- 1Graduate School of China Academy of Chinese Medical Sciences, Beijing, China
- 2Graduate School of Hebei University of Chinese Medicine, Shijiazhuang, China
As the global male infertility rate continues to rise, there is an urgent imperative to investigate the underlying causes of sustained deterioration in sperm quality. The gut microbiota emerges as a pivotal factor in host health regulation, with mounting evidence highlighting its dual influence on semen. This review underscores the interplay between the Testis-Gut microbiota axis and its consequential effects on sperm. Potential mechanisms driving the dual impact of gut microbiota on sperm encompass immune modulation, inflammatory responses mediated by endotoxins, oxidative stress, antioxidant defenses, gut microbiota-derived metabolites, epigenetic modifications, regulatory sex hormone signaling. Interventions such as probiotics, prebiotics, synbiotics, fecal microbiota transplantation, and Traditional natural herbal extracts are hypothesized to rectify dysbiosis, offering avenues to modulate gut microbiota and enhance Spermatogenesis and motility. Future investigations should delve into elucidating the mechanisms and foundational principles governing the interaction between gut microbiota and sperm within the Testis-Gut microbiota Axis. Understanding and modulating the Testis-Gut microbiota Axis may yield novel therapeutic strategies to enhance male fertility and combat the global decline in sperm quality.
1 Introduction
Infertility is becoming a widespread global issue, and the World Health Organization estimates that 12.6–17.5% of couples globally encounter fertility challenges, with male factors contributing to 30–50% of cases of reduced fertility (Cox et al., 2022; Fainberg and Kashanian, 2019; Eisenberg et al., 2023), causing social, psychological, and marital problems for couples. Male reproductive impairment can arise from factors impacting sperm production, quality, function, or transport (Tournaye et al., 2017). Given that male fertility hinges on both sperm quantity and quality, semen quality serves as a crucial indicator of male reproductive health and is closely linked to fertility. A retrospective analysis of semen samples collected globally throughout the 20th and 21st centuries has revealed a significant decline in male sperm concentration and total sperm count, a trend that is accelerating in the 21st century (Levine et al., 2023). With the acceleration of global male infertility, there is an urgent need to investigate the potential causes and mechanisms of this continuous decline, and take preventive measures to protect male reproductive health from further deterioration.
The gut microbiota (GM) represents a significant component of the gastrointestinal tract, often termed the “second human genome” due to its vast repertoire of over 3 million genes, compared to the approximately 23,000 genes found in the human genome. It is recognized as the host’s endocrine organ. This extensive bacterial community plays a critical role in preserving the equilibrium between the host’s internal and external environments, thereby serving as a pivotal determinant of host health (De Vos et al., 2022; Lloyd-Price et al., 2016). The GM constitutes a complex and dynamically changing microbial community. Across the life cycle of mammals, evolution has occurred in conjunction with this microbiota (Argaw-Denboba et al., 2024), and mounting research underscores the pivotal role of GM in human physiological functions and disease progression. The indigenous GM fulfills distinct functions in host nutrient metabolism, xenobiotic and drug metabolism, preservation of the structural integrity of the gut mucosal barrier, immunomodulation, and defense against pathogens (Jandhyala et al., 2015). Research indicates that nearly all regions of the human body harbor microorganisms, and various organs can communicate through the GM (Gilbert et al., 2018; Schmidt et al., 2018). Furthermore, different individuals can also share connections via their GM. A recent study (Argaw-Denboba et al., 2024) highlights the pivotal role of the GM in mediating intergenerational health outcomes across paternal lineages in mice. Disruption in the ecological balance of the paternal GM has been linked to alterations in the male reproductive system, including compromised leptin signaling, changes in testicular metabolite profiles, and the redistribution of small RNA payloads in sperm. These changes increase the risk of developmental disorders and premature mortality in offspring, directly impacting their overall health.
Recent studies have demonstrated the significant influence of GM on sperm. Increasing research indicates an interplay between GM and the male reproductive system, highlighting its pivotal role in reproductive health (Hao et al., 2022; Yan et al., 2022; Jin et al., 2024; Li et al., 2022c; Li et al., 2024). Numerous studies have examined how GM impacts semen from dual perspectives (Ding et al., 2020; Lundy et al., 2021). On one hand, GM like Lactic acid bacteria, Bacteroidetes, and Ruminococcus (UCG011) can enhance sperm production, motility, and semen quality. (Fu et al., 2023). On the other hand, imbalanced GM can disrupt sperm production and reduce motility. The negative correlation between sperm motility and some “bad bacteria” or GM dysbiosis has been identified, for example Bacteroidetes Prevotella, Enterococcus faecalis, and GM dysbiosis caused by high-fat diet or otheres. The potential mechanism of GM influencing on sperm includes GM metabolites or bacterial cells regulating host intestinal homeostasis, host metabolism, that finally affects host reproductive function (Fu et al., 2023; Lv et al., 2024).
The influence of GM on sperm is evident, however, the specific influencing mechanisms require further elucidation. Accordingly, our team maintains a focus on investigating the gut-testis axis (Zou et al., 2024). By thoroughly reviewing existing literature, we aim to uncover potential mechanisms through which GM affects semen. This exploration intents to stimulate researchers’ interest on investigating the connections and reciprocal influences between GM and reproductive disorders, including sperm health, testicular function, and sex hormone regulation.
2 Potential mechanisms of GM affecting sperm
The GM comprises numerous species and interacts with multiple systems in the body, exhibiting complex potential mechanisms of action (Chen et al., 2021). This section focuses on elucidating the dual effects of GM on semen, exploring its role in mediating immune and inflammatory responses via endotoxins, oxidative stress, antioxidant protection, microbiota-derived metabolites, epigenetic modifications, regulation of sex hormones, and modulation of the blood-testis barrier.
2.1 GM mediates immune and inflammatory responses via endotoxins
Endotoxin is a potential pathway through which the intestinal microbiota mediates immune and inflammatory responses that affect sperm generation and reproductive function (Noguchi et al., 2017; Tremellen et al., 2018; Khanmohammad et al., 2021). Endotoxin is a component of the intestinal microbiota, particularly gram-negative bacteria, which use lipopolysaccharides (LPS) as cytoderm, that is effective activator of inflammation. Upon activation of the immune system, inflammatory mediators such as cytokines (e.g., tumor necrosis factor, interleukin-6) and chemokines (Chen et al., 2017; Silva et al., 2018) are typically released, triggering inflammatory responses that can affect sperm generation and function (Rizzetto et al., 2018; Maynard et al., 2012; Brown et al., 2019; Wei et al., 2024).
Dysbiosis of the GM can lead to the release of endotoxins into the intestine due to damage of gram-negative bacteria (Zhao et al., 2019; Schoeler and Caesar, 2019), which will compromise the intestinal barrier. This allows endotoxins to enter the circulation and activate immune responses, thereby mediating inflammatory reactions (Mohr et al., 2022; Di Lorenzo et al., 2019; Candelli et al., 2021). These reactions include releasing key pro-inflammatory cytokines, activating genes involved in inflammation and immune responses, that decrease sperm motility (O'Doherty et al., 2016; Parker and Palladino, 2017). For example, LPS from Escherichia coli can stimulate immune responses in healthy male mice, leading to the production of pro-inflammatory cytokines such as IL-17A, mediating immune and inflammatory responses in testicular tissue. This results in widespread necrosis of testicular parenchyma, damage to the epithelial cells of seminiferous tubules, reduction in testosterone levels within the testes, ultimately impairing testicular tissue, decreasing sperm production, reducing motility, and enhancing DNA fragmentation (Khanmohammad et al., 2021; Folliero et al., 2022; Li et al., 2024). Additionally, LPS-induced epididymitis in rats exhibits leukocyte infiltration and fibrosis in the caudal epididymis, downregulating the expression of rat-specific β-defensin SPAG11E, disrupting SPAG11E binding with sperm, damaging blood-epididymal barrier permeability, and sperm viability (Cao et al., 2010; Wang et al., 2019). Research by Brecchia G and others (Brecchia et al., 2010; Collodel et al., 2012) demonstrates that LPS-mediated subacute inflammation can disrupt rabbit testicular structure and sperm membrane integrity. After 30 days of LPS exposure, rabbit sperm membrane integrity and the number of necrotic sperm are severely affected, peaking at the end of the 56-day spermatogenic cycle. Supplementation with testicular vitamin K may help inhibit inflammatory signal transduction and improve LPS-induced reduction in testicular testosterone synthesis, maintaining stable testosterone levels (Takumi et al., 2011).
2.2 Oxidative stress and antioxidant protection
Sperm are susceptible to oxidative stress (OS), which refers to the imbalance between the generation of reactive oxygen species (ROS) and the cellular antioxidant defense systems (Barati et al., 2020). Spermiogenesis involves an oxidative process that requires controlled levels of ROS to trigger phosphorylation. Thus, at physiological concentrations, ROS are essential for normal sperm function, playing critical roles in sperm maturation, capacitation, hyperactivation, and acrosome reaction processes. However, excessive ROS can lead to OS, causing structural and functional damage to sperm cells, manifested as impaired energy metabolism, protein oxidation, lipid peroxidation, and DNA damage, ultimately resulting in reduced sperm motility and viability (Du Plessis et al., 2015; Aitken, 2017).
The GM can influence the host’s antioxidant defense system, thereby affecting sperm production and motility (Uchiyama et al., 2022; Magill and MacDonald, 2023). Antioxidant enzymes such as superoxide dismutase (SOD), glutathione peroxidase (GPX), peroxiredoxin (PRDX), thioredoxin, and glutathione-S-transferase exhibit antioxidant activity, neutralizing free radicals and other oxidative stressors to reduce oxidative damage. Certain probiotics or specific bacterial strains can produce antioxidants like glutathione and superoxide dismutase, which are essential for generating healthy sperm, maintaining sperm quality to ensure vitality, energy acquisition, and DNA integrity, thereby protecting sperm from oxidative harm (O'Flaherty and Scarlata, 2022; Oliveira et al., 2024; Wang et al., 2017). Studies indicate that PRDX regulates ROS levels, preventing oxidative stress during human sperm maturation processes (Lee et al., 2017) Further research by Fernandez MC (Fernandez and O'Flaherty, 2018; Fernandez et al., 2019) and others has highlighted peroxiredoxin 6 as a key antioxidant enzyme maintaining human sperm vitality and DNA integrity. Peroxiredoxin 6 regulates the phosphoinositide 3-kinase (PI3K) /protein kinase B (AKT) pathway to eliminate excessive ROS and maintain sperm vitality, thereby preventing oxidative damage.
Supplementation with antioxidants such as vitamins E and C, selenium, glutathione, coenzyme Q10, carotenoids, and l-carnitine can modulate GM, reducing sperm damage induced by oxidative stress (Beygi et al., 2021; Li et al., 2023). For instance, selenium (Se), a renowned antioxidant, significantly influences gut microbial composition, male sperm quality, and fertility. Research indicates associations between selenium binding protein (SeAlb), Escherichia/Shigella species, and glutathione peroxidase (GPx) (Rayman, 2012; Ramírez-Acosta et al., 2022). Studies by Sun et al. (2023) and Zeng et al. (2024), and others have shown that selenium gluconate (SeGlu) derivatives, novel organic selenium compounds, reduce the abundance of detrimental bacteria such as Rikenella, Barnesiella, Tenacibaculum, Acinetobacter, Bacteroides, and Alistipes, while increasing beneficial microbes like Intestinimonas, Christensenella, Coprococcus, Butyrivibrio, Clostridium, Ruminococcus, Lactobacillus, and Lactococcus. This supplementation enhances rat sperm quality by reducing harmful bacterial colonization, modulating GM, and decreasing sperm damage induced by oxidative stress.
Furthermore, dysbiosis of GM increases oxidative stress within the host, making cells more susceptible to oxidative damage, triggering immune responses, inflammation, and other pathological changes (Ferro et al., 2020), impairing sperm production and function. Dysbiosis-induced LPS induce oxidative stress-mediated mitochondrial damage in sperm, leading to significant mitochondrial ultrastructural changes and increased mitochondrial reactive oxygen species. This abnormal activation of oxidative phosphorylation (OXPHOS) and mitochondrial membrane lipid peroxidation result in sperm oxidative damage, reducing boar sperm motility and vitality (He et al., 2017). Research has shown that glyphosate (GLY) -induced dysbiosis of GM increases local interleukin (IL) -17A production (Liu et al., 2021), subsequently activating testicular oxidative damage, manifesting as impaired testicular structure, decreased sperm vitality, and increased sperm deformity rates.
2.3 Metabolites of GM
The influence of GM metabolites on host health extends to semen quality. GM produce a diverse array of metabolites with varied biological activities. These metabolites can be categorized into three main types based on their origins (Figure 1): Metabolites directly synthesized by GM from dietary sources, including short-chain fatty acids (SCFAs), polyunsaturated fatty acids (PUFAs), and amino acid derivatives; Metabolites initially produced by the host and subsequently modified by GM, such as secondary bile acids and hydroxysteroid dehydrogenase (HSDH); Metabolites synthesized de novo, such as LPS and vitamin K (Liu et al., 2022a; Lv et al., 2024). Alterations in GM composition can impact the levels of these metabolites, consequently influencing sperm production and quality (Wang et al., 2023a). For instance, decreased levels of RuminococcaceeNK4A214_group in the gut correlate with reduced bile acid levels, impairing spermatogenesis and decreasing spermatogenic cell counts (Zhang et al., 2021). Moreover, the GM-derived metabolite 3-hydroxyphenylacetic acid (3-HPAA) has been shown to inhibit ferroptosis-mediated mechanisms and promote spermatogenesis in aging mice (Jin et al., 2023). Supplementation with dietary fiber enhances GM composition in boars, stimulating the production of SCFAs and thereby improving sperm production and semen quality (Lin et al., 2022). This review focuses on the impact of key GM metabolites such as SCFAs, secondary bile acids, tryptophan and indole derivatives, and vitamins on sperm health.
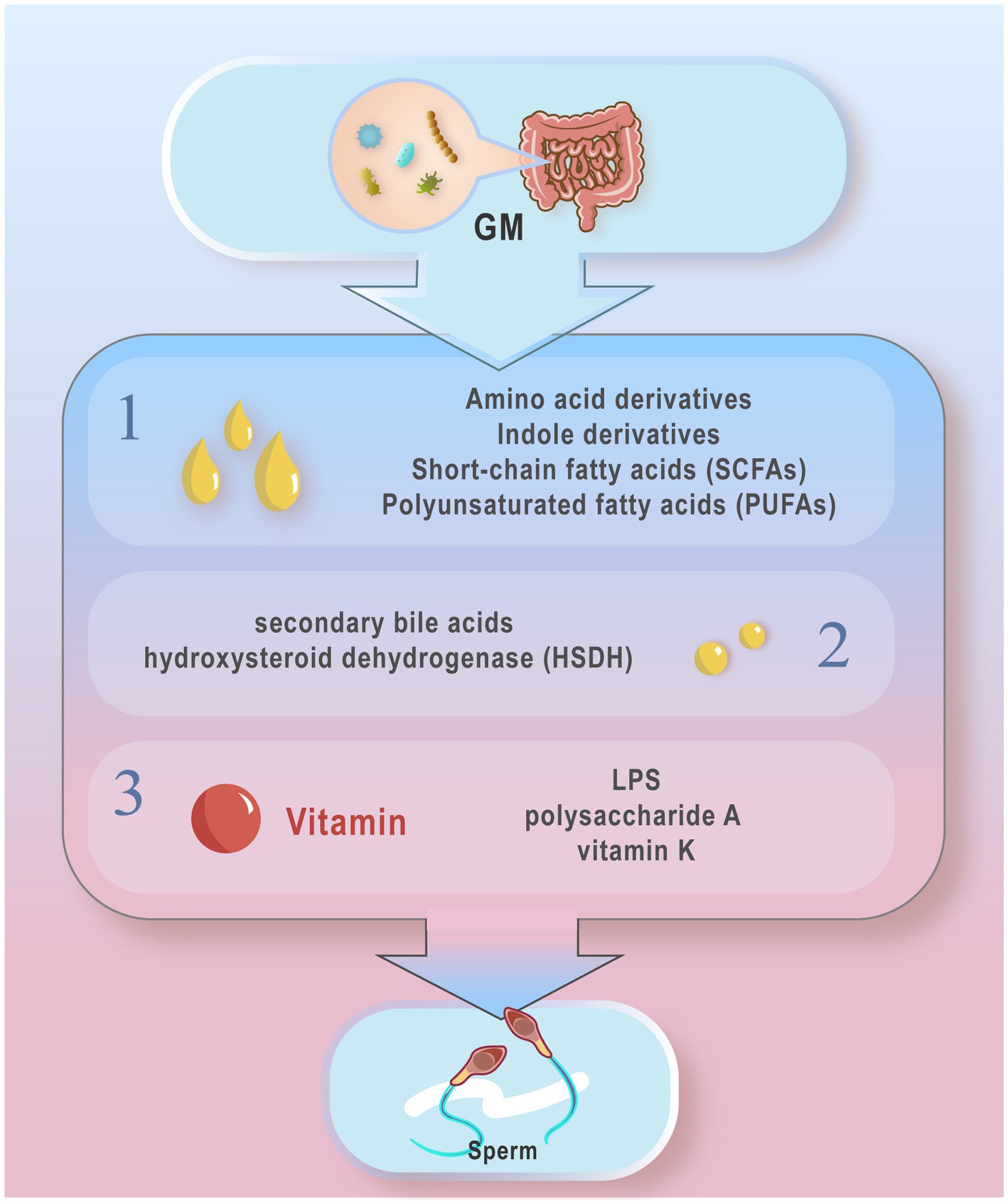
Figure 1. Typical gut microbiota metabolites from different sources. According to different sources, these metabolites can be divided into three main types: 1. Metabolites directly produced by the gut microbiota from the diet: short chain fatty acids (SCFAs), polyunsaturated fatty acids (PUFAs) amino acid derivatives, and indole derivatives; 2. Metabolites produced by the host and modified by the gut micro- biota, secondary bile acids and hydroxysteroid dehydrogenases (HSDH); 3 Metabolites synthesized from novel LPS, polysaccharide A, and vitamin K, etc. The metabolites of GM may influence the host’s Spermatogenesis and motility.
SCFAs are metabolites produced by GM, particularly probiotics and Bacillus subtilis, through the fermentation of cellulose and unabsorbed carbohydrates. They play a crucial role in regulating gut homeostasis and influencing health and disease outcomes (van der Hee and Wells, 2021; Fusco et al., 2023). SCFAs are involved in regulating sperm production and motility; for instance, dietary supplementation with sodium butyrate (SB) in roosters has been shown to enhance semen volume, sperm motility, sperm concentration, and reduce abnormal sperm percentages. Additionally, it enhances the enzyme activity of GPx and SOD in adult roosters at 45 weeks, promoting testosterone secretion and testicular growth (Alhaj et al., 2018). SCFAs can improve intestinal microbiota altered by a high-fat diet (HFD), regulate lipid metabolism to enhance spermatogenesis, and improve semen volume and fertility by producing n-3 polyunsaturated fatty acids (Hao et al., 2022). They also increase beneficial enterobacteria, reduce harmful bacteria, elevate levels of acetic acid and butyric acid in feces, and enhance blood levels of testosterone, DHA, EPA, promoting spermatogenesis, and improving sperm concentration and vitality in type 2 diabetes (Yan et al., 2022; Zhou et al., 2023).
Secondary bile acids are a type of bile acid formed after metabolism by GM. They significantly influence host metabolism and immune response by modulating bile acid pool circulation and overall fat metabolism (Fogelson et al., 2023). Altering the GM structure affects bile acid metabolism, which in turn influences host metabolism and immune response (Tian et al., 2020; Lee et al., 2024). Bile acids may impact sperm production and quality through their regulatory effects on host metabolism and immune response. Research indicates that heat stress-induced dysbiosis of GM impairs spermatogenesis by altering secondary bile acid metabolism in the gut (He et al., 2024). Moreover, Aspergillus fumigatus regulates secondary bile acid metabolism by promoting colonization of bile salt hydrolase (BSH) metabolizing bacteria, thereby enhancing retinol absorption in the host gut and improving testicular retinoid levels, which further improves spermatogenesis. Zhang et al. (2022) found that reduced levels of RuminococcaceeNK4A214_group lead to decreased bile acid levels, causing abnormal vitamin A metabolism in the intestine and resulting in abnormal sperm.
Tryptophan is an amino acid metabolized into indole, a primary product of tryptophan metabolism. In the intestine, GM further metabolizes indole into various derivatives such as indole-3-propionic acid (IPA) and 3-hydroxyindole, which significantly influence host health, disease, and aging (Wang et al., 2024a; Gupta et al., 2023). IPA inhibits GM dysbiosis and intestinal endotoxin leakage (Zhao et al., 2019). Indole-derived metabolites upregulate CatSper protein expression, enhance testosterone secretion, and increase StAR protein expression to mitigate testicular injury induced by Cisplatin (II), inhibit OS and inflammation, and restore sex hormone levels (Afsar et al., 2022). The potential effects of tryptophan and its derivatives on sperm warrant further investigation.
2.4 Epigenetic modifications induced by GM and their impact on host physiology
The GM and epigenetic processes are dynamic and influenced by environmental factors and diet (Li et al., 2022c). Epigenetic modifications refer to chemical alterations of certain parts of the genome that do not involve changes in the DNA sequence itself. These modifications alter the structure or modification status of DNA and its associated proteins, thereby regulating gene expression levels and functions. They include DNA methylation, histone modifications, chromatin remodeling, and modifications mediated by non-coding RNAs (Skvortsova et al., 2018; Xavier et al., 2019).
Epigenetic regulation is considered an effective mechanism by which the GM influences host physiological functions (Wang et al., 2024b). GM metabolites can induce epigenetic modifications, such as changes in DNA methylation and micro-RNA expression. Studies have shown that gut microbes like lactobacilli and bifidobacteria can influence DNA methylation by affecting the bioavailability of folate they produce (Ashonibare et al., 2024). Kumar et al. (2014) demonstrated that GM dominated by Firmicutes or Bacteroidetes correlates with differences in the methylation status of gene promoters associated with cardiovascular disease.
The GM may influence sperm genetic quality and offspring health through effects on host gene expression and epigenetic modifications. These effects can manifest in various ways, including changes in DNA methylation patterns or regulation of histone modifications, thereby impacting genetic stability and phenotypic characteristics of sperm (Woo and Alenghat, 2022; Ashonibare et al., 2024). For instance Liu et al. (2022b) found that water extracts of black tea alter tissue gene expression through GM modulation, changing the levels of major epigenetic modifications (DNA methylation) and regulating imprinting genes’ DNA methylation in sperm of high-fat diet-fed mice. The impact of GM on sperm via epigenetic modifications is evident but requires further investigation for clarification.
2.5 GM’s role in regulating sex hormones and spermatogenesis
The GM influences the host’s endocrine system, including regulation of sex hormones by affecting the hypothalamic–pituitary-gonadal (HPG) axis (Wang and Xie, 2022). Sex hormones are crucial for spermatogenesis and sperm activity, and dysbiosis of the GM may lead to abnormal changes in hormone levels, affecting sperm quality and quantity. Within the HPG axis, the hypothalamus coordinates the pulsatile release of gonadotropin-releasing hormone (GnRH), activating the pituitary-gonadal axis. GnRH stimulates the pituitary gland to produce luteinizing hormone (LH) and follicle-stimulating hormone (FSH), which are vital for male reproductive processes. LH regulates Leydig cell function and testosterone secretion, while FSH promotes germ cell division and sperm production, supporting the energy metabolism of testicular germ cells (Kaprara and Huhtaniemi, 2018).
The GM can modulate hormone levels through various pathways (He et al., 2021). Studies by Ashonibare (Ashonibare et al., 2024) suggest that the GM can directly influence the synthesis of hormone-related enzymes and participate in the enterohepatic circulation of hormones, thereby affecting the hypothalamic–pituitary-testicular (HPT) axis. Research by Shin JH (Shin et al., 2019) indicates that men with higher testosterone levels have a more diverse gut microbial community compared to others, with abundances of Bacteroides, Dorea, Ruminococcus, and Clostridium significantly correlating with testosterone levels. Similarly, research by Yan et al. (2024) shows that within the male GM, species like Coprobacter, Ruminococcus2, Barnesiella, Actinomyces, and Bifidobacterium are negatively correlated with sex hormone-binding globulin (SHBG) levels, whereas α-Proteobacteria are positively correlated.
The GM may be a primary regulatory factor in testosterone production and metabolism. Deng C (Li et al., 2024) and others propose interactions between testosterone and the GM, suggesting testosterone may regulate spermatogenesis through the blood-testis barrier (BTB). Tang (Tang et al., 2024) further supports Deng’s findings, showing that viscumin affects the immune microenvironment of the testes, downregulating serum testosterone levels in male mice by inhibiting Akkermansia, disrupting guanosine metabolism. Supplementation of guanosine restores testosterone secretion by repairing the BTB and serum lipopolysaccharide levels. Clostridium scindens American Type Culture Collection 35,704 converts primary bile acids into toxic secondary bile acids and converts glucocorticoids into testosterone by side-chain cleavage (Ridlon et al., 2013). Adolescent Bifidobacterium strains with 20β-HSDH activity can alter glucocorticoid metabolism in the gut, potentially serving as probiotics for testosterone-dependent diseases (Doden et al., 2019). Poutahidis et al. (2014) and colleagues demonstrate that male mice fed purified Lactobacillus have larger testes and higher serum testosterone levels compared to controls. Moreover, feeding mice with Lactobacillus reuteri significantly increases testosterone levels after 5 months, with significant enhancement in seminiferous tubule cross-sectional profiles and interstitial cell proliferation in the testes.
Furthermore, the GM can regulate the permeability of the BTB, influencing hormone levels and thereby modulating sperm production and motility. The BTB is a critical ultrastructure in the testes supporting meiosis and post-meiotic spermatogenic cell development (Cheng and Mruk, 2012). Dysbiosis of the GM can increase inflammation, regulating oxidative stress-related enzyme activity, testosterone levels, and BTB permeability (Guo et al., 2024). Al-Asmakh et al. (2014) and others demonstrate that the microbiota regulates BTB permeability through modulation of intercellular adhesion, secreting high levels of butyrate, which restores BTB integrity in germ-free (GF) mice and normalizes levels of cell adhesion proteins, with intercellular adhesion molecules (ICAMs) being critical regulatory molecules for spermatogenesis (Xiao et al., 2013).
3 Intervention methods: correcting dysbiosis of GM
3.1 Prebiotics, probiotics, and synbiotics
Prebiotics refer to specific non-digestible food components beneficial to humans; Probiotics are live microorganisms in the gut; and synbiotics is composed of a mixture of prebiotics and probiotics. Prebiotics can stimulate the growth and activity of beneficial gut flora to improve host health. Probiotics confer health benefits to the host by colonizing the intestinal tract, rebalancing GM, and inhibiting the growth of harmful bacteria. Synbiotics offer a broader and more comprehensive probiotic effect through synergistic interactions of multiple strains (Ashonibare et al., 2024; Swanson et al., 2020; Gibson et al., 2017; Hill et al., 2014).Prebiotics, probiotics, and synbiotics have the potential to rectify dysbiosis of GM, influencing various host functions through colonization, pathogen eradication, and induction of host cell responses, thereby serving as microbial management tools to enhance host health (Sanders et al., 2019; Yadav et al., 2022) (Figure 2).
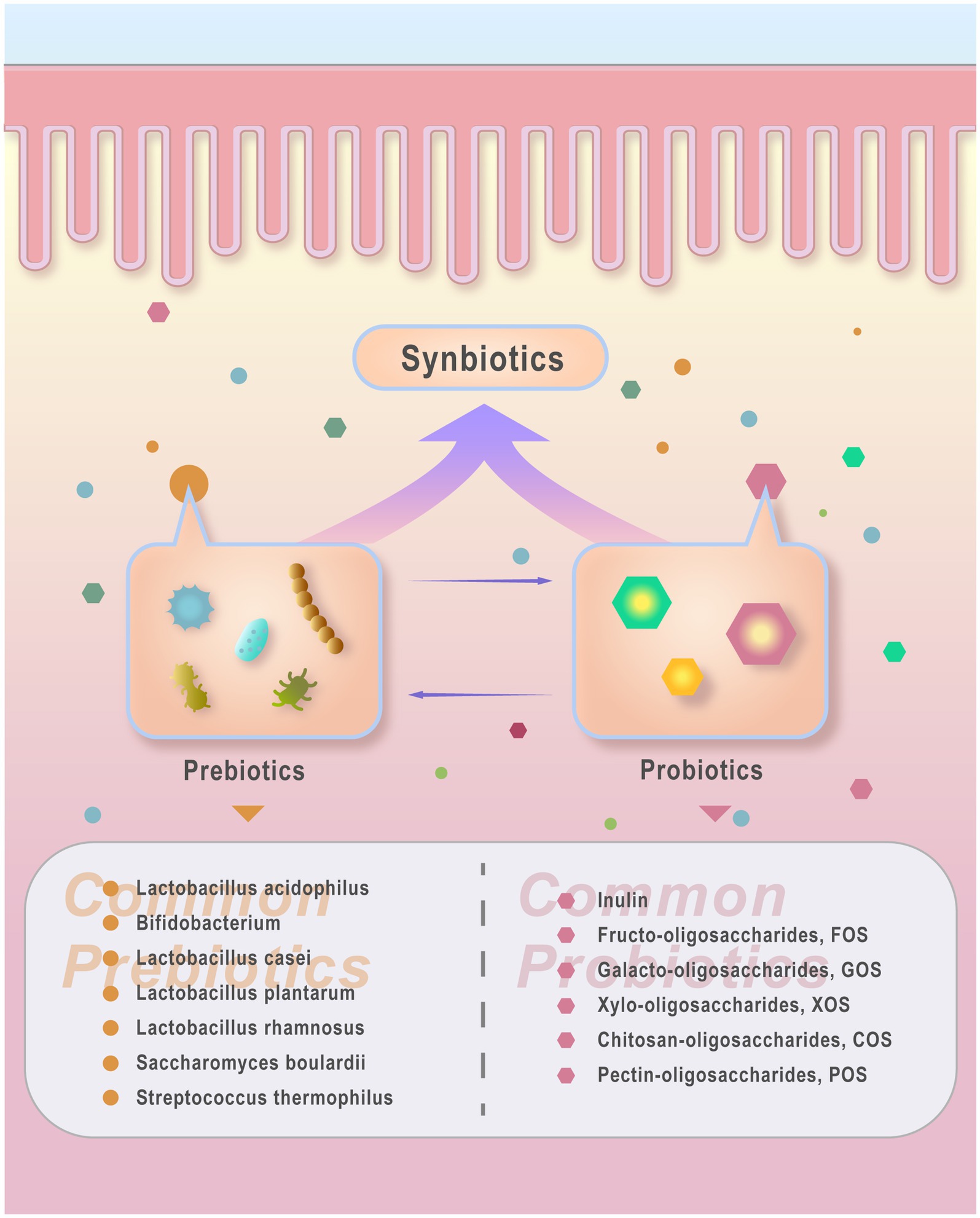
Figure 2. probiotics, prebiotics and synbiotics. Probiotics refer to beneficial live microorganisms that improve host health conditions. Common types include Lacto- bacillus acidophilus, Bifidobacterium, Lactobacillus casei, Lactobacillus plantarum, Lactobacillus rhamnosus, and yeast such as Saccharomyces boulardii. Other probiotics like Streptococcus thermophilus are also recognized for their beneficial effects on host health. Prebiotics refer to food components that cannot be digested or absorbed by the host but can be utilized by beneficial gut bacteria, thereby promoting the growth or activity of probiotics. Common prebiotics include Inulin, fructo-oligosaccharides (FOS), galacto-oligosaccharides (GOS), xylo-oligosac- chandes (XOS), chitosan-oligosaccharides (COS), and pectin-oligosaccharides (POS). Synbiotics refer to products combining probiotics and prebiotics that coexist and interact synergistically. Synbiotics contribute to enhancing gut microbiota by delivering probiotics (live beneficial microorganisms) and prebiotics (compounds that foster probiotic growth).
Supplementation with prebiotics, probiotics, and synbiotics can ameliorate OS and inflammation, adjust sex hormone levels, thereby improving sperm quality. Reshaping of GM following probiotic supplementation reduces proliferation of pathogenic bacteria, enhances intestinal barrier function, decreases oxidative stress, restores balance of SCFAs, and improves testicular function by repairing seminiferous tubule structure and increasing spermatogonial stem cells (Wu et al., 2024b), while also reducing gut-derived inflammatory mediators circulating in the bloodstream (Cai et al., 2023). For instance, supplementation with Lactobacillus rhamnosus NCDC-610 [and Lactobacillus fermentum NCDC-400 with prebiotics such as fructooligosaccharides (FOS)] enhances activities of catalase and superoxide dismutase, IL-6, IL-10, and tumor necrosis factor-alpha (TNF-α), thereby improving oxidative stress and inflammation, mitigating sperm defects induced by restraint stress, and enhancing gut health (Akram et al., 2023). Studies by Akram et al. (2022), Dardmeh et al. (2017), and others similarly demonstrate that supplementation with Lactobacillus fermentum NCDC 400 and Lactobacillus rhamnosus NCDC 610, Lactobacillus rhamnosus PB01, along with FOS, can reduce OS damage, maintain testosterone concentrations, restore testicular structure, and improve sperm vitality and motility parameters in diet-induced obesity models.
Research indicates that synbiotics (Lactobacillus paracasei + arabinoxylan oligosaccharides + FOS + L-glutamine) can regulate FSH, LH, and testosterone levels in idiopathic oligoasthenoteratozoospermia patients and improve semen volume and sperm quality/quantity (Maretti and Cavallini, 2017). Khan et al. (2024) and others have also shown that supplementation with Lactobacillus rhamnosus, Bifidobacterium, and galactooligosaccharides can enhance immature male Japanese quail estrogen, testosterone, FSH, and LH steroid hormone receptor expression through GM modulation, increase catalase to improve oxidative stress, promote testicular weight, and gonadosomatic index (GSI). FamiLact (probiotics + prebiotics) can alleviate oxidative stress, improve sperm concentration, vitality, and abnormal morphology, and reduce sperm DNA damage (Abbasi et al., 2021). Further research by Mahiddine et al. (2023) indicates that supplementation with Lactobacillus rhamnosus for 6 weeks increases relative abundance of Actinobacteria, Bacillus, and Streptomyces while decreasing Clostridium and Enterococcus, thereby enhancing sperm kinetic parameters, vitality, and acrosome integrity, and upregulating mRNA levels of genes associated with DNA repair and antioxidation.
3.2 Fecal microbiota transplantation
Fecal microbiota transplantation (FMT) involves transferring GM derived from healthy donor feces into the gastrointestinal tract of patients to treat dysbiosis-related diseases by altering GM composition. The efficacy of FMT may be linked to the specific implantation of donor phages (Wang et al., 2022; Liu et al., 2023). Increasingly valued and recognized as a novel treatment method to enhance semen quality, FMT has gained attention (Hao et al., 2022).
FMT has shown potential to mitigate inflammation and improve testicular diseases in male mice induced by GM dysbiosis from microplastics (MPs), thereby enhancing semen quality (Zhang et al., 2023; Wen et al., 2022). It can also alleviate male obesity and fertility decline caused by a HFD by enhancing systemic and testicular metabolism. For instance, studies by Hao et al. (2022), Hao et al. (2022), Yan et al. (2022) and others demonstrated that modifying GM through FMT combined with alginate oligosaccharides (AOS) (A10-FMT) improved reduced semen quality (sperm concentration and vitality) caused by a high-fat diet. A10-FMT enhanced blood metabolism and increased beneficial GM such as lactobacilli and allobacilli, including small intestinal lactobacilli, thereby elevating blood and/or testicular levels of butyric acid, docosahexaenoic acid (DHA), eicosapentaenoic acid (EPA), and testosterone, promoting spermatogenesis, and thereby improving sperm concentration, vitality, and semen quality affected by type 1 (T1D) and type 2 diabetes (T2D) through the gut-microbiota-testis axis (Hao et al., 2022).
Given the limitations in acceptance and reproductive feasibility of fecal transplantation in clinical practice, researchers have explored alternative approaches for FMT, such as transplanting viral groups, bacterial communities (e.g., phage transplantation), and fungal groups (e.g., Candida genus). Future advancements in FMT are anticipated to focus more on transplanting specific components of fecal microbiota, such as bacterial or viral components (Lam et al., 2022; Wu et al., 2023; Yu et al., 2023). Consequently, future developments like fecal bacteriophage transplantation (FBT) and fecal virome transplantation (FVT) offer potential avenues to modulate GM to enhance sperm production and motility.
3.3 Traditional natural herbal extracts
Traditional natural herbs have been widely used in clinical treatment and health care in many countries and regions (Jia et al., 2022) The GM and traditional natural herbs can interact synergistically, with herbs capable of modulating GM composition (An et al., 2019). They enhance sperm production and motility through mechanisms such as elevating SCFA levels, regulating bile acid metabolism, reducing trimethylamine oxide production, and mitigating inflammatory factor release (Li et al., 2021).
Ginseng, widely used in clinical settings, is noted for its energizing effects and fatigue-reducing properties. Research indicates that ginsenosides (Zhang et al., 2024) significantly enhance bile acid enterohepatic circulation via the FXR/CYP7A1 pathway, restore GM diversity, rebalance the Firmicutes/Bacteroidetes ratio, and ameliorate sperm damage and density (Ji et al., 2024). Chestnut polysaccharides (CPs) improve the testicular microenvironment, notably increasing germ cell counts in seminiferous tubules, adjusting GM composition by enriching Firmicutes, Proteobacteria, Bacteroidetes, Actinobacteria, and other phyla. Studies suggest that CPs metabolize through steroid hormone biosynthesis to enhance sperm production (Sun et al., 2022; Yu et al., 2020). Rhodiola rosea glycoside (Wang et al., 2023b; Wang et al., 2024c) inhibits LPS entry into the circulatory system, activates SCFA receptor mRNA expression, fortifies the intestinal barrier, alleviates orchitis, and enhances semen quality via GM regulation and metabolite adjustment. Cornus officinalis glycoside alleviates diabetes-induced testicular injury by inhibiting the AGEs-RAGE-p38 MAPK pathway, modulates intestinal flora, markedly reverses flora distribution, increases testosterone, LH, and FSH levels, and improves sperm count and vitality (Liu et al., 2021; Chen et al., 2016).
Cordyceps militaris, a parasitic fungus with medicinal properties, is utilized in food and medicine. Cordyceps polysaccharides (SeCMP) extracts exhibit structural diversity (Wu et al., 2024a) and repair intestinal mucosal damage from LPS. By augmenting lactobacilli abundance while reducing Akkermansia and Bacteroidetes, SeCMP mitigates intestinal microbiota imbalance (Wu et al., 2022). SeCMP corrects metabolic disorders, enhances testosterone synthesis in mice, raises androgen levels, increases seminiferous tubule area, thereby boosting sperm concentration and vitality in mice (Lin et al., 2022; Lin et al., 2007; Huang et al., 2023). Furthermore, SeCMP decreases rumen cocci abundance in infertile male rats, increases Romboutsia abundance, lowers serum LPS levels, and enhances sperm production by restoring intestinal microbiota diversity and inhibiting epididymitis in infertile male rats (Sheng et al., 2023).Traditional natural herbal resources are abundant and can effectively regulate GM and sperm quality. There is great potential to improve sperm quality by regulating GM, with further exploration needed regarding its application value.
In addition to the aforementioned measures, improving male fertility through gut microbiota regulation remains an ongoing area of research. Modifying lifestyle habits could potentially enhance gut microbiota, restore its balance, and improve semen quality. Lin et al. have demonstrated that dietary fiber supplements can positively affect gut microbiota and boost SCFA production, which in turn improves sperm production and semen quality (Lin et al., 2022). Conversely, chronic alcohol consumption can disrupt gut microbiota, leading to metabolic disorders, increased serum endotoxins and inflammatory cytokines, orchitis, abnormal gene expression, and ultimately, reduced sperm quality (Liu et al., 2022b).
4 Conclusion and outlook
The GM exerts dual effects on sperm through endotoxin-mediated immune and inflammatory responses, oxidative stress and antioxidant protection, metabolites of GM, epigenetic modifications, regulatory sex hormones. Prebiotics, probiotics, symbiotics, fecal microbiota transplantation, and Traditional natural herbal extracts offer potential for rectifying dysbiosis in the GM and regulating spermatogenesis and motility (Figure 3). Due to the unclear mechanisms through which specific GM and their metabolites influence sperm quality, methods aimed at enhancing male fertility by modulating the GM remain experimental, and clinical evidence is still needed. Future research should investigate the specific effects of particular GM and their metabolites on sperm quality, as well as explore the regulatory and mechanistic roles of different prebiotics, probiotics, and traditional medicines on GM and sperm quality. Given the influence of GM on sperm, continued focus on the Testis-Gut microbiota Axis is warranted, emphasizing interconnections and mutual impacts in future research directions. The diversity of GM species and the complexity of their mechanisms underscore the extensive journey ahead in this field.
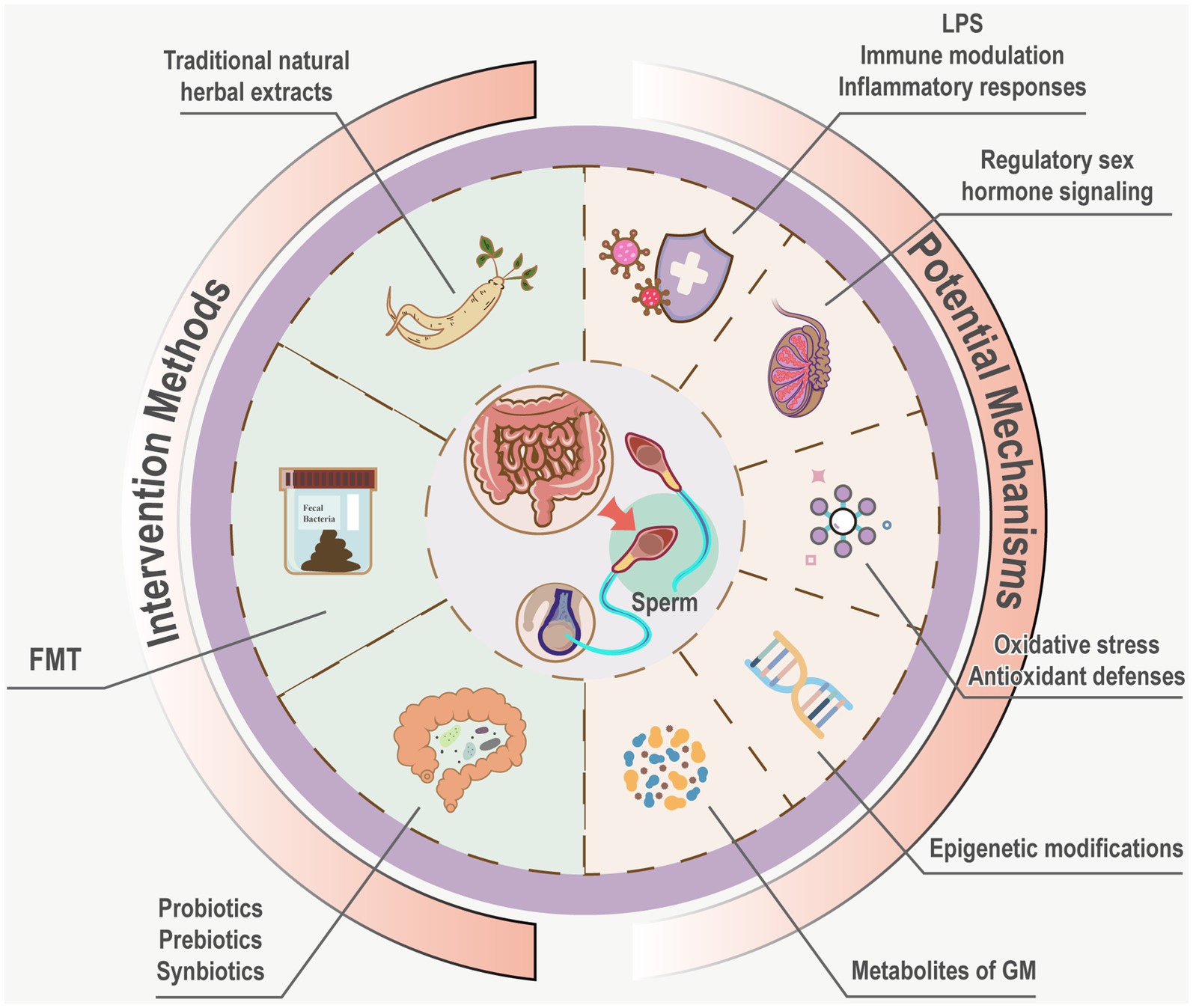
Figure 3. Potential mechanisms and intervention measures of GM influence sperm. Potential mechanisms of GM on sperm encompass immune modulation, inflammatory responses mediated by endo- toxins, oxidative stress, antioxidant defenses, metabolites of GM, epigenetic modifications, regulatory sex hormone signaling Interventions such as probiotics, prebiotics, synbiotics, FMT, and Traditional natural herbal extracts are hypothesized to rectify dysbiosis, offering avenues to modulate gut microbiota and enhance spermatogenesis and motility.
Author contributions
WKC: Conceptualization, Data curation, Formal analysis, Funding acquisition, Investigation, Methodology, Project administration, Resources, Software, Supervision, Validation, Visualization, Writing – original draft, Writing – review & editing. HDZ: Conceptualization, Data curation, Formal analysis, Investigation, Methodology, Project administration, Software, Supervision, Validation, Visualization, Writing – original draft, Writing – review & editing. HRX: Conceptualization, Formal analysis, Investigation, Methodology, Software, Supervision, Validation, Visualization, Writing – original draft, Writing – review & editing. RC: Conceptualization, Data curation, Formal analysis, Writing – original draft, Writing – review & editing. HKZ: Conceptualization, Data curation, Writing – original draft, Writing – review & editing. YPZ: Conceptualization, Data curation, Formal analysis, Writing – original draft, Writing – review & editing. JYZ: Conceptualization, Formal analysis, Funding acquisition, Investigation, Methodology, Project administration, Resources, Supervision, Writing – original draft, Writing – review & editing.
Funding
The authors declare that financial support was received for the research, authorship, and publication of this article. This research was funded by the National Natural Science Foundation of China (82274337), Science and Technology Innovation Project of China Academy of Chinese Medical Sciences (No.CI2021A02207, No.CI2023C018YL), Capital’s Funds for Health Improvement and Research (CFH 2022–2-4271).
Acknowledgments
The authors thank Adobe Illustrator for providing a drawing platform to complete all figures in this review.
Conflict of interest
The authors declare that the research was conducted in the absence of any commercial or financial relationships that could be construed as a potential conflict of interest.
Publisher’s note
All claims expressed in this article are solely those of the authors and do not necessarily represent those of their affiliated organizations, or those of the publisher, the editors and the reviewers. Any product that may be evaluated in this article, or claim that may be made by its manufacturer, is not guaranteed or endorsed by the publisher.
References
Abbasi, B., Abbasi, H., and Niroumand, H. (2021). Synbiotic (FamiLact) administration in idiopathic male infertility enhances sperm quality, DNA integrity, and chromatin status: a triple-blinded randomized clinical trial. Int. J. Reprod. Biomed. 19, 235–244. doi: 10.18502/ijrm.v19i3.8571
Afsar, T., Razak, S., Trembley, J. H., Khan, K., Shabbir, M., Almajwal, A., et al. (2022). Prevention of testicular damage by indole derivative MMINA via upregulated StAR and CatSper channels with coincident suppression of oxidative stress and inflammation: in Silico and in vivo validation. Antioxidants 11:2063. doi: 10.3390/antiox11102063
Aitken, R. J. (2017). Reactive oxygen species as mediators of sperm capacitation and pathological damage. Mol. Reprod. Dev. 84, 1039–1052. doi: 10.1002/mrd.22871
Akram, M., Ali, S. A., Behare, P., and Kaul, G. (2022). Dietary intake of probiotic fermented milk benefits the gut and reproductive health in mice fed with an obesogenic diet. Food Funct. 13, 737–752. doi: 10.1039/d1fo02501e
Akram, M., Ali, S. A., and Kaul, G. (2023). Probiotic and prebiotic supplementation ameliorates chronic restraint stress-induced male reproductive dysfunction. Food Funct. 14, 8558–8574. doi: 10.1039/d3fo03153e
Al-Asmakh, M., Stukenborg, J. B., Reda, A., Anuar, F., Strand, M. L., Hedin, L., et al. (2014). The gut microbiota and developmental programming of the testis in mice. PLoS One 9:e103809. doi: 10.1371/journal.pone.0103809
Alhaj, H. W., Li, Z., Shan, T., Dai, P., Zhu, P., Li, Y., et al. (2018). Effects of dietary sodium butyrate on reproduction in adult breeder roosters. Anim. Reprod. Sci. 196, 111–119. doi: 10.1016/j.anireprosci.2018.07.002
An, X., Bao, Q., Di, S., Zhao, Y., Zhao, S., Zhang, H., et al. (2019). The interaction between the gut microbiota and herbal medicines. Biomed. Pharmacother. 118:109252. doi: 10.1016/j.biopha.2019.109252
Argaw-Denboba, A., Schmidt, T. S. B., Di Giacomo, M., Ranjan, B., Devendran, S., Mastrorilli, E., et al. (2024). Paternal microbiome perturbations impact offspring fitness. Nature 629, 652–659. doi: 10.1038/s41586-024-07336-w
Ashonibare, V. J., Akorede, B. A., Ashonibare, P. J., Akhigbe, T. M., and Akhigbe, R. E. (2024). Gut microbiota-gonadal axis: the impact of gut microbiota on reproductive functions. Front. Immunol. 15:1346035. doi: 10.3389/fimmu.2024.1346035
Barati, E., Nikzad, H., and Karimian, M. (2020). Oxidative stress and male infertility: current knowledge of pathophysiology and role of antioxidant therapy in disease management. Cell. Mol. Life Sci. 77, 93–113. doi: 10.1007/s00018-019-03253-8
Beygi, Z., Forouhari, S., Mahmoudi, E., Hayat, S. M. G., and Nourimand, F. (2021). Role of oxidative stress and antioxidant supplementation in male fertility. Curr. Mol. Med. 21, 265–282. doi: 10.2174/1566524020999200831123553
Brecchia, G., Cardinali, R., Mourvaki, E., Collodel, G., Moretti, E., Dal Bosco, A., et al. (2010). Short- and long-term effects of lipopolysaccharide-induced inflammation on rabbit sperm quality. Anim. Reprod. Sci. 118, 310–316. doi: 10.1016/j.anireprosci.2009.06.016
Brown, E. M., Kenny, D. J., and Xavier, R. J. (2019). Gut microbiota regulation of T cells during inflammation and autoimmunity. Annu. Rev. Immunol. 37, 599–624. doi: 10.1146/annurev-immunol-042718-041841
Cai, H., Qin, D., Liu, Y., Guo, X., Liu, Y., Ma, B., et al. (2023). Remodeling of gut microbiota by probiotics alleviated heat stroke-induced necroptosis in male germ cells. Mol. Nutr. Food Res. 67:e2300291. doi: 10.1002/mnfr.202300291
Candelli, M., Franza, L., Pignataro, G., Ojetti, V., Covino, M., Piccioni, A., et al. (2021). Interaction between lipopolysaccharide and gut microbiota in inflammatory bowel diseases. Int. J. Mol. Sci. 22:6242. doi: 10.3390/ijms22126242
Cao, D., Li, Y., Yang, R., Wang, Y., Zhou, Y., Diao, H., et al. (2010). Lipopolysaccharide-induced epididymitis disrupts epididymal beta-defensin expression and inhibits sperm motility in rats. Biol. Reprod. 83, 1064–1070. doi: 10.1095/biolreprod.109.082180
Chen, Y., Wang, J., Zhang, Q., Xiang, Z., Li, D., and Han, X. (2017). Microcystin-leucine arginine exhibits immunomodulatory roles in testicular cells resulting in orchitis. Environmental Poll. 229, 964–975. doi: 10.1016/j.envpol.2017.07.081
Chen, Y., Wu, Y., Gan, X., Liu, K., Lv, X., Shen, H., et al. (2016). Iridoid glycoside from Cornus officinalis ameliorated diabetes mellitus-induced testicular damage in male rats: involvement of suppression of the AGEs/RAGE/p38 MAPK signaling pathway. J. Ethnopharmacol. 194, 850–860. doi: 10.1016/j.jep.2016.10.079
Chen, Y., Zhou, J., and Wang, L. (2021). Role and mechanism of gut microbiota in human disease. Front. Cell. Infect. Microbiol. 11:625913. doi: 10.3389/fcimb.2021.625913
Cheng, C. Y., and Mruk, D. D. (2012). The blood-testis barrier and its implications for male contraception. Pharmacol. Rev. 64, 16–64. doi: 10.1124/pr.110.002790
Collodel, G., Castellini, C., del Vecchio, M. T., Cardinali, R., Geminiani, M., Rossi, B., et al. (2012). Effect of a bacterial lipopolysaccharide treatment on rabbit testis and ejaculated sperm. Reprod. Domestic Animals 47, 372–378. doi: 10.1111/j.1439-0531.2011.01882.x
Cox, C. M., Thoma, M. E., Tchangalova, N., Mburu, G., Bornstein, M. J., Johnson, C. L., et al. (2022). Infertility prevalence and the methods of estimation from 1990 to 2021: a systematic review and meta-analysis. Hum. Reprod. Open 2022:hoac051. doi: 10.1093/hropen/hoac051
Dardmeh, F., Alipour, H., Gazerani, P., van der Horst, G., Brandsborg, E., and Nielsen, H. I. (2017). Lactobacillus rhamnosus PB01 (DSM 14870) supplementation affects markers of sperm kinematic parameters in a diet-induced obesity mice model. PLoS One 12:e0185964. doi: 10.1371/journal.pone.0185964
de Vos, W. M., Tilg, H., Van Hul, M., and Cani, P. D. (2022). Gut microbiome and health: mechanistic insights. Gut 71, 1020–1032. doi: 10.1136/gutjnl-2021-326789
Di Lorenzo, F., De Castro, C., Silipo, A., and Molinaro, A. (2019). Lipopolysaccharide structures of gram-negative populations in the gut microbiota and effects on host interactions. FEMS Microbiol. Rev. 43, 257–272. doi: 10.1093/femsre/fuz002
Ding, N., Zhang, X., Zhang, X. D., Jing, J., Liu, S. S., Mu, Y. P., et al. (2020). Impairment of spermatogenesis and sperm motility by the high-fat diet-induced dysbiosis of gut microbes. Gut 69, 1608–1619. doi: 10.1136/gutjnl-2019-319127
Doden, H. L., Pollet, R. M., Mythen, S. M., Wawrzak, Z., Devendran, S., Cann, I., et al. (2019). Structural and biochemical characterization of 20β-hydroxysteroid dehydrogenase from Bifidobacterium adolescentis strain L2-32. J. Biol. Chem. 294, 12040–12053. doi: 10.1074/jbc.RA119.009390
Du Plessis, S. S., Agarwal, A., Halabi, J., and Tvrda, E. (2015). Contemporary evidence on the physiological role of reactive oxygen species in human sperm function. J. Assist. Reprod. Genet. 32, 509–520. doi: 10.1007/s10815-014-0425-7
Eisenberg, M. L., Esteves, S. C., Lamb, D. J., Hotaling, J. M., Giwercman, A., Hwang, K., et al. (2023). Male infertility. Nat. Rev. Dis. Primers 9:49. doi: 10.1038/s41572-023-00459-w
Fainberg, J., and Kashanian, J. A. (2019). Recent advances in understanding and managing male infertility. F1000Research 8:7076. doi: 10.12688/f1000research.17076.1
Fernandez, M. C., and O'Flaherty, C. (2018). Peroxiredoxin 6 is the primary antioxidant enzyme for the maintenance of viability and DNA integrity in human spermatozoa. Hum. Reprod. 33, 1394–1407. doi: 10.1093/humrep/dey221
Fernandez, M. C., Yu, A., Moawad, A. R., and O'Flaherty, C. (2019). Peroxiredoxin 6 regulates the phosphoinositide 3-kinase/AKT pathway to maintain human sperm viability. Mol. Hum. Reprod. 25, 787–796. doi: 10.1093/molehr/gaz060
Ferro, D., Baratta, F., Pastori, D., Cocomello, N., Colantoni, A., Angelico, F., et al. (2020). New insights into the pathogenesis of non-alcoholic fatty liver disease: gut-derived lipopolysaccharides and oxidative stress. Nutrients 12:2762. doi: 10.3390/nu12092762
Fogelson, K. A., Dorrestein, P. C., Zarrinpar, A., and Knight, R. (2023). The gut microbial bile acid modulation and its relevance to digestive health and diseases. Gastroenterology 164, 1069–1085. doi: 10.1053/j.gastro.2023.02.022
Folliero, V., Santonastaso, M., Dell'Annunziata, F., De Franciscis, P., Boccia, G., Colacurci, N., et al. (2022). Impact of Escherichia coli outer membrane vesicles on sperm function. Pathogens 11:782. doi: 10.3390/pathogens11070782
Fu, Z. D., Wang, Y., and Yan, H. L. (2023). Male infertility risk and gut microbiota: a Mendelian randomization study. Front. Microbiol. 14:1228693. doi: 10.3389/fmicb.2023.1228693
Fusco, W., Lorenzo, M. B., Cintoni, M., Porcari, S., Rinninella, E., Kaitsas, F., et al. (2023). Short-chain fatty-acid-producing Bacteria: key components of the human gut microbiota. Nutrients 15:2211. doi: 10.3390/nu15092211
Gibson, G. R., Hutkins, R., Sanders, M. E., Prescott, S. L., Reimer, R. A., Salminen, S. J., et al. (2017). Expert consensus document: the international scientific Association for Probiotics and Prebiotics (ISAPP) consensus statement on the definition and scope of prebiotics. Nat. Rev. Gastroenterol. Hepatol. 14, 491–502. doi: 10.1038/nrgastro.2017.75
Gilbert, J. A., Blaser, M. J., Caporaso, J. G., Jansson, J. K., Lynch, S. V., and Knight, R. (2018). Current understanding of the human microbiome. Nat. Med. 24, 392–400. doi: 10.1038/nm.4517
Guo, Q., Cheng, Y., Li, T., Huang, J., Li, J., Zhang, Z., et al. (2024). The gut microbiota contributes to the development of LPS-induced Orchitis by disrupting the blood-testosterone barrier in mice. Reprod. Sci. doi: 10.1007/s43032-024-01613-9
Gupta, S. K., Vyavahare, S., Duchesne Blanes, I. L., Berger, F., Isales, C., and Fulzele, S. (2023). Microbiota-derived tryptophan metabolism: impacts on health, aging, and disease. Exp. Gerontol. 183:112319. doi: 10.1016/j.exger.2023.112319
Hao, Y., Feng, Y., Yan, X., Chen, L., Ma, X., Tang, X., et al. (2022). Gut microbiota-testis Axis: FMT mitigates high-fat diet-diminished male fertility via improving systemic and testicular metabolome. Microbiol. Spectr. 10:e0002822. doi: 10.1128/spectrum.00028-22
Hao, Y., Feng, Y., Yan, X., Chen, L., Zhong, R., Tang, X., et al. (2022). Gut microbiota-testis axis: FMT improves systemic and testicular micro-environment to increase semen quality in type 1 diabetes. Mol. Med. 28:45. doi: 10.1186/s10020-022-00473-w
He, B., Guo, H., Gong, Y., and Zhao, R. (2017). Lipopolysaccharide-induced mitochondrial dysfunction in boar sperm is mediated by activation of oxidative phosphorylation. Theriogenology 87, 1–8. doi: 10.1016/j.theriogenology.2016.07.030
He, S., Li, H., Yu, Z., Zhang, F., Liang, S., Liu, H., et al. (2021). The gut microbiome and sex hormone-related diseases. Front. Microbiol. 12:711137. doi: 10.3389/fmicb.2021.711137
He, G., Zhang, B., Yi, K., Chen, T., Shen, C., Cao, M., et al. (2024). Heat stress-induced dysbiosis of the gut microbiota impairs spermatogenesis by regulating secondary bile acid metabolism in the gut. Sci. Total Environ. 937, 937:173305. doi: 10.1016/j.scitotenv.2024.173305
Hill, C., Guarner, F., Reid, G., Gibson, G. R., Merenstein, D. J., Pot, B., et al. (2014). Expert consensus document. The international scientific Association for Probiotics and Prebiotics consensus statement on the scope and appropriate use of the term probiotic. Nat. Rev. Gastroenterol. Hepatol. 11, 506–514. doi: 10.1038/nrgastro.2014.66
Huang, S., Zou, Y., Tang, H., Zhuang, J., Ye, Z., Wei, T., et al. (2023). Cordyceps militaris polysaccharides modulate gut microbiota and improve metabolic disorders in mice with diet-induced obesity. J. Sci. Food Agric. 103, 1885–1894. doi: 10.1002/jsfa.12409
Jandhyala, S. M., Talukdar, R., Subramanyam, C., Vuyyuru, H., Sasikala, M., and Nageshwar Reddy, D. (2015). Role of the normal gut microbiota. World J. Gastroenterol. 21, 8787–8803. doi: 10.3748/wjg.v21.i29.8787
Ji, X., Yu, L., Han, C., Gao, H., Cai, Y., Li, J., et al. (2024). Investigating the effects of rare ginsenosides on hyperuricemia and associated sperm damage via nontargeted metabolomics and gut microbiota. J. Ethnopharmacol. 332:118362. doi: 10.1016/j.jep.2024.118362
Jia, Q., He, Q., Yao, L., Li, M., Lin, J., Tang, Z., et al. (2022). Utilization of physiologically based pharmacokinetic modeling in pharmacokinetic study of natural medicine: An overview. Molecules 27:8670. doi: 10.3390/molecules27248670
Jin, Z., Cao, Y., Wen, Q., Zhang, H., Fang, Z., Zhao, Q., et al. (2024). Dapagliflozin ameliorates diabetes-induced spermatogenic dysfunction by modulating the adenosine metabolism along the gut microbiota-testis axis. Sci. Rep. 14:641. doi: 10.1038/s41598-024-51224-2
Jin, Z., Yang, Y., Cao, Y., Wen, Q., Xi, Y., Cheng, J., et al. (2023). The gut metabolite 3-hydroxyphenylacetic acid rejuvenates spermatogenic dysfunction in aged mice through GPX4-mediated ferroptosis. Microbiome 11:212. doi: 10.1186/s40168-023-01659-y
Kaprara, A., and Huhtaniemi, I. T. (2018). The hypothalamus-pituitary-gonad axis: Tales of mice and men. Metab. Clin. Exp. 86, 3–17. doi: 10.1016/j.metabol.2017.11.018
Khan, A., Kango, N., and Srivastava, R. (2024). Impact of dietary probiotics on the immune and reproductive physiology of pubertal male Japanese quail (Coturnix coturnix japonica) administered at the onset of pre-puberty. Probiot. Antimicrob. Proteins. doi: 10.1007/s12602-023-10209-9
Khanmohammad, K. R., Khalili, M. B., Sadeh, M., Talebi, A. R., Astani, A., Shams, A., et al. (2021). The effect of lipopolysaccharide from uropathogenic Escherichia coli on the immune system, testis tissue, and spermatozoa of BALB/c mice. Clin. Exp. Reprod. Med. 48, 105–110. doi: 10.5653/cerm.2020.03888
Kumar, H., Lund, R., Laiho, A., Lundelin, K., Ley, R. E., Isolauri, E., et al. (2014). Gut microbiota as an epigenetic regulator: pilot study based on whole-genome methylation analysis. MBio 5, e02113–e02114. doi: 10.1128/mBio.02113-14
Lam, S., Bai, X., Shkoporov, A. N., Park, H., Wu, X., Lan, P., et al. (2022). Roles of the gut virome and mycobiome in faecal microbiota transplantation. Lancet Gastroenterol. Hepatol. 7, 472–484. doi: 10.1016/S2468-1253(21)00303-4
Lee, D., Moawad, A. R., Morielli, T., Fernandez, M. C., and O'Flaherty, C. (2017). Peroxiredoxins prevent oxidative stress during human sperm capacitation. Mol. Hum. Reprod. 23, 106–115. doi: 10.1093/molehr/gaw081
Lee, M. H., Nuccio, S. P., Mohanty, I., Hagey, L. R., Dorrestein, P. C., Chu, H., et al. (2024). How bile acids and the microbiota interact to shape host immunity. Nat. Rev. Immunol. doi: 10.1038/s41577-024-01057-x
Levine, H., Jørgensen, N., Martino-Andrade, A., Mendiola, J., Weksler-Derri, D., Jolles, M., et al. (2023). Temporal trends in sperm count: a systematic review and meta-regression analysis of samples collected globally in the 20th and 21st centuries. Hum. Reprod. Update 29, 157–176. doi: 10.1093/humupd/dmac035
Li, X., Cheng, W., Shang, H., Wei, H., and Deng, C. (2022c). The interplay between androgen and gut microbiota: is there a microbiota-gut-testis Axis. Reprod. Sci. 29, 1674–1684. doi: 10.1007/s43032-021-00624-0
Li, Y., Ji, X., Wu, H., Li, X., Zhang, H., and Tang, D. (2021). Mechanisms of traditional Chinese medicine in modulating gut microbiota metabolites-mediated lipid metabolism. J. Ethnopharmacol. 278:114207. doi: 10.1016/j.jep.2021.114207
Li, H., Li, N., Lu, Q., Yang, J., Zhao, J., Zhu, Q., et al. (2022b). Chronic alcohol-induced dysbiosis of the gut microbiota and gut metabolites impairs sperm quality in mice. Front. Microbiol. 13:1042923. doi: 10.3389/fmicb.2022.1042923
Li, D., Li, Y., Yang, S., Lu, J., Jin, X., and Wu, M. (2022c). Diet-gut microbiota-epigenetics in metabolic diseases: from mechanisms to therapeutics. Biomed. Pharmacother. 153:113290. doi: 10.1016/j.biopha.2022.113290
Li, Y., Liu, Y., Chen, Y., Yao, C., Yu, S., Qu, J., et al. (2024). Combined effects of polystyrene nanoplastics and lipopolysaccharide on testosterone biosynthesis and inflammation in mouse testis. Ecotoxicol. Environ. Saf. 273:116180. doi: 10.1016/j.ecoenv.2024.116180
Li, Y., Ma, H., and Wang, J. (2024). Effects of polycyclic aromatic hydrocarbons on the gut-testis axis. Ecotoxicol. Environ. Saf. 280:116539. doi: 10.1016/j.ecoenv.2024.116539
Li, X. Y., Meng, L., Shen, L., and Ji, H. F. (2023). Regulation of gut microbiota by vitamin C, vitamin E and β-carotene. Food Res. Int. 169:112749. doi: 10.1016/j.foodres.2023.112749
Lin, S., Hsu, W. K., Tsai, M. S., Hsu, T. H., Lin, T. C., Su, H. L., et al. (2022). Effects of Cordyceps militaris fermentation products on reproductive development in juvenile male mice. Sci. Rep. 12:13720. doi: 10.1038/s41598-022-18066-2
Lin, W. H., Tsai, M. T., Chen, Y. S., Hou, R. C., Hung, H. F., Li, C. H., et al. (2007). Improvement of sperm production in subfertile boars by Cordyceps militaris supplement. Am. J. Chin. Med. 35, 631–641. doi: 10.1142/S0192415X07005120
Lin, Y., Wang, K., Che, L., Fang, Z., Xu, S., Feng, B., et al. (2022). The improvement of semen quality by dietary Fiber intake is positively related with gut microbiota and SCFA in a boar model. Front. Microbiol. 13:863315. doi: 10.3389/fmicb.2022.863315
Liu, J. B., Chen, K., Li, Z. F., Wang, Z. Y., and Wang, L. (2022a). Glyphosate-induced gut microbiota dysbiosis facilitates male reproductive toxicity in rats. Sci. Total Environ. 805:150368. doi: 10.1016/j.scitotenv.2021.150368
Liu, X., Hu, G., Wang, A., Long, G., Yang, Y., Wang, D., et al. (2022b). Black tea reduces diet-induced obesity in mice via modulation of gut microbiota and gene expression in host tissues. Nutrients 14:1635. doi: 10.3390/nu14081635
Liu, L., Shu, A., Zhu, Y., and Chen, Y. (2021). Cornuside alleviates diabetes mellitus-induced testicular damage by modulating the gut microbiota. Evid. Complement. Alternat. Med. 2021, 5301942–5301913. doi: 10.1155/2021/5301942
Liu, J., Tan, Y., Cheng, H., Zhang, D., Feng, W., and Peng, C. (2022b). Functions of gut microbiota metabolites, current status and future perspectives. Aging Dis. 13, 1106–1126. doi: 10.14336/AD.2022.0104
Liu, Q., Xu, Z., Dai, M., Su, Q., Leung Chan, F. K., and Ng, S. C. (2023). Faecal microbiota transplantations and the role of bacteriophages. Clin. Microbiol. Infect. 29, 689–694. doi: 10.1016/j.cmi.2022.11.012
Lloyd-Price, J., Abu-Ali, G., and Huttenhower, C. (2016). The healthy human microbiome. Genome Med. 8:51. doi: 10.1186/s13073-016-0307-y
Lundy, S. D., Sangwan, N., Parekh, N. V., Selvam, M. K. P., Gupta, S., McCaffrey, P., et al. (2021). Functional and taxonomic Dysbiosis of the gut, urine, and semen microbiomes in male infertility. Eur. Urol. 79, 826–836. doi: 10.1016/j.eururo.2021.01.014
Lv, S., Huang, J., Luo, Y., Wen, Y., Chen, B., Qiu, H., et al. (2024). Gut microbiota is involved in male reproductive function: a review. Front. Microbiol. 15:1371667. doi: 10.3389/fmicb.2024.1371667
Magill, R. G., and MacDonald, S. M. (2023). Male infertility and the human microbiome. Front. Reprod. Health 5:1166201. Published 2023 Jun 9. doi: 10.3389/frph.2023.1166201
Mahiddine, F. Y., You, I., Park, H., and Kim, M. J. (2023). Management of dog sperm parameters and gut microbiota composition with Lactobacillus rhamnosus supplementation. Vet. Res. Commun. 47, 1629–1640. doi: 10.1007/s11259-023-10116-y
Maretti, C., and Cavallini, G. (2017). The association of a probiotic with a prebiotic (Flortec, Bracco) to improve the quality/quantity of spermatozoa in infertile patients with idiopathic oligoasthenoteratospermia: a pilot study. Andrology 5, 439–444. doi: 10.1111/andr.12336
Maynard, C. L., Elson, C. O., Hatton, R. D., and Weaver, C. T. (2012). Reciprocal interactions of the intestinal microbiota and immune system. Nature 489, 231–241. doi: 10.1038/nature11551
Mohr, A. E., Crawford, M., Jasbi, P., Fessler, S., and Sweazea, K. L. (2022). Lipopolysaccharide and the gut microbiota: considering structural variation. FEBS Lett. 596, 849–875. doi: 10.1002/1873-3468.14328
Noguchi, J., Watanabe, S., Nguyen, T. Q., Kikuchi, K., and Kaneko, H. (2017). Development of a lipopolysaccharide (LPS) -supplemented adjuvant and its effects on cell-mediated and humoral immune responses in male rats immunized against sperm. J. Reprod. Dev. 63, 111–115. doi: 10.1262/jrd.2016-144
O'Doherty, A. M., Di Fenza, M., and Kölle, S. (2016). Lipopolysaccharide (LPS) disrupts particle transport, cilia function and sperm motility in an ex vivo oviduct model. Sci. Rep. 6:24583. doi: 10.1038/srep24583
O'Flaherty, C., and Scarlata, E. (2022). Oxidative stress and reproductive function: the protection of mammalian spermatozoa against oxidative stress. Reproduction 164, F67–F78. doi: 10.1530/REP-22-0200
Oliveira, L. C. S. L., Costa, E. C., Martins, F. D. G., Rocha, A. S. D., and Brasil, G. A. (2024). Probiotics supplementation in the treatment of male infertility: a systematic review. JBRA Assist. Reprod. 28, 341–348. doi: 10.5935/1518-0557.20240013
Parker, M. I., and Palladino, M. A. (2017). MicroRNAs downregulated following immune activation of rat testis. Amer. J. Reprod. Immunol. 77:2673. doi: 10.1111/aji.12673
Poutahidis, T., Springer, A., Levkovich, T., Qi, P., Varian, B. J., Lakritz, J. R., et al. (2014). Probiotic microbes sustain youthful serum testosterone levels and testicular size in aging mice. PLoS One 9:e84877. doi: 10.1371/journal.pone.0084877
Ramírez-Acosta, S., Selma-Royo, M., Collado, M. C., Navarro-Roldán, F., Abril, N., and García-Barrera, T. (2022). Selenium supplementation influences mice testicular selenoproteins driven by gut microbiota. Sci. Rep. 12:4218. doi: 10.1038/s41598-022-08121-3
Rayman, M. P. (2012). Selenium and human health. Lancet 379, 1256–1268. doi: 10.1016/S0140-6736(11)61452-9
Ridlon, J. M., Ikegawa, S., Alves, J. M., Zhou, B., Kobayashi, A., Iida, T., et al. (2013). Clostridium scindens: a human gut microbe with a high potential to convert glucocorticoids into androgens. J. Lipid Res. 54, 2437–2449. doi: 10.1194/jlr.M038869
Rizzetto, L., Fava, F., Tuohy, K. M., and Selmi, C. (2018). Connecting the immune system, systemic chronic inflammation and the gut microbiome: the role of sex. J. Autoimmun. 92, 12–34. doi: 10.1016/j.jaut.2018.05.008
Sanders, M. E., Merenstein, D. J., Reid, G., Gibson, G. R., and Rastall, R. A. (2019). Probiotics and prebiotics in intestinal health and disease: from biology to the clinic. Nat. Rev. Gastroenterol. Hepatol. 16, 605–616. doi: 10.1038/s41575-019-0173-3
Schmidt, T. S. B., Raes, J., and Bork, P. (2018). The human gut microbiome: from association to modulation. Cell 172, 1198–1215. doi: 10.1016/j.cell.2018.02.044
Schoeler, M., and Caesar, R. (2019). Dietary lipids, gut microbiota and lipid metabolism. Rev. Endocr. Metab. Disord. 20, 461–472. doi: 10.1007/s11154-019-09512-0
Sheng, W., Xu, W., Ding, J., Lu, B., Liu, L., He, Q., et al. (2023). Guijiajiao (Colla Carapacis et Plastri, CCP) prevents male infertility via gut microbiota modulation. Chin. J. Nat. Med. 21, 403–410. doi: 10.1016/S1875-5364(23)60471-6
Shin, J. H., Park, Y. H., Sim, M., Kim, S. A., Joung, H., and Shin, D. M. (2019). Serum level of sex steroid hormone is associated with diversity and profiles of human gut microbiome. Res. Microbiol. 170, 192–201. doi: 10.1016/j.resmic.2019.03.003
Silva, E. J. R., Ribeiro, C. M., Mirim, A. F. M., Silva, A. A. S., Romano, R. M., Hallak, J., et al. (2018). Lipopolysaccharide and lipotheicoic acid differentially modulate epididymal cytokine and chemokine profiles and sperm parameters in experimental acute epididymitis. Sci. Rep. 8:103. doi: 10.1038/s41598-017-17944-4
Skvortsova, K., Iovino, N., and Bogdanović, O. (2018). Functions and mechanisms of epigenetic inheritance in animals. Nat. Rev. Mol. Cell Biol. 19, 774–790. doi: 10.1038/s41580-018-0074-2
Sun, H., Lv, B., Zhu, H., Zeng, Z., El-Ashram, S., Li, J., et al. (2023). Selenized glucose improves rat semen quality by improving the gut microbiota and serum metabolome. Food Funct. 14, 5105–5119. doi: 10.1039/d3fo00692a
Sun, Z. Y., Yu, S., Tian, Y., Han, B. Q., Zhao, Y., Li, Y. Q., et al. (2022). Chestnut polysaccharides restore impaired spermatogenesis by adjusting gut microbiota and the intestinal structure. Food Funct. 13, 425–436. doi: 10.1039/d1fo03145g
Swanson, K. S., Gibson, G. R., Hutkins, R., Reimer, R. A., Reid, G., Verbeke, K., et al. (2020). The international scientific Association for Probiotics and Prebiotics (ISAPP) consensus statement on the definition and scope of synbiotics. Nat. Rev. Gastroenterol. Hepatol. 17, 687–701. doi: 10.1038/s41575-020-0344-2
Takumi, N., Shirakawa, H., Ohsaki, Y., Ito, A., Watanabe, T., Giriwono, P. E., et al. (2011). Dietary vitamin K alleviates the reduction in testosterone production induced by lipopolysaccharide administration in rat testis. Food Funct. 2, 406–411. doi: 10.1039/c1fo10058k
Tang, L., Yang, X., Zhou, M., Feng, L., Ji, C., Liang, J., et al. (2024). Inhibition of inosine metabolism of the gut microbiota decreases testosterone secretion in the testis. mSystems 9:e0013824. doi: 10.1128/msystems.00138-24
Tian, Y., Gui, W., Koo, I., Smith, P. B., Allman, E. L., Nichols, R. G., et al. (2020). The microbiome modulating activity of bile acids. Gut Microbes 11, 979–996. doi: 10.1080/19490976.2020.1732268
Tournaye, H., Krausz, C., and Oates, R. D. (2017). Novel concepts in the aetiology of male reproductive impairment. Lancet Diabetes Endocrinol. 5, 544–553. doi: 10.1016/S2213-8587(16)30040-7
Tremellen, K., McPhee, N., Pearce, K., Benson, S., Schedlowski, M., and Engler, H. (2018). Endotoxin-initiated inflammation reduces testosterone production in men of reproductive age. Am. J. Physiol. Endocrinol. Metab. 314, E206–E213. doi: 10.1152/ajpendo.00279.2017
Uchiyama, J., Akiyama, M., Hase, K., Kumagai, Y., and Kim, Y. G. (2022). Gut microbiota reinforce host antioxidant capacity via the generation of reactive sulfur species. Cell Rep. 38:110479. doi: 10.1016/j.celrep.2022.110479
van der Hee, B., and Wells, J. M. (2021). Microbial regulation of host physiology by short-chain fatty acids. Trends Microbiol. 29, 700–712. doi: 10.1016/j.tim.2021.02.001
Wang, N., Chen, L., Yi, K., Zhang, B., Li, C., and Zhou, X. (2024a). The effects of microbiota on reproductive health: a review. Crit. Rev. Food Sci. Nutr. 64, 1486–1507. doi: 10.1080/10408398.2022.2117784
Wang, G., Fan, Y., Zhang, G., Cai, S., Ma, Y., Yang, L., et al. (2024b). Microbiota-derived indoles alleviate intestinal inflammation and modulate microbiome by microbial cross-feeding. Microbiome 12:59. doi: 10.1186/s40168-024-01750-y
Wang, Z., Li, L., Li, W., Yan, H., and Yuan, Y. (2024c). Salidroside alleviates furan-induced impaired gut barrier and inflammation via gut microbiota-SCFA-TLR4 signaling. J. Agric. Food Chem. 72, 16484–16495. doi: 10.1021/acs.jafc.4c02433
Wang, Z., Li, L., Yan, H., Li, W., Pang, Y., and Yuan, Y. (2023a). Salidroside ameliorates furan-induced testicular inflammation in relation to the gut-testis Axis and intestinal apoptosis. J. Agric. Food Chem. 71, 17968–17987. doi: 10.1021/acs.jafc.3c06587
Wang, F., Liu, W., Jiang, Q., Gong, M., Chen, R., Wu, H., et al. (2019). Lipopolysaccharide-induced testicular dysfunction and epididymitis in mice: a critical role of tumor necrosis factor alpha†. Biol. Reprod. 100, 849–861. doi: 10.1093/biolre/ioy235
Wang, M., Ren, C., Wang, P., Cheng, X., Chen, Y., Huang, Y., et al. (2023b). Microbiome-metabolome reveals the contribution of the gut-testis Axis to sperm motility in sheep (Ovis aries). Animals 13:996. doi: 10.3390/ani13060996
Wang, Y., Wu, Y., Wang, Y., Xu, H., Mei, X., Yu, D., et al. (2017). Antioxidant properties of probiotic Bacteria. Nutrients 9:521. doi: 10.3390/nu9050521
Wang, Y., and Xie, Z. (2022). Exploring the role of gut microbiome in male reproduction. Andrology 10, 441–450. doi: 10.1111/andr.13143
Wang, Y., Zhang, S., Borody, T. J., and Zhang, F. (2022). Encyclopedia of fecal microbiota transplantation: a review of effectiveness in the treatment of 85 diseases. Chin. Med. J. 135, 1927–1939. doi: 10.1097/CM9.0000000000002339
Wei, M., Liu, H., Wang, Y., Sun, M., and Shang, P. (2024). Mechanisms of male reproductive sterility triggered by Dysbiosis of intestinal microorganisms. Life 14:694. doi: 10.3390/life14060694
Wen, S., Zhao, Y., Liu, S., Yuan, H., You, T., and Xu, H. (2022). Microplastics-perturbed gut microbiota triggered the testicular disorder in male mice: via fecal microbiota transplantation. Environ. Poll. 309:119789. doi: 10.1016/j.envpol.2022.119789
Woo, V., and Alenghat, T. (2022). Epigenetic regulation by gut microbiota. Gut Microbes 14:2022407. doi: 10.1080/19490976.2021.2022407
Wu, N., Ge, X., Yin, X., Yang, L., Chen, L., Shao, R., et al. (2024a). A review on polysaccharide biosynthesis in Cordyceps militaris. Int. J. Biol. Macromol. 260:129336. doi: 10.1016/j.ijbiomac.2024.129336
Wu, S., Wu, Q., Wang, J., Li, Y., Chen, B., Zhu, Z., et al. (2022). Novel selenium peptides obtained from selenium-enriched Cordyceps militaris alleviate Neuroinflammation and gut microbiota Dysbacteriosis in LPS-injured mice. J. Agric. Food Chem. 70, 3194–3206. doi: 10.1021/acs.jafc.1c08393
Wu, D., Zhang, C., Liu, Y., Yao, J., Yang, X., Wu, S., et al. (2023). Beyond faecal microbiota transplantation, the non-negligible role of faecal virome or bacteriophage transplantation. J. Microbiol. Immunol. Infect. 56, 893–908. doi: 10.1016/j.jmii.2023.02.005
Wu, J., Zhou, T., Shen, H., Jiang, Y., Yang, Q., Su, S., et al. (2024b). Mixed probiotics modulated gut microbiota to improve spermatogenesis in bisphenol A-exposed male mice. Ecotoxicol. Environ. Saf. 270:115922. doi: 10.1016/j.ecoenv.2023.115922
Xavier, M. J., Roman, S. D., Aitken, R. J., and Nixon, B. (2019). Transgenerational inheritance: how impacts to the epigenetic and genetic information of parents affect offspring health. Hum. Reprod. Update 25, 519–541. doi: 10.1093/humupd/dmz017
Xiao, X., Mruk, D. D., and Cheng, C. Y. (2013). Intercellular adhesion molecules (ICAMs) and spermatogenesis. Hum. Reprod. Update 19, 167–186. doi: 10.1093/humupd/dms049
Yadav, M. K., Kumari, I., Singh, B., Sharma, K. K., and Tiwari, S. K. (2022). Probiotics, prebiotics and synbiotics: safe options for next-generation therapeutics. Appl. Microbiol. Biotechnol. 106, 505–521. doi: 10.1007/s00253-021-11646-8
Yan, X., Feng, Y., Hao, Y., Zhong, R., Jiang, Y., Tang, X., et al. (2022). Gut-testis Axis: microbiota prime metabolome to increase sperm quality in young type 2 diabetes. Microbiol. Spectr. 10:e0142322. doi: 10.1128/spectrum.01423-22
Yan, Z., Zheng, Z., Xia, T., Ni, Z., Dou, Y., and Liu, X. (2024). Causal relationship between gut microbiome and sex hormone-binding globulin: a bidirectional two-sample Mendelian randomization study. Amer. J. Reprod. Immunol. 91:e13824. doi: 10.1111/aji.13824
Yu, Y., Wang, W., and Zhang, F. (2023). The next generation fecal microbiota transplantation: to transplant Bacteria or Virome. Adv. Sci. 10:e2301097. doi: 10.1002/advs.202301097
Yu, S., Zhao, Y., Zhang, F. L., Li, Y. Q., Shen, W., and Sun, Z. Y. (2020). Chestnut polysaccharides benefit spermatogenesis through improvement in the expression of important genes. Aging 12, 11431–11445. doi: 10.18632/aging.103205
Zeng, Z., Lv, B., Tang, Y. E., Sun, H., Li, S., He, Y., et al. (2024). Effects of dietary selenized glucose on intestinal microbiota and tryptophan metabolism in rats: assessing skatole reduction potential. Environ. Res. 252:118874. doi: 10.1016/j.envres.2024.118874
Zhang, Y., Hou, B., Liu, T., Wu, Y., and Wang, Z. (2023). Probiotics improve polystyrene microplastics-induced male reproductive toxicity in mice by alleviating inflammatory response. Ecotoxicol. Environ. Saf. 263:115248. doi: 10.1016/j.ecoenv.2023.115248
Zhang, T., Sun, P., Geng, Q., Fan, H., Gong, Y., Hu, Y., et al. (2022). Disrupted spermatogenesis in a metabolic syndrome model: the role of vitamin a metabolism in the gut-testis axis. Gut 71, 78–87. doi: 10.1136/gutjnl-2020-323347
Zhang, C., Xiong, B., Chen, L., Ge, W., Yin, S., Feng, Y., et al. (2021). Rescue of male fertility following faecal microbiota transplantation from alginate oligosaccharide-dosed mice. Gut 70, 2213–2215. doi: 10.1136/gutjnl-2020-323593
Zhang, K. X., Zhu, Y., Song, S. X., Bu, Q. Y., You, X. Y., Zou, H., et al. (2024). Ginsenoside Rb1, compound K and 20 (S) -Protopanaxadiol attenuate high-fat diet-induced hyperlipidemia in rats via modulation of gut microbiota and bile acid metabolism. Molecules 29:1108. doi: 10.3390/molecules29051108
Zhao, Z. H., Xin, F. Z., Xue, Y., Hu, Z., Han, Y., Ma, F., et al. (2019). Indole-3-propionic acid inhibits gut dysbiosis and endotoxin leakage to attenuate steatohepatitis in rats. Exp. Mol. Med. 51, 1–14. doi: 10.1038/s12276-019-0304-5
Zhou, Y., Wei, Z., Tan, J., Sun, H., Jiang, H., Gao, Y., et al. (2023). Alginate oligosaccharide extends the service lifespan by improving the sperm metabolome and gut microbiota in an aging Duroc boars model. Front. Cell. Infect. Microbiol. 13:1308484. doi: 10.3389/fcimb.2023.1308484
Keywords: gut microbiota, spermatogenesis, sperm motility, testis-gut microbiota axis, dual impact, mechanism, intervention measures
Citation: Chen W, Zou H, Xu H, Cao R, Zhang H, Zhang Y and Zhao J (2024) The potential influence and intervention measures of gut microbiota on sperm: it is time to focus on testis-gut microbiota axis. Front. Microbiol. 15:1478082. doi: 10.3389/fmicb.2024.1478082
Edited by:
Lifeng Zhu, Nanjing University of Chinese Medicine, ChinaReviewed by:
Peihai Zhang, Chengdu University of Traditional Chinese Medicine, ChinaWei Wei, China Academy of Chinese Medical Sciences, China
Baofang Jin, Southeast University, China
Copyright © 2024 Chen, Zou, Xu, Cao, Zhang, Zhang and Zhao. This is an open-access article distributed under the terms of the Creative Commons Attribution License (CC BY). The use, distribution or reproduction in other forums is permitted, provided the original author(s) and the copyright owner(s) are credited and that the original publication in this journal is cited, in accordance with accepted academic practice. No use, distribution or reproduction is permitted which does not comply with these terms.
*Correspondence: Jiayou Zhao, emhhb2ppYXlvdTUyMEAxMjYuY29t
†These authors have contributed equally to this work