- 1College of Food Science and Light Industry, Nanjing Tech University, Nanjing, China
- 2Institute of Applied Microbiology, Xinjiang Academy of Agricultural Sciences/ Xinjiang Key Laboratory of Special Environmental Microbiology, Urumqi, China
- 3College of Life Sciences, Xinjiang Normal University, Urumqi, China
- 4School of Chemistry and Molecular Engineering, Nanjing Tech University, Nanjing, China
- 5School of Pharmaceutical Sciences, Nanjing Tech University, Nanjing, China
- 6China Astronaut Research and Training Center, Beijing, China
- 7State Key Laboratory of Materials-Oriented Chemical Engineering, College of Food Science and Light Industry, Nanjing Tech University, Nanjing, China
The ability of Deinococcus bacteria to survive in harsh environments, such as high radiation, extreme temperature, and dryness, is mainly attributed to the generation of unique pigments, especially carotenoids. Although the limited number of natural pigments produced by these bacteria restricts their industrial potential, metabolic engineering and synthetic biology can significantly increase pigment yield and expand their application prospects. In this study, we review the properties, biosynthetic pathways, and functions of key enzymes and genes related to these pigments and explore strategies for improving pigment production through gene editing and optimization of culture conditions. Additionally, studies have highlighted the unique role of these pigments in antioxidant activity and radiation resistance, particularly emphasizing the critical functions of deinoxanthin in D. radiodurans. In the future, Deinococcus bacterial pigments will have broad application prospects in the food industry, drug production, and space exploration, where they can serve as radiation indicators and natural antioxidants to protect astronauts’ health during long-term space flights.
1 Introduction
Color plays a pivotal role in food production and processing, serving to facilitate product identification, preserve sensory quality, enhance original colors, preserve nutritional value, and improve consumer acceptance (Ghosh et al., 2024). Therefore, for many foods, adding food colorants prior to final packaging or consumption is an indispensable component of their formulation. Chemically synthesized colorants have gained popularity as they offer advantages such as cost-effectiveness in production, high tinctorial strength, and robust chemical stability (Sen et al., 2019). However, concerns regarding potential adverse effects associated with synthetic colorants, including hyperactivity in children, allergenicity, toxicological issues, and carcinogenicity problems, have prompted the prohibition of several synthetic food colorants (Sen et al., 2019). This has subsequently led to a transition from the use of synthetic food colorants to natural ones. Natural pigments derived from plants, microorganisms, minerals, insects, and marine organisms present promising alternatives to synthetic food colorants (Park et al., 2020). Among them, microbes produce a variety of pigments that can be used as additives, antioxidants, color intensifiers, and functional food ingredients (Rajendran et al., 2023).
Bacterial pigments are a diverse class of compounds produced by microorganisms during their physiological processes and are widely present in nature (Venil et al., 2013). These pigments not only play important roles in the ecological adaptation of bacteria but also have a variety of potential industrial applications (Orlandi et al., 2022). Bacterial pigments include carotenoids, melanin, phycobilin, and flavonoids. Among them, carotenoids have attracted much attention due to their diverse physiological functions and unique physicochemical properties (Celedón and Díaz, 2021). Carotenoids are a class of polyene compounds composed of 40 or 50 carbon atoms that are widely found in plants, algae, fungi, and bacteria (Maoka, 2020; Giani et al., 2024). They not only give organisms bright colors but also possess various biological functions such as antioxidant activity, photoprotection, and immune regulation (Esteban et al., 2015; Maoka, 2020; Flieger et al., 2024). In bacteria, the synthesis pathways and functions of carotenoids are diverse. They involve the synergistic action of multiple genes and enzymes, which makes the study of bacterial carotenoids one of the hotspots (Liang et al., 2018; Wurtzel, 2019).
Currently, extremophiles have emerged as promising bioresources for the production of diverse pigments due to their involvement in crucial cellular functions necessary for survival in extreme habitats (Jeong et al., 2021). The phylum Deinococcus–Thermus is one group of extremophiles that possesses the ability to synthesize carotenoids, resulting in the formation of colonies exhibiting red, orange, and pink colors (Tian and Hua, 2010). Compared to other carotenoids, those produced by Deinococcus species demonstrate excellent scavenging activity against singlet oxygen and hydrogen peroxide, thereby exhibiting enhanced antioxidant effects (Jeong et al., 2021). Consequently, they have garnered attention as the next-generation organic compounds and hold great potential as natural ingredients in food supplements.
Here, we review the bacterial pigments known as carotenoids, which are found in Deinococcus bacteria either naturally or through genetic engineering. We introduce their physicochemical properties and applications in various fields. The biosynthetic pathway of carotenoids is analyzed, and the key enzymes involved in the pathway are introduced individually. In addition, we discuss metabolic engineering techniques used to enhance carotenoid production in Deinococcus, including genetic engineering and modifications to culture conditions aimed at improving yield. We also explore gene editing technology for producing other types of carotenoids and heterologous expression of pathway genes for carotenoid production. Finally, the roles of these carotenoids in conferring stress resistance to Deinococcus bacteria were summarized, highlighting their importance in combating oxidative stress.
2 Extremophilic Deinococcus bacteria
There are numerous types of bacteria on Earth, and they exist in nearly all environments (Poli et al., 2017). Specifically, certain bacteria can even survive in extreme environments such as high temperatures, high pressures, and acidic or alkaline conditions (Shu and Huang, 2022). The radiation environment is an extreme condition because it can damage the genetic material DNA and other biological macromolecules of living organisms (Romanovskaya et al., 2002). Deinococcus radiodurans (D. radiodurans) can tolerate lethal doses of ionizing radiation and ultraviolet radiation (Nayak et al., 2021). It belongs to the Deinococcaceae family, and bacteria in this family play an important role in bioremediation due to their high tolerance to radiation (Basu, 2022). Most bacteria in the genus Deinococcus are Gram-positive but have the distinctive feature of Gram-negative bacteria, that is, the cells are coated with an outer membrane. The majority of these bacteria present positive on the Gram stain test, with a few exceptions, such as D. grandis. In addition, these bacteria all possess a thick sacculus characteristic of Gram-positive bacteria (Griffiths and Gupta, 2004). In terms of colony morphology, colonies belonging to this genus appear smooth, raised, and round-shaped with regular edges. Moreover, the colors of these colonies are rich and diverse, ranging from pink to red due to the unique carotenoids produced by them.
Deinococcus radiodurans and other bacteria of the genus Deinococcus are highly radioresistant and can survive in ionizing radiation at doses up to 5 kilogray (kGy). In contrast, radiation doses of a few hundred gray are sufficient to kill most known bacterial species, such as Escherichia coli (E. coli), while 5–10 gray is lethal for most vertebrates, including humans (Daly, 2012). An in-depth study of D. radiodurans will not only reveal the mechanism of radiation resistance in Deinococcus but also provide valuable insights into radiation resistance or sensitivity in other organisms. At present, most studies suggest that factors affecting the radiation resistance of bacteria mainly include the DNA repair system (Villa et al., 2021; Han et al., 2022), oxidative stress system (Han et al., 2020; Chen et al., 2021), and metabolic regulation system (Lim et al., 2019; Jeong et al., 2021). The oxidative stress system comprises non-enzymatic antioxidant systems such as carotenoids, bacillus thiol, and manganese complexes (Sun et al., 2010; Tian and Hua, 2010; Luan et al., 2014). Deinoxanthin, the major carotenoid in D. radiodurans, plays a crucial role in its antioxidant capacity and resistance against DNA damage (Sadowska-Bartosz and Bartosz, 2023). This carotenoid contributes significantly to cell defense systems and aids bacterial survival and proliferation in harsh environments (Günay and Kuzucu, 2023). Further studies have also revealed that D. radiodurans enhances its resistance to environmental stress through its carotenoid synthesis pathway, providing new insights into the environmental adaptability of microorganisms (Tapia et al., 2021).
Carotenoids produced by Deinococcus bacteria not only contribute to their survival in extreme environments but also have become a research hotspot due to their potential health benefits. For example, deinoxanthin produced by D. sp. UDEC-P1 showed inhibitory effects on certain cancer cells, suggesting the potential of these pigments as supplements for anticancer therapies (Tapia et al., 2021). In addition, owing to its excellent antioxidant properties, deinoxanthin has also been investigated as a potential ingredient in food additives and nutraceuticals (Jeong et al., 2021). Through genetic and metabolic engineering techniques, researchers have successfully enhanced the production capacity of deinoxanthin in D. radiodurans, laying the foundation for its commercial application.
3 Bacterial pigments in the genus Deinococcus
The colonies of most strains in the Deinococcus genus are orange or pink, a characteristic attributed to their ability to produce pigments. These pigments, produced by Deinococcus, serve diverse functions and are among the primary factors that enable these radiation-resistant bacteria to defend themselves against intense radiation and extremely high temperatures.
3.1 Deinoxanthin
3.1.1 Physicochemical properties of deinoxanthin
Deinoxanthin, a distinctive carotenoid, was first identified in D. radiodurans, which is a bacterium renowned for its remarkable resistance to ionizing radiation and other environmental stresses. The chemical structure of deinoxanthin is (all-E)-2,1′-dihydroxy-3′,4′-didehydro-1′,2′-dihydro-β, ψ-carotene-4-one-1 (Sadowska-Bartosz and Bartosz, 2023), which consists of an unsaturated carbon chain with a hydroxyl group on the end carbon atom (C-1′) and a hexatomic ring containing a hydroxyl group and a ketonic group on the C-2 and C-4 of the ring, respectively (Figure 1). The unique chemical structure forms the basis for its bio-function, as it contains 12 conjugated double bonds in the unsaturated carbon chain along with functional groups such as the hydroxyl group and ketonic group on the terminal ring (Figure 1). The antioxidant capacity of carotenoids is related to the length of their conjugated double bond system and the presence of functional groups (Albrecht et al., 2000). For example, bacterioruberin pigments with 13 conjugated double bonds exhibited superior antioxidant properties compared to β-carotene, which only comprises nine conjugated double bonds (Noby et al., 2023). Moreover, studies suggest that pigment color is also linked to the conjugated system, which can absorb specific wavelengths of light and thus produce its special color (Britton, 1995). It has been reported that conjugated systems with less than eight double bonds appear colorless to the human eyes and only absorb in the UV region. For example, there are 11 conjugated double bonds in the β-carotenoid and zeaxanthin with yellow color but none of them in the colorless phytoene, while the red color was observed in bacterioruberin due to the longer conjugated double bond system (Palanisamy and Ramalingam, 2024).
3.1.2 Antioxidant effect of deinoxanthin
The effects of ionizing radiation (e.g., γ-radiation and X-ray) on microbial cells are mainly caused by direct and indirect effects. The direct effect is that ionizing radiation can directly penetrate the cell membrane and destroy genetic material. The indirect effect is the radiolysis of water inside and outside the cells into reactive oxygen species (ROS), thereby indirectly damaging DNA, RNA, and protein (Figure 2) (Tian et al., 2004; Sadowska-Bartosz and Bartosz, 2023). The ROS-scavenging system is vital for D. radiodurans to decrease the impact of ROS damage, which consists of antioxidant enzymes (e.g., superoxide dismutase and catalase) and non-enzymatic antioxidants (e.g., carotenoids and metal ions). Carotenoids are strong ROS scavengers, especially singlet oxygen among non-enzymatic antioxidants (Tian et al., 2009). Deinoxanthin is a more effective ROS scavenger than other well-known carotenes, xanthophylls, and ascorbic acids (Cheng et al., 2014; Kim et al., 2021). It can react directly with free radicals and neutralize them (Yu et al., 2015). This effect is related to the conjugated double bond system in deinoxanthin that stabilizes unstable electrons formed by free radicals. Structural analysis of the S-layer deinoxanthin-binding complex in D. radiodurans further underscores the importance of deinoxanthin in the bacterium’s ability to withstand environmental challenges while highlighting its role in protective mechanisms against such challenges (Farci et al., 2022).
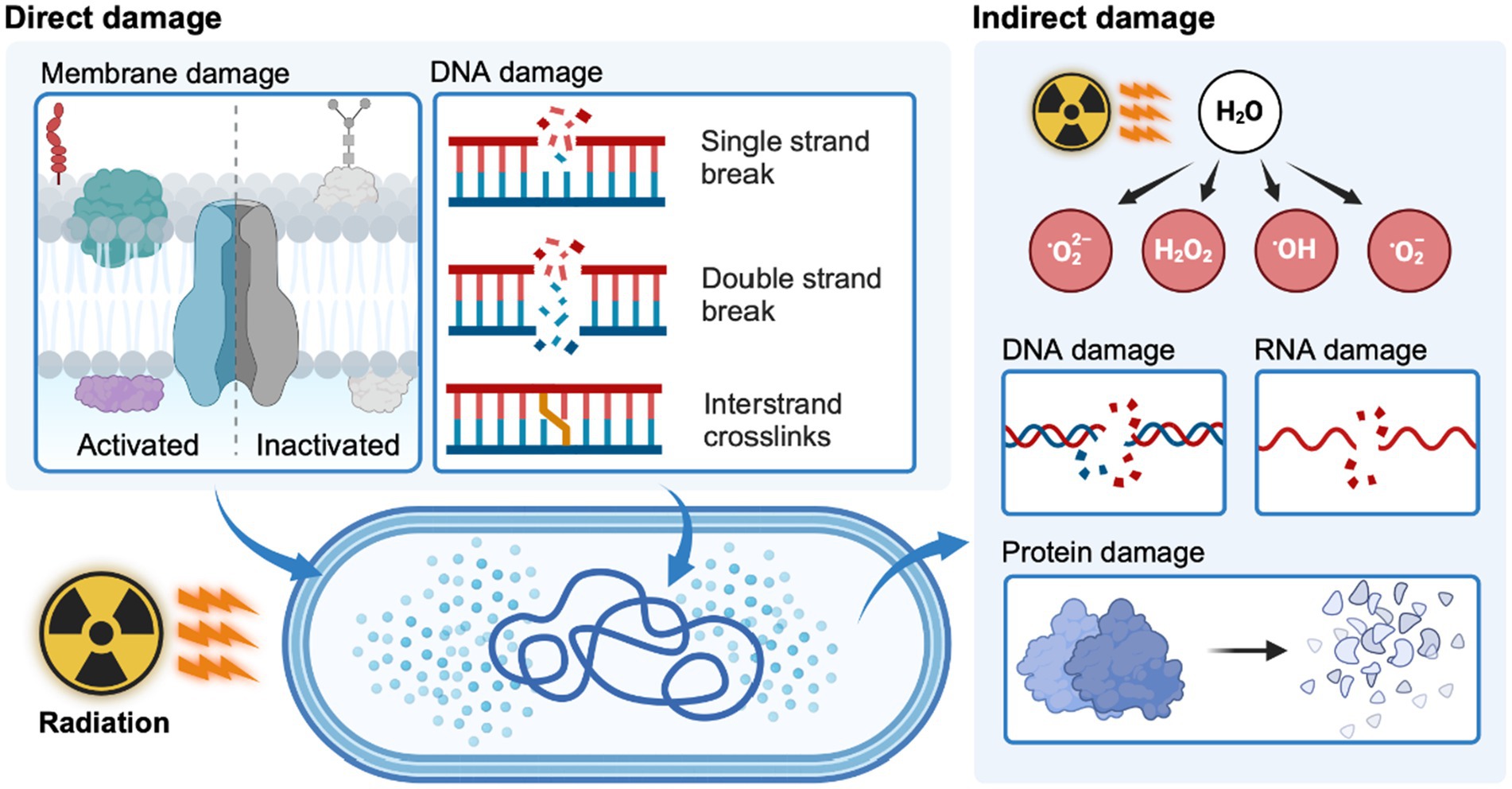
Figure 2. Mechanisms of direct and indirect radiation damage in bacteria. Created with BioRender.com.
3.1.3 Anticancer ability of deinoxanthin
Carotenoids such as β-carotene, lycopene, and lutein can induce apoptosis of a variety of cancer cells in animals and have an efficient effect in inhibiting carcinogenesis (Tanaka et al., 2012). The scavenging ability of deinoxanthin for active oxygen is stronger than that of these carotenoids. Therefore, it is also very important to study the anticancer effect of deinoxanthin. Current research has found that deinoxanthin can induce apoptosis in cancer cells such as liver cancer (Choi et al., 2014), colon cancer (Choi et al., 2014), prostate cancer (Choi et al., 2014), osteosarcoma (Tapia et al., 2021), and breast cancer (Tian et al., 2018; Günay and Kuzucu, 2023). Choi et al. (2014) found that deinoxanthin induced apoptosis through chromatin condensation and nuclear fragmentation in HepG2 liver cancer, HT-29 colon cancer, and PC-3 prostate cancer cells by releasing BAX and activating Caspase-3. Similarly, Tapia et al. (2021) found that deinoxanthin significantly reduced the proliferation activity of Saos-2 osteosarcoma cells by approximately 37.1%. In addition, tamoxifen has shown strong efficacy against breast cancer, and combining deinoxanthin with tamoxifen can enhance its anti-proliferation activity on cancer cells by leveraging its potential antioxidant effect and apoptosis-inducing ability (Günay and Kuzucu, 2023).
3.1.4 Deinoxanthin derivatives
In addition to the well-known deinoxanthin, D. radiodurans also produces a unique carotenoid identified as (all-E)-1′-hydroxy-3′,4′-didehydro-1′,2′,2,3-quahydro-β, ψ-carotene-4-one-1 (Yang, 2021). The characterization of this carotenoid, named 2-deoxydeinoxanthin, is the precursor of deinoxanthin (Sadowska-Bartosz and Bartosz, 2023). The structural difference between this pigment and deinoxanthin is that the hydroxyl group on C-2 of the benzene ring is replaced by hydrogen. Despite lacking a hydroxyl group on C-2, it still contains many conjugated double bonds, suggesting its potential ability to resist ROS based on previous research. However, due to the absence of a hydroxyl group on C-2, its antioxidant capacity is theoretically weaker than that of deinoxanthin. Yang et al. found that 2-deoxydeinoxanthin could effectively scavenge radicals, including superoxide anion (O2−) and hydroxyl radical (·OH), thereby aiding in maintaining cell survival and recovery when exposed to radiation damage (Yang, 2021). Comparing the antioxidant and oxygen-free radical scavenging abilities of deinoxanthin and 2-deoxydeinoxanthin revealed that although the antioxidant capacity was weaker than that of deinoxanthin, 2-deoxydeinoxanthin still exhibited similar radiation protection effects (Yang, 2021). It plays a key role in D. radiodurans’ antioxidant defense system, particularly against oxidative stress caused by radiation exposure. These findings further emphasize the crucial role of carotenoids in D. radiodurans, especially in its extraordinary mechanism of radiation resistance.
3.2 Lycopene
3.2.1 Physicochemical properties of lycopene
Lycopene belongs to the carotenoid family of compounds that are found in fruits, vegetables, and green plants. Like other carotenoids, lycopene is a natural pigment synthesized by plants and microorganisms that absorbs light during photosynthesis and protects them from photosensitization (Roldán-Gutiérrez and Castro, 2007). It is a non-cyclic carotenoid with a molecular formula of C40H56 and a molecular weight of 536.85 Da (Surmanidze et al., 2024). Being lipophilic, it appears as a red pigment that absorbs light in the visible range. The λmax of lycopene in petroleum ether solution is 472 nm with ε% of 3,450 (Rodríguez-Rodríguez et al., 2020). It consists of an 8-isoprene unit chain containing 40 carbons with 11 conjugated double bonds and two non-conjugated double bonds (Figure 1) (Wang, 2012). Similar to other carotenoids, their double bonds can be converted from trans to single or multiple cis isomers through exposure to light, thermal energy, or chemical reactions. However, due to the lack of β-ionone ring structure in the lycopene molecule, it does not have provitamin A activity (Ashraf et al., 2022).
3.2.2 Lycopene and human health
Due to the special structure of lycopene, this pigment has strong antioxidant properties and anticancer activity, making it widely used in the food and medical industries (Khan et al., 2021). Studies have provided an overview of the mechanism of action of lycopene (Long et al., 2024). The main biological function of lycopene is its antioxidant properties. Research shows that consuming lycopene from the diet can increase its levels in circulation and tissues (Przybylska, 2020). As an antioxidant, it scavenges ROS and reduces oxidative stress and damage to cellular components including lipids, proteins, RNA, and DNA, therefore diminishing the risk of certain diseases such as cardiovascular disease, cancer, and osteoporosis by acting as a potent antioxidant (Caseiro et al., 2020; Kulawik et al., 2023). Numerous studies have demonstrated the association between lycopene intake and prevention/treatment of cancer. For example, daily consumption of lycopene may lower the risk of gut-related cancers as well as prostate cancer (Kapala et al., 2022). In addition, similar to deinoxanthin, lycopene exhibits a certain effect on breast cancer by inhibiting the proliferation of human MCF-7 breast cancer cells (Santos et al., 2018). Apart from its role in cancer treatment, lycopene also confers benefits for cardiovascular disease (Przybylska and Tokarczyk, 2022). Overall, lycopene has shown great potential in maintaining human health.
4 Deinoxanthin biosynthesis
4.1 Synthetic pathway
Deinoxanthin is the main carotenoid product in the Deinococcus strains. Its synthesis begins with the general lycopene biosynthesis pathway, which involves multiple enzymes (Figure 3) (Du et al., 2023). Under the catalysis of various enzymes, the structure of lycopene becomes more complex, containing circular ends and multiple oxidative functional groups. First, under the catalysis of lycopene cyclase (CrtLm), the end of the lycopene molecule forms a β-ring to produce γ-carotene. Subsequently, through the catalysis of C-1′,2′-hydratase (CruF) and C-3′,4′-desaturase (CrtD), a double bond between C-1′ and C-2′ of γ-carotene is hydrated, and a hydroxyl group is added to C-1′, forming a double bond between C-3′ and C-4′. Additionally, carotene ketolase (CrtO) can introduce ketone groups at the C-4 position of γ-carotene and its derivatives. With these three enzymes acting together, γ-carotene is converted into 2-deoxydeinoxanthin, which serves as a precursor for deinoxanthin. Finally, 2-β-hydroxylase (DR2473) adds a hydroxyl group to the β-ring (C-2) of 2-deoxydeinoxanthin to generate deinoxanthin as its final product.
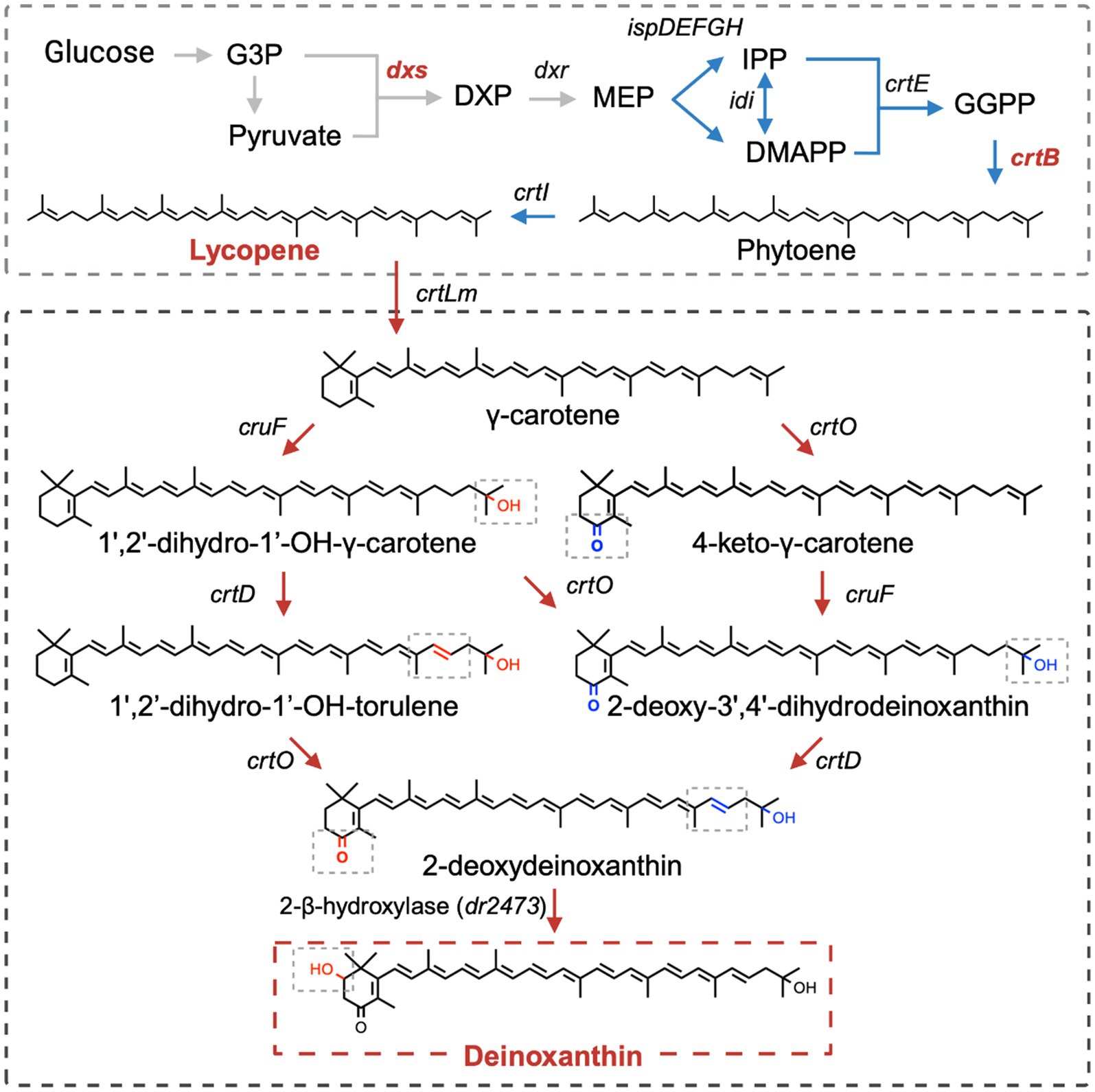
Figure 3. Deinoxanthin metabolic pathway (G3P: glyceraldehyde 3-phosphate; DXP: 1-deoxy-D-xylulose-5-phosphate; MEP: methylerythritol-4-phosphate; IPP: isopentenyl diphosphate; DMAPP: dimethylallyl diphosphate; GGPP: geranylgeranyl diphosphate; dxs: DXP synthase; dxr: DXP reductoisomerase; ispD: 4-(cytosine-5-phosphate)-2-C-methyl-D-erythritol (CDP-ME) synthase; ispE: CDP-ME kinase; ispF: 2-C-methyl-D-erythritol-2,4-diphosphate (MECP) synthase; ispG: 1-hydroxy-2-methyl-2-(E)-butenyl-4-diphosphate (HMBPP) synthase; ispH: HMBPP reductase; idi: isopentenyl diphosphate isomerase; crtE: GGPP synthase; crtB: phytoene synthase; crtI: phytoene desaturase; crtLm: lycopene cyclase; cruF: C-1′,2′-hydratase; crtD: C-3′,4′-desaturase; crtO: carotene ketolase). Created with BioRender.com.
4.2 Synthetic enzymes
4.2.1 Lycopene cyclase
The structure of some natural carotenoids is asymmetric and difficult to obtain by chemical synthesis (Su et al., 2023). However, there is a biological solution to solve this problem using enzymes. Deinococcus bacteria possess a type of lycopene cyclase (CrtLm), which is responsible for the cyclization of lycopene into various carotenoid products. CrtLm was identified in D. radiodurans by co-expressing crtLm with the crtEIB genes from Pantoea stewartii in E. coli. It is noteworthy that, unlike the CrtY-type lycopene β-cyclases that cyclize lycopene at both ends to produce β-lycopene, CrtLm selectively interacts with lycopene and asymmetrically cyclizes it into γ-carotene (Tao et al., 2004).
4.2.2 C-1′,2′-hydratase
The end modification of monocyclic carotenoids requires carotenoid C-1′,2′-hydratase (CruF) to achieve C-1′,2′ hydration. A CruF type carotenoid-1′,2′-hydratase has been identified from D. radiodurans and D. geothermalis, which shows little homology with the CrtC type carotenoid hydratase mainly found in photosynthetic bacteria (Frigaard et al., 2004; Sun et al., 2009b). Phylogenetic analysis reveals that CruF homologs are clustered separately in a clade and have a distant evolutionary relationship with the CrtC homologs. The cruF gene in Deinococcus may have been obtained from a common ancestor or through horizontal gene transfer between bacteria (Sun et al., 2009b). The hydration process catalyzed by CruF is a key step in the carotenoid biosynthesis pathway, and the mechanism by which CruF converts carotenoids such as γ-carotene into hydroxyl compounds involves a crucial interaction between water and carbon–carbon double bond (Sun et al., 2009b).
4.2.3 C-3′,4′-desaturase
The crtD gene in D. radiodurans (dr2250) is crucial for the desaturation process, specifically catalyzing the C-3′,4′-desaturation of the monocyclic precursor of deinoxanthin (Tian et al., 2008). This enzyme does not affect acyclic carotenoids. The C-3′,4′-desaturase (CrtD) from D. radiodurans specifically acts on carotenoids with a hydroxyl group at the C-1′ position. This specificity indicates that the enzyme preferentially modifies carotenoids that have already been modified by hydroxylation, rather than acting on initial carotenoid structure. Thus, the catalysis of CrtD during deinoxanthin synthesis always follows the reaction catalyzed by CruF. CrtD participates in the biosynthesis of deinoxanthin by catalyzing the desaturation at position C-3′,4′ on the carotenoid backbone. This action is critical in forming deinoxanthin, which contributes to the notable antioxidant properties and radiation resistance of D. radiodurans (Tian et al., 2008). Studies on similar enzymes in other bacteria have shown that CrtD-like enzymes could exhibit varied activities based on carotenoid structure. For instance, in Rubrivivax gelatinosus, a similar enzyme shows a preference for certain hydroxyspheroidene derivatives, highlighting the importance of specific structural features for enzyme activity (Steiger et al., 2000).
4.2.4 Carotene ketolase
Carotene ketolase (CrtO) from D. radiodurans is capable of adding a keto group to the C-4 position of the β-ring of γ-carotene or its derivatives, producing ketoleted carotenoids. CrtO is part of the phytoene desaturase (CrtI) family, which includes carotene desaturases and carotenoid oxidases (Sun et al., 2009a). In Synechocystis sp., CrtO primarily acts as a mono-ketolase but can also produce small amounts of diketo canthaxanthin. The enzyme utilizes an oxidized quinone as a co-factor, and the catalytic process begins with a hydride transfer to the quinone, followed by a stabilization reaction forming a hydroxy group. The keto group is formed through two subsequent hydroxylations at the same C-atom and subsequent water elimination (Breitenbach et al., 2013). The mutation in CrtO led to a significant decrease in the ability of D. radiodurans R1 to convert γ-carotene derivatives into ketolated carotenoids. Moreover, this mutation resulted in crtOΔR1 exhibiting greater sensitivity to oxidative stress induced by hydrogen peroxide (H₂O₂) treatment compared to the wild-type strain. This increased sensitivity is attributed to the absence of the C-4 keto group on the carotenoids, which plays a critical role in their antioxidant activity. Additionally, carotenoid extracts from the mutant demonstrated lower radical scavenging activity, underscoring the importance of the C-4 keto group in the antioxidant defense mechanisms of D. radiodurans (Sun et al., 2009a). When expressed in E. coli, CrtO from D. radiodurans demonstrates its ability to convert accumulated β-carotene into ketocarotenoids, such as canthaxanthin. This indicates that functional expression of CrtO can be achieved in a heterologous host, potentially facilitating the production of valuable ketocarotenoids using a more controllable and scalable system (Tao and Cheng, 2004).
4.2.5 2-β-hydroxylase
The 2-β-hydroxylase (DR2473) in D. radiodurans plays a crucial role in the synthesis of deinoxanthin, which is the final step in its biosynthesis pathway. 2-β-hydroxylase belongs to the class of cytochrome P450 monooxygenases that use electrons from NAD(P)H to activate molecular oxygen and perform specific oxidative attacks on structurally inactive carbon-hydrogen bonds of various chemicals (Werck-Reichhart and Feyereisen, 2000). In D. radiodurans, 2-β-hydroxylase adds a hydroxyl group to the β-ring (C-2) of 2-deoxydeinoxanthin to generate the final product of deinoxanthin (Zhou et al., 2015). Mutation of the gene dr2473 encoding cytochrome P450 CYP287A1 leads to an accumulation of 2-deoxydeinoxanthin in D. radiodurans, suggesting that 2-β-hydroxylase can further convert it into other products. In addition, the Δdr2473 mutant exhibits increased sensitivity to UV radiation and oxidative stress compared to wild-type strain D. radiodurans R1, indicating that the hydroxyl group at the C-2 position is important for antioxidant activity (Zhou et al., 2015).
5 Pathway modification and heterologous expression
Metabolic engineering, an important technique in microbial bioengineering, has been widely used to enhance the production of specific metabolites, optimize biosynthetic pathways, and introduce new metabolic functions. Deinococcus has recently emerged as a promising target for microbial metabolic engineering due to its adaptability to extreme environments (Figure 4) (Tian et al., 2009). The production capacity of carotenoids in this genus is not only related to its remarkable radiation resistance but also attracts attention for potential applications in the pharmaceutical, food, and cosmetic industries (Cheng et al., 2014; Sadowska-Bartosz and Bartosz, 2023). However, the limited number of carotenoids naturally produced by these bacteria limits their industrial potential. Homologous expression of carotenoid products using Deinococcus as a chassis and heterologous expression of key exogenous genes in Deinococcus are widely utilized to enhance carotenoid production. Therefore, pathway modification (homologous expression) and heterologous expression based on metabolic engineering play crucial roles in improving carotenoid production.
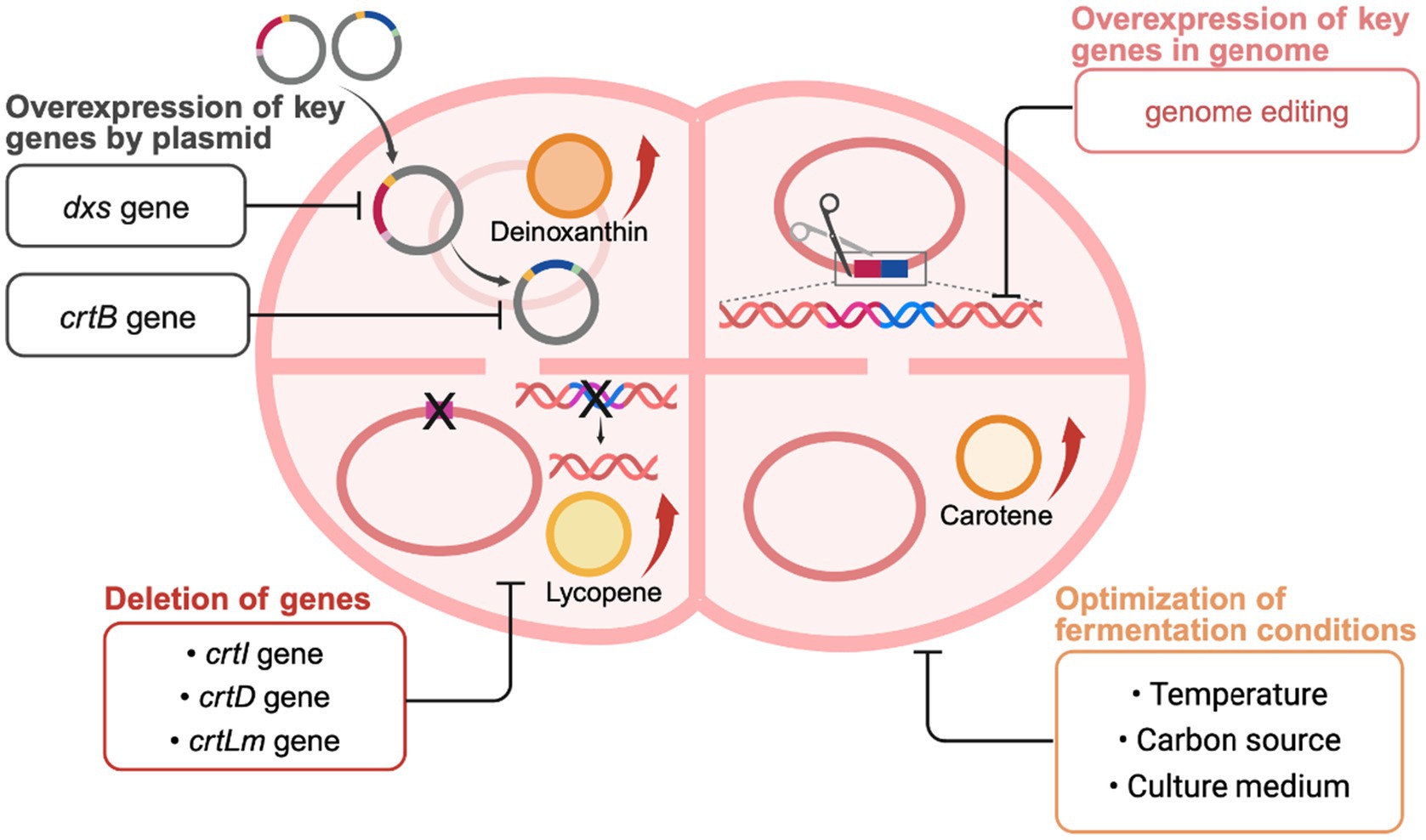
Figure 4. Metabolic engineering strategies for carotenoid biosynthesis in Deinococcus. Created with BioRender.com.
5.1 Metabolic engineering of pigment synthesis in Deinococcus
5.1.1 Overexpression of key genes
The overexpression of key genes is a direct way to increase the production of target metabolites. In Deinococcus bacteria, the activities of key enzymes in the carotenoid biosynthesis pathway, such as phytoene synthase (CrtB), directly affect the accumulation of end products. By increasing the expression of these enzymes through genetic engineering, it is possible to significantly increase the intracellular concentration of carotenoids. In carotenoid-producing strain, both the dxs gene encoding 1-deoxy-D-xylulose-5-phosphate synthase and crtB gene encoding phytoene synthase are considered rate-limiting steps in their respective carotenoid biosynthesis pathway (Rosas-Saavedra and Stange, 2016). However, genetic manipulation of the Deinococcus genus is difficult due to limitations in studying this genus, such as repetitive cloning steps and time-consuming processes. Additionally, the lack of tools for rescuing antibiotic resistance genes makes it impossible to create multiple knockout strains (Jeong et al., 2017). The application of Cre-lox-based rapid and efficient multiple knockout system in D. radiodurans R1 provides a new solution to this problem (Jeong et al., 2017). Based on this approach, the metabolic flux of deinoxanthin was enhanced by inserting crtB and dxs genes into the genome of D. radiodurans R1 (Jeong et al., 2021). The overexpression of crtB and dxs genes did not cause a burden on bacterial growth, and the deinoxanthin production of the engineered strain was 177.29 ± 8.4 mg/L, which was 200% higher than that of the wild-type strain. By comparing and analyzing mRNA expression levels for both genes, the optimal temperature was determined, and the carbon source was further optimized to significantly improve deinoxanthin production. The deinoxanthin production of the engineered strain was (394 ± 17.6) mg/L (102 ± 11.1 mg/g DCW) (Jeong et al., 2021). These studies on the overexpression of key genes provide a basis for the modification of metabolic pathways in Deinococcus bacteria. The overexpression of both crtB and dxs genes is necessary for subsequent studies on how to produce high amounts of carotenoids.
5.1.2 Deletion of genes
The shunting of non-target carotenoids was reduced by knocking down specific Crt-like genes, thereby increasing the production of target carotenoids. For example, deleting the gene crtI that encodes phytoene desaturase cuts off the pathway for converting phytoene to downstream carotenoids, allowing for the accumulation of phytoene. The engineered strain using 10 g/L of fructose as a carbon source achieved a phytoene yield of 0.413 ± 0.023 mg/L (Jeong et al., 2018). Deleting the carotenoid 3′,4′-desaturase gene crtD and overexpressing crtB and dxs genes increased phytoene production in the engineered strain by 1,080% (4.46 ± 0.19 mg/L) (Jeong et al., 2017). Moreover, the crtLm gene encoding lycopene cyclase in D. radiodurans R1 was deleted to prevent the synthesis of γ-carotene, so that bacteria unable to produce lycopene could now produce it instead. Lycopene production was increased to 373.5 mg/L by constructing plasmids overexpressing crtB and dxs genes (Kang et al., 2020). Given the complexity of carotenoid metabolic pathways, one effective method for metabolic engineering to produce specific carotenoids is redirecting metabolic flow toward a specific pathway and deleting bypass genes.
5.1.3 Optimization of fermentation conditions
The optimization of fermentation conditions is also a key factor in improving product yield. By finding the optimal growth conditions for microorganisms or providing abundant nutrients, the cell concentration can be increased to enhance the production of target products. To improve deinoxanthin production, cultivation conditions, especially temperature and carbon sources, have been optimized. The groR promoter in D. radiodurans has shown varying gene expression abilities at different temperatures (Schmid and Lidstrom, 2002). Therefore, regulation of crtB and dxs genes with the groR promoter was used to improve deinoxanthin production by optimizing fermentation temperature. At the optimal temperature (37°C), mRNA expression levels of crtB and dxs in the engineered strain were increased by 18 and 13 times, respectively, resulting in a deinoxanthin production of 256.5 ± 13.8 mg/L, which was 290% higher than that of the wild-type strain (Jeong et al., 2021). Additionally, replacing glucose with sucrose as the carbon source further enhanced deinoxanthin production in the engineered strain to reach 394 ± 17.6 mg/L, which was 446% higher than that of the wild-type strain (Jeong et al., 2021). Similarly, in D. sp. AJ005, the total carotenoid yield was increased by optimizing medium composition and sucrose concentrations. Specifically, a TGY medium containing 40 g/L of sucrose resulted in a total carotenoid yield that was 650% higher than that obtained without sucrose (Choi et al., 2019). As discussed in this review, the external conditions required for simulating extremophile habitats are an important consideration for their industrial production.
5.2 Heterologous expression of pigment synthesis genes in Deinococcus
In recent years, the use of genetic engineering techniques to exogenously express specific genes in model microorganisms for the production of target compounds has become a research hotspot in synthetic biology. As one of the model microorganisms, E. coli has been widely used in various biosynthetic studies due to its clear genetic background, easy genetic manipulation, and rapid growth (Adamczyk and Reed, 2017). To achieve efficient carotenoid production, the carotenoid synthesis genes from Deinococcus bacteria are introduced into E. coli and other model bacteria (Jin et al., 2015; Xu X. et al., 2018). Through molecular biological methods and bioengineering techniques, we can optimize the biosynthetic pathway of carotenoids and improve their expression and activity in host cells (Mori et al., 2022).
Deinococcus wulumuqiensis R12 has a strong antioxidant capacity and can produce deinoxanthin to improve radiation resistance (Wang et al., 2010; Xu et al., 2013). Three genes encoding carotenoid synthetase (crtE, crtB, and crtI) were identified from D. wulumuqiensis R12 to construct a polycistronic plasmid harboring these putative lycopene biosynthesis genes (crtEBI) for the production of lycopene. These genes were co-expressed in E. coli using the optimized Shine–Dalgarno (SD) region to increase lycopene yield and expressed in E. coli with different arrangements to study the effect of gene order. After optimizing the culture conditions, a high yield of lycopene was obtained, with the highest yield being 688 mg/L in the gene order crtI-E-B (Jin et al., 2015; Xu X. et al., 2018). However, an early study found that the gene order crtEBI (6.2 mg/g DCW) had the highest yield of lycopene, while crtI-E-B had the lowest yield (0.17 mg/g DCW) (Zhang et al., 2015). The reason for this opposite result may be attributed to differences in gene origin, as crtI-E-B had its highest yield from D. wulumuqiensis R12, while another study used three genes from three bacteria belonging to the genus Pantoea, respectively. In order to obtain E. coli with high lycopene production, experiments were conducted to find suitable plasmids for lycopene production and E. coli host strains (Xu J. et al., 2018). The lycopene synthesis genes crtE, crtB, and crtI from R12 were integrated into three different plasmids and then transferred into three different E. coli hosts, resulting in nine engineered strains. After the optimization of conditions, it was found that the selection of host and plasmid greatly impacted lycopene production, where medium copy number plasmids were more suitable than those with high or low copy numbers (Xu J. et al., 2018).
The production of deinoxanthin still needs to be industrialized at present, and research on heterologous expression of the deinoxanthin synthesis pathway has not been reported. Heterologous expression and metabolic engineering of deinoxanthin derived from Deinococcus will provide more experimental support for the further industrial production of deinoxanthin in more robust chassis cells.
6 Enhancement of bacterial resistance to environmental stress
Pigments play an important role in the survival and adaptation of bacteria, especially in response to external environmental stresses (Rao et al., 2017; Moors et al., 2021). In nature, many bacteria can produce different pigments, among which carotenoids are a class of pigment molecules that are ubiquitous and have diverse functions. Carotenoids not only give bacteria specific colors but also play key roles in antioxidant activity, photoprotection, and immune regulation (Chew and Park, 2004; Sipka and Maroti, 2018). By inhibiting ROS production and scavenging free radicals, carotenoids protect bacterial cells from damage caused by environmental stresses such as ultraviolet rays, oxidants, and high salt concentrations (Jones and Baxter, 2017). In recent years, with the progress of metabolic engineering technology, genetically modified strains capable of synthesizing carotenoids have been developed to significantly improve their tolerance to environmental stress (Albrecht et al., 2000). These engineered strains not only provide valuable models for basic research but also show broad prospects in industrial production and bioremediation applications (Albrecht et al., 2000). For example, introducing the carotenoid synthesis pathway into engineered bacteria exhibits enhanced viability and adaptability under conditions of high salt concentration, high temperature, and in the presence of oxidants. In addition, these modification techniques can be used to enhance the performance of industrial strains while improving the yield and stability of biological products.
Carotenoids in Deinococcus bacteria serve as non-enzymatic antioxidants, enabling them to resist oxidative toxicity in the external environment. D. radiodurans exhibited five orders of magnitude higher resistance to 10 mM H2O2 compared to E. coli, which does not produce deinoxanthin (Wang and Schellhorn, 1995). When D. radiodurans and its colorless mutant were exposed to different concentrations of H2O2, it was found that the wild-type strain showed no significant change in bacterial survival after treatment with various concentrations of H2O2 (0.83, 1.67, 4, and 5 mM), while the colorless mutant displayed a dose-dependent increase in sensitivity toward H2O2. At a concentration of 5 mM, it was found to be 100-fold more sensitive than the wild-type strain (Qi et al., 2020). In addition, genetic engineering techniques were used to knock out crtB and crtI genes involved in the deinoxanthin pathway of D. radiodurans R1, resulting in two colorless mutants that exhibited increased sensitivity to H2O2, ionizing radiation and UV (Zhang et al., 2007; Yang, 2021).
Further studies have found that the functional groups of deinoxanthin directly affect the antioxidant capacity of bacteria. When the gene for the last enzyme in the synthetic pathway (2-β-hydroxylase) is knocked out, the product is 2-deoxydeinoxanthin, with a hydroxyl group at C-2 of deinoxanthin. The LD90 of the mutant R1Δd2473 decreased from 71.2 mM/30 min in the wild type to 54.6 mM/30 min, indicating that deinoxanthin has a higher antioxidant capacity than 2-deoxydeinoxanthin (Zhou et al., 2015). The survival rate of the mutant R1Δd2473 was reduced by 90% at a UV irradiation dose of 1,000 J·m−2 compared to the wild type. The presence of a keto group at C-4 position in γ-carotene derivatives, formed by γ-carotene ketolase (CrtO), is crucial for this antioxidant activity (Sun et al., 2009a). Mutants lacking this keto group show increased sensitivity to oxidative stress and lower radical-scavenging activity, indicating the importance of structure of deinoxanthin in its antioxidant function as well. The product of R1ΔcrtD mutant is 3′,4′-dihydrodeinoxanthin, which lacks a double bond between C-3′ and C-4′. The loss of this functional group significantly weakens the antioxidant capacity of 3′,4′-dihydrodeinoxanthin compared to deinoxanthin (p < 0.05) (Tian et al., 2008). Nevertheless, its antioxidant capacity is still higher than that of astaxanthin (Jeong et al., 2021).
7 Conclusion and prospects
This article reviews the synthesis and engineering of Deinococcus pigments, especially carotenoids, as well as their role in coping with environmental stress. First, we introduce the unique carotenoid synthesis pathway of Deinococcus species, including the functions and mechanisms of key enzymes. Next, strategies for improving carotenoid production through metabolic engineering are discussed, such as overexpression of key genes, gene knockout, and optimization of fermentation conditions. Finally, we explore the key role of these carotenoids in bacterial resistance to oxidative stress and use genetic modification and heterologous expression techniques to enable other bacteria to synthesize these carotenoids and enhance their stress resistance. In conclusion, carotenoids in Deinococcus not only play important roles in their survival and environmental adaptability but also have potential industrial applications. An in-depth study of these bacterial pigments will not only reveal their unique biological mechanisms but also provide new ideas and methods for biotechnology and industrial applications. Future research in pigment production by Deinococcus bacteria can be carried out from the following aspects:
1. Gene regulation and metabolic pathway optimization. Future studies should further reveal the gene regulation mechanism of carotenoid synthesis in Deinococcus species and optimize metabolic pathways to improve pigment production. For example, gene editing technologies such as CRISPR/Cas9 can be used to precisely regulate the expression of key enzyme genes and increase the amount of carotenoid accumulation. Additionally, metabolic flux analysis can be used to identify and eliminate metabolic bottlenecks for further improvement in pigment production.
2. Synthetic biology and heterologous expression. The development of synthetic biology provides a new idea for the heterologous synthesis of carotenoids. The carotenoid synthesis gene from Deinococcus is cloned into industrial microorganisms such as E. coli, and efficient production is achieved by optimizing the expression vector and fermentation conditions. In addition, new pigments and derivatives are developed using modular design and combinatorial biosynthetic pathways.
3. Application in space exploration. Carotenoids from Deinococcus bacteria are highly radioresistant, and previous studies have shown their excellent performance in space exploration, which holds significant potential for practical applications (Ott et al., 2019, 2020). For example, these pigments could serve as radiation indicators to monitor real-time levels of radiation exposure for astronauts and equipment. In addition, these pigments can also act as natural antioxidants to protect the health of astronauts during long-term spaceflight.
4. Biotechnology and pharmaceutical applications. Carotenoids from Deinococcus bacteria have broad prospects for applications in food, pharmaceutical, and cosmetic fields. For example, the antioxidant and anticancer properties of deinoxanthin can be exploited to develop new functional foods and nutritional supplements. At the same time, these pigments can also be used as natural substitutes for synthetic pigments to meet the market demand for natural products.
Author contributions
YW: Conceptualization, Funding acquisition, Methodology, Validation, Writing – original draft. JL: Conceptualization, Methodology, Validation, Writing – original draft. YY: Methodology, Writing – original draft. LZ: Formal analysis, Investigation, Writing – original draft. ML: Formal analysis, Investigation, Writing – review & editing. ZZ: Funding acquisition, Writing – review & editing. QX: Validation, Writing – review & editing. LJ: Funding acquisition, Writing – review & editing.
Funding
The author(s) declare that financial support was received for the research, authorship, and/or publication of this article. This study was supported by the National Natural Science Foundation of China (2021YFC2102700 and U2106228), the Jiangsu Synergetic Innovation Center for Advanced Bio-Manufacture (XTC2205), the Natural Science Foundation of the Jiangsu Higher Education Institutions of China (23KJB550007), the Project funded by China Postdoctoral Science Foundation (2023M741669), the Jiangsu Funding Program for Excellent Postdoctoral Talent (2023ZB025), the Natural Science Foundation of Xinjiang Uygur Autonomous Region (2022D01E19), and the Agricultural Science and Technology Innovation Platform Capacity Improvement Project of Xinjiang Academy of Agricultural Sciences (Pt005).
Conflict of interest
The authors declare that the research was conducted in the absence of any commercial or financial relationships that could be construed as a potential conflict of interest.
Publisher’s note
All claims expressed in this article are solely those of the authors and do not necessarily represent those of their affiliated organizations, or those of the publisher, the editors and the reviewers. Any product that may be evaluated in this article, or claim that may be made by its manufacturer, is not guaranteed or endorsed by the publisher.
References
Adamczyk, P. A., and Reed, J. L. (2017). Escherichia coli as a model organism for systems metabolic engineering. Curr. Opin. Syst. Biol. 6, 80–88. doi: 10.1016/j.coisb.2017.11.001
Albrecht, M., Takaichi, S., Steiger, S., Wang, Z., and Sandmann, G. (2000). Novel hydroxycarotenoids with improved antioxidative properties produced by gene combination in Escherichia coli. Nat. Biotechnol. 18, 843–846. doi: 10.1038/78443
Ashraf, W., Latif, A., Zhang, T., Zhang, J., Wang, C., Rehman, A., et al. (2022). Technological advancement in the processing of lycopene: a review. Food Rev. Int. 38, 857–883. doi: 10.1080/87559129.2020.1749653
Basu, B. (2022). The radiophiles of Deinococcaceae family: resourceful microbes for innovative biotechnological applications. Curr. Res. Microb. Sci. 3:100153. doi: 10.1016/j.crmicr.2022.100153
Breitenbach, J., Gerjets, T., and Sandmann, G. (2013). Catalytic properties and reaction mechanism of the CrtO carotenoid ketolase from the cyanobacterium Synechocystis sp. PCC 6803. Arch. Biochem. Biophys. 529, 86–91. doi: 10.1016/j.abb.2012.11.003
Britton, G. (1995). Structure and properties of carotenoids in relation to function. FASEB J. 9, 1551–1558. doi: 10.1096/fasebj.9.15.8529834
Caseiro, M., Ascenso, A., Costa, A., Creagh-Flynn, J., Johnson, M., and Simoes, S. (2020). Lycopene in human health. LWT 127:109323. doi: 10.1016/j.lwt.2020.109323
Celedón, R. S., and Díaz, L. B. (2021). Natural pigments of bacterial origin and their possible biomedical applications. Microorganisms 9:739. doi: 10.3390/microorganisms9040739
Chen, A., Hernandez-Vargas, J., Han, R., Cortazar-Martinez, O., Gonzalez, N., Patel, S., et al. (2021). Small RNAs as a new platform for tuning the biosynthesis of silver nanoparticles for enhanced material and functional properties. ACS Appl. Mater. Interfaces 13, 36769–36783. doi: 10.1021/acsami.1c07400
Cheng, J., Zhang, Z., Zheng, Z., Lv, G., Wang, L., Tian, B., et al. (2014). Antioxidative and hepatoprotective activities of deinoxanthin-rich extract from Deinococcus radiodurans R1 against carbon tetrachloride-induced liver injury in mice. Trop. J. Pharm. Res. 13, 581–586. doi: 10.4314/tjpr.v13i4.14
Chew, B. P., and Park, J. S. (2004). Carotenoid action on the immune response. J. Nutr. 134, 257S–261S. doi: 10.1093/jn/134.1.257S
Choi, Y. J., Hur, J. M., Lim, S., Jo, M., Kim, D. H., and Choi, J. I. (2014). Induction of apoptosis by deinoxanthin in human cancer cells. Anticancer Res. 34, 1829–1835 Available at: https://ar.iiarjournals.org/content/34/4/1829.
Choi, J. Y., Lee, K., and Lee, P. C. (2019). Characterization of carotenoid biosynthesis in newly isolated Deinococcus sp. AJ005 and investigation of the effects of environmental conditions on cell growth and carotenoid biosynthesis. Mar. Drugs 17:705. doi: 10.3390/md17120705
Daly, M. J. (2012). Death by protein damage in irradiated cells. DNA Repair 11, 12–21. doi: 10.1016/j.dnarep.2011.10.024
Du, B., Sun, M., Hui, W., Xie, C., and Xu, X. (2023). Recent advances on key enzymes of microbial origin in the lycopene biosynthesis pathway. J. Agric. Food Chem. 71, 12927–12942. doi: 10.1021/acs.jafc.3c03942
Esteban, R., Moran, J. F., Becerril, J. M., and García-Plazaola, J. I. (2015). Versatility of carotenoids: an integrated view on diversity, evolution, functional roles and environmental interactions. Environ. Exp. Bot. 119, 63–75. doi: 10.1016/j.envexpbot.2015.04.009
Farci, D., Haniewicz, P., Sanctis, D., Lesu, L., Kereiche, S., Winterhalter, M., et al. (2022). The cryo-EM structure of the S-layer deinoxanthin-binding complex of Deinococcus radiodurans informs properties of its environmental interactions. J. Biol. Chem. 298:102031. doi: 10.1016/j.jbc.2022.102031
Flieger, J., Raszewska-Famielec, M., Radzikowska-Büchner, E., and Flieger, W. (2024). Skin protection by carotenoid pigments. Int. J. Mol. Sci. 25:1431. doi: 10.3390/ijms25031431
Frigaard, N. U., Maresca, J. A., Yunker, C. E., Jones, A. D., and Bryant, D. A. (2004). Genetic manipulation of carotenoid biosynthesis in the green sulfur bacterium Chlorobium tepidum. J. Bacteriol. 186, 5210–5220. doi: 10.1128/jb.186.16.5210-5220.2004
Ghosh, S., Sarkar, T., Chakraborty, R., Shariati, M. A., and Simal-Gandara, J. (2024). Nature’s palette: an emerging frontier for coloring dairy products. Crit. Rev. Food Sci. Nutr. 64, 1508–1552. doi: 10.1080/10408398.2022.2117785
Giani, M., Pire, C., and Martinez-Espinosa, R. (2024). Bacterioruberin: biosynthesis, antioxidant activity, and therapeutic applications in cancer and immune pathologies. Mar. Drugs 22:167. doi: 10.3390/md22040167
Griffiths, E., and Gupta, R. S. (2004). Distinctive protein signatures provide molecular markers and evidence for the monophyletic nature of the Deinococcus-Thermus phylum. J. Bacteriol. 186, 3097–3107. doi: 10.1128/jb.186.10.3097-3107.2004
Günay, N., and Kuzucu, M. (2023). Agonistic effects of deinoxanthin on tamoxifen antiproliferative activity on HER2 positive breast cancer: an in vitro study on MDA-MB-453. Erzincan Univ. J. Sci. Technol. 16, 138–154. doi: 10.18185/erzifbed.1224499
Han, R., Fang, J., Jiang, J., Gaidamakova, E. K., Tkava, R., Daly, M. J., et al. (2020). Signal recognition particle RNA contributes to oxidative stress response in Deinococcus radiodurans by modulating catalase localization. Front. Microbiol. 11:613571. doi: 10.3389/fmicb.2020.613571
Han, R., Jiang, J., Fang, J., and Contreras, L. M. (2022). PNPase and RhlB interact and reduce the cellular availability of oxidized RNA in Deinococcus radiodurans. Microbiol. Spectr. 10, e02140–e02122. doi: 10.1128/spectrum.02140-22
Jeong, S. W., Kang, C. K., and Choi, Y. J. (2018). Metabolic engineering of Deinococcus radiodurans for the production of phytoene. J. Microbiol. Biotechnol. 28, 1691–1699. doi: 10.4014/jmb.1808.08019
Jeong, S. W., Kim, J. H., Kim, J. W., Kim, C. Y., Kim, S. Y., and Choi, Y. J. (2021). Metabolic engineering of extremophilic bacterium Deinococcus radiodurans for the production of the novel carotenoid deinoxanthin. Microorganisms 9:44. doi: 10.3390/microorganisms9010044
Jeong, S. W., Yang, J. E., Im, S., and Choi, Y. J. (2017). Development of Cre-lox based multiple knockout system in Deinococcus radiodurans R1. Korean J. Chem. Eng. 34, 1728–1733. doi: 10.1007/s11814-017-0082-5
Jin, W., Xu, X., Jiang, L., Zhang, Z., Li, C., and Huang, H. (2015). Putative carotenoid genes expressed under the regulation of Shine-Dalgarno regions in Escherichia coli for efficient lycopene production. Biotechnol. Lett. 37, 2303–2310. doi: 10.1007/s10529-015-1922-1
Jones, D. L., and Baxter, B. K. (2017). DNA repair and photoprotection: mechanisms of overcoming environmental ultraviolet radiation exposure in halophilic archaea. Front. Microbiol. 8:1882. doi: 10.3389/fmicb.2017.01882
Kang, C. K., Jeong, S. W., Yang, J. E., and Choi, Y. J. (2020). High-yield production of lycopene from corn steep liquor and glycerol using the metabolically engineered Deinococcus radiodurans R1 strain. J. Agric. Food Chem. 68, 5147–5153. doi: 10.1021/acs.jafc.0c01024
Kapala, A., Szlendak, M., and Motacka, E. (2022). The anti-cancer activity of lycopene: a systematic review of human and animal studies. Nutrients 14:5152. doi: 10.3390/nu14235152
Khan, U. M., Sevindik, M., Zarrabi, A., Nami, M., Ozdemir, B., Kaplan, D. N., et al. (2021). Lycopene: food sources, biological activities, and human health benefits. Oxidative Med. Cell. Longev. 2021:2713511. doi: 10.1155/2021/2713511
Kim, W., Kim, M., Hong, M., and Park, W. (2021). Killing effect of deinoxanthins on cyanobloom-forming Microcystis aeruginosa: eco-friendly production and specific activity of deinoxanthins. Environ. Res. 200:111455. doi: 10.1016/j.envres.2021.111455
Kulawik, A., Cielecka-Piontek, J., and Zalewski, P. (2023). The importance of antioxidant activity for the health-promoting effect of lycopene. Nutrients 15:3821. doi: 10.3390/nu15173821
Liang, M., Zhu, J., and Jiang, J. (2018). Carotenoids biosynthesis and cleavage related genes from bacteria to plants. Crit. Rev. Food Sci. Nutr. 58, 2314–2333. doi: 10.1080/10408398.2017.1322552
Lim, S., Jung, J. H., Blanchard, L., and Groot, A. D. (2019). Conservation and diversity of radiation and oxidative stress resistance mechanisms in Deinococcus species. FEMS Microbiol. Rev. 43, 19–52. doi: 10.1093/femsre/fuy037
Long, Y., Paengkoum, S., Lu, S., Niu, X., Thongpea, S., Taethaisong, N., et al. (2024). Physicochemical properties, mechanism of action of lycopene and its application in poultry and ruminant production. Front. Vet. Sci. 11:1364589. doi: 10.3389/fvets.2024.1364589
Luan, H., Meng, N., Fu, J., Chen, X., Xu, X., Feng, Q., et al. (2014). Genome-wide transcriptome and antioxidant analyses on gamma-irradiated phases of Deinococcus radiodurans R1. PLoS One 9:e85649. doi: 10.1371/journal.pone.0085649
Maoka, T. (2020). Carotenoids as natural functional pigments. J. Nat. Med. 74, 1–16. doi: 10.1007/s11418-019-01364-x
Moors, K. A., Ott, E., Weckwerth, W., and Milojevic, T. (2021). Proteomic response of Deinococcus radiodurans to short-term real microgravity during parabolic flight reveals altered abundance of proteins involved in stress response and cell envelope functions. Life 12:23. doi: 10.3390/life12010023
Mori, H., Kataoka, M., and Yang, X. (2022). Past, present, and future of genome modification in Escherichia coli. Microorganisms 10:1835. doi: 10.3390/microorganisms10091835
Nayak, T., Sengupta, I., and Dhal, P. K. (2021). A new era of radiation resistance bacteria in bioremediation and production of bioactive compounds with therapeutic potential and other aspects: an in-perspective review. J. Environ. Radioact. 237:106696. doi: 10.1016/j.jenvrad.2021.106696
Noby, N., Khattab, S. N., and Soliman, N. A. (2023). Sustainable production of bacterioruberin carotenoid and its derivatives from Arthrobacter agilis NP20 on whey-based medium: optimization and product characterization. Bioresour. Bioprocess. 10:46. doi: 10.1186/s40643-023-00662-3
Orlandi, V. T., Martegani, E., Giaroni, C., Baj, A., and Bolognese, F. (2022). Bacterial pigments: a colorful palette reservoir for biotechnological applications. Biotechnol. Appl. Biochem. 69, 981–1001. doi: 10.1002/bab.2170
Ott, E., Fuchs, F. M., Moeller, R., Hemmersbach, R., Kawaguchi, Y., Yamagishi, A., et al. (2019). Molecular response of Deinococcus radiodurans to simulated microgravity explored by proteometabolomic approach. Sci. Rep. 9:18462. doi: 10.1038/s41598-019-54742-6
Ott, E., Kawaguchi, Y., Kolbl, D., Rabbow, E., Rettberg, P., Mora, M., et al. (2020). Molecular repertoire of Deinococcus radiodurans after 1 year of exposure outside the international Space Station within the Tanpopo mission. Microbiome 8:150. doi: 10.1186/s40168-020-00927-5
Palanisamy, M., and Ramalingam, S. (2024). Microbial bacterioruberin: A comprehensive review. Indian J. Microbiol., 1–25. In Press. doi: 10.1007/s12088-024-01312-8
Park, S. A., Ahn, S. Y., and Choi, K. Y. (2020). Functional microbial pigments isolated from Chryseobacterium and Deinococcus species for bio-paint application. Biotechnol. Bioprocess Eng. 25, 394–402. doi: 10.1007/s12257-019-0372-3
Poli, A., Finore, I., Romano, I., Gioiello, A., Lama, L., and Nicolaus, B. (2017). Microbial diversity in extreme marine habitats and their biomolecules. Microorganisms 5:25. doi: 10.3390/microorganisms5020025
Przybylska, S. (2020). Lycopene–a bioactive carotenoid offering multiple health benefits: a review. Int. J. Food Sci. Technol. 55, 11–32. doi: 10.1111/ijfs.14260
Przybylska, S., and Tokarczyk, G. (2022). Lycopene in the prevention of cardiovascular diseases. Int. J. Mol. Sci. 23:1957. doi: 10.3390/ijms23041957
Qi, H., Wang, W., He, J., Ma, Y., Xiao, F., and He, S. (2020). Antioxidative system of Deinococcus radiodurans. Res. Microbiol. 171, 45–54. doi: 10.1016/j.resmic.2019.11.002
Rajendran, P., Somasundaram, P., and Dufossé, L. (2023). Microbial pigments: eco-friendly extraction techniques and some industrial applications. J. Mol. Struct. 1290:135958. doi: 10.1016/j.molstruc.2023.135958
Rao, M. P. N., Xiao, M., and Li, W. J. (2017). Fungal and bacterial pigments: secondary metabolites with wide applications. Front. Microbiol. 8:1113. doi: 10.3389/fmicb.2017.01113
Rodríguez-Rodríguez, E., Beltrán-de-Miguel, B., Samaniego-Aguilar, K. X., Sánchez-Prieto, M., Estévez-Santiago, R., and Olmedilla-Alonso, B. (2020). Extraction and analysis by HPLC-DAD of carotenoids in human faeces from Spanish adults. Antioxidants 9:484. doi: 10.3390/antiox9060484
Roldán-Gutiérrez, J. M., and Castro, M. D. L. (2007). Lycopene: the need for better methods for characterization and determination. TrAC. Trends Anal. Chem. 26, 163–170. doi: 10.1016/j.trac.2006.11.013
Romanovskaya, V., Rokitko, P., Mikheev, A., Gushcha, N. I., Malashenko, Y. R., and Chernaya, N. A. (2002). The effect of γ-radiation and desiccation on the viability of the soil bacteria isolated from the alienated zone around the Chernobyl nuclear power plant. Microbiology 71, 608–613. doi: 10.1023/A:1020575223365
Rosas-Saavedra, C., and Stange, C. (2016). Biosynthesis of carotenoids in plants: enzymes and color. Subcell. Biochem. 79, 35–69. doi: 10.1007/978-3-319-39126-7_2
Sadowska-Bartosz, I., and Bartosz, G. (2023). Antioxidant defense of Deinococcus radiodurans: how does it contribute to extreme radiation resistance? Int. J. Radiat. Biol. 99, 1803–1829. doi: 10.1080/09553002.2023.2241895
Santos, R. C., Ombredane, A. S., Souza, J. M. T., Vasconcelos, A. G., Placido, A., Amorim, A. G. N., et al. (2018). Lycopene-rich extract from red guava (Psidium guajava L.) displays cytotoxic effect against human breast adenocarcinoma cell line MCF-7 via an apoptotic-like pathway. Food Res. Int. 105, 184–196. doi: 10.1016/j.foodres.2017.10.045
Schmid, A. K., and Lidstrom, M. E. (2002). Involvement of two putative alternative sigma factors in stress response of the radioresistant bacterium Deinococcus radiodurans. J. Bacteriol. 184, 6182–6189. doi: 10.1128/jb.184.22.6182-6189.2002
Sen, T., Barrow, C. J., and Deshmukh, S. K. (2019). Microbial pigments in the food industry-challenges and the way forward. Front. Nutr. 6:7. doi: 10.3389/fnut.2019.00007
Shu, W. S., and Huang, L. N. (2022). Microbial diversity in extreme environments. Nat. Rev. Microbiol. 20, 219–235. doi: 10.1038/s41579-021-00648-y
Sipka, G., and Maroti, P. (2018). Photoprotection in intact cells of photosynthetic bacteria: quenching of bacteriochlorophyll fluorescence by carotenoid triplets. Photosynth. Res. 136, 17–30. doi: 10.1007/s11120-017-0434-3
Steiger, S., Astier, C., and Sandmann, G. (2000). Substrate specificity of the expressed carotenoid 3,4-desaturase from Rubrivivax gelatinosus reveals the detailed reaction sequence to spheroidene and spirilloxanthin. Biochem. J. 349, 635–640. doi: 10.1042/bj3490635
Su, B., Deng, M. R., and Zhu, H. (2023). Advances in the discovery and engineering of gene targets for carotenoid biosynthesis in recombinant strains. Biomol. Ther. 13:1747. doi: 10.3390/biom13121747
Sun, Z., Shen, S., Tian, B., Wang, H., Xu, Z., Wang, L., et al. (2009a). Functional analysis of γ-carotene ketolase involved in the carotenoid biosynthesis of Deinococcus radiodurans. FEMS Microbiol. Lett. 301, 21–27. doi: 10.1111/j.1574-6968.2009.01794.x
Sun, Z., Shen, S., Wang, C., Wang, H., Hu, Y., Jiao, J., et al. (2009b). A novel carotenoid 1,2-hydratase (CruF) from two species of the non-photosynthetic bacterium Deinococcus. Microbiology 155, 2775–2783. doi: 10.1099/mic.0.027623-0
Sun, H., Xu, G., Zhan, H., Chen, H., Sun, Z., Tian, B., et al. (2010). Identification and evaluation of the role of the manganese efflux protein in Deinococcus radiodurans. BMC Microbiol. 10, 1–8. doi: 10.1186/1471-2180-10-319
Surmanidze, N., Vanidze, M., Djafaridze, I., Davitadze, R., Qarcivadze, I., Khakhutaishvili, M., et al. (2024). Optimization of the method of ultrasonic extraction of lycopene with a green extract from the fruit of Elaeagnus umbellata, common in Western Georgia. Food Sci. Nutr. 12, 3593–3601. doi: 10.1002/fsn3.4030
Tanaka, T., Shnimizu, M., and Moriwaki, H. (2012). Cancer chemoprevention by carotenoids. Molecules 17, 3202–3242. doi: 10.3390/molecules17033202
Tao, L., and Cheng, Q. (2004). Novel beta-carotene ketolases from non-photosynthetic bacteria for canthaxanthin synthesis. Mol. Gen. Genomics. 272, 530–537. doi: 10.1007/s00438-004-1083-8
Tao, L., Picataggio, S., Rouviere, P. E., and Cheng, Q. (2004). Asymmetrically acting lycopene beta-cyclases (CrtLm) from non-photosynthetic bacteri. Mol. Gen. Genomics. 271, 180–188. doi: 10.1007/s00438-003-0969-1
Tapia, C., Lopez, B., Astuya, A., Becerra, J., Gugliandolo, C., and Parra, B. (2021). Antiproliferative activity of carotenoid pigments produced by extremophile bacteria. Nat. Prod. Res. 35, 4638–4642. doi: 10.1080/14786419.2019.1698574
Tian, B., and Hua, Y. (2010). Carotenoid biosynthesis in extremophilic Deinococcus–Thermus bacteria. Trends Microbiol. 18, 512–520. doi: 10.1016/j.tim.2010.07.007
Tian, B., Li, J., Pang, R., Dai, S., Li, T., Weng, Y., et al. (2018). Gold nanoparticles biosynthesized and functionalized using a hydroxylated tetraterpenoid trigger gene expression changes and apoptosis in cancer cells. ACS Appl. Mater. Interfaces 10, 37353–37363. doi: 10.1021/acsami.8b09206
Tian, B., Sun, Z., Shen, S., Wang, H., Jiao, J., Wang, L., et al. (2009). Effects of carotenoids from Deinococcus radiodurans on protein oxidation. Lett. Appl. Microbiol. 49, 689–694. doi: 10.1111/j.1472-765X.2009.02727.x
Tian, B., Sun, Z., Xu, Z., Shen, S., Wang, H., and Hua, Y. (2008). Carotenoid 3′, 4′-desaturase is involved in carotenoid biosynthesis in the radioresistant bacterium Deinococcus radiodurans. Microbiology 154, 3697–3706. doi: 10.1099/mic.0.2008/021071-0
Tian, B., Wu, Y., Sheng, D., Zheng, Z., Gao, G., and Hua, Y. (2004). Chemiluminescence assay for reactive oxygen species scavenging activities and inhibition on oxidative damage of DNA in Deinococcus radiodurans. Luminescence 19, 78–84. doi: 10.1002/bio.761
Venil, C. K., Zakaria, Z. A., and Ahmad, W. A. (2013). Bacterial pigments and their applications. Process Biochem. 48, 1065–1079. doi: 10.1016/j.procbio.2013.06.006
Villa, J. K., Han, R., Tsai, C. H., Chen, A., Sweet, P., Franco, G., et al. (2021). A small RNA regulates pprM, a modulator of pleiotropic proteins promoting DNA repair, in Deinococcus radiodurans under ionizing radiation. Sci. Rep. 11:12949. doi: 10.1038/s41598-021-91335-8
Wang, X. (2012). Lycopene metabolism and its biological significance. Am. J. Clin. Nutr. 96, 1214S–1222S. doi: 10.3945/ajcn.111.032359
Wang, W., Mao, J., Zhang, Z., Tang, Q., Xie, Y., Zhu, J., et al. (2010). Deinococcus wulumuqiensis sp. nov., and Deinococcus xibeiensis sp. nov., isolated from radiation-polluted soil. Int. J. Syst. Evol. Microbiol. 60, 2006–2010. doi: 10.1099/ijs.0.015917-0
Wang, P., and Schellhorn, H. E. (1995). Induction of resistance to hydrogen peroxide and radiation in Deinococcus radiodurans. Can. J. Microbiol. 41, 170–176. doi: 10.1139/m95-023
Werck-Reichhart, D., and Feyereisen, R. (2000). Cytochromes P450: a success story. Genome Biol. 1:REVIEWS3003. doi: 10.1186/gb-2000-1-6-reviews3003
Wurtzel, E. T. (2019). Changing form and function through carotenoids and synthetic biology. Plant Physiol. 179, 830–843. doi: 10.1104/pp.18.01122
Xu, X., Jiang, L., Zhang, Z., Shi, Y., and Huang, H. (2013). Genome sequence of a gamma-and UV-ray-resistant strain, Deinococcus wulumuqiensis R12[J]. Genome Announc. 1:e00206. doi: 10.1128/genomea.00206-13
Xu, X., Tian, L., Xu, J., Xie, C., Jiang, L., and Huang, H. (2018). Analysis and expression of the carotenoid biosynthesis genes from Deinococcus wulumuqiensis R12 in engineered Escherichia coli. AMB Expr. 8:94. doi: 10.1186/s13568-018-0624-1
Xu, J., Xu, X., Xu, Q., Zhang, Z., Jiang, L., and Huang, H. (2018). Efficient production of lycopene by engineered E. coli strains harboring different types of plasmids. Bioprocess Biosyst. Eng. 41, 489–499. doi: 10.1007/s00449-017-1883-y
Yang, Q. (2021). Crucial roles of carotenoids as bacterial endogenous defense system for bacterial radioresistance of Deinococcus radiodurans. bioRxiv :2021.05.26.445811. doi: 10.1101/2021.05.26.445811
Yu, H., Cao, L., Li, F., Wu, Q., Li, Q., Wang, S., et al. (2015). The antioxidant mechanism of nitroxide TEMPO: scavenging with glutathionyl radicals. RSC Adv. 5, 63655–63661. doi: 10.1039/C5RA06129F
Zhang, L., Yang, Q., Luo, X., Fang, C., Zhang, Q., and Tang, Y. (2007). Knockout of crtB or crtI gene blocks the carotenoid biosynthetic pathway in Deinococcus radiodurans R1 and influences its resistance to oxidative DNA-damaging agents due to change of free radicals scavenging ability. Arch. Microbiol. 188, 411–419. doi: 10.1007/s00203-007-0262-5
Zhang, S., Zhao, X., Tao, Y., and Lou, C. (2015). A novel approach for metabolic pathway optimization: oligo-linker mediated assembly (OLMA) method. J. Biol. Eng. 9:23. doi: 10.1186/s13036-015-0021-0
Keywords: Deinococcus , carotenoid, deinoxanthin, metabolic engineering, antioxidation
Citation: Wang Y, Liu J, Yi Y, Zhu L, Liu M, Zhang Z, Xie Q and Jiang L (2024) Insights into the synthesis, engineering, and functions of microbial pigments in Deinococcus bacteria. Front. Microbiol. 15:1447785. doi: 10.3389/fmicb.2024.1447785
Edited by:
Xuejiao An, Jiangxi Agricultural University, ChinaReviewed by:
Runhua Han, University of Manitoba, CanadaRosa María Martínez-Espinosa, University of Alicante, Spain
Copyright © 2024 Wang, Liu, Yi, Zhu, Liu, Zhang, Xie and Jiang. This is an open-access article distributed under the terms of the Creative Commons Attribution License (CC BY). The use, distribution or reproduction in other forums is permitted, provided the original author(s) and the copyright owner(s) are credited and that the original publication in this journal is cited, in accordance with accepted academic practice. No use, distribution or reproduction is permitted which does not comply with these terms.
*Correspondence: Zhidong Zhang, emhhbmd6aGVlZG9uZ0B4YWFzLmFjLmNu; Qiong Xie, eGllcWlvQHNpbmEuY29t; Ling Jiang, amlhbmdsaW5nQG5qdGVjaC5lZHUuY24=
†These authors have contributed equally to this work