- 1Guizhou University of Traditional Chinese Medicine, Guiyang, China
- 2State Key Laboratory of Dao-di Herbs, Beijng, China
- 3Faculty of Biology, University of Freiburg, Freiburg, Germany
Medicinal herbs have been increasingly used for therapeutic purposes against a diverse range of human diseases worldwide. However, inevitable contaminants, including mycotoxins, in medicinal herbs can cause serious problems for humans despite their health benefits. The increasing consumption of medicinal plants has made their use a public health problem due to the lack of effective surveillance of the use, efficacy, toxicity, and quality of these natural products. Radix Dipsaci is commonly utilized in traditional Chinese medicine and is susceptible to contamination with mycotoxins. Here, we evaluated the mycotoxins, mycobiota and toxigenic fungi in the traditional medicine Radix Dipsaci. A total of 28 out of 63 Radix Dipsaci sample batches (44.4%) were found to contain mycotoxins. Among the positive samples, the contamination levels of AFB1, AFG1, AFG2, and OTA in the positive samples ranged from 0.52 to 32.13 μg/kg, 5.14 to 20.05 μg/kg, 1.52 to 2.33 μg/kg, and 1.81 to 19.43 μg/kg respectively, while the concentrations of ZEN and T-2 were found to range from 2.85 to 6.33 μg/kg and from 2.03 to 2.53 μg/kg, respectively. More than 60% of the contaminated samples were combined with multiple mycotoxins. Fungal diversity and community were altered in the Radix Dipsaci contaminated with various mycotoxins. The abundance of Aspergillus and Fusarium increased in the Radix Dipsaci contaminated with aflatoxins (AFs) and ZEN. A total of 95 strains of potentially toxigenic fungi were isolated from the Radix Dipsaci samples contaminated with mycotoxins, predominantly comprising Aspergillus (73.7%), Fusarium (20.0%), and Penicillium (6.3%). Through morphological identification, molecular identification, mycotoxin synthase gene identification and toxin production verification, we confirmed that AFB1 and AFG1 primarily derive from Aspergillus flavus, OTA primarily derives from Aspergillus westerdijkiae, ZEN primarily derives from Fusarium oxysporum, and T-2 primarily derives from Fusarium graminearum in Radix Dipsaci. These data can facilitate our comprehension of prevalent toxigenic fungal species and contamination levels in Chinese herbal medicine, thereby aiding the establishment of effective strategies for prevention, control, and degradation to mitigate the presence of fungi and mycotoxins in Chinese herbal medicine.
Highlights
• Radix Dipsaci is susceptible to contamination by a combination of AFB1, AFG1, AFG2, OTA, ZEN and T-2.
• Fungal diversity and community were altered in the Radix Dipsaci contaminated with different mycotoxins.
• The main sources of mycotoxins in Radix Dipsaci are Aspergillus flavus, Aspergillus westerdijkiae, Fusarium oxysporum, and Fusarium graminearum.
1 Introduction
Medicinal herbs have been increasingly used for therapeutic purposes against a diverse range of human diseases worldwide. Reports suggest that an estimated 80% of the global populace continues to depend on botanical medicines (Sen and Samanta, 2015); furthermore, a significant majority of pharmaceuticals originate from plant materials or are synthesized from plant-derived compounds (Markov et al., 2017; England et al., 2023). However, the presence of inevitable contaminants, such as mycotoxins, within medicinal herbs can pose significant health risks to humans, notwithstanding their therapeutic advantages. Throughout the processes of harvesting, handling, storage, and distribution, medicinal plants are vulnerable to contamination by diverse fungi, potentially leading to spoilage and the generation of mycotoxin (Ashiq et al., 2014). The escalating utilization of medicinal plants could precipitate an augmented intake of mycotoxins; hence, the contamination of these plants with mycotoxins poses a threat to human health.
Owing to the complex cultivation processes and specialized processing requirements, traditional Chinese medicinal herbs are particularly susceptible to contamination by toxigenic fungi during their production phase (Long et al., 2021). Traditional Chinese herbal medicine is mainly contaminated by toxigenic fungi such as Aspergillus, Fusarium, and Penicillium (Han et al., 2012). According to the relevant studies, Aspergillus can produce AFs including AFB1, AFB2, AFG1, and AFG2, along with ochratoxins (Abrar et al., 2013; Schrenk et al., 2020). Fusarium species are recognized for synthesize zearalenone (ZEN), T-2, deoxynivalenol, and fumonisins (Gavrilova et al., 2023; Marin et al., 2013; Stockmann-Juvala and Savolainen, 2008; Yao and Long, 2020). The Penicillium is capable of synthesizing citrinin (Perrone and Susca, 2017; Rundberget et al., 2004). The mycotoxins mentioned often exhibit carcinogenic, teratogenic, mutagenic, hepatorenal toxicity, immunotoxicity, neurotoxicity and other highly significant toxicological effects (Fang et al., 2022; Liew and Sabran, 2022). The survey reveals the pervasive presence of mycotoxin contamination, with approximately 25% of global agricultural products being annually subjected to such contamination (Moretti et al., 2017). The safety of Chinese herbal medicine has garnered significant attention, making the prevention and control of mycotoxin pollution a focal point in current research. The accidental consumption of traditional Chinese medicinal herbs contaminated with mycotoxins poses health risks (Singh et al., 2008). The improvement in living quality has led to an increased focus on health, consequently drawing attention to the issue of mycotoxin pollution in Chinese herbal medicine. Identifying the source of mycotoxin contamination in Chinese herbal medicine is a critical initial step towards ensuring the safety of these medicinal herbs.
Root herbs, owing to their direct contact with soil, exhibit a heightened susceptibility to infections by toxigenic fungi relative to other herbal categories. Additionally, root herbs, characterized by their dense texture, demonstrate resistance to the eradication of fungal spores via conventional processing techniques (Chen et al., 2013). Under favorable environmental conditions, mycotoxins synthesized by toxigenic fungi can accumulate in Chinese herbs. Radix Dipsaci, is derived from the dried root of Dipsacus asper Wall.ex Henry (Wu et al., 2022), is renowned for its liver and kidney tonifying properties, muscle and bone strengthening effects, fracture healing abilities, and its use in treating osteoporosis (Du et al., 2023; Tao et al., 2020). The processing of Radix Dipsaci, categorized as a medicinal root herb, necessitates undergoing a “sweating” treatment (Tao et al., 2020). However, the high temperature and humidity environment provided during this process can easily promote the growth of toxic fungi. Currently, research into the detection of mycotoxins and the identification of toxigenic fungi within Radix Dipsaci remains profoundly uncharted.
Studies have found that Radix Dipsaci can improve osteoporosis by promoting osteogenic differentiation of bone marrow stromal cells, increasing bone mineral density and changing bone histomorphology (Ke et al., 2016). In recent years, several researchers have discovered that Radix Dipsaci has the potential to inhibit neuronal apoptosis in the brains of patients with Alzheimer’s disease and enhance their cognitive function (Gao et al., 2018). Yang et al. (2024) discovered that contamination of Radix Dipsaci with AFB1 resulted in a diminished capacity to enhance mineral density and mineral salt content in the bones of diseased mice, while also impeding the generation of newborn neurons in the hippocampus, consequently leading to cognitive decline. The pharmacodynamic effects of Radix Dipsaci significantly compromised by the presence of mycotoxin contamination. However, current research on Radix Dipsaci primarily focuses on processing, chemical composition, pharmacological effects, and other related aspects. There are limited reports regarding the impact of mycotoxin contamination on Radix Dipsaci quality. In this study, we conducted systematic surveillance of randomly selected Radix Dipsaci samples to identify those contaminated with mycotoxins. To elucidate the potential impacts of mycotoxins on Radix Dipsaci’s fungal community, we assessed alterations in fungal community following mycotoxin contamination. By employing morphological and molecular identification techniques, alongside mycotoxin synthase gene analysis and toxin production verification, we delineated the types and levels of mycotoxins produced by each toxigenic fungus. Our results provide valuable insights that could guide the development of strategies for the prevention, control, and remediation of contaminants, ultimately ensuring the safety of the traditional medicinal herb, Radix Dipsaci.
2 Materials and methods
2.1 Radix Dipsaci sampling and detection of mycotoxins
The Radix Dipsaci samples were purchased from Guiyang Taisheng medicinal material market, Guangxi Yulin herbal medicine market, Anguo herbal medicine market of Hebei Province, Bozhou herbal medicine market of Anhui Province, Hehuachi herbal medicine market of Chengdu Sichuan Province, and camphor tree herbal medicine market of Jiangxi Province from September to December 2020. We collected 9–12 batchs of Radix Dipsaci samples in each herbal medicine market using a five-point sampling method, yielding a total of 63 batchs Radix Dipsaci samples. A minimum of 5 kg was procured for each batch.
The test substance and reference substance were prepared according to the method described by Ge et al. (2024): the Radix Dipsaci sample was extracted with acetonitrile/water/acetic acid (80/19/1, v/v/v), and purified material was added to remove impurities, evaporated to near dryness under nitroge, and finally redissolved with acetonitrile (50% v/v) to obtain the test substance solution. Standards for AFB1 (lot No. MSS1003), AFG1 (lot No. MSS1005), AFG2 (lot No. MSS1006), OTA (lot No. MSS1020), ZEN (lot No. MSS1024), and T-2 (lot No. MSS1023) were sourced from Qingdao Puribang Co., Ltd. (Qingdao, China). The reference substance was prepared with 50% acetonitrile solution, and finally 400 ng/mL mixed standard substance of AFB1, AFG1, AFG2, OTA, T-2 and 500 ng/mL ZEN was obtained.
Mycotoxin detection in Radix Dipsaci utilized high performance liquid chromatography mass spectrometry (HPLC-MS/MS, SCIEX-QTRAP 5500 tandem quadrupole mass spectrometry, SCIEX, United States), following the methodology outlined in Zhao et al. (2021) and Ge et al. (2024): the column temperature was maintained at 40°C; the mobile phase was composed of 0.1% formic acid (Fisher, United States) as eluent A and 0.1% acetonitrile (Fisher, United States) as eluent B; the flow rate was established at a constant value of 0.3 mL/min; the volume of injection was 5.00 μL. The linear gradient elution program was as follows: from 0 to 3 min, the concentration of solution B increased linearly from 15 to 35%; from 3 to 5 min, the concentration of solution B increased linearly from 35 to 45%; from 5 to 7 min, it increased linearly from 45 to 50%; linear equilibrium was maintained during the period from 7 to 7.5 min. The mass spectrometer was operated in positive ionization mode (ESI+) using an electrospray source. The parameters for the analysis of the mycotoxin, including m/z values, collision energy of parent ions, primary daughter ions, and selection criteria, are presented in Supplementary Table S1.
The analytical method for mycotoxin detection was validated according to the method described by Ge et al. (2024), and the standard curve was established. The signal-to-noise ratio S/N ≥3 was used as the limit of detection (LOD), and S/N ≥10 was used as the limit of quantification (LOQ). The method’s repeatability, instrument’s precision and stability were assessed by measuring the mycotoxin content, the peak area of six consecutive injections, and the relative standard deviation of the mycotoxin content detected at 0, 2, 4, 6, 12, and 24 h. The method accuracy was evaluated by conducting sample recovery experiments to determine the recovery rate and relative standard deviation of each mycotoxin.
2.2 Microbiome sequencing and analysis
The extraction and amplification of fungal DNA from Radix Dipsaci were conducted in accordance with the methods detailed in a previous report (He et al., 2023). The amplification of the ITS regions of the fungal 18S rRNA genes utilized primers ITS1F (5′-CTTGGTCATTTAGAGGAAGTAA-3′) and ITS2R (5′-GCTGCGTTCTTCATCGATGC-3′). Fungal PCR was conducted following the protocol in the previous study (He et al., 2023). The PCR products were detected through 2% agarose gel electrophoresis. Purified amplicons were pooled in equimolar ratios and paired-end sequenced on an Illumina MiSeq platform (Illumina, San Diego, United States) according to the standard protocols described by Majorbio Bio-Pharm Technology Co., Ltd. (Shanghai, China). The raw sequencing reads have been deposited into the NCBI Sequence Read Archive (BioProject ID PRJNA1103735).
Sequences in the library were clustered using USearch, operational taxonomic units (OTUs) were clustered at 97% similarity for non-repetitive sequences (excluding individual sequences), and chimeras were eliminated during clustering to derive representative sequences of OTUs. Utilizing the OTUs information, rarefaction curves and alpha diversity indices including observed OTUs, Ace, Chao richness and Shannon and Simpson index were calculated with Mothur v1.30.1. The similarity among the microbial communities in different samples was assessed through principal coordinate analysis principal coordinate analysis (PCoA) based on Bray–Curtis dissimilarity using Vegan v2.5-3 package.
2.3 Isolation and identification of potentially toxigenic fungi
2.3.1 Isolation of potentially toxigenic fungi
Accurately weighing 15 g of the Radix Dipsaci contaminated with mycotoxins using an analytical balance into a conical bottle of 135 mL sterile water. The Radix Dipsaci sample powder was thoroughly dispersed by shaking for 20 min in a shaking incubator (GZX-400EF, Tianjin Test Instrument Co., Ltd., China). Serial dilutions ranging from 10−1 to 10−4 were meticulously prepared from the samples, following the documented procedure in Su et al. (2018). The prepared dilutions were inoculated on Potato Dextrose Agar (PDA) media, which consisted of 200 g of potato, 20 g of glucose (Tianjin Yongda Chemical Reagent Co., Ltd., China), 15 g of agar (Soleibao Biotechnology Co., Ltd., China), and 0.1 g of kanamycin (Soleibao Biotechnology Co., Ltd., China) dissolved in 1 L of water (Wei et al., 2023). Subsequently, the samples were incubated at a constant temperature of 28°C for a duration ranging from 5 to 7 days, throughout which the morphology of the colonies was meticulously observed and documented (Chen et al., 2020). Pure cultures were successfully isolated after multiple rounds of purification, and these pure cultures were then stored at a refrigerated temperature of 4°C.
2.3.2 Morphological and molecular identification of potentially toxigenic fungi
Macroscopic characteristics, such as the growth rate, morphological alterations of mycelium, and the texture and coloration of purified fungal colonies, were systematically observed with the naked eye. The microscopic characteristics, such as conidia and conidiophores of the toxigenic fungi, were observed using a microscope (BX-41, Olympus Corporation Limited, Japan).
Fresh mycelia were collected from PDA surfaces and fungal DNA extraction was carried out following the protocol described in He et al. (2023). Amplification of the ITS2 region within the fungal DNA gene was achieved using primers ITS1 (5′-TCCGTAGGAACCTGCGG-3′) and ITS4 (5′-TCCTCCGCTTATTGATATGC-3′). PCR amplifications were carried out in a 20 μL reaction volume using the C1000Touch™ Thermal Cycler (Bio-Rad, United States). The system comprised 0.4 μL of each forward primer (ITS1) and reverse primer (ITS4), 2.0 μL of DNA template, 0.2 μL of EasyTaq DNA polymerase, 2.0 μL of 10 × EasyTaq buffer, 1.5 μL of 2.5 mM dNTPs, and 13.5 μL of H2O. The thermocycler was programmed for 35 cycles: denaturation at 95°C for 30 s, annealing at 58°C for 40 s, elongation at 72°C for 1 min, and final elongation at 72°C for 10 min. The PCR products were detected using the ChemiDocTM Touch Imaging System (Bio-Rad, United States) for digital imaging gel analysis. Following the PCR amplification, samples were sent to Shanghai Shenggong Biological Company (Shanghai, China) for sequencing. The resulting sequences were subjected to analysis via the BLAST program1 hosted on the NCBI website, to identify the genera and species of the fungal isolates. Then, the toxigenic fungi were subsequently subjected to phylogenetic analysis using reference sequences derived from ITS rDNA and closely related taxa available in GenBank. The fungus Saccharomyces cerevisiae was used as an outgroup for phylogenetic analyses. The phylogenetic analysis of sequences retrieved from GenBank, and the construction of phylogenetic trees were conducted using the MEGA 5.0 software. The evolutionary history was inferred using the neighbor-joining method, and the p-distance method was employed to calculate the evolutionary distance. The robustness of the branches was assessed through 1,000 bootstrap replications.
2.4 Analysis of key gene expression in mycotoxin synthesis
DNA extraction from fungal samples was conducted according to the protocol in He et al. (2023). The conserved regions of AFs, OTA, T-2, and ZEN were selected for the design of specific primers targeting their synthesis key genes Aflr, PKS, Tri7, and PKS14. PCR primers are detailed in Supplementary Table S2. The extracted fungal DNA was utilized as templates for PCR reactions to detect the presence of Aflr, PKS, Tri7, and PKS14 in purified toxigenic fungi. The PCR reaction was conducted using a 20 μL reaction system, and the amplification procedure is detailed in section 2.3.2.
2.5 Verification of mycotoxins production
The isolated toxigenic fungi were introduced onto a substrate composed of irradiated sterilized rice and Radix Dipsaci, with the moisture level adjusted to 30%, followed by incubation at a temperature of 25°C for a duration of 14 days. After incubation, all cultures were dehydrated at 45°C and ground into a fine powder. Accurately weigh 2 g of powder using an analytical balance into a centrifuge tube. The centrifugal tube was supplemented with 10.0 mL of carbinol (lot No. 200343, Fresher, United States) containing 70% concentration. After thorough oscillation and centrifugation, 5 mL of the supernatant was transferred to a 10 mL volumetric flask. The sample, which was filtered using a 0.22 μm polytetrafluoroethylene-ethylene filter, was injected into the HPLC-MS/MS system for analysis.
2.6 Statistical analysis
Statistical analysis of the data was performed with GraphPad Prism 8.0 (GraphPad, La Jolla, CA, United States). Results were presented as mean ± SEM. Group differences were evaluated for significance with one-way ANOVA for normally distributed data, as determined by the Kolmogorov–Smirnov test, or with the Kruskal–Wallis test for non-normally distributed data. Significance was defined as p < 0.05. The results of statistical analysis are shown in Supplementary Tables S3, S4.
3 Result
3.1 Occurrence of mycotoxins in Radix Dipsaci
The UPLC-MS/MS method was utilized to detect mycotoxins in the Radix Dipsaci samples, revealing that out of the 63 batches samples, mycotoxins were identified in 28 batches, indicating a contamination rate of 44.4%. Among them, 11 batches were found to be contaminated with a single mycotoxin (AFB1, AFG1, OTA), while 17 batches were discovered to be contaminated with two or more mycotoxins (Figure 1A). The most prevalent mycotoxins detected in the positive samples were AFB1 (34.92%), OTA (19.04%), and AFG1 (14.28%) (Figure 1B). The contamination levels of AFB1, AFG1, AFG2, and OTA in the positive samples ranged from 0.52 to 32.13 μg/kg, 5.14 to 20.05 μg/kg, 1.52 to 2.33 μg/kg, and 1.81 to 19.43 μg/kg respectively, while the concentrations of ZEN and T-2 were found to range from 2.85 to 6.33 μg/kg and from 2.03 to 2.53 μg/kg, respectively. Among the samples contaminated with mycotoxins, 13 batches samples exhibited AFB1 levels exceeding 5 μg/kg, while in 5 batches samples OTA levels surpassed 10 μg/kg. Moreover, in 3 batches samples both AFB1 and AFG1 exceeded the threshold of 10 μg/kg. The ZEN contamination level in the sample was below 500 μg/kg, which meets the requirements of the Chinese pharmacopoeia (2020 edition) (Figure 1C). The findings imply that the Radix Dipsaci is prone to contamination by diverse mycotoxins.
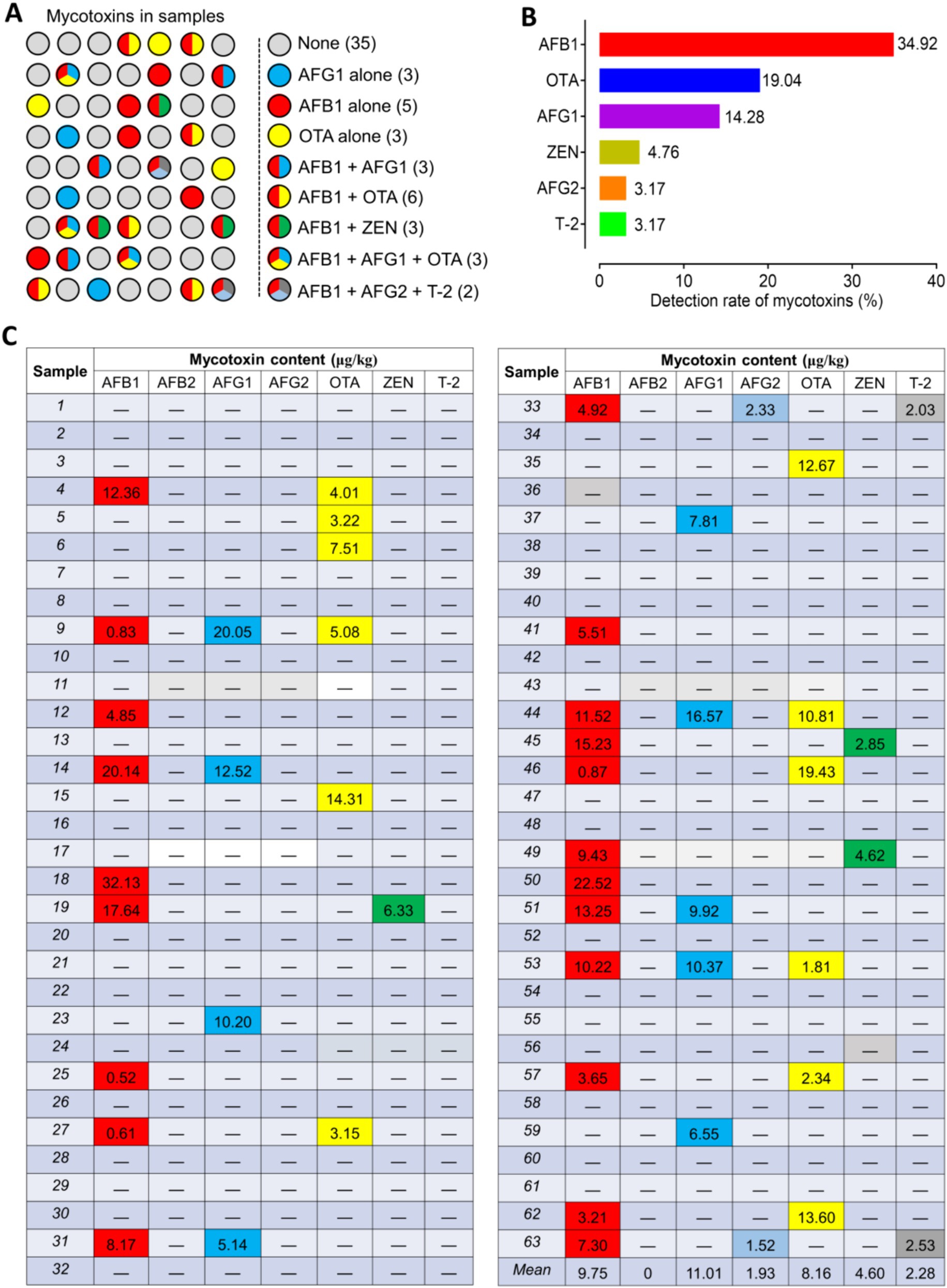
Figure 1. Occurrence of mycotoxins in Radix Dipsaci. (A) Determination of mycotoxins in 63 batches of Radix Dipsaci from major herbal medicine markets in five parts of China. None, samples were not contaminated with any mycotoxins; AFG1 alone, samples contaminated only with aflatoxin G1 (AFG1); AFB1 alone, samples contaminated only with aflatoxin B1 (AFB1); OTA alone, samples contaminated only with ochratoxin A (OTA); AFB1 + AFG1, samples contaminated with both AFB1 and AFG1; AFB1 + OTA, samples contaminated with both AFB1 and OTA; AFB1 + ZEN, samples contaminated with both AFB1 and zearalenone (ZEN); AFB1 + AFG1 + OTA, samples contaminated with AFB1, AFG1 and OTA; AFB1 + AFG2 + T-2, samples contaminated with both AFB1, aflatoxin G2 (AFG2) and T-2 toxin (T-2). (B) Percentages of samples contaminated with AFB1, OTA, AFG1, ZEN, AFG2 and T-2. (C) Levels of mycotoxins contamination of 63 batches Radix Dipsaci samples.
3.2 Fungal diversity and community were altered in the Radix Dipsaci contaminated with different mycotoxins
We prepared Radix Dipsaci samples that were not contaminated with mycotoxins or contaminated with different mycotoxins for conducting microbiome sequencing. Fungal alpha diversity was assessed through computation of Chao, Ace, Shannon, and Simpson indices at the operational taxonomic unit (OTU) level. The findings indicated a marked reduction in Ace and Chao indices of fungi in Radix Dipsaci contaminated with AFB1 alone, AFG1 alone, OTA alone, AFB1 + OTA, AFB1 + ZEN, or AFB1 + AFG1 + OTA, compared to non-contaminated samples, (p < 0.001) (Figures 2A,B), indicating that mycotoxins contamination reduced the richness of fungi in Radix Dipsaci. A notable decline in the Shannon index and a substantial rise in the Simpson index of fungi were observed in Radix Dipsaci contaminated with AFB1 alone, AFG1 alone, OTA alone, AFB1 + OTA, AFB1 + ZEN, or AFB1 + AFG1 + OTA, in contrast to non-contaminated samples, (p < 0.05) (Figures 2C,D), indicating that mycotoxins contamination reduced fungal diversity in Radix Dipsaci. We observed a lower number of fungal OTUs in all samples contaminated with mycotoxins when compared to those of the samples not contaminated with mycotoxins (Figure 2E). Additionally, we identified 65 OTUs common to all Radix Dipsaci samples and 20, 31, 20, 22, 24, 19, 39 and 41unique OTUs in samples contaminated with AFB1 alone, AFG1 alone, OTA alone, AFB1 + AFG1, AFB1 + OTA, AFB1 + ZEN, AFB1 + AFG1 + OTA and AFB1 + AFG2 + T-2 respectively (Figure 2E). We also found that the dominant fungi in samples not contaminated with mycotoxins were fungi of the genus Gibberella, the dominant fungi in samples contaminated with AFs and OTA were fungi of the genus Aspergillus, the dominant fungi in samples contaminated with ZEN and T-2 were fungi of the genus Fusarium (Figure 2F).
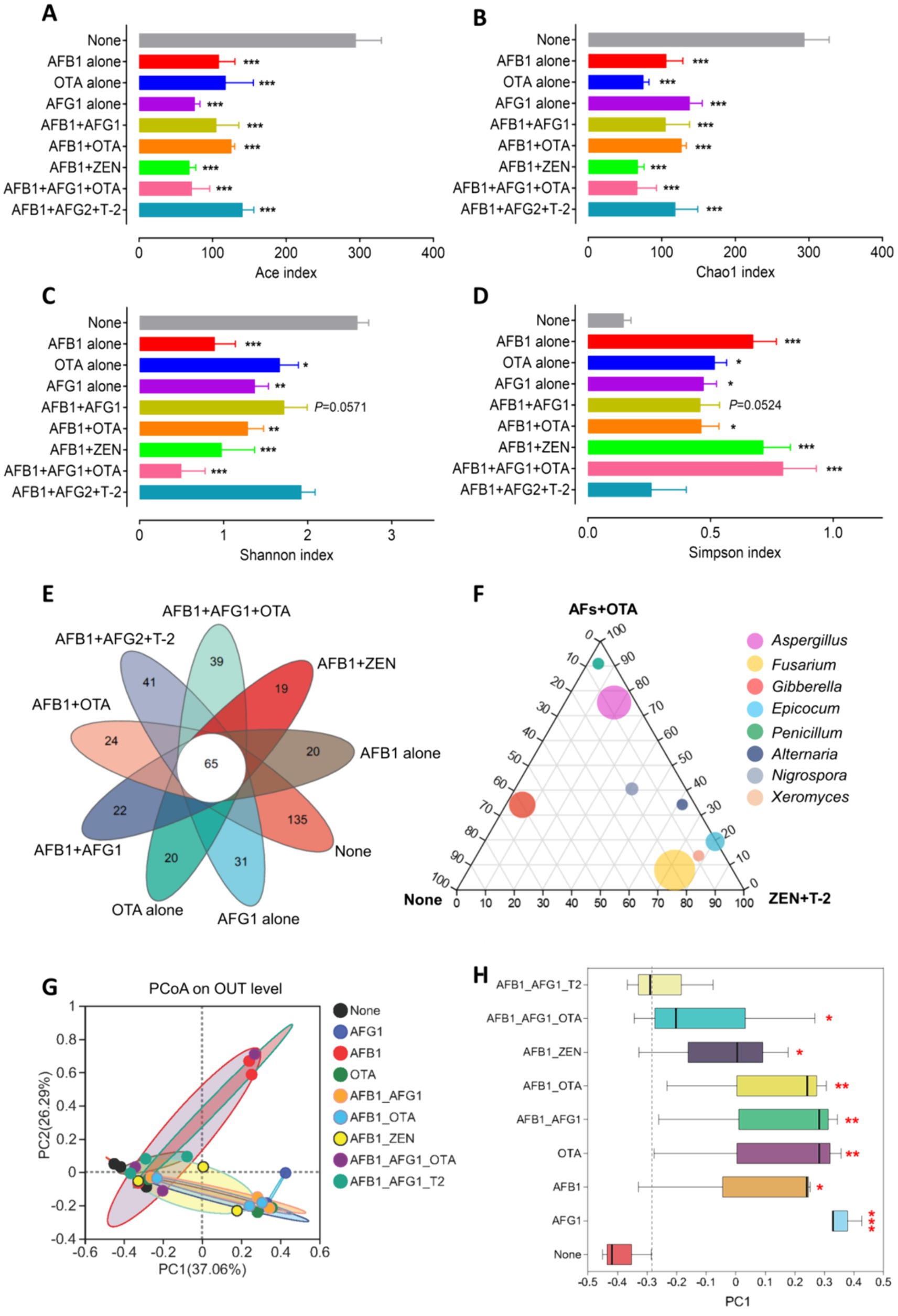
Figure 2. Fungal diversities were altered in the Radix Dipsaci contaminated mycotoxins. (A–D) Alpha diversity at the level of OTUs in fungal communities in Radix Dipsaci samples not contaminated with mycotoxins or contaminated with different mycotoxins. Microbial diversity was quantified using Chao’s, Ace’s, Shannon’s and Simpson’s diversity indices (n = 3). (E) Wayne’s analysis showed the changes in the number of operational taxonomic unit (OTU) of fungi in Radix Dipsaci samples contaminated with different mycotoxins. (F) The difference of fungal genus among none, AFs + OTA, and ZEN + T-2 evaluated using the two-tailed Wilcoxon test. (G,H) Principal component analysis (PCoA) of beta diversity in fungal communities in Radix Dipsaci samples not contaminated with mycotoxins or contaminated with different mycotoxins, based on Bray–Curtis distances. Data are mean ± SEM (n = 3). *p < 0.05, **p < 0.01, and ***p < 0.001 compared with none group by one-way ANOVA with Tukey’s multiple comparison post hoc test.
Beta diversity was analyzed through principal coordinate analysis (PCoA) plots using nonphylogenetic Bray–Curtis metrics to assess differences in microbial composition (OTU) among these groups (Figure 2G). A distinct segregation was evident between the samples contaminated with AFB1 alone, AFG1 alone, OTA alone, AFB1 + AFG1, AFB1 + OTA, AFB1 + ZEN, and AFB1 + AFG1 + OTA, and the mycotoxin-free samples along the primary axis of the first principal component (PC1), p < 0.05 (Figure 2H), indicating that contamination with mycotoxins can alter the composition of the fungal community. For instance, at the genus level, a notable rise in the prevalence of Aspergillus fungi was observed in samples contaminated solely with AFB1, AFG1, AFB1 + AFG1, AFB1 + OTA, and AFB1 + AFG1 + OTA. Conversely, there was a significant surge in the abundance of Fusarium fungi in samples contaminated with OTA alone, AFG1 alone, AFB1 + AFG1, and AFB1 + OTA, (p < 0.001) (Figures 3A–E). These results indicate that fungal diversity and community were altered, and the abundance of Aspergillus and Fusarium were increased in the Radix Dipsaci contaminated with different mycotoxins.
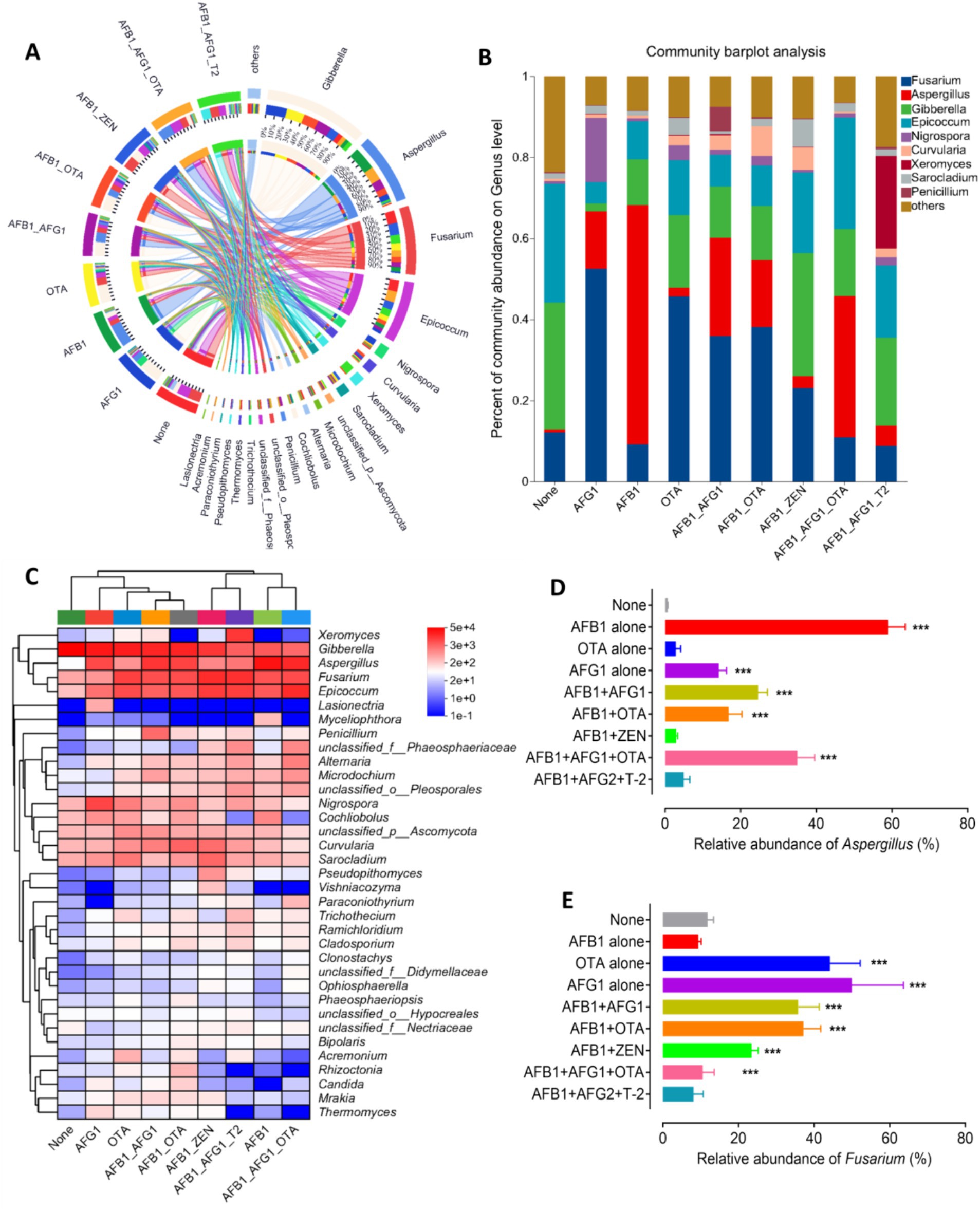
Figure 3. Fungal communities were altered in the Radix Dipsaci contaminated mycotoxins. (A,B) Relative abundances of fungal genus in Radix Dipsaci samples not contaminated or contaminated with different mycotoxins. (C) The difference of fungal genus among Radix Dipsaci samples not contaminated or contaminated with different mycotoxins. (D,E) Relative abundances of Aspergillus spp. and Fusarium spp. in Radix Dipsaci samples not contaminated or contaminated with different mycotoxins. Data are mean ± SEM (n = 3). ***p < 0.001 compared with none group by one-way ANOVA with Tukey’s multiple comparison post hoc test.
3.3 Isolation and identification of potentially toxigenic fungi in Radix Dipsaci
Ninety-five strains of toxigenic fungi were isolated from 28 batches of Radix Dipsaci contaminated with various mycotoxins. A greater number of Aspergillus strains were isolated from Radix Dipsaci samples contaminated with AFG1, AFB1, OTA, AFB1 + OTA, AFB1 + AFG1 and AFB1 + AFG1 + OTA, resulting in a total of 64 Aspergillus species being identified. More Fusarium strains were isolated from the Radix Dipsaci samples contaminated with AFB1 + ZEN and AFB1 + AFG2 + T-2, resulting in a total of 8 Fusarium species (Figure 4A).
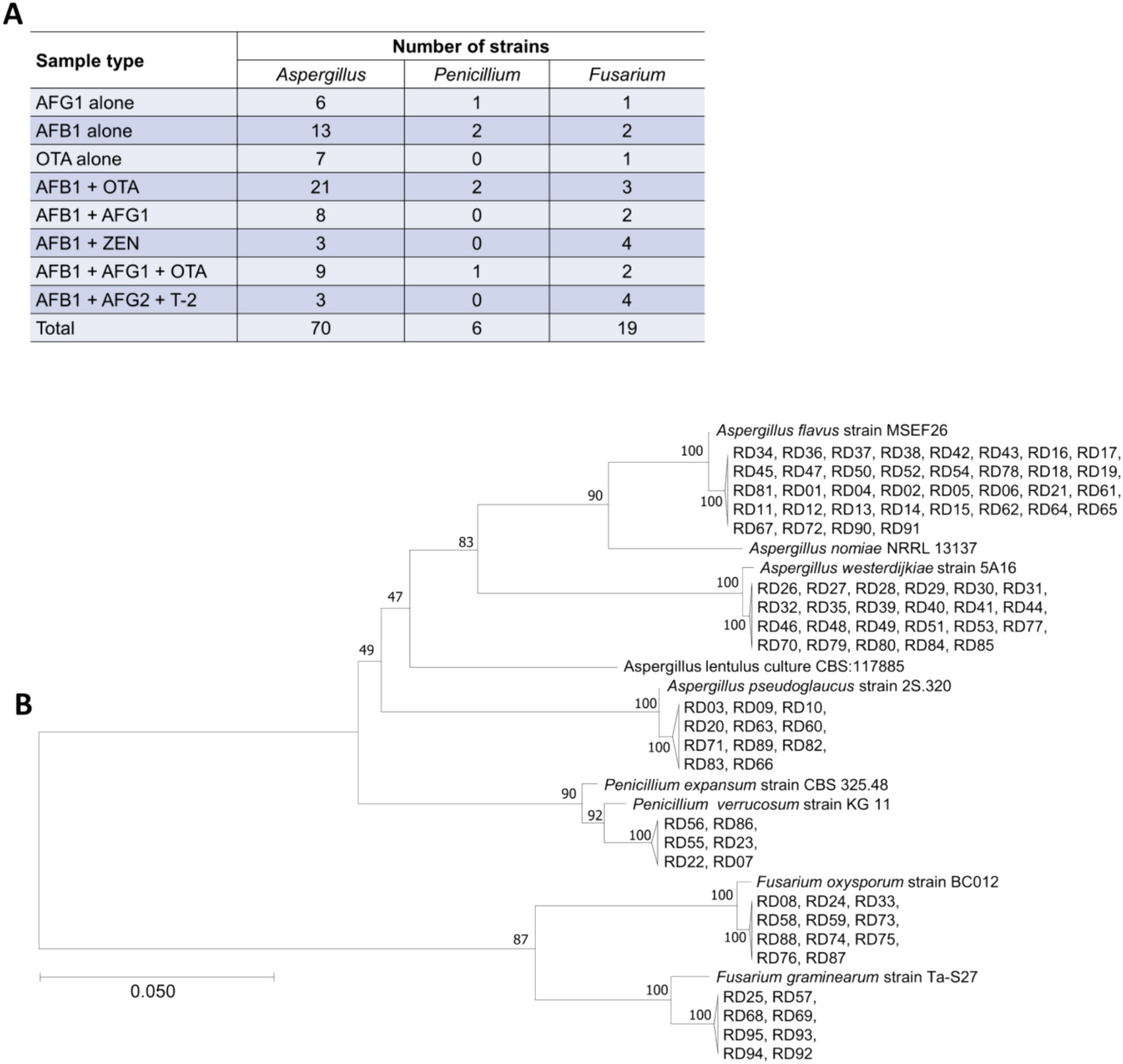
Figure 4. Isolation and molecular identification of potentially toxigenic fungi from Radix Dipsaci contaminated with mycotoxins. (A) The quantities of Aspergillus spp., Penicillium spp. and Fusarium spp. isolated from Radix Dipsaci sample contaminated with various mycotoxins. (B) Molecular identification of potentially toxigenic fungi from Radix Dipsaci contaminated with mycotoxins. Phylogenetic tree based on gene sequences of specific fungi, inferred by using the maximum likelihood method, on 36 A. flavus, 23 A. westerdijkiae, 11 A. pseudoglaucus, 11 F. oxysporum, eight F. graminearum and six P. verrucosum isolated from Radix Dipsaci, compared to reference sequences for corresponding species.
Through the phylogenetic tree indicated that 36 potentially toxigenic fungal strains shared a close genetic relationship with A. flavus strain MSEF26, showing a 100% sequence homology. The 23 toxigenic fungal strains showed a 100% similarity with A. westerdijkiae strain 5A16. The homology between the six toxigenic fungal strains and Penicillium verrucosum strain KG11 was determined to be 92%. The 11 potentially toxigenic fungal strains exhibited a 100% homology with F. oxysporum strain BC012. The eight toxigenic fungal strains demonstrated a 100% similarity with F. graminearum strain Ta-S27 (Figure 4B).
The purified strains were observed for pigment and morphological characteristics (as showed Supplementary Figure S1). The colonies of P. verrucosum showed displaying green coloration, white margins, and beige undersides. The conidia exhibited displaying a spherical to subspherical shape. The colony of A. flavus showed featuring a loosely structured pale-yellow surface, the mycelium exhibited accompanied by flask-shaped at the apex. The colony of A. westerdijkiae showed velvety texture. The apical capsule of the conidium was spherical, with the conidia also being spherical in shape. The colony of Aspergillus pseudoglaucus exhibited rapid growth on PDA and was floccose, flat, brilliant greenish yellow. The reverse side of the colony appeared pale yellow to brilliant yellow. The colony of F. graminearum diffuse aerial mycelium that was white on the front but had reddish pigmentation on the reverse side of the culture plate. The F. oxysporum colony was white to lavender, with red pigment precipitation from the back side of the plate. Both large and small conidia were observed in the colonies. The small conidia were ovoid. The large conidia were sickle - shaped.
The results revealed that A. flavus exhibited the highest frequency of isolation from the samples contaminated with AFG1 or AFB1. The predominant toxigenic fungi isolated from the samples contaminated with OTA was A. westerdijkiae. A higher frequency of occurrence of A. flavus and A. westerdijkiae was noted in samples contaminated with AFB1 and OTA. Moreover, the presence of A. flavus, F. oxysporum, and F. graminearum was observed more frequently in samples contaminated with AFB1 and ZEN or T-2 (Figure 5A).
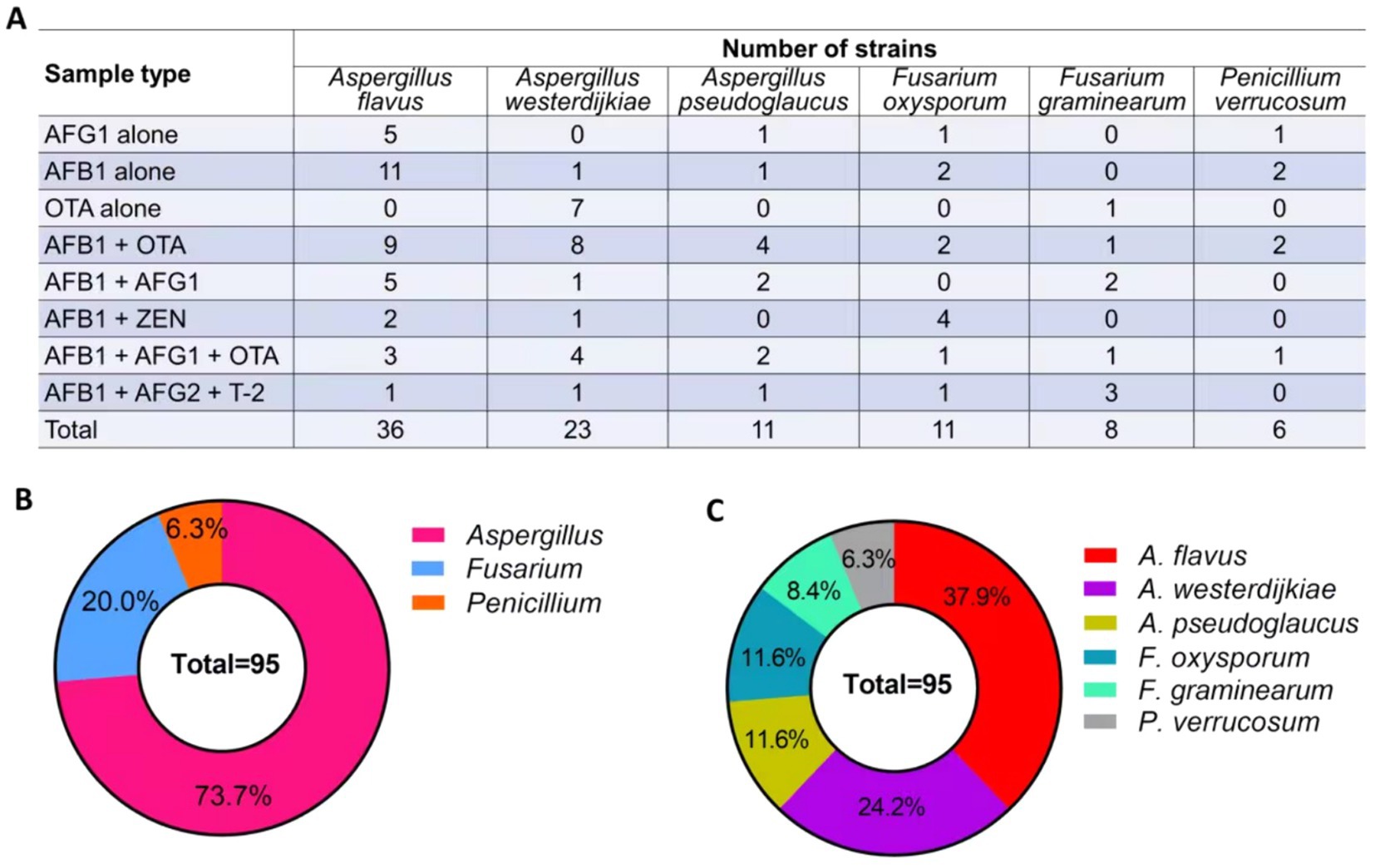
Figure 5. Quantity and frequency of potentially toxigenic fungi in Radix Dipsaci. (A) The quantity of potentially toxigenic fungi isolated from Radix Dipsaci contaminated with mycotoxins. (B,C) The frequency of potentially toxigenic fungi isolated from Radix Dipsaci contaminated with mycotoxins.
A total of 95 strains of fungi were isolated from the Radix Dipsaci samples contaminated with mycotoxins, predominantly comprising Aspergillus (73.7%), Fusarium (20.0%), and Penicillium (6.3%) (Figure 5B). Subsequent investigations unveiled that a total of 36 strains of A. flavus were isolated from the contaminated samples, constituting approximately 37.9% of the overall strain population. Twenty-three strains of A. westerdijkiae were isolated from the contaminated samples, accounting for 24.2%. A. pseudoglaucus and F. oxysporum strains were evenly distributed, with each species comprising 11 strains, accounting for 11.6% respectively. The presence of eight strains of F. graminearum accounted for 8.4%, while the presence of six strains of P. verrucosum accounted for 6.3% (Figure 5C).
3.4 Identification of key genes for mycotoxins biosynthesis of toxigenic fungi in Radix Dipsaci
The pivotal genes Aflr, PKS, Tri7, and PKS14 associated with the biosynthesis of AFs, OTA, T-2, and ZEN were identified through PCR amplification. The results indicated the presence of the Aflr gene in A. flavus, implying its capability for AFs production. The detection of the PKS14 gene in F. oxysporum implies the potential for ZEN production by this fungus. The identification of the PKS gene in A. westerdijkiae indicates the potential for OTA production by this fungus. The presence of the Tri7 gene in F. graminearum indicates its potential for T-2 biosynthesis (Figure 6).
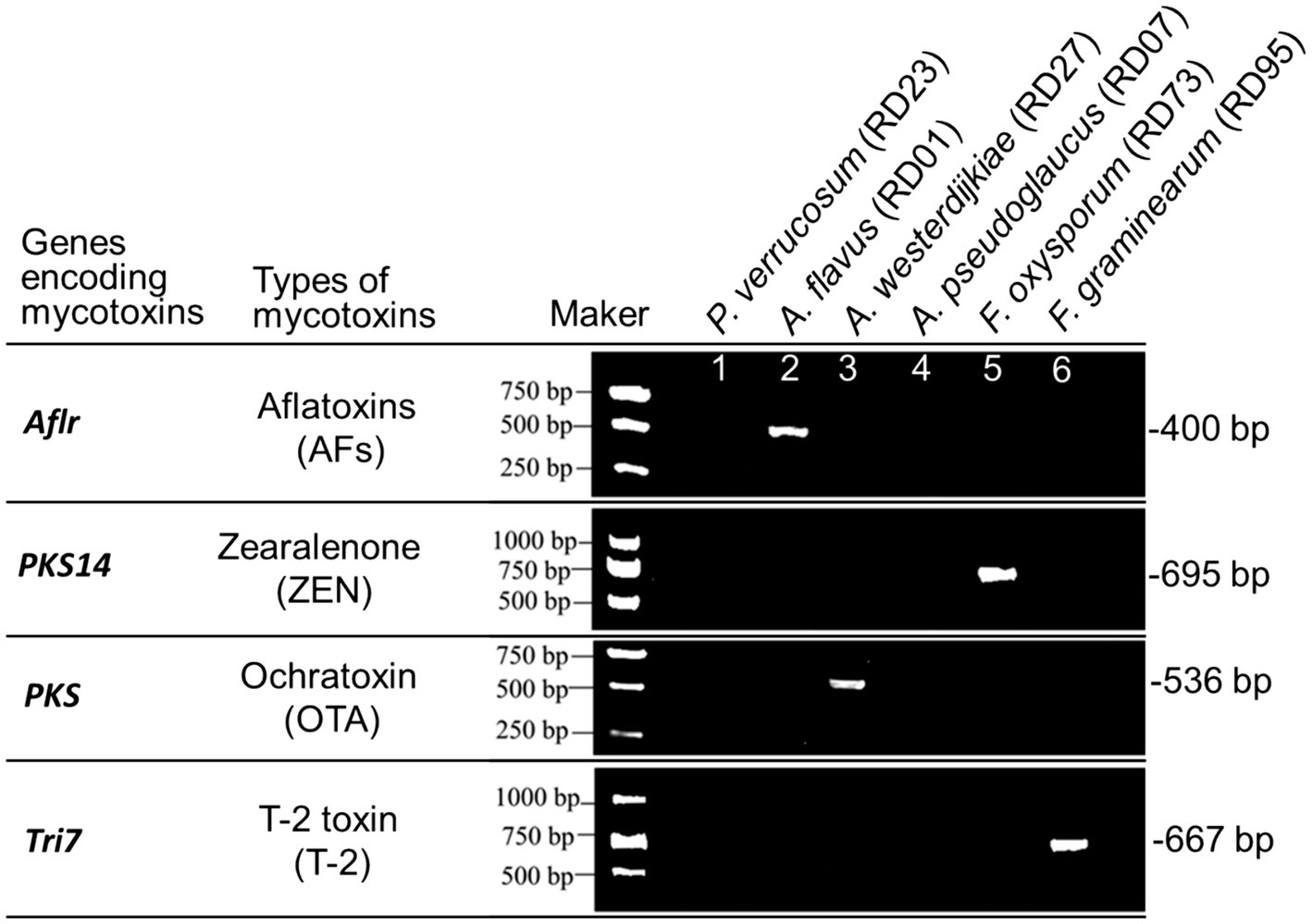
Figure 6. PCR identification of key genes for mycotoxins biosynthesis in potentially toxigenic fungi from Radix Dipsaci.
3.5 Verification of mycotoxins production for toxigenic fungi in Radix Dipsaci
The production of mycotoxins by fungi expressing key genes involved in mycotoxin biosynthesis on rice and the Radix Dipsaci matrix was identified through the utilization of an immunoaffinity column coupled with HPLC-MS/MS (Supplementary Figure S2). The results uncovered that A. flavus simultaneously produced AFB1 and AFG1 in both the rice and Radix Dipsaci matrices. In the rice matrix, the levels of AFB1 and AFG1 produced were 0.61 ± 0.20 μg/kg and 0.89 ± 0.21 μg/kg, respectively, whereas in the Radix Dipsaci matrix, they were 0.18 ± 0.05 μg/kg and 0.13 ± 0.04 μg/kg, respectively. A. westerdijkiae demonstrated the ability to produce OTA in both the rice matrix and Radix Dipsaci matrix, with concentrations of 0.72 ± 0.16 μg/kg in the rice matrix and 0.06 ± 0.02 μg/kg in the Radix Dipsaci matrix. F. oxysporum exhibited the capacity to produce ZEN in both the rice matrix and Radix Dipsaci matrix, with ZEN contents of 4.02 ± 0.42 μg/kg in the rice matrix and 2.15 ± 0.36 μg/kg in the Radix Dipsaci matrix. The production of T-2 by F. graminearum in the rice matrix was below the limit of quantification, and no detectable levels of T-2 were observed in the Radix Dipsaci matrix (Tables 1, 2).
4 Discussion
Contamination of Chinese herbal medicine with mycotoxins can reduce or even lose its therapeutic effect, which is potentially harmful to human health. The current imperative in ensuring the quality and safety of Chinese herbal medicines lies in the meticulous identification of sources and circumstances surrounding mycotoxin contamination within these medicinal products. In the present study, we assessed the mycotoxins, mycobiota, and toxigenic fungi present in the traditional medicinal herb Radix Dipsaci. Our findings demonstrate that Radix Dipsaci is prone to contamination by a combination of AFB1, AFG1, AFG2, OTA, ZEN, and T-2. The fungal diversity and community were altered in the Radix Dipsaci contaminated with diverse mycotoxins. There was an increase in the abundance of Aspergillus and Fusarium in Radix Dipsaci contaminated with AFs and ZEN. Through the utilization of morphological identification, molecular identification, mycotoxin synthase gene identification, and toxin production verification, we validated that AFB1 and AFG1 mainly originated from A. flavus, OTA primarily originated from A. westerdijkiae, ZEN primarily originated from F. oxysporum, and T-2 primarily originated from F. graminearum in Radix Dipsaci. This study signifies a groundbreaking endeavor in the identification of mycotoxins and their toxigenic fungi in Radix Dipsaci, to the best of our knowledge.
Inadequate handling during the processing and storage of traditional Chinese herbal medicine may lead to contamination by toxigenic fungi, resulting in quality degradation and jeopardizing safe clinical usage (Chen et al., 2020). Studies have shown that root herbs are particularly vulnerable to mycotoxin contamination. Huang et al. reported that out of 31 batches of Glycyrrhizae Radix et Rhizoma samples, mycotoxin contamination was detected in 17 batches, resulting in a detection rate of 54.8% (Huang et al., 2018). In this study, out of 36 batches of Radix Dipsaci samples, 28 batches were found to be contaminated with toxigenic fungi, resulting in an overall contamination rate of 44.4%. The elevated mycotoxin contamination in Radix Dipsaci and Glycyrrhizae Radix et Rhizoma could be attributed to its classification as a root herb with an extended growth cycle typically exceeding 3 years, necessitating prolonged soil contact during development, thus facilitating the colonization of toxigenic fungi from the soil. Moreover, sweating during processing predisposes Radix Dipsaci to toxigenic fungal growth. The coarse exterior of the herb promotes the adhesion of fungal spores, heightening its vulnerability to contamination throughout storage and transit. Consequently, Radix Dipsaci is susceptible to potential toxigenic fungal contamination across the phases of cultivation, processing, storage, and transit.
The invasion of toxigenic fungi into medicinal materials results in the release of mycotoxins, which serve to inhibit the growth of competing fungi, thereby enabling the toxigenic fungi to access more nutrients. Consequently, the invasion of toxigenic fungi and the production of mycotoxins can disrupt the microbial community of the host (Pfliegler et al., 2019). Our investigation revealed a noteworthy reduction in the Ace, Chao, and Shannon indices of fungal diversity, alongside a marked increase in the Simpson index, within mycotoxin-contaminated Radix Dipsaci, in contrast to uncontaminated samples. These findings indicate a diminishment in fungal richness and diversity due to mycotoxin contamination in Radix Dipsaci. These results suggest that contamination by mycotoxins decreases the richness and diversity of fungi in Radix Dipsaci. The results of beta diversity analysis revealed a distinct separation between the samples contaminated with individual mycotoxins (AFB1, AFG1, OTA), combinations of mycotoxins (AFB1 + AFG1, AFB1 + OTA, AFB1 + ZEN, AFB1 + AFG1 + OTA), and the uncontaminated samples along the principal component axis, indicating that mycotoxin contamination has the potential to alter the fungal community structure. We also observed that the predominant fungi in samples contaminated with AFs and OTA were of the genus Aspergillus, a significant source of toxigenic fungi responsible for various AFs and ochratoxins (Jedidi et al., 2017). The dominant fungi in samples contaminated with ZEN and T-2 were fungi of the genus Fusarium, which belong to a major source of toxigenic fungus responsible for various mycotoxins including ZEN, T-2, and deoxynivalenol (Tima et al., 2016). We observed a significant increase in the abundance of Aspergillus and Fusarium in mycotoxin-contaminated Radix Dipsaci, indicating that the mycotoxins in Radix Dipsaci may originate from Aspergillus and Fusarium fungi.
Studies have shown that the most carcinogenic mycotoxins are AFs, encompassing AFB1, AFB2, AFG1, and AFG2 (Wong and Hsieh, 1976). The contamination of AFs is a prevalent issue in Chinese herbal medicines, with the direct contact between the roots and soil of root Chinese herbal medicines being particularly susceptible to AFs contamination. Han et al. (2010) detected 16 batches contaminated with AFs out of a total of 30 batches of Chinese medicinal herbs, which included Puerariae Lobatae Radix, leading to a detection rate of 53.3%. Liu et al. (2012) revealed that the incidence rates of AFB1, AFB2, AFG1, and AFG2 in traditional Chinese medicine samples contaminated with AFs were 15.52, 14.37, 6.32, and 2.30% respectively. In this study, Radix Dipsaci was identified as a type of herbal root with an observed occurrence rate of AFs at 52.37%. Among the positive samples, AFB1, AFG1 and AFG2 were detected at rates of 34.92, 14.28 and 3.17%, respectively. The results showed that rhizome herbs such as Radix Dipsaci and Puerariae Lobatae Radix were very sensitive to AFs, particularly AFB1. The nutrient-rich properties of root herbs, such as Radix Dipsaci, may create an optimal growth environment for toxigenic fungi, thereby facilitating their contamination by AFs. Scientific literature has documented at least 16 Aspergillus species capable of producing AFs (Frisvad et al., 2019). A. flavus and A. parasiticus are recognized as the primary producers of AFs (Abrar et al., 2013). In this study, A. flavus was also isolated from Radix Dipsaci contaminated with AFB1 or AFG1. A. flavus exhibited a high frequency of isolation and expressed Aflr, a pivotal synthetic gene responsible for aflatoxin synthesis. Furthermore, it was shown that A. flavus had the ability to consistently produce AFB1 and AFG1. The results suggest that A. flavus contamination is the main factor contributing to the presence of AFB1 and AFG1 in Radix Dipsaci.
The group of ochratoxins includes OTA, ochratoxin B, and ochratoxin C (Qileng et al., 2018). Among these, OTA is the most toxic, demonstrating nephrotoxic, hepatotoxic, teratogenic, neurotoxic, and carcinogenic effects in animals (Tarazona et al., 2018; Yu et al., 2018). Currently, the issue of OTA contamination in traditional Chinese herbal medicine is increasingly severe, garnering significant attention from relevant stakeholders in society. Trucksess et al. (2007) identified OTA contamination in 29 out of 39 batches of Zingiberis Rhizoma Recens, resulting in a positive sample incidence rate of 74.4%; among the 10 batches of Ginseng Radix et Rhizoma, OTA contamination was detected in four batches, with a detection rate of 40.0%. The results of this study revealed that the detection rate of OTA in Radix Dipsaci, a type of root herb, was 19.04%. The susceptibility of Radix Dipsaci, Zingiberis Rhizoma Recens, and Ginseng Radix et Rhizoma to OTA contamination may be attributed to the high protein, polysaccharide, and starch content in root herbs like Radix Dipsaci. These components serve as carbon sources for toxigenic fungi, facilitating their growth and proliferation, ultimately leading to OTA contamination. The fungi identified in relevant studies as producers of OTA include A. westerdijkiae, A. steynii, A. niger, and P. verrucosum (Bui-Klimke and Wu, 2015; Gil-Serna et al., 2015). A. westerdijkiae was isolated from Radix Dipsaci samples contaminated with OTA. Subsequent investigations revealed that A. westerdijkiae exhibited the expression of PKS, a crucial synthetic gene responsible for OTA synthesis, and demonstrated continuous production of OTA in rice and Radix Dipsaci matrices, respectively. The results indicate that the contamination of A. westerdijkiae is the primary contributing factor to the presence of OTA in Radix Dipsaci.
ZEN is widely distributed and shares structural similarities with endogenous estrogen, enabling it to competitively bind to estrogen receptors (Gao et al., 2018; Sun et al., 2022). This interaction disrupts hormone synthesis and metabolism, leading to aberrant estrogenic effects, damage to reproductive organs, and disturbances in reproductive hormone levels (Santos-Cruz et al., 2023; Takemura et al., 2007). Studies have found that agricultural products such as Chinese herbs are susceptible to ZEN contamination. Liao et al. discovered that 28.16% of the samples from 103 batches of Chinese medicinal herbs, including Nelumbos Semen and Coicis Semen were contaminated with ZEN. Notably, the ZEN content in five batches of Coicis Semen samples exceeded the maximum residue limit (Liao et al., 2023). The presence of ZEN in small amounts has been detected in samples of Ginseng Radix et Rhizoma and Panacis Quinquefolii Radix, and the crude extract of Ginseng Radix et Rhizoma roots in accordance with related studies (Gray et al., 2004; Trucksess and Scott, 2008). Our study revealed that only three out of the 36 batches of Radix Dipsaci tested positive for ZEN contamination, resulting in a low detection rate of 4.76%. The findings indicated that seed herbs, such as Coicis Semen, exhibited higher susceptibility to ZEN contamination compared to root herbs, such as Radix Dipsaci. The susceptibility of seed herbs to ZEN contamination may be attributed to their high content of rich fats and proteins. Currently, it has been demonstrated that ZEN is a toxic secondary metabolite generated by multiple strains of Fusarium, including F. graminearum, F. verticillioides, F. oxysporum and F. acuminatum (Ropejko and Twaruzek, 2021). F. oxysporum was isolated from Radix Dipsaci contaminated with ZEN. Subsequent investigations unveiled that F. oxysporum displayed the expression of PKS14, a key biosynthetic gene responsible for ZEN production, and demonstrated sustained synthesis of ZEN in both rice and Radix Dipsaci matrices. The findings suggest that the presence of ZEN in Radix Dipsaci is primarily attributed to contamination by F. oxysporum.
T-2 is the most toxic type A trichothecene mycotoxin, exhibiting cytotoxic, genotoxic, reproductive toxic and neurotoxic effects (Li et al., 2022; Yang et al., 2021). The contamination of T-2 was found to be particularly prevalent in traditional Chinese herbs. Boško et al. (2022) analyzed the contamination of Silybi Fructus samples with T-2, and it was found that all the examined samples were contaminated. Similarly, Wang et al. (2014) discovered that out of 17 batches of Puerariae lobatae Radix samples, two batches were contaminated with T-2, resulting in a contamination rate of 11.8%. The current study detected the existence of T-2 contamination in two batches of samples, with a positive sample incidence rate of 3.17%. The findings indicated that fruiting herbs exhibited a higher susceptibility to T-2 contamination, whereas root herbs demonstrated a lower susceptibility. The presence of fruit herbs may contribute to a more conducive growth environment for toxigenic fungi, thereby leading to their T-2 contamination. T-2 is a type A trichothecene toxin produced by various Fusarium species, including F. graminearum, F. lateritium, F. oxysporum and F. tricinctum (Rabie et al., 1986). F. graminearum was isolated from Radix Dipsaci samples contaminated with T-2, which exhibited the expression of Tri7, a crucial gene involved in T-2 synthesis, and demonstrated its capability to produce T-2 in rice matrix. The findings suggest that contamination by F. graminearum may the primary factor contributing to the presence of T-2 in Radix Dipsaci.
Chinese herbal medicines are susceptible to mycotoxins, the presence of a substantial quantity of fungal spores in the planting soil, combined with high temperature and humidity conditions, creates an optimal environment for fungal growth (Gonzalez-Curbelo and Kabak, 2023; Ahmad et al., 2022). The presence of excess water in herbal medicine can additionally facilitate the proliferation of fungi. Inadequate implementation of comprehensive quality control measures, detection methods, risk assessment, and monitoring systems are also contributing factors. The current primary objective is to effectively manage mycotoxins in Chinese herbs, such as Radix Dipsaci, to ensure the quality and safety of Chinese herbs. Therefore, it is crucial to employ rational pesticide and fertilizer usage, implement thorough disinfection and soil improvement measures prior to planting, maintain appropriate water content levels during storage, conduct regular quality testing of medicinal materials, promptly isolate and treat contaminated varieties, develop industry standards and practices for mycotoxins, establish comprehensive standards for monitoring and testing the quality of traditional Chinese medicine, as well as regularly assess and monitor the risk of mycotoxin contamination in order to effectively prevent mycotoxin.
5 Conclusion
The findings indicate that Radix Dipsaci is susceptible to contamination by a combination of aflatoxins, OTA, ZEN, and T-2. The detection rate of aflatoxin exceeded the standard limit in 20.63% of the samples, and the OTA toxin in 7.93% of the samples exceeded the limit standard. Neither ZEN nor T-2 exceeded the standard limit. Aflatoxins, OTA, ZEN, and T-2 primarily originate from A. flavus, A. westerdijkiae, F. oxysporum and F. graminearum in Radix Dipsaci. Mycotoxins contamination has been implicated in the alteration of fungal communities within Radix Dipsaci, especially the increase of the abundance of Aspergillus and Fusarium. To ensure the safety of Radix Dipsaci, future work is needed to monitor more mycotoxins, such as fumonisins and deoxynivalenol. Our findings presented in this study contribute significantly to a more comprehensive understanding of the causal agents responsible for mycotoxin contamination in Radix Dipsaci and their potential implications for consumer safety. The available data also offers valuable insights into the monitoring and control of other Chinese medicinal herbs that may potentially be contaminated by toxigenic fungi.
Data availability statement
The datasets presented in this study can be found in online repositories. The names of the repository/repositories and accession number(s) can be found in the article/Supplementary material.
Author contributions
MH: Investigation, Methodology, Software, Validation, Writing – original draft, Writing – review & editing. LW: Methodology, Writing – original draft. DS: Investigation, Methodology, Validation, Writing – review & editing. QY: Visualization, Writing – review & editing. CX: Data curation, Writing – review & editing. LG: Conceptualization, Supervision, Writing – review & editing. MW: Methodology, Validation, Writing – review & editing. CK: Software, Writing – review & editing. JZ: Conceptualization, Funding acquisition, Methodology, Project administration, Supervision, Writing – review & editing. TZ: Funding acquisition, Project administration, Resources, Writing – review & editing.
Funding
The author(s) declare that financial support was received for the research, authorship, and/or publication of this article. This work was supported by the Scientific and Technological Innovation Project of China Academy of Chinese Medical Science (CI2021B013); the University Science and Technology Innovation Team of the Guizhou Provincial Department of Education [Qian-Jiao-Ji (2023)071]; the Research Platform Team Project of the Education Department of Guizhou Province [Qian-Jiao-Ji (2022)021]; the National and Provincial Scientific and Technological Innovation Talent Team of Guizhou University of Traditional Chinese Medicine [Gui-Zhong-Yi TD He-Zi (2022)003]; and the Graduate Education Innovation Program of Guizhou University of Traditional Chinese Medicine (YCXKYS2023007).
Acknowledgments
The authors are grateful to Creaducate Consulting GmbH (Munich, Germany) for editorial assistance.
Conflict of interest
The authors declare that the research was conducted in the absence of any commercial or financial relationships that could be construed as a potential conflict of interest.
Publisher’s note
All claims expressed in this article are solely those of the authors and do not necessarily represent those of their affiliated organizations, or those of the publisher, the editors and the reviewers. Any product that may be evaluated in this article, or claim that may be made by its manufacturer, is not guaranteed or endorsed by the publisher.
Supplementary material
The Supplementary material for this article can be found online at: https://www.frontiersin.org/articles/10.3389/fmicb.2024.1454683/full#supplementary-material
Footnotes
References
Abrar, M., Anjum, F. M., Butt, M. S., Pasha, I., Randhawa, M. A., Saeed, F., et al. (2013). Aflatoxins: biosynthesis, occurrence, toxicity, and remedies. Crit. Rev. Food Sci. Nutr. 53, 862–874. doi: 10.1080/10408398.2011.563154
Ahmad, R. R., Bano, H., Ahmad, P. S., Perveen, K., Al Masoudi, L. M., Saud, A. S., et al. (2022). Anthropogenic impacts on phytosociological features and soil microbial health of Colchicum luteum L. an endangered medicinal plant of North Western Himalaya. Saudi J. Biol. Sci. 29, 2856–2866. doi: 10.1016/j.sjbs.2022.01.011
Ashiq, S., Hussain, M., and Ahmad, B. (2014). Natural occurrence of mycotoxins in medicinal plants: a review. Fungal Genet. Biol. 66, 1–10. doi: 10.1016/j.fgb.2014.02.005
Boško, R., Pernica, M., Belakova, S., Bjelkova, M., and Pluhackova, H. (2022). Determination of T-2 and HT-2 toxins in seed of milk thistle [Silybum marianum (L.) Gaertn.] using immunoaffinity column by UPLC-MS/MS. Toxins 14:258. doi: 10.3390/toxins14040258
Bui-Klimke, T. R., and Wu, F. (2015). Ochratoxin and human health risk: a review of the evidence. Crit. Rev. Food Sci. Nutr. 55, 1860–1869. doi: 10.1080/10408398.2012.724480
Chen, L., Guo, W., Zheng, Y., Zhou, J., Liu, T., Chen, W., et al. (2020). Occurrence and characterization of fungi and mycotoxins in contaminated medicinal herbs. Toxins 12:30. doi: 10.3390/toxins12010030
Chen, A. J., Tang, D., Zhou, Y. Q., Sun, B. D., Li, X. J., Wang, L. Z., et al. (2013). Identification of ochratoxin A producing fungus associated with fresh and dry liquorice. PLoS One 8:e78285. doi: 10.1371/journal.pone.0078285
Du, W., Lv, Y., Wu, H., Li, Y., Tang, R., Zhao, M., et al. (2023). Research on the effect of Dipsaci Radix before and after salt-processed on kidney yang deficiency syndrome rats and the preliminary mechanism study through the BMP-Smad signaling pathway. J. Ethnopharmacol. 312:116480. doi: 10.1016/j.jep.2023.116480
England, C., TrejoMartinez, J., PerezSanchez, P., Karki, U., and Xu, J. (2023). Plants as biofactories for therapeutic proteins and antiviral compounds to combat COVID-19. Life 13:617. doi: 10.3390/life13030617
Fang, M., Hu, W., and Liu, B. (2022). Protective and detoxifying effects conferred by selenium against mycotoxins and livestock viruses: a review. Front. Vet. Sci. 9:956814. doi: 10.3389/fvets.2022.956814
Frisvad, J. C., Hubka, V., Ezekiel, C. N., Hong, S. B., Novakova, A., Chen, A. J., et al. (2019). Taxonomy of aspergillus section flavi and their production of aflatoxins, ochratoxins and other mycotoxins. Stud. Mycol. 93, 1–63. doi: 10.1016/j.simyco.2018.06.001
Gao, X., Xiao, Z., Li, C., Zhang, J., Zhu, L., Sun, L., et al. (2018). Prenatal exposure to zearalenone disrupts reproductive potential and development via hormone-related genes in male rats. Food Chem. Toxicol. 116, 11–19. doi: 10.1016/j.fct.2018.04.011
Gavrilova, O. P., Gagkaeva, T. Y., Orina, A. S., and Gogina, N. N. (2023). Diversity of Fusarium species and their mycotoxins in cereal crops from the asian territory of Russia. Dokl. Biol. Sci. 508, 9–19. doi: 10.1134/S0012496622700156
Ge, Y., Wang, L., Su, D., Yuan, Q., Xiao, C., Hu, M., et al. (2024). The sweating process promotes toxigenic fungi expansion and increases the risk of combined contamination of mycotoxins in Radix Dipsaci. Front. Microbiol. 15:1394774. doi: 10.3389/fmicb.2024.1394774
Gil-Serna, J., Patino, B., Cortes, L., Gonzalez-Jaen, M. T., and Vazquez, C. (2015). Aspergillus steynii and Aspergillus westerdijkiae as potential risk of ota contamination in food products in warm climates. Food Microbiol. 46, 168–175. doi: 10.1016/j.fm.2014.07.013
Gonzalez-Curbelo, M. A., and Kabak, B. (2023). Occurrence of mycotoxins in dried fruits worldwide, with a focus on aflatoxins and ochratoxin A: a review. Toxins 15:576. doi: 10.3390/toxins15090576
Gray, S. L., Lackey, B. R., Tate, P. L., Riley, M. B., and Camper, N. D. (2004). Mycotoxins in root extracts of American and Asian ginseng bind estrogen receptors alpha and beta. Exp. Biol. Med. 229, 560–568. doi: 10.1177/153537020422900615
Han, Z., Ren, Y., Zhu, J., Cai, Z., Chen, Y., Luan, L., et al. (2012). Multianalysis of 35 mycotoxins in traditional Chinese medicines by ultra-high-performance liquid chromatography-tandem mass spectrometry coupled with accelerated solvent extraction. J. Agric. Food Chem. 60, 8233–8247. doi: 10.1021/jf301928r
Han, Z., Zheng, Y., Luan, L., Cai, Z., Ren, Y., and Wu, Y. (2010). An ultra-high-performance liquid chromatography-tandem mass spectrometry method for simultaneous determination of aflatoxins B1, B2, G1, G2, M1 and M2 in traditional Chinese medicines. Anal. Chim. Acta 664, 165–171. doi: 10.1016/j.aca.2010.02.009
He, H., Xu, J., Zhou, T., Yang, Y., Yang, C., Xiao, C., et al. (2023). Metabolomic and microbiomic insights into color changes during the sweating process in Dipsacus asper. Front. Microbiol. 14:1195088. doi: 10.3389/fmicb.2023.1195088
Huang, X., Wang, S., Mao, D., Miao, S., Hu, Q., and Ji, S. (2018). Optimized QuEChERS method combined with UHPLC-MS/MS for the simultaneous determination of 15 mycotoxins in liquorice. J. AOAC Int. 101, 633–642. doi: 10.5740/jaoacint.17-0365
Jedidi, I., Cruz, A., Gonzalez-Jaen, M. T., and Said, S. (2017). Aflatoxins and ochratoxin and their Aspergillus causal species in Tunisian cereals. Food Addit. Contam. B 10, 51–58. doi: 10.1080/19393210.2016.1247917
Ke, K., Li, Q., Yang, X., Xie, Z., Wang, Y., Shi, J., et al. (2016). Asperosaponin VI promotes bone marrow stromal cell osteogenic differentiation through the PI3K/AKT signaling pathway in an osteoporosis model. Sci. Rep. 6:35233. doi: 10.1038/srep35233
Li, S. J., Zhang, G., Xue, B., Ding, Q., Han, L., Huang, J. C., et al. (2022). Toxicity and detoxification of T-2 toxin in poultry. Food Chem. Toxicol. 169:113392. doi: 10.1016/j.fct.2022.113392
Liao, X., Li, Y., Long, N., Xu, Q., Li, P., Wang, J., et al. (2023). Multi-mycotoxin detection and human exposure risk assessment in medicinal foods. Food Res. Int. 164:112456. doi: 10.1016/j.foodres.2023.112456
Liew, W. P., and Sabran, M. R. (2022). Recent advances in immunoassay-based mycotoxin analysis and toxicogenomic technologies. J. Food Drug Anal. 30, 549–561. doi: 10.38212/2224-6614.3430
Liu, L., Jin, H., Sun, L., Ma, S., and Lin, R. (2012). Determination of aflatoxins in medicinal herbs by high-performance liquid chromatography-tandem mass spectrometry. Phytochem. Anal. 23, 469–476. doi: 10.1002/pca.2343
Long, N., Liu, J., Liao, X., Jia, B., Liu, J., Zhou, L., et al. (2021). Fungal communities in Nelumbinis semen characterized by high-throughput sequencing. Int. J. Food Microbiol. 359:109428. doi: 10.1016/j.ijfoodmicro.2021.109428
Marin, D. E., Pistol, G. C., Neagoe, I. V., Calin, L., and Taranu, I. (2013). Effects of zearalenone on oxidative stress and inflammation in weanling piglets. Food Chem. Toxicol. 58, 408–415. doi: 10.1016/j.fct.2013.05.033
Markov, A. V., Sen’Kova, A. V., Warszycki, D., Salomatina, O. V., Salakhutdinov, N. F., Zenkova, M. A., et al. (2017). Soloxolone methyl inhibits influenza virus replication and reduces virus-induced lung inflammation. Sci. Rep. 7:13968. doi: 10.1038/s41598-017-14029-0
Moretti, A., Logrieco, A. F., and Susca, A. (2017). Mycotoxins: an underhand food problem. Methods Mol. Biol. 1542, 3–12. doi: 10.1007/978-1-4939-6707-0_1
Perrone, G., and Susca, A. (2017). Penicillium species and their associated mycotoxins. Methods Mol. Biol. 1542, 107–119. doi: 10.1007/978-1-4939-6707-0_5
Pfliegler, W. P., Pocsi, I., Gyori, Z., and Pusztahelyi, T. (2019). The Aspergilli and their mycotoxins: metabolic interactions with plants and the soil biota. Front. Microbiol. 10:2921. doi: 10.3389/fmicb.2019.02921
Qileng, A., Wei, J., Lu, N., Liu, W., Cai, Y., Chen, M., et al. (2018). Broad specificity photoelectrochemical immunoassay for the simultaneous detection of ochratoxin A, ochratoxin B and ochratoxin C. Biosens. Bioelectron. 106, 219–226. doi: 10.1016/j.bios.2018.02.004
Rabie, C. J., Sydenham, E. W., Thiel, P. G., Lubben, A., and Marasas, W. F. (1986). T-2 toxin production by Fusarium acuminatum isolated from oats and barley. Appl. Environ. Microbiol. 52, 594–596. doi: 10.1128/aem.52.3.594-596.1986
Ropejko, K., and Twaruzek, M. (2021). Zearalenone and its metabolites-general overview, occurrence, and toxicity. Toxins. 13. doi: 10.3390/toxins13010035
Rundberget, T., Skaar, I., and Flaoyen, A. (2004). The presence of Penicillium and Penicillium mycotoxins in food wastes. Int. J. Food Microbiol. 90, 181–188. doi: 10.1016/s0168-1605(03)00291-5
Santos-Cruz, L. F., Ponciano-Gomez, A., Torres-Gregorio, J. T., Ramirez-Cruz, B. G., Vazquez-Gomez, G., Hernandez-Portilla, L. B., et al. (2023). Zearalenone does not show genotoxic effects in the Drosophila melanogaster wing spot test, but it induces oxidative imbalance, development, and fecundity alterations. Toxins 15:358. doi: 10.3390/toxins15060358
Schrenk, D., Bodin, L., Chipman, J. K., Del, M. J., Grasl-Kraupp, B., Hogstrand, C., et al. (2020). Risk assessment of ochratoxin A in food. EFSA J. 18:e6113. doi: 10.2903/j.efsa.2020.6113
Sen, T., and Samanta, S. K. (2015). Medicinal plants, human health and biodiversity: a broad review. Adv. Biochem. Eng. Biotechnol. 147, 59–110. doi: 10.1007/10_2014_273
Singh, P., Srivastava, B., Kumar, A., and Dubey, N. K. (2008). Fungal contamination of raw materials of some herbal drugs and recommendation of Cinnamomum camphora oil as herbal fungitoxicant. Microb. Ecol. 56, 555–560. doi: 10.1007/s00248-008-9375-x
Stockmann-Juvala, H., and Savolainen, K. (2008). A review of the toxic effects and mechanisms of action of fumonisin B1. Hum. Exp. Toxicol. 27, 799–809. doi: 10.1177/0960327108099525
Su, C., Hu, Y., Gao, D., Luo, Y. I., Chen, A. J., Jiao, X., et al. (2018). Occurrence of toxigenic fungi and mycotoxins on root herbs from Chinese markets. J. Food Prot. 81, 754–761. doi: 10.4315/0362-028X.JFP-17-405
Sun, Y., Qi, S., Dong, X., Qin, M., Zhang, Y., and Wang, Z. (2022). Colorimetric aptasensor targeting zearalenone developed based on the hyaluronic acid-DNA hydrogel and bimetallic MOFzyme. Biosens. Bioelectron. 212:114366. doi: 10.1016/j.bios.2022.114366
Takemura, H., Shim, J. Y., Sayama, K., Tsubura, A., Zhu, B. T., and Shimoi, K. (2007). Characterization of the estrogenic activities of zearalenone and zeranol in vivo and in vitro. J. Steroid Biochem. Mol. Biol. 103, 170–177. doi: 10.1016/j.jsbmb.2006.08.008
Tao, Y., Chen, L., and Yan, J. (2020). Traditional uses, processing methods, phytochemistry, pharmacology and quality control of Dipsacus asper Wall. ex C.B. Clarke: a review. J. Ethnopharmacol. 258:112912. doi: 10.1016/j.jep.2020.112912
Tarazona, A., Gomez, J. V., Gavara, R., Mateo-Castro, R., Gimeno-Adelantado, J. V., Jimenez, M., et al. (2018). Risk management of ochratoxigenic fungi and ochratoxin A in maize grains by bioactive EVOH films containing individual components of some essential oils. Int. J. Food Microbiol. 269, 107–119. doi: 10.1016/j.ijfoodmicro.2018.02.002
Tima, H., Racz, A., Guld, Z., Mohacsi-Farkas, C., and Kisko, G. (2016). Deoxynivalenol, zearalenone and T-2 in grain based swine feed in Hungary. Food Addit. Contam. B 9, 275–280. doi: 10.1080/19393210.2016.1213318
Trucksess, M. W., and Scott, P. M. (2008). Mycotoxins in botanicals and dried fruits: a review. Food Addit. Contam. A 25, 181–192. doi: 10.1080/02652030701567459
Trucksess, M. W., Weaver, C. M., Oles, C. J., Rump, L. V., White, K. D., Betz, J. M., et al. (2007). Use of multitoxin immunoaffinity columns for determination of aflatoxins and ochratoxin A in ginseng and ginger. J. AOAC Int. 90, 1042–1049. doi: 10.1093/jaoac/90.4.1042
Wang, S., Cheng, L., Ji, S., and Wang, K. (2014). Simultaneous determination of seventeen mycotoxins residues in Puerariae lobatae radix by liquid chromatography-tandem mass spectrometry. J. Pharm. Biomed. Anal. 98, 201–209. doi: 10.1016/j.jpba.2014.05.037
Wei, G., Guo, X., Liang, Y., Liu, C., Zhang, G., Liang, C., et al. (2023). Occurrence of fungi and mycotoxins in herbal medicines and rapid detection of toxin-producing fungi. Environ. Pollut. 333:122082. doi: 10.1016/j.envpol.2023.122082
Wong, J. J., and Hsieh, D. P. (1976). Mutagenicity of aflatoxins related to their metabolism and carcinogenic potential. Proc. Natl. Acad. Sci. U.S.A. 73, 2241–2244. doi: 10.1073/pnas.73.7.2241
Wu, H., Lv, Y., Wei, F., Li, C., Ge, W., and Du, W. (2022). Comparative analysis of anti-osteoporosis efficacy in Radix Dipsaci before and after processing with salt based on spectrum-effect relationship. J. Pharm. Biomed. Anal. 221:115078. doi: 10.1016/j.jpba.2022.115078
Yang, C., Jiang, W., Su, D., Yang, C., Yuan, Q., Kang, C., et al. (2024). Contamination of the traditional medicine Radix Dipsaci with aflatoxin b1 impairs hippocampal neurogenesis and cognitive function in a mouse model of osteoporosis. Ecotoxicol. Environ. Saf. 283:116831. doi: 10.1016/j.ecoenv.2024.116831
Yang, X., Liu, P., Zhang, X., Zhang, J., Cui, Y., Song, M., et al. (2021). T-2 toxin causes dysfunction of sertoli cells by inducing oxidative stress. Ecotoxicol. Environ. Saf. 225:112702. doi: 10.1016/j.ecoenv.2021.112702
Yao, Y., and Long, M. (2020). The biological detoxification of deoxynivalenol: a review. Food Chem. Toxicol. 145:111649. doi: 10.1016/j.fct.2020.111649
Yu, Z., Wu, F., Tian, J., Guo, X., and An, R. (2018). Protective effects of compound ammonium glycyrrhizin, L‑arginine, silymarin and glucurolactone against liver damage induced by ochratoxin A in primary chicken hepatocytes. Mol. Med. Rep. 18, 2551–2560. doi: 10.3892/mmr.2018.9285
Zhao, D. T., Gao, Y. J., Zhang, W. J., Bi, T. C., Wang, X., Ma, C. X., et al. (2021). Development a multi-immunoaffinity column LC-MS-MS method for comprehensive investigation of mycotoxins contamination and co-occurrence in traditional Chinese medicinal materials. J. Chromatogr. B 1178:122730. doi: 10.1016/j.jchromb.2021.122730
Keywords: traditional Chinese medicinal herbs, Radix Dipsaci , toxigenic fungi, mycotoxins, aflatoxins
Citation: Hu M, Wang L, Su D, Yuan Q, Xiao C, Guo L, Wang M, Kang C, Zhang J and Zhou T (2024) Evaluation of mycotoxins, mycobiota and toxigenic fungi in the traditional medicine Radix Dipsaci. Front. Microbiol. 15:1454683. doi: 10.3389/fmicb.2024.1454683
Edited by:
Laurent Dufossé, Université de la Réunion, FranceReviewed by:
Carlos Augusto Fernandes Oliveira, University of São Paulo, BrazilAbhay K. Pandey, North Bengal Regional R & D Center, India
Copyright © 2024 Hu, Wang, Su, Yuan, Xiao, Guo, Wang, Kang, Zhang and Zhou. This is an open-access article distributed under the terms of the Creative Commons Attribution License (CC BY). The use, distribution or reproduction in other forums is permitted, provided the original author(s) and the copyright owner(s) are credited and that the original publication in this journal is cited, in accordance with accepted academic practice. No use, distribution or reproduction is permitted which does not comply with these terms.
*Correspondence: Jinqiang Zhang, NTUyNDUwMzc0QHFxLmNvbQ==; Tao Zhou, dGFvemhvdTg4QDE2My5jb20=