- 1Graduate Program in Biochemistry and Biophysics, Rensselaer Polytechnic Institute, Troy, NY, United States
- 2Marine Biology Research Division, Scripps Institution of Oceanography, University of California, San Diego, La Jolla, CA, United States
- 3Department of Biological Sciences, Rensselaer Polytechnic Institute, Troy, NY, United States
Introduction: The molecular mechanisms underlying pressure adaptation remain largely unexplored, despite their significance for understanding biological adaptation and improving sterilization methods in the food and beverage industry. The heat shock response leads to a global stabilization of the proteome. Prior research suggested that the heat shock regulon may exhibit a transcriptional response to high-pressure stress.
Methods: In this study, we investigated the pressure-dependent heat shock response in E. coli strains using plasmid-borne green fluorescent protein (GFP) promoter fusions and fluorescence fluctuation microscopy.
Results: We quantitatively confirm that key heat shock genes-rpoH, rpoE, dnaK, and groEL - are transcriptionally upregulated following pressure shock in both piezosensitive Escherichia coli and a more piezotolerant laboratory-evolved strain, AN62. Our quantitative imaging results provide the first single cell resolution measurements for both the heat shock and pressure shock transcriptional responses, revealing not only the magnitude of the responses, but also the biological variance involved. Moreover, our results demonstrate distinct responses in the pressure-adapted strain. Specifically, PgroEL is upregulated more than PdnaK in AN62, while the reverse is true in the parental strain. Furthermore, unlike in the parental strain, the pressure-induced upregulation of PrpoE is highly stochastic in strain AN62, consistent with a strong feedback mechanism and suggesting that RpoE could act as a pressure sensor.
Discussion: Despite its capacity to grow at pressures up to 62 MPa, the AN62 genome shows minimal mutations, with notable single nucleotide substitutions in genes of the transcriptionally important b subunit of RNA polymerase and the Rho terminator. In particular, the mutation in RNAP is one of a cluster of mutations known to confer rifampicin resistance to E. coli via modification of RNAP pausing and termination efficiency. The observed differences in the pressure and heat shock responses between the parental MG1655 strain and the pressure-adapted strain AN62 could arise in part from functional differences in their RNAP molecules.
Introduction
In recent years it has become clear that a majority of microbial life exists in a diverse range of environments, most of which are inhospitable to humans (Merino et al., 2019). Among the characteristics possessed by organisms that thrive in the deep biosphere is piezotolerant or piezophilic growth; the ability to grow or preferentially grow, respectively, at high hydrostatic or lithostatic pressures. In addition to piezotolerant/philic adaptation to grow under high pressure, mesophiles can acquire pressure resistance to survive brief but large pressure shocks (Malone et al., 2006; Van Boeijen et al., 2010; Vanlint et al., 2011, 2012). This process poses a major problem for high pressure processing (HPP) of foods, which is a multibillion-dollar industry projected to grow significantly over time as pressure treatment, unlike temperature sterilization, allows for the retention of food taste and texture (Huang et al., 2017). Beyond mere resistance to pressure, E. coli has been observed to acquire the ability to grow under high pressure in a laboratory setting (Marietou et al., 2015). In this study, adaptive laboratory evolution (ALE) was used to develop E. coli strain AN62, which is capable of growth up to 62 MPa. Only 17 mutations were found in the genome of AN62 (Supplementary Table S1; Allemann et al., 2024).
All cellular components respond to increasing pressure (Bartlett, 2002; Oger and Jebbar, 2010; Gayán et al., 2017). Beyond effects on individual molecules, pressure leads to increased activity of promoters recognized by the general stress response sigma factor RpoS (σS), which has been implicated in pressure resistance (Vanlint et al., 2013b). Notably, sub-lethal pressure shock has been shown to elicit the upregulation of numerous E. coli heat shock proteins (HSPs), including DnaK and GroEL (Welch et al., 1993), as well as the transcriptional induction of HSP genes post sub-lethal pressure shock (Aertsen et al., 2004). Upregulation of HSPs also leads to improved bacterial survival during a lethal pressure shock (Aertsen et al., 2004). The gene for the heat shock regulated extra-cytoplasmic stress response sigma factor rpoE (produces σE or RpoE) is also transcriptionally induced and enhances viability following lethal pressure exposure (Malone et al., 2006). Similar examinations of pressure-induced transcriptional heat shock responses, as well as observations of cross resistance between heat and pressure shocks have reinforced the hypothesis that the heat shock response is important for high pressure adaptation and survival and underscores the importance of proteostasis for bacterial survival and growth under stress (Aertsen and Michiels, 2007; Vanlint et al., 2013a; Gayán et al., 2016).
The ultimate outcome of the heat shock response is to upregulate two key groups of HSPs: the DnaK/DnaJ and GroEL/GroES chaperone systems (Saito and Uchida, 1978; Kusukawa and Yura, 1988; Lipinska et al., 1988). When the proteome is destabilized, DnaK works in tandem with its co-transcribed chaperone, DnaJ, and a nucleotide exchange factor, GrpE, as an unfoldase to disaggregate and partially unfold misfolded or aggregated proteins (Slepenkov and Witt, 2002). In contrast, under homeostatic conditions DnaK sequesters RpoH (σ32), the main heat shock sigma factor, thereby repressing its transcriptional activation activity (Johnson et al., 1989; Straus et al., 1990; Gamer et al., 1992; Gamer et al., 1996). It has also been shown that numerous proteins require DnaK to fold properly or maintain their proper folding (Calloni et al., 2012). GroEL functions as a large multimeric complex with GroES and is required for the folding of several important proteins defined as class IV substrates (Fujiwara et al., 2010) and to refold unfolded or misfolded proteins (Kerner et al., 2005). It has been proposed that DnaK may act as a filter for GroEL selectivity (Kerner et al., 2005; Calloni et al., 2012).
The heat shock response is heavily regulated, particularly at the transcriptional level via the alteration of utilized sigma factors and promoters in HSP gene promoter regions (Figure 1). Thermal induction of HSP genes, including dnaK/dnaJ and groEL/groES (Cowing et al., 1985; Cowing and Gross, 1989), is achieved by a large increase in the quantity of the heat shock sigma factor RpoH (Grossman et al., 1987), a normally very unstable protein (Tilly et al., 1989). The promoter region of the rpoH gene is complex, allowing its expression to be driven by either the main sigma factor RpoD (σ70) or by RpoE (σE) (Erickson et al., 1987; Wang and Kaguni, 1989a), a secondary heat shock sigma factor (Rouviere et al., 1995). The regulation of RpoE at the transcriptional and post translational levels depends on changes in the amount of unfolded proteins; particularly those associated with the cell membrane and periplasm (Raina et al., 1995; Missiakas et al., 1997). Like that of rpoH, the rpoE promoter region is controlled by multiple sigma factors, RpoD, RpoS and RpoE, along with sigma factors unrelated to the heat shock response (Klein et al., 2016). It is important to note that rpoD also experiences a heat shock response, whereby its expression can be driven by RpoE or RpoH in addition to RpoD (Burton et al., 1983; Taylor et al., 1984; Grossman et al., 1985). Note that other transcription factors, unrelated to the heat shock response and not shown in Figure 1, also contribute to the regulation of alternative sigma factor and HSP transcriptional regulation (Wang and Kaguni, 1989b; Kallipolitis and Valentin-Hansen, 1998; Landini et al., 2014; Klein et al., 2016; Ishihama, 2017; Rome et al., 2018).
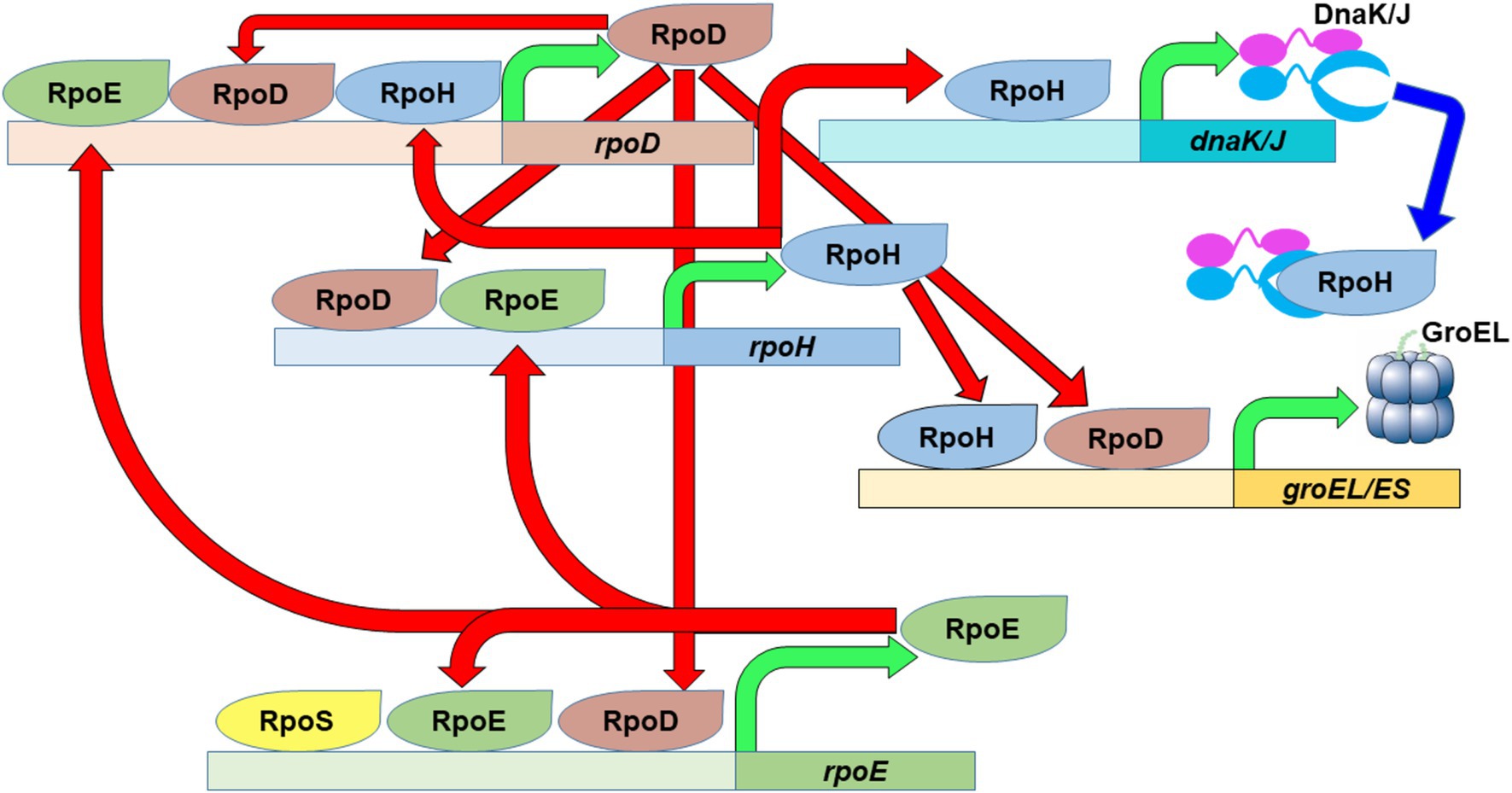
Figure 1. The heat shock response in E. coli. The main housekeeping sigma factor, RpoD, as well as the primary (RpoH) and secondary (RpoE) heat shock sigma factors possess complex promoter regions that allow them to fine tune their expression based on the needs of the cell. An increase in the amount of RpoH will eventually lead to increased expression of specific chaperon systems (DnaK/J and GroEL/ES) in order to stabilize the proteome after temperature upshift. Green arrows depict transcription of the designated gene to produce the specified protein product. Red arrows depict the transcriptional activation activity of the specified sigma factors. Blue arrow depict depicts repression activity of the specified chaperones.
In the present study, we sought to confirm and quantitatively characterize, at single cell resolution, the pressure-induced transcriptional heat shock response in E. coli. To this end we generated plasmid borne green fluorescent protein (GFP) promoter fusion constructs of four key heat shock genes: those encoding the chaperones, dnaK and groEL, and the two alternative sigma factors, rpoE and rpoH. We then quantified the transcriptional response of each promoter to heat and pressure shock in both the E. coli K-12 strain MG1655 (Blattner et al., 1997) and its derived high pressure-adapted strain, AN62 (Marietou et al., 2015; Allemann et al., 2024). Quantification of the absolute GFP concentration in single cells prior to and after heat or pressure shock was carried out using a particle counting imaging approach called two photon scanning number and brightness microscopy (sN&B), which was specifically designed to perform quantitative measurements in live cells with minimal photobleaching, low background fluorescence, single cell resolution, and the ability to differentiate between an increase in the number of cells vs. an increase in the fluorescence intensity per cell (Digman et al., 2008; Ferguson et al., 2011, 2012; Royer, 2019). Our results confirmed that E. coli mounts a heat shock response at the transcriptional level when exposed to pressure shock, and that for some promoters the response to pressure shock differs in magnitude from the response to heat shock. We also found that upregulation of PrpoH was consistently larger after pressure shock compared to heat shock in both MG1655 and AN62, which underscores the importance of the pressure-induced heat shock response, even for organisms that can grow under high pressure. Finally, we show that the transcriptional pressure shock response is distinct for the chaperone genes dnaK and groEL in E. coli MG1655 and AN62. Pressure-induced dnaK upregulation is stronger in MG1655, while that of groEL is more pronounced in AN62. These observations suggest that producing more GroEL than DnaK might provide a selective advantage for growth under pressure.
Materials and methods
Strain construction
GFP transcriptional fusions were constructed for four major heat shock genes: the chaperone-encoding dnaK and groEL genes, and the alternate σ factor-encoding rpoE and rpoH genes. They were cloned into plasmid pMS201, which is maintained as a low copy number plasmid (Zaslaver et al., 2006; Table 1). For each reporter fusion, the full-length promoter region, encompassing all known promoters for each gene, was utilized (hereafter referred to as promoter fusion for simplicity). All plasmids were purchased from Horizon Discovery and transformed into E. coli K12 strains MG1655 or AN62. Cells were made chemically competent via the Transformation Storage Solution (TSS) method (Chung and Miller, 1993). pMS201 utilizes 50 μg/mL kanamycin for plasmid selection.
An exception was the transcriptional fusion of GFPmut2 being driven by the arabinose inducible promoter PBAD. It was generated via Gibson assembly using the plasmid pBAD24 as a backbone and GFPmut2 as the insert and transformed into E. coli strain MG1655. Unlike pMS201, pBAD24 utilizes 100 μg/mL ampicillin for selection.
The next day, transformants were re-streaked onto selective plates containing the necessary antibiotic and verified by PCR. Clonal isolates verified by PCR were grown to mid-upper log phase and 1 mL of culture was preserved in 25% (v/v) glycerol and stored at −80°C. Plasmid sequence integrity was also verified via whole plasmid sequencing (Primordium Labs) (Supplementary Table S2) after being harvested from 1 mL of mid-upper log phase cultures from clonal isolates using a Zymo Research ZR-Plasmid Miniprep™-Classic kit. Unless otherwise stated, all culturing and recovery steps were done in Luria Broth (LB) containing per liter 10 g Tryptone, 10 g sodium chloride, and 5 g yeast extract supplemented with the correct antibiotic for plasmid selection.
Cell culture
E. coli AN62 and its mesophilic ancestor MG1655 were used for sN&B experiments. Unless otherwise stated, all culturing was done in LB medium supplemented with 50 μg/mL kanamycin. For heat shock experiments, cells were grown at 30°C at 180 rpm. For pressure shock experiments, cells were grown at 37°C at 180 rpm to decrease the likelihood of high-pressure inactivation (Aertsen et al., 2004). After overnight growth, MG1655 cells were diluted 1:100 fresh and AN62 cells were diluted 1:10 into medium. AN62 cells were diluted significantly less than MG1655 to skip their long lag phase (Marietou et al., 2015). All cultures were allowed to grow to mid-log phase (OD600 nm = 0.4–0.5). At mid log phase, two aliquots of 600 μL of cells were removed from the culture. One aliquot was prepared for imaging without any shock, while the other aliquot was subjected to either a heat or pressure shock. We verified balanced growth conditions, as results were similar when cells were grown after a 1:10,000-fold dilution.
Heat and pressure shocks
For heat shock, cells were placed in a 42°C water bath for 15 min and then prepared for imaging. For pressure shock, cells were transferred to a quartz cuvette and sealed with a DuraSeal cap and an O-ring. The cuvette was then placed inside a high-pressure cell and pressurized to 60 MPa (600 bar). The setup for the high-pressure cell has been previously described (Jenkins et al., 2018). The pressurization was performed in increments of 20 MPa (200 bar), with a brief equilibration period of 5 s at each pressure. Cells were pressurized for 15 min and kept at 34°C (The limit of the temperature regulation unit attached to the high-pressure cell). Depressurization was performed in the same manner as pressurization. After pressure shock, cells were transferred from the cuvette to a sterile Eppendorf tube and prepared for imaging.
Cell preparation for imaging
Both aliquots were prepared for imaging using an agarose pad setup that has been previously described (Ferguson et al., 2011; Supplemental methods) with modifications. Briefly, cells were centrifuged at 7000 rpm for 2 min and resuspended in 3–5 μL of minimal M9 medium supplemented with 0.4% glucose (Supplementary Table S3). Cells were then plated on a 66 μL, 2% agarose pad made with the same supplemented M9 medium. Cells were allowed to equilibrate on the surface of the pad for 5 min, then a poly lysine coated No. 1 coverslip (VWR) was placed over top of the cells for 1 min before sealing the cells inside. The cells were then placed in an autofluor holder for imaging.
Because of the short time frame (under 10 min) between the end of the shock and mounting on the microscope, it is extremely unlikely that there was any significant amount of growth of the cells. This prevented any significant loss of GFP due to dilution from cell division. It is also unlikely there was any significant protease degradation since GFP has been observed to possess a long half-life in cells (Tombolini et al., 2006). This timeline also allows for rapid measurement of the response that occurred during or immediately after the shock and avoids any pleotropic effects due to differences in growth rates between MG1655 and AN62.
Two photon excitation fluorescence fluctuation microscopy
Imaging was performed on an ISS Alba fast scanning mirror fluctuation microscope (ISS, Champaign, IL) equipped with 2-photon laser excitation (Mai Tai Ti: Sapphire, Newport-SpectraPhysics, Mountain View, CA). 930 nm excitation light (with an average power of 15.2 mW) was focused through a 60 × 1.2NA water immersion objective (Nikon APO VC) onto a No. 1 coverslip. All images were 20 μm x 20 μm. A 735 nm low-pass dichroic filter (Chroma Technology Corporation, Rockingham, VT, USA) was used to filter infrared light from the emitted light. Emitted light was further filtered with a 530/43 nm bandpass filter just before reaching the detector - an avalanche photodiode (Perkin Elmer). At the start of each experiment, 28 nM fluorescein was used to assess the quality of the laser alignment through Fluorescence Correlation Spectroscopy (FCS) and by determining the effective volume of the 2-photon point spread function (PSF) at both 780 nm and at 930 nm (12 mW and 49 mW excitation power, respectively).
All imaging was performed at atmospheric pressure, precluding reversible pressure dependent fluorescence intensity changes in GFP itself. Moreover, GFP is extremely pressure stable and does not unfold until above 1,050 MPa (10 kbar) (Ehrmann et al., 2001; Scheyhing et al., 2002). In the present work, pressure shocks were performed at much lower pressure, 60 MPa. Moreover, we have shown previously that there is no irreversible effect of pressure up to 100 MPa on the molecular brightness (= quantum yield or counts per second per molecule) of GFP (Bourges et al., 2020) in live bacterial cells.
Scanning number and brightness (sN&B) imaging and analyses
sN&B was developed to allow for quantitative analysis of the number of fluorescent molecules in living cells (Digman et al., 2008; Ferguson et al., 2011, 2012). To perform sN&B measurements, a series of very rapid raster scans are obtained (for these experiments, 25 frames were acquired) for each field of view (FOV). A pixel dwell time of 40 μs was used, which is faster than the diffusion time of GFP in cells (~5 μm2/s,) (Ferguson et al., 2011) to allow for measurement of the fluorescence fluctuations. The average fluorescence intensity, <FGFP>, of the diffusing GFP molecules and the variance of their fluorescence, σ2, were used to calculate the shot noise corrected molecular brightness of GFP (eGFP) at each pixel in each bacterial cell according to equation 1.
Then eGFP was averaged across all bacterial cells to provide the average molecular brightness of GFP (<eGFP>). Using the average molecular brightness of GFP, the absolute number of GFP molecules (nGFP) within the effective volume (Veff) defined by the point spread function (PSF) of the excitation laser was determined for each pixel in each bacterial cell from the average fluorescence over all scans at that pixel according to equation 2.
Values of nGFP were averaged over all quantified pixels within each cell, <nGFP>, and correspond to the absolute concentration of GFP (number of GFP molecules in the Veff) in each cell.
In some cases, GFP expression was so high that it saturated the detectors. In these cases, the excitation intensity was lowered such that the detected fluorescence intensity was sufficiently below the limit of the detector. To accurately compare data acquired with different excitation intensities, the fluorescence intensities were first normalized to the highest excitation intensity according to equation 3.
where Fnorm is the normalized fluorescence intensity, Fi is the initial fluorescence intensity, Enorm is the normalized excitation intensity, and Ei is the initial excitation intensity. Background subtraction and sN&B analyses (see below) were only carried out after fluorescence intensity normalization, as the background fluorescence was always measured with Enorm.
sN&B analyses were carried out using the Patrack software (Espenel et al., 2008) to manually segment cells for single cell resolution. Prior to calculation of GFP brightness and number, background fluorescence, determined from imaging strain MG1655 or AN62 with no GFP producing plasmids, was subtracted from the fluorescence intensity at each pixel. To avoid artfacts that arise from imaging along the boundaries of cells due to the diffraction-limited PSF, only the central pixels were used to determine the average fluorescence intensity in each cell. The distribution of the <nGFP> value for each cell from all FOV for a given condition was then plotted and compared between populations of cells that received no shock or a heat or pressure shock. From the averages of the histogram distributions, the percent change in promoter activity after either heat or pressure shock was calculated and averaged for 3 separate experiments for each strain and condition. Pairwise T tests were then performed for all promoter fusion strains under all conditions (Supplementary Table S4).
Protein structure visualization
Protein structure files were taken from the protein databank (PDB). Files were then viewed in pymol (The PyMOL Molecular Graphics System, Version 3.0 Schrödinger, LLC), and key residues were emphasized using visualization tools in the software.
Results
Quantification of the transcriptional response to heat shock for heat shock genes
To quantify the transcriptional response to heat shock we performed 2-photon sN&B imaging on E. coli strains MG1655 and AN62 bearing GFP plasmid-borne promoter fusions of the four major heat shock genes dnaK, groEL, rpoE, and rpoH. Because the mRNA and protein produced is the same (GFP), beyond short 5’-UTR regions specific to each promoter, for all promoters in both strains, these experiments monitor directly changes in promoter activity, as opposed to differences in the amount of RNA transcript or HS protein produced. The heat shock transcriptional response was characterized before (at 30°C) and after a 15-min 42°C heat shock similar to previous heat shock studies (Gross et al., 1984; Taylor et al., 1984; Grossman et al., 1984; Erickson et al., 1987). Single cell resolution was achieved via manual cell segmentation as described in the Methods section. Dividing the fluorescence intensity averaged over all central pixels in each cell by the molecular brightness of GFP, eGFP, calculated using equation 1, yielded the average absolute number of GFP molecules in the Veff in each cell, <nGFP> equation 2 which corresponds to the absolute concentration of GFP in each cell. Both MG1655 (Figure 2A) and AN62 (Figure 2C) exhibited basal levels of expression prior to heat shock due to RNA polymerase recruitment via σ70 (or σ32 in the case of dnaK). In some cases, GFP expression was so high that the excitation intensity was decreased to avoid oversaturation of the detector. To ensure comparability between all promoter fusions, fluorescence intensity values were normalized to the highest excitation intensity using equation 3 (see Methods section). Additionally, since these strains bear the promoter GFP fusions on plasmids, the initial expression levels (intensities) for repeat experiments varied, as well as between strains and promoters. Thus, intensities could not be compared either between promoters or strains. Rather, it is the magnitude of the fractional change in expression after shock that is significant and should be compared.
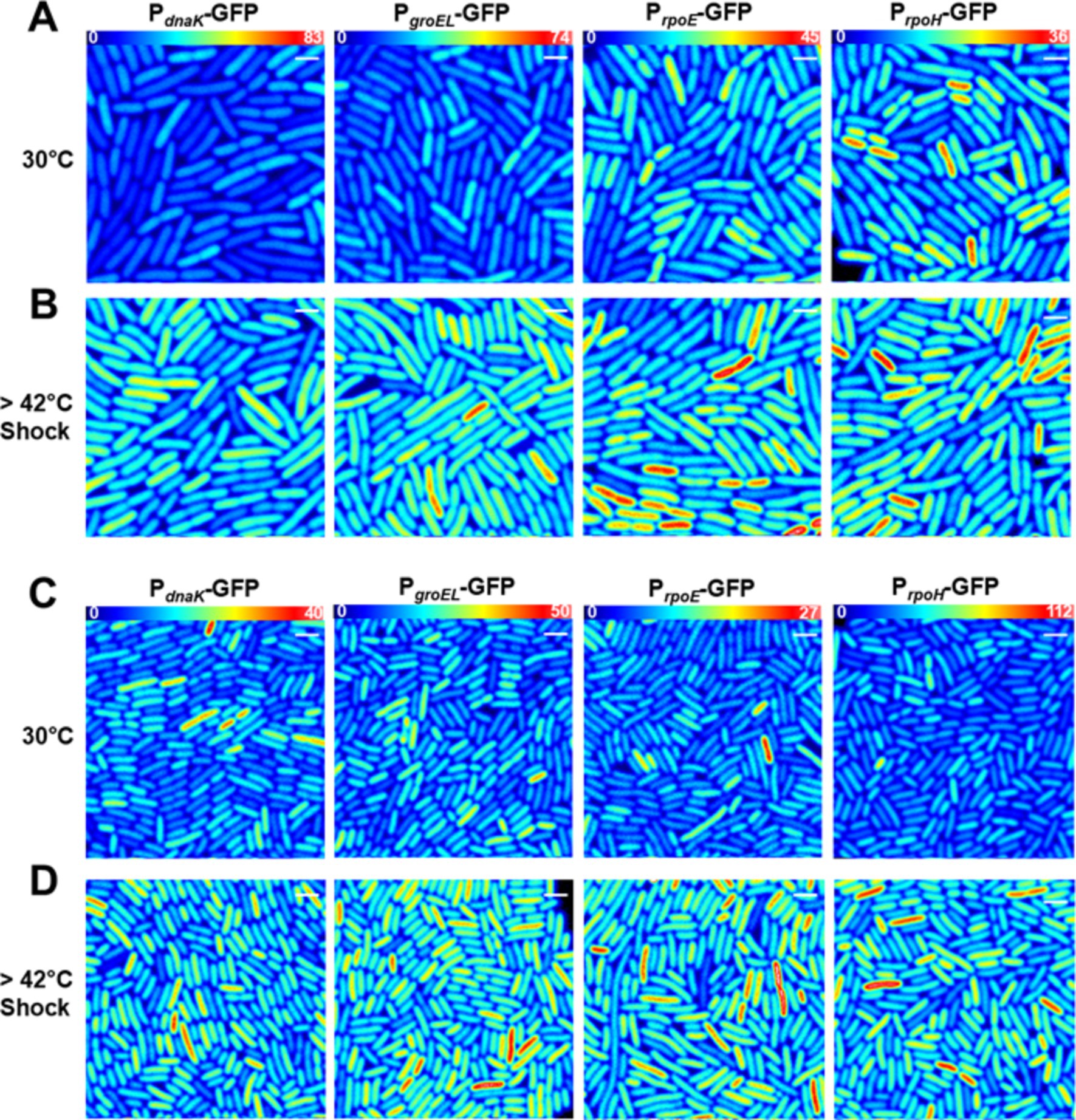
Figure 2. Transcriptional heat shock response in single cells. Results are presented in (A,B) E. coli MG1655 or (C,D) the E. coli AN62 strain. Representative fluorescence intensity images for each promoter fusion after growth at 30°C (A,C) without any shock and (B,D) after a 15-min, 42°C heat shock. Full intensity scales are (A,B) MG1655 PdnaK (0–83), MG1655 PgroEL (0–74), MG1655 PrpoE (0–45), and MG1655 PrpoH (0–36). (C,D) AN62 PdnaK (0–40), AN62 PgroEL (0–50), AN62 PrpoE (0–27), and AN62 PrpoH (0–112). Spatial scale bars (white) are 2 μm.
After heat shock, an increase in promoter activity, as evidenced by an increase in the value of <nGFP > for each cell, was observed for all promoter fusion constructs in both the MG1655 (Figure 2B) and AN62 (Figure 2D) strains. Histograms of <nGFP > for all promoter fusion constructs in both the MG1655 and AN62 strains showed a clear increase in expression upon heat shock (Figure 3). Only the PdnaK and PrpoE promoter fusions exhibited any significant change in the width of the distributions, corresponding to an increase in biological noise after heat shock (Figures 3A,C). Interestingly, the heat shock transcriptional responses of the chaperone promoters, PdnaK (47%) and PgroEL (45%) were stronger than those of the alternative sigma factor promoters, PrpoH (21%) and PrpoE (28%) (p values in Supplementary Table S4; Figure 4). The magnitudes of the transcriptional heat shock responses observed here are consistent with previous studies (Erickson et al., 1987; Riehle et al., 2003; Gunasekera et al., 2008; Ying et al., 2013, 2015; Kim et al., 2020). Since the responses are transient, the actual timing of our measurements after heat shock (~8–10 min) could impact the measured magnitude of the response in comparison to prior results. Note also that post-transcriptional (protein level) HS responses have been shown to be much larger than transcriptional HS responses (Lemaux et al., 1978; Herendeen et al., 1979; Erickson et al., 1987). In contrast to the parental strain, in AN62, the heat shock response of PdnaK (27%) was only about half as large as that of PgroEL (50%) and was also significantly smaller than the responses of both alternative sigma factor promoters, PrpoH (36%) and PrpoE (37%) (Figure 4; p values in Supplementary Table S4). Comparing AN62 to MG1655, PdnaK was upregulated much less after heat shock in the pressure-adapted strain, and the promoters for the alternative sigma factors, PrpoH and PrpoE, were upregulated significantly more (Supplementary Table S4). Taken together, all promoter fusions in both the MG1655 and AN62 strains exhibited robust, yet distinct, transcriptional heat shock responses.
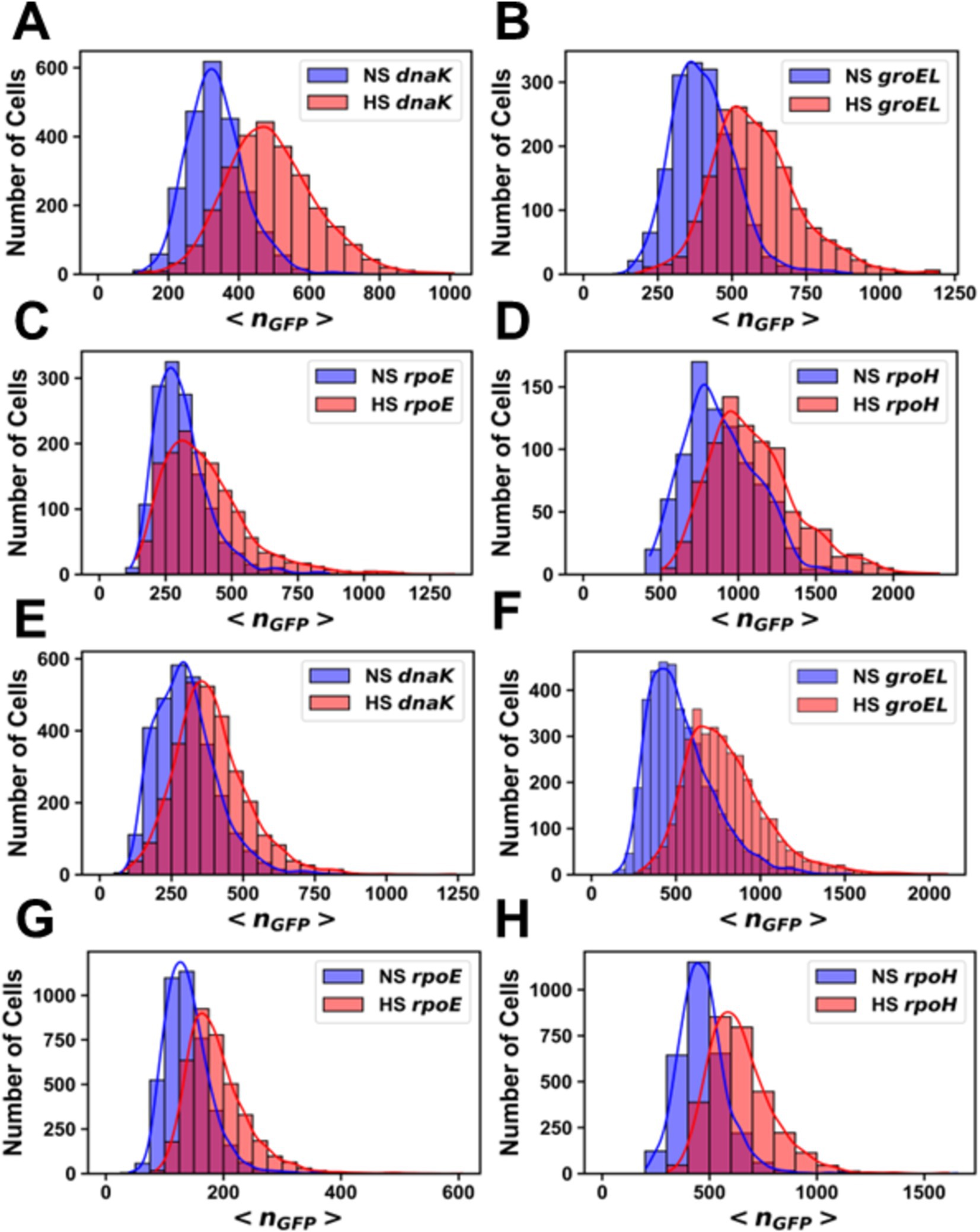
Figure 3. Histograms of the number of molecules of GFP per cell before and after heat shock. Promoter fusions for (A) MG1655 PdnaK, (B) MG1655 PgroEL, (C) MG1655 PrpoE, (D) MG1655 PrpoH, (E) AN62 PdnaK, (F) AN62 PgroEL, (G) AN62 PrpoE and (H) AN62 PrpoH. Cells that received a heat shock (HS) are colored red, and cells that did not receive a heat shock are colored blue (NS). Cells were grown at 30°C prior to heat shock at 42°C for 15 min. The absolute numbers of GFP molecules were determined by sN&B analysis. Note that data are plotted on different x and y axes for different experiments due to differences in basal levels (plasmid copy number and intrinsic promoter activity). Axes have been optimized to allow comparison of the shock vs. no shock samples.
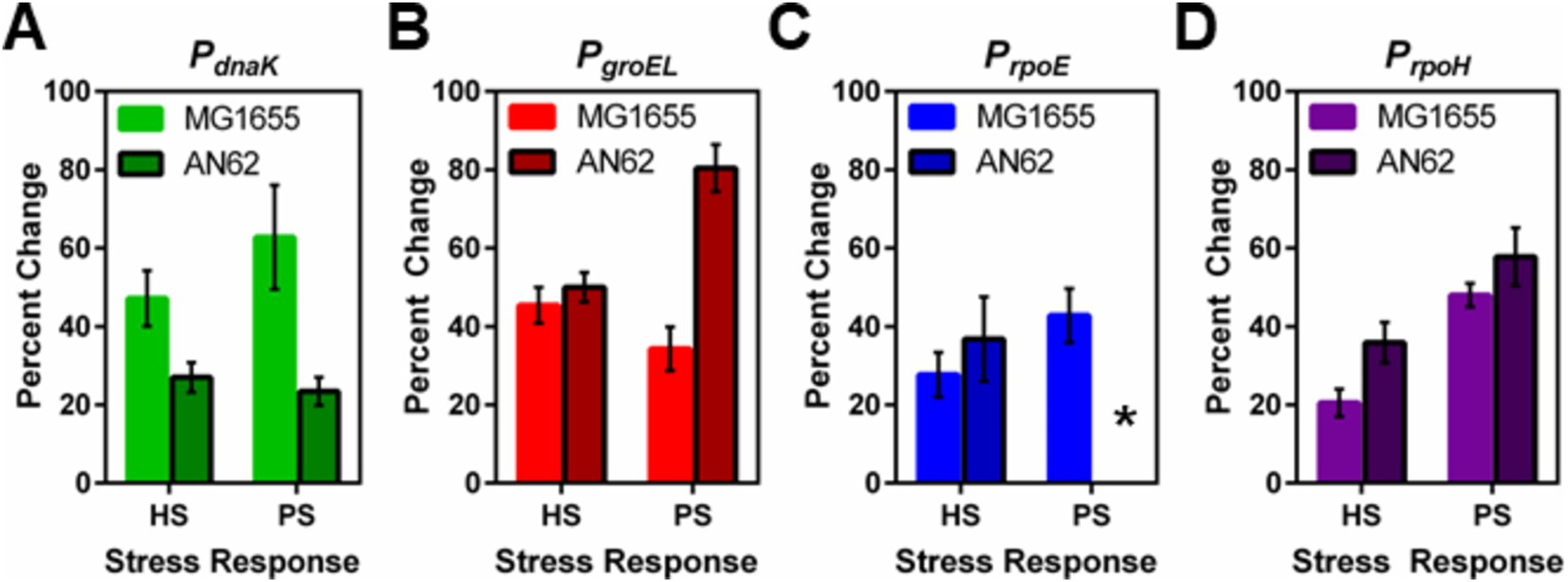
Figure 4. Comparison between the heat and pressure shock responses in E. coli MG1655 and AN62. The percent change in the number of molecules of GFP produced after heat and pressure shocks are compared for the promoter fusions for (A) PdnaK, (B) PgroEL, (C) PrpoE, and (D) PrpoH. Because of the stochastic response to pressure shock for the PrpoE promoter in AN62, no percent change was calculated, indicated by the asterisk. Error bars are one standard deviation of the average of three biological replicates.
Heat shock genes exhibit a transcriptional response to pressure shock
It has been reported that E. coli mounts a heat shock response after a pressure shock (Welch et al., 1993; Aertsen et al., 2004). To quantify this pressure-induced heat shock response, each promoter fusion strain was subjected to a 15-min 60 MPa pressure shock after growth at 37°C. The magnitude of the pressure shock, 60 MPa, was chosen because it is a sub-lethal pressure shock for MG1655 E. coli and is just below the maximum pressure at which the piezotolerant AN62 strain will grow (Marietou et al., 2015). Because AN62 is piezotolerant and not piezophilic, we hypothesized that a 60 MPa pressure shock would still act as a stressor for this strain. Similar to the results above for heat shock, all promoters exhibited basal levels of transcriptional activity (Figures 5A,C) when grown at 0.1 MPa (atmospheric pressure), although as noted above, differences in plasmid copy numbers between strains and within strains for different experiments precludes direct comparison of the basal levels. In general, fluorescence intensity values for basal expression were higher at 37°C compared to 30°C (Figures 3, 6). Due to the especially large amount of basal GFP expression from some promoters, the excitation intensity was lowered to avoid saturation of the detectors and the fluorescence intensity was normalized equation 3. Note that raw intensity values are shown in the images. After pressure shock and return to atmospheric pressure, the absolute concentration of GFP, <nGFP>, produced from all promoter fusions increased in both MG1655 and AN62, as indicated by the warmer colored cells in the fluorescence intensity heat map images (Figures 5B,D). Note that GFP structure and fluorescence is not affected by 60 MPa pressure in vitro (Ehrmann et al., 2001; Scheyhing et al., 2002), and that we have shown previously that GFP fluorescence, itself, is not perturbed by pressure shock in vivo (Bourges et al., 2020). Moreover, we confirmed in this study that pressure-induced upregulation was not a general phenomenon, as expression of GFP from the non-heat shock, PBAD, promoter in presence of arabinose showed no change after pressure shock (Supplementary Figure S1).
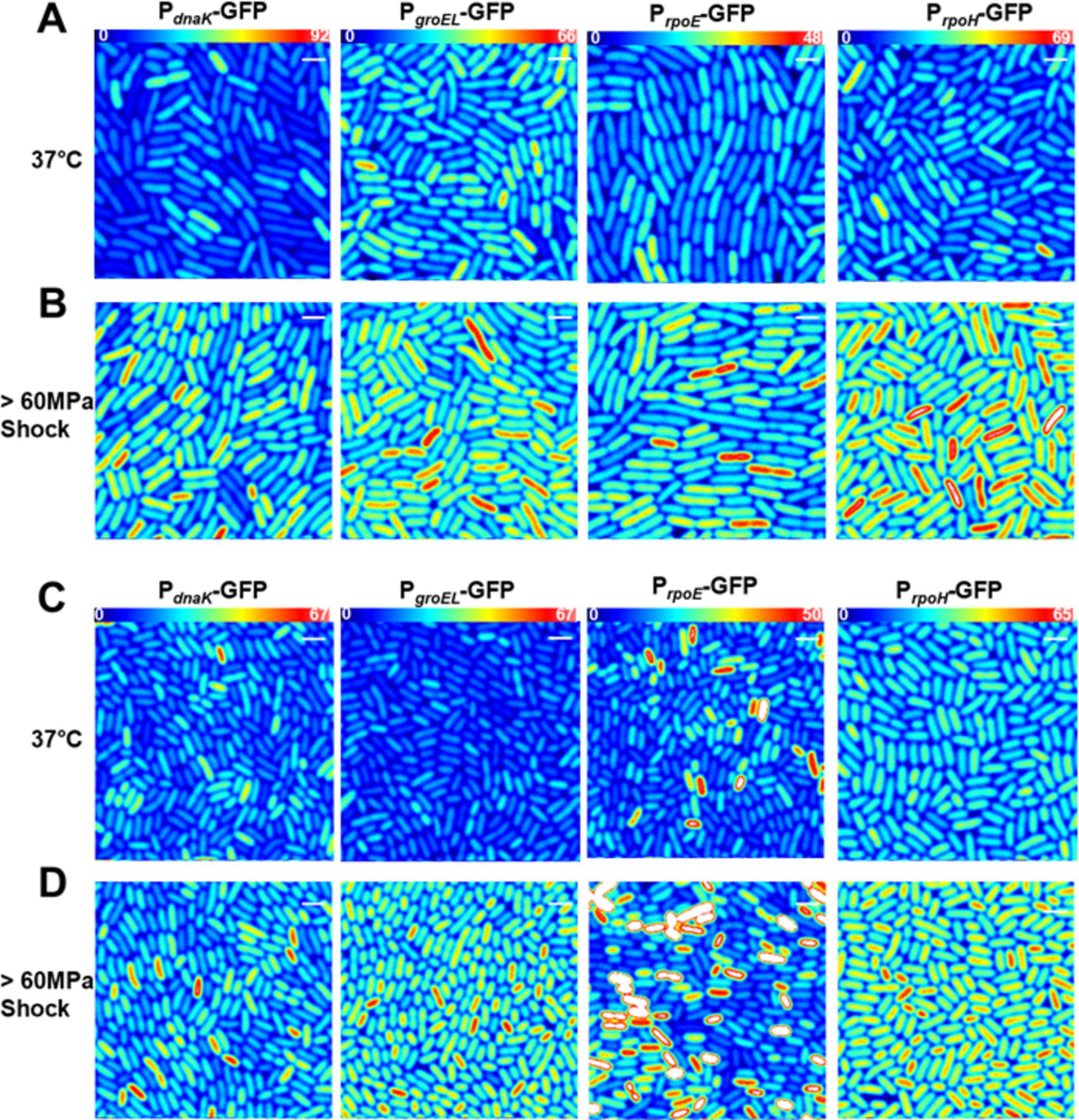
Figure 5. Transcriptional pressure-induced heat shock response in single cells in (A,B) E. coli MG1655 or (C,D) the E. coli AN62 strain. Representative fluorescence Intensity images for each promoter fusion after growth at 37°C (A,C) without any shock and (B,D) after a 15 min, 60 MPa pressure shock. Full intensity scales are (A,B) MG1655 PdnaK (0–92), MG1655 PgroEL (0–66), MG1655 PrpoE (0–48), and MG1655 PrpoH (0–69). (C,D) AN62 PdnaK (0–67), AN62 PgroEL (0–67), AN62 PrpoE (0–50), and AN62 PrpoH (0–65). Spatial scale bars (white) are 2 μm.
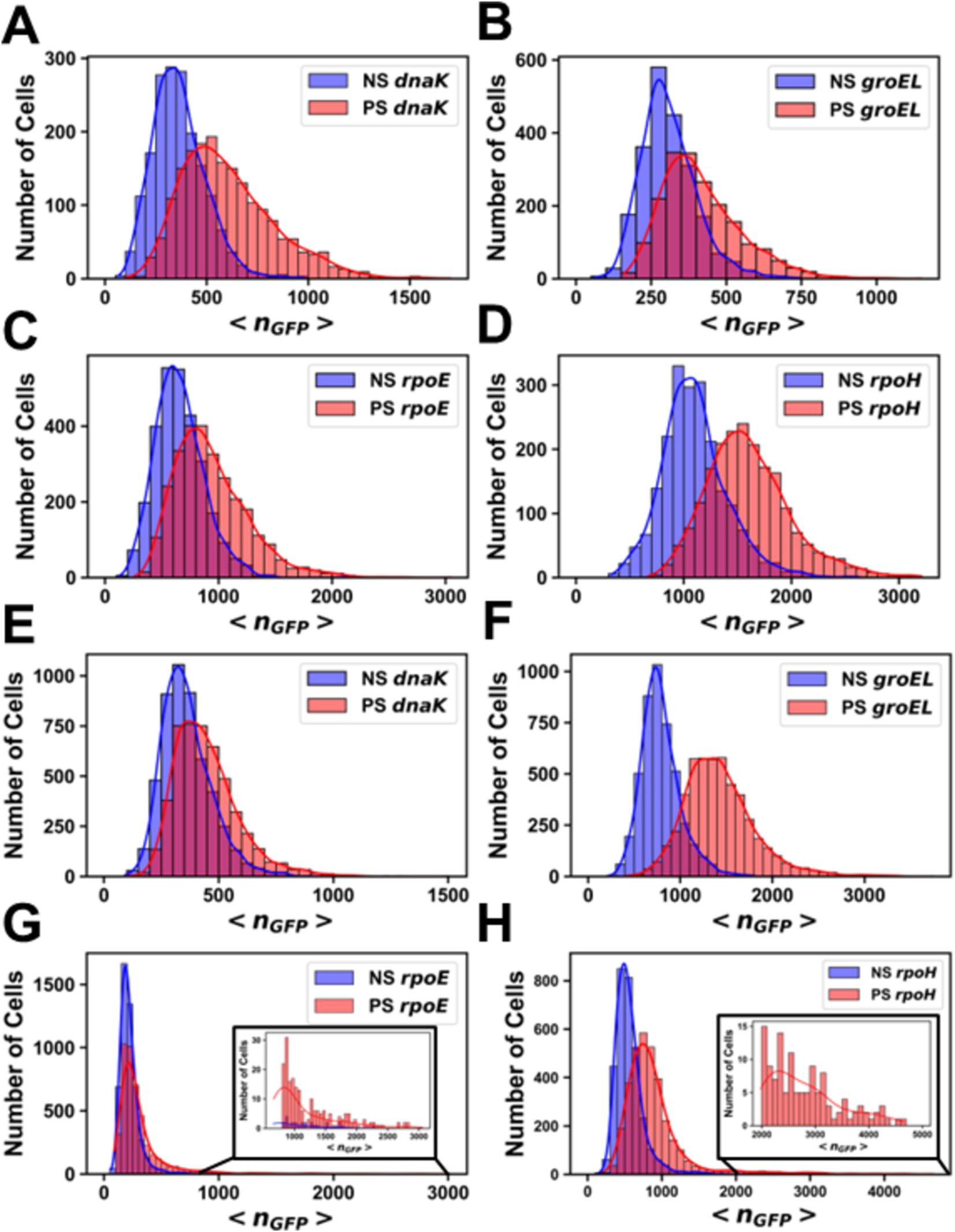
Figure 6. Histograms of the number of molecules of GFP per cell before and after pressure shock. Promoter fusions for (A) MG1655 PdnaK, (B) MG1655 PgroEL, (C) MG1655 PrpoE, (D) MG1655 PrpoH, (E) AN62 PdnaK, (F) AN62 PgroEL, (G) AN62 PrpoE and (H) AN62 PrpoH. Cells that received a pressure shock (PS) are colored red, and cells that did not receive a pressure shock are colored blue (NS). Cells were grown at 37°C prior to pressure shock at 60 MPa for 15 min. The absolute numbers of GFP molecules were determined by sN&B analysis. Note that different x and y axes are used due to the different total numbers of cells at any given nGFP value for each experiment and also the different ranges of protein concentrations measured. Axes have been optimized to allow comparison of the shock vs. no shock samples.
Analysis by sN&B yielded the distributions of <nGFP> per cell before and after pressure shock (Figure 6). In the MG1655 strain after pressure shock, PdnaK activity increased the most (63%), while the increase for PgroEL (34%), PrpoE (43%), and PrpoH (48%) were smaller and similar to each other (p-values in Supplementary Table S4; Figure 4). In addition, all the MG1655 promoter fusions exhibited a significant increase in both the mean and the variance of promoter expression distributions after pressure shock (Figures 6A–D). In strain AN62, as observed for the heat shock response, PdnaK activity increased the least (23%), while PgroEL activity increased the most (80%) (p values in Supplementary Table S4; Figure 4). The increased activity of the alternative sigma factor promoter, PrpoH, (58%) was intermediate. Prior to pressure shock, the cell-to-cell variance in PrpoE activity in strain AN62 was significant (Figure 5C). Furthermore, PrpoE and PrpoH displayed highly stochastic expression patterns after pressure shock, as evidenced by the large tail on the distributions extending far beyond the mean (Figures 5C,D, 6G,H, insets). For strain PrpoE, after pressure shock, many cells exhibited little to no response, while ~30–40% of cells responded very dramatically to pressure, increasing the number of molecules of GFP by up to ~10-fold beyond the mean prior to shock (Figures 6G,H, insets). In particular, because the PrpoE response was so heterogeneous, the percent change in promoter activity is not particularly informative and for this reason is not provided (Figure 4C). Interestingly, in strain AN62, the pressure-induced heat shock response of PgroEL was larger than its response to temperature, with larger increases in both the mean and the variance of the <nGFP> distributions (Figures 4, 6E,F; p values in Supplementary Table S4). Moreover, the responses to pressure shock of the two chaperone promoters were inversed in strain AN62 compared to strain MG1655 (Figures 4A,B). In AN62, PgroEL showed a larger pressure-induced heat shock response than PdnaK while in MG1655 PdnaK experienced a larger increase in promoter activity after pressure shock than PgroEL (p values in Supplementary Table S4).
The heat shock response to pressure is distinct from the response to heat
We were interested to compare the heat-induced heat shock response in both strains to their pressure-induced heat shock responses to probe for any differences in mechanism. For PdnaK, while we did not observe any statistically significant larger pressure-induced heat shock response compared to the heat-induced response in either strain, MG1655 clearly demonstrated a more robust response from PdnaK to both heat and pressure shocks than AN62 (Figure 4A; p values in Supplementary Table S4). PgroEL in the AN62 strain showed a much stronger response to pressure shock than to heat shock, while in MG1655, there was a slightly stronger response to heat shock than pressure shock (Figure 4B; p values in Supplementary Table S4). Only in strain MG1655 did PrpoE exhibit a general upregulation response to pressure, although this promoter responded to heat shock in both MG1655 and AN62 (Figure 4C). In contrast, in strain AN62 the response to pressure of PrpoE was highly stochastic (Figure 6G). Of all the promoter fusions studied, only the promoter for the main heat shock sigma factor, PrpoH, showed a larger response to pressure shock than to heat shock in both strains (Figure 4; p values in Supplementary Table S4).
Discussion
Both Escherichia coli MG1655 and pressure-adapted AN62 exhibit a pressure-induced transcriptional heat shock response
It has been shown previously that in E. coli strain MG1655 there is an increase in DnaK and GroEL protein levels during pressure shock (Welch et al., 1993). A rather long-term transcriptional heat shock response to pressure shock in this strain has been reported for dnaK, lon, and clpPX (Aertsen et al., 2004). We have confirmed and quantified a transcriptional pressure-induced heat shock response for several key heat shock promoters, PdnaK, PgroEL, PrpoH, and PrpoE in both MG1655, as well as for strain AN62, adapted in the laboratory to grow at high pressure (Marietou et al., 2015). We note that the single cell resolution and timescale of our observations (performed <10 min after the shock) is distinct from previous studies. It is important to note, as well, that in our studies, the observed upregulation of promoter activity is not due to a change in mRNA stability [as was the case for transcription from the PrpoH during heat shock (Morita et al., 1999)], since our readout for the activity of all promoters in all conditions is the number of GFP molecules produced (i.e., the same GFP mRNA, differing only in the 5’UTR for each promoter).
The transcriptional response to pressure shock is unique and adaptable
The transcriptional pressure-induced heat shock response is distinct from the heat shock response. For strain MG1655, pressure shock elicited an equivalent (PdnaK) or stronger transcriptional upregulation than heat shock for all promoters. In strain AN62, the transcriptional pressure shock dependent heat shock response was complex. It was found to be more robust for PgroEL and PrpoH than heat shock in either strain, while the response to either heat or pressure shock for PdnaK was the smallest. Interestingly, PrpoE and to a lesser extent, PrpoH, responded stochastically to pressure shock in strain AN62. It is well established that higher pressures disfavor protein aggregation (disaggregation being the main function of DnaK), while favoring protein unfolding (refolding being the main function of GroEL). It is conceivable that, whatever the underlying mechanism, increased GroEL production in strain AN62 could confer some advantage for growth at high pressure.
We wondered what might be the molecular basis for these distinct transcriptional responses to pressure shock in AN62 relative to the parent strain? The most direct mechanisms would implicate transcription, itself, with any differences between promoters arising from differential transcription of their 5’UTR regions, since the coding region corresponds in all cases to GFP (Supplementary Table S2). Strain AN62 harbors only 12 mutations in coding regions of its genome, in addition to five intergenic mutations, three of which are near the gene for tRNA-Gly (Supplementary Table S1; Allemann et al., 2024). Of the mutations in coding sequences, only three affect proteins directly implicated in transcription. The others involve transporters and metabolic enzymes. Of those mutations in genes coding for proteins implicated in transcription, one is a transcriptional activator for the cysteine regulon, which is not involved in the HS response. Another is found in the rho terminator gene. However, rho mutations are unlikely to be implicated in differential HS promoter activity since no rho termination sites are present in the 5’UTR regions of the HS GFP promoter fusions (Supplementary Table S2; Naville et al., 2011).
In contrast, the mutation in rpoB which leads to an amino acid substitution (glutamine to histidine) at position 148 in the β-subunit of RNA polymerase (RNAP) could conceivably contribute to the observed differential responses of the two strains to pressure shock. The Q148➔H mutation is very close to the transcription bubble and the nascent mRNA, as shown in the structure of the E. coli RNAP initiation complex (Figures 7A,B; Zuo and Steitz, 2015). The large number of internal cavities in the RNAP structure (Figure 7C), particularly between the open complex bubble and the mutation, could render this region, and thus RNAP activity, pressure-sensitive, affecting differentially the WT and AN62 enzymes.
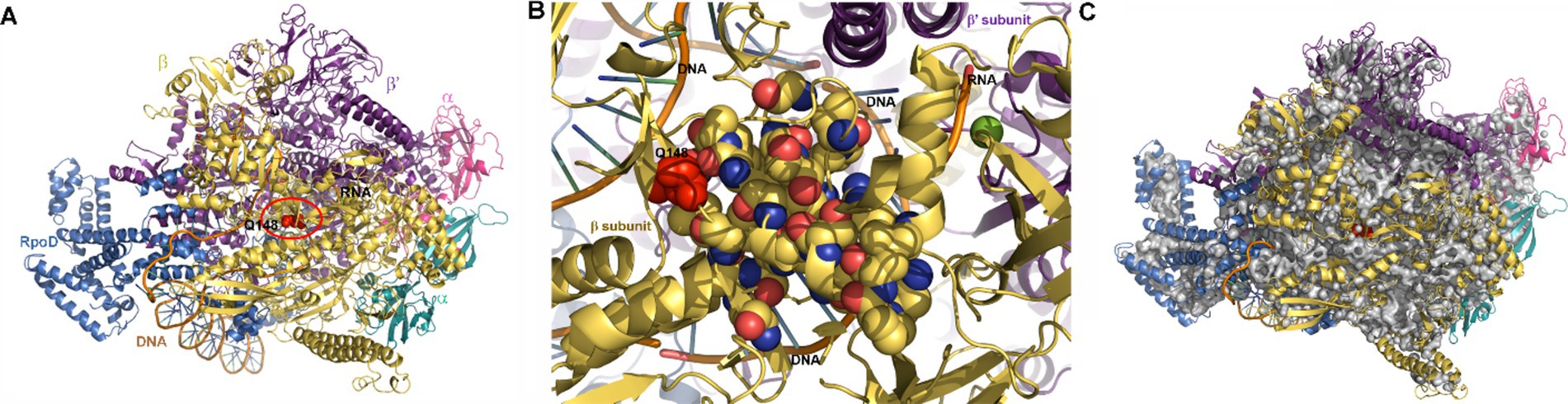
Figure 7. Visualization of RNA polymerase initiation complex. (A) The structure of E. coli RNA polymerase transcription initiation complex (Zuo and Steitz, 2015) visualized using Pymol (see methods section). Note that residue in the β subunit (yellow) of WT RNAP (BQ148) that is mutated to H in AN62 is shown in red spheres and inside a red circle. The α, β and β’ subunits are labeled according to their color. The ω subunit is at the back and not visible in this view. The transcribed and non-transcribed DNA, as well as the nascent RNA are also labeled. (B) Zoomed in image of the cluster of mutations in the RpoB subunit of E. coli RNAP that confer rifampicin resistance. Note that the 21 mutations conferring resistance to rifampicin (yellow CPK spheres), including Q148 in WT RNAP (red spheres and also labeled) are found in the vicinity of the transcription bubble and the mRNA transcript. DNA and RNA are shown in orange ribbon, while bases are shown as blue-green sticks. (C) Internal cavities in RNAP. Cavities were calculated using Pymol with a detection radius of 4 solvent molecules and a detection cutoff of 3 solvent molecules. Cavities are shown in gray and Q148 in red spheres. (A,C) The α subunit of RpoB is colored in magenta, the α’ subunit in aqua, the β subunit is colored yellow, the β’ subunit in violet and the RpoD subunit in blue.
While additional stress response mechanisms could certainly contribute to the distinct pressure-induced heat shock responses in strain AN62, the hypothesis that the Q148H mutation in rpoB might contribute to this phenomenon is supported by the fact that this substitution is one of over 20 single site mutations located within the rifampicin binding site of RNAP known to confer rifampicin resistance to E. coli (Jin et al., 1988; Jun and Gross, 1988; Goldstein, 2014; Molodtsov et al., 2017). The resistance conferring (Rifr) mutations, in addition to altering the affinity for rifampicin, lead to significant changes in transcriptional initiation, pausing, elongation and termination efficiency in absence of drug, and have been used to elucidate RNAP functional mechanisms (Jun and Gross, 1988; Jun and Gross, 1989; Landick et al., 1990; Molodtsov et al., 2017; Meenakshi and Hussain Munavar, 2018). Rifr mutations in the β subunit of RNAP have been shown to have pleiotropic effects, as well. They lead to slow growth (Jun and Gross, 1989; Reynolds, 2000), which is known to be strongly dependent upon transcriptional capacity (Izard et al., 2015; Zhang et al., 2020). Indeed, the growth rate of AN62 is slower than that of MG1655 (Marietou et al., 2015). Moreover, Rifr mutations in the RNAP β subunit have been shown to result in both upregulation and down-regulation of hundreds of genes (Meenakshi and Hussain Munavar, 2018). Interestingly, Rifr mutations (including one, R143L, quite close to the Q148H substitution in AN62) were selected in absence of rifampicin in a laboratory evolution experiment that involved adaptation to growth at high temperature (Rodríguez-Verdugo et al., 2013).
In contrast to similar sizes for AN62 and the parental MG1655 strains reported previously (Marietou et al., 2015), we have observed consistently that the cells in strain AN62 are significantly smaller (50%) when grown at atmospheric pressure. The discrepancy may stem from the fact that cells were fixed before imaging in the previous study. While the mechanism underlying the difference in size is outside the scope of the current study, we offer one possible hypothesis. Cell division in E. coli is licensed by DNA replication, but size is controlled by a division adder, i.e., sufficient accumulation (relative to growth rate) of initiators and precursors required for cell division and maintenance of their production proportional to volume growth (Si et al., 2019). Thus, the smaller size in strain AN62 could result from differential scaling between growth (which, as noted above, is slower than the parental strain) and the rate of production of proteins required for division (e.g., FtsZ). Interestingly, ftsZ and ftsA (which recruits FtsZ to the septum) are among the genes shown to be upregulated by certain Rifr mutations, while the gene for a repressor of division, sulA, was found to be the most strongly downregulated (Meenakshi and Hussain Munavar, 2018). Future work will be aimed at testing the role of the rpoB mutation in supporting growth of strain AN62 at high pressure.
RpoE may act as a pressure sensor for the pressure-induced heat shock response
As noted above, upregulation of PrpoE in AN62 after pressure shock was limited and strongly stochastic compared to MG1655, where it is upregulated robustly. While more work is needed to understand this differential expression pattern for the two strains, we hypothesize the difference may at least partially arise from differences in membrane composition of the two strains. Under homeostatic conditions, RpoE is sequestered at the membrane by the integral membrane protein RseA and is only released upon stress to the membrane and/or extra cytoplasmic/membrane proteins (Peñas et al., 1997; Missiakas et al., 1997; Klein et al., 2016). Membranes are very susceptible to pressure changes (e.g., Lakowicz and Thompson, 1983; Winter and Jeworrek, 2009; Winnikoff et al., 2024), with significant decreases in fluidity resulting from increased pressure. We hypothesize that the pressure-induced decrease in membrane fluidity, could lead to release of RpoE, which would then upregulate rpoH and its own expression. Since the membranes of the AN62 strain contain a larger fraction of unsaturated fatty acids than the MG1655 strain (20.02% 18:1 ω7c vs. 9.5%) (Marietou et al., 2015), the membrane of AN62 may experience less membrane stress due to pressure shock, resulting in the observed limited rpoE upregulation in the pressure adapted strain. The very strong expression in the small fraction of AN62 cells that do respond to pressure shock could arise from differences in RNAP function at high pressure in the pressure-adapted strain.
Concluding remarks
The present results both confirm and quantify a pressure-induced transcriptional heat shock response in E. coli. This response to pressure shock, is distinct from the heat shock response and distinct between the parent and pressure-adapted strain for several promoters. Our results suggest that a rifampicin resistance mutation in the β subunit of RNAP in the pressure-adapted strain could contribute to the differential responses. Another intriguing hypothesis that stems from our observations is that RpoE and its anti-sigma factors may act as a membrane-linked pressure sensors to aid in activating the pressure-induced heat shock response in the parent strain, while the different membrane composition in AN62 could protect the pressure-adapted strain. Taken together, our results point to the importance of transcription and membrane stability in pressure adaptation and provide a foundation for future studies aimed at understanding organismal adaptation to, and even preference for, high pressure.
Data availability statement
The original contributions presented in the study are publicly available. This data can be found here: 10.6084/m9.figshare.27310554.
Author contributions
CC: Data curation, Formal analysis, Investigation, Visualization, Writing – original draft, Writing – review & editing. LF: Investigation, Methodology, Resources, Writing – review & editing. SC: Formal analysis, Investigation, Writing – review & editing. PD: Formal analysis, Investigation, Writing – review & editing. DB: Conceptualization, Funding acquisition, Methodology, Resources, Supervision, Writing – review & editing. CR: Conceptualization, Funding acquisition, Methodology, Project administration, Resources, Supervision, Writing – review & editing.
Funding
The author(s) declare that financial support was received for the research, authorship, and/or publication of this article. This study was supported by National Science Foundation grants MCB 2019471 to CR and MCB 2019455 to DB.
Conflict of interest
The authors declare that the research was conducted in the absence of any commercial or financial relationships that could be construed as a potential conflict of interest.
Publisher’s note
All claims expressed in this article are solely those of the authors and do not necessarily represent those of their affiliated organizations, or those of the publisher, the editors and the reviewers. Any product that may be evaluated in this article, or claim that may be made by its manufacturer, is not guaranteed or endorsed by the publisher.
Supplementary material
The Supplementary material for this article can be found online at: https://www.frontiersin.org/articles/10.3389/fmicb.2024.1470617/full#supplementary-material
References
Aertsen, A., and Michiels, C. W. (2007). The high-pressure shock response in Escherichia coli: a short survey. High Pressure Res. 27, 121–124. doi: 10.1080/08957950601076450
Aertsen, A., Vanoirbeek, K., De Spiegeleer, P., Sermon, J., Hauben, K., Farewell, A., et al. (2004). Heat shock protein-mediated resistance to high hydrostatic pressure in Escherichia coli. Appl. Environ. Microbiol. 70, 2660–2666. doi: 10.1128/AEM.70.5.2660-2666.2004
Allemann, M. N., Ma, S., Sztain, T., Bartholow, T. G., Marshall, I. P. G., McCammon, A., et al. (2024). Adaptation to high pressure; insights from the genome of an evolved Escherichia coli strain with increased Piezotolerance. Available at: https://www.biorxiv.org/content/biorxiv/early/2024/09/25/2024.09.24.613341.full.pdf (Accessed September 25, 2024).
Bartlett, D. H. (2002). Pressure effects on in vivo microbial processes. Biochim. Biophys. Acta 1595, 367–381. doi: 10.1016/S0167-4838(01)00357-0
Blattner, F. R., Plunkett, G. 3rd, Bloch, C. A., Perna, N. T., Burland, V., Riley, M., et al. (1997). The complete genome sequence of Escherichia coli K-12. Science 277, 1453–1462. doi: 10.1126/science.277.5331.1453
Bourges, A. C., Lazarev, A., Declerck, N., Rogers, K. L., and Royer, C. A. (2020). Quantitative high-resolution imaging of live microbial cells at high hydrostatic pressure. Biophys. J. 118, 2670–2679. doi: 10.1016/j.bpj.2020.04.017
Bourges, A. C., Torres Montaguth, O. E., Ghosh, A., Tadesse, W. M., Declerck, N., Aertsen, A., et al. (2017). High pressure activation of the Mrr restriction endonuclease in Escherichia coli involves tetramer dissociation. Nucleic Acids Res. 45, 5323–5332. doi: 10.1093/nar/gkx192
Burton, Z. F., Gross, C. A., Watanabe, K. K., and Burgess, R. R. (1983). The operon that encodes the sigma subunit of RNA polymerase also encodes ribosomal protein S21 and DNA primase in E. coli K12. Cell 32, 335–349. doi: 10.1016/0092-8674(83)90453-1
Calloni, G., Chen, T., Schermann, S. M., Chang, H. C., Genevaux, P., Agostini, F., et al. (2012). DnaK functions as a central hub in the E. coli chaperone network. Cell Rep. 1, 251–264. doi: 10.1016/j.celrep.2011.12.007
Chung, C. T., and Miller, R. H. (1993). Preparation and storage of competent Escherichia coli cells. Methods Enzymol. 218, 621–627. doi: 10.1016/0076-6879(93)18045-E
Cowing, D. W., Bardwell, J. C., Craig, E. A., Woolford, C., Hendrix, R. W., and Gross, C. A. (1985). Consensus sequence for Escherichia coli heat shock gene promoters. Proc. Natl. Acad. Sci. U.S. A. 82, 2679–2683. doi: 10.1073/pnas.82.9.2679
Cowing, D. W., and Gross, C. A. (1989). Interaction of Escherichia cob RNA polymerase holoenzyme containing σ32 with heat shock promoters DNase I Footprinting and methylation protection. J. Mol. Biol. 210, 513–520. doi: 10.1016/0022-2836(89)90127-7
Digman, M. A., Dalal, R., Horwitz, A. F., and Gratton, E. (2008). Mapping the number of molecules and brightness in the laser scanning microscope. Biophys. J. 94, 2320–2332. doi: 10.1529/biophysj.107.114645
Ehrmann, M. A., Scheyhing, C. H., and Vogel, R. F. (2001). In vitro stability and expression of green fluorescent protein under high pressure conditions. Lett. Appl. Microbiol. 32, 230–234. doi: 10.1046/j.1472-765X.2001.00892.x
Erickson, J. W., Vaughn, V., Walter, W. A., Neidhardt, F. C., and Gross, C. A. (1987). Regulation of the promoters and transcripts of RpoH, the Escherichia coli heat shock regulatory gene. Genes Dev. May, 419–432. doi: 10.1101/gad.1.5.419
Espenel, C., Margeat, E., Dosset, P., Arduise, C., Le Grimellec, C., Royer, C. A., et al. (2008). Single-molecule analysis of CD9 dynamics and partitioning reveals multiple modes of interaction in the Tetraspanin web. J. Cell Biol. 182, 765–776. doi: 10.1083/jcb.200803010
Ferguson, M. L., Le Coq, D., Jules, M., Aymerich, S., Declerck, N., and Royer, C. A. (2011). Absolute quantification of gene expression in individual bacterial cells using two-photon fluctuation microscopy. Anal. Biochem. 419, 250–259. doi: 10.1016/j.ab.2011.08.017
Ferguson, M. L., Le Coq, D., Jules, M., Aymerich, S., Radulescu, O., Declerck, N., et al. (2012). Reconciling molecular regulatory mechanisms with noise patterns of bacterial metabolic promoters in induced and repressed states. Proc. Natl. Acad. Sci. U.S. A. 109, 155–160. doi: 10.1073/pnas.1110541108
Fujiwara, K., Ishihama, Y., Nakahigashi, K., Soga, T., and Taguchi, H. (2010). A systematic survey of in vivo obligate chaperonin-dependent substrates. EMBO J. 29, 1552–1564. doi: 10.1038/emboj.2010.52
Gamer, J., Bujard, H., and Bukau, B. (1992). Physical interaction between heat shock proteins DnaK, DnaJ, and GrpE and the bacterial heat shock transcription factor sigma 32. Cell 69, 833–842. doi: 10.1016/0092-8674(92)90294-m
Gamer, J., Multhaup, G., Tomoyasu, T., McCarty, J. S., Rudiger, S., Schonfeld, H.-J., et al. (1996). A cycle of binding and release of the DnaK, DnaJ and GrpE chaperones regulates activity of the Escherichia coli heat shock transcription factor sigma32. EMBO J. 15, 607–617. doi: 10.1002/j.1460-2075.1996.tb00393.x
Gayán, E., Cambré, A., Michiels, C. W., and Aertsen, A. (2016). Stress-induced evolution of heat resistance and resuscitation speed in Escherichia coli O157:H7 ATCC 43888. Appl. Environ. Microbiol. 82, 6656–6663. doi: 10.1128/AEM.02027-16
Gayán, E., Govers, S. K., and Aertsen, A. (2017). Impact of high hydrostatic pressure on bacterial Proteostasis. Biophys. Chem. 231, 3–9. doi: 10.1016/j.bpc.2017.03.005
Goldstein, B. P. (2014). Resistance to rifampicin: a review. J. Antibiot. 67, 625–630. doi: 10.1038/ja.2014.107
Gross, C., Grossman, A., Liebke, H., Walter, W., and Burgess, R. (1984). Effects of the mutant sigma allele RpoD800 on the synthesis of specific macromolecular components of the Escherichia coli K12 cell. Mol. Biol. 172, 283–300. doi: 10.1016/S0022-2836(84)80027-3
Grossman, A. D., Erickson, J. W., and Gross, C. A. (1984). The HtpR gene product of E. coli is a sigma factor for heat-shock promoters. Cell 38, 383–390. doi: 10.1016/0092-8674(84)90493-8
Grossman, A. D., Straus, D. B., Walter, W. A., and Gross, C. A. (1987). σ32 synthesis can regulate the synthesis of heat shock proteins in Escherichia coli. Genes Dev. 1, 179–184. doi: 10.1101/gad.1.2.179
Grossman, A. D., Taylor, W. E., Burton, Z. F., Burgess, R. R., and Gross, C. A. (1985). Stringent response in Escherichia coli induces expression of heat shock proteins. J. Mol. Biol. 186, 357–365. doi: 10.1016/0022-2836(85)90110-X
Gunasekera, T. S., Csonka, L. N., and Paliy, O. (2008). Genome-wide transcriptional responses of Escherichia coli K-12 to continuous osmotic and heat stresses. J. Bacteriol. 190, 3712–3720. doi: 10.1128/JB.01990-07
Herendeen, S. L., Vanbogelen, R. A., and Neidhardt, F. C. (1979). Levels of major proteins of Escherichia coli during growth at different temperatures. J. Bacteriol. 139, 185–194. doi: 10.1128/jb.139.1.185-194.1979
Huang, H. W., Jie, W. S., Kai, L. J., Shyu, Y. T., and Wang, C. Y. (2017). Current status and future trends of high-pressure processing in food industry. Food Control 72, 1–8. doi: 10.1016/j.foodcont.2016.07.019
Ishihama, A. (2017). Building a complete image of genome regulation in the model organism Escherichia coli. J. Gen. Appl. Microbiol. 63, 311–324. doi: 10.2323/jgam.2017.01.002
Izard, J., Gomez, C. D. C., Balderas, D. R., Lacour, S., Song, X., Yang, Y., et al. (2015). A synthetic growth switch based on controlled expression of RNA polymerase. Mol. Syst. Biol. 11:840. doi: 10.15252/msb.20156382
Jenkins, K. A., Fossat, M. J., Zhang, S., Rai, D. K., Klein, S., Gillilan, R., et al. (2018). The consequences of cavity creation on the folding landscape of a repeat protein depend upon context. Proc. Natl. Acad. Sci. USA 115, E8153–E8161. doi: 10.1073/pnas.1807379115
Jin, J., Ding, M. C., Friedman, D. I., Nakamura, Y., Walter, W. A., and Gross, C. A. (1988). Effects of rifampicin resistant RpoB mutations on Antitermination and interaction with NusA in Escherichia coli. J. Mol. Biol. 204, 247–261. doi: 10.1016/0022-2836(88)90573-6
Johnson, C., Chandrasekhar, G. N., and Georgopoulos, C. (1989). Escherichia coli DnaK and GrpE heat shock proteins interact both in vivo and in vitro. J. Bacteriol. 171, 1590–1596. doi: 10.1128/jb.171.3.1590-1596.1989
Jun, D., and Gross, C. A. (1988). Mapping and sequencing of mutations in the Escherichia coli RpoB gene that Lead to rifampicin resistance. J. Mol. Biol. 202, 45–58. doi: 10.1016/0022-2836(88)90517-7
Jun, D. J., and Gross, C. A. (1989). Characterization of the pleiotropic phenotypes of rifampin-resistant RpoB mutants of Escherichia coli. J. Bacteriol. 171, 5229–5231.
Kallipolitis, B. H., and Valentin-Hansen, P. (1998). Transcription of RpoH, encoding the Escherichia coli heat-shock regulator σ32, is negatively controlled by the CAMP-CRP/CytR nucleoprotein complex. Mol. Microbiol. 29, 1091–1099. doi: 10.1046/j.1365-2958.1998.00999.x
Kerner, M. J., Naylor, D. J., Ishihama, Y., Maier, T., Chang, H. C., Stines, A. P., et al. (2005). Proteome-wide analysis of chaperonin-dependent protein folding in Escherichia coli. Cell 122, 209–220. doi: 10.1016/j.cell.2005.05.028
Kim, S., Kim, Y., Suh, D. H., Lee, C. H., Yoo, S. M., Lee, S. Y., et al. (2020). Heat-responsive and time-resolved transcriptome and metabolome analyses of Escherichia coli uncover Thermo-tolerant mechanisms. Sci. Rep. 10:17715. doi: 10.1038/s41598-020-74606-8
Klein, G., Stupak, A., Biernacka, D., Wojtkiewicz, P., Lindner, B., and Raina, S. (2016). Multiple transcriptional factors regulate transcription of the RpoE gene in Escherichia coli under different growth conditions and when the lipopolysaccharide biosynthesis is defective. J. Biol. Chem. 291, 22999–23019. doi: 10.1074/jbc.M116.748954
Kusukawa, N., and Yura, T. (1988). Heat shock protein GroE of Escherichia coli: key protective roles against thermal stress. Genes Dev. 2, 874–882. doi: 10.1101/gad.2.7.874
Lakowicz, J. R., and Thompson, R. B. (1983). Differential polarized phase Fluorometric studies of phospholipid bilayers under high hydrostatic pressure. Biochim. Biophys. Acta 732, 359–371. doi: 10.1016/0005-2736(83)90052-4
Landick, R., Stewart, J., and Lee, D. N. (1990). Amino acid changes in conserved regions of the 13-subunit of Escherichia coli RNA polymerase alter transcription pausing and termination. Genes Dev. 4, 1623–1636. doi: 10.1101/gad.4.9.1623
Landini, P., Egli, T., Wolf, J., and Lacour, S. (2014). SigmaS, a major player in the response to environmental stresses in Escherichia coli: role, regulation and mechanisms of promoter recognition. Environ. Microbiol. Rep. 6, 1–13. doi: 10.1111/1758-2229.12112
Lemaux, P. G., Herendeen, S. L., Bloch, P. L., and Neidhardt, F. C. (1978). Transient rates of synthesis of individual polypeptides in E. coli following temperature shifts. Cell 13, 427–434. doi: 10.1016/0092-8674(78)90317-3
Lipinska, B., King, J., Ang, D., and Georgopoulos, C. (1988). Sequence analysis and transcriptional regulation of the Escherichia coli GrpE gene, encoding a heat shock protein. Nucleic Acids Res. 16, 7545–7562. doi: 10.1093/nar/16.15.7545
Malone, A. S., Chung, Y. K., and Yousef, A. E. (2006). Genes of Escherichia coli O157:H7 that are involved in high-pressure resistance. Appl. Environ. Microbiol. 72, 2661–2671. doi: 10.1128/AEM.72.4.2661-2671.2006
Marietou, A., Nguyen, A. T., Allen, E. E., and Bartlett, D. H. (2015). Adaptive laboratory evolution of Escherichia coli K-12 MG1655 for growth at high hydrostatic pressure. Front. Microbiol. 5:749. doi: 10.3389/fmicb.2014.00749
Meenakshi, S., and Hussain Munavar, M. (2018). Evidence for up and down regulation of 450 genes by RpoB12 (Rif) mutation and their implications in complexity of transcription modulation in Escherichia coli. Microbiol. Res. 212-213, 80–93. doi: 10.1016/j.micres.2018.04.009
Merino, N., Aronson, H. S., Bojanova, D. P., Feyhl-Buska, J., Wong, M. L., Zhang, S., et al. (2019). Living at the extremes: extremophiles and the limits of life in a planetary context. Front. Microbiol. 10:780. doi: 10.3389/fmicb.2019.00780
Missiakas, D., Mayer, M. P., Lemaire, M., Georgopoulos, C., and Raina, S. (1997). Modulation of the Escherichia coli σ(E) (RpoE) heat-shock transcription-factor activity by the RseA, RseB and RseC proteins. Mol. Microbiol. 24, 355–371. doi: 10.1046/j.1365-2958.1997.3601713.x
Molodtsov, V., Scharf, N. T., Stefan, M. A., Garcia, G. A., and Murakami, K. S. (2017). Structural basis for Rifamycin resistance of bacterial RNA polymerase by the three Most clinically important RpoB mutations found in Mycobacterium tuberculosis. Mol. Microbiol. 103, 1034–1045. doi: 10.1111/mmi.13606
Morita, M., Kanemori, M., Yanagi, H., and Yura, T. (1999). Heat-induced synthesis of Sigma32 in Escherichia coli: structural and functional dissection of RpoH MRNA secondary structure. J. Bacteriol. 181, 401–410. doi: 10.1128/JB.181.2.401-410.1999
Naville, M., Ghuillot-Gaudeffroy, A., Marchais, A., and Gautheret, D. (2011). ARNold: a web tool for the prediction of rho-independent transcription terminators. RNA Biol. 8, 11–13. doi: 10.4161/rna.8.1.13346
Oger, P. M., and Jebbar, M. (2010). The many ways of coping with pressure. Res. Microbiol. 161, 799–809. doi: 10.1016/j.resmic.2010.09.017
Peñas, L., De, A., Connolly, L., and Gross, C. A. (1997). The σ(E)-mediated response to Extracytoplasmic stress in Escherichia coli is transduced by RseA and RseB, two negative regulators of σ(E). Mol. Microbiol. 24, 373–385. doi: 10.1046/j.1365-2958.1997.3611718.x
Raina, S., Missiakas, D., and Georgopoulos, C. (1995). The RpoE gene encoding the σ(E) (σ24) heat shock sigma factor of Escherichia coli. EMBO J. 14, 1043–1055. doi: 10.1002/j.1460-2075.1995.tb07085.x
Reynolds, M. G. (2000). Compensatory evolution in rifampin-resistant Escherichia coli. Genetics 156, 1471–1481. doi: 10.1093/genetics/156.4.1471
Riehle, M. M., Bennett, A. F., Lenski, R. E., and Long, A. D. (2003). Evolutionary changes in heat-inducible gene expression in lines of Escherichia coli adapted to high temperature. Physiol. Genomics 14, 47–58. doi: 10.1152/physiolgenomics.00034.2002
Rodríguez-Verdugo, A., Gaut, B. S., and Tenaillon, O. (2013). Evolution of Escherichia coli rifampicin resistance in an antibiotic-free environment during thermal stress. BMC Evol. Biol. 13:50. doi: 10.1186/1471-2148-13-50
Rome, K., Borde, C., Taher, R., Cayron, J., Lesterlin, C., Gueguen, E., et al. (2018). The two-component system ZraPSR is a novel ESR that contributes to intrinsic antibiotic tolerance in Escherichia coli. J. Mol. Biol. 430, 4971–4985. doi: 10.1016/j.jmb.2018.10.021
Rouviere, P. E., De, A., Penas, L., Mecsas, J., Chi Zen, L., Rudd, K. E., et al. (1995). rpoE, the gene encoding the second heat-shock sigma factor, σE, in Escherichia coli. EMBO J. 14, 1032–1042. doi: 10.1002/j.1460-2075.1995.tb07084.x
Royer, C. A. (2019). Characterizing proteins in their cellular environment: Examples of recent advances in quantitative fluorescence microscopy. Protein Sci. 28, 1210–1221. doi: 10.1002/pro.3630
Saito, H., and Uchida, H. (1978). Organization and expression of the DnaJ and DnaK genes of Escherichia coli K12. Mol. Gen. Genomics. 164, 1–8. doi: 10.1007/BF00267592
Scheyhing, C. H., Meersman, F., Ehrmann, M. A., Heremans, K., and Vogel, R. F. (2002). Temperature-pressure stability of green fluorescent protein: a Fourier transform infrared spectroscopy study. Biopolymers 65, 244–253. doi: 10.1002/bip.10237
Si, F., Le Treut, G., Sauls, J. T., Vadia, S., Levin, P. A., and Jun, S. (2019). Mechanistic origin of cell-size control and homeostasis in Bacteria. Curr. Biol. 29, 1760–1770.e7. doi: 10.1016/j.cub.2019.04.062
Slepenkov, S. V., and Witt, S. N. (2002). The unfolding story of the Escherichia coli Hsp70 DnaK: is DnaK a Holdase or an Unfoldase? Mol. Microbiol. 45, 1197–1206. doi: 10.1046/j.1365-2958.2002.03093.x
Straus, D., Walter, W., and Gross, C. A. (1990). DnaK, DnaJ and GrpE heat shock proteins negatively regulate heat shock gene expression by controlling the synthesis and stability of σ32. Genes Dev. 4, 2202–2209. doi: 10.1101/gad.4.12a.2202
Taylor, W. E., Straus, D. B., Grossman, A. D., Burton, Z. F., Gross, C. A., and Burgess, R. R. (1984). Transcription from a heat-inducible promoter causes heat shock regulation of the sigma subunit of E. coli RNA polymerase. Cell 38, 371–381. doi: 10.1016/0092-8674(84)90492-6
Tilly, K., Spence, J., and Georgopoulos, C. (1989). Modulation of stability of the Escherichia coli heat shock regulatory factor σ32. J. Bacteriol. 171, 1585–1589. doi: 10.1128/jb.171.3.1585-1589.1989
Tombolini, R., Unge, A., Davey, M. E., Bruijn, F. J., and Jansson, J. K. (2006). Flow cytometric and microscopic analysis of GFP-tagged Pseudomonas Fluorescens Bacteria. FEMS Microbiol. Ecol. 22, 17–28. doi: 10.1111/j.1574-6941.1997.tb00352.x
Van Boeijen, I. K., Chavaroche, A. A., Valderrama, W. B., Moezelaar, R., Zwietering, M. H., and Abee, T. (2010). Population diversity of Listeria Monocytogenes LO28: phenotypic and genotypic characterization of variants resistant to high hydrostatic pressure. Appl. Environ. Microbiol. 76, 2225–2233. doi: 10.1128/AEM.02434-09
Vanlint, D., Mitchell, R., Bailey, E., Meersman, F., McMillan, P. F., Michiels, C. W., et al. (2011). Rapid acquisition of gigapascal-high-pressure resistance by Escherichia coli. MBio 2, e00130–e00110. doi: 10.1128/mBio.00130-10
Vanlint, D., Pype, B. J., Rutten, N., Vanoirbeek, K. G., Michiels, C. W., and Aertsen, A. (2013a). Loss of cAMP/CRP regulation confers extreme high hydrostatic pressure resistance in Escherichia coli O157: H7. Int. J. Food Microbiol. 166, 65–71. doi: 10.1016/j.ijfoodmicro.2013.06.020
Vanlint, D., Rutten, N., Govers, S. K., Michiels, C. W., and Aertsen, A. (2013b). Exposure to high hydrostatic pressure rapidly selects for increased RpoS activity and general stress-resistance in Escherichia coli O157: H7. Int. J. Food Microbiol. 163, 28–33. doi: 10.1016/j.ijfoodmicro.2013.02.001
Vanlint, D., Rutten, N., Michiels, C. W., and Aertsen, A. (2012). Emergence and stability of high-pressure resistance in different food-borne pathogens. Appl. Environ. Microbiol. 78, 3234–3241. doi: 10.1128/AEM.00030-12
Wang, Q. P., and Kaguni, J. M. (1989a). A novel sigma factor is involved in expression of the rpoH ene of Escherichia coli. J. Bacteriol. 171, 4248–4253. doi: 10.1128/jb.171.8.4248-4253.1989
Wang, Q. P., and Kaguni, J. M. (1989b). DnaA protein regulates transcription of the rpoH gene of Escherichia coli. J. Biol. Chem. 264, 7338–7344. doi: 10.1016/S0021-9258(18)83238-0
Welch, T. J., Farewell, A., Neidhardt, F. C., and Bartlett, D. H. (1993). Stress response of Escherichia coli to elevated hydrostatic pressure. J. Bacteriol. 175, 7170–7177. doi: 10.1128/jb.175.22.7170-7177.1993
Winnikoff, J. R., Milshteyn, D., Vargas-Urbano, S. J., Pedraza-Joya, M. A., Armando, A. M., Quehenberger, O., et al. (2024). Homeocurvature adaptation of phospholipids to pressure in deep-sea invertebrates. Science 384, 1482–1488. doi: 10.1126/science.adm7607
Winter, R., and Jeworrek, C. (2009). Effect of pressure on membranes. Soft Matter 5, 3157–3173. doi: 10.1039/b901690b
Ying, B. W., Matsumoto, Y., Kitahara, K., Suzuki, S., Ono, N., Furusawa, C., et al. (2015). Bacterial transcriptome reorganization in thermal adaptive evolution. BMC Genomics 16:802. doi: 10.1186/s12864-015-1999-x
Ying, B. W., Seno, S., Kaneko, F., Matsuda, H., and Yomo, T. (2013). Multilevel comparative analysis of the contributions of genome reduction and heat shock to the Escherichia coli transcriptome. BMC Genomics 14:25. doi: 10.1186/1471-2164-14-25
Zaslaver, A., Bren, A., Ronen, M., Itzkovitz, S., Kikoin, I., Shavit, S., et al. (2006). A comprehensive library of fluorescent transcriptional reporters for Escherichia coli. Nat. Methods 3, 623–628. doi: 10.1038/nmeth895
Zhang, Q., Brambilla, E., Li, R., Shi, H., Lagomarsino, M. C., and Sclavi, B. (2020). A decrease in transcription capacity limits growth rate upon translation inhibition. mSystems 5:e00575-20. doi: 10.1128/mSystems
Keywords: pressure, transcriptional heat shock response, number and brightness microscopy, adaptation, E. coli
Citation: Coffin CH, Fisher LA, Crippen S, Demers P, Bartlett DH and Royer CA (2024) Response and adaptation of the transcriptional heat shock response to pressure. Front. Microbiol. 15:1470617. doi: 10.3389/fmicb.2024.1470617
Edited by:
Xue-Gong Li, Chinese Academy of Sciences (CAS), ChinaReviewed by:
Wei-Jia Zhang, Chinese Academy of Sciences (CAS), ChinaJunwei Cao, Shanghai Ocean University, China
Li Jie, Institute of Microbiology Chinese Academy of Science, China
Horia Todor, University of California, San Francisco, United States
Copyright © 2024 Coffin, Fisher, Crippen, Demers, Bartlett and Royer. This is an open-access article distributed under the terms of the Creative Commons Attribution License (CC BY). The use, distribution or reproduction in other forums is permitted, provided the original author(s) and the copyright owner(s) are credited and that the original publication in this journal is cited, in accordance with accepted academic practice. No use, distribution or reproduction is permitted which does not comply with these terms.
*Correspondence: Douglas H. Bartlett, ZGJhcnRsZXR0QHVjc2QuZWR1; Catherine A. Royer, cm95ZXJjQHJwaS5lZHU=
†These authors have contributed equally to this work