- 1Laboratory of Food Microbiology and Mycotoxicology, Department of Food Engineering, School of Animal Science and Food Engineering, University of São Paulo, Pirassununga, Brazil
- 2School of Physics, Engineering and Technology, University of York, York, United Kingdom
- 3Department for Gaseous Electronics, Jožef Stefan Institute, Ljubljana, Slovenia
Introduction: Microbial contamination remains a vital challenge across the food production chain, particularly due to mycotoxins—secondary metabolites produced by several genera of fungi such as Aspergillus, Fusarium, Alternaria, and Penicillium. These toxins, including aflatoxins, fumonisins, ochratoxins, and trichothecenes (nivalenol, deoxynivalenol, T2, HT-2). These contaminants pose severe risks to human and animal health, with their potential to produce a variety of different toxic effects. Notably, up to 50% of global cereal production is affected by mycotoxin contamination, leading to significant economic losses. Current research focuses on innovative technologies to mitigate mycotoxins, with cold atmospheric pressure plasma emerging as a promising decontamination method.
Method: This systematic review aimed at describing recent advances in the application of cold atmospheric plasma for the decontamination of toxigenic fungi and mycotoxins.
Results and discussion: Cold atmospheric plasma offers a sustainable and cost effective solution to preserve food quality while inactivating toxigenic fungi and degrading mycotoxins. Through the generation of reactive oxygen and nitrogen species, cold plasma disrupts fungal cell integrity, hinders spore germination, and inhibits toxin biosynthesis. Additionally, cold atmospheric plasma-driven degradation of mycotoxins involves structural modifications, breaking key molecular bonds that reduce toxicity. The effectiveness of cold plasma depends on operational parameters and the specific characteristics of the treated food, with notable efficacy in degrading aflatoxin B1 and deoxynivalenol by converting them into less toxic substances and inhibiting their spores and DNA responsible for their biosynthesis. While the data demonstrates that cold atmospheric plasma has minimal impact on food composition, further research is needed to fully assess the nature of the degradation products of mycotoxins, its influence on food quality attributes and to optimize application strategies for different products.
1 Introduction
Microbial contamination is a major concern throughout the food supply chain, posing significant challenges to food production (Khaneghah et al., 2019). Among microbial contaminants, filamentous fungi are found on various food and feed products and are capable of producing toxic secondary metabolites known as mycotoxins, which present a significant threat to human health. The most common mycotoxigenic fungi include genera such as Aspergillus, Fusarium, Alternaria, and Penicillium (Khaneghah et al., 2019; Nesic et al., 2021).
Mycotoxin contamination remains a persistent challenge in agriculture, especially in cereal grains, and can occur during pre- and post-harvest stages, as well as during processing, packaging, and storage of products (Ali et al., 2023a). The most common mycotoxins found in food and feed products include aflatoxins (AFs), fumonisins (FBs), ochratoxins (OTs), zearalenone (ZEN), patulin (PAT), and trichothecenes such as nivalenol, deoxynivalenol (DON), HT-2 and T-2 toxins (Nesic et al., 2021). Fusarium mycotoxins, such as DON, FBs, and ZEN, are known to contaminate grains in the field during the pre-harvest stage of crop cultivation. In contrast, AFs produced by Aspergillus and OTs produced by Penicillium may appear later due to improper post-harvest practices, including inadequate storage and transport (Neme and Mohammed, 2017). The growth of mycotoxigenic fungi and the production of mycotoxins are influenced by increased temperature and moisture. As a result, higher levels of mycotoxin contamination are strongly correlated with global climate change, which also impacts the global economy. Food transported over long distances is exposed to varying local climates, extended transport, and prolonged storage times, all of which contribute to increased contamination risks (Moretti et al., 2019). It is estimated that 25–50% of global cereal production is affected by significant mycotoxin levels, with 5–10% reaching concentrations considered irremediable (Haque et al., 2020; Abrunhosa et al., 2016). Such contamination not only compromises product quality but also poses serious safety risks and is therefore subject to strict regulatory standards (Khaneghah et al., 2019). Recently, there has been increased focus on so-called emerging mycotoxins, which are either masked or modified forms of already identified types or have been newly discovered. These toxic compounds are not yet regulated but, like other mycotoxins, can occur frequently and at high concentrations in food and feed. Emerging mycotoxins include Fusarium metabolites such as enniatins (ENNs), fusaproliferin (FP), and beauvericin (BEA), as well as Alternaria spp. mycotoxins and ergot alkaloids produced by Claviceps spp (Gruber-Dorninger et al., 2017).
When mycotoxin levels exceed the recommended limits (EFSA, 2004; EU, 2023), they can cause acute or chronic toxic effects, with carcinogenicity being one of the most concerning outcomes (Richard, 2007). From a risk perspective, several mycotoxins have been classified based on their carcinogenic potential by the International Agency for Research on Cancer (IARC, 2023). Aflatoxin B1 (AFB1) is categorized as Group 1 (carcinogenic to humans), fumonisin B1 (FB1) and ochratoxin A (OTA) as Group 2B (possibly carcinogenic to humans), while ZEN and DON are placed in Group 3 (not classifiable as to their carcinogenicity to humans) (IARC, 2023; Schrenk et al., 2020; Ostry et al., 2017; EFSA, 2004). In addition to genotoxicity and carcinogenicity, mycotoxins are known to cause a range of other toxic effects. Human exposure, particularly in young children, is especially concerning due to their immature detoxification systems and increased vulnerability to these toxins (Knutsen et al., 2017; Fakhri et al., 2019). Exposure to FB1 has been linked to neural tube defects and cancer (Alvito and Pereira-da-Silva, 2022; Schrenk et al., 2022), while ZEN is known to cause estrogenic disturbances and degenerative syndromes, affecting both growth and reproductive health in humans and animals (Borutova et al., 2012; Schoevers et al., 2012). The T-2 toxin can inhibit protein, RNA, and DNA synthesis, leading to apoptosis, necrosis, lipid peroxidation, and adverse hematopoietic effects (Arcella et al., 2017). DON disrupts eukaryotic cells and mitochondria, with chronic exposure linked to intestinal microbiota imbalance and health complications (Knutsen et al., 2017; Ali et al., 2024; Arce-López et al., 2021; Akbari et al., 2017). OTA is recognized for its nephrotoxic, neurotoxic, teratogenic, and carcinogenic properties (Clark and Snedeker, 2006; Majeed et al., 2017).
Preventive measures including good agriculture practices, appropriate grain handling after harvest and proper storage are key factors to avoid fungal development and mycotoxin production (Haque et al., 2020). However, these practices may not be effective once mycotoxins have formed. Therefore, efforts have been directed toward developing effective strategies to alleviate or eliminate toxins and their metabolites from food materials (Neuenfeldt et al., 2023). Effective decontamination approaches must minimize both toxigenic fungi and mycotoxins levels, without compromising food quality and safety. So far, various methods—including chemical, biological, physical—have been explored in the food industry for mycotoxin detoxification (Marshall et al., 2020; Basso et al., 2023; Ali et al., 2023b; Naeem et al., 2024). Chemical treatments, such as ammonization, ozone, hydrogen peroxide, and sodium bisulfite among others, have been widely applied to detoxify mycotoxins in various food matrices. However, these methods may reduce nutritional quality, leave harmful residues, or only partially remove toxins, raising concerns over safety and consumer acceptance (Nahle et al., 2022; Mir et al., 2021; Khan, 2024). Biological methods involving microorganisms or enzymes to adsorb or degrade toxins offer safer alternatives but can face challenges with production consistency (Loi et al., 2023; Mir et al., 2021). Physical methods, such as thermal treatments, can effectively reduce microbial loads and toxins but may alter sensory and nutritional properties, or achieve uneven heating in grains (Naeem et al., 2024; Anderson, 2019). In contrast, innovative approaches to mycotoxin detoxification such as non-thermal technologies are anticipated to have a pivotal role in the food supply chain, ensuring the preservation of food quality while minimizing environmental impacts and maintaining economic viability (Hojnik et al., 2020). Recently, research has increasingly focused on cold atmospheric pressure plasma (CAP) as a promising approach for mycotoxin decontamination of food, aligning with these critical requirements (Patriarca and Pinto, 2017; Gavahian et al., 2021). CAP has demonstrated effectiveness in reducing microbial loads and detoxifying mycotoxins across diverse food types, especially in low-moisture products like cereal grains (Sun et al., 2014; Mir et al., 2021; Naeem et al., 2024). Although some studies have outlined CAP applications for fungi and mycotoxin decontamination (Gavahian and Cullen, 2019; Neuenfeldt et al., 2023; Misra et al., 2018; Hojnik et al., 2017), there is no available systematic reviews on this subject. Therefore, the objective of the present study was to systematically review recent literature on the application of CAP for the decontamination of commonly occurring mycotoxins in foodstuffs, discussing its potential and challenges for broader adoption in the food industry.
2 Search strategy
A literature search was conducted according to Cochrane protocols and the preferred reporting items for systematic review and meta-analysis (PRISMA) guidelines (Shamseer et al., 2015) across PubMed, ScienceDirect, Web of Science, Scopus and Google Scholar to identify relevant studies on the use of CAP for decontamination of main mycotoxins found in food products published in the past 10 years. The following key terms were employed: “Mycotoxins” OR “Aflatoxins” OR “Fumonisins” OR “Ochratoxin A” OR “Zearalenone” OR “Patulin” OR “Trichothecenes” OR “Deoxynivalenol” OR “T-2 Toxin” OR “HT-2 Toxin” OR “Atmospheric pressure plasma technology” OR “Cold atmospheric plasma” OR “Decontamination” OR “Detoxification” OR “Degradation.”
To optimize search precision and account for database-specific limitations, the terms were grouped into five search combinations:
1. “Cold atmospheric plasma” AND “Mycotoxins” AND “Aflatoxins” OR “Deoxynivalenol” AND “Degradation.”
2. “Atmospheric pressure plasma technology” AND “Mycotoxins” OR “Fumonisins” OR “Patulin” AND “Decontamination.”
3. “Cold atmospheric plasma” AND “Ochratoxin A” OR “Zearalenone” AND “Degradation” OR “Detoxification.”
4. “Atmospheric pressure plasma technology” AND “Mycotoxins” OR “Trichothecenes” OR “Toxin HT-2” AND “Detoxification.”
5. “Cold atmospheric plasma” AND “Mycotoxins” OR “Toxin T-2” OR “Trichothecenes” AND “Decontamination.”
After screening titles and abstracts for relevance, full-text articles were assessed for eligibility based on the following criteria: (1) availability of full text, (2) original research studies (excluding reviews), (3) detailed experimental procedures, (4) precise analytical methods and (5) publication in English. The initial search yielded 4,679 articles, from which 3,237 articles were excluded immediately for not meeting criterion 2, as they were not original studies. Additionally, 200 articles were removed as duplicates, resulting in a total of 1,242 unique publications on the application of CAP. Following another screening, 1,181 articles were excluded for describing CAP applications for secondary fungal metabolites other than main mycotoxins that commonly occur in food products or involving products unrelated to the food sector. Further 26 articles were excluded due to incomplete descriptions of the methods used or because they focused on comparisons between different analytical methods. Finally, 5 articles that used CAP in combination with other decontamination methods were also excluded. At the end of the screening process, a total of 4,650 articles were excluded, as outlined in Figure 1. Ultimately, 30 studies met the inclusion criteria and were included in this review.
3 Principles of cold atmospheric plasma technologies
Plasma, known as the 4th state of matter, is created when sufficient energy, in the form of heat or an electric field, is applied to a gas, causing free electrons to be accelerated to high energies. Collisions between electrons and neutral gas atoms or molecules can result in ionization, if a sufficient number of ionization events occur the process becomes self-sustaining and a plasma is formed (Neuenfeldt et al., 2023). Atmospheric pressure plasmas can be categorized as either “thermal,” where a local thermodynamic equilibrium is maintained among the electrons and heavy particles (e.g., ions and neutrals), or “non-thermal,” characterized by thermodynamic imbalance, whereby electrons are at a significantly higher temperature. CAP falls within the non-thermal category (Pankaj and Keener, 2017).
Two essential components are required for plasma generation: a high-voltage source and a reactor. The high-voltage source is used to establish a high electric field, which accelerates electrons to the high energies needed to cause ionization, this is typically done in a reactor employing two electrodes, a powered electrode and ground (Niemira, 2012; Ojha et al., 2021). Key parameters affecting the efficacy of the system relate to the form of the applied voltage (e.g., direct current, radio-frequency, pulsed, microwave etc.), and the particular configuration of the reactor (e.g., plasma jet, parallel plate etc.). Once plasma forms in the reactor, the energetic electrons take part in a plethora of reactions causing dissociation and excitation of the background gas creating a complex mixture including ions, free radicals, electrons, and UV photons. For example, in humid air plasma it is known that over 50 unique chemical species are created, which take part in over 600 reactions (Sakiyama et al., 2012). Of particular importance are the reactive neutral species, commonly termed reactive oxygen species (ROS) and reactive nitrogen species (RNS) (Hertwig et al., 2018; Misra and Jo, 2017). Beyond the key physical parameters of the plasma system, a large number of factors affect ROS and RNS generation and therefore the efficacy of the plasma in degrading mycotoxin contamination. For example, plasma generation parameters, such as energy input, play a key role, as the electrical power used for plasma generation directly affects the energy available for the chemical ionization, dissociation, and excitation processes. These energy-dependent processes determine the types and densities of reactive species formed (Shimizu et al., 2012). The energy distribution of electrons is another vital factor, as it governs the nature of reactions within the plasma. Specifically, the electron energy distribution function (EEDF) influences whether molecular dissociation or ionization occurs (Abdel-Fattah, 2013). The frequency of the applied voltage, whether direct current (DC), radio frequency (RF), or microwave, also impacts the energy distribution of electrons and ions. Abdel-Fattah (2013) demonstrated that higher frequencies led to increased peak electron densities, a finding supported by Naidis (2014), who observed elevated densities of reactive species at higher voltage amplitudes and frequencies. In a helium-air plasma model, Naidis (2014) noted that higher voltage amplitudes and frequencies increased NO and O3 densities while reducing O atom concentrations, whereas the hydroxy radical (OH) density remained relatively stable.
The feed gas composition is another critical determinant of plasma chemistry, as it defines the atoms and molecules participating in plasma formation. Variations in feed gases, such as helium (He), argon (Ar), nitrogen (N2), air, or oxygen (O2), result in distinct reactive species profiles (Naidis, 2014; Han et al., 2016; Dünnbier et al., 2013). The introduction of specific gases can significantly influence reactive species generation. For example, Han et al. (2016) observed a gradual increase in hydrogen peroxide (H2O2) production with the addition of oxygen, a process linked to enhanced generation of ROS, including OH radicals. Gas flow rate also affects the formation and distribution of reactive species such as NO and HO2. Increased flow rates promote the spread of OH radicals, affecting their interaction sites (Hasan and Walsh, 2017). Interaction with ambient air further modulates plasma chemistry, introducing nitrogen, oxygen, and water vapor into the system, which contributes to RNS and ROS formation (Naidis, 2014; Morabit et al., 2021).
Electrode geometry and material significantly impact the electric field distribution, which influence plasma characteristics. Changes in electrode geometry, for instance diameter, alter the breakdown voltage and power deposition within the plasma discharge (Hasan and Walsh, 2017). The chemical composition of plasma also depends on the distance between electrodes and the target. Variations in these distances modify the ionization region, affecting the generation and transport of ROS and RNS (Morabit et al., 2021). Moreover, the density of reactive species decreases as the distance between the plasma jet and the target sample increases (Zhang et al., 2019). Plasma interactions with either a biological, liquid, or polymeric sample, can catalyze or inhibit specific reactions, altering the chemical composition near the sample surface (Morabit et al., 2021; Kovaèeviæ et al., 2018). In some types likely liquid samples, these effects are particularly pronounced, as the presence of the liquid alters reactive species composition and modifies the potential gap near the target surface (Kovaèeviæ et al., 2018).
Historically, CAP generation was constrained to low-pressure conditions, requiring the use of a vacuum chamber and thus limiting its applications for the treatment of food products. However, advancements in technology now allow for the stable and non-thermal generation of plasma at atmospheric pressure, leading to extensive research across various scientific fields (Pankaj and Keener, 2017). In specific reactor configurations, such as plasma jets, the mass transport of reactive species is facilitated by gas flow, enabling the effects of plasma to extend to regions well beyond the electrodes. Due to the limited area covered by a single plasma jet, multiple jets or motion systems are often used to treat larger areas or volumes (Feizollahi et al., 2020).
The effectiveness of CAP in fungal inactivation depends on several factors, including the type of plasma generator, the gas utilized, the fungal strains involved, and the test matrix (Mošovská et al., 2019; Wu et al., 2021). Additionally, specific equipment parameters, such as frequency (ranging from 0 Hz to several GHz), discharge voltage (1 kV to over 100 kV), and power (ranging from W to kW), significantly influence CAP’s impact (Ojha et al., 2021). While numerous studies highlight the importance of these parameters, there remains no consensus among recent research regarding the optimal ranges for CAP treatment. The variability in these parameters, as applied to mycotoxin degradation in food, is further discussed according to the specific CAP types examined in the studies.
3.1 Indirect plasma treatment
As previously described, a wide range of different plasma reactors have been used to examine the impact of plasma exposure on mycotoxin contamination. Typically, these can be categorized as either direct—meaning the plasma directly interacts with the target, or indirect—meaning the plasma is generated remotely from the target and only the neutral species interact with the target. This is a fundamental difference, as the spatial separation between plasma and target acts as a spatial filter for reactive species, meaning only the less reactive agents, such as ozone, play a role in the degradation process.
Of the many indirect plasma treatment systems reported in the literature, the surface barrier discharge (SBD) has been widely adopted. This particular configuration belongs to the dielectric barrier discharge (DBD) family of devices, and typically employs a dielectric material sandwiched between a powered and grounded electrode, illustrated in Figure 2A. In this indirect scenario, the plasma region is in the form of a thin layer situated mm to cm from the target. When ambient air is used as the working gas, highly reactive species such as H, N and O form in the discharge layer and are transported toward the target via diffusion and convection (Dickenson et al., 2018; Hasan et al., 2017; Dickenson et al., 2017; Hasan and Walsh, 2017). Owing to their high chemical reactivity, they rapidly react beyond the discharge region to form more stable states such as O3 and N2O. Other indirect treatment modalities include plasma jets where the target is placed at a distance from the generated plasma plume and plasma “activated” liquids (Naidis, 2014; Morabit et al., 2021). In a plasma activated liquid, either direct or indirect plasma exposure can be used to first treat a liquid, such as water, introducing a complex chemical cocktail; with the liquid being subsequently applied to the target. Typically, this process generates an acidic solution, owing to abundant nitrogen-based species whilst containing hydrogen peroxide and others. Such solutions have been shown to possess disinfectant properties, making them effective for surface decontamination (Neuenfeldt et al., 2023; Han et al., 2023; Perinban et al., 2022).
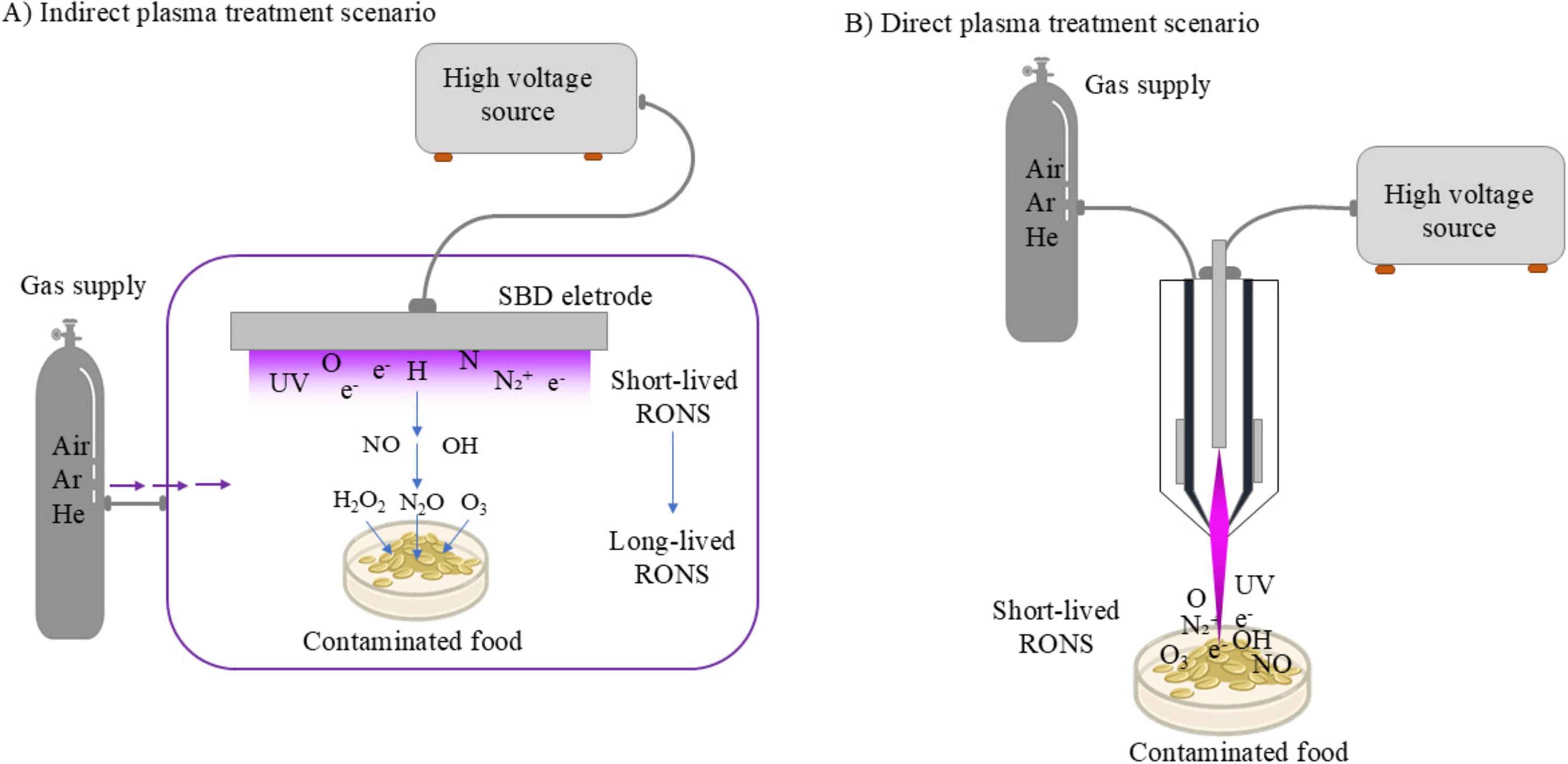
Figure 2. Schematic representation of indirect (A) and direct (B) plasma treatments illustrating mechanisms for decontaminating food matrices.
3.2 Direct plasma treatment
In contrast to the in-direct treatment scenario, the direct application of CAP to a target enables the entire reactive chemistry created within the discharge to play a role in the decontamination process (Figure 2B). Plasma reactors, such as plasma jets and parallel plates, facilitate the direct exposure of a target to a plasma (Harikrishna et al., 2023). Both plasma jets and parallel plates can take the form of a DBD or employ other approaches to inhibit the glow-to-arc transition, such as radio-frequency excitation (Park et al., 2000; Polito and Kushner, 2024). Through these methods it is possible to maintain discharge stability and a non-thermal characteristic. While such systems can offer enhanced efficacy over their indirect counterparts in microbial inactivation (Wan et al., 2019), due primarily to the enhanced flux and composition of RONS arriving at the target, they are also more complex and typically more difficult to control. When a target is electrically conductive, such as most food stuffs, it is inherently part of the electrical circuit and therefore plays a role in dictating the specific composition of the plasma. Plasma jets can overcome this by physically separating the plasma generation region from the application region, but typically require significant gas flows and/or operation in noble gases such as Argon and Helium (Morabit et al., 2021).
4 Application of cold atmospheric plasma in inactivating toxigenic fungi and mycotoxin biosynthesis
In foodstuffs and other substrates, fungal sporulation and germination evolve until a fully developed fungus. For this process, the fungus and its spores nurture on the target food matrix and introduce it with different chemical agents such as damaging enzymes, and self-defense metabolites including mycotoxins (Ali et al., 2023a). The fungus cellular integrity, its cellular metabolic processes and the enzymatic events are essential in establishing this cycle. However, injury to any or all the essential components, leads to an end with the fungus sporulation and germination with no more production of toxins. Inhibiting such mechanisms has been tempted with modern technologies including CAP application on certain foods. Figure 3 highlights the mechanisms underlying the inactivation of toxigenic fungi and inhibition of mycotoxin biosynthesis by CAP technologies.
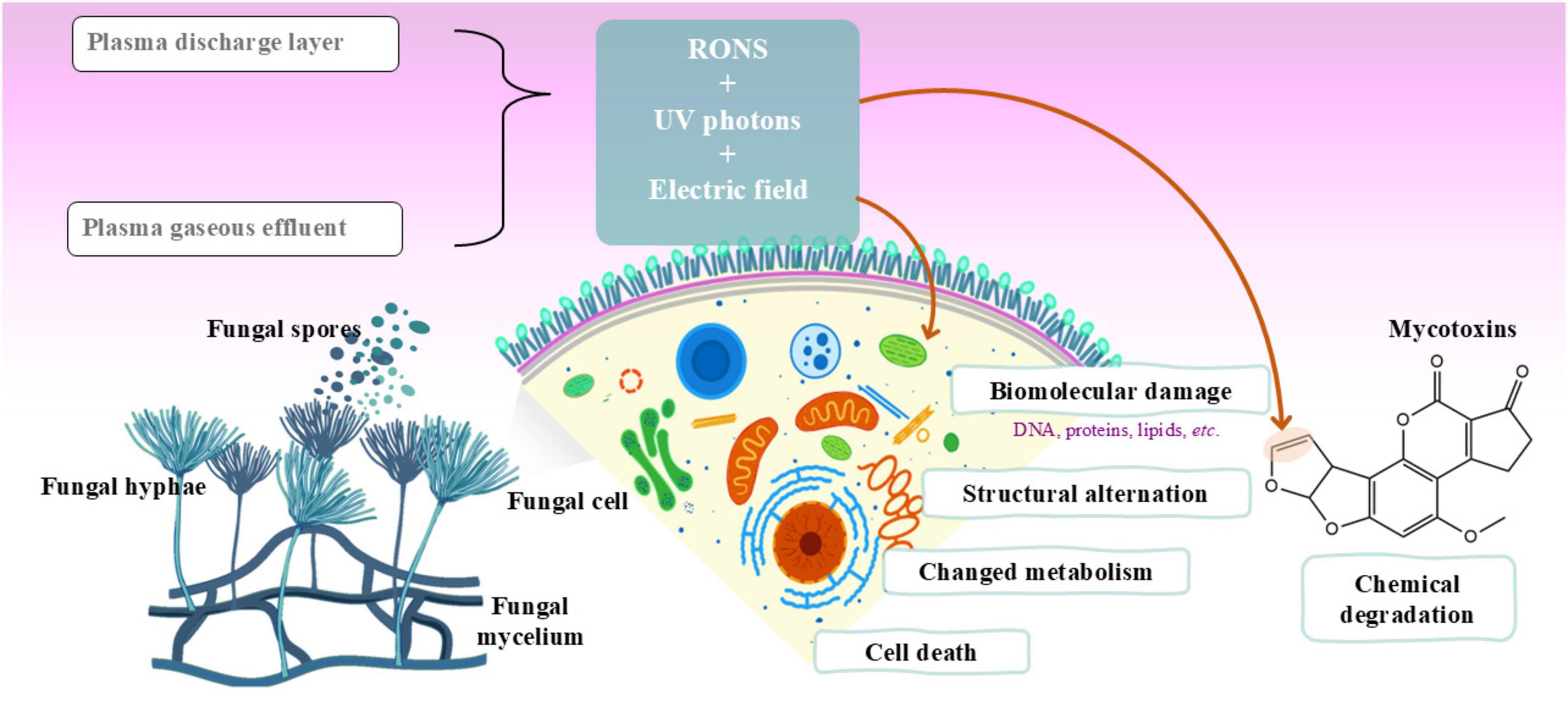
Figure 3. Mechanisms involved in the inactivation of toxigenic fungi and the inhibition or degradation of mycotoxins induced by cold atmospheric plasma (CAP) technology.
The antimicrobial properties of CAP are well-established, making this technology an appealing option for controlling mycotoxigenic fungi and decontaminating the mycotoxins they produce. Table 1 summarizes the main findings from studies focused on the inactivation of toxigenic fungi and inhibition of mycotoxin biosynthesis. CAP generates different reactive species like ROS, RNS and radicals (Hertwig et al., 2018; Misra and Jo, 2017), which are capable of damaging spores and avoid their germination. To do so, the chemical species are directed to induce oxidative stress on the outer layers of the target spores. The oxidative stress further causes a disruption to the cellular metabolic events, and cellular membrane together with its essential DNA, protein, and lipid components, resulting in declined growth of fungus and a reduced production of toxins (Zhao X. et al., 2024). The exposure of A. ochraceus to CAP caused ruptures and desiccations in its spores, with significant decline in their viability (Hoppanová et al., 2020). Membrane rupture and desiccation allow the cellular contents to escape from the damaged cell and eventual cell death occurs. In addition, CAP can lead to poor production or lack of energy, ultimately causing spore prevention and cell death, as described for Clostridioides difficile (Kaur et al., 2020). CAP can also interfere in the metabolic pathways and enzyme events needed to spore development (Hojnik et al., 2017).
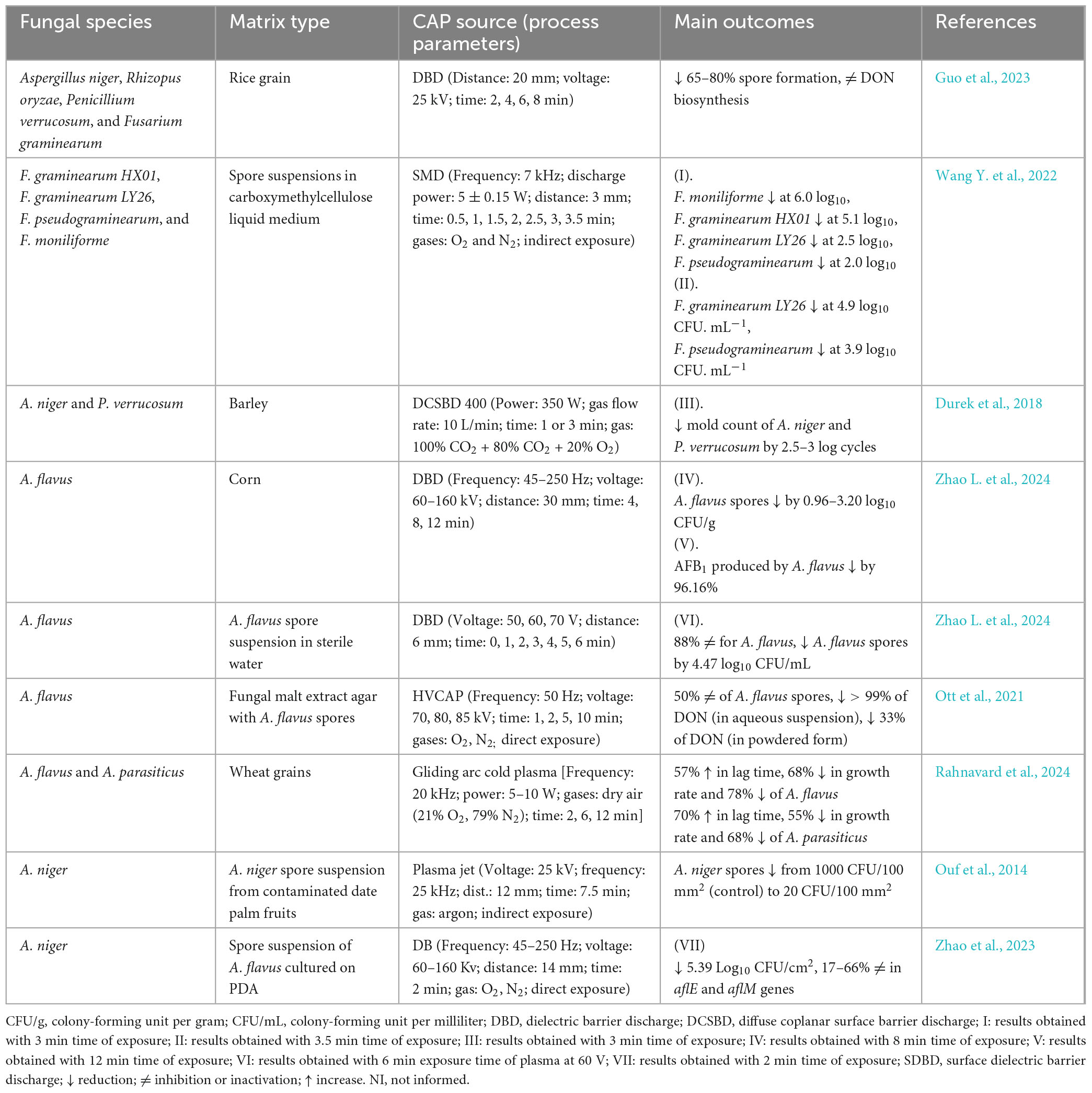
Table 1. Summary of main outcomes from studies using cold atmospheric pressure plasma (CAP) in decontaminating toxigenic fungi.
The inhibition efficacy of CAP created with DBD was evaluated in a case of decontaminating A. niger, P. verrucosum, F. graminearum, and Rhizopus oryzae on rice grains (Guo et al., 2023). Promising results were achieved after 4 min of exposure, significantly reducing fungal mycelial growth across all tested species, with increased efficacy observed at a longer exposure time (Table 1). The study demonstrated a significant inhibitory effect on spore germination, particularly in the case of F. graminearum and R. oryzae, for which a 4-min exposure resulted in a 65–80% reduction in spore viability (Guo et al., 2023). However, the efficiency of CAP against spores varies according to the fungal genus and spore size (Wang Y. et al., 2022; Zhao X. et al., 2024). The larger spore sizes are shown to correlate well with a lower CAP inactivation efficiency (Wen et al., 2017; Wen et al., 2019; Wen et al., 2020). By studying the inactivation ability of CAP on Fusarium species, Wang Y. et al. (2022) found that smaller sized spores were more susceptible to CAP reactive species due to their larger specific surface area, resulting in more effective inactivation. Additionally, spore germination rates dropped below 10% following to 2 min of exposure to CAP generated with a dielectric barrier surface micro-discharge (SMD). Regarding DON levels relative to the dry weight of mycelium (μg/g), CAP treatment resulted in a 30–48% reduction (Wang Y. et al., 2022).
CAP’s action can alter spore morphology, leading to structural damage such as increased roughness surface, membrane fractures, and size reduction. The observed membrane damage, confirmed by monitoring intracellular nucleic acid and protein leakage, indicates that increasing CAP exposure beyond 2 min progressively compromises membrane integrity (Wang Y. et al., 2022). CAP also inhibits mycotoxin biosynthesis by downregulating the expression of the genes responsible for toxin production. Ouf et al. (2014) found that CAP generated with a double atmospheric pressure argon plasma jet (DAPACP) or plasma jet affected the biosynthesis of FB2 and OTA in treated A. niger spores, through disruptions in the microbial DNA. This was also investigated in a study by Zhao et al. (2023), where aflatoxin production was analyzed at the biomolecular level, focusing on the gene expressions involved in aflatoxin biosynthesis. Among the 12 genes associated with AFB1 production, CAP generated with the dielectric barrier (DB) specifically reduced the expression of aflE and aflM, indicating CAP’s role in inhibiting aflatoxin synthesis. These findings highlight the importance of advancing our understanding of CAP’s impact on fungal activity and mycotoxin production at the molecular level.
5 Mycotoxins degradation by cold atmospheric plasma
Mycotoxin reduction through the application of CAP technologies occur mainly by degradation where a target toxin is broken down into less or non-toxic chemical components (Wang et al., 2024). Mycotoxins’ degradation through CAP is primarily influenced by the type of chemical reactions, the reactive species generated by the apparatus, and UV radiation. This combination leads to cleavage of the molecular structure of toxin, resulting in the formation of transformation products (TPs) (Feizollahi et al., 2020). When CAP induces the cleavage of the specific site on the mycotoxin molecule responsible for its toxicity, it can be assumed that this process leads to the degradation of the mycotoxin and the formation of less toxic TPs. In line, reduction effects following CAP application have been demonstrated for AFs and some other mycotoxins (Figures 4–8), as shown in Tables 2, 3, respectively.
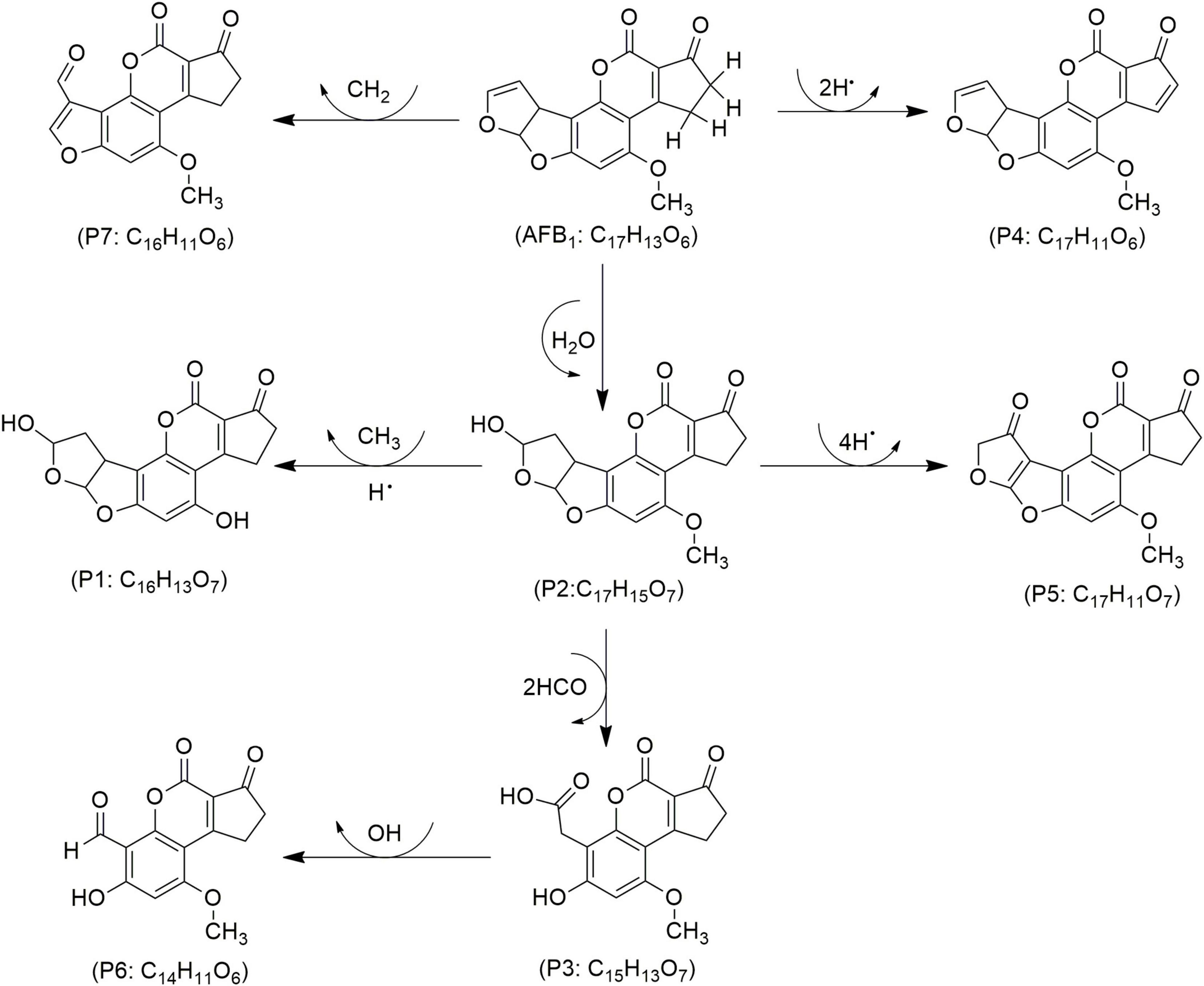
Figure 4. Proposed mechanisms and degradation products (P) of aflatoxin B1 (AFB1) in food under cold atmospheric plasma (CAP) treatment, adapted from Zhao et al. (2023).
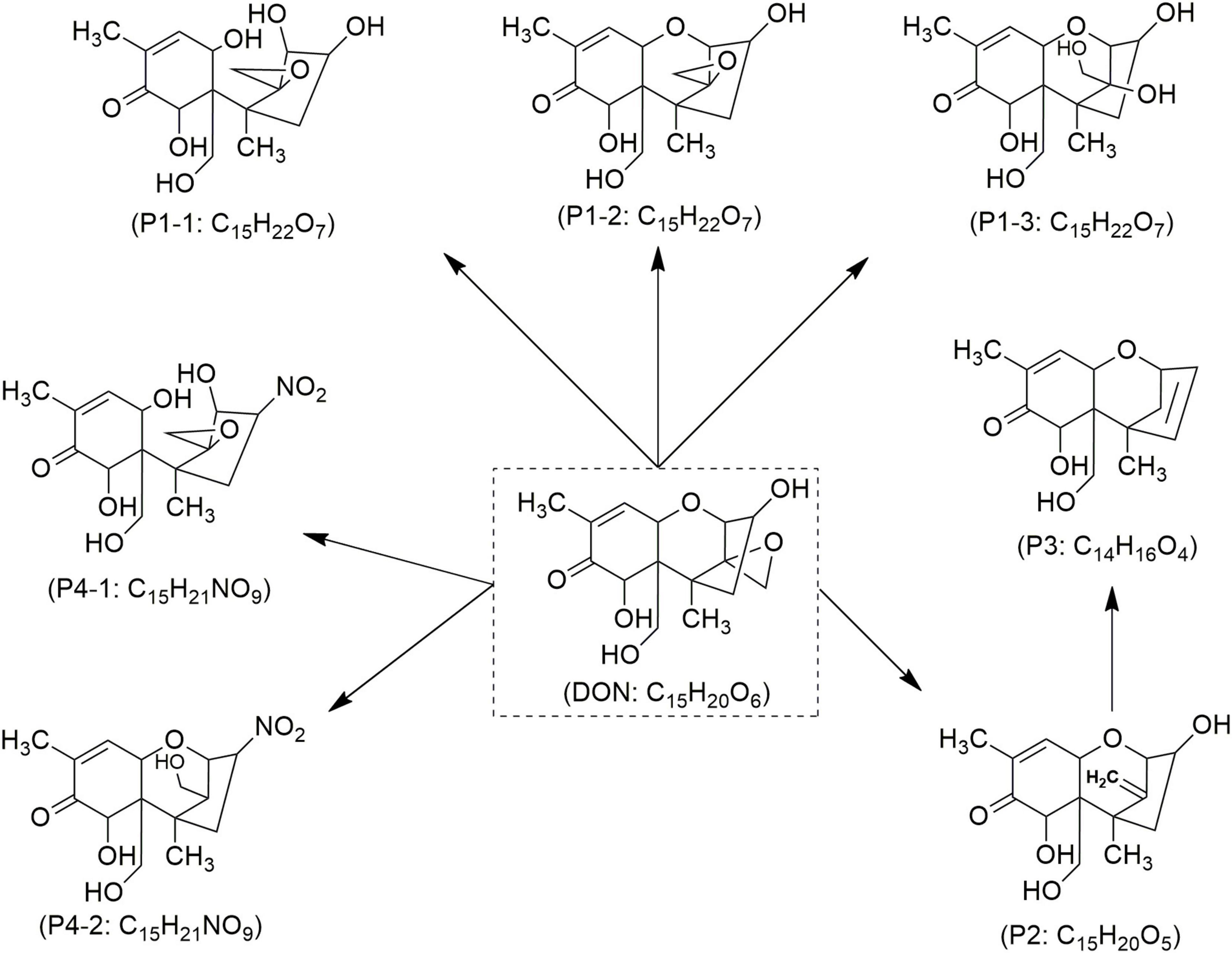
Figure 5. Proposed mechanisms and degradation products (P) of deoxynivalenol (DON) in food under cold atmospheric plasma (CAP) treatment, adapted from Chen et al. (2022).
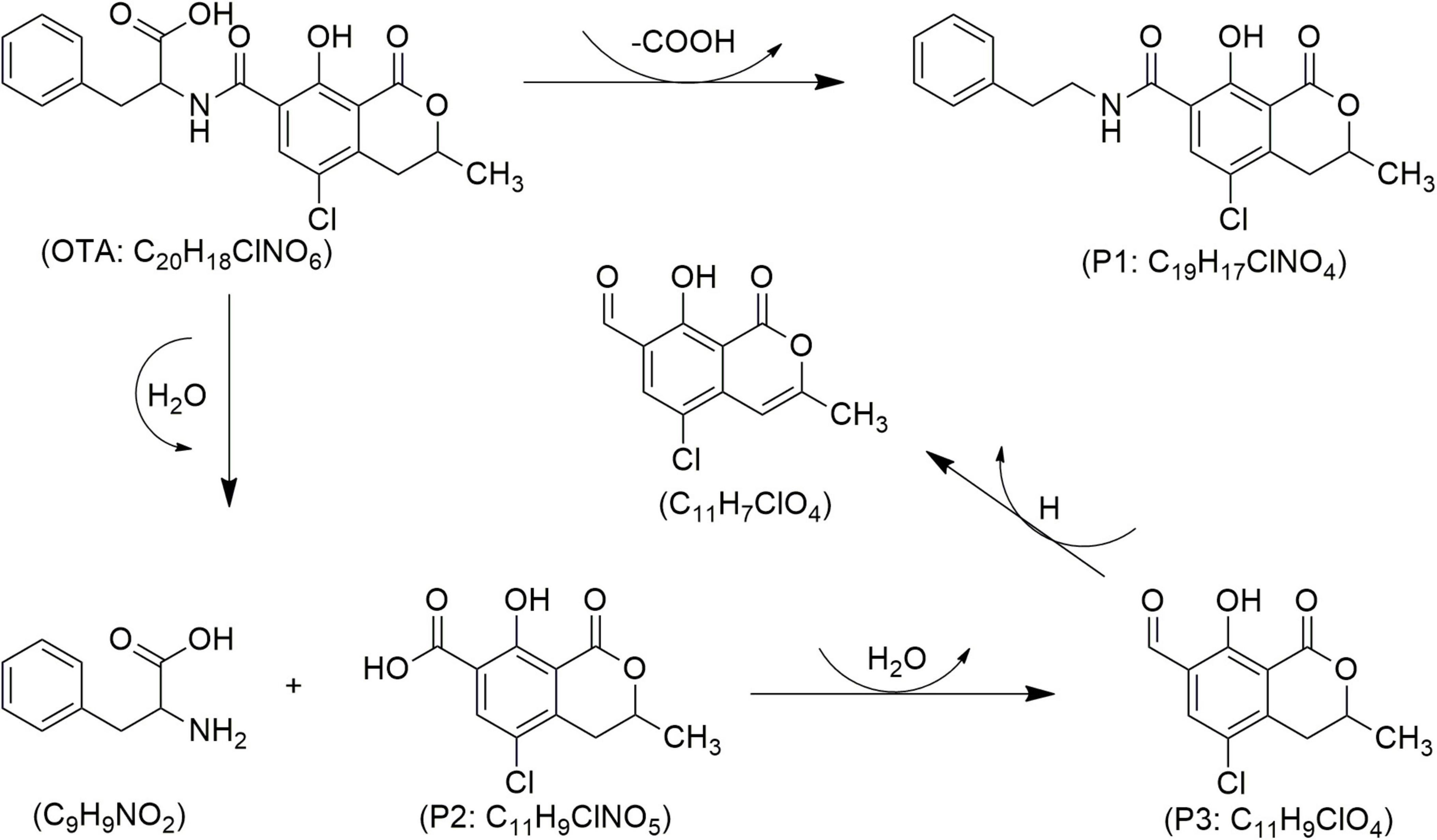
Figure 6. Proposed mechanisms and degradation products (P) of ochratoxin A (OTA) in food under cold atmospheric plasma (CAP) treatment, adapted from Wang et al. (2024).
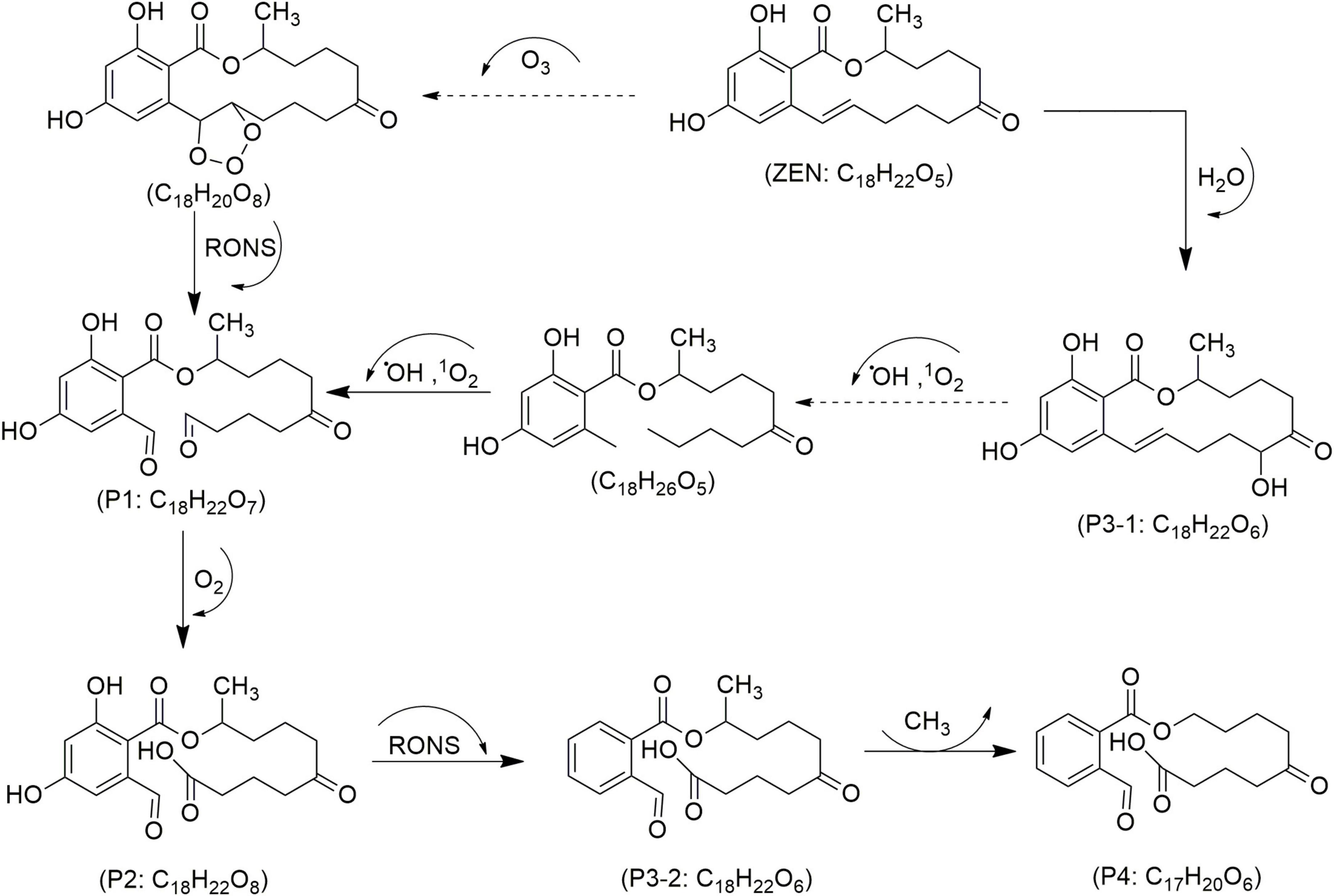
Figure 7. Proposed mechanisms and degradation products (P) of zearalenone (ZEN) in food under cold atmospheric plasma (CAP) treatment, adapted from Liu et al. (2024).
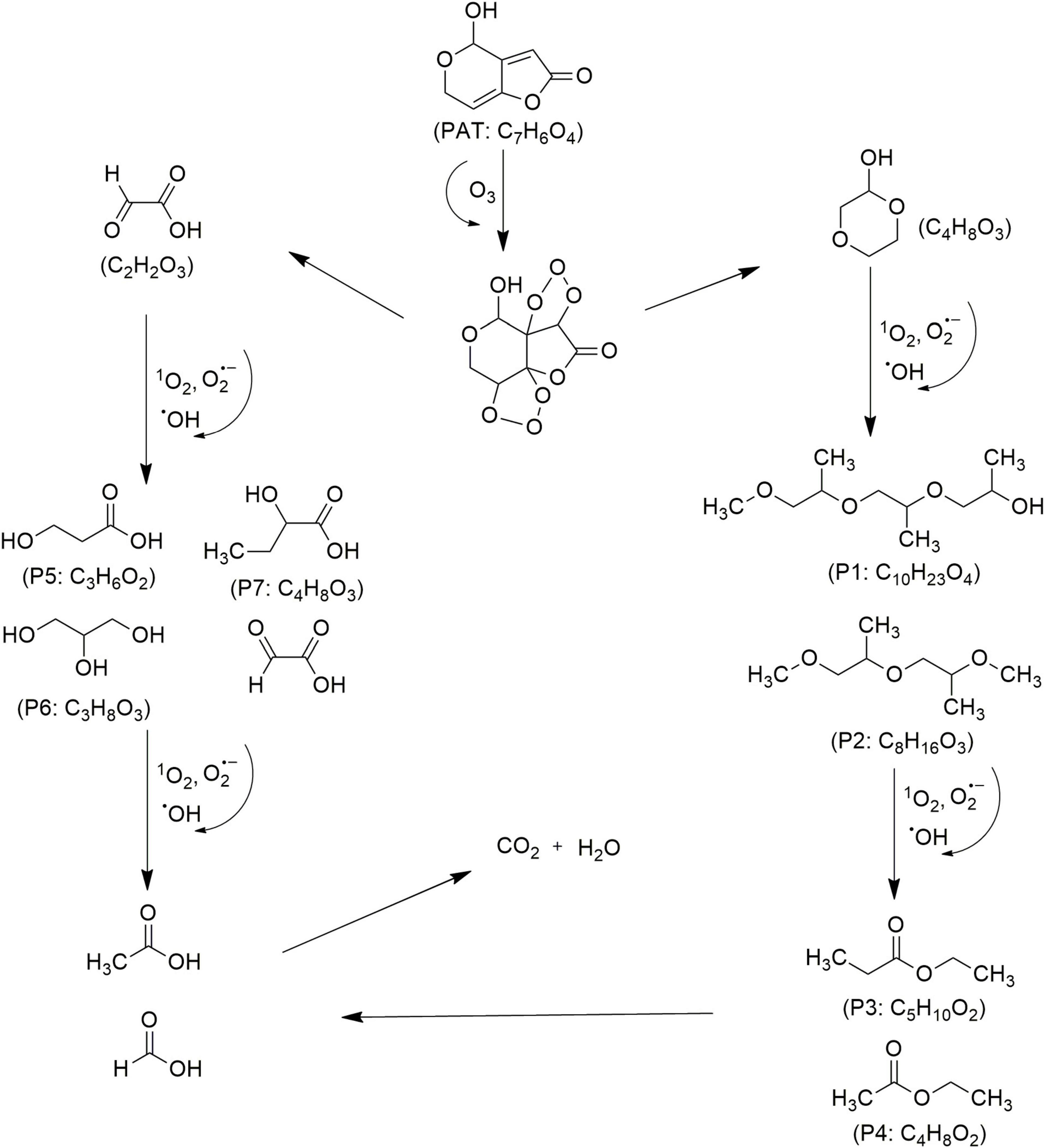
Figure 8. Proposed mechanisms and degradation products (P) of patulin (PAT) in food under cold atmospheric plasma (CAP) treatment, adapted from Shirazi et al. (2025) and Xue et al. (2021).
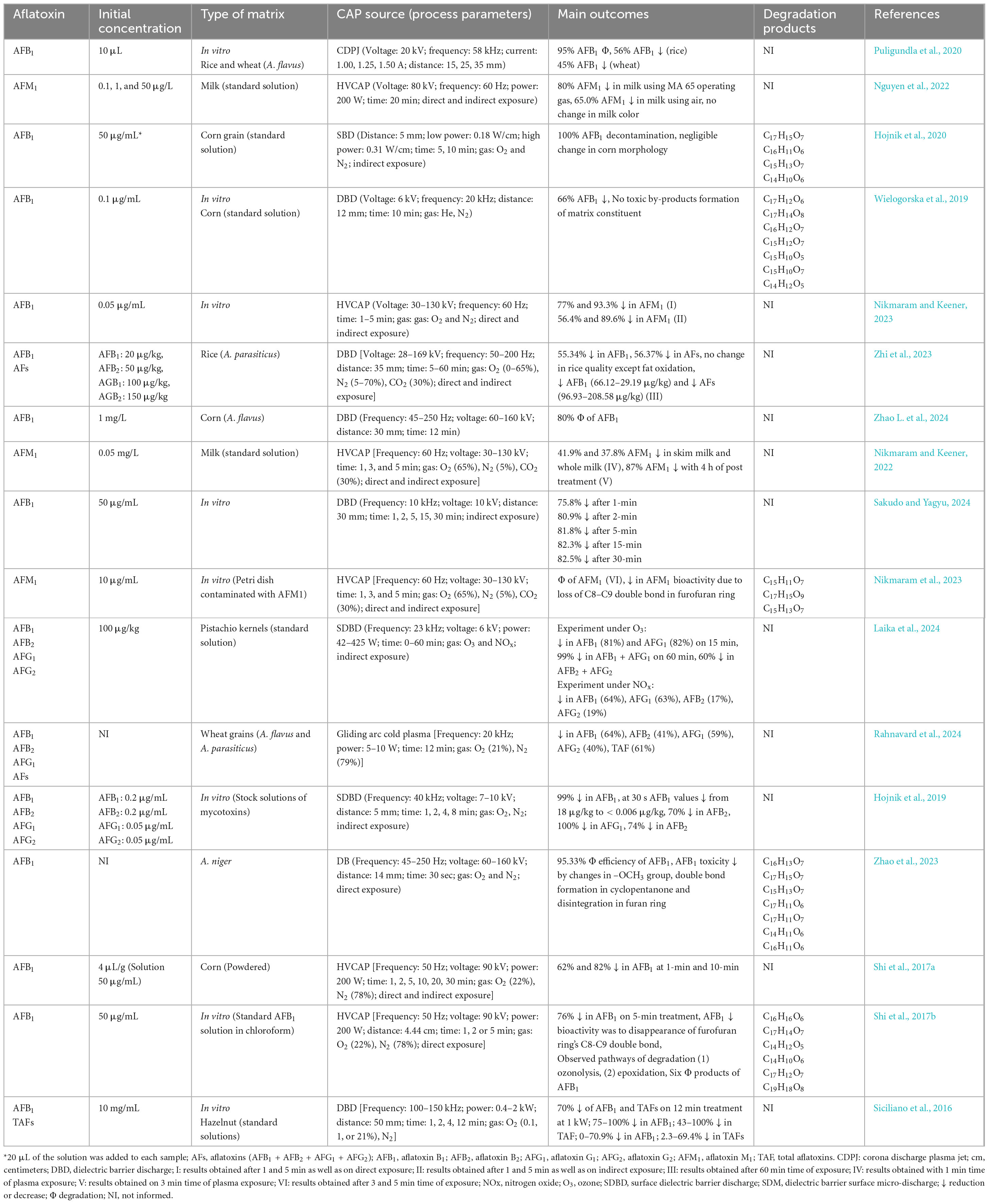
Table 2. Summary of main outcomes from studies using cold atmospheric pressure plasma (CAP) in decontaminating aflatoxins.
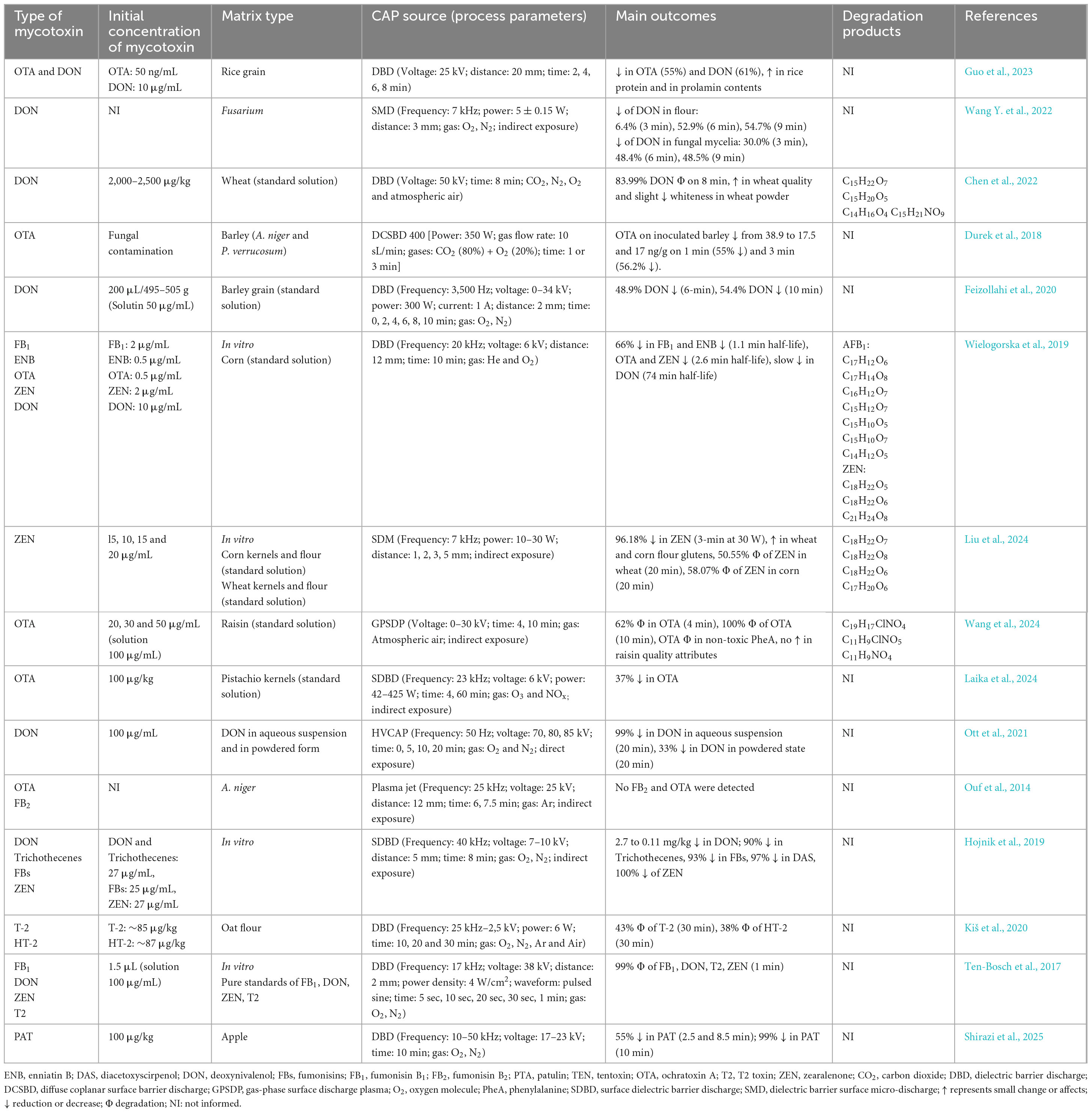
Table 3. Summary of main outcomes from studies using cold atmospheric pressure plasma (CAP) in decontaminating other mycotoxins.
The toxicity of AFB1 is attributed to the double bond between carbons 8 and 9 (C8 = C9) in furan ring, and disrupting this bond produces less toxic degraded products (Hojnik et al., 2021), depicted in Figure 4. In a study by Nguyen et al. (2022), CAP’s effect on aflatoxin M1 (AFM1) was investigated, reporting reductions across all treatments, with the most significant decrease of 60% occurring after 20 min of exposure to a high voltage generated CAP (Table 2). Similarly, Nikmaram and Keener (2022) achieved an even higher AFM1 degradation rate of 87% with just 4 min of CAP exposure (30–130 kV, 60 Hz) in milk. The analysis in this study indicated three TPs resulted from AFM1 degradation (i.e., C15H11O7, C17H15O9, and C15H13O7). Each TP was formed as a result from CAP induced chemical modifications in furofuran ring, particularly by the disruption of the double bond between C8 and C9, leading to a reduction in toxicity of the molecule (Nikmaram et al., 2023).
In pistachio kernels treated with ozone-based CAP, AFB1 and AFG1 were reduced by 81 and 82% within 15 min, respectively, reaching 99% after 60 min, while CAP with NOx reduced AFB1 and AFG1 by 64 and 63%, respectively (Laika et al., 2024). In wheat, CAP treatment led to 61% reduction in AFs, with specific decreases of 64, 41, 59 and 40% for AFB1, AFB2, AFG1 and AFG2, respectively (Rahnavard et al., 2024). The reduction rate of AFB1 increased from 62 to 82% in corn powder treated by a high voltage CAP for one to 10 min (Shi et al., 2017a). A 5-min treatment of high voltage CAP, revealed 76% degradation in AFB1 in standard solutions, suggesting ozonolysis and epoxidation that led to the breakdown of C8 = C9 in furofuran, which resulted in six TPs–e.g., C16H16O6, C17H14O7, C14H12O5, C14H10O6, C17H12O7, and C19H18O8 (Shi et al., 2017b; Table 2).
Hojnik et al. (2020) achieved complete AFB1 degradation in corn grains after 5–10 min treatment of CAP generated with SBD. Except for a negligible alteration in sample morphology, CAP application led to AFB1 degradation based on its four TPs such as C17H15O7, C16H11O6, C15H13O7, and C14H10O6 (Hojnik et al., 2020). In another study, a 30-s treatment of CAP with SDBD demonstrated 99% reduction in AFB1 and 70% in AFB2, while 100 and 74% reductions were observed for AFG1 and AFG2, respectively (Hojnik et al., 2019). In rice inoculated with A. parasiticus, CAP reduced AFs by 56.4% after 60 min with a DBD system using a mixture of O2, N2, and CO2. However, minor fat oxidation was noted probably because of insource generated reactive species (Zhi et al., 2023). In another study, corn inoculated with A. flavus and treated with CAP at intervals of 2, 4, 8, and 12 min showed progressive AFB1 reductions of 23.4, 41.8, 64.4, and 80.0% (Zhao L. et al., 2024). Additionally, AFB1 degradation by CAP in an A. niger cell suspension reached 95.3% within 30 s. The data indicated a disintegrated chemical structure of AFB1, resulting in seven TPs designated as products (Ps) in Figure 4, e.g., C16H13O7 (P1), C17H15O7 (P2), C15H13O7 (P3), C17H11O6 (P4), C17H11O7 (P5), C14H11O6 (P6) and C16H11O6 (P7) (Zhao et al., 2023). Previously, Wielogorska et al. (2019) obtained a 66% reduction of AFB1 after treatment by CAP generated with DBD demonstrated, mainly due to a high voltage in oxygen-rich environment that boosted oxygen radical and ozone formation, thereby enhancing peroxidation. This reaction primarily modified the terminal furans, with lactone and methoxy groups unchanged, indicating selective site reactivity with a net result of seven TPs such as C17H12O6, C17H14O8, C16H12O7, C15H12O7, C15H10O5, C15H10O7, and C14H12O5, respectively (Wielogorska et al., 2019). Similarly, wheat and rice inoculated with A. flavus, treated with CAP generated by CDPJ using variable frequency, current and electrodes distance, revealed remarkably a reduction of 95% of AFB1 of which 45 to 56% were assigned to wheat and rice samples (Puligundla et al., 2020). Moreover, DBD-based CAP for a 12 min treatment of hazelnuts showed the reduction rates between 43 and 100% for AFs, and 75 to 100% for AFB1 (Siciliano et al., 2016). Sakudo and Yagyu (2024) reported time-dependent reductions in AFB1, achieving 75.8% decreased within 1 min and up to 82.5% over 30 min of CAP exposure.
Studies have demonstrated the ability of CAP to decontaminate other types of mycotoxins (Table 3). Wang Y. et al. (2022) investigated the impact of CAP generated with SMD on Fusarium graminearum and observed a significant reduction in DON production across all samples evaluated, with results varying according to exposure duration. Notably, a 6-min CAP treatment resulted in over 50% reduction in DON levels. Similarly, Chen et al. (2022) reported that applying CAP generated with DBD at 50 kV to wheat for 8 min led to a DON degradation of over 62–84%. The authors mentioned that seven TPs of DON (Figure 5) were confirmed according to four molecular formulas, e.g., C15H22O7 (P1/1–3), C15H20O5 (P2), C14H16O4 (P3), and C15H21NO9 (P4/1–2) (Chen et al., 2022). Among these, C15H20O5 (P2), known as de-epoxy deoxynivalenol (DOM-1), was noted for its reduced toxicity compared to its parent DON molecule (Figure 5). DON’s toxicity is primarily associated with the hydroxyl (-OH) group at C3, the double bond between C9 and C10, and the sigma bond between C12 and C13 in epoxy group. Since the degradation products lack these or some of these chemical features, are considered as less harmful (Chen et al., 2022). Notably, the breakdown of the C12-C13 epoxy ring may be more prevalent in acidic environments, suggesting that food matrices with lower pH may facilitate this process (Guo et al., 2023). In the same study, it was also demonstrated that CAP generated with DBD effectively reduces the levels of both DON and OTA. The highest reduction was obtained after 8 min of treatment, reaching 61 and 56% reduction rates for DON and OTA, respectively (Guo et al., 2023). Regarding OTA, CAP treatment promotes the hydrolysis of the amide bond, thus resulting in the formation of less toxic compounds like phenylalanine (C9H9NO2) and ochratoxin-α (OT-α: P2) (Figure 6). It is also notable that ozone generated by CAP can oxidize the chlorinated ring in the OTA moiety, which leads to its breakdown into amino acids or free chlorine, further contributing to detoxification (Guo et al., 2023; Wang L. et al., 2022). Wang et al. (2024) demonstrated the effectiveness of CAP generated with GPSDP in degrading OTA in raisin, achieving 62% degradation at a four-min treatment and reaching 100% with a 10-min treatment (Table 3), without affecting matrix quality. Three OTA degradation products such as C19H17ClNO4 (P1), C11H9ClNO5 (P2) and C11H9ClO4 (P3) were identified (Figure 6), likely resulting from ozone, peroxides, and free radicals, as detailed elsewhere (Guo et al., 2023; Wang L. et al., 2022; Wang et al., 2024).
In corn containing six mycotoxins and treated with CAP using a DBD system, Wielogorska et al. (2019) observed 60–66% reductions of AFB1 and FB1 within 10 min. The study led to the identification of TPs only for AFB1 (e.g., C17H12O6, C17H14O8, C16H12O7, C15H12O7, C15H10O5, C15H10O7 and C14H12O5) and ZEN (e.g., C18H22O5, C18H22O6 and C21H24O8). Helium plasma enhanced the degradation efficiency when compared with mixed nitrogen-oxygen, primarily due to increased oxygen radical formation and a preference for peroxidation reactions (Wielogorska et al., 2019). A three-min CAP treatment using SDM at 30 watts achieved 96.2% degradation of ZEN in corn and wheat kernels, as well as their flour. However, individual treatments of wheat and corn alone over 20 min showed ZEN reductions of 50.5–58.1%, with no significant changes to gluten in either matrix. Four ZEN degradation products, presented as C18H22O7 (P1), C18H22O8 (P2), C18H22O6 (P3/1–2) and C17H20O6 (P4) in Figure 7, were identified, primarily from oxidative cleavage of the olefinic C11 = C12 bond, following well-explored pathways (Liu et al., 2024). Key factors in ZEN degradation included irradiation distance, treatment time, RONS, and free radical oxidation.
The use of CAP for mycotoxin decontamination has primarily focused on the widely consumed cereals, grains, and their derivatives, with limited attention given to perishable products like fruits and vegetables. Research on perishable matrices has mainly addressed microbial control and shelf-life extension, leaving the potential for mycotoxin mitigation largely unexplored (Yinxin et al., 2022; Pinela and Ferreira, 2017; Yarabbi et al., 2023). A recent study highlighted the effectiveness of CAP generated with DBD in degrading PAT in fresh-cut apple slices while simultaneously enhancing their quality attributes (Shirazi et al., 2025). Treatment with 23 kV for 2.5 and 8.5 min gave 55 to 99% reduction of PAT to the undetectable levels, demonstrating the importance of high voltage and optimized exposure time. The degradation was attributed to ROS produced during plasma discharge, which facilitated oxidative breakdown into seven TPs (Figure 8) including C10H23O4 (P1), C8H16O3 (P2), C5H10O2 (P3), C4H8O2 (P4), C3H6O2 (P5), C3H8O3 (P6), and C4H8O3 (P7) (Shirazi et al., 2025; Xue et al., 2021). These findings highlight the dual benefits of CAP in mitigating mycotoxins and enhancing food quality. The demonstrated efficacy of CAP for perishable products like fresh-cut fruits provides a foundation for broader applications and indicates the potential for further research and development in food safety and preservation strategies.
The efficiency of CAP in degrading different mycotoxins and reducing their toxicity depends on its ability to induce structural modifications in the target mycotoxin molecules, particularly at the molecular sites responsible for their toxicity. Degradation is confirmed only when less toxic molecules are generated. However, despite the significance of understanding the toxicity of degradation products, only 7 out of the 30 studies reviewed provided data on the by-products of the given toxins degraded by the CAP technology. In conformity with these results, the TPs mentioned and those newly emerging should be comprehensively studied for their potential toxicities and risks. While challenging, accurately measuring the maximum number of detectable TPs requires highly sensitive methods, likely the use of both high-resolution mass spectrometry (HRMS) and low-resolution MS, for inclusive proof of identities, validation, and quantification of all novel TPs. To ensure compliance with the guidelines set by the World Health Organization (WHO) and the Food and Agriculture Organization (FAO), both in vitro and in vivo studies are essential to assess the overall toxicological profiles of the TPs generated by CAP treatments.
6 Effects of cold atmospheric plasma on the food matrix
Assessing the impact of CAP on food products is crucial, particularly regarding their physicochemical, sensory, and nutritional properties. Research indicates that CAP treatment does not significantly alter the nutritional composition of food matrices. Studies on raw materials like rice and wheat, as well as processed products like wheat flour, show that starch content remains largely unaffected by CAP exposure (Guo et al., 2023; Chen et al., 2022; Zhi et al., 2023; Zhao L. et al., 2024).
Regarding the sensory attributes, specifically color, CAP treatment of rice revealed no significant changes in its characteristic appearance (Zhi et al., 2023). In the case of milk, however, Nikmaram and Keener (2022) observed a non-perceptible color change in skim milk, while a slight but perceptible yellowing occurred in whole milk. This yellowing effect is likely due to the oxidation of fats and proteins induced by CAP which is a source of highly reactive species (Ali et al., 2023b; Segat et al., 2015).
The limited impact of CAP on the food matrices may also be attributed to its inability to penetrate deeply into the product. For example, in rice, the presence of husks reduces CAP’s effectiveness (Guo et al., 2023). Similar results were observed in corn kernels, where analytical methods such as X-ray photoelectron spectroscopy (XPS) and secondary ion mass spectrometry (SIMS) demonstrated that 8 min treatment with air plasma generated with SBD led to the slight oxidation of corn kernel surface, while scanning electron microscopy (SEM) showed no significant differences between CAP-treated and control samples (Hojnik et al., 2020).
CAP treatment can have varying effects on the food protein composition. While there were significant differences in specific proteins, such as prolamin and globulin, between the CAP-treated and control samples, the overall protein content remained unaffected. These findings are consistent with those of Zhi et al. (2023), where even prolonged exposure to CAP (60 min) did not result in significant changes. Regarding fatty acid (FA) content, CAP treatment was observed to increase the number of FA types over time, generating 3 to 5 new FAs. However, the primary FA components in wheat showed no significant difference compared to the control, suggesting that CAP does not alter the total FA content (Guo et al., 2023). In contrast, Zhi et al. (2023) reported a significant upsurge of 275.3% in free FA content in rice, indicating that CAP can indeed influence such composition. These findings collectively indicate that the application of CAP using different system parameters and exposure times had none or negligible qualitative effects on food matrices, as described for various items including dairy products (milk), and cereal grains (e.g., rice, wheat, corn).
7 Challenges of cold atmospheric plasma application in the food industry
CAP is an emerging technology in food mycotoxin decontamination due to its high efficiency, environmentally friendly nature, and dual functionality in degrading toxins and inhibiting the growth of toxigenic microorganisms (Marshall et al., 2020; Alizadeh et al., 2021; Ouf et al., 2014; Wang et al., 2020). Despite these advantages, several challenges obstruct its industrial application. Scalability remains a major challenge, while the DBD model, as the easiest of all possible options and most used in research, presents significant obstacles for industrial implementation (Neuenfeldt et al., 2023; Guo et al., 2023). The lack of standardized range for key parameters such as discharge frequency, voltage, and power, also complicate its optimization for large-scale use (Neuenfeldt et al., 2023). Future advancements should prioritize the development of modular, scalable CAP systems and the establishment of standardized operational parameters to broaden CAP’s applicability while achieving consistent and reproducible outcomes. The cost of CAP equipment, energy demands, and limited penetration of reactive species in complex food matrices remains another barrier to be addressed (Chakka et al., 2021). Designing cost-effective, energy-efficient CAP devices integrated with mechanisms like agitation systems or rotating conveyor belts could enhance reactive species distribution, improve decontamination efficiency, and facilitate industrial applications.
The literature highlights a well-understood degradation pathway for mycotoxins like AFB1 and DON. However, there is limited knowledge regarding the degradation products and cytotoxicity of other important mycotoxins, such as AFM1 and OTA (Hojnik et al., 2020; Deng et al., 2020). Additionally, research on the effects of CAP on the physicochemical, nutritional, and sensory properties of food matrices is scarce, underscoring the need for further investigation in this area while maximizing CAP efficiency (Guo et al., 2023). Taken together, overcoming these obstacles will need interdisciplinary collaboration across engineering, toxicology, and food science. Such efforts will be pivotal in advancing CAP as a sustainable, scalable, and reliable technology in the food sector.
8 Concluding remarks and future perspectives
This systematic review confirms the effectiveness of CAP in degrading mycotoxins such as AFB1 and DON. The extensive knowledge of the degradation compounds and their reduced toxicity underscores CAP’s potential in food safety applications. However, the review highlights a significant gap in research regarding the cytotoxicity of these degradation by-products. Future studies should focus on the cytotoxicological assessment of by-products from the breakdown of a broader range of mycotoxins, including AFs, FBs, OTs, ZEN, DON, T-2, HT-2 and PAT. CAP also shows promise in preventing mycotoxin contamination by inhibiting the growth of toxigenic fungi. The technology effectively targets fungal spores and damages the DNA responsible for mycotoxin biosynthesis, offering a dual approach to both degrading existing mycotoxins and preventing their formation. Despite its potential, the industrial application of CAP faces several challenges, particularly regarding the practical viability of large-scale implementation. Key obstacles include the need for more robust equipment design and the optimization of operational parameters, as there is currently no consensus on the best practices for these variables. The literature suggests that CAP has a minimal impact on the nutritional and sensory qualities of food, making it an attractive option for the food industry. However, research in this area remains limited, highlighting the need for more studies to fully understand the CAP efficacy on different food matrices. As a cutting-edge method for mycotoxin decontamination, addressing these research gaps is crucial for advancing the development and industrial applicability of CAP. Further exploration will enhance the efficiency of decontamination processes, ultimately contributing to the production of safer food products on a larger scale.
Data availability statement
The original contributions presented in this study are included in this article/supplementary material, further inquiries can be directed to the corresponding author.
Author contributions
AO: Conceptualization, Investigation, Writing – original draft, Writing – review and editing. SA: Formal Analysis, Writing – review and editing. CC: Validation, Writing – review and editing. SU: Investigation, Writing – original draft. KP: Investigation, Writing – original draft. JW: Visualization, Writing – review and editing. NH: Visualization, Writing – review and editing. CO: Funding acquisition, Supervision, Writing – review and editing.
Funding
The authors declare that financial support was received for the research, authorship, and/or publication of this article. This work was supported by Fundação de Amparo à Pesquisa do Estado de São Paulo (FAPESP) under grant numbers: 2022/03952-1, 2023/05989-2, and 2024/00896-9, as well as Coordenação de Aperfeiçoamento de Pessoal de Nível Superior - Brasil (CAPES) - Financial Code 001.
Acknowledgments
We greatly acknowledge the Fundação de Amparo à Pesquisa do Estado de São Paulo (FAPESP) and Coordenação de Aperfeiçoamento de Pessoal de Nível Superior - Brasil (CAPES).
Conflict of interest
The authors declare that the research was conducted in the absence of any commercial or financial relationships that could be construed as a potential conflict of interest.
Generative AI statement
The authors declare that no Generative AI was used in the creation of this manuscript.
Publisher’s note
All claims expressed in this article are solely those of the authors and do not necessarily represent those of their affiliated organizations, or those of the publisher, the editors and the reviewers. Any product that may be evaluated in this article, or claim that may be made by its manufacturer, is not guaranteed or endorsed by the publisher.
References
Abdel-Fattah, E. (2013). Investigation of capacitively coupled argon plasma driven at various frequencies and validation of surface waves excitation. Phys. Lett. A. 377, 297–302. doi: 10.1016/j.physleta.2012.11.014
Abrunhosa, L., Morales, H., Soares, C., Calado, T., Vila-Chã, A. S., Pereira, M., et al. (2016). A review of mycotoxins in food and feed products in Portugal and estimation of probable daily intakes. Crit. Rev. Food Sci. Nutr. 56, 249–265. doi: 10.1080/10408398.2012.720619
Akbari, P., Braber, S., Varasteh, S., Alizadeh, A., Garssen, J., and Fink-Gremmels, J. (2017). The intestinal barrier as an emerging target in the toxicological assessment of mycotoxins. Arch. Toxicol. 91, 1007–1029. doi: 10.1007/s00204-016-1794-8
Ali, S., Franco, B. B., Rezende, V. T., Freire, L. G. D., de Paiva, E. L., Haikal, M. C. F., et al. (2024). Exposure assessment of children to dietary mycotoxins: a pilot study conducted in Ribeirão Preto, São Paulo, Brazil. Food Res. Int. 180:114087. doi: 10.1016/j.foodres.2024.114087
Ali, S., Freire, L. G. D., Rezende, V. T., Noman, M., Ullah, S., and Abdullah, et al. (2023a). Occurrence of mycotoxins in foods: unraveling the knowledge gaps on their persistence in food production systems. Foods 12:4314. doi: 10.3390/foods12234314
Ali, S., Rezende, V. T., Ullah, S., de Paiva, E. L., Tonin, F. G., and Abdullah, et al. (2023b). Food processing and challenges in the food production and quality: the foodomics approach. Food Biosci. 56:103217. doi: 10.1016/j.fbio.2023.103217
Alizadeh, A. M., Hashempour-Baltork, F., Khaneghah, A. M., and Hosseini, H. (2021). New perspective approaches in controlling fungi and mycotoxins in food using emerging and green technologies. Curr. Opin. Food Sci. 39, 7–15. doi: 10.1016/j.cofs.2020.12.006
Alvito, P., and Pereira-da-Silva, L. (2022). Mycotoxin exposure during the first 1000 days of life and its impact on children’s health: a clinical overview. Toxins 14:189. doi: 10.3390/toxins14030189
Anderson, N. M. (2019). Recent advances in low moisture food pasteurization. Curr. Opin. Food Sci. 29, 109–115. doi: 10.1016/j.cofs.2018.11.001
Arcella, D., Gergelova, P., Innocenti, M. L., and Steinkellner, H. (2017). Human and animal dietary exposure to T-2 and HT-2 toxin. EFSA J. 15:e04972. doi: 10.2903/j.efsa.2017.4972
Arce-López, B., Lizarraga, E., López De Mesa, R., and González-Peñas, E. (2021). Assessment of exposure to mycotoxins in Spanish children through the analysis of their levels in plasma samples. Toxins 13:150. doi: 10.3390/toxins13020150
Basso, A. B. G., Ali, S., Corassin, C. H., Rosim, R. E., and Oliveria, C. A. F. (2023). Individual and combined decontamination effect of fermentation and ultrasound on aflatoxin B1 in wheat-based doughs: a preliminary study. Qual. Assur. Saf. Crop. 15, 96–103. doi: 10.15586/qas.v15i3.1244
Borutova, R., Aragon, Y. A., Nährer, K., and Berthiller, F. (2012). Co-occurrence and statistical correlations between mycotoxins in feedstuffs collected in the Asia–Oceania in 2010. Anim. Feed Sci. Technol. 178, 190–197. doi: 10.1016/j.anifeedsci.2012.09.015
Chakka, A. K., Sriraksha, M. S., and Ravishankar, C. N. (2021). Sustainability of emerging green non-thermal technologies in the food industry with food safety perspective: a review. Lwt 151:112140. doi: 10.1016/j.lwt.2021.112140
Chen, X., Qiu, Y., Zhang, J., Guo, Y., Ding, Y., and Lyu, F. (2022). Degradation efficiency and products of deoxynivalenol treated by cold plasma and its application in wheat. Food Control 136:108874. doi: 10.1016/j.foodcont.2022.108874
Clark, H. A., and Snedeker, S. M. (2006). Ochratoxin A: its cancer risk and potential for exposure. J. Toxicol. Environ. Health B Crit. Rev. 9, 265–296. doi: 10.1080/15287390500195570
Deng, L.-Z., Tao, Y., Mujumdar, A. S., Pan, Z., Chen, C., Yang, X.-H., et al. (2020). Recent advances in non-thermal decontamination technologies for microorganisms and mycotoxins in low-moisture foods. Trends Food Sci. Technol. 106, 104–112. doi: 10.1016/j.tifs.2020.10.012
Dickenson, A., Britun, N., Nikiforov, A., Leys, C., Hasan, M. I., and Walsh, J. L. (2018). The generation and transport of reactive nitrogen species from a low temperature atmospheric pressure air plasma source. Phys. Chem. Chem. Phys. 20, 28499–28510. doi: 10.1039/c8cp05762a
Dickenson, A., Morabit, Y., Hasan, M. I., and Walsh, J. L. (2017). Directional mass transport in an atmospheric pressure surface barrier discharge. Sci. Rep. 7:14003. doi: 10.1038/s41598-017-14117-1
Dünnbier, M., Schmidt-Bleker, A., Winter, J., Wolfram, M., Hippler, R., Weltmann, K.-D., et al. (2013). Ambient air particle transport into the effluent of a cold atmospheric-pressure argon plasma jet investigated by molecular beam mass spectrometry. J. Phys. D Appl. Phys. 46:435203. doi: 10.1088/0022-3727/46/43/435203
Durek, J., Schlüter, O., Roscher, A., Durek, P., and Fröhling, A. (2018). Inhibition or stimulation of ochratoxin A synthesis on inoculated barley triggered by diffuse coplanar surface barrier discharge plasma. Front. Microbiol. 9:2782. doi: 10.3389/fmicb.2018.02782
EFSA (2004). Opinion of the scientific panel on contaminants in the food chain on a request from the commission related to aflatoxin B1 as undesirable substance in animal feed. EFSA J. 39, 1–27. doi: 10.2903/j.efsa.2004.39
EU (2023). Commission Regulation (EU) 2023/915 of 25 April 2023 on Maximum Levels for Certain Contaminants in Food and Repealing Regulation (EC) No 1881/2006. Brussels: European Union.
Fakhri, Y., Rahmani, J., Oliveira, C. A. F., Franco, L. T., Corassin, C. H., Saba, S., et al. (2019). Aflatoxin M1 in human breast milk: a global systematic review, meta-analysis, and risk assessment study (Monte Carlo simulation). Trends Food Sci. Technol. 88, 333–342. doi: 10.1016/j.tifs.2019.03.013
Feizollahi, E., Iqdiam, B., Vasanthan, T., Thilakarathna, M. S., and Roopesh, M. S. (2020). Effects of atmospheric-pressure cold plasma treatment on deoxynivalenol degradation, quality parameters, and germination of barley grains. Appl. Sci. 10:3530. doi: 10.3390/app10103530
Gavahian, M., and Cullen, P. J. (2019). Cold plasma as an emerging technique for mycotoxin-free food: efficacy, mechanisms, and trends. Crit. Rev. Food Sci. Nutr. 56, 193–214. doi: 10.1080/87559129.2019.1630638
Gavahian, M., Mathad, G. N., Oliveira, C. A. F., and Khaneghah, A. M. (2021). Combinations of emerging technologies with fermentation: interaction effects for detoxification of mycotoxins? Food Res. Int. 141:110104. doi: 10.1016/j.foodres.2021.110104
Gruber-Dorninger, C., Novak, B., Nagl, V., and Berthiller, F. (2017). Emerging mycotoxins: beyond traditionally determined food contaminants. J. Agric. Food Chem. 65, 7052–7070. doi: 10.1021/acs.jafc.6b03413
Guo, J., He, Z., Ma, C., Li, W., Wang, J., Lin, F., et al. (2023). Evaluation of cold plasma for decontamination of molds and mycotoxins in rice grain. Food Chem. 402:134159. doi: 10.1016/j.foodchem.2022.134159
Han, L., Boehm, D., Amias, E., Milosavljeviæ, V., Cullen, P. J., and Bourke, P. (2016). Atmospheric cold plasma interactions with modified atmosphere packaging inducer gases for safe food preservation. Innov. Food Sci. Emerg. Technol. 38, 384–392. doi: 10.1016/j.ifset.2016.09.026
Han, Q.-Y., Wen, X., Gao, J.-Y., Zhong, C.-S., and Ni, Y.-Y. (2023). Application of plasma-activated water in the food industry: a review of recent research developments. Food Chem. 405:134797. doi: 10.1016/j.foodchem.2022.134797
Haque, M. A., Wang, Y., Shen, Z., Li, X., Saleemi, M. K., and He, C. (2020). Mycotoxin contamination and control strategy in human, domestic animal, and poultry: a review. Microb. Pathog. 142:104095. doi: 10.1016/j.micpath.2020.104095
Harikrishna, S., Anil, P. P., Shams, R., and Dash, K. K. (2023). Cold plasma as an emerging nonthermal technology for food processing: a comprehensive review. J. Agric. Food Res. 14:100747. doi: 10.1016/j.jafr.2023.100747
Hasan, M. I., and Walsh, J. L. (2017). Influence of gas flow velocity on the transport of chemical species in an atmospheric pressure air plasma discharge. Appl. Phys. Lett. 110:134102. doi: 10.1063/1.4979178
Hasan, M. I., Morabit, Y., Dickenson, A., and Walsh, J. L. (2017). Impact of electrode geometry on an atmospheric pressure surface barrier discharge. Appl. Phys. Lett. 110:264101. doi: 10.1063/1.4985030
Hertwig, C., Meneses, N., and Mathys, A. (2018). Cold atmospheric pressure plasma and low energy electron beam as alternative nonthermal decontamination technologies for dry food surfaces: a review. Trends Food Sci. Technol. 77, 131–142. doi: 10.1016/j.tifs.2018.05.011
Hojnik, N., Cvelbar, U., Tavèar-Kalcher, G., Walsh, J. L., and Križaj, I. (2017). Mycotoxin decontamination of food: cold atmospheric pressure plasma versus “classic” decontamination. Toxins 9:151. doi: 10.3390/toxins9050151
Hojnik, N., Modic, M., Tavèar-Kalcher, G., Babiè, J., Walsh, J. L., and Cvelbar, U. (2019). Mycotoxin decontamination efficacy of atmospheric pressure air plasma. Toxins 11:219. doi: 10.3390/toxins11040219
Hojnik, N., Modic, M., Walsh, J. L., Žigon, D., Javornik, U., Plavec, J., et al. (2021). Unravelling the pathways of air plasma induced aflatoxin B1 degradation and detoxification. J. Hazard. Mater. 403:123593. doi: 10.1016/j.jhazmat.2020.123593
Hojnik, N., Modic, M., Žigon, D., Kovaè, J., Jurov, A., Dickenson, A., et al. (2020). Cold atmospheric pressure plasma-assisted removal of aflatoxin B1 from contaminated corn kernels. Plasma Process. Polym. 18:2000163. doi: 10.1002/ppap.202000163
Hoppanová, L., Dylíková, J., Kováèik, D., Medvecká, V., Ïurina, P., Kryštofová, S., et al. (2020). The effect of cold atmospheric pressure plasma on Aspergillus ochraceus and ochratoxin A production. Antonie van Leeuwenhoek 113, 1479–1488. doi: 10.1007/s10482-020-01457-8
IARC, (2023). Agents Classified by the IARC Monographs, 1–134 – IARC Monographs on the Identification of Carcinogenic Hazards to Humans. Available online at: https://monographs.iarc.who.int/agents-classified-by-the-iarc/ (Accessed November 30, 2024).
Kaur, M., Hüberli, D., and Bayliss, K. L. (2020). Cold plasma: exploring a new option for management of postharvest fungal pathogens, mycotoxins and insect pests in Australian stored cereal grain. Crop Pasture Sci. 71, 715–724. doi: 10.1071/CP20078
Khan, R. (2024). Mycotoxins in food: occurrence, health implications, and control strategies-A comprehensive review. Toxicon 248:108038. doi: 10.1016/j.toxicon.2024.108038
Khaneghah, A. M., Fakhri, Y., Gahruie, H. H., Niakousari, M., and Sant’ana, A. (2019). Mycotoxins in cereal-based products during 24 years (1983–2017): a global systematic review. Trends Food Sci. Technol. 91, 95–105. doi: 10.1016/j.tifs.2019.06.007
Kiš, M., Miloševiæ, S., Vuliæ, A., Herceg, Z., Vukušiæ, T., and Pleadin, J. (2020). Efficacy of low pressure DBD plasma in the reduction of T-2 and HT-2 toxin in oat flour. Food Chem. 316:126372. doi: 10.1016/j.foodchem.2020.126372
Knutsen, H. K., Alexander, J., Barregård, L., Bignami, M., Brüschweiler, B., Ceccatelli, S., et al. (2017). Risks to human and animal health related to the presence of deoxynivalenol and its acetylated and modified forms in food and feed. EFSA J. 15:e04718. doi: 10.2903/j.efsa.2017.4718
Kovaèeviæ, V. V., Sretenoviæ, G. B., Slikboer, E., Guaitella, O., Sobota, A., and Kuraica, M. M. (2018). The effect of liquid target on a nonthermal plasma jet—Imaging, electric fields, visualization of gas flow, and optical emission spectroscopy. J. Phys. D Appl. Phys. 51:065202. doi: 10.1088/1361-6463/aaa288
Laika, J., Viteritti, E., Molina-Hernandez, J. B., Sergi, M., Neri, L., Laurita, R., et al. (2024). Efficiency of cold atmospheric plasma under ozone (O3) and nitrogen oxide (NOx) regimes on the degradation of aflatoxins and ochratoxin A in solid state and in spiked pistachio kernels. Food Control 159:110286. doi: 10.1016/j.foodcont.2024.110286
Liu, M., Feng, J., Fan, Y., Yang, X., Chen, R., Xu, C., et al. (2024). Application of atmospheric cold plasma for zearalenone detoxification in cereals: kinetics, mechanisms, and cytotoxicity analysis. J. Adv. Res. doi: 10.1016/j.jare.2024.04.024 [Epub ahead of print].
Loi, M., Logrieco, A. F., Pusztahelyi, T., Leiter, É, Hornok, L., and Pócsi, I. (2023). Advanced mycotoxin control and decontamination techniques in view of an increased aflatoxin risk in Europe due to climate change. Front. Microbiol. 13:1085891. doi: 10.3389/fmicb.2022.1085891
Majeed, M., Khaneghah, A. M., Kadmi, Y., Khan, M. U., and Shariati, M. A. (2017). Assessment of ochratoxin A in commercial corn and wheat products. Curr. Res. Nutr. Food Sci. 14, 116–120. doi: 10.2174/15734013136661703301558231
Marshall, H., Meneely, J. P., Quinn, B., Zhao, Y., Bourke, P., Gilmore, B. F., et al. (2020). Novel decontamination approaches and their potential application for post-harvest aflatoxin control. Trends Food Sci. Technol. 106, 489–496. doi: 10.1016/j.tifs.2020.11.001
Mir, S. A., Dar, B. N., Shah, M. A., Sofi, S. A., Hamdani, A. M., Oliveira, C. A. F., et al. (2021). Application of new technologies in decontamination of mycotoxins in cereal grains: challenges, and perspectives. Food Chem. Toxicol. 148:111976. doi: 10.1016/j.fct.2021.111976
Misra, N. N., and Jo, C. (2017). Applications of cold plasma technology for microbiological safety in meat industry. Trends Food Sci. Technol. 64, 74–86. doi: 10.1016/j.tifs.2017.04.005
Misra, N. N., Yadav, B., Roopesh, M. S., and Jo, C. (2018). Cold plasma for effective fungal and mycotoxin control in foods: mechanisms, inactivation effects, and applications. Comprehens. Rev. Food Sci. Food Saf. 18, 106–120. doi: 10.1111/1541-4337.12398
Morabit, Y., Hasan, M. I., Whalley, R. D., Robert, E., Modic, M., and Walsh, J. L. (2021). A review of the gas and liquid phase interactions in low-temperature plasma jets used for biomedical applications. Eur. Phys. J. D. 75, 01–26. doi: 10.1140/epjd/s10053-021-00015-1
Moretti, A., Pascale, M., and Logrieco, A. F. (2019). Mycotoxin risks under a climate change scenario in Europe. Trends Food Sci. Technol. 84, 38–40. doi: 10.1016/j.tifs.2018.03.008
Mošovská, S., Medvecká, V., Gregov, M., Tomeková, J., Valík, L., Mikulajová, A., et al. (2019). Plasma inactivation of Aspergillus flavus on hazelnut surface in a diffuse barrier discharge using different working gases. Food Control 104, 256–261. doi: 10.1016/j.foodcont.2019.05.003
Naeem, I., Ismail, A., Riaz, M., Aziz, M., Akram, K., Shahzad, M. A., et al. (2024). Aflatoxins in the rice production chain: a review on prevalence, detection, and decontamination strategies. Food Res. Int. 188:114441. doi: 10.1016/j.foodres.2024.114441
Nahle, S., El Khoury, A., Savvaidis, I., Chokr, A., Louka, N., and Atoui, A. (2022). Detoxification approaches of mycotoxins: by microorganisms, biofilms and enzymes. Int. J. Food Contamin. 9:3. doi: 10.1186/s40550-022-00089-2
Naidis, G. V. (2014). Production of active species in cold helium–air plasma jets. Plasma Sourc. Sci. Technol. 23:065014. doi: 10.1088/0963-0252/23/6/065014
Neme, K., and Mohammed, A. (2017). Mycotoxin occurrence in grains and the role of postharvest management as a mitigation strategy. A review. Food Control 78, 412–425. doi: 10.1016/j.foodcont.2017.03.012
Nesic, K., Habschied, K., and Mastanjevic, K. (2021). Possibilities for the biological control of mycotoxins in food and feed. Toxins 13:198. doi: 10.3390/toxins13030198
Neuenfeldt, N. H., Silva, L. P., Pessoa, R. S., and Rocha, L. O. (2023). Cold plasma technology for controlling toxigenic fungi and mycotoxins in food. Curr. Opin. Food Sci. 52:101045. doi: 10.1016/j.cofs.2023.101045
Nguyen, T., Palmer, J., Phan, N., Shi, H., Keener, K., and Flint, S. (2022). Control of aflatoxin M1 in skim milk by high voltage atmospheric cold plasma. Food Chem. 386:132814. doi: 10.1016/j.foodchem.2022.132814
Niemira, B. A. (2012). Cold plasma for sustainable food production and processing. Annu. Rev. Food Sci. Technol. 3, 125–142. doi: 10.1146/annurev-food-022811-101132
Nikmaram, N., and Keener, K. M. (2022). Degradation of aflatoxin M1 in skim and whole milk using high voltage atmospheric cold plasma (HVACP) and quality assessment. Food Res. Int. 162:112009. doi: 10.1016/j.foodres.2022.112009
Nikmaram, N., and Keener, K. M. (2023). Aflatoxin M1 degradation using high voltage atmospheric cold plasma (HVACP) technology. Crit. Rev. Food Sci. Nutr. 63, 503–515. doi: 10.1080/01919512.2023.2173556
Nikmaram, N., Brückner, L., Cramer, B., Humpf, H.-U., and Keener, K. M. (2023). Degradation products of aflatoxin M1 (AFM1) formed by high voltage atmospheric cold plasma (HVACP) treatment. Toxicon 230:107160. doi: 10.1016/j.toxicon.2023.107160
Ojha, S., Fröhling, A., Durek, J., Ehlbeck, J., Tiwari, B. K., Schlüter, O. K., et al. (2021). Principles and application of cold plasma in food processing. Innov. Food Process. Technol. 1, 519–540. doi: 10.1016/B978-0-08-100596-5.23033-3
Ostry, V., Malir, F., Toman, J., and Grosse, Y. (2017). Mycotoxins as human carcinogens—the IARC Monographs classification. Mycotoxin Res. 33, 65–73. doi: 10.1007/s12550-016-0265-7
Ott, L. C., Appleton, H. J., Shi, H., Keener, K., and Mellata, M. (2021). High voltage atmospheric cold plasma treatment inactivates Aspergillus flavus spores and deoxynivalenol toxin. Food Microbiol. 95:103669. doi: 10.1016/j.fm.2020.103669
Ouf, S. A., Basher, A. H., and Mohamed, A.-A. H. (2014). Inhibitory effect of double atmospheric pressure argon cold plasma on spores and mycotoxin production of Aspergillus niger contaminating date palm fruits. J. Sci. Food Agric. 95, 3204–3210. doi: 10.1002/jsfa.7060
Pankaj, S. K., and Keener, K. M. (2017). Cold plasma: background, applications and current trends. Curr. Opin. Food Sci. 16, 49–52. doi: 10.1016/j.cofs.2017.07.008
Park, J., Henins, I., Herrmann, H. W., Selwyn, G. S., Jeong, J. Y., Hicks, R. F., et al. (2000). An atmospheric pressure plasma source. Appl. Phys. Lett. 76, 288–290. doi: 10.1063/1.125724
Patriarca, A., and Pinto, V. P. (2017). Prevalence of mycotoxins in foods and decontamination. Curr. Opin. Food Sci. 14, 50–60. doi: 10.1016/j.cofs.2017.01.011
Perinban, S., Orsat, V., Lyew, D., and Raghavan, V. (2022). Effect of plasma activated water on Escherichia coli disinfection and quality of kale and spinach. Food Chem. 397:133793. doi: 10.1016/j.foodchem.2022.133793
Pinela, J., and Ferreira, I. C. F. R. (2017). Nonthermal physical technologies to decontaminate and extend the shelf-life of fruits and vegetables: trends aiming at quality and safety. Crit. Rev. Food Sci. Nutr. 57, 2095–2111. doi: 10.1080/10408398.2015.1046547
Polito, J., and Kushner, M. J. (2024). A hierarchal model for bacterial cell inactivation in solution by direct and indirect treatment using cold atmospheric plasmas. J. Phys. D Appl. Phys. 57:405207. doi: 10.1088/1361-6463/ad5f3b
Puligundla, P., Lee, T., and Mok, C. (2020). Effect of corona discharge plasma jet treatment on the degradation of aflatoxin B1 on glass slides and in spiked food commodities. LWT 124:108333. doi: 10.1016/j.lwt.2019.108333
Rahnavard, M. A., Zare, D., Nassiri, S. M., Taghvaei, H., and Fazaeli, M. (2024). Impact of gliding arc cold plasma on deactivating aflatoxin and post-treatment fungal growth on wheat grains. Food Control 164:110597. doi: 10.1016/j.foodcont.2024.110597
Richard, J. L. (2007). Some major mycotoxins and their mycotoxicoses—An overview. Int. J. Food Microbiol. 119, 3–10. doi: 10.1016/j.ijfoodmicro.2007.07.019
Sakiyama, Y., Graves, D. B., Chang, H.-W., Shimizu, T., and Morfill, G. E. (2012). Plasma chemistry model of surface microdischarge in humid air and dynamics of reactive neutral species. J. Phys. D Appl. Phys. 45:425201. doi: 10.1088/0022-3727/45/42/425201
Sakudo, A., and Yagyu, Y. (2024). Degradation of toxins derived from foodborne pathogens by atmospheric-pressure dielectric-barrier discharge. Int. J. Mol. Sci. 25:5986. doi: 10.3390/ijms25115986
Schoevers, E. J., Santos, R. R., Colenbrander, B., Fink-Gremmels, J., and Roelen, B. A. (2012). Transgenerational toxicity of Zearalenone in pigs. Reprod. Toxicol. 34, 110–119. doi: 10.1016/j.reprotox.2012.03.004
Schrenk, D., Bignami, M., Bodin, L., Chipman, J. K., del Mazo, J., Grasl-Kraupp, B., et al. (2022). Assessment of information as regards the toxicity of fumonisins for pigs, poultry and horses. EFSA J. 20:e07534. doi: 10.2903/j.efsa.2022.7534
Schrenk, D., Bignami, M., Bodin, L., Chipman, J. K., Del Mazo, J., Grasl-Kraupp, B., et al. (2020). Risk assessment of aflatoxins in food. EFSA J. 18:e06040. doi: 10.2903/j.efsa.2020.6040
Segat, A., Misra, N. N., Cullen, P. J., and Innocente, N. (2015). Atmospheric pressure cold plasma (ACP) treatment of whey protein isolate model solution. Innovative Food Science & Emerging Technologies. 29, 247–254. doi: 10.1016/j.ifset.2015.03.014
Shamseer, L., Moher, D., Clarke, M., Ghersi, D., Liberati, A., Petticrew, M., et al. (2015). Preferred reporting items for systematic review and meta-analysis protocols (PRISMA-P) 2015: elaboration and explanation. BMJ 349:g7647. doi: 10.1136/bmj.g7647
Shi, H., Cooper, B., Stroshine, R. L., Ileleji, K. E., and Keener, K. M. (2017b). Structures of degradation products andq degradation pathways of aflatoxin B1 by high-voltage atmospheric cold plasma (HVACP) treatment. J. Agric. Food Chem. 65, 6222–6230. doi: 10.1021/acs.jafc.7b01604
Shi, H., Ileleji, K., Stroshine, R. L., Keener, K., and Jensen, J. L. (2017a). Reduction of aflatoxin in corn by high voltage atmospheric cold plasma. Food Bioproc. Technol. 10, 1042–1052. doi: 10.1007/s11947-017-1873-8
Shimizu, T., Sakiyama, Y., Graves, D. B., Zimmermann, J. L., and Morfill, G. E. (2012). The dynamics of ozone generation and mode transition in air surface micro-discharge plasma at atmospheric pressure. N. J. Phys. 14:103028. doi: 10.1088/1367-2630/14/10/103028
Shirazi, S., Ramezan, Y., Moslehishad, M., Ghomi Marzdashti, H., and Mirsaeedghazi, H. (2025). Effects of cold atmospheric plasma on patulin degradation, polyphenol oxidase inactivation, and other physicochemical properties of fresh-cut apple slices during storage. Food Chem. 465:142017. doi: 10.1016/j.foodchem.2024.142017
Siciliano, I., Spadaro, D., Prelle, A., Vallauri, D., Cavallero, M. C., Garibaldi, A., et al. (2016). Use of cold atmospheric plasma to detoxify hazelnuts from aflatoxins. Toxins 8:125. doi: 10.3390/toxins8050125
Sun, S., Anderson, N. M., and Keller, S. (2014). Atmospheric pressure plasma treatment of black peppercorns inoculated with Salmonella and held under controlled storage. J. Food Sci. 79, E2441–E2446. doi: 10.1111/1750-3841.12696
Ten-Bosch, L., Pfohl, K., Avramidis, G., Wieneke, S., Viöl, W., and Karlovsky, P. (2017). Plasma-based degradation of mycotoxins produced by Fusarium, Aspergillus, and Alternaria species. Toxins 9:97. doi: 10.3390/toxins9030097
Wan, Z., Pankaj, S. K., Mosher, C., and Keener, K. M. (2019). Effect of high voltage atmospheric cold plasma on inactivation of Listeria innocua on Queso Fresco cheese, cheese model and tryptic soy agar. LWT 102, 268–275. doi: 10.1016/j.lwt.2018.11.096
Wang, L., Liu, X., Cai, R., Ge, Q., Zhao, Z., Yue, T., et al. (2022). Detoxification of ochratoxin A by pulsed light in grape juice and evaluation of its degradation products and safety. Innov. Food Sci. Emerg. Technol. 78:103024. doi: 10.1016/j.ifset.2022.103024
Wang, X., Wang, S., Yan, Y., Wang, W., Zhang, L., and Zong, W. (2020). The degradation of Alternaria mycotoxins by dielectric barrier discharge cold plasma. Food Control 117:107333. doi: 10.1016/j.foodcont.2020.107333
Wang, Y., Li, B., Shang, H., Ma, R., Zhu, Y., Yang, X., et al. (2022). Effective inhibition of fungal growth, deoxynivalenol biosynthesis and pathogenicity in cereal pathogen Fusarium spp. by cold atmospheric plasma. Chem. Eng. J. 437:135307. doi: 10.1016/j.cej.2022.135307
Wang, Y., Zheng, Y., Wang, J., Mu, L., Zhang, W., Huang, M., et al. (2024). Effect of plasma on ochratoxin degradation and raisin properties. J. Food Eng. 375:112051. doi: 10.1016/j.jfoodeng.2024.112051
Wen, G., Liang, Z., Xu, X., Cao, R., Wan, Q., and Ji, G. (2020). Inactivation of fungal spores in water using ozone: kinetics, influencing factors and mechanisms. Water Res. 185:116218. doi: 10.1016/j.watres.2020.116218
Wen, G., Xu, X., Zhu, H., Huang, T., and Ma, J. (2017). Inactivation of four genera of dominant fungal spores in groundwater using UV and UV/PMS: efficiency and mechanisms. Chem. Eng. J. 328, 619–628. doi: 10.1016/j.cej.2017.07.05
Wen, G., Zhao, D., Xu, X., Chen, Z., Huang, T., and Ma, J. (2019). Inactivation of fungi from four typical genera in groundwater using PMS/Cl? system: efficacy, kinetics and mechanisms. Chem. Eng. J. 357, 567–578. doi: 10.1016/j.cej.2018.09.195
Wielogorska, E., Ahmed, Y., Meneely, J., Graham, W. G., Elliott, C. T., and Gilmore, B. F. (2019). A holistic study to understand the detoxification of mycotoxins in maize and impact on its molecular integrity using cold atmospheric plasma treatment. Food Chem. 301:125281. doi: 10.1016/j.foodchem.2019.125281
Wu, Y., Cheng, J.-H., and Sun, D.-W. (2021). Blocking and degradation of aflatoxins by cold plasma treatments: applications and mechanisms. Trends Food Sci. Technol. 109, 647–661. doi: 10.1016/j.tifs.2021.01.053
Xue, M., Wang, T., Sun, Q., Qu, G., Jia, H., and Zhu, L. (2021). Insights into the highly efficient detoxification of the biotoxin patulin in water by discharge plasma oxidation. Chem. Eng. J. 411:128432. doi: 10.1016/j.cej.2021.128432
Yarabbi, H., Soltani, K., Sangatash, M. M., Yavarmanesh, M., and Zenoozian, M. S. (2023). Reduction of microbial population of fresh vegetables (carrot, white radish) and dried fruits (dried fig, dried peach) using atmospheric cold plasma and its effect on physicochemical properties. J. Agric. Food Res. 14:100789. doi: 10.1016/j.jafr.2023.100789
Yinxin, L., Can, Z., Menglu, H., Cui, S., Jinping, C., Jingyu, W., et al. (2022). Effect of cold atmospheric plasma on the gray mold rot of postharvest mulberry fruit. Food Control 137:108906. doi: 10.1016/j.foodcont.2022.108906
Zhang, K., Perussello, C. A., Milosavljeviæ, V., Cullen, P. J., Sun, D.-W., and Tiwari, B. K. (2019). Diagnostics of plasma reactive species and induced chemistry of plasma treated foods. Crit. Rev. Food Sci. Nutr. 59, 812–825. doi: 10.1080/10408398.2018.1564731
Zhao, L., Sheng, X., Li, S., Yan, W., Qian, J., Wang, J., et al. (2024). Assessment of non-thermal plasma for decontamination against Aspergillus flavus and aflatoxin B1 in maize. Food Control 163:110521. doi: 10.1016/j.foodcont.2024.110521
Zhao, L., Wang, J., Sheng, X., Li, S., Yan, W., Qian, J., et al. (2023). Non-thermal plasma inhibited the growth and aflatoxins production of Aspergillus flavus, degraded aflatoxin B1 and its potential mechanisms. Chem. Eng. J. 475:146017. doi: 10.1016/j.cej.2023.146017
Zhao, X., Wang, N., Lu, Y., Li, Y., Zhao, T., Xu, J., et al. (2024). Effects of cold plasma on the growth and aflatoxin production of Aspergillus flavus. Food Biosci. 61:104552. doi: 10.1016/j.fbio.2024.104552
Keywords: CAP technology, mycotoxins, contamination, foodstuffs, detoxificationprotect
Citation: Oliveira ACD, Ali S, Corassin CH, Ullah S, Pereira KN, Walsh JL, Hojnik N and Oliveira CAF (2025) Application of cold atmospheric plasma for decontamination of toxigenic fungi and mycotoxins: a systematic review. Front. Microbiol. 15:1502915. doi: 10.3389/fmicb.2024.1502915
Received: 27 September 2024; Accepted: 09 December 2024;
Published: 03 January 2025.
Edited by:
Gustavo Cordero-Bueso, University of Cádiz, SpainReviewed by:
Abhay K. Pandey, North Bengal Regional R&D Center, IndiaShengqian Sun, Yantai Institute of Technology, China
Mengyue Guo, Chinese Academy of Medical Sciences and Peking Union Medical College, China
Copyright © 2025 Oliveira, Ali, Corassin, Ullah, Pereira, Walsh, Hojnik and Oliveira. This is an open-access article distributed under the terms of the Creative Commons Attribution License (CC BY). The use, distribution or reproduction in other forums is permitted, provided the original author(s) and the copyright owner(s) are credited and that the original publication in this journal is cited, in accordance with accepted academic practice. No use, distribution or reproduction is permitted which does not comply with these terms.
*Correspondence: Carlos Augusto Fernandes de Oliveira, Y2FybG9zYWZAdXNwLmJy
†ORCID: Amanda Cristina Dias de Oliveira, orcid.org/0000-0001-6991-6218; Sher Ali, orcid.org/0000-0002-4604-9702; Carlos Humberto Corassin, orcid.org/0000-0001-9826-9224; Sana Ullah, orcid.org/0009-0002-7086-4894; Karina Nascimento Pereira, orcid.org/0000-0002-9609-7964; Carlos Augusto Fernandes de Oliveira, orcid.org/0000-0001-5779-5287