- 1Microbial Chemistry, Department of Chemistry-Ångström Laboratory, Uppsala University, Uppsala, Sweden
- 2Department of Chemical Engineering, Loughborough University, Loughborough, United Kingdom
Thermophilic acetogens are gaining recognition as potent microbial cell factories, leveraging their unique metabolic capabilities to drive the development of sustainable biotechnological processes. These microorganisms, thriving at elevated temperatures, exhibit robust carbon fixation abilities via the linear Wood-Ljungdahl pathway to efficiently convert C1 substrates, including syngas (CO, CO2 and H2) from industrial waste gasses, into acetate and biomass via the central metabolite acetyl-CoA. This review summarizes recent advancements in metabolic engineering and synthetic biology efforts that have expanded the range of products derived from thermophilic acetogens after briefly discussing their autotrophic metabolic diversity. These discussions highlight their potential in the sustainable bioproduction of industrially relevant compounds. We further review the remaining challenges for implementing efficient and complex strain engineering strategies in thermophilic acetogens, significantly limiting their use in an industrial context.
1 Introduction
As anthropocentric industrial activities accelerate the climate change, sustainable alternatives for manufacturing essential chemical commodities are urgently needed. Microbial biotechnology processes stand out as promising solutions due to their inherent robustness, adaptability, and less energy-intensive nature as compared to traditional chemical synthesis and fossil fuel-based methods (Ko et al., 2020; Cho et al., 2022). The development of reliable and efficient genetic tools, supporting various metabolic engineering strategies to expand and rewire microbial metabolic networks, has also allowed to further establish microbial cell factories as key production platforms. Acetogenic bacteria are becoming increasingly relevant in the current climate crisis context due to their autotrophic ability to utilize CO2 as their sole carbon source; thus, holding great promise to mitigate global warming by abating greenhouse gas emissions. In particular, these bacteria can assimilate a combination of H2, CO2, and CO (i.e., syngas) (Liew et al., 2016) released by diverse industrial processes, hence offering the possibility to utilize industrial waste gas streams. Thus, acetogens can significantly contribute to industrial carbon capture and utilization efforts, alongside other non-biological strategies (McLaughlin et al., 2023; Yusuf and Ibrahim, 2023).
Although acetogens are very diverse in their metabolic capabilities, these anaerobic Gram-positive bacteria all rely on the Wood-Ljungdahl pathway (WLP) (Drake et al., 2008; Ragsdale, 2008), also known as the reductive acetyl-CoA pathway for carbon assimilation. They use the WLP to convert CO2 into the central metabolite acetyl-CoA, which is then channeled into both biomass and acetate formation. Operating at the thermodynamic limit of life (Schuchmann and Müller, 2014), acetogens have evolved intricate energy-conserving mechanisms to thrive autotrophically with the WLP. While significant progresses in terms of genetic engineering efforts and understanding of autotrophic processes have been achieved for mesophilic acetogens, their thermophilic counterparts remain largely understudied. However, thermophilic acetogens warrant a greater attention due to their unique advantages for large-scale cultivation and industrial bioprocesses, such as high turnover rates and reduced gas cooling requirements and contamination risks in bioreactors.
Despite their attractive characteristics, genetic and metabolic engineering of thermophilic acetogens for commodity bioproduction presents considerable challenges. Their metabolism is inherently constrained and the lack of efficient genetic tools complicates strain engineering. This review will discuss progresses and challenges of engineering thermophilic acetogens as microbial cell factories.
2 Thermophilic acetogenic isolates and their metabolism
2.1 Thermophilic acetogenic metabolism
Acetogenesis can be defined as the ability to convert two molecules of CO2 into acetyl-CoA through the WLP (Schuchmann and Müller, 2016). Although the WLP is present in methanogens, this review focuses on homoacetogens which utilize this metabolic pathway for energy conservation. In these organisms, the WLP, described in details elsewhere (Ragsdale, 2008), consists of two converging branches, the methyl and carbonyl branches (Figure 1), which provide the methyl and carbonyl groups, respectively, for acetyl-CoA formation. This pathway requires an essential enzyme, the CO dehydrogenase/acetyl-CoA synthase (CODH-ACS) to form acetyl-CoA that is needed for biomass formation. Additionally, acetyl-CoA is converted into acetate, releasing one molecule of ATP. As formate conversion in the methyl branch requires one molecule of ATP, the net gain of ATP in the WLP is zero, placing acetogenic metabolism at the thermodynamic limit of life. Under autotrophy, the WLP is vital for carbon fixation, but this pathway is also active during heterotrophic growth as it participates into energy conservation and acts as a crucial electron sink.
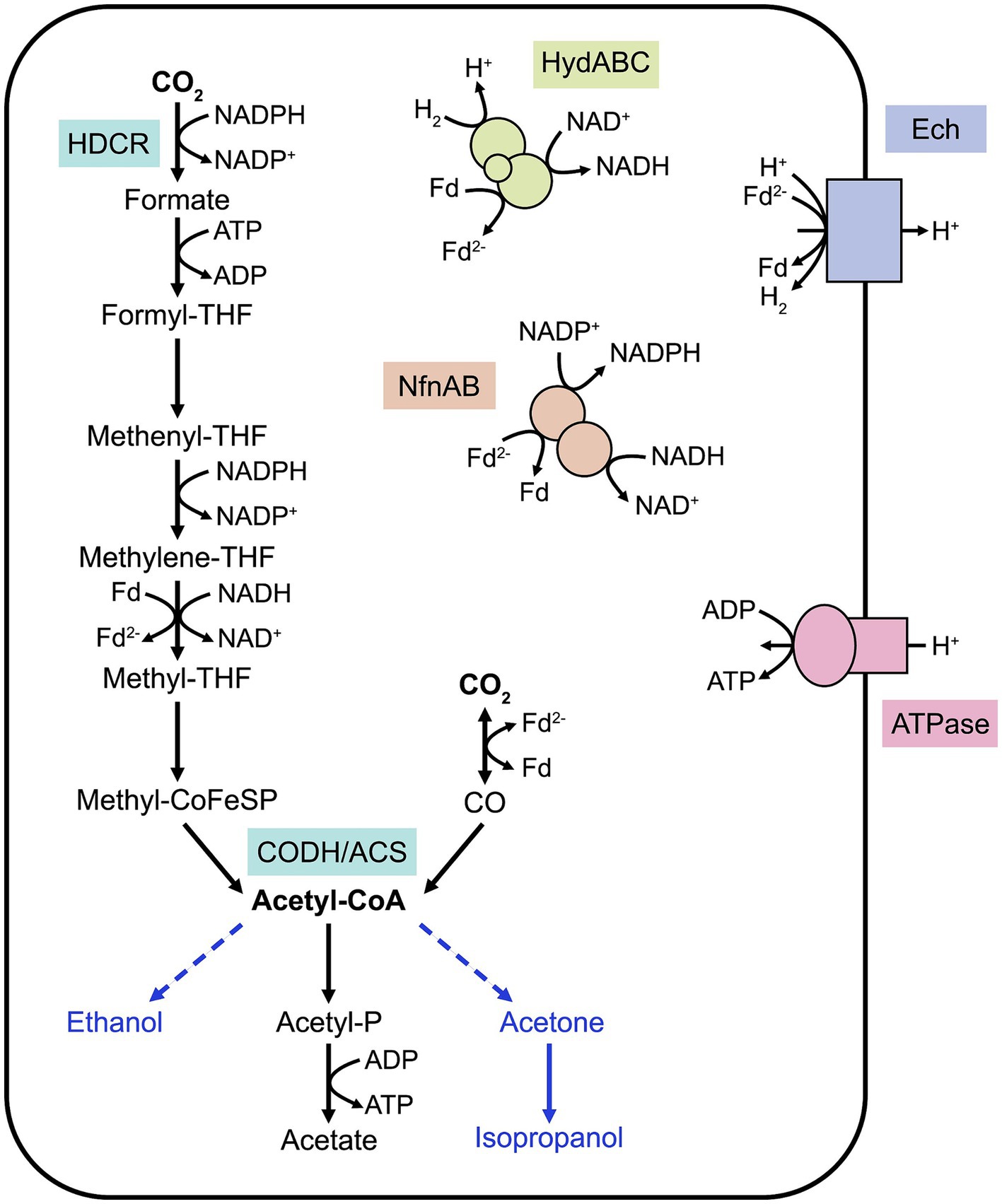
Figure 1. Simplified schematic of the Wood-Ljungdahl pathway in M. thermoacetica. The converging methyl and carbonyl branches allow CO2 conversion into acetyl-CoA, shuttled into biomass or acetate formation. Key enzymes of the WLP are represented. Known energy-conserving mechanisms are illustrated. Heterologous pathways for compound bioproduction are represented with blue arrows. Note that cofactor stoichiometry is not included in this figure and that cofactors and energy-conserving mechanisms differ between acetogens. HDCR, H2-dependent carbon dioxide reductase; CODH/ACS, CO dehydrogenase/acetyl-CoA synthase; THF, tetrahydrofolate; Fd, ferredoxin; HydABC, electron-bifurcating hydrogenase; NfnAB, electron-bifurcating transhydrogenase.
Energy-conserving mechanisms have evolved to regenerate cofactors and are highly species-specific, as reviewed elsewhere (Basen and Müller, 2017). Briefly, most thermophilic acetogens rely on a membrane-bound energy-converting hydrogenase (Ech) to create a proton gradient across the membrane, which is utilized for ATP synthesis by an F1F0 ATPase (Figure 1). This proton translocation is also coupled to the oxidation of reduced ferredoxin, provided by the electron-bifurcating hydrogenase HydABC (Wang et al., 2013). NADPH, involved in the carbonyl branch of the WLP, is provided by the electron-bifurcating transhydrogenase NfnAB, with the concomitant conversion of reduced ferredoxin and NADH. Some of these processes and their stoichiometry remain unclear in thermophilic acetogens. In addition, acetogens can utilize a variety of electron carriers, enabling them to conserve energy by metabolizing diverse substrates such as pentoses, alcohols or organic acids (Basen and Müller, 2017). This metabolic flexibility is believed to be advantageous in their natural environments, where they compete with methanogens and sulfate-reducing bacteria. Acetogenic thermophiles important for industrial biotechnology applications are described in more details in the following sections.
2.2 Moorella sp.
To date, only a few acetogenic thermophiles have been isolated (Table 1). Most of these acetogens belong to the Moorella genus, with the first species, M. thermoacetica, isolated in the 1940s (Fontaine et al., 1942). M. thermoacetica has become the model Moorella species, and was key to describing and characterizing the WLP. Additional Moorella strains have since been isolated (Jia et al., 2023), and continuous discovery of new species highlights their diversity. Notably, the taxonomy of Moorella species still remains uncertain, as previously distinct species have recently been proposed to be the same species based on sequencing data (Redl et al., 2020).
Moorella sp. grow optimally at 55–60°C (Table 1) although pH and salinity parameters differ between species. They catabolize a variety of substrates, with several species reported to utilize methanol (Santaella et al., 2023). Both M. thermoacetica and M. thermoautotrophica are capable of microbial electrosynthesis (Yu et al., 2017; Chen et al., 2018; Ha et al., 2022), converting electricity and CO2 into high-value organic acids. This ability has, for example, been harnessed for acetate formation by M. thermoautotrophica, with the supply of electricity through metal electrodes, and further improved by embedding cells with carbon nanoparticules (Yu et al., 2017) and increasing cell permeability (Chen et al., 2018).
In addition to the energy-conserving mechanisms described above, the presence of quinones and cytochromes in Moorella sp. is unique among acetogens (Rosenbaum and Müller, 2021); however their roles remain unclear. Although a possible function as electron carriers in the electron transport chain, for example during lactate metabolism, has been proposed (Rosenbaum and Müller, 2021, 2023; Rosenbaum et al., 2021), further evidence is required to elucidate the role and importance of quinones and cytochromes for energy conservation in Moorella sp.
2.3 Thermoanaerobacter sp.
Among Thermoanaerobacter species, only T. kivui has been reported to fix CO2 through the WLP as other Thermoanaerobacter sp. do not contain CODH/ACS and hydrogen-dependent carbon dioxide reductase (HDCR) enzymes, essential to the WLP (Basen and Müller, 2017). Evolutionary emergence of acetogenic capabilities in this species remains unclear. In contrast to other thermophilic acetogens, T. kivui has a fast doubling time (~2 h) under H2 + CO2 conditions (Weghoff and Müller, 2016) and is naturally competent (Basen et al., 2018), simplifying laboratory cultivation and DNA uptake, which makes it particularly promising for industrial applications. It has been adapted for growth on CO and syngas (Weghoff and Müller, 2016), later shown to be supported by the presence of cooS, coding for a monofunctional CO dehydrogenase and essential for growth on CO (Jain et al., 2022). Recently, it has been reported that T. kivui can utilize mannitol in a CO2-dependent manner through expression of a mannitol-1-phosphate dehydrogenase (Moon et al., 2019, 2020).
While many unknowns remain regarding energy-conserving mechanisms and electron carriers involved in the WLP in T. kivui, genome analysis suggests that this organism relies on a proton (H+) gradient created by the Ech hydrogenase to drive ATP synthesis, similar to Moorella sp. (Hess et al., 2014). Electron carriers necessary for several enzymes involved in the WLP and energy conservation have been elucidated in cell-free extracts (Katsyv et al., 2021a), identifying, for example, NADP+-specificity of the methylene-THF dehydrogenase involved in the carbonyl branch of the WLP. However, the identity of electron carriers for other enzymes such as the electron-bifurcating hydrogenase HydABC remains unclear. In addition, the structure of the hydrogen-dependent carbon dioxide reductase (HDCR), which converts H2 and CO2 into formate in a high-turnover reaction, has now been elucidated with cryo-electron microscopy (Dietrich et al., 2022), providing a strong fundamental knowledge for this key enzyme. Interestingly, the formation of long HDCR filaments at the plasma membrane in T. kivui cells significantly enhanced enzymatic activity. Unsurprisingly, a Δhdcr mutant was unable to grow autotrophically without formate supplementation in the medium (Jain et al., 2020). However, this phenotype was also observed under heterotrophy, highlighting the importance of the HDCR enzyme and the WLP for both autotrophy and heterotrophy.
2.4 Thermacetogenium sp.
Although Thermacetogenium phaeum is able to produce acetyl-CoA from CO2 with the WLP (Basen and Müller, 2017), this species exhibits poor growth in axenic cultures, reaching low maximal cell densities (Keller et al., 2019a). Instead, it preferentially grows in syntrophic cultures with the methanogen Methanothermobacter thermautotrophicus. In this syntrophic scenario, T. phaeum reverts the WLP for acetate consumption, a unique property not observed in other acetogens (Hattori et al., 2005). Genome analysis suggests that T. phaeum’s energy-conserving mechanisms and autotrophic metabolism differ significantly from other acetogens to accommodate for its bidirectional WLP (Oehler et al., 2012). In particular, ATP synthesis under both acetate formation and consumption raises several thermodynamic questions that are yet to be elucidated. A periplasmically oriented and quinone-dependent formate dehydrogenase has been proposed to allow WLP reversibility in T. phaeum (Keller et al., 2019a) although additional work is needed to elucidate energy-conserving mechanisms during both metabolic processes. Recently, the presence of pathways for methanol and ethanol degradation in T. phaeum have been proposed from proteomics and enzymatic activities, suggesting similar stoichiometries to the mesophilic acetogen Acetobacterium woodii (Keller et al., 2019b). This work also identified bacterial microcompartments involved in ethanolamine utilization in this thermophile. While progresses have been made toward understanding T. phauem’s metabolism, much more work is needed to uncover how this acetogen can revert the WLP, which has potential for industrial acetate valorization.
2.5 Aceticella sp.
The new thermophilic acetogenic species Aceticella autotrophica was recently isolated from a Russian terrestrial hot spring (Frolov et al., 2023). This species is the first obligate autotroph identified among acetogens and is unable to grow under heterotrophic conditions. Comparative genomics suggested that this strict autotrophic requirement results from the loss of genes involved in carbohydrate metabolism and sugar transport. Interestingly, while the species belongs to the Thermoanaerobacterales order, it has evolved unique features contrasting to its evolutionary counterparts. It shares the most similarity with T. kivui and contains a WLP gene cluster. Much more work is needed to characterize this new acetogen.
2.6 Archaeoglobus sp.
In addition to the aforementioned bacterial species, several mesophilic archaea (Loh et al., 2020; Schöne et al., 2022) are also acetogens. Archaeoglobus fulgidus is, to date, the only thermophilic archaeon reported to grow as an acetogen (Henstra et al., 2007). This species is primarily studied for its sulfate-reducing ability and piezophilic lifestyle (Oliver et al., 2020) but has been adapted to grow on CO (Henstra et al., 2007). CO adaptation eliminated A. fulgidus’s long lag-phase and was later investigated through transcriptomics analysis upon growth on CO (Hocking et al., 2014, 2015). This work proposed a scheme for energy conservation during the acetogenic growth of A. fulgidus by a F420H2:quinone oxidoreductase complex. Other Archaeoglobus species were explored but could not grow as acetogens, although key genes required for acetogenesis were present. Further work is thus needed to understand why A. fulgidus has the unique ability to grow as an acetogen and better-characterize its associated mechanisms.
3 Engineering thermophilic acetogens as microbial cell factories
3.1 Development and applications of genetic tools
Native acetogenic properties are extremely valuable for climate mitigation by fixing high CO2 concentrations in sustainable bioprocesses. However, to further expand their potential, reliable and efficient genetic tools must be developed to enable targeted strain engineering efforts that are crucial to rewire the metabolism for maximized compound bioproduction and heterologous pathway expression. Currently, genetic methods available for thermophilic acetogens are extremely limited. Successful genetic engineering has only been reported in M. thermoacetica and T. kivui although other acetogenic thermophiles have unique valuable properties.
In M. thermoacetica, genetic insertions have been performed using uracil/5-fluoroorotic acid (5-FOA) counterselection through deletion and reinsertion of pyrF (Iwasaki et al., 2013; Kita et al., 2013; Rahayu et al., 2017; Kato et al., 2021, 2024), encoding an orotodine 5′-phosphate decarboxylase. The ΔpyrF mutant becomes auxotrophic for uracil and resistant for 5-FOA, allowing transformant selection without antibiotic pressure. Reintroduction of pyrF, concurrently with the gene(s) of interest, restores uracil biosynthesis in the resulting mutant strain. This strategy has been applied to establish ethanol (Rahayu et al., 2017), acetone (Kato et al., 2021) and isopropanol (Kato et al., 2024) production in M. thermoacetica through expression of an aldehyde dehydrogenase, an acetone operon (consisting of a CoA transferase, a thiolase and an acetoacetate decarboxylase) and a secondary alchohol dehydrogenase, respectively. As these pathways branch from acetyl-CoA, disruption of acetate formation through deletion of the phosphotransacetylases PduL1 and PduL2 has been beneficial to redirect carbon flux toward compound biosynthesis (Kato et al., 2021). In addition to uracil auxotrophy, antibiotic selection by expressing a thermostable kanamycin resistant gene has also been reported (Iwasaki et al., 2013). This strategy has allowed the development of a self-replicating plasmid, harboring the pRKU1 replicon from Thermotoga maritima (Bourgade et al., 2022) and subsequently applied for ethanol production in proof-of-concept experiments. This self-replicating plasmid offers the possibility to rapidly and transiently test genetic constructs, ideal for, for example, CRISPR-Cas tools. Furthermore, to date, only one promoter, the strong constitutive promoter for glyceraldehyde-3-phosphate dehydrogenase (Kita et al., 2013) has been used for heterologous gene expression in M. thermoacetica. Thus, additional promoters are needed to expand the genetic toolbox to tailor target gene expression in this industrially important host.
In naturally competent T. kivui, a similar uracil/5-FOA counterselection technique has been adapted by deleting pyrE, encoding an orotate phosphoribosyltransferase involved in uracil biosynthesis (Basen et al., 2018). This method has primarily been used in fundamental studies to explore enzymatic functions of metabolic relevance. For example, Δhdcr, ΔcooS and ΔmtlD mutants enabled to investigate formate formation, CO metabolism and mannitol consumption, respectively in T. kivui (Moon et al., 2019; Jain et al., 2020, 2022). pyrE-mediated genetic insertions have also allowed overexpression of the native pfor1 (Katsyv et al., 2021b) and mtlD (Moon et al., 2019) genes, coding for a pyruvate:ferredoxin oxidoreductase and a mannitol-1-phosphate dehydrogenase, respectively, for protein purification from T. kivui cells. However, this method has not been reported for insertion and expression of heterologous genes yet but should allow successful pathway implementation in T. kivui in a similar manner to M. thermoacetica. A recent study successfully expressed the thermostable fluorescent reporter pFAST from a self-replicating plasmid, establishing a reporter assay for genetic part testing (Hocq et al., 2023). This tool was applied for promoter characterization to identify new strong constitutive promoters, such as the novel promoter pPtaTkv for target gene expression in T. kivui. Interestingly, promoters from mesophilic acetogens were also functional in T. kivui, suggesting genetic part transferability. The authors isolated more stable versions of the replicon to promote plasmid propagation through adaptive laboratory evolution under antibiotic selection, significantly expanding T. kivui genetic toolbox.
Genetic tools are currently not available for other thermophilic acetogens but the development of such tools would greatly expand their industrial potential. It is worth noting that beyond microbial cell factories, thermophilic acetogens can also offer promising thermostable enzymes of industrial interest. For example, the recently characterized T. kivui HDCR (Dietrich et al., 2022) stands out as a promising biocatalyst for H2 storage. An alternative approach to using whole-cell biocatalysis has recently been reported to store H2 into formate (Schwarz and Müller, 2020). Additionally, the pyruvate:ferredoxin oxidoreductase can also be purified directly from T. kivui and is a promising auxiliary enzyme for enzymatic assays requiring reduced ferredoxin (Fd2−) difficult to provide otherwise (Katsyv et al., 2021b). Extensive research efforts on A. fulgidus have focused on characterizing various enzymes such as Argonaute (Manakova et al., 2024) or ferritin (Palombarini et al., 2021), highlighting its potential for enzymatic and therapeutic applications.
3.2 Engineering challenges
As described above, only two thermophilic acetogens have been genetically modified to date. While these efforts mark a significant step toward unlocking their potential, more complex genetic tools are needed to support extensive strain engineering of these thermophiles. Additionally, developing genetic methods for the other isolated thermophilic acetogens is crucial to accelerate their industrial potential for sustainable bioprocesses. However, several challenges remain for efficient genetic engineering of thermophilic acetogens. Successful transformation is currently hindered by multiple factors, such as difficulty of transformant selection and DNA entry into the host. Growth on plates has been reported as problematic for several acetogens (Sanford and Woolston, 2022). For instance, M. thermoacetica seems unable to grow on plates under antibiotic selective pressure (Bourgade et al., 2022). Instead, a rolling strategy in Hungate tubes has been used (Kita et al., 2013) which could result in high proportion of false transformants. In contrast, T. kivui is well adapted for growth on plates and can even tolerate brief exposure to oxygen at room temperature (Basen et al., 2018). The ability of other thermophilic acetogens to grow on plates is unknown but may restrict isolating positive transformants.
Many prokaryotes employ restriction-modification (RM) systems to protect themselves against invading foreign DNA (Vasu and Nagaraja, 2013). These systems recognize specific DNA sequences with associated methylation patterns to induce DNA cleavage; thereby, preventing foreign DNA entry into the host. Many acetogens possess these RM systems (Bourgade et al., 2021), which must be circumvented for a successful DNA entry into these hosts. For M. thermoacetica, bypassing of native RM systems has been reported by expressing three native genes encoding RM systems in an E. coli strain to protect cargo DNA prior to transformation into M. thermoacetica (Kita et al., 2013; Jia et al., 2023). This method may be applied for DNA insertion into other Moorella species or thermophilic acetogens.
Although genomic integration tends to enhance strain stability, it is often time-consuming and unsuitable for rapid construct testing or transient expression for CRISPR-based methods. Instead, self-replicating plasmids, able to propagate independently of chromosomal replication, are valuable genetic tools. However, these plasmids require compatible replicons for plasmid replication with the host’s machinery, often difficult to identify. Recently, a self-replicating shuttle vector was developed for M. thermoacetica using the pRKU1 replicon from Thermotoga maritima (Bourgade et al., 2022), which may be compatible with other closely related Moorella sp. However, additional work is needed to better understand this plasmid behavior in M. thermoacetica. T. kivui has previously been transformed with pMU131 replicon from Thermoanaerobacterium saccharolyticum (Basen et al., 2018). This replicon was later shown to be unstable at higher temperatures and subsequently improved through adapted laboratory evolution to increase its stability (Hocq et al., 2023).
In addition to replicons, genetic parts that are essential for achieving tuneable gene expression levels in thermophilic acetogens are poorly characterized. To date, only one promoter, from the glyceraldehyde-3-phosphate dehydrogenase has been used in M. thermoacetica to drive strong constitutive expression of heterologous genes (Kita et al., 2013). More promoters of varying strengths are needed to precisely control heterologous expression and metabolic output. Promoter characterization may prove difficult under thermophilic and anaerobic conditions, which render many fluorescent reporters non-functional. However, a reporter assay was recently developed for T. kivui with the O2-independent FAST system, allowing promoter characterization at high temperatures (Hocq et al., 2023) and is possibly compatible with other thermophilic acetogens. Ribosome-binding sites have yet to be characterized in these organisms but would be useful for achieving predictable translation levels.
Thermophily, while advantageous for metabolic efficiency and industrial applications, complicates genetic engineering work by limiting the pool of candidate enzymes that are functional at high temperatures. A thermostable kanR gene from Streptococcus faecalis has allowed kanamycin selection in M. thermoacetica (Iwasaki et al., 2013). Similarly, a thermostable acetone operon was engineered for M. thermoacetica by selecting candidate enzymes from other thermophilic prokaryotes (Kato et al., 2021). As exemplified by FAST (Hocq et al., 2023), exploring enzyme thermostability is crucial when working with thermophilic acetogens.
Finally, acetogenic metabolism is highly constrained by energy limitations and cofactor availability. These constraints significantly limit metabolic engineering possibilities, preventing expression of ATP-demanding pathways in these hosts. In particular, most pathways successfully implemented in acetogens stem from acetyl-CoA, therefore competing with ATP-yielding acetate formation. However, increasing target compound biosynthesis by abolishing acetate formation poses a problem for ATP synthesis during autotrophy and is often unviable for the host. For example, a M. thermoacetica Δpdul1Δpdul2::aldh strain, producing ethanol instead of acetate, was unable to grow autotrophically on H2:CO2 (Takemura et al., 2021). Instead, ethanol was produced autotrophically with Δpdul2::aldh strain under CO supplementation, allowing ATP synthesis by decreased acetate formation using alternative routes. Another engineered M. thermoacetica strain required an additional electron acceptor to produce acetone autotrophically (Takemura et al., 2023). This work identified dimethyl sulfoxide as the strongest electron acceptor by enhancing ATP synthesis under H2:CO2 conditions.
4 Conclusion and outlook
Acetogens can fix CO2 into acetyl-CoA with the WLP, making them promising chassis organisms for large-scale biological CO2 fixation and compound bioproduction—a pivotal step toward mitigating climate change. Thermophilic acetogens offer additional advantages over their mesophilic counterparts by, for example, reducing gas cooling requirements and contamination risks in industrial bioprocesses. Several species with unique properties have now been isolated at temperatures above 55°C. However, most of them remain understudied, potentially due to the difficulty of cultivating and studying them under standard laboratory conditions. Consequently, significant knowledge gaps regarding their metabolism and physiology, in particular energy-conserving mechanisms remain. However, recent research efforts have started elucidating their metabolic processes, primarily in M. thermoacetica and T. kivui. Further work is, therefore, needed to fully understand their metabolism in order to design appropriate metabolic engineering strategies for industrial applications.
Moreover, M. thermoacetica and T. kivui have recently been engineered for heterologous compound biosynthesis and fundamental studies, respectively, paving the way for thermophilic acetogenic microbial cell factories. However, the genetic toolkit currently available for manipulating acetogens is limited, which further prevents complex strain engineering efforts. In particular, characterized genetic parts and thermostable enzymes are missing but are key elements for metabolic engineering. Significant genetic work is therefore needed to establish thermophilic acetogens as robust microbial cell factories for simultaneous CO2 fixation and compound biosynthesis.
Author contributions
BB: Writing – review & editing, Writing – original draft, Visualization, Conceptualization. MAI: Writing – review & editing, Project administration, Funding acquisition, Conceptualization.
Funding
The author(s) declare financial support was received for the research, authorship, and/or publication of this article. BB acknowledges funding from Formas-A Swedish Research Council for Sustainable Development (project no. 2021-01669). MAI acknowledges support from the EPSRC/BBSRC NIBB Environmental Biotechnology Network (EBNet) PoC grant (project no. POC202311).
Conflict of interest
The authors declare that the research was conducted in the absence of any commercial or financial relationships that could be construed as a potential conflict of interest.
Publisher’s note
All claims expressed in this article are solely those of the authors and do not necessarily represent those of their affiliated organizations, or those of the publisher, the editors and the reviewers. Any product that may be evaluated in this article, or claim that may be made by its manufacturer, is not guaranteed or endorsed by the publisher.
References
Alves, J. I., van Gelder, A. H., Alves, M. M., Sousa, D. Z., and Plugge, C. M. (2013). Moorella stamsii sp. nov., a new anaerobic thermophilic hydrogenogenic carboxydotroph isolated from digester sludge. Int. J. Syst. Evol. Microbiol. 63, 4072–4076. doi: 10.1099/ijs.0.050369-0
Balk, M., Van Gelder, T., Weelink, S. A., and Stams, A. J. M. (2008). (per)chlorate reduction by the thermophilic bacterium Moorella perchloratireducens sp. nov., isolated from underground gas storage. Appl. Environ. Microbiol. 74, 403–409. doi: 10.1128/AEM.01743-07
Balk, M., Weijma, J., Friedrich, M. W., and Stams, A. J. M. (2003). Methanol utilization by a novel thermophilic homoacetogenic bacterium, Moorella mulderi sp. nov., isolated from a bioreactor. Arch. Microbiol. 179, 315–320. doi: 10.1007/s00203-003-0523-x
Basen, M., Geiger, I., and Henke, L. (2018). A genetic system for the thermophilic acetogenic bacterium Thermoanaerobacter kivui. Appl. Environ. Microbiol. 84, 1–11. doi: 10.1128/AEM.02210-17
Basen, M., and Müller, V. (2017). “Hot” acetogenesis. Extremophiles 21, 15–26. doi: 10.1007/s00792-016-0873-3
Bourgade, B., Millard, J., Humphreys, C. M., Minton, N. P., and Islam, M. A. (2022). Enabling ethanologenesis in Moorella thermoacetica through construction of a replicating shuttle vector. Fermentation 8:585. doi: 10.3390/fermentation8110585
Bourgade, B., Minton, N. P., and Islam, M. A. (2021). Genetic and metabolic engineering challenges of C1-gas fermenting acetogenic chassis organisms. FEMS Microbiol. Rev. 45, 1–20. doi: 10.1093/femsre/fuab008
Chen, S., Fang, Y., Jing, X., Luo, H., Chen, J., and Zhou, S. (2018). Enhanced electrosynthesis performance of Moorella thermoautotrophica by improving cell permeability. Bioelectrochemistry 121, 151–159. doi: 10.1016/j.bioelechem.2018.02.003
Cho, J. S., Kim, G. B., Eun, H., Moon, C. W., and Lee, S. Y. (2022). Designing microbial cell factories for the production of chemicals. JACS Au 2, 1781–1799. doi: 10.1021/jacsau.2c00344
Dietrich, H. M., Righetto, R. D., Kumar, A., Wietrzynski, W., Trischler, R., Schuller, S. K., et al. (2022). Membrane-anchored HDCR nanowires drive hydrogen-powered CO2 fixation. Nature 607, 823–830. doi: 10.1038/s41586-022-04971-z
Drake, H. L., Gößner, A. S., and Daniel, S. L. (2008). Old acetogens, new light. Ann. N. Y. Acad. Sci. 1125, 100–128. doi: 10.1196/annals.1419.016
Fontaine, F. E., Peterson, W. H., McCoy, E., Johnson, M. J., and Ritter, G. J. (1942). A new type of glucose fermentation by Clostridium thermoaceticum. J. Bacteriol. 43, 701–715. doi: 10.1128/jb.43.6.701-715.1942
Frolov, E. N., Elcheninov, A. G., Gololobova, A. V., Toshchakov, S. V., Novikov, A. A., Lebedinsky, A. V., et al. (2023). Obligate autotrophy at the thermodynamic limit of life in a new acetogenic bacterium. Front. Microbiol. 14:1185739. doi: 10.3389/fmicb.2023.1185739
Ha, B. N., Pham, D. M., Masuda, D., Kasai, T., and Katayama, A. (2022). Humin-promoted microbial electrosynthesis of acetate from CO2 by Moorella thermoacetica. Biotechnol. Bioeng. 119, 3487–3496. doi: 10.1002/bit.28238
Hattori, S., Galushko, A. S., Kamagata, Y., and Schink, B. (2005). Operation of the CO dehydrogenase/acetyl coenzyme a pathway in both acetate oxidation and acetate formation by the syntrophically acetate-oxidizing bacterium Thermacetogenium phaeum. J. Bacteriol. 187, 3471–3476. doi: 10.1128/JB.187.10.3471-3476.2005
Hattori, S., Kamagata, Y., Hanada, S., and Shoun, H. (2000). Thermacetogenium phaeum gen. Nov., sp. nov., a strictly anaerobic, thermophilic, syntrophic acetate-oxidizing bacterium. Int. J. Syst. Evol. Microbiol. 50, 1601–1609. doi: 10.1099/00207713-50-4-1601
Henstra, A. M., Dijkema, C., and Stams, A. J. M. (2007). Archaeoglobus fulgidus couples CO oxidation to sulfate reduction and acetogenesis with transient formate accumulation. Environ. Microbiol. 9, 1836–1841. doi: 10.1111/j.1462-2920.2007.01306.x
Hess, V., Poehlein, A., Weghoff, M. C., Daniel, R., and Müller, V. (2014). A genome-guided analysis of energy conservation in the thermophilic, cytochrome-free acetogenic bacterium Thermoanaerobacter kivui. BMC Genomics 15, 1–14. doi: 10.1186/1471-2164-15-1139
Hocking, W. P., Roalkvam, I., Magnussen, C., Stokke, R., and Steen, I. H. (2015). Assessment of the carbon monoxide metabolism of the hyperthermophilic sulfate-reducing archaeon Archaeoglobus fulgidus VC-16 by comparative transcriptome analyses. Archaea 2015:235384. doi: 10.1155/2015/235384
Hocking, W. P., Stokke, R., Roalkvam, I., and Steen, I. H. (2014). Identification of key components in the energy metabolism of the hyperthermophilic sulfate-reducing archaeon Archaeoglobus fulgidus by transcriptome analyses. Front. Microbiol. 5, 1–20. doi: 10.3389/fmicb.2014.00095
Hocq, R., Bottone, S., Gautier, A., and Pflügl, S. (2023). A fluorescent reporter system for anaerobic thermophiles. Front. Bioeng. Biotechnol. 11:1226889. doi: 10.3389/fbioe.2023.1226889
Iwasaki, Y., Kita, A., Sakai, S., Takaoka, K., Yano, S., Tajima, T., et al. (2013). Engineering of a functional thermostable kanamycin resistance marker for use in Moorella thermoacetica ATCC39073. FEMS Microbiol. Lett. 343, 8–12. doi: 10.1111/1574-6968.12113
Jain, S., Dietrich, H. M., Müller, V., and Basen, M. (2020). Formate is required for growth of the thermophilic acetogenic bacterium Thermoanaerobacter kivui lacking hydrogen-dependent carbon dioxide reductase (HDCR). Front. Microbiol. 11:59. doi: 10.3389/fmicb.2020.00059
Jain, S., Katsyv, A., Basen, M., and Müller, V. (2022). The monofunctional CO dehydrogenase CooS is essential for growth of Thermoanaerobacter kivui on carbon monoxide. Extremophiles 26, 4–12. doi: 10.1007/s00792-021-01251-y
Jia, D., Deng, W., Hu, P., Jiang, W., and Gu, Y. (2023). Thermophilic Moorella thermoacetica as a platform microorganism for C1 gas utilization: physiology, engineering, and applications. Bioresour. Bioprocess. 10:61. doi: 10.1186/s40643-023-00682-z
Kato, J., Matsuo, T., Takemura, K., Kato, S., Fujii, T., Wada, K., et al. (2024). Isopropanol production via the thermophilic bioconversion of sugars and syngas using metabolically engineered Moorella thermoacetica. Biotechnol. Biofuels Bioprod. 17, 1–12. doi: 10.1186/s13068-024-02460-1
Kato, J., Takemura, K., Kato, S., Fujii, T., Wada, K., Iwasaki, Y., et al. (2021). Metabolic engineering of Moorella thermoacetica for thermophilic bioconversion of gaseous substrates to a volatile chemical. AMB Express 11:59. doi: 10.1186/s13568-021-01220-w
Katsyv, A., Jain, S., Basen, M., and Müller, V. (2021a). Electron carriers involved in autotrophic and heterotrophic acetogenesis in the thermophilic bacterium Thermoanaerobacter kivui. Extremophiles 25, 513–526. doi: 10.1007/s00792-021-01247-8
Katsyv, A., Schoelmerich, M. C., Basen, M., and Müller, V. (2021b). The pyruvate:ferredoxin oxidoreductase of the thermophilic acetogen, Thermoanaerobacter kivui. FEBS Open Bio 11, 1332–1342. doi: 10.1002/2211-5463.13136
Keller, A., Schink, B., and Müller, N. (2019a). Energy-conserving enzyme systems active during syntrophic acetate oxidation in the thermophilic bacterium Thermacetogenium phaeum. Front. Microbiol. 10:2785. doi: 10.3389/fmicb.2019.02785
Keller, A., Schink, B., and Müller, N. (2019b). Alternative pathways of acetogenic ethanol and methanol degradation in the thermophilic anaerobe Thermacetogenium phaeum. Front. Microbiol. 10:423. doi: 10.3389/fmicb.2019.00423
Kita, A., Iwasaki, Y., Sakai, S., Okuto, S., Takaoka, K., Suzuki, T., et al. (2013). Development of genetic transformation and heterologous expression system in carboxydotrophic thermophilic acetogen Moorella thermoacetica. J. Biosci. Bioeng. 115, 347–352. doi: 10.1016/j.jbiosc.2012.10.013
Ko, Y.-S., Kim, J. W., Lee, J. A., Han, T., Kim, G. B., Park, J. E., et al. (2020). Tools and strategies of systems metabolic engineering for the development of microbial cell factories for chemical production. Chem. Soc. Rev. 49, 4615–4636. doi: 10.1039/d0cs00155d
Leigh, J. A., Mayer, F., and Wolfe, R. S. (1981). Acetogenium kivui, a new thermophilic hydrogen-oxidizing acetogenic bacterium. Arch. Microbiol. 129, 275–280. doi: 10.1007/BF00414697
Liew, F., Martin, M. E., Tappel, R. C., and Heijstra, B. D. (2016). Gas fermentation — a flexible platform for commercial scale production of low-carbon-fuels and chemicals from waste and renewable feedstocks. Front. Microbiol. 7:694. doi: 10.3389/fmicb.2016.00694
Loh, Q. H., Hervé, V., and Brune, A. (2020). Metabolic potential for reductive acetogenesis and a novel energy-conserving [NiFe] hydrogenase in Bathyarchaeia from termite guts — a genome-centric analysis. Front. Microbiol. 11:635786. doi: 10.3389/fmicb.2020.635786
Manakova, E., Golovinas, E., Pocevičiūte, R., Sasnauskas, G., Silanskas, A., Rutkauskas, D., et al. (2024). The missing part: the Archaeoglobus fulgidus Argonaute forms a functional heterodimer with an N-L1-L2 domain protein. Nucleic Acids Res. 52, 2530–2545. doi: 10.1093/nar/gkad1241
McLaughlin, H., Littlefield, A. A., Menefee, M., Kinzer, A., Hull, T., Sovacool, B. K., et al. (2023). Carbon capture utilization and storage in review: sociotechnical implications for a carbon reliant world. Renew. Sust. Energ. Rev. 177, 1–41. doi: 10.1016/j.rser.2023.113215
Moon, J., Henke, L., Merz, N., and Basen, M. (2019). A thermostable mannitol-1-phosphate dehydrogenase is required in mannitol metabolism of the thermophilic acetogenic bacterium Thermoanaerobacter kivui. Environ. Microbiol. 21, 3728–3736. doi: 10.1111/1462-2920.14720
Moon, J., Jain, S., Müller, V., and Basen, M. (2020). Homoacetogenic conversion of mannitol by the thermophilic acetogenic bacterium Thermoanaerobacter kivui requires external CO2. Front. Microbiol. 11:571736. doi: 10.3389/fmicb.2020.571736
Nepomnyashchaya, Y. N., Slobodkina, G. B., Baslerov, R. V., Chernyh, N. A., Bonch-Osmolovskaya, E. A., Netrusov, A. I., et al. (2012). Moorella humiferrea sp. nov., a thermophilic, anaerobic bacterium capable of growth via electron shuttling between humic acid and Fe(III). Int. J. Syst. Evol. Microbiol. 62, 613–617. doi: 10.1099/ijs.0.029009-0
Oehler, D., Poehlein, A., Leimbach, A., Müller, N., Daniel, R., Gottschalk, G., et al. (2012). Genome-guided analysis of physiological and morphological traits of the fermentative acetate oxidizer Thermacetogenium phaeum. BMC Genomics 13:723. doi: 10.1186/1471-2164-13-723
Oliver, G. C., Cario, A., and Rogers, K. L. (2020). Rate and extent of growth of a model extremophile, Archaeoglobus fulgidus, under high hydrostatic pressures. Front. Microbiol. 11:1023. doi: 10.3389/fmicb.2020.01023
Palombarini, F., Masciarelli, S., Incocciati, A., Liccardo, F., Di Fabio, E., Iazzetti, A., et al. (2021). Self-assembling ferritin-dendrimer nanoparticles for targeted delivery of nucleic acids to myeloid leukemia cells. J. Nanobiotechnol. 19, 1–12. doi: 10.1186/s12951-021-00921-5
Ragsdale, S. W. (2008). Enzymology of the wood-Ljungdahl pathway of acetogenesis. Ann. N. Y. Acad. Sci. 1125, 129–136. doi: 10.1196/annals.1419.015
Rahayu, F., Kawai, Y., Iwasaki, Y., Yoshida, K., Kita, A., Tajima, T., et al. (2017). Thermophilic ethanol fermentation from lignocellulose hydrolysate by genetically engineered Moorella thermoacetica. Bioresour. Technol. 245, 1393–1399. doi: 10.1016/j.biortech.2017.05.146
Redl, S., Poehlein, A., Esser, C., Bengelsdorf, F. R., Jensen, T., Jendresen, C. B., et al. (2020). Genome-based comparison of all species of the genus Moorella, and status of the species Moorella thermoacetica and Moorella thermoautotrophica. Front. Microbiol. 10:3070. doi: 10.3389/fmicb.2019.03070
Rosenbaum, F. P., and Müller, V. (2021). Energy conservation under extreme energy limitation: the role of cytochromes and quinones in acetogenic bacteria. Extremophiles 25, 413–424. doi: 10.1007/s00792-021-01241-0
Rosenbaum, F. P., and Müller, V. (2023). Moorella thermoacetica: a promising cytochrome-and quinone-containing acetogenic bacterium as platform for a CO2-based bioeconomy. Green Carbon 1, 2–13. doi: 10.1016/j.greenca.2023.06.002
Rosenbaum, F. P., Poehlein, A., Egelkamp, R., Daniel, R., Harder, S., Schlüter, H., et al. (2021). Lactate metabolism in strictly anaerobic microorganisms with a soluble NAD+-dependent l-lactate dehydrogenase. Environ. Microbiol. 23, 4661–4672. doi: 10.1111/1462-2920.15657
Sanford, P. A., and Woolston, B. M. (2022). Expanding the genetic engineering toolbox for the metabolically flexible acetogen Eubacterium limosum. J. Ind. Microbiol. Biotechnol. 49, 1–11. doi: 10.1093/jimb/kuac019
Santaella, N. V., Sousa, D. Z., and Stams, A. J. M. (2023). Moorella caeni sp. nov., isolated from thermophilic anaerobic sludge from a methanol-fed reactor. Int. J. Syst. Evol. Microbiol. 73, 1–10. doi: 10.1099/ijsem.0.005905
Schöne, C., Poehlein, A., Jehmlich, N., Adlung, N., Daniel, R., von Bergen, M., et al. (2022). Deconstructing Methanosarcina acetivorans into an acetogenic archaeon. Proc. Natl. Acad. Sci. USA 119, 1–7. doi: 10.1073/pnas.2113853119
Schuchmann, K., and Müller, V. (2014). Autotrophy at the thermodynamic limit of life: a model for energy conservation in acetogenic bacteria. Nat. Rev. Microbiol. 12, 809–821. doi: 10.1038/nrmicro3365
Schuchmann, K., and Müller, V. (2016). Energetics and application of heterotrophy in acetogenic bacteria. Appl. Environ. Microbiol. 82, 4056–4069. doi: 10.1128/AEM.00882-16
Schwarz, F. M., and Müller, V. (2020). Whole-cell biocatalysis for hydrogen storage and syngas conversion to formate using a thermophilic acetogen. Biotechnol. Biofuels 13, 32–11. doi: 10.1186/s13068-020-1670-x
Slobodkin, A., Reysenbach, A. L., Mayer, F., and Wiegel, J. (1997). Isolation and characterization of the homoacetogenic thermophilic bacterium Moorella glycerini sp. nov. Int. J. Syst. Bacteriol. 47, 969–974. doi: 10.1099/00207713-47-4-969
Slobodkina, G. B., Merkel, A. Y., Kuchierskaya, A. A., and Slobodkin, A. I. (2022). Moorella sulfitireducens sp. nov., a thermophilic anaerobic bacterium isolated from a terrestrial thermal spring. Extremophiles 26, 1–9. doi: 10.1007/s00792-022-01285-w
Stetter, K. O. (1988). Archaeoglobus fulgidus gen. Nov., sp. nov.: a new taxon of extremely thermophilic archaebacteria. Syst. Appl. Microbiol. 10, 172–173. doi: 10.1016/S0723-2020(88)80032-8
Takemura, K., Kato, J., Kato, S., Fujii, T., Wada, K., Iwasaki, Y., et al. (2023). Enhancing acetone production from H2 and CO2 using supplemental electron acceptors in an engineered Moorella thermoacetica. J. Biosci. Bioeng. 136, 13–19. doi: 10.1016/j.jbiosc.2023.04.001
Takemura, K., Kato, J., Kato, S., Fujii, T., Wada, K., Iwasaki, Y., et al. (2021). Autotrophic growth and ethanol production enabled by diverting acetate flux in the metabolically engineered Moorella thermoacetica. J. Biosci. Bioeng. 132, 569–574. doi: 10.1016/j.jbiosc.2021.08.005
Vasu, K., and Nagaraja, V. (2013). Diverse functions of restriction-modification systems in addition to cellular defense. Microbiol. Mol. Biol. Rev. 77, 53–72. doi: 10.1128/MMBR.00044-12
Wang, S., Huang, H., Kahnt, J., and Thauer, R. K. (2013). A reversible electron-bifurcating ferredoxin-and NAD-dependent [FeFe]-hydrogenase (HydABC) in Moorella thermoacetica. J. Bacteriol. 195, 1267–1275. doi: 10.1128/JB.02158-12
Weghoff, M. C., and Müller, V. (2016). CO metabolism in the thermophilic acetogen Thermoanaerobacter kivui. Appl. Environ. Microbiol. 82, 2312–2319. doi: 10.1128/AEM.00122-16
Wiegel, J., Braun, M., and Gottschalk, G. (1981). Clostridium thermoautotrophicum species novum, a thermophile producing acetate from molecular hydrogen and carbon dioxide. Curr. Microbiol. 5, 255–260. doi: 10.1007/BF01571158
Yu, L., Yuan, Y., Tang, J., and Zhou, S. (2017). Thermophilic Moorella thermoautotrophica-immobilized cathode enhanced microbial electrosynthesis of acetate and formate from CO2. Bioelectrochemistry 117, 23–28. doi: 10.1016/j.bioelechem.2017.05.001
Keywords: acetogen, thermophile, Wood-Ljungdahl pathway, Moorella, Thermoanaerobacter, cell factory, genetic tools
Citation: Bourgade B and Islam MA (2024) Progresses and challenges of engineering thermophilic acetogenic cell factories. Front. Microbiol. 15:1476253. doi: 10.3389/fmicb.2024.1476253
Edited by:
Sean Michael Scully, University of Akureyri, IcelandReviewed by:
Simone Antonio De Rose, University of Exeter, United KingdomCopyright © 2024 Bourgade and Islam. This is an open-access article distributed under the terms of the Creative Commons Attribution License (CC BY). The use, distribution or reproduction in other forums is permitted, provided the original author(s) and the copyright owner(s) are credited and that the original publication in this journal is cited, in accordance with accepted academic practice. No use, distribution or reproduction is permitted which does not comply with these terms.
*Correspondence: M. Ahsanul Islam, bS5pc2xhbUBsYm9yby5hYy51aw==