- 1School of Basic Medical Sciences, Health Science Center, Ningbo University, Ningbo, China
- 2Wenzhou Central Blood Station, Wenzhou, China
- 3Key Laboratory of Laboratory Medicine, Ministry of Education, Zhejiang Provincial Key Laboratory of Medical Genetics, College of Laboratory Medicine and Life Sciences, Wenzhou Medical University, Wenzhou, China
- 4Ningbo Municipal Center for Disease Control and Prevention, Ningbo, China
Introduction: Japanese encephalitis virus (JEV) and Zika virus (ZIKV) pose a severe threat to human health. Our previous research results, as well as those of other research groups, indicated that antibodies (Abs) induced by JEV infection or JEV vaccine vaccination could enhance ZIKV infection in vitro and exacerbate the mortality of ZIKV-infected mice, vice versa, which is known as antibody-dependent enhancement (ADE). Although studies on other flaviviruses revealed that altering the amino acid residues located in the fusion loop (FL) of envelope (E) protein can reduce the level of flavivirus-cross-reactive Abs, thereby abating the ADE of heterologous flavivirus infection, it is unclear whether this strategy is equally applicable to JEV.
Methods: In this study, we constructed recombinant adenoviruses and nucleotide-modified mRNA-lipid nanoparticle (LNP) encoding JEV wild-type E protein or E protein mutant (designated as Ad5-JEV-EWT and Ad5-JEV-Emut; JEV-EWT mRNA-LNP, and JEV-Emut mRNA-LNP). We evaluated the immunogenicity of these vaccine candidates in mice and the capacity of vaccine-immune mouse sera to neutralize JEV infection or mediate ADE of ZIKV infection in vitro and in vivo.
Results: Ad5-JEV-Emut or JEV-Emut mRNA-LNP immunization induced ZIKV-cross-reactive Ab response which is dramatically lower than that induced by Ad5-JEV-EWT and JEV-EWT mRNA-LNP, respectively. The levels of JEV-neutralizing Abs induced by Ad5-JEV-Emut or JEV-Emut mRNA-LNP are comparable to that induced by Ad5-JEV-EWT and JEV-EWT mRNA-LNP, respectively. The ability of Abs induced by Ad5-JEV-Emut to enhance ZIKV infection in vitro is attenuated as compared with that induced by Ad5-JEV-EWT. Moreover, JEV-Emut mRNA-LNP immunization elicited potent T cell response similar to JEV-EWT mRNA-LNP in mice. Mice immunized with each mRNA-LNP exhibited lower level of serum viral load than the mock-immunized mice post JEV challenge. Mice receiving JEV-EWT mRNA-LNP-immune mouse sera exhibited ADE post ZIKV challenge whereas passively transferred JEV-Emut mRNA-LNP-immune mouse sera did not lead to obvious ADE of ZIKV infection in recipient mice. Most importantly, maternally acquired Abs did not enhance the mortality of 1-day-old neonates born to JEV-Emut mRNA-LNP-immunized mice post ZIKV challenge.
Discussion: These results suggest that optimizing the FL sequence of JEV could significantly reduce the level of JEV/ZIKV-cross-reactive Abs and abrogate the ADE of ZIKV infection, providing a promising strategy to develop effective and safety JEV vaccine.
1 Introduction
Japanese encephalitis virus (JEV), as a mosquito-borne pathogen belonging to the genus flavivirus within the family Flaviviridae, is identified as a primary etiological agent of viral encephalitis (Sharma et al., 2021). As reported by the World Health Organization (WHO) in 2019, JEV accounts for approximately 68,000 annual cases globally (Campbell et al., 2011). JEV is mainly transmitted via Culex tritaeniorhynchus bite and predominantly endemic in 24 countries/territories including China, Japan, South Korea, etc. (Kumar et al., 2021). It was estimated that around three billion individuals are at the risk of JEV infection (Liu et al., 2020). Patients infected with JEV can show a variety of symptoms, from mild fever to severe hemorrhagic, encephalitic manifestations or even death (Huang et al., 2021). Previous studies indicated that the mortality rate for patients with Japanese encephalitis ranges from 20 to 30%. Furthermore, among the survivors, 30 to 50% will experience permanent neurological sequelae (Campbell et al., 2011; Solomon et al., 2002). To date, four types of JEV vaccines are issued for use: the mouse brain-derived inactivated JEV vaccine, the Vero cell-derived inactivated JEV vaccine, the cell culture-derived live-attenuated JEV vaccine, and the live recombinant (chimeric) vaccine (Hills et al., 2014; Batchelor and Petersen, 2015). Same to JEV, Zika virus (ZIKV) belongs to the genus flavivirus, family Flaviviridae. By July 2019, the cases of ZIKV infection were reported among 87 countries/territories (Fong and Chu, 2022). Different to JEV, the transmission vectors for ZIKV are Aedes aegypti and Aedes albopictus mosquitoes (Westaway et al., 1985). In addition to vector-borne transmission via mosquito bites, ZIKV can be transmitted sexually (Govero et al., 2016); moreover, vertical transmission of ZIKV can occur from mother to fetus during pregnancy, leading to congenital Zika syndromes, including fetal/newborn microcephaly (Rubin et al., 2016; Rasmussen et al., 2016; Mlakar et al., 2016). Roughly 80% of adults infected with ZIKV are asymptomatic, with the remaining patients exhibiting symptoms ranging from mild non-specific symptoms such as fever, joint and muscle pain, to severe neurological disorders such as Guillain–Barré syndrome (Musso et al., 2014; Cao-Lormeau et al., 2016; Dirlikov et al., 2016; Schuler-Faccini et al., 2016). Currently, there are no specific therapeutic agents or vaccines available for the treatment or prevention of ZIKV infection.
The phenomenon of antibody-dependent enhancement (ADE) refers to a scenario where, under certain conditions, one virus infection can be enhanced by antibodies (Abs) induced by a related virus (Bournazos et al., 2020). ADE in dengue virus (DENV) research field was first described by Halstead et al. (1973a,b). They observed that individuals with pre-existing immunity to one serotype of DENV are more likely to develop severe forms of the disease, such as dengue shock syndrome and dengue hemorrhagic fever, upon subsequent infection with a heterologous serotype (Watanabe et al., 2015; Muhammad Azami et al., 2020). Up to date, ADE was widely identified among many flaviviruses of the family Flaviviridae (Bardina et al., 2017; Chen et al., 2020; He et al., 2020; Zhang et al., 2020; Katzelnick et al., 2017; Katzelnick et al., 2020). For instance, mice receiving sera from patients infected with West Nile virus (WNV) exhibited a markedly higher mortality rate than mice given with naïve sera upon subsequent ZIKV infection (Bardina et al., 2017). In addition, transfer of sera obtained from DENV-infected patients significantly increased the mortality of ZIKV-infected mice (Bardina et al., 2017). Our recent study also indicated that 1-day-old pups born to JEV-immunized mice exhibited an increased mortality and elevated viremia than the newborns born to the naïve mothers after ZIKV attack (Chen et al., 2020). Moreover, our findings were subsequently confirmed by the experimental results of other labs (He et al., 2020; Zhang et al., 2020). Similarly, serum Abs from ZIKV-immunized mice could cross-recognize JEV antigen and increased the mortality of recipient mice post JEV challenge (Chen et al., 2020). The ADE phenomenon of DENV through animal experiments was confirmed by a clinical cohort study (Katzelnick et al., 2017). The results of another clinical cohort study by this research group also revealed that individuals infected with ZIKV are more likely to develop severe disease after infecting with DENV (Katzelnick et al., 2020). Considering that many flaviviruses (particularly JEV and ZIKV) have the overlapping epidemic areas and the fact that JEV vaccines is widely used among populations in multiple countries/territories, the ADE issue between JEV and ZIKV is of particular concern. Therefore, developing or optimizing flavivirus vaccine is a necessary measure.
The envelope (E) protein of flavivirus is located in the viral membrane and plays a pivotal role in the process of flavivirus infecting host cell. E protein could facilitate virus to bind to the receptor of susceptible cell and mediate the membrane fusion between virus and cell (Zhang et al., 2017). In fact, the extracellular part of E protein is composed of three domains: Domain I (EDI), Domain II (EDII), and Domain III (EDIII). The B-cell epitopes are predominantly located in EDI and EDIII (Tabata et al., 2023). EDII mainly stabilizes the homodimeric configuration of E protein and harbors a highly conserved fusion loop (FL) sequence among flaviviruses, mediating virus-cell membrane fusion (Chiou et al., 2012; Chiou et al., 2008). Previous studies reported that the FL sequence contains a critical epitope that is involved in the induction of flavivirus-cross-reactive Abs, resulting in the ADE of heterologous flavivirus infection (de Alwis et al., 2014; Gunawardana and Shaw, 2018; Vogt et al., 2011). Recently, a number of studies have demonstrated that replacing the different amino acid sites of FL could reduce the production of flavivirus-cross-reactive Abs, and even alleviate ADE between flaviviruses (Vogt et al., 2011; Crill et al., 2007; Berneck et al., 2020; Zhang et al., 2006; Rockstroh et al., 2015). However, it is unclear whether optimizing the FL sequence of JEV can reduce the production of Abs that have ADE effects on ZIKV infection.
In the present study, to clarify whether optimizing the FL sequence of JEV E protein can reduce the production of ZIKV-cross-reactive Abs and attenuate the ADE activity of JEV-induced Abs against ZIKV infection, we generated recombinant adenoviruses and mRNA-lipid nanoparticle (mRNA-LNP) on the basis of the amino acid sequences of JEV wild-type E protein and E protein mutant. Our data show here, (1) the ability of Abs induced by Ad5-JEV-Emut or JEV-Emut mRNA-LNP to cross-recognize ZIKV antigen is significantly reduced; (2) the neutralizing activity of Ad5-JEV-Emut-immune or JEV-Emut mRNA-LNP-immune mouse sera against JEV infection is comparable to the Abs induced by Ad5-JEV-EWT and JEV-EWT mRNA-LNP, respectively; (3) the ability of Abs induced by Ad5-JEV-Emut to mediate ADE of ZIKV infection in vitro is attenuated; (4) JEV-Emut mRNA-LNP immunization induced a comparable level of CD8+ T cell response to JEV-EWT mRNA-LNP; (5) JEV-Emut mRNA-LNP immunization can protect mice against JEV challenge, characterized by the significant reduction of serum viral load; (6) passive transfer of the JEV-Emut mRNA-LNP-immune mouse sera does not increase the mortality of recipient mice post ZIKV challenge; (7) maternally acquired anti-JEV Abs does not increase the mortality of pups born to JEV-Emut mRNA-LNP-immunized mice after ZIKV challenge.
2 Materials and methods
2.1 Cell lines, viruses and reagents
Vero cell was purchased from American Type Culture Center (ATCC) and maintained in RPMI-1640 medium supplemented with 10% fetal bovine serum and 1% penicillin/streptomycin. HEK293T cell was obtained from ATCC and cultured using MEM medium supplemented with 10% fetal bovine serum and 1% penicillin/streptomycin. Both JEV (strain ZJ14-52, GenBank Accession No. MK558811) and ZIKV (strain Zhejiang04, GenBank Accession No. KX117076.1) were propagated in Vero cells and the viral stocks were tittered using plaque-forming assay (PFA) as described below. The viral titer was expressed as plaque-forming unit (PFU)/mL. HRP-conjugated goat anti-mouse IgG and FITC-conjugated goat anti-mouse IgG were purchased from Proteintech (Chicago, America). 3,3′,5,5′-Tetramethylbenzidine (TMB) was purchased from Macklin (Shanghai, China). Methyl cellulose M450 was purchased from Ourchem (Beijing, China). Isopropyl-β-D-thiogalactoside (IPTG) was purchased from Solarbio (Beijing, China). Polyetherimide (PEI) transfection reagent was purchased from GlpBio (California, United States).
2.2 Mice
Six-week-old male and female wild-type (WT) C57BL/6 mice were purchased from the Animal Model Research Center at Nanjing University, China, and were maintained and bred in the Animal Center of Ningbo University, China. The experiments were performed in strict accordance with the animal welfare act and public health service policy on humane care and use of laboratory animals and were approved by the ethical committee of Ningbo University (No. 2020-55). Sample sizes were estimated on the basis of our previous studies. Animal experiments were randomized but not blinded.
2.3 Peptide synthesis
Three previously identified H-2b-restricted CD8+ T cell epitopes in ZIKV (ZIKV-E4–12, ZIKV-E57–65, and ZIKV-E420–428) and their variants in JEV (JEV-E4–12, JEV-E57–65, and JEV-E416–424) (Chen et al., 2020) were synthesized by ChinaPeptides with a purity >95% (Supplementary Table S1).
2.4 Expression of viral proteins
The genes (optimized for E. coli codon usage) encoding the extracellular domain of E protein of JEV (E406, aa 1-406) and ZIKV (E410, aa 1-410) were synthesized by GeneWiz (Suzhou, China) and cloned into the pET21a expression vectors, each featuring a 6 × His tag at the 3′ terminal of gene sequence. pET21a-JEV-E406 and pET21a-ZIKV-E410 were, respectively transformed into E. coli BL21 strain, and protein expression was induced using IPTG at a final concentration of 1 mM. The recombinant JEV-E406 and ZIKV-E410 proteins were purified using a Ni-NTA (TransGen Biotech, Beijing, China), followed by further concentrated through a 10 kDa ultrafiltration tube (Millipore, Darmstadt, Germany). The protein purity was assessed by SDS-PAGE and the protein concentration was quantified using a BCA kit (TransGen Biotech).
2.5 Construction of recombinant adenoviruses
The genes (optimized for Homo sapiens codon usage) encoding the complete amino acid sequences of wild-type E protein (EWT, 500 aa) or E protein mutant (Emut, 500 aa, with W101A and F108A amino acid substitutions in the FL sequence) of JEV were synthesized by GeneWiz and cloned into the pCAGGS plasmid, resulting in recombinant plasmids pCAGGS-JEV-EWT and pCAGGS-JEV-Emut. After dual enzyme digestion of the recombinant plasmid and pShuttle-CMV plasmid, the purified gene fragment was ligated with the empty pShuttle-CMV plasmid and transformed into competent bacteria Stbl3 strain to produce recombinant pShuttle-CMV plasmids (pShuttle-CMV-JEV-EWT or pShuttle-CMV-JEV-Emut). The linearized plasmid pShuttle-CMV-JEV-EWT or pShuttle-CMV-JEV-Emut was electroporated into competent E. coli BJ5183 strain containing a human adenovirus type 5 (Ad5) genomic backbone plasmid (pAdEasy-1) for homologous recombination, and the recombinant Ad5 plasmids (Ad5-JEV-EWT and Ad5-JEV-Emut) were screened out. The recombinant Ad5 plasmids were transfected into HEK293T cell to produce recombinant Ad5 which was then tittered by checking the GFP-positive cell using confocal microscope on the basis of Vero cell. The titer of recombinant Ad5 was expressed as infectious unit (IFU) per ml. Recombinant adenoviruses were used to infect Vero cells and the expression of JEV-E protein in the cell supernatant was evaluated using WB. JEV-E406-immune mouse sera were used as the primary antibody and HRP-conjugated goat anti-mouse IgG mAb worked as the secondary antibody.
2.6 Generation of mRNA sequences encoding JEV-E protein in vitro
To prepare a mRNA sequence encoding JEV-E protein in vitro, we designed a DNA sequence consisting of T7 promoter, a 5′ UTR sequence, a Kozak sequence, a signal peptide sequence, gene sequence encoding JEV-EWT or JEV-Emut (W101A and F108A substitutions in the FL sequence), a 3′ UTR sequence, and a poly A tail from 5′ terminal to 3′ terminal, respectively (Supplementary Table S2). The DNA sequence was engineered into an optimized sequence with as much as more codons containing A or G (Supplementary Table S2) and cloned into the eukaryotic plasmid pBluescript II KS (+) to produce recombinant plasmids pBluescript II KS (+)-JEV-EWT and pBluescript II KS (+)-JEV-Emut. Using the linearized pBluescript II KS (+)-JEV-EWT or pBluescript II KS (+)-JEV-Emut as template, RNA was produced using an in vitro transcription kit after supplementing with N1-methyl-pseudouridine and other components. Finally, the 5′ terminal of RNA was capped using capping reagent kit (Novoprotein, Suzhou, China) to generate functional JEV-EWT mRNA and JEV-Emut mRNA.
2.7 Transfection of 293T cells with mRNA
293T cells were seeded to cell culture plate (10 cm2) and incubated for 24 h in a 37°C/5%CO2 incubator. Next day, the cell supernatant was discarded and MEM medium without FBS was added to the cells. Four hours later, 10 μg mRNA was diluted in PBS to reach 0.5 mL. 0.5 mL PEI (20 μg) was added to diluted mRNA and incubated for 20 min at room temperature. The PEI/mRNA mixture was added to cells and transfected for 4 h. After discarding the transfection reagent, complete MEM medium (with 10% FBS) was added to the cells and incubated for 2 days in the incubator. The cell supernatant was harvested for detecting JEV-E protein using WB. JEV-E406-immune mouse sera were used as the primary antibody and HRP-conjugated goat anti-mouse IgG mAb worked as the secondary antibody.
2.8 Production of mRNA-lipid-nanoparticle
The purified mRNA was diluted into citrate buffer (pH4, 50 mM) to form the aqueous phase with a final concentration of 170 μg/mL. Briefly, 4-(N,N-dimethylamino) butyric acid (dilinoleic) methyl ester (Dlin-MC3-DMA; cationic lipid), Diethyl phosphatidylcholine (DSPC; non-cationic lipid), Cholesterol (sterol), and 1, 2-dimyristoyl-rac-glycero-3-methoxypolyethylene glycol-2000 (DMG-PEG2000; PEG-modified lipid) were dissolved into ethanol to make the organic phase, reaching the final concentration of 6.25 mM, 1.25 mM, 4.8125 mM, 0.1875 mM, respectively. The aqueous phase and the organic phase were mixed at a volume ratio of 3:1 and passed through a Microflow S microfluidic nanomedicine fabrication instrument at a flow rate of 12 mL/min to produce JEV-EWT mRNA-LNP or JEV-Emut mRNA-LNP. The zeta potential and polydispersity of mRNA-LNP were determined using particle size analyzer. The particle size of mRNA-LNP was measured using transmission electron microscope. The mRNA-LNP was cleaved using 2% Triton X-100 and the amount of released mRNA was measured for calculating the mRNA encapsulation rate.
2.9 Animal immunization
Recombinant adenovirus and JEV-E mRNA-LNP were used to immunize C57BL/6 mice. As for recombinant adenovirus immunization, 6-week-old male mice were subcutaneously (s.c.) injected with Ad5-JEV-EWT or Ad5-JEV-Emut (1 × 108 IFU/mouse). The mice receiving PBS were used as control. Two weeks later, the mice were boosted with the same recombinant adenovirus. Two weeks after the second immunization, the mice were sacrificed and the mouse sera were collected for ELISA, plaque-reduction neutralization test (PRNT), and in vitro ADE assay. The mRNA-LNP was used to immunize adult (6-week-old) or young (1-week-old) mice. In one protocol, 6-week-old male or female mice were injected s.c. with JEV-EWT mRNA-LNP or JEV-Emut mRNA-LNP (5 or 50 μg mRNA/mouse) twice at a two-week interval. The mice receiving PBS were used as control. Two weeks after the second immunization, the male mice were sacrificed and the mouse sera were used for ELISA, PRNT, and passive transfer experiments. The splenocytes were used for ELISpot assay. The immunized female mice were mated with 10-week-old naïve male mice. Approximate 3 weeks later, the 1-day-old neonates born to the mRNA-LNP-immunized mice were injected s.c. with 1,000 PFU of ZIKV, and the mouse weight and mortality were recorded daily for 28 days. In another protocol, 1-week-old neonates were injected s.c. with mRNA-LNP (0.1 or 1 μg mRNA/mouse) and boosted with the same mRNA-LNP 2 weeks later. Two weeks after the second immunization, some immunized mice were sacrificed and the mouse sera were used for ELISA and PRNT; the remaining mice were injected s.c. with 1 × 105 PFU of JEV. Three days later, the mice were sacrificed and the mouse sera were harvested for viral load detection using PFA.
2.10 ELISA
The antigen-reactive IgG in mouse sera (recombinant adenovirus-infected mice; mRNA-LNP-immunized adult mice; mRNA-LNP-immunized young mice) was detected using indirect ELISA. In brief, the high-affinity ELISA plates were coated with recombinant JEV-E406 or ZIKV-E410 proteins (20 μg/mL, 100 μL/well) and incubated for 1.5 h at 37°C. The plates were then blocked for 1.5 h at 37°C using 5% non-fat milk/PBS solution. Serially diluted mouse sera were added to the plates and incubated for 1.5 h at 37°C. HRP-conjugated Goat anti-mouse IgG (1:2,000 dilution) was added to the plate and incubated for 1.5 h at 37°C. The plate was developed with fresh TMB solution for 10 min at room temperature. The OD450 value of each well was measured using a microplate reader.
2.11 Plaque reduction neutralization test
The ability of recombinant adenovirus-immune or mRNA-LNP-immune mouse sera to neutralize JEV in vitro was evaluated on Vero cells using PRNT. In brief, Vero cells were seeded in each well (1 × 105 cells/well) of 24-well cell culture plate and incubated for 24 h in a 37°C/5% CO2 incubator. Mouse sera (recombinant adenovirus-infected mice; mRNA-LNP-immunized adult mice; mRNA-LNP-immunized young mice) were 3-fold serially diluted in the well (240 μL/well) of 96-well culture plate, followed by addition of 50 μL of JEV solution (containing 50 PFU), and incubated for 1 h at 37°C. After discarding the supernatant of each well, the mixture of serum/virus was added to Vero cell and infected for 1 h at 37°C. After discarding the viral solution, the cells were overlaid with 1% methyl cellulose/medium and incubated for 3 days in a 37°C/5% CO2 incubator. The cells were fixed with 4% paraformaldehyde for 1 h at room temperature and stained with 0.5% crystal violet solution for 10 min at room temperature. The plaques of each well were counted and the NT50 (the highest serum dilution that can reduce viral plaques by 50%) titers were calculated.
2.12 ADE assay
The potential of mouse sera to enhance the infection of ZIKV was assessed on the basis of K562 cells. K562 cells were plated to each well (2 × 104 cells/well) of U-bottom 96-well cell culture plates and incubated for 24 h in the 37°C/5% CO2 incubator. Mouse sera (Ad5-JEV-EWT-immune, Ad5-JEV-Emut-immune) were serially diluted in the well (50 μL/well) of 96-well plate and 50 μL of ZIKV (containing 1,000 PFU) were added to each well, and incubated at 37°C for 1 h. After centrifuged for 10 min at 2,000 rpm, the supernatant of K562 cells was discarded, followed by addition of the serum/virus mixture. After 1-h infection at 37°C, the viral solution was discarded and fresh medium was added to cells. After 24-h incubation, the cells were collected and fixed with 4% paraformaldehyde for 40 min at room temperature. The cells were then permeabilized with 0.5% Triton X-100/PBS for 40 min at temperature. The cells were incubated with viral antigen-specific antibody (ZIKV-E410-immune mouse serum, 1:200 dilution) for 1 h at room temperature, followed by incubation with FITC-labeled goat anti-mouse IgG mAb (1:1000 dilution) for 1 h at room temperature. The percentage of FITC-positive cells among each sample was determined using flow cytometry.
2.13 Mouse IFNγ ELISpot assay
Mouse splenocytes were plated to each well (1 × 106/100 μL/well) of 96-well precoated ELISpot plate (DAKEWE, coated with anti-mouse IFNγ mAb), followed by addition of individual peptide (2 μg/well). Wells with cells but without peptide were served as negative control. After 24 h incubation in a 37°C/CO2 incubator, the plates were incubated sequentially with biotinylated anti-mouse IFN-γ mAb, HRP-conjugated streptavidin, and developed with 3-amino-9-ethylcarbazole color development solution. The colored spots of each well were counted and the results were expressed as the number of spot-forming cell (SFC) per 1 × 106 mouse splenocytes. The presence of >20 SFCs/well with a stimulation index of >2 (index = ratio of test SFCs to control SFCs) was considered a positive response.
2.14 Passive transfer experiments
The effect of JEV-E mRNA-LNP-immune mouse sera on JEV or ZIKV infection in vivo was determined using passive transfer experiments. Different volume (3 or 15 μL) of either JEV-EWT mRNA-LNP-immune mouse sera or JEV-Emut mRNA-LNP-immune mouse sera were injected s.c. into 1-day-old mice. Two hours later, the mice were injected s.c. with 10 PFU of JEV or 1,000 PFU of ZIKV. The mouse weights and mortality were recorded daily for 14 days and 28 days, respectively.
2.15 Tissue viral loads detection
The titers of viral stock and the viral load in mouse sera were determined using PFA. Briefly, Vero cells are seeded to each well (1 × 105 cells/well) of 24-well cell culture plate and incubated for 24 h in a 37°C/5% CO2 incubator. After discarding the cell supernatant, 240 μL of serially diluted viral stock or mouse serum was added to cells and the plate was incubated for 1 h at 37°C. After discarding the samples, the cells were overlaid with 1% Methyl cellulose/medium and incubated for 3–5 days in a 37°C/5% CO2 incubator. The cells were fixed with 4% paraformaldehyde for 1 h at room temperature and stained with 0.5% crystal violet solution for 10 min at room temperature. The numbers of plaque in each well were counted and the viral load in mouse serum was expressed as PFU/mL.
2.16 Statistical analysis
All data were analyzed using Prism 8 software (GraphPad Software, La Jolla, CA, United States) and expressed as the means ± SEM. The comparison between group means and survival data were analyzed using the Mann–Whitney test and a log-rank test, respectively. p < 0.05 was considered statistically significant.
3 Results
3.1 Production of recombinant adenovirus encoding JEV-E protein
To explore whether optimizing the FL sequence of the JEV-E protein affects the production of ZIKV-cross-reactive Abs, we initially designed the amino acid sequence of JEV-E protein mutant (JEV-Emut) by incorporating two amino acid substitutions to the wild-type E protein (JEV-EWT). With the assistance of shuttle plasmid, the genes encoding the JEV-EWT or JEV-Emut were successfully transferred into the human Ad5 genomic backbone plasmid to produce a recombinant adenovirus plasmid carrying the JEV-EWT gene or JEV-Emut gene. After transfecting the linearized recombinant adenovirus plasmid into 293T cells, recombinant adenovirus particles (having an average diameter of around 100 nm) were identified in the cytoplasm of cells using transmission electron microscopy (Figures 1A,B). We collected the cell supernatants and purified the recombinant adenoviruses Ad5-JEV-EWT and Ad5-JEV-Emut. Two recombinant adenoviruses were sent to company GeneWiz for sequencing, and the inserted nucleotide sequences showed no nucleotide loss, insertion, or mutation as compared to our original sequences (data not shown). Both Ad5-JEV-EWT and Ad5-JEV-Emut were used to infect Vero cells and the presence of JEV-E protein in the cell supernatant was determined using WB (Figure 1C). Since the recombinant adenovirus carries the gene encoding GFP, the expression of GFP in the Vero cells infected with Ad5-JEV-EWT or Ad5-JEV-Emut was observed using confocal microscopy and the titer of recombinant adenovirus was determined by counting GFP-positive cells (Figures 1D,E).
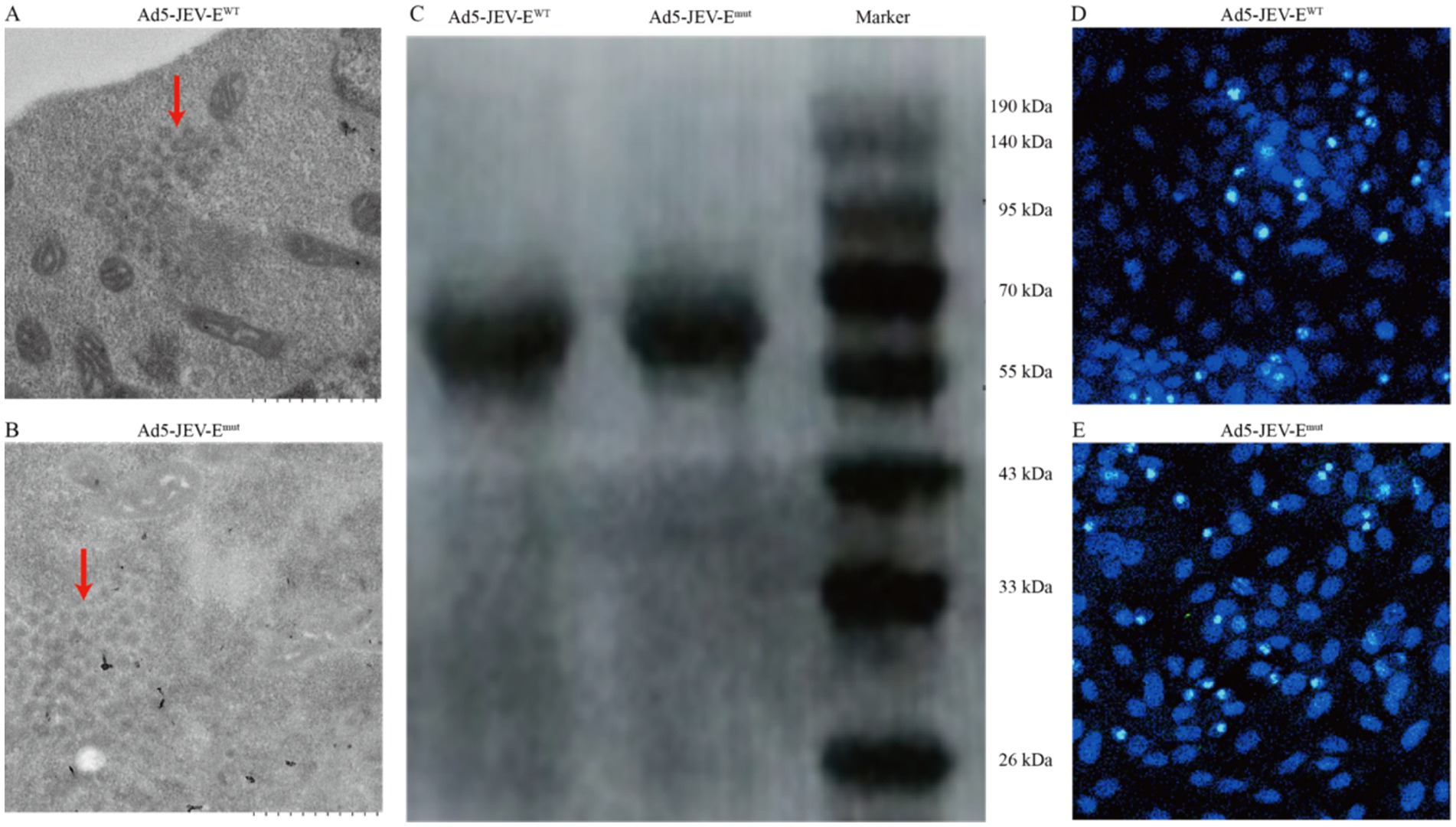
Figure 1. Generation of recombinant adenovirus encoding JEV-E protein. (A,B) 293T cells were transfected with recombinant adenovirus plasmids JEV-EWT or JEV-Emut and the viral particles of recombinant adenovirus in the cells were observed using transmission electron microscopy. (C) Vero cells were infected with recombinant adenoviruses Ad5-JEV-EWT or Ad5-JEV-Emut and the expression of JEV-E protein was determined using WB. (D,E) Vero cells were infected with recombinant adenoviruses Ad5-JEV-EWT or Ad5-JEV-Emut and the GFP-positive cells were observed using confocal microscopy.
3.2 Generation of JEV-E mRNA-LNP in vitro
We further designed DNA sequence for producing mRNA in vitro and cloned it into pBluscript II KS (+) plasmid, resulting into pBluscript II KS (+)-JEV-EWT and pBluscript II KS (+)-JEV-Emut (Figure 2A). On the basis of linearized recombinant plasmid, we proceeded to generate mRNA encoding JEV-EWT or JEV-Emut with a length of 1875 bp (Figure 2B; Supplementary Figure S1). Following transfection of Vero cells with either JEV-EWT mRNA or JEV-Emut mRNA, the expression levels of the E protein were assessed using WB, displaying a similar expression proficiency (Figure 2C). We next encapsulated the mRNA within four components (cationic lipid, non-cationic lipid, cholesterol, and PEG-modified lipid) and produced the mRNA-LNP. The transmission electron micrograph showed that the mRNA-LNPs have an average diameter of around 100 nm (Figures 2D,E).
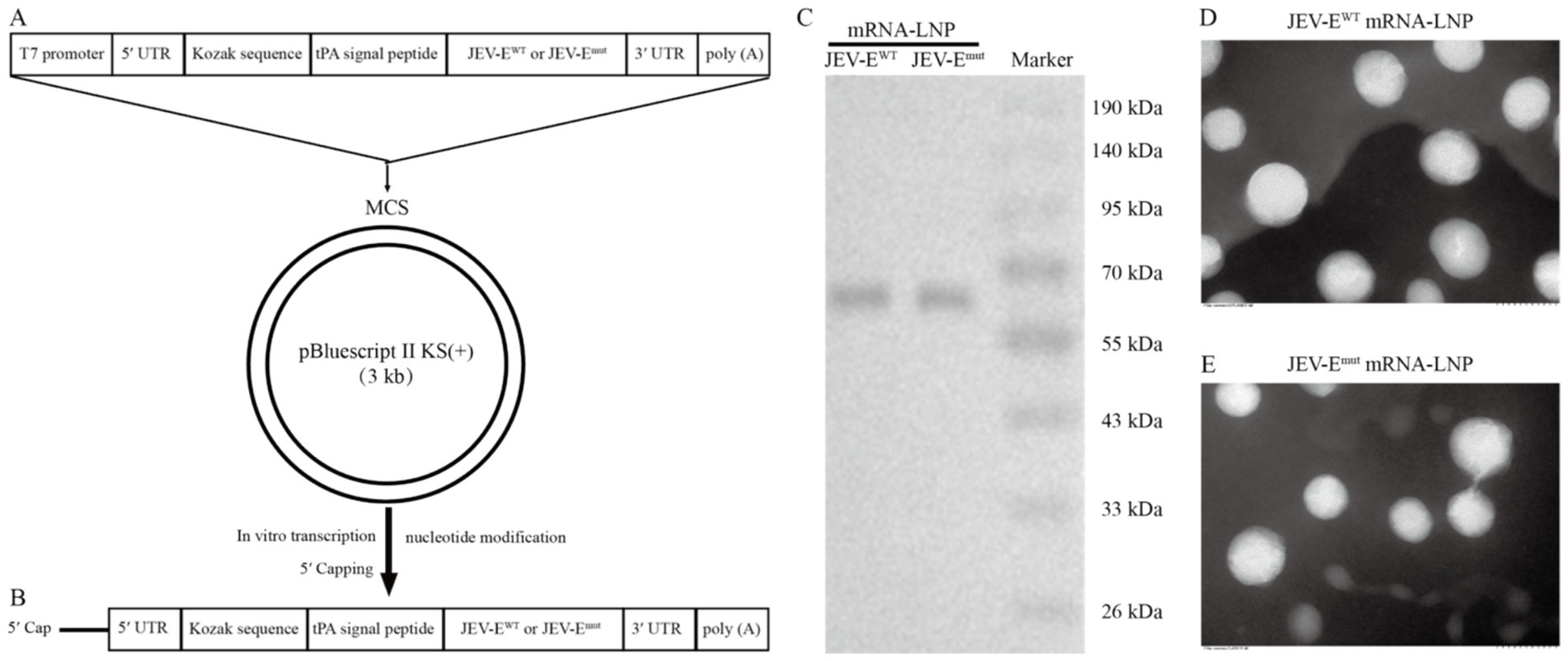
Figure 2. Generation of JEV-E mRNA-LNP. (A) The gene map of DNA used for in vitro transcription of RNA. (B) Transcription of RNA in vitro using linearized recombinant plasmid as template. (C) JEV-EWT mRNA or JEV-Emut mRNA was transfected into 293T cells using PEI transfection reagent and the expression of E protein was detected using WB. (D,E) The mRNA-LNP particle size was analyzed using transmission electron microscopy.
3.3 Optimizing the fusion loop sequence of JEV attenuates the production of ZIKV-cross-reactive antibodies
We next immunized 6-week-old mice with recombinant adenoviruses Ad5-JEV-EWT or Ad5-JEV-Emut, and found that each recombinant adenovirus immunization induced the production of JEV-E-reactive IgG (Supplementary Figures S2A,B), which could cross-recognize ZIKV-E protein. Interestingly, the ability of Ad5-JEV-Emut-immune mouse serum Abs to recognize ZIKV-E protein was significantly weaker than that of Ad5-JEV-EWT-immune mouse serum Abs (Supplementary Figures S2C,D). Next, 6-week-old mice were immunized with different doses of JEV-EWT mRNA or JEV-Emut mRNA in the form of LNP twice at a two-week internal, and the levels of JEV-E protein-reactive IgG and cross-reactive Abs against ZIKV-E protein were quantified using an ELISA. The results demonstrated that whether it is 5 μg or 50 μg, JEV-EWT mRNA-LNP and JEV-Emut mRNA-LNP induced a similar level of JEV-E-reactive antibody response (Figures 3A,B). However, 50 μg of JEV-Emut mRNA induced a significantly lower level of ZIKV-E-cross-reactive antibody response compared to JEV-EWT mRNA (Figures 3C,D). Considering 10-week-old mice resist JEV infection and have no obvious viremia post JEV infection whereas 5-week-old mice are susceptible to JEV infection and can maintain viremia for several days post JEV infection (data not shown), we then immunized 1-week-old young mice with different doses of JEV-EWT mRNA-LNP or JEV-Emut mRNA-LNP twice at a two-week interval and determined the JEV-E protein-reactive IgG in the mouse sera at 2 weeks post the second immunization. Similar Ab response against JEV-E protein and ZIKV-E protein were observed in mouse sera (Figures 3E–H). These data indicate that the substitution of amino acid residues among the FL of JEV can significantly attenuate the induction of ZIKV-E-cross-reactive Abs, which is not affected by mouse age. We next evaluate the cellular immune response induced by JEV-E mRNA-LNP immunization by detecting the frequencies of peptide-specific T cells in the splenocytes of JEV-E mRNA-LNP-immunized mice. The results show that 5 μg JEV-EWT mRNA induced a similar T cell immune response with 5 μg JEV-Emut mRNA while 50 μg JEV-EWT mRNA induced a stronger T cell immune response than 50 μg JEV-Emut mRNA (Figures 4A,B). But, 50 μg mRNA did not significantly increase T cell response than 5 μg mRNA (Figures 4C,D). These results indicate that substitution of amino acid residues in the FL sequence of JEV can significantly reduce the level of ZIKV-cross-reactive antibody response, but does not affect the humoral and cellular immune responses against JEV antigens.
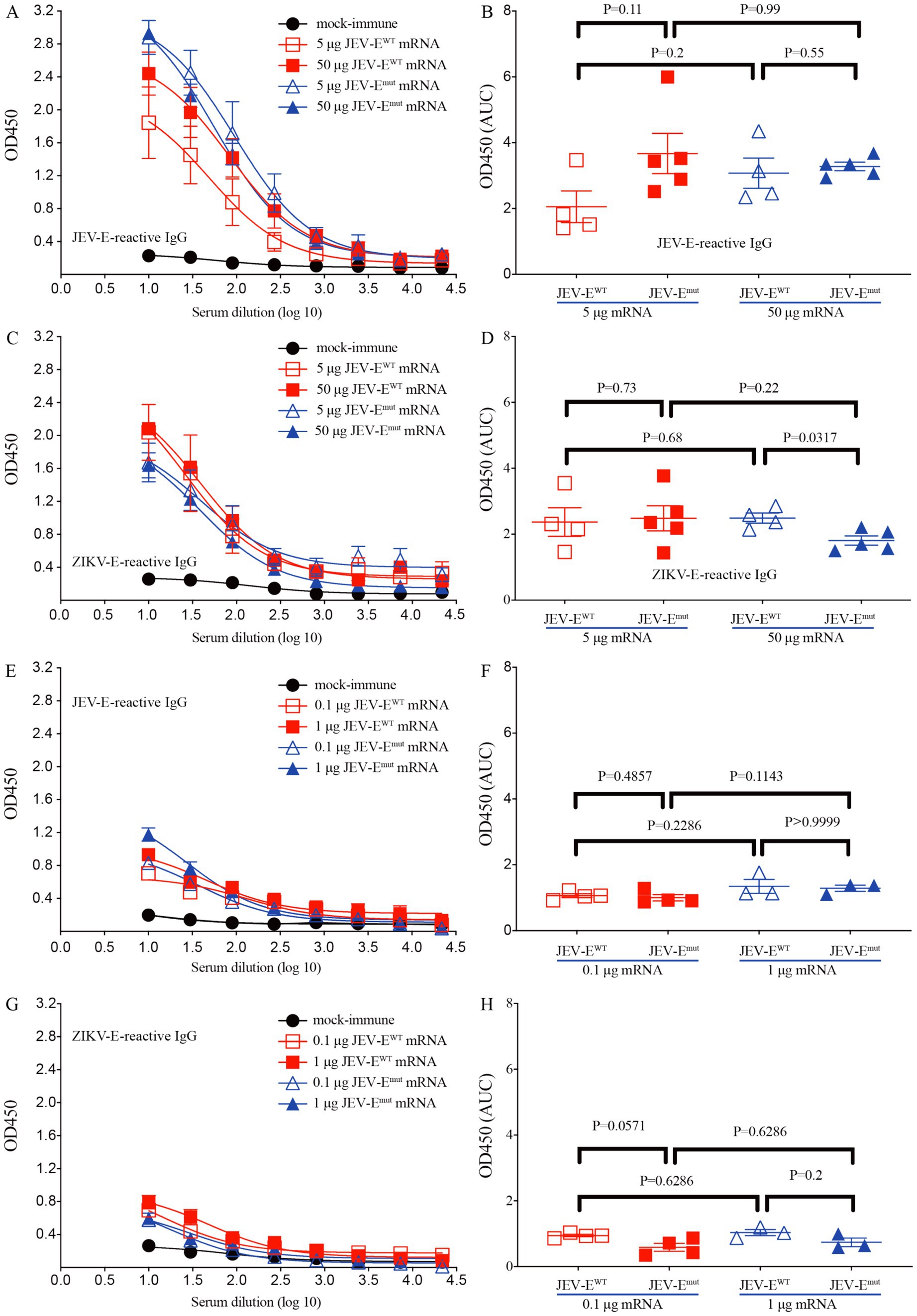
Figure 3. JEV-Emut mRNA-LNP immunization induces low level of ZIKV-E-cross-reactive IgG response. Different doses of JEV-EWT mRNA-LNP or JEV-Emut mRNA-LNP (5 or 50 μg mRNA/adult mouse; 0.1 or 1 μg mRNA/young mice) were used to immunize adult (6-week-old) mice or young (1-week-old) mice twice as described in materials and methods. (A–D) Mouse sera were collected from mock-immune, JEV-EWT mRNA-LNP-immune, and JEV-Emut mRNA-LNP-immune adult mice. (E–H) Mouse sera were collected from mock-immune, JEV-EWT mRNA-LNP-immune, and JEV-Emut mRNA-LNP-immune young mice. The levels of JEV-E406- and ZIKV-E410-reactive IgG were measured using ELISA. Data are presented as the mean ± SEM. *p < 0.05 by two-tailed Mann–Whitney U test. “AUC”: area under curve.
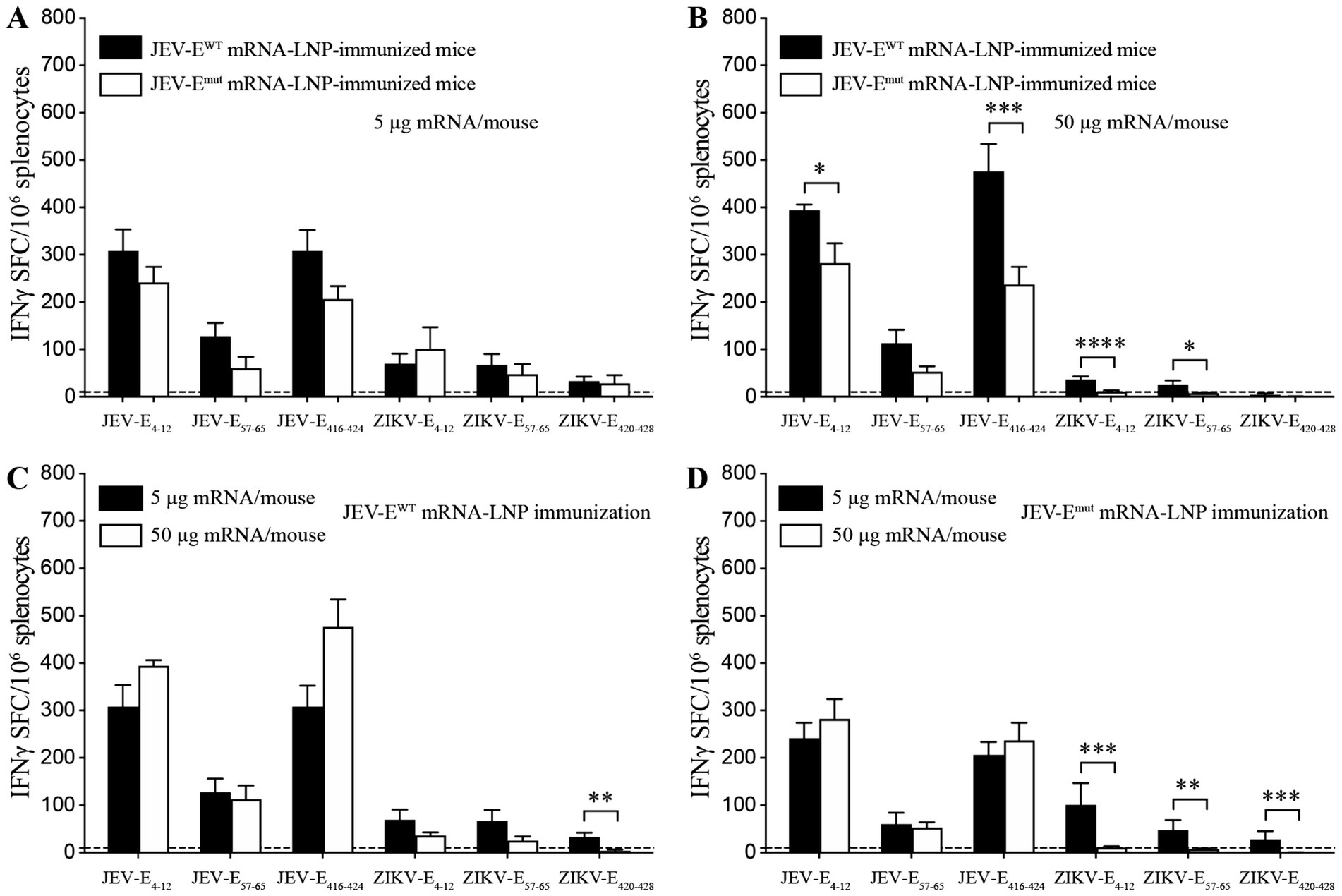
Figure 4. JEV-E mRNA-LNP immunization induces T cell response. (A–D) C57BL/6 mice were immunized with JEV-EWT mRNA-LNP or JEV-Emut mRNA-LNP as described in Materials and Methods. Twenty-eight days later, the splenocytes were stimulated in vitro with the indicated CD8+ T cell epitopes from JEV or the corresponding ZIKV variants. The frequencies of IFNγ-producing CD8+ T cells (SFC) were detected using IFNγ ELIspot assays. Data are presented as the mean ± SEM. *p < 0.05, **p < 0.01, and ***p < 0.001 by two-tailed Mann–Whitney U test.
3.4 Ad5-JEV-Emut-immune mouse serum abs mediate weak ADE of ZIKV infection in vitro
Due to the resistance of K562 cells to ZIKV infection and its inability to be directly infected, the ZIKV-binding Abs can mediate the virus entry to cells by binding to the Fc receptors on the surface of K562 cells, making it a commonly used cell line for performing in vitro ADE assay (Chen et al., 2020). Therefore, we compared the differences in enhancing ZIKV infection in vitro between Ad5-JEV-EWT-immune mouse sera and Ad5-JEV-Emut-immune mouse sera based on K562 mice. As shown in Figures 5A,B, Ad5-JEV-EWT-immune mouse serum has a strong enhancing effect on ZIKV infection, and enhanced infection fold decreases continuously with serum diluted. When the serum is diluted at 1:10, the infection of ZIKV is increased by about 16 folds. Although Ad5-JEV-Emut-immune mouse serum still has an enhancing effect on ZIKV infection, there is a significant reduction compared to Ad5-JEV-EWT-immune mouse serum in total. When the serum is diluted at 1:10, it enhances ZIKV infection by about 8 folds.
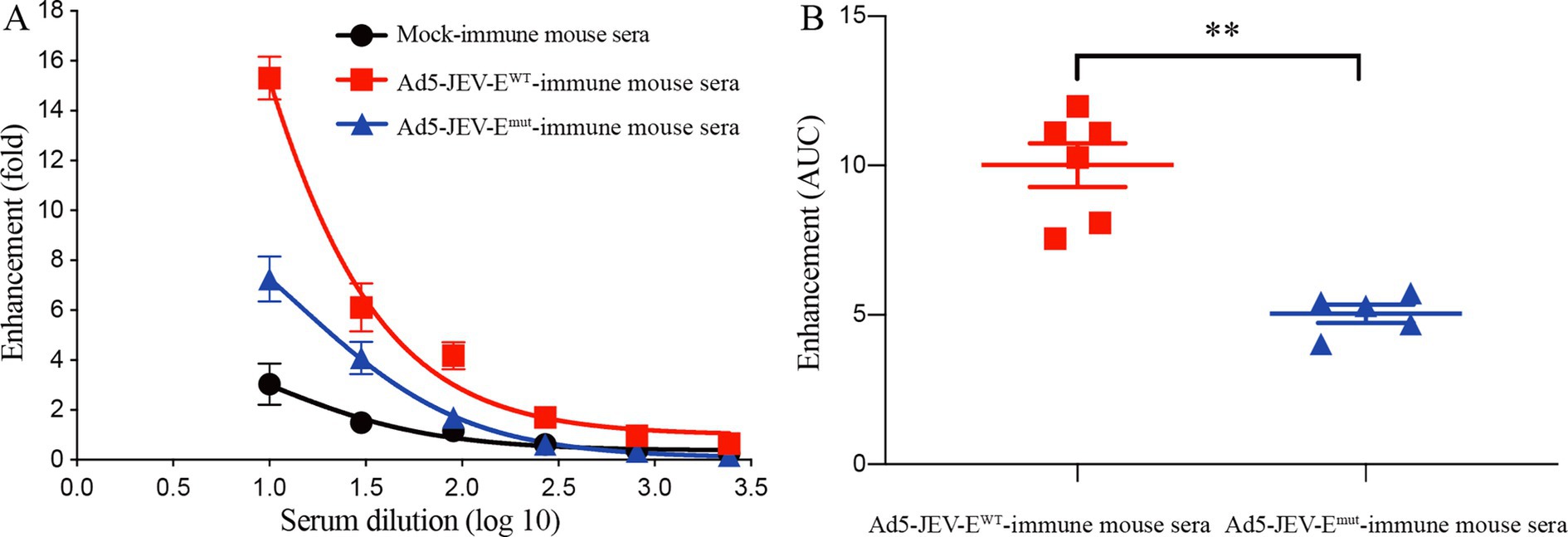
Figure 5. Antibodies induced by Ad5-JEV-Emut mediate attenuated ADE of ZIKV infection in vitro. (A,B) K562 cells were infected with ZIKV at the absence or presence of diluted Ad5-JEV-EWT-immune mouse serum or Ad5-JEV-Emut-immune mouse serum. The percent infection of K562 cells were determined by detecting ZIKV antigen-positive cells using flow cytometry. Data are presented as the mean ± SEM. **p < 0.01 by two-tailed Mann–Whitney U test.
3.5 Optimizing the fusion loop sequence of JEV does not affect the level of JEV-neutralizing antibodies
In light of the aforementioned substitution of two amino acids within the FL region of the E protein were shown to attenuate the induction of ZIKV-cross-reactive Abs, we were then propelled to investigate the potential impact of such amino acid substitutions on the production of neutralizing Abs against JEV using PRNT. The results demonstrate that sera from mice immunized with Ad5-JEV-Emut effectively neutralized JEV in vitro, with a NT50 titer of around 1:199 which is significantly higher than that of Ad5-JEV-EWT-immune mouse sera (NT50 titer is about 1:70, Figures 6A,B). Interestingly, sera from 6-week-old or 1-week-old mice immunized with JEV-EWT mRNA-LNP or JEV-Emut mRNA-LNP exhibit high level of JEV-neutralizing antibody response. The NT50 titers are 1:199 versus 1:933, 1:468 versus 1:588, respectively (Figures 6C–F). These results suggest that substitution of amino acid residues in the FL sequence of JEV does not affect the level of JEV-neutralizing antibody response induced by JEV-Emut.
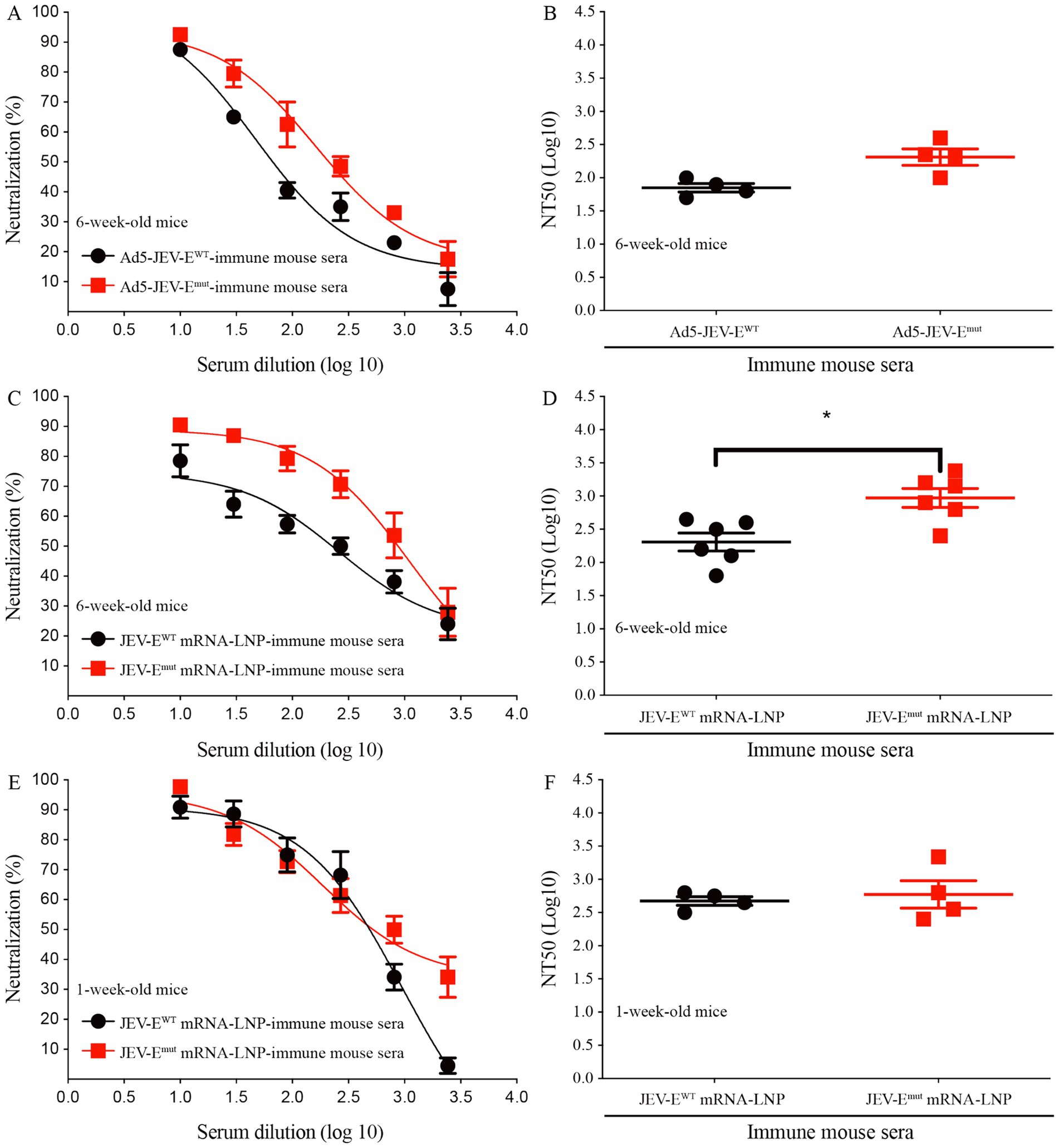
Figure 6. Optimizing the fusion loop sequence of JEV does not reduce the production of JEV-neutralizing antibodies. (A–F) Ad5-JEV-EWT-immune, Ad5-JEV-Emut-immune, JEV-EWT mRNA-LNP-immune, JEV-Emut mRNA-LNP-immune mouse sera were tested for neutralization of JEV infection of Vero cells using plaque-reduction neutralization test. Data are presented as the mean ± SEM. *p < 0.05 by two-tailed Mann–Whitney U test.
3.6 JEV-E mRNA-LNP immunization protects mice against JEV challenge
To evaluate whether two types of JEV-E mRNA-LNP-immune mouse sera can protect recipient mice from JEV attack, we injected 15 μL of JEV-EWT mRNA-LNP or JEV-Emut mRNA-LNP-immune mouse sera into 1-day-old neonatal mice, and then attacked them with JEV, followed by mouse survival study. As shown in Figures 7A,B, the percent survival of recipient mice transferred with 15 μL of JEV-EWT mRNA-LNP-immune mouse sera or JEV-Emut mRNA-LNP-immune mouse sera was significantly higher than that of the control group (55.5% versus 0 and 37.5% versus 0, respectively).
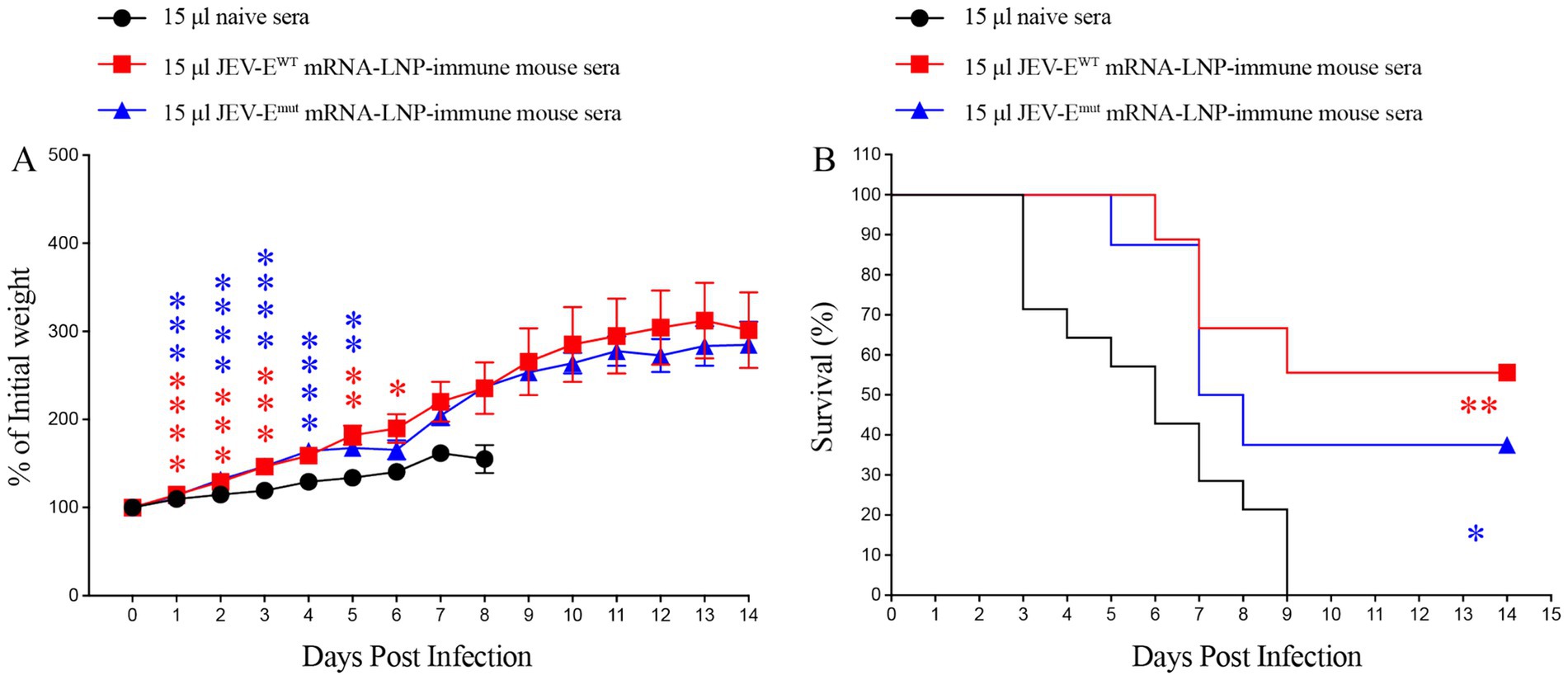
Figure 7. Transfer of JEV-E mRNA-LNP-immune mouse sera protects recipient mice against JEV attack. One-day-old naive C57BL/6 mice were injected s.c. with 15 μL of pooled mock-immune or JEV-EWT mRNA-LNP-immune or JEV-Emut mRNA-LNP-immune mouse sera. Two hours later, the mice were injected s.c. with 10 PFU of JEV. Mouse weights and survival were recorded daily for up to 14 days. Data are presented as the mean ± SEM. *p < 0.05, **p < 0.01, ***p < 0.001, and ****p < 0.0001 by two-tailed Mann–Whitney U test (A) or log-rank test (B).
Given the efficacy observed in passive serum transfer assays, whereas mouse-immune sera conferred protection against lethal JEV challenges, this next experiment aimed to ascertain whether our developed mRNA vaccine candidates could directly protect mice from JEV. To this end, one-week-old mice were immunized with 1 μg of JEV-EWT mRNA or JEV-Emut mRNA for twice and then challenged with JEV 2 weeks after the second immunization. Three days post JEV attack, the mouse sera were collected for viral load analysis. The data demonstrated that both mRNA-LNP immunization significantly shielded the mice from JEV infection. PFA results revealed that the levels of viral load in the sera of mice immunized with either JEV-EWT mRNA-LNP or JEV-Emut mRNA-LNP were substantially lower as compared to those in naïve mice (43 PFU/mL versus 501 PFU/mL, p = 0.0148; 26 PFU/mL versus 501 PFU/mL, p = 0.0093, respectively), confirming the protective efficacy of the JEV-E mRNA-LNP against JEV infection (Figure 8). These results indicate that mRNA constructed based on E protein mutant with optimized FL sequences showed no significant difference in protecting mice against ZIKV attacks compared to mRNA encoding wild-type E protein of JEV.
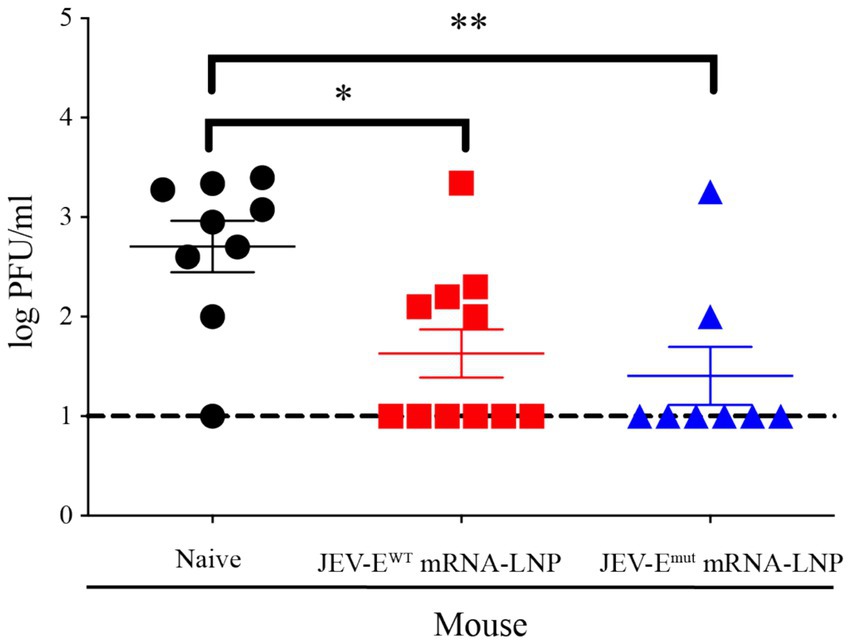
Figure 8. JEV-E mRNA-LNP immunization reduce the tissue viral loads of JEV-infected mice. One-week-old young mice were immunized with JEV-E mRNA-LNP as described in Materials and Methods. The immunized mice were challenged with 1 × 105 PFU of JEV. Three days post infection, the mice were sacrificed and the mouse sera were collected for detecting viral load using PFA. Data are presented as the mean ± SEM. *p < 0.05 and **p < 0.01 by two-tailed Mann–Whitney U test.
3.7 Transfer of JEV-Emut mRNA-LNP-immune sera does not enhance ZIKV infection in recipient mice
Considering a significant decrease in the level of ZIKV-cross-reactive antibody induced by JEV-Emut mRNA-LNP, we next to explore whether the reduction of ZIKV-cross-reactive antibody response can potentially affect the occurrence of ADE of ZIKV infection in vivo. Specifically, we injected 3 or 15 μL of pooled JEV-EWT mRNA-LNP- or JEV-Emut mRNA-LNP-immune mouse sera into 1-day-old neonates and performed mouse survival study post ZIKV challenge. As a result, 1-day-old mice receiving 3 μL of pooled naive mouse sera all died within 22 days after ZIKV infection (Figures 9A,B). However, 1-day-old mice given with 3 μL of JEV-EWT mRNA-LNP-immune mouse sera showed an accelerated death (all die within 14 days after ZIKV challenge) (Figures 9A,B). But, the 1-day-old mice transferred with 3 μL of JEV-Emut mRNA-LNP-immune mouse sera all died at around 19 days after ZIKV infection and exhibited a significant reduction of weight loss compared with mice receiving naive mouse sera at multiple timepoints post ZIKV infection (Figures 9A,B).
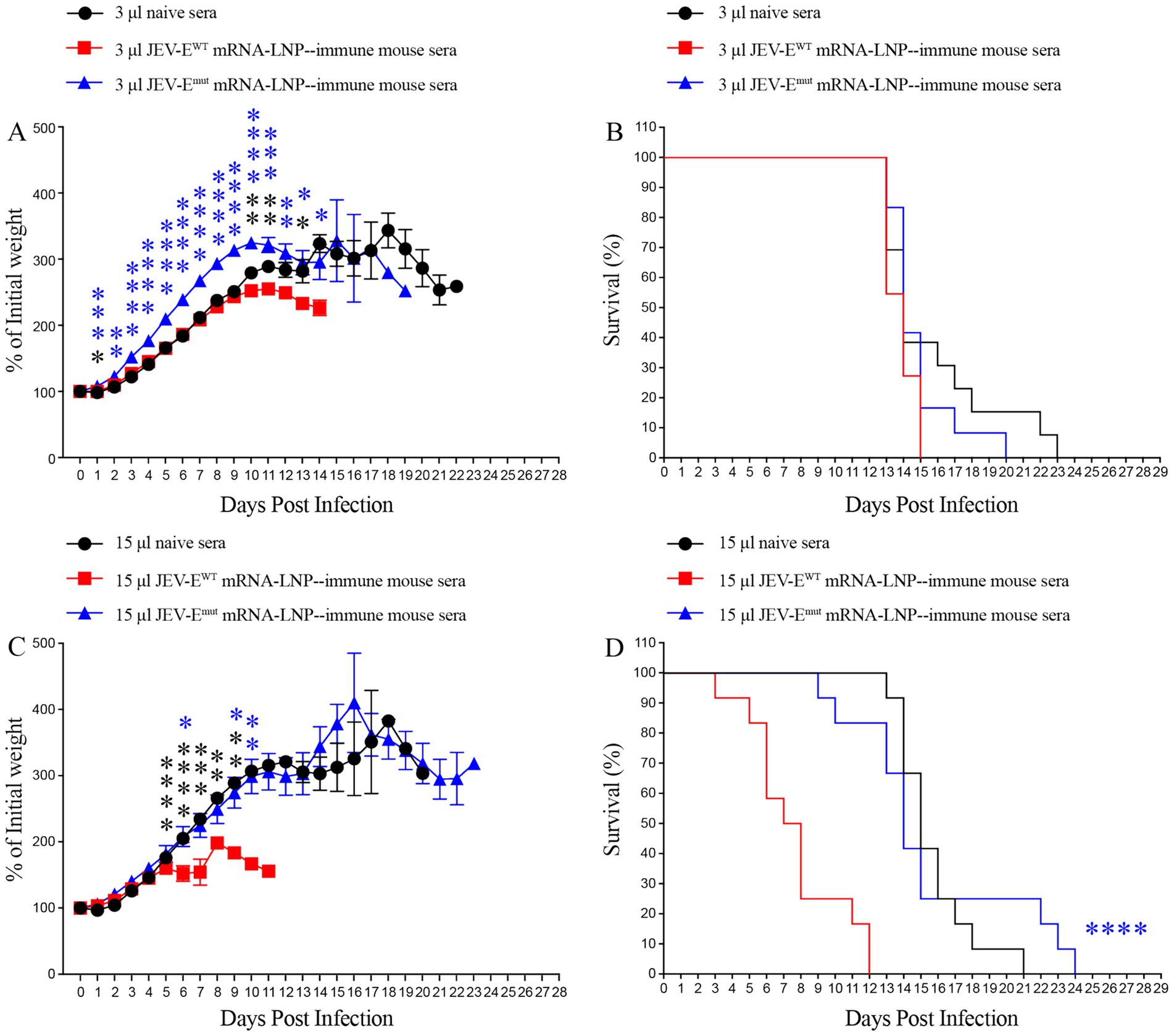
Figure 9. Transfer of JEV-Emut mRNA-LNP-immune mouse sera do not accelerate the death of ZIKV-infected mice. One-day-old naive C57BL/6 mice were injected s.c. with 3 μL or 15 μL of pooled mock-immune or JEV-EWT mRNA-LNP-immune or JEV-Emut mRNA-LNP-immune mouse sera. Two hours later, the mice were injected s.c. with 1,000 PFU of ZIKV. Mouse weights and survival were recorded daily for up to 28 days. Data are presented as the mean ± SEM. *p < 0.05, **p < 0.01, ***p < 0.001, and ****p < 0.0001 by two-tailed Mann–Whitney U test (A,C) or log-rank test (B,D).
Next, 1-day-old pups injected with 15 μL of naive mouse serum also died within 20 days after ZIKV infection. However, 1-day-old pups given with 15 μL of JEV-EWT mRNA-LNP-immune mouse serum exhibited a significant acceleration of death (all died within 11 days after ZIKV infection). However, 1-day-old pups receiving 15 μL of JEV-Emut mRNA-LNP-immune mouse serum died around 23 days after ZIKV infection. Compared to JEV-Emut mRNA-LNP-immune mouse serum, JEV-EWT mRNA-LNP-immune mouse serum significantly accelerated the death of 1-day-old mice (Figures 9C,D). These results indicate that the Abs induced by mRNA encoding wild-type E protein of JEV can exacerbate ZIKV infection in mice at the specific doses, leading to earlier death of mouse, representing speculated ADE of ZIKV infection. The Abs induced by mRNA encoding the E protein mutant of JEV did not have an obvious enhancing effect on ZIKV infection, suggesting optimized JEV mRNA vaccine is safer than unmodified mRNA vaccine.
3.8 Maternally acquired ZIKV-reactive Ab did not increase the mortality of pups born to mRNA-LNP-immune mice
Although our passive transfer experiment results showed that Abs against the JEV-EWT protein can accelerate the death of ZIKV-infected mice at certain doses, but, do anti JEV-EWT Abs have ADE against ZIKV infection in the real world? If so, whether the Abs induced by optimized mRNA vaccine will not mediate ADE of ZIKV infection? Considering that IgG in the mother’s body can across the placenta and enter the body of fetus or newborn, as well as that many women who have been infected with JEV or vaccinated with JEV vaccine will have children who may subsequently be infected with ZIKV, we then evaluate the impact of anti-JEV Abs obtained by the pups from the female mice vaccinated with either JEV-EWT mRNA-LNP or JEV-Emut mRNA-LNP on subsequent ZIKV infection. The results showed that the 1-day-old mice born to JEV-Emut mRNA-LNP-immunized female mice loss less weight compared to 1-day-old mice born to naive females post ZIKV challenge (Figure 10A). Approximately 6% of 1-day-old mice born to naive female mice survived ZIKV infection, while all 1-day-old mice born to JEV-EWT mRNA-LNP-immunized female mice died within 17 days post ZIKV challenge. In comparison, around 14% of 1-day-old mice born to JEV-Emut mRNA-LNP-immunized female mice survived ZIKV infection (Figure 10). These results suggest that maternally acquired Abs from JEV-EWT mRNA-LNP-immunized female mice can accelerate the death of 1-day-old mice during ZIKV infection, while the maternally acquired Abs against JEV-Emut protein do not exacerbate the death of 1-day-old mice infected with ZIKV, but rather slightly improve the percent survival of mice, suggesting that Abs induced by JEV-Emut mRNA immunization may not make the host develop severe diseases after ZIKV infection.
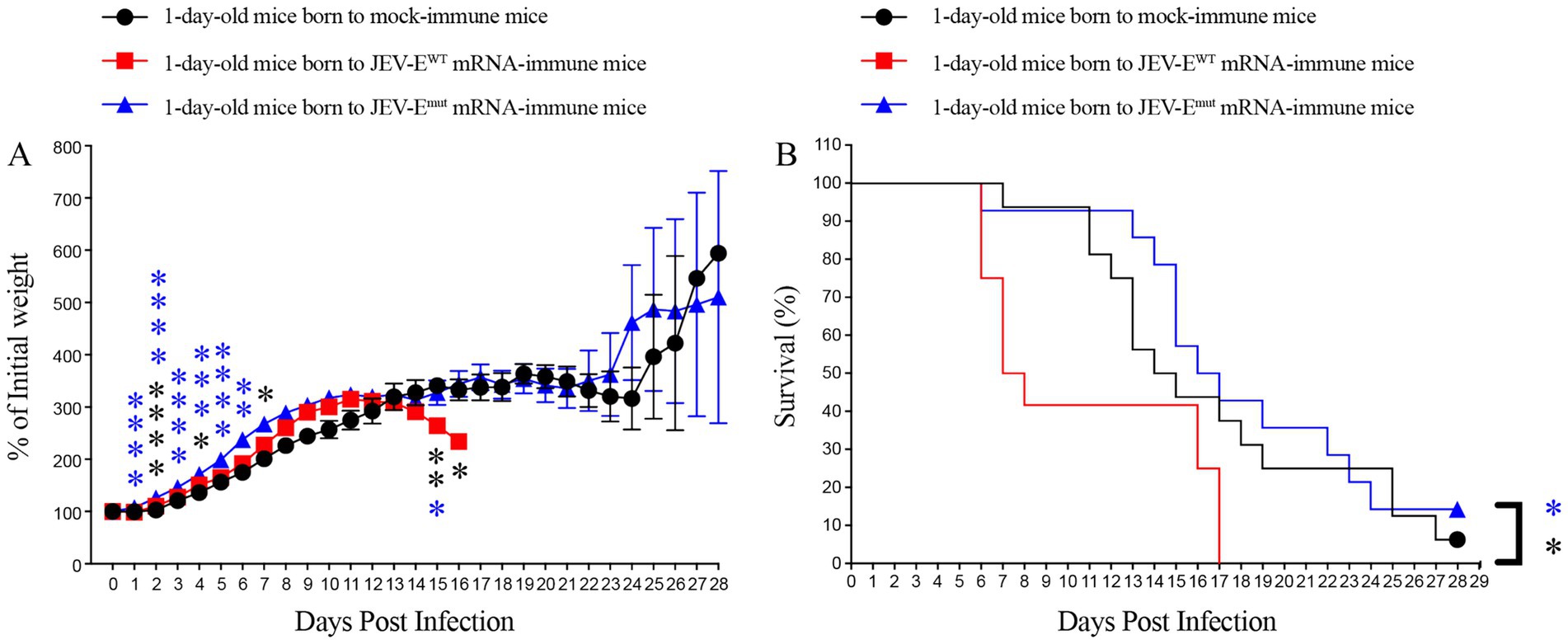
Figure 10. Maternally acquired ZIKV-reactive antibody did not increase the mortality of pups born to mRNA-LNP-immune mice. Six-week-old female C57BL/6 mice were immunized with JEV-EWT mRNA-LNP or JEV-Emut mRNA-LNP as described in materials and methods. Four weeks later, mock-immune or JEV-E mRNA-LNP-immune females were mated with 10-week-old naive male mice. One-day-old pups born to JEV-E mRNA-LNP-immune females were injected s.c. with 1,000 PFU of ZIKV. Mouse weights and survival were recorded daily for up to 28 days. Data are presented as the mean ± SEM. *p < 0.05, **p < 0.01, ***p < 0.001, and ****p < 0.0001 by two-tailed Mann–Whitney U test (A) or log-rank test (B).
4 Discussion
Fusion loop sequence (98-111 aa of E protein) is a highly conserved sequence among many flaviviruses (Supplementary Table S3). The FL sequence (DRGWGNGCGLFGKG) of DENV is same to that of ZIKV while there is one amino acid residue variation (L108F) in the FL sequence of JEV (DRGWGNGCGFFGKG). FL has strong immunogenicity and responsible for the induction of flavivirus-cross-reactive Abs (de Alwis et al., 2014; Gunawardana and Shaw, 2018; Vogt et al., 2011). In the present study, we replaced the amino acid W at position 101 and the amino acid F at position 108 in the FL sequence of JEV with amino acid A and obtain an Emut protein sequence. We then constructed the recombinant adenovirus and mRNA-LNP encoding JEV-EWT and JEV-Emut. Our in vitro and in vivo experimental results showed that the levels of JEV-neutralizing Abs induced by either Ad5-JEV-Emut or JEV-Emut mRNA-LNP did not decrease as compared with vaccine candidates encoding JEV-EWT, while the levels of Abs reactive to ZIKV antigen and its ADE effect on ZIKV infection were significantly reduced. Considering that our and other research groups’ previous results indicate that JEV infection- or vaccine inoculation-induced Abs have an ADE effect on ZIKV infection (Chen et al., 2020; He et al., 2020; Zhang et al., 2020), thus, optimized JEV vaccines may have broad application value in the future.
In the past several decade years, a growing number of studies have highlighted that ADE phenomenon is commonly present between different serotypes of the same flavivirus [such as dengue virus (DENV)], as well as between various flavivirus species (Bardina et al., 2017; Chen et al., 2020; He et al., 2020; Zhang et al., 2020; Katzelnick et al., 2017; Katzelnick et al., 2020). Considering that most flavivirus cross-reactive Abs are produced against the highly conserved FL domain in the viral E protein, researchers have made some attempts to optimize the FL sequence, and have achieved some expected results (Berneck et al., 2020; Zhang et al., 2006; Rockstroh et al., 2015). An earlier study showed that a single substitution (L107F) within the FL of West Nile virus abolished binding ability of a non-neutralizing Mab (3D9) which is broadly cross-reactive with other flaviviruses (Zhang et al., 2006). Later, Rockstroh et al. (2015), generated recombinant E proteins which contain four mutations (T76R, Q77E, W101R, L107R) in the FL domain of four DENV serotypes. The specific mixtures of these antigen mutants exhibit reduced cross-reactivity against heterologous flaviviruses. Berneck et al. (2020), expressed an E protein mutant carrying four mutations (T76A, Q77G, W101R and L107R) in and near the FL of ZIKV and observed that this antigen mutant elicits Abs with equal neutralizing capacity as the wildtype E protein. But, this mutant antigen induces much less serological cross-reactivity and does not cause ADE of DENV infection.
In addition, although two studies identified that DENV virus-like particles carrying W101A and F108A substitutions were not recognized by cross-reactive antibodies, but the researchers did not evaluate whether the capacity of this DENV VLP mutant to induce ADE-mediating antibodies will be attenuated (Lai et al., 2013; Tsai et al., 2013). Moreover, whether these substitutions (W101A and F108A) affect the production of neutralizing antibodies also needs to be considered. For instance, researchers introduced four amino acid mutations (T76R, Q77E, W101R, and L107R) into the E protein of ZIKV and prepared mRNA-LNP encoding prM-E. The ADE effect of the vaccine-induced antibodies on DENV infection was significantly attenuated, but the level of ZIKV-neutralizing antibodies induced by the vaccine was also weakened (Richner et al., 2017). In the present study, we designed an E protein mutant by introducing W101A and F108A substitutions in the FL sequence of the JEV viral E protein and constructed recombinant adenovirus and mRNA vaccine because these two types of vaccines can induce strong humoral and cellular immunity. In the preparation of mRNA vaccines, we prioritize choosing codons rich in adenine (A) or guanine (G) to replace codons containing cytidine (C) and uridine (U), as this strategy can increase the stability of RNA. As expected, these substitutions we introduced in the FL sequence of JEV did not affect the production of JEV-neutralizing antibodies, but significantly reduced the production of ZIKV-cross-reactive antibodies and attenuated the ADE of ZIKV infection. These results indicate that optimizing the amino acid sequence of the FL sequence of the flavivirus has the potential to reduce the potential ADE of the vaccine against heterologous flavivirus infections. Previous studies showed that CD8+ T cell response induced by JEV infection or vaccine inoculation plays a protective activity against JEV and ZIKV infection (Chen et al., 2020; Zhang et al., 2020). In this study, we found that JEV-E mRNA-LNP immunization could induce IFNγ-secreting T cell response reactive with both JEV and ZIKV peptides. Thus, vaccines constructed using this strategy should have safe profile and have broad application scenarios in many countries/territories, especially in areas where multiple flaviviruses are co-circulating.
Recombinant adenovirus is better than recombinant protein in immune response especially cellular immune response, a property that underscores the feasibility of vaccines based on recombinant adenovirus vectors, as evidenced by extensive human trials. Owing to their non-replicating nature within the host organism, recombinant adenovirus vaccines minimize safety concerns, thereby offering a promising avenue for both prophylactic and therapeutic immunization strategies. Recombinant adenovirus vaccine is currently extensively studied in the prevention of many infectious diseases, including COVID-19, chikungunya fever, and hepatitis C (Agrawal et al., 2019; Lyke et al., 2022; Cao et al., 2022). An mRNA vaccine is a vaccine that prevents or treats a disease by introducing into the body a mRNA that expresses a specific antigen, thereby inducing an immune response against the pathogen or antigenic protein. The unique advantages of mRNA technology are as follows: firstly, due to the standardized process of mRNA vaccine production, rapid development of a new vaccine against emerging pathogen is feasible by simply substituting the mRNA sequence specific to the specific pathogen. This makes the design and production of mRNA vaccines more convenient and cost-effective compared to traditional vaccines (Schlake et al., 2012; Pardi et al., 2018; Linares-Fernandez et al., 2020). Secondly, mRNA sequence inherently possesses adjuvant properties because it can activate innate immune responses to produce cytokines, thereby assisting adaptive immune response. In contrast, traditional protein-based vaccines require additional adjuvants to achieve similar effects (Iavarone et al., 2017). Thirdly, mRNA vaccines, devoid of live viruses, eliminate the risk of infection in vaccine recipients. Moreover, the chemical structure of mRNA, being distinct from DNA, minimizes the likelihood of integration into the host’s DNA genome (Sahin et al., 2014). Given that mRNA is inherently susceptible to degradation by nucleases, and further compounded by its propensity for self-hydrolysis, naked mRNA exhibits instability. Encapsulating mRNA within LNPs serves to shield it from nuclease degradation, concurrently facilitating the delivery of mRNA.
Developing engineered vaccines based on mutations in the FL sequence of the E protein of the JEV is of paramount importance for several reasons. Firstly, there is a considerable geographic overlap between the endemic regions of JEV and ZIKV. In these epidemic regions (particularly Southeast Asian and East Asian countries/territories), the majority of the population has already been infected with JEV or vaccinated with JEV vaccine, and the Abs induced by JEV may have potential ADE effects on subsequent ZIKV infections (Chen et al., 2020). The antibodies generated from JEV vaccination persist in the body longer than the duration of cellular immunity (Wang et al., 2020), which may increase the risk of ADE in individuals infected with heterologous flavivirus. Therefore, it is necessary to optimize the present JEV vaccine. Secondly, individuals with compromised immune systems, such as HIV patients, exhibit weaker cellular immunity, rendering them more susceptible to severe ZIKV infections.
5 Conclusion
In summary, our results indicate that indicated amino acid substitutions within the FL region of the JEV E protein exert a negligible impact on the production of JEV-neutralizing Abs, but, significantly reduce the levels of cross-reactive Abs against ZIKV, thereby serving as a strategic approach in the development of safer JEV vaccines.
Data availability statement
The original contributions presented in the study are included in the article/Supplementary material, further inquiries can be directed to the corresponding author.
Ethics statement
The animal study was approved by the Ethical Committee of Ningbo University. The study was conducted in accordance with the local legislation and institutional requirements.
Author contributions
JgZ: Data curation, Software, Writing – original draft. CH: Software, Validation, Writing – original draft. YL: Formal analysis, Investigation, Methodology, Writing – original draft. MC: Software, Writing – original draft. JiZ: Supervision, Writing – original draft. DC: Visualization, Writing – original draft. HN: Writing – original draft. JW: Funding acquisition, Resources, Writing – review & editing.
Funding
The author(s) declare that financial support was received for the research, authorship, and/or publication of this article. The study was supported by Natural Science Foundation of Zhejiang Province (LY22C010004), National Natural Science Foundation of China (32070162 and 31870159), Natural Science Foundation of Ningbo Municipality (2022J131), One Health Interdisciplinary Research Project of Ningbo University (HY202403), and K. C. Wong Magna Fund in Ningbo University.
Conflict of interest
The authors declare that the research was conducted in the absence of any commercial or financial relationships that could be construed as a potential conflict of interest.
Publisher’s note
All claims expressed in this article are solely those of the authors and do not necessarily represent those of their affiliated organizations, or those of the publisher, the editors and the reviewers. Any product that may be evaluated in this article, or claim that may be made by its manufacturer, is not guaranteed or endorsed by the publisher.
Supplementary material
The Supplementary material for this article can be found online at: https://www.frontiersin.org/articles/10.3389/fmicb.2024.1472824/full#supplementary-material
References
Agrawal, B., Gupta, N., Vedi, S., Singh, S., Li, W., Garg, S., et al. (2019). Heterologous immunity between adenoviruses and hepatitis C virus (HCV): recombinant adenovirus vaccine vectors containing antigens from unrelated pathogens induce cross-reactive immunity against HCV antigens. Cells 8:507. doi: 10.3390/cells8050507
Bardina, S. V., Bunduc, P., Tripathi, S., Duehr, J., Frere, J. J., Brown, J. A., et al. (2017). Enhancement of Zika virus pathogenesis by preexisting antiflavivirus immunity. Science 356, 175–180. doi: 10.1126/science.aal4365
Batchelor, P., and Petersen, K. (2015). Japanese encephalitis: a review of clinical guidelines and vaccine availability in Asia. Trop. Dis. Travel Med. Vaccines 1:11. doi: 10.1186/s40794-015-0013-6
Berneck, B. S., Rockstroh, A., Fertey, J., Grunwald, T., and Ulbert, S. (2020). A recombinant Zika virus envelope protein with mutations in the conserved fusion loop leads to reduced antibody cross-reactivity upon vaccination. Vaccines 8:603. doi: 10.3390/vaccines8040603
Bournazos, S., Gupta, A., and Ravetch, J. V. (2020). The role of IgG Fc receptors in antibody-dependent enhancement. Nat. Rev. Immunol. 20, 633–643. doi: 10.1038/s41577-020-00410-0
Campbell, G. L., Hills, S. L., Fischer, M., Jacobson, J. A., Hoke, C. H., Hombach, J. M., et al. (2011). Estimated global incidence of Japanese encephalitis: a systematic review. Bull. World Health Organ. 89, 766–774. doi: 10.2471/BLT.10.085233
Cao, L., Wang, W., Sun, W., Zhang, J., Han, J., Xie, C., et al. (2022). Construction and evaluation of recombinant adenovirus candidate vaccines for chikungunya virus. Viruses 14:1779. doi: 10.3390/v14081779
Cao-Lormeau, V. M., Blake, A., Mons, S., Lastere, S., Roche, C., Vanhomwegen, J., et al. (2016). Guillain-Barre syndrome outbreak associated with Zika virus infection in French Polynesia: a case-control study. Lancet 387, 1531–1539. doi: 10.1016/S0140-6736(16)00562-6
Chen, D., Duan, Z., Zhou, W., Zou, W., Jin, S., Li, D., et al. (2020). Japanese encephalitis virus-primed CD8+ T cells prevent antibody-dependent enhancement of Zika virus pathogenesis. J. Exp. Med. 217:e20192152. doi: 10.1084/jem.20192152
Chiou, S. S., Crill, W. D., Chen, L. K., and Chang, G. J. (2008). Enzyme-linked immunosorbent assays using novel Japanese encephalitis virus antigen improve the accuracy of clinical diagnosis of flavivirus infections. Clin. Vaccine Immunol. 15, 825–835. doi: 10.1128/CVI.00004-08
Chiou, S. S., Fan, Y. C., Crill, W. D., Chang, R. Y., and Chang, G. J. (2012). Mutation analysis of the cross-reactive epitopes of Japanese encephalitis virus envelope glycoprotein. J. Gen. Virol. 93, 1185–1192. doi: 10.1099/vir.0.040238-0
Crill, W. D., Trainor, N. B., and Chang, G. J. (2007). A detailed mutagenesis study of flavivirus cross-reactive epitopes using West Nile virus-like particles. J. Gen. Virol. 88, 1169–1174. doi: 10.1099/vir.0.82640-0
de Alwis, R., Williams, K. L., Schmid, M. A., Lai, C. Y., Patel, B., Smith, S. A., et al. (2014). Dengue viruses are enhanced by distinct populations of serotype cross-reactive antibodies in human immune sera. PLoS Pathog. 10:e1004386. doi: 10.1371/journal.ppat.1004386
Dirlikov, E., Major, C. G., Mayshack, M., Medina, N., Matos, D., Ryff, K. R., et al. (2016). Guillain-Barré syndrome during ongoing Zika virus transmission—Puerto Rico, January 1–July 31, 2016. MMWR Morb. Mortal Wkly. Rep. 65, 910–914. doi: 10.15585/mmwr.mm6534e1
Fong, Y. D., and Chu, J. J. H. (2022). Natural products as Zika antivirals. Med. Res. Rev. 42, 1739–1780. doi: 10.1002/med.21891
Govero, J., Esakky, P., Scheaffer, S. M., Fernandez, E., Drury, A., Platt, D. J., et al. (2016). Zika virus infection damages the testes in mice. Nature 540, 438–442. doi: 10.1038/nature20556
Gunawardana, S. A., and Shaw, R. H. (2018). Cross-reactive dengue virus-derived monoclonal antibodies to Zika virus envelope protein: Panacea or Pandora’s box? BMC Infect. Dis. 18:641. doi: 10.1186/s12879-018-3572-0
Halstead, S. B., Chow, J. S., and Marchette, N. J. (1973a). Immunological enhancement of dengue virus replication. Nat. New Biol. 243, 24–26
Halstead, S. B., Shotwell, H., and Casals, J. (1973b). Studies on the pathogenesis of dengue infection in monkeys. II. Clinical laboratory responses to heterologous infection. J. Infect. Dis. 128, 15–22. doi: 10.1093/infdis/128.1.15
He, X., Lang, X., Yu, J., Zhu, L., Qin, Z., Liu, X., et al. (2020). The effects of Japanese encephalitis virus antibodies on Zika virus infection. Med. Microbiol. Immunol. 209, 177–188. doi: 10.1007/s00430-020-00658-2
Hills, S., Martin, R., Marfin, A., and Fischer, M. (2014). Control of Japanese encephalitis in Asia: the time is now. Expert Rev. Anti-Infect. Ther. 12, 901–904. doi: 10.1586/14787210.2014.929498
Huang, L., Li, H., Ye, Z., Xu, Q., Fu, Q., Sun, W., et al. (2021). Berbamine inhibits Japanese encephalitis virus (JEV) infection by compromising TPRMLs-mediated endolysosomal trafficking of low-density lipoprotein receptor (LDLR). Emerg. Microbes Infect. 10, 1257–1271. doi: 10.1080/22221751.2021.1941276
Iavarone, C., O’Hagan, D. T., Yu, D., Delahaye, N. F., and Ulmer, J. B. (2017). Mechanism of action of mRNA-based vaccines. Expert Rev. Vaccines 16, 871–881. doi: 10.1080/14760584.2017.1355245
Katzelnick, L. C., Gresh, L., Halloran, M. E., Mercado, J. C., Kuan, G., Gordon, A., et al. (2017). Antibody-dependent enhancement of severe dengue disease in humans. Science 358, 929–932. doi: 10.1126/science.aan6836
Katzelnick, L. C., Narvaez, C., Arguello, S., Lopez Mercado, B., Collado, D., Ampie, O., et al. (2020). Zika virus infection enhances future risk of severe dengue disease. Science 369, 1123–1128. doi: 10.1126/science.abb6143
Kumar, K., Ong, H. K., Tan, W. S., Arshad, S. S., and Ho, K. L. (2021). Immunological analysis of nodavirus capsid displaying the domain III of Japanese encephalitis virus envelope protein. Pharmaceutics 13:1826. doi: 10.3390/pharmaceutics13111826
Lai, C. Y., Williams, K. L., Wu, Y. C., Knight, S., Balmaseda, A., Harris, E., et al. (2013). Analysis of cross-reactive antibodies recognizing the fusion loop of envelope protein and correlation with neutralizing antibody titers in Nicaraguan dengue cases. PLoS Negl. Trop. Dis. 7:e2451. doi: 10.1371/journal.pntd.0002451
Linares-Fernandez, S., Lacroix, C., Exposito, J. Y., and Verrier, B. (2020). Tailoring mRNA vaccine to balance innate/adaptive immune response. Trends Mol. Med. 26, 311–323. doi: 10.1016/j.molmed.2019.10.002
Liu, W., Fu, S., Ma, X., Chen, X., Wu, D., Zhou, L., et al. (2020). An outbreak of Japanese encephalitis caused by genotype Ib Japanese encephalitis virus in China, 2018: a laboratory and field investigation. PLoS Negl. Trop. Dis. 14:e0008312. doi: 10.1371/journal.pntd.0008312
Lyke, K. E., Atmar, R. L., Islas, C. D., Posavad, C. M., Szydlo, D., Paul Chourdhury, R., et al. (2022). Rapid decline in vaccine-boosted neutralizing antibodies against SARS-CoV-2 Omicron variant. Cell Rep. Med. 3:100679. doi: 10.1016/j.xcrm.2022.100679
Mlakar, J., Korva, M., Tul, N., Popovic, M., Poljsak-Prijatelj, M., Mraz, J., et al. (2016). Zika virus associated with microcephaly. N. Engl. J. Med. 374, 951–958. doi: 10.1056/NEJMoa1600651
Muhammad Azami, N. A., Takasaki, T., Kurane, I., and Moi, M. L. (2020). Non-human primate models of dengue virus infection: a comparison of viremia levels and antibody responses during primary and secondary infection among old world and new world monkeys. Pathogens 9:247. doi: 10.3390/pathogens9040247
Musso, D., Nilles, E. J., and Cao-Lormeau, V. M. (2014). Rapid spread of emerging Zika virus in the Pacific area. Clin. Microbiol. Infect. 20, O595–O596. doi: 10.1111/1469-0691.12707
Pardi, N., Hogan, M. J., Porter, F. W., and Weissman, D. (2018). mRNA vaccines—a new era in vaccinology. Nat. Rev. Drug Discov. 17, 261–279. doi: 10.1038/nrd.2017.243
Rasmussen, S. A., Jamieson, D. J., Honein, M. A., and Petersen, L. R. (2016). Zika virus and birth defects—reviewing the evidence for causality. N. Engl. J. Med. 374, 1981–1987. doi: 10.1056/NEJMsr1604338
Richner, J. M., Himansu, S., Dowd, K. A., Butler, S. L., Salazar, V., Fox, J. M., et al. (2017). Modified mRNA vaccines protect against Zika virus infection. Cell 168, 1114–1125.e10. doi: 10.1016/j.cell.2017.02.017
Rockstroh, A., Barzon, L., Pacenti, M., Palu, G., Niedrig, M., and Ulbert, S. (2015). Recombinant envelope-proteins with mutations in the conserved fusion loop allow specific serological diagnosis of dengue-infections. PLoS Negl. Trop. Dis. 9:e0004218. doi: 10.1371/journal.pntd.0004218
Rubin, E. J., Greene, M. F., and Baden, L. R. (2016). Zika virus and microcephaly. N. Engl. J. Med. 374, 984–985. doi: 10.1056/NEJMe1601862
Sahin, U., Kariko, K., and Tureci, O. (2014). mRNA-based therapeutics—developing a new class of drugs. Nat. Rev. Drug Discov. 13, 759–780. doi: 10.1038/nrd4278
Schlake, T., Thess, A., Fotin-Mleczek, M., and Kallen, K. J. (2012). Developing mRNA-vaccine technologies. RNA Biol. 9, 1319–1330. doi: 10.4161/rna.22269
Schuler-Faccini, L., Ribeiro, E. M., Feitosa, I. M., Horovitz, D. D., Cavalcanti, D. P., Pessoa, A., et al. (2016). Possible association between Zika virus infection and microcephaly—Brazil, 2015. MMWR Morb. Mortal Wkly. Rep. 65, 59–62. doi: 10.15585/mmwr.mm6503e2
Sharma, K. B., Vrati, S., and Kalia, M. (2021). Pathobiology of Japanese encephalitis virus infection. Mol. Asp. Med. 81:100994. doi: 10.1016/j.mam.2021.100994
Solomon, T., Dung, N. M., Kneen, R., Thao le, T. T., Gainsborough, M., Nisalak, A., et al. (2002). Seizures and raised intracranial pressure in Vietnamese patients with Japanese encephalitis. Brain 125, 1084–1093. doi: 10.1093/brain/awf116
Tabata, K., Itakura, Y., Ariizumi, T., Igarashi, M., Kobayashi, H., Intaruck, K., et al. (2023). Development of flavivirus subviral particles with low cross-reactivity by mutations of a distinct antigenic domain. Appl. Microbiol. Biotechnol. 107, 7515–7529. doi: 10.1007/s00253-023-12817-5
Tsai, W. Y., Lai, C. Y., Wu, Y. C., Lin, H. E., Edwards, C., Jumnainsong, A., et al. (2013). High-avidity and potently neutralizing cross-reactive human monoclonal antibodies derived from secondary dengue virus infection. J. Virol. 87, 12562–12575. doi: 10.1128/JVI.00871-13
Vogt, M. R., Dowd, K. A., Engle, M., Tesh, R. B., Johnson, S., Pierson, T. C., et al. (2011). Poorly neutralizing cross-reactive antibodies against the fusion loop of West Nile virus envelope protein protect in vivo via Fcγ receptor and complement-dependent effector mechanisms. J. Virol. 85, 11567–11580. doi: 10.1128/JVI.05859-11
Wang, R., Fan, D., Wang, L., Li, Y., Zhou, H., Gao, N., et al. (2020). Neutralizing antibody rather than cellular immune response is maintained for nearly 20 years among Japanese encephalitis SA14-14-2 vaccinees in an endemic setting. Infect. Genet. Evol. 85:104476. doi: 10.1016/j.meegid.2020.104476
Watanabe, S., Chan, K. W., Wang, J., Rivino, L., Lok, S. M., and Vasudevan, S. G. (2015). Dengue virus infection with highly neutralizing levels of cross-reactive antibodies causes acute lethal small intestinal pathology without a high level of viremia in mice. J. Virol. 89, 5847–5861. doi: 10.1128/JVI.00216-15
Westaway, E. G., Brinton, M. A., Gaidamovich, S., Horzinek, M. C., Igarashi, A., Kaariainen, L., et al. (1985). Flaviviridae. Intervirology 24, 183–192. doi: 10.1159/000149642
Zhang, X., Jia, R., Shen, H., Wang, M., Yin, Z., and Cheng, A. (2017). Structures and functions of the envelope glycoprotein in flavivirus infections. Viruses 9:338. doi: 10.3390/v9110338
Zhang, S., Li, L., Woodson, S. E., Huang, C. Y., Kinney, R. M., Barrett, A. D., et al. (2006). A mutation in the envelope protein fusion loop attenuates mouse neuroinvasiveness of the NY99 strain of West Nile virus. Virology 353, 35–40. doi: 10.1016/j.virol.2006.05.025
Keywords: Japanese encephalitis virus, Zika virus, mRNA vaccine, recombinant adenovirus, antibody-dependent enhancement
Citation: Zhu J, He C, Liu Y, Chen M, Zhang J, Chen D, Ni H and Wen J (2024) An engineered Japanese encephalitis virus mRNA-lipid nanoparticle immunization induces protective immunity in mice. Front. Microbiol. 15:1472824. doi: 10.3389/fmicb.2024.1472824
Edited by:
Sreelekshmy Mohandas, National Institute of Virology (ICMR), IndiaReviewed by:
Muhammad Suleman, University of Veterinary and Animal Sciences, PakistanOksana Palchevska, University of Alabama at Birmingham, United States
Copyright © 2024 Zhu, He, Liu, Chen, Zhang, Chen, Ni and Wen. This is an open-access article distributed under the terms of the Creative Commons Attribution License (CC BY). The use, distribution or reproduction in other forums is permitted, provided the original author(s) and the copyright owner(s) are credited and that the original publication in this journal is cited, in accordance with accepted academic practice. No use, distribution or reproduction is permitted which does not comply with these terms.
*Correspondence: Jinsheng Wen, d2Vuamluc2hlbmdAbmJ1LmVkdS5jbg==
†These authors have contributed equally to this work