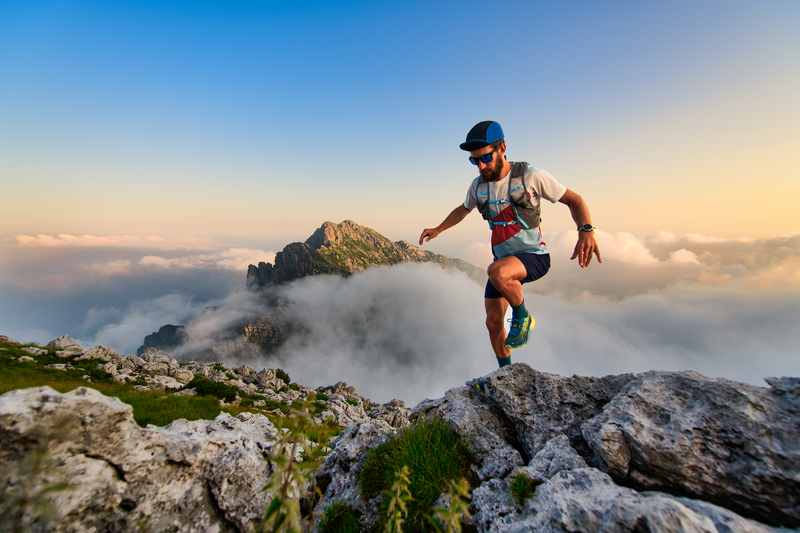
94% of researchers rate our articles as excellent or good
Learn more about the work of our research integrity team to safeguard the quality of each article we publish.
Find out more
ORIGINAL RESEARCH article
Front. Microbiol.
Sec. Terrestrial Microbiology
Volume 15 - 2024 | doi: 10.3389/fmicb.2024.1454861
This article is part of the Research Topic Forest Microbiome: Dynamics and Interactions in the Anthropocene Era View all 19 articles
The final, formatted version of the article will be published soon.
You have multiple emails registered with Frontiers:
Please enter your email address:
If you already have an account, please login
You don't have a Frontiers account ? You can register here
The Ironwood tree (Casuarina equisetifolia) holds a significant ecological role in Guam where a decline in Ironwood trees was first documented in 2002. Studies have linked the Ironwood tree decline (IWTD) to bacteria from the Ralstonia solanacearum complex and wetwood bacteria, specifically Klebsiella oxytoca and Klebsiella variicola. Presence of termites was first found to be associated with IWTD in 2010; however, the role of termites in IWTD is still not clear. The Asian subterranean termite, Coptotermes gestroi (Wasmann) (Blattodea: Rhinotermitidae) frequently attacks Ironwood trees. As workers of this soil-dwelling species of the lower termites harbor a diverse microbial community in their bodies, we examined whether C. gestroi workers carry IWTD-associated bacteria and could, therefore, act as vectors. We described the bacterial community in C. gestroi workers using 16S rRNA gene sequencing and tested the impact of factors related to the location and health of the Ironwood tree the termites were collected from on termite bacterial diversity. Feeding assays were performed to assess if workers show preference in consumption depending on the amount of Ralstonia and wetwood bacteria in the food source. Health of Ironwood trees and level of site management impacted the bacterial composition of C. gestroi termite workers attacking the trees. Although C. gestroi workers equally consumed food sources with high and low Ralstonia and wetwood bacteria load in lab experiments, Ralstonia and other IWTD-related bacteria were not detected in considerable amounts in termite workers collected from trees. Thus, C. gestroi workers are not a vector for bacteria associated with IWTD in Guam.
Keywords: termite1, bacterial taxonomy2, diversity analysis3, metataxanomics4, Amplicon sequencing5, 16S6
Received: 25 Jun 2024; Accepted: 22 Nov 2024.
Copyright: © 2024 Setia, Chen, Schlub and Husseneder. This is an open-access article distributed under the terms of the Creative Commons Attribution License (CC BY). The use, distribution or reproduction in other forums is permitted, provided the original author(s) or licensor are credited and that the original publication in this journal is cited, in accordance with accepted academic practice. No use, distribution or reproduction is permitted which does not comply with these terms.
* Correspondence:
Claudia Husseneder, Department of Entomology, Louisiana State University Agricultural Center, United States
Disclaimer: All claims expressed in this article are solely those of the authors and do not necessarily represent those of their affiliated organizations, or those of the publisher, the editors and the reviewers. Any product that may be evaluated in this article or claim that may be made by its manufacturer is not guaranteed or endorsed by the publisher.
Research integrity at Frontiers
Learn more about the work of our research integrity team to safeguard the quality of each article we publish.