HSL; Numbers on the condition axis refer to biological replicates. *Statistically significant difference.
The LAMP-CRISPR-Cas13a technique produced very good results regarding the detection of the cyclase APECO1 present in several type I CBASS systems (n = 13) and the cyclase contained in the type II CBASS systems located in PAIs (n = 2). The technique was able to detect the cyclase APECO1 gene in all of the K. pneumoniae strains containing it, except strain number 1. It also produced negative results for the K. pneumoniae strains without CBASS systems and with type II CBASS systems and for the strains containing type I CBASS systems with a different cyclase (Figure 4A). Importantly, the application of a bioinformatic assay to the type I CBASS system in strain 1 indicated that the cyclase APECO1 located in this system had only seven nucleotides in common with the crRNA molecule, required for Cas13a detection. In addition, the technique successfully detected the presence of the cyclase in the type II CBASS systems contained in PAIs in the 10ST340-VIM1 and K3325S K. pneumoniae strains (Figure 4B). Based on the results obtained, we estimated that the technique exhibits 92.3% sensitivity and 100% specificity [99% confidence interval (CI)] for the detection of the cyclase APECO1 gene as well as 100% sensitivity and specificity [99% confidence interval (CI)] for the identification of the cyclase gene contained in type II CBASS systems located in PAIs (Figure 4C).
Figure 4. Results of the detection of type I and II CBASS systems using the LAMP-CRISPR-Cas13a technology. (A) Strips for detection of the K. pneumoniae strains harboring the APECO1 gene; (B) Strips for detection of the K. pneumoniae strains containing the cyclase gene in type II CBASS system; (C) APECO1 gene detection (A) and table showing the specificity and sensitivity of the LAMP-CRISPR-Cas13a technique, determined by processing data in (A, B).
The results of the in silico analysis support the possibility that GIs are essential elements in the HGT for the optimization of bacterial fitness and adaptation, as more than 50% of the genes found in the 309 PAIs were MGE or were involved in DRV (Figure 1A). More than 20% of the proteins contained in the 309 PAIs were related to a single defense mechanism, that is, APD (Figure 1B; Fillol-Salom et al., 2022). This finding is consistent with those of Fillol-Salom et al. (2022), who demonstrated that PICIs are PAIs responsible for the transfer of defense genes.
A study of the complete APD systems harbored in the 17 most widespread PAIs revealed that these PAIs may be more important for bacterial fitness than previously considered, as the most prevalent anti-phage systems detected have a low cost to bacterial fitness (Figure 1B). Indeed, we did not detect any complete CRISPR-Cas system, in accordance with the increasingly prevalent idea that this APD system has a high cost for bacterial fitness, thus explaining its unexpectedly low prevalence across the bacterial domain (Zaayman and Wheatley, 2022). The cell surface modification and the defense systems harbored in prophages were notable findings, as preventing phage adsorption is the first line of defense in bacteria, and the main representative system. Prophages are widely spread and provide bacterial defense systems, which explains why almost all MGEs harboring APD proteins are prophages (Duan et al., 2024; Rousset et al., 2022). We also detected other common anti-phage systems: TA, RM, and CBASS APD systems. Abi systems have been detected in a wide variety of microorganisms, although their abundance is difficult to assess owing to the high level of diversity (Bernheim and Sorek, 2020).
Analysis of the CBASS systems contained in the 48 genomes of the K. pneumoniae strains identified almost twice as many type I CBASS systems as type II CBASS systems. Importantly, the fact that only type II CBASS systems were found in PAI sequences may indicate that this type of CBASS system is more important for bacterial fitness. This may also explain why the cyclase APECO1 is present in a high proportion of all type I CBASS systems (87%), while the presence of the cyclase involved in type II systems is more variable. According to the aforementioned explanation, the pore-forming effector function was homogeneously observed in the type I CBASS systems, but the effector harbored in the type II CBASS systems may be related to pore-forming or to phospholipase enzymatic function. Finally, the second signal GMPAMPc seems to be widespread as it is contained in all the CBASS systems detected.
Since being described in 2020 by Cohen et al., CBASS systems have been detected in the genomes of many prokaryotes, which demonstrates their widespread importance in enabling bacteria to resist phage replication (Cohen et al., 2019). The functional study of the type II CBASS system located in a PAI harbored in the 10ST340-VIM1 K. pneumoniae strain was assessed, due to the role of the PAIs in spreading resistance mechanisms and the fact that the 10ST340-VIM1 isolate contained fewer APD systems than the K3325S strain. The study focused on regulation by the QS network as well as on the role of the CBASS systems in the proliferation and cellular viability levels. The qRT-PCR assay showed that induction of the QS by AHL C6-HSL led to overexpression of the CBASS system in the 10ST340-VIM1 K. pneumoniae strain, indicating that detection of a threat to the bacterial community via the QS network provokes a signal that finally leads to activation of defense systems, some involved in anti-phage resistance, as previously described by Barrio-Pujante et al. (2024) (Figure 2). We also demonstrated that stimulation of the QS network causes a significant reduction in bacterial growth rates when the strain harbors a functional CBASS system, corroborating the aforementioned findings (Figure 3A), as CBASS is an Abi defense system. However, the lower growth rate of the 10ST340-VIM1Δcyclase K. pneumoniae strains suggests that the APD system may be involved in other mechanisms that improve bacterial fitness when growth conditions are suitable (Figure 3C).
Given the importance of novel therapeutic strategies like phage therapy, a reliable protocol for the rapid detection of bacterial resistance mechanisms, such as CBASS systems, is crucial for developing effective phage cocktails or combining these with other therapies to improve patient outcomes. We have demonstrated the value of the LAMP-CRISPR-Cas13a technique for detecting the most widespread CBASS types (I and II). This method has exhibited high sensitivity and specificity, except in detecting the cyclase APECO1 gene in type I CBASS systems. In this case, detection is impeded because one of 13 isolates harbors a modified version of the gene in the crRNA hybridization region, further highlighting the specificity of LAMP-CRISPR-Cas13a.
Building on our previous study, LAMP-CRISPR-Cas13a has proven highly effective for detecting bacterial resistance genes, including those conferring resistance to antibiotics and phages (Ortiz-Cartagena et al., 2023). The sensitivity and cost-effectiveness of the method are comparable to those of the molecular gold standard, RT-PCR (Ortiz-Cartagena et al., 2022, 2023). Furthermore, the high cost of RT-PCR, as well as the time requirements, and the need for specialized personnel and equipment make the method impractical for point-of-care (POC) testing, unlike CRISPR-Cas-based methods (Ortiz-Cartagena et al., 2022, 2023; Wu et al., 2021). Indeed, a CRISPR-Cas12a platform has been successfully used to detect β-lactamase-producing bacteria (KPC, NDM, and OXA), with a lower limit of detection (attomolar) than the CRISPR-Cas13a system (Curti et al., 2020; Wu et al., 2021). This may be because Cas12 targets double-stranded DNA (dsDNA), which is inherently more stable than RNA. However, the CRISPR-Cas12a protocol relies on RPA for isothermal amplification, which is less specific than LAMP, and uses a fluorescent readout, making it more costly than the LAMP-CRISPR-Cas13a method (Ortiz-Cartagena et al., 2022, 2023).
In summary, the study findings demonstrate the regulatory role of the QS network in the Abi system CBASS. Moreover, we propose an innovative biotechnological application for the LAMP-CRISPR-Cas13a technique as an ideal tool for optimizing phage therapy, as it could enable the detection of a particular APD mechanism, thus predicting the potential inefficacy of a therapeutic phage. The use of the LAMP-CRISPR-Cas13a technique is therefore proposed as a promising means of improving treatment selection and patient prognosis. Moreover, the study findings demonstrate the regulatory role of the QS network in the CBASS family of Abi systems.
The raw data supporting the conclusions of this article will be made available by the authors, without undue reservation.
CO-C: Investigation, Methodology, Writing – original draft. PF-G: Investigation, Methodology, Conceptualization, Funding acquisition, Writing – review & editing. LA: Methodology, Conceptualization, Formal analysis, Writing – review & editing. LB: Data curation, Writing – review & editing, Methodology. DP-M: Methodology, Writing – review & editing, Conceptualization, Investigation. IB: Conceptualization, Investigation, Writing – review & editing, Data curation. LF-G: Conceptualization, Writing – review & editing, Investigation, Methodology. CI-Q: Investigation, Writing – original draft. FF-C: Writing – review & editing. AB-P: Conceptualization, Writing – review & editing, Investigation, Visualization. BA: Investigation, Writing – review & editing, Formal analysis, Software. JC-M: Writing – review & editing. MT: Writing – review & editing, Conceptualization, Funding acquisition, Resources, Supervision, Validation.
The author(s) declare that financial support was received for the research and/or publication of this article. This research was supported by Project PI22/00323 from María Tomás funded by Instituto de Salud Carlos III (ISCIII) and co-funded by the European Union. The research was also supported by CIBER-Centro de Investigación Biomédica en Red (CB21/13/00068 and CB21/13/00095) (CIBERINFEC) and MePRAM Project (PMP22/00092) from Instituto de Salud Carlos III, Ministerio de Ciencia e Innovación and Unión Europea-NextGenera4onEU and Promoción of Research-European Regional Development Fund “A way of Making Europe”. Projects of Excellence from Galician Innovation Agency (GAIN, Exp. number IN607D 2021-10). Laura Fernández-García was supported by Xunta de Galicia Postdoctoral Grant IN606C-2024/004 and Antonio Barrio-Pujante Xunta de Galicia Predoctoral Grant IN606A-2023/017.
We thank the Reference Laboratory, Program for the Prevention and Control of Healthcare-Associated Infections and Antimicrobial Stewardship in Andalucía (PIRASOA, Servicio Andaluz de Salud), for their collaboration.
The authors declare that the research was conducted in the absence of any commercial or financial relationships that could be construed as a potential conflict of interest.
The author(s) declare that no Gen AI was used in the creation of this manuscript.
All claims expressed in this article are solely those of the authors and do not necessarily represent those of their affiliated organizations, or those of the publisher, the editors and the reviewers. Any product that may be evaluated in this article, or claim that may be made by its manufacturer, is not guaranteed or endorsed by the publisher.
Ajulo, S., and Awosile, B. (2024). Global antimicrobial resistance and use surveillance system (GLASS 2022): investigating the relationship between antimicrobial resistance and antimicrobial consumption data across the participating countries. PLoS ONE 19:e0297921. doi: 10.1371/journal.pone.0297921
Audrey, B., Cellier, N., White, F., Jacques, P.-É., and Burrus, V. (2023). A systematic approach to classify and characterize genomic islands driven by conjugative mobility using protein signatures. Nucl. Acids Res. 51, 8402–8412. doi: 10.1093/nar/gkad644
Barrio-Pujante, A., Bleriot, I., Blasco, L., Fernández-Garcia, L., Pacios, O., Ortiz-Cartagena, C., et al. (2024). Regulation of anti-phage defense mechanisms by using cinnamaldehyde as a quorum sensing inhibitor. Front. Microbiol. 15:1416628. doi: 10.3389/fmicb.2024.1416628
Bernheim, A., and Sorek, R. (2020). The pan-immune system of bacteria: antiviral defence as a community resource. Nat. Rev. Microbiol. 18, 113–119. doi: 10.1038/s41579-019-0278-2
Bleriot, I., Blasco, L., Pacios, O., Fernández-García, L., López, M., Ortiz-Cartagena, C., et al. (2023b). Proteomic study of the interactions between phages and the bacterial host Klebsiella pneumoniae. Microbiol. Spectr. 11:e03974–e03922. doi: 10.1128/spectrum.03974-22
Bleriot, I., Pacios, O., Blasco, L., Fernández-García, L., López, M., Ortiz-Cartagena, C., et al. (2023a). Improving phage therapy by evasion of phage resistance mechanisms. JAC-Antimicrob. Resist. 6:dlae017. doi: 10.1093/jacamr/dlae017
Chen, J. S., Ma, E., Harrington, L. B., Da Costa, M., Tian, X., Palefsky, J. M., et al. (2018). CRISPR-Cas12a target binding unleashes indiscriminate single-stranded DNase activity. Science 360, 436–439. doi: 10.1126/science.aar6245
Cohen, D., Melamed, S., Millman, A., Shulman, G., Oppenheimer-Shaanan, Y., Kacen, A., et al. (2019). Cyclic GMP–AMP signalling protects bacteria against viral infection. Nature 574, 691–695. doi: 10.1038/s41586-019-1605-5
Curti, L. A., Pereyra-Bonnet, F., Repizo, G. D., Fay, J. V., Salvatierra, K., Blariza, M. J., et al. (2020). CRISPR-based platform for carbapenemases and emerging viruses detection using Cas12a (Cpf1) effector nuclease. Emerg. Microb. Infect. 9, 1140–1148. doi: 10.1080/22221751.2020.1763857
Díaz, M. L. (2017). Estudio del “quorum sensing” en “Acinetobacter baumannii”: Implicaciones Clínicas (eds. M. M. Tomás Carmona and G. Bou Arévalo). Programa de Doctorado en Ciencias de la Salud (RD99/2011). Instituto de Investigación Biomédica (INIBIC). Coruña: Complejo Hospitalario Universitario A Coruña- Universidad de A Coruñaeds.
Dobrindt, U., Hochhut, B., Hentschel, U., and Hacker, J. (2004). Genomic islands in pathogenic and environmental microorganisms. Nat. Rev. Microbiol. 2, 414–424. doi: 10.1038/nrmicro884
Doron, S., Melamed, S., Ofir, G., Leavitt, A., Lopatina, A., Keren, M., et al. (2018). Systematic discovery of antiphage defense systems in the microbial pangenome. Science 359:eaar4120. doi: 10.1126/science.aar4120
Duan, N., Hand, E., Pheko, M., Sharma, S., and Emiola, A. (2024). Structure-guided discovery of anti-CRISPR and anti-phage defense proteins. Nat. Commun. 15:649. doi: 10.1038/s41467-024-45068-7
Fillol-Salom, A., Rostøl, J. T., Ojiogu, A. D., Chen, J., Douce, G., Humphrey, S., et al. (2022). Bacteriophages benefit from mobilizing pathogenicity islands encoding immune systems against competitors. Cell 185, 3248–3262.e20. doi: 10.1016/j.cell.2022.07.014
Finn, R. D., Clements, J., and Eddy, S. R. (2011). HMMER web server: interactive sequence similarity searching. Nucleic Acids Res. 39, W29–37. doi: 10.1093/nar/gkr367
Genoud, V., Stortz, M., Waisman, A., Berardino, B. G., Verneri, P., Dansey, V., et al. (2021). Extraction-free protocol combining proteinase K and heat inactivation for detection of SARS-CoV-2 by RT-qPCR. PLoS ONE 16:e0247792. doi: 10.1371/journal.pone.0247792
Georjon, H., and Bernheim, A. (2023). The highly diverse antiphage defence systems of bacteria. Nat. Rev. Microbiol. 21, 686–700. doi: 10.1038/s41579-023-00934-x
Gomes, A. É. I., Stuchi, L. P., Siqueira, N. M. G., Henrique, J. B., Vicentini, R., Ribeiro, M. L., et al. (2018). Selection and validation of reference genes for gene expression studies in Klebsiella pneumoniae using Reverse Transcription Quantitative real-time PCR. Sci. Rep. 8:9001. doi: 10.1038/s41598-018-27420-2
Grundmann, H., Glasner, C., Albiger, B., Aanensen, D. M., Tomlinson, C. T., Andrasević, A. T., et al. (2017). Occurrence of carbapenemase-producing Klebsiella pneumoniae and Escherichia coli in the European survey of carbapenemase-producing Enterobacteriaceae (EuSCAPE): a prospective, multinational study. Lancet Infect. Dis. 17, 153–163. doi: 10.1016/S1473-3099(16)30257-2
Huang, T.-W., Lam, I., Chang, H.-Y., Tsai, S.-F., Palsson, B. O., and Charusan, P. (2014). Capsule deletion via a λ-Red knockout system perturbs biofilm formation and fimbriae expression in Klebsiella pneumoniae MGH 78578. BMC Res. Notes 7:13. doi: 10.1186/1756-0500-7-13
Humphrey, S., San Millán, Á., Toll-Riera, M., Connolly, J., Flor-Duro, A., Chen, J., et al. (2021). Staphylococcal phages and pathogenicity islands drive plasmid evolution. Nat. Commun. 12:5845. doi: 10.1038/s41467-021-26101-5
Jonas, O. B., Irwin, A., Berthe, F. C. J., Le Gall, F. G., and Marquez, P. V. (2017). Drug-resistant infections : a threat to our economic future (Vol. 2 of 2): final report (English). HNP/Agriculture Global Antimicrobial Resistance Initiative. Washington, DC: World Bank Group. Available online at: http://documents.worldbank.org/curated/en/323311493396993758
Kellner, M. J., Koob, J. G., Gootenberg, J. S., Abudayyeh, O. O., and Zhang, F. (2019). SHERLOCK: nucleic acid detection with CRISPR nucleases. Nat. Protoc. 14, 2986–3012. doi: 10.1038/s41596-019-0210-2
Leamon, J. H., and Rothberg, J. M. (2009). “DNA sequencing and genomics,” in Encyclopedia of Microbiology, 3rd Edn, ed. M. Schaechter (Academic Press), 148–161. doi: 10.1016/B978-012373944-5.00024-9
Lin, D. M., Koskella, B., and Lin, H. C. (2017). Phage therapy: an alternative to antibiotics in the age of multi-drug resistance. World J. Gastrointest. Pharmacol. Ther. 8, 162–173. doi: 10.4292/wjgpt.v8.i3.162
Millman, A., Melamed, S., Amitai, G., and Sorek, R. (2020). Diversity and classification of cyclic-oligonucleotide-based anti-phage signalling systems. Nat. Microbiol. 5, 1608–1615. doi: 10.1038/s41564-020-0777-y
Murray, C. J. L., Ikuta, K. S., Sharara, F., Swetschinski, L., Robles Aguilar, G., Gray, A., et al. (2022). Global burden of bacterial antimicrobial resistance in 2019: a systematic analysis. Lancet 399, 629–655. doi: 10.1016/S0140-6736(21)02724-0
Oliveira, P. H., Touchon, M., and Rocha, E. P. C. (2016). Regulation of genetic flux between bacteria by restriction-modification systems. Proc. Natl. Acad. Sci. U. S. A. 113, 5658–5663. doi: 10.1073/pnas.1603257113
Ortiz-Cartagena, C., Fernández-García, L., Blasco, L., Pacios, O., Bleriot, I., López, M., et al. (2022). Reverse transcription-loop-mediated isothermal amplification-CRISPR-Cas13a technology as a promising diagnostic tool for SARS-CoV-2. Microbiol. Spectr. 10:e0239822. doi: 10.1128/spectrum.02398-22
Ortiz-Cartagena, C., Pablo-Marcos, D., Fernández-García, L., Blasco, L., Pacios, O., Bleriot, I., et al. (2023). CRISPR-Cas13a-based assay for accurate detection of OXA-48 and GES carbapenemases. Microbiol. Spectr. 11:e0132923. doi: 10.1128/spectrum.01329-23
Pacheco, T., Gomes, A. É. I., Siqueira, N. M. G., Assoni, L., Darrieux, M., Venter, H., et al. (2021). SdiA, a quorum-sensing regulator, suppresses fimbriae expression, biofilm formation, and quorum-sensing signaling molecules production in Klebsiella pneumoniae. Front. Microbiol. 12:597735. doi: 10.3389/fmicb.2021.597735
Pacios, O., Blasco, L., Bleriot, I., Fernandez-Garcia, L., González Bardanca, M., Ambroa, A., et al. (2020). Strategies to combat multidrug-resistant and persistent infectious diseases. Antibiotics 9:65. doi: 10.3390/antibiotics9020065
Payne, L. J., Meaden, S., Mestre, M. R., Palmer, C., Toro, N., Fineran, P. C., et al. (2022). PADLOC: a web server for the identification of antiviral defence systems in microbial genomes. Nucleic Acids Res. 50, W541–W550. doi: 10.1093/nar/gkac400
Ramsay, J., Colombi, E., Terpolilli, J., and Ronson, C. (2023). “Symbiosis islands,” in Reference Module in Life Sciences (Amsterdam: Elsevier). doi: 10.1016/B978-0-12-822563-9.00071-8
Reyes, J., Aguilar, A. C., and Caicedo, A. (2019). Carbapenem-resistant Klebsiella pneumoniae: microbiology key points for clinical practice. IJGM Volume 12, 437–446. doi: 10.2147/IJGM.S214305
Rousset, F., Depardieu, F., Miele, S., Dowding, J., Laval, A.-L., Lieberman, E., et al. (2022). Phages and their satellites encode hotspots of antiviral systems. Cell Host Microbe. 30, 740–753.e5. doi: 10.1016/j.chom.2022.02.018
Seemann, T. (2014). Prokka: rapid prokaryotic genome annotation. Bioinformatics 30, 2068–2069. doi: 10.1093/bioinformatics/btu153
Singh, K., Biswas, A., Chakrabarti, A. K., and Dutta, S. (2023). Phage therapy as a protective tool against pathogenic bacteria: how far we are? Curr. Pharm. Biotechnol. 24, 1277–1290. doi: 10.2174/1389201024666221207114047
Soares, S. C., Geyik, H., Ramos, R. T. J., De Sá, P. H. C. G., Barbosa, E. G. V., Baumbach, J., et al. (2016). GIPSy: genomic island prediction software. J. Biotechnol. 232, 2–11. doi: 10.1016/j.jbiotec.2015.09.008
Söding, J., Biegert, A., and Lupas, A. N. (2005). The HHpred interactive server for protein homology detection and structure prediction. Nucleic Acids Res. 33, W244–248. doi: 10.1093/nar/gki408
Tamura, K., Stecher, G., and Kumar, S. (2021). MEGA11: molecular evolutionary genetics analysis version 11. Mol. Biol. Evol. 38, 3022–3027. doi: 10.1093/molbev/msab120
Tang, K. W. K., Millar, B. C., and Moore, J. E. (2023). Antimicrobial resistance (AMR). Br. J. Biomed. Sci. 80:11387. doi: 10.3389/bjbs.2023.11387
Wang, J., Chitsaz, F., Derbyshire, M. K., Gonzales, N. R., Gwadz, M., Lu, S., et al. (2023). The conserved domain database in 2023. Nucleic Acids Res. 51, D384–D388. doi: 10.1093/nar/gkac1096
Wang, L., and Zhang, L. (2023). The arms race between bacteria CBASS and bacteriophages. Front. Immunol. 14:1224341. doi: 10.3389/fimmu.2023.1224341
WHO (2024). WHO Bacterial Priority Pathogens List Bacterial Pathogens of Public Health Importance, to Guide Research, Development, and Strategies to Prevent and Control Antimicrobial Resistance. Geneva: World Health Organization.
Wu, Y., Battalapalli, D., Hakeem, M. J., Selamneni, V., Zhang, P., Draz, M. S., et al. (2021). Engineered CRISPR-Cas systems for the detection and control of antibiotic-resistant infections. J. Nanobiotechnol. 19:401. doi: 10.1186/s12951-021-01132-8
Zaayman, M., and Wheatley, R. M. (2022). Fitness costs of CRISPR-Cas systems in bacteria. Microbiology 168:1209. doi: 10.1099/mic.0.001209
Keywords: phage resistance, CBASS systems, CRISPR, Cas13a, Purification-free
Citation: Ortiz-Cartagena C, Fernández-Grela P, Armán L, Blasco L, Pablo-Marcos D, Bleriot I, Fernández-García L, Ibarguren-Quiles C, Fernández-Cuenca F, Barrio-Pujante A, Aracil B, Calvo-Montes J and Tomás M (2025) The LAMP-CRISPR-Cas13a technique for detecting the CBASS mechanism of phage resistance in bacteria. Front. Microbiol. 16:1550534. doi: 10.3389/fmicb.2025.1550534
Received: 23 December 2024; Accepted: 03 March 2025;
Published: 24 March 2025.
Edited by:
Lin Chen, Nanyang Technological University, SingaporeReviewed by:
Malgorzata Barbara Lobocka, Polish Academy of Sciences (PAN), PolandCopyright © 2025 Ortiz-Cartagena, Fernández-Grela, Armán, Blasco, Pablo-Marcos, Bleriot, Fernández-García, Ibarguren-Quiles, Fernández-Cuenca, Barrio-Pujante, Aracil, Calvo-Montes and Tomás. This is an open-access article distributed under the terms of the Creative Commons Attribution License (CC BY). The use, distribution or reproduction in other forums is permitted, provided the original author(s) and the copyright owner(s) are credited and that the original publication in this journal is cited, in accordance with accepted academic practice. No use, distribution or reproduction is permitted which does not comply with these terms.
*Correspondence: María Tomás, bWEuZGVsLm1hci50b21hcy5jYXJtb25hQHNlcmdhcy5lcw==
Disclaimer: All claims expressed in this article are solely those of the authors and do not necessarily represent those of their affiliated organizations, or those of the publisher, the editors and the reviewers. Any product that may be evaluated in this article or claim that may be made by its manufacturer is not guaranteed or endorsed by the publisher.
Research integrity at Frontiers
Learn more about the work of our research integrity team to safeguard the quality of each article we publish.